- Neurological Sciences and Cerebrovascular Research Laboratory, Department of Neurology and Stroke Center, La Paz University Hospital, Neuroscience Area of Hospital La Paz Institute for Health Research (IdiPAZ), Universidad Autónoma de Madrid, Madrid, Spain
Introduction
The recovery of the patient is the goal of the neuroscientists after a stroke. To achieve this recovery, there is a crucial need for increasing the understanding of the pathophysiological mechanisms that spontaneously engage early after stroke, which involve excitotoxicity, free radical damage, increased glutamate concentrations, and inflammation, leading to cell death. To assume that only neurons are vulnerable to these pathophysiological responses is simplistic. Stroke affects all components of the neurovascular unit, which consists of endothelial cells, pericytes, neurons, glial cells, white matter fiber tracts, myelin, and extracellular matrix proteins. It is, therefore, important to focus on brain protection as a whole rather than neuroprotection in isolation (1).
In the last 50 years, we have gained significant insights into the molecular mechanisms of recovery after stroke, in addition to the damage mechanisms. Due to its plasticity, the brain has the ability to reorganize its function and structure, which involves processes of self-protection and self-repair. Brain plasticity is a very complex process that involves adaptive structural and functional changes in the brain, including neurogenesis, synaptogenesis, angiogenesis, oligodendrogenesis, and astrogliosis modulation, and promotes collateral circulation, processes that begin immediately after stroke (2–4). Such is the self-repair ability brain that the stroke-induced neurogenesis is not only limited to the subventricular zone and hippocampal dentate gyrus. It has also been revealed that several additional areas of the brain promote mammalian adult neurogenesis, which include the hypothalamus, striatum, substantia nigra, cortex, and amygdala (5). The neural stem cells of the neurogenic areas generate new neural cells that migrate to the lesion site and become mature neurons, orchestrating neurological repair through nerve repair, neuron polarization, axonal sprouting and pruning, neurite outgrowth, and myelin repair, promoting post-stroke recovery (4). However, in the unfavorable microenvironment that occurs in the lesion, most new cells do not survive (4). Given that this self-repair capacity is limited, there has been a growing interest in the potential for brain plasticity-inducing interventions to enhance post-stroke recovery through rehabilitation, trophic factors, cell therapy, and extracellular vesicles. These therapeutic approaches currently hold great promise by targeting the mechanisms involved in brain plasticity (2, 6–8) (Figure 1). The beneficial effects of rehabilitation therapies in stroke recovery are well-known (9), although there are still certain aspects to be clarified, such as the optimal time to start rehabilitation, as well as its intensity and duration. Other approaches that involve brain stimulation, such as transcranial magnetic and electrical stimulation, can enhance recovery and post-stroke plasticity (10), and innovations with exoskeletons and brain-machine interfaces (11) have opened up new research lines. However, we would like to focus on other novel and promising strategies, such as the administration of trophic factors, stem cells, and extracellular vesicles.
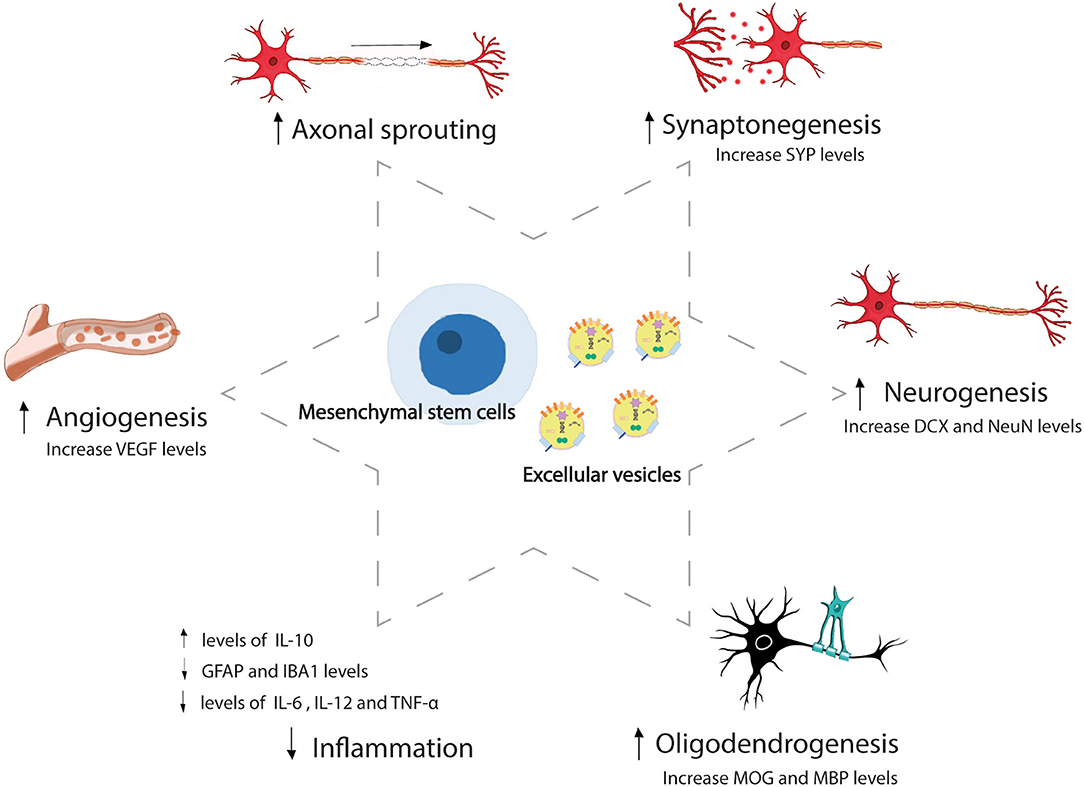
Figure 1. Mesenchymal stem cell and extracellular vesicles-induced brain repair process after stroke. Mesenchymal stem cells and extracellular vesicles administration-induced brain plasticity that involves adaptive structural and functional changes, including angiogenesis, axonal sprouting, synaptogenesis, neurogenesis, oligodendrogenesis, and inflammation modulation after stroke. SYP, synaptophysin; DCX, doublecortin; NeuN, neuronal nuclear protein; MOG, myelin-oligodendrocyte glycoprotein; MBP, myelin basic protein; IL, interleukin; GFAP, glial fibrillary acidic protein; IBA, ionized calcium-binding adapter molecule; TNF, tumor necrosis factor; VEGF, vascular endothelial growth factor.
Trophic Factors as Therapeutic Strategy to Promote Brain Plasticity
In recent years, trophic factors have generated a great deal of interest in the clinical context, given that their administration is not restricted to a narrow therapeutic window. Several trophic factors, including erythropoietin, brain-derived neurotrophic factor, granulocyte-colony-stimulating factor, vascular endothelial growth factor, fibroblast growth factor, epidermal growth factor, and heparin-binding epidermal growth factor, have anti-inflammatory and anti-excitotoxic protective properties and have demonstrated efficacy in promoting neurogenesis and angiogenesis, stimulating progenitor cell proliferation, preventing blood-brain barrier (BBB) disruption and ultimately promoting functional recovery in experimental stroke models (12–15). However, a number of these approaches were lost in translation from a bench to a bedside (14, 15), while others have not yet been tested in clinical trials.
Cell Therapy: The Factory of Trophic Factors and Key Molecules to Improve Recovery
But why settle for administering a single factor when we can administer the whole arsenal? This is where stem cell therapy plays an important role. Stem cells can secrete various trophic factors and key molecules, promote brain plasticity, and reduce overall inflammation. In particular, mesenchymal stem cells (MSCs) from bone marrow or adipose tissue have demonstrated efficacy in experimental animal stroke models (16–23). These positive findings are translated to clinical trials, where MSCs and other cells (such as bone marrow mononuclear cells) have demonstrated safety in patients with stroke (24–30) and even efficacy in promoting improvement in white matter injuries at 1 year (31). Perhaps, one of the most stimulating findings in stem cell translational research is the discovery of an abundant quantity of MSCs within adipose tissue. Adipose tissue-derived MSCs (AD-MSCs) are of special interest, not only due to their abundance but also their relative ease of obtention through procedures such as liposuction and abdominoplasty, which obviate the ethical concerns with embryonic MSCs. Due to immunoprivileged characteristics of this cell type, allogeneic administration is possible, in our opinion (32). Treatment can, therefore, be administered at an early stage, which is crucial for a disease where delays result in irrevocable loss of brain function. The administration of AD-MSCs in the acute phase could inhibit the aforementioned pathophysiological mechanisms that are activated early after stroke, thereby participating not only in the repair processes of the neurovascular unit but also in its protection. In terms of clinical feasibility, the intravenous route for delivering AD-MSCs is attractive, given its low invasiveness, low risk, and greater comfort for the patient. Using this route, AD-MSCs are unable to reach the brain due to their lack of ability in crossing the BBB; however, they exert their beneficial therapeutic actions by delivering their secretome from the peripheral organs where they are confined (19). MSCs exert their action by the release of key molecules or extracellular vesicles (EVs) by paracrine effects (33), rather than through differentiation to replace damaged neurons (16, 18).
Extracellular Vesicles: The Novel Strategy to Enhance Brain Recovery
Extracellular vesicles are released from all cell types, harbor important molecules such as proteins, DNA, lipids, mRNAs, and microRNAs, and participate in cell-to-cell communication. EVs can act as an active principle promoting several mechanisms of recovery after stroke, including brain plasticity. It has been shown that the intravenous administration of MSC-derived EVs promotes functional recovery and brain plasticity in an ischemic stroke rat model (34–37). Moreover, our group found that an intravenous administration of EVs-improved outcomes by promoting the processes involved in white matter repair in subcortical stroke in rats (35). Other authors have also shown that EVs induce higher axonal density and neurite remodeling (34), new formation of endothelial cells (36), sprouting of new capillaries, and higher endothelial integrity (37). MSC-derived EVs are, therefore, a promising approach for repairing the components of the neurovascular unit to promote overall post-stroke recovery (38, 39).
MSC-derived EVs are able to go one step farther than MSCs as a therapeutic strategy, given that MSC-EVs can cross the BBB, resolve cell-related problems, such as immune compatibility, tumor formation, and vascular occlusion, and can be stored in hospital settings without the need for toxic cryopreservative agents, offering an approach for acute ischemic stroke. Due to their small size, MSC-EVs can be saved from phagocytosis by macrophages. In addition, selective manipulation of their cargo by bioengineering can lead to individualized medicine (40, 41). There is, currently, only one ongoing clinical trial aimed at assaying the efficacy of the allogeneic administration of MSC-derived EVs enriched by miR-124 for improving the recovery of patients with acute ischemic stroke, registered in clinicaltrials.gov (Identifier: NCT03384433). A previous study using an animal model of stroke demonstrated that the administration of EVs loaded with miR-124 promoted cortical neural progenitors and neurogenesis (42). These functions of the microRNA content of EVs make them important not only as treatment but also as biomarkers. We showed that circulating EVs from patients with stroke contain miRNA and proteins related to risk factors and etiology, post-ischemic immune response, endogenous protection, and angiogenesis (43). We also observed differences in the levels of the microRNA content of EVs according to the topography of the stroke (subcortical and cortical-subcortical ischemic stroke) and related to improved recovery after stroke (44). Given the progress of research on EVs, further information on brain-derived EVs under stroke conditions is necessary (45). The content of EVs can be used as biomarkers that improve our understanding of the mechanisms by which EVs act in stroke to help develop new therapeutic strategies and find new molecular targets for this neurological disease.
Discussion
The experimental studies in animal model and clinical trials have indicated that intravenously administered MSC therapy seems to be a promising therapeutic strategy after stroke (16–23, 26–28, 30). However, there are still some unresolved issues that have to be investigated such as the most appropriate administration timing, the doses required for successful recovery (46), and the dose regimen (single-dose or repeat-doses) that reach the higher threshold of brain repair after stroke. Likewise, the limitations of cell therapy will be determined by the results of clinical trials.
Moreover, MSC-derived EVs have some important advantages that can be exploited when translating a therapeutic strategy for stroke. EVs provide a great feature as a drug delivery system, given their ability to cross the BBB (40). Thanks to the development of delivery system technologies, MSC-derived EVs can be engineered and designed to carry specific therapeutic molecules (47) according to brain tissue repair needs, avoiding molecules that could induce adverse effects, moving toward personalized medicine.
Beyond the proven beneficial outcomes with the MSC-derived EV treatment in experimental animal models of stroke (34–39), many aspects are yet to be resolved about the production of EVs for their use in clinical practice, such us large-scale production, conditions in different physiologically environments, and standardized experimental protocol for extracting EVs to provide batch uniformity according to GMP regulations (40, 48, 49).
In conclusion, we are facing a disease that has a high incidence and prevalence and results in major disability. However, we are also finding new and promising therapeutic options, such as trophic factors, cell therapy, and EVs that can positively contribute to stroke recovery by improving brain plasticity. For EVs, their ability to cross the BBB and their editable cargo provide live information on molecules that participate in damage and post-stroke repair, which could lead to personalized and precision medicine.
Author Contributions
All authors listed have made a substantial, direct, and intellectual contribution to the work, and approved it for publication.
Funding
This work was sponsored by a grant from Miguel Servet (CP15/00069; CPII20/00002 to MG-F), Miguel Servet (CP20/00024 to LO-O), and the INVICTUS PLUS network grant (RD16/0019/0005) from the Carlos III Health Institute Health Care Research Fund and was co-funded by the European Regional Development Fund (ERDF). We appreciate Morote Traducciones S. L. support for their editing assistance.
Conflict of Interest
The authors declare that the research was conducted in the absence of any commercial or financial relationships that could be construed as a potential conflict of interest.
Publisher's Note
All claims expressed in this article are solely those of the authors and do not necessarily represent those of their affiliated organizations, or those of the publisher, the editors and the reviewers. Any product that may be evaluated in this article, or claim that may be made by its manufacturer, is not guaranteed or endorsed by the publisher.
References
1. Díez-Tejedor E, Gutiérrez-Fernández M. Why do we say ‘neuroprotection' in stroke when we mean ‘brain protection or cerebroprotection'? Eur Stroke J. (2019) 4:281–82. doi: 10.1177/2396987319831928
2. Gutiérrez M, Merino JJ, Alonso de Leciñana M, Díez-Tejedor E. Cerebral protection, brain repair, plasticity and cell therapy in ischemic stroke. Cerebrovasc Dis. (2009) 27:177–86. doi: 10.1159/000200457
3. Detante O, Jaillard A, Moisan A, Barbieux M, Favre IM, Garambois K, et al. Biotherapies in stroke. Rev Neurol. (2014) 170:779–98. doi: 10.1016/j.neurol.2014.10.005
4. Cuartero MI, García-Culebras A, Torres-López C, Medina V, Fraga E, Vázquez-Reyes S, et al. Post-stroke neurogenesis: friend or foe? Front Cell Dev Biol. (2021) 9:657846. doi: 10.3389/fcell.2021.657846
5. Jurkowski MP, Bettio L, Woo EK, Patten A, Yau SY, Gil-Mohapel J. Beyond the hippocampus and the SVZ: adult neurogenesis throughout the brain. Front Cell Neurosci. (2020) 14:576444. doi: 10.3389/fncel.2020.576444
6. Zhang RL, Zhang ZG, Zhang L, Chopp M. Proliferation and differentiation of progenitor cells in the cortex and the subventricular zone in the adult rat after focal cerebral ischemia. Neuroscience. (2001) 105:33–41. doi: 10.1016/S0306-4522(01)00117-8
7. Thored P, Arvidsson A, Cacci E, Ahlenius H, Kallur T, Darsalia V, et al. Persistent production of neurons from adult brain stem cells during recovery after stroke. Stem Cells. (2006) 24:739–47. doi: 10.1634/stemcells.2005-0281
8. Wang L, Xiong X, Zhang L, Shen J. Neurovascular unit: a critical role in ischemic stroke. CNS Neurosci Ther. (2021) 27:7–16. doi: 10.1111/cns.13561
9. Ting WK, Fadul FA, Fecteau S, Ethier C. Neurostimulation for stroke rehabilitation. Front Neurosci. (2021) 15:649459. doi: 10.3389/fnins.2021.649459
10. Braun RG, Wittenberg GF. Motor recovery: how rehabilitation techniques and technologies can enhance recovery and neuroplasticity. Semin Neurol. (2021) 41:167–76. doi: 10.1055/s-0041-1725138
11. Nizamis K, Athanasiou A, Almpani S, Dimitrousis C, Astaras A. Converging robotic technologies in targeted neural rehabilitation: a review of emerging solutions and challenges. Sensors. (2021) 21:2084. doi: 10.3390/s21062084
12. Gutiérrez-Fernández M, Fuentes B, Rodríguez-Frutos B, Ramos-Cejudo J, Vallejo-Cremades MT, Díez-Tejedor E. Trophic factors and cell therapy to stimulate brain repair after ischaemic stroke. J Cell Mol Med. (2012) 16:2280–90. doi: 10.1111/j.1582-4934.2012.01575.x
13. Ramos-Cejudo J, Gutiérrez-Fernández M, Otero-Ortega L, Rodríguez-Frutos B, Fuentes B, Vallejo-Cremades MT, et al. Brain-derived neurotrophic factor administration mediated oligodendrocyte differentiation and myelin formation in subcortical ischemic stroke. Stroke. (2015) 46:221–8. doi: 10.1161/STROKEAHA.114.006692
14. Chan SJ, Love C, Spector M, Cool SM, Nurcombe V, Lo EH. Endogenous regeneration: engineering growth factors for stroke. Neurochem Int. (2017) 107:57–65. doi: 10.1016/j.neuint.2017.03.024
15. Dordoe C, Chen K, Huang W, Chen J, Hu J, Wang X, et al. Roles of fibroblast growth factors and their therapeutic potential in treatment of ischemic stroke. Front Pharmacol. (2021) 12:671131. doi: 10.3389/fphar.2021.671131
16. Gutiérrez-Fernández M, Rodríguez-Frutos B, Alvarez-Grech J, Vallejo-Cremades MT, Expósito-Alcaide M, Merino J, et al. Functional recovery after hematic administration of allogenic mesenchymal stem cells in acute ischemic stroke in rats. Neuroscience. (2011) 175:394–405. doi: 10.1016/j.neuroscience.2010.11.054
17. Gutiérrez-Fernández M, Rodríguez-Frutos B, Ramos-Cejudo J, Otero-Ortega L, Fuentes B, Vallejo-Cremades MT, et al. Comparison between xenogeneic and allogeneic adipose mesenchymal stem cells in the treatment of acute cerebral infarct: proof of concept in rats. J TranslMed. (2015) 13:46. doi: 10.1186/s12967-015-0406-3
18. Otero-Ortega L, Gutiérrez-Fernández M, Ramos-Cejudo J, Rodríguez-Frutos B, Fuentes B, Sobrino T, et al. White matter injury restoration after stem cell administration in subcortical ischemic stroke. Stem Cell Res Ther. (2015) 6:121. doi: 10.1186/s13287-015-0111-4
19. Rodríguez-Frutos B, Otero-Ortega L, Gutiérrez-Fernández M, Fuentes B, Ramos-Cejudo J, Díez-Tejedor E. Stem cell therapy and administration routes after stroke. Transl Stroke Res. (2016) 7:378–87. doi: 10.1007/s12975-016-0482-6
20. Laso-García F, Diekhorst L, Gómez-de Frutos MC, Otero-Ortega L, Fuentes B, Ruiz-Ares G, et al. Cell-based therapies for stroke: promising solution or dead end? Mesenchymal stem cells and comorbidities in preclinical stroke research. Front Neurol. (2019) 10:332. doi: 10.3389/fneur.2019.00332
21. Gómez-de Frutos MC, Laso-García F, Diekhorst L, Otero-Ortega L, Fuentes B, Jolkkonen J, et al. RESSTORE consortium. Intravenous delivery of adipose tissue-derived mesenchymal stem cells improves brain repair in hyperglycemic stroke rats. Stem Cell Res Ther. (2019) 10:212. doi: 10.1186/s13287-019-1322-x
22. Tobin MK, Stephen TKL, Lopez KL, Pergande MR, Bartholomew AM, Cologna SM, et al. Activated mesenchymal stem cells induce recovery following stroke via regulation of inflammation and oligodendrogenesis. J Am Heart Assoc. (2020) 9:e013583. doi: 10.1161/JAHA.119.013583
23. Li W, Shi L, Hu B, Hong Y, Zhang H, Li X, et al. Mesenchymal stem cell-based therapy for stroke: current understanding and challenges. Front Cell Neurosci. (2021) 15:628940. doi: 10.3389/fncel.2021.628940
24. Battistella V, de Freitas GR, da Fonseca LM, Mercante D, Gutfilen B, Goldenberg RC, et al. Safety of autologous bone marrow mononuclear cell transplantation in patients with nonacute ischemic stroke. Regen Med. (2011) 6:45–52. doi: 10.2217/rme.10.97
25. Moniche F, Gonzalez A, Gonzalez-Marcos JR, Carmona M, Pinero P, Espigado I, et al. Intra-arterial bone marrow mononuclear cells in ischemic stroke: a pilot clinical trial. Stroke. (2012) 43:2242–44. doi: 10.1161/STROKEAHA.112.659409
26. Diez-Tejedor E, Gutierrez-Fernandez M, Martinez-Sanchez P, Rodriguez-Frutos B, Ruiz-Ares G, Lara ML, et al. Reparative therapy for acute ischemic stroke with allogeneic mesenchymal stem cells from adipose tissue: a safety assessment: a phase II randomized, double-blind, placebo-controlled, single-center, pilot clinical trial. J Stroke Cerebrovasc Dis. (2014) 23:2694–700. doi: 10.1016/j.jstrokecerebrovasdis.2014.06.011
27. Steinberg GK, Kondziolka D, Wechsler LR, Lunsford LD, Coburn ML, Billigen JB, et al. Clinical outcomes of transplanted modified bone marrow-derived mesenchymal stem cells in stroke: a phase 1/2a study. Strok. (2016) 47:1817–24. doi: 10.1161/STROKEAHA.116.012995
28. Hess DC, Wechsler LR, Clark WM, Savitz SI, Ford GA, Chiu D, et al. Safety and efficacy of multipotent adult progenitor cells in acute ischaemic stroke (MASTERS): a randomised, double-blind, placebo-controlled, phase 2 trial. Lancet Neurol. (2017) 16:360–8. doi: 10.1016/S1474-4422(17)30046-7
29. Vahidy FS, Haque ME, Rahbar MH, Zhu H, Rowan P, Aisiku IP, et al. Intravenous bone marrow mononuclear cells for acute ischemic stroke: safety, feasibility, and effect size from a phase I clinical trial. Stem Cells. (2019) 37:1481–91. doi: 10.1002/stem.3080
30. Chung JW, Chang WH, Bang OY, Moon GJ, Kim SJ, Kim SK, et al. STARTING-2 collaborators. efficacy and safety of intravenous mesenchymal stem cells for ischemic stroke. Neurology. (2021) 96:e1012–3. doi: 10.1212/WNL.0000000000011440
31. Haque ME, Hasan KM, George S, Sitton C, Boren S, Arevalo OD, et al. Longitudinal neuroimaging evaluation of the corticospinal tract in patients with stroke treated with autologous bone marrow cells. Stem Cells Transl Med. (2021) 10:943–55. doi: 10.1002/sctm.20-0369
32. Gutiérrez-Fernández M, Otero-Ortega L, Ramos-Cejudo J, Rodríguez-Frutos B, Fuentes B, Díez-Tejedor E. Adipose tissue-derived mesenchymal stem cells as a strategy to improve recovery after stroke. Expert Opin Biol Ther. (2015) 15:873–81. doi: 10.1517/14712598.2015.1040386
33. Satani N, Savitz SI. Is immunomodulation a principal mechanism underlying how cell-based therapies enhance stroke recovery? Neurotherapeutics. (2016) 13:775–82. doi: 10.1007/s13311-016-0468-9
34. Xin H, Li Y, Cui Y, Yang JJ, Zhang ZG, Chopp M. Systemic administration of exosomes released from mesenchymal stromal cells promote functional recovery and neurovascular plasticity after stroke in rats. J Cereb Blood Flow Metab. (2013) 33:1711–5. doi: 10.1038/jcbfm.2013.152
35. Otero-Ortega L, Laso-García F, Gómez-de Frutos MD, Rodríguez-Frutos B, Pascual-Guerra J, Fuentes B, et al. White matter repair after extracellular vesicles administration in an experimental animal model of subcortical stroke. Sci Rep. (2017) 7:44433. doi: 10.1038/srep44433
36. Doeppner TR, Herz J, Gorgens A, Schlechter J, Ludwig AK, Radtke S, et al. Extracellular vesicles improve post-stroke neuroregeneration and prevent postischemic immunosuppression. Stem Cells Transl Med. (2015) 4:1131–43. doi: 10.5966/sctm.2015-0078
37. Chen KH, Chen CH, Wallace CG, Yuen CM, Kao GS, Chen YL, et al. Intravenous administration of xenogenic adipose-derived mesenchymal stem cells (ADMSC) and ADMSC-derived exosomes markedly reduced brain infarct volume and preserved neurological function in rat after acute ischemic stroke. Oncotarget. (2016) 7:74537–56. doi: 10.18632/oncotarget.12902
38. Doeppner TR, Bähr M, Giebel B, Hermann DM. Immunological and non-immunological effects of stem cell-derived extracellular vesicles on the ischaemic brain. Ther Adv Neurol Disord. (2018) 11:1756286418789326. doi: 10.1177/1756286418789326
39. Davis C, Savitz SI, Satani N. Mesenchymal stem cell derived extracellular vesicles for repairing the neurovascular unit after ischemic stroke. Cells. (2021) 10:767. doi: 10.3390/cells10040767
40. Otero-Ortega L, Laso-García F, Gómez-de Frutos M, Fuentes B, Diekhorst L, Díez-Tejedor E, Gutiérrez-Fernández M. Role of exosomes as a treatment and potential biomarker for stroke. Transl Stroke Res. (2019) 10:241–9. doi: 10.1007/s12975-018-0654-7
41. Bang OY, Kim EH. mesenchymal stem cell-derived extracellular vesicle therapy for stroke: challenges and progress. Front Neurol. (2019) 10:211. doi: 10.3389/fneur.2019.00211
42. Yang J, Zhang X, Chen X, Wang L, Yang G. Exosome mediated delivery of miR-124 promotes neurogenesis after ischemia. Mol Ther Nucleic Acids. (2017) 7:278–87. doi: 10.1016/j.omtn.2017.04.010
43. Otero-Ortega L, Alonso-López E, Pérez-Mato M, Laso-García F, Gómez-de Frutos MC, Diekhorst L, et al. Similarities and differences in extracellular vesicle profiles between ischaemic stroke and myocardial infarction. Biomedicines. (2020) 9:8. doi: 10.3390/biomedicines9010008
44. Otero-Ortega L, Alonso-López E, Pérez-Mato M, Laso-García F, Gómez-de Frutos MC, Diekhorst L, et al. Circulating extracellular vesicle proteins and microRNA profiles in subcortical and cortical-subcortical ischaemic stroke. Biomedicines. (2021) 9:786. doi: 10.3390/biomedicines9070786
45. Brenna S, Altmeppen HC, Mohammadi B, Rissiek B, Schlink F, Ludewig P, et al. Characterization of brain-derived extracellular vesicles reveals changes in cellular origin after stroke and enrichment of the prion protein with a potential role in cellular uptake. J Extracell Vesicles. (2020) 9:1809065. doi: 10.1080/20013078.2020.1809065
46. Doeppner TR, Hermann DM. Mesenchymal stem cells in the treatment of ischemic stroke: progress and possibilities. Stem Cells Cloning. (2010) 3:157–63. doi: 10.2147/SCCAA.S7820
47. Dolcetti E, Bruno A, Guadalupi L, Rizzo FR, Musella A, Gentile A, et al. Emerging role of extracellular vesicles in the pathophysiology of multiple sclerosis. Int J Mol Sci. (2020) 21:7336. doi: 10.3390/ijms21197336
48. Ha D, Yang N, Nadithe V. Exosomes as therapeutic drug carriers and delivery vehicles across biological membranes: current perspectives and future challenges. Acta Pharm Sin B. (2016) 6:287–96. doi: 10.1016/j.apsb.2016.02.001
Keywords: brain plasticity, recovery, stroke, trophic factors, stem cell, extracellular vesicles
Citation: Otero-Ortega L, Gutiérrez-Fernández M and Díez-Tejedor E (2021) Recovery After Stroke: New Insight to Promote Brain Plasticity. Front. Neurol. 12:768958. doi: 10.3389/fneur.2021.768958
Received: 01 September 2021; Accepted: 18 October 2021;
Published: 19 November 2021.
Edited by:
Juan Francisco Arenillas, Hospital Clínico Universitario de Valladolid, SpainReviewed by:
Roza M. Umarova, University of Freiburg Medical Center, GermanyCopyright © 2021 Otero-Ortega, Gutiérrez-Fernández and Díez-Tejedor. This is an open-access article distributed under the terms of the Creative Commons Attribution License (CC BY). The use, distribution or reproduction in other forums is permitted, provided the original author(s) and the copyright owner(s) are credited and that the original publication in this journal is cited, in accordance with accepted academic practice. No use, distribution or reproduction is permitted which does not comply with these terms.
*Correspondence: Exuperio Díez-Tejedor, ZXh1cGVyaW8uZGllekBzYWx1ZC5tYWRyaWQub3Jn; María Gutiérrez-Fernández, bWd1dGllcnJlemZlcm5hbmRlekBzYWx1ZC5tYWRyaWQub3Jn