- Department of Neurology, Union Hospital, Tongji Medical College, Huazhong University of Science and Technology, Wuhan, China
After long-term use of levodopa, Parkinson's patients almost inevitably develop dyskinesia, a kind of drug side effect manifesting as uncontrollable choreic movements and dystonia, which could be crippling yet have limited therapeutic options. Transcranial magnetic stimulation is the most widely studied non-invasive neuromodulation technology to treat levodopa-induced dyskinesia. Many studies have shown that transcranial magnetic stimulation has beneficial effects on levodopa-induced dyskinesia and is patient-tolerable, barely with reported adverse effects. Changes in brain connectivity, neuroplasticity, neurotransmitter, neurorestoration, and blood flow modulation could play crucial roles in the efficacy of transcranial magnetic stimulation for levodopa-induced dyskinesia. The appearance of new modes and application for emerging targets are possible solutions for transcranial magnetic stimulation to achieve sustained efficacy. Since the sample size in all available studies is small, more randomized double-blind controlled studies are needed to elucidate the specific treatment mechanisms and optimize treatment parameters.
Introduction
Parkinson's disease (PD) is a neurodegenerative disease characterized by the degeneration of substantia nigra dopaminergic neurons. Levodopa is the mainstay drug choice in the clinical management of PD. However, long-term levodopa supplements convert Parkinson's patients from akinetic state to hyperkinetic state, namely levodopa-induced dyskinesia (LID), with its severity ranging from mild and barely noticeable to severely disabled. After 4–6 years of levodopa administration, the occurrence rate of dyskinesia is 40%, while after 15 years, the occurrence rates can be up to 94% (1).
Modifying dopaminergic therapy to provide more continuous dopaminergic stimulation is helpful for the management of LID. Apart from dopaminergic drugs, amantadine is currently regarded as the most effective drug for LID treatment (2). Although the efficacy of amantadine has been proved to be long-lasting and remarkable, its use might induce/exacerbate unacceptable hallucinations and also is contraindicated in patients with end-stage renal disease (3). Discontinuation of this drug is even associated with a significant risk of worsening dyskinesias (3). These undesirable effects limited the long-term use of amantadine. Several other chemicals targeting adenosine, adrenergic, glutamatergic, and serotonergic receptors have significantly decreased dyskinesias in animal models but not in parkinsonian patients (3). For patients refractory to medical management, neurosurgical approaches are also the procedure of choice. Among them, deep brain stimulation has been widely studied and recommended as one priority procedure for LID patients who need surgery. However, deep brain stimulation costs much, needs regular follow-up appointments over several years, and bears a danger of possible adverse effects after electrode placement (4). Surgical ablation of the globus pallidus has been reported to have remarkable efficacy in treating contralateral dyskinetic symptoms, while its efficacy is more petite than bilateral subthalamic nucleus deep brain stimulation in one case of a randomized controlled trial (4). A new minimally invasive approach using magnetic resonance-guided focused ultrasound to ablate globus pallidus has only been shown to improve dyskinesia in a case report (4).
In contrast, another non-invasive procedure, transcranial magnetic stimulation (TMS), has been applied to treat LID since 2005 (5) and has shown some benefits to a certain extent in several studies. TMS might be a promising neuromodulation skill to improve LID. The purpose of the present review is to discuss the main points of TMS in the management of LID and related mechanisms to allow for a better understanding of its potential uses.
An Outline for Studies Concerning Transcranial Magnetic Stimulation in the Management of LID
Studies Utilizing Repetitive Transcranial Magnetic Stimulation
A pilot study subjected 8 PD patients with LID to 1 day of 15-min, low-frequency (1 Hz) rTMS (LF-rTMS) over the supplementary motor area (SMA) during apomorphine infusion (5). Mean (average of two raters) dyskinesia was significantly lower immediately and 15 min after the LF-rTMS sessions but not 30 min afterward (5). Brusa et al. conducted the same LF-rTMS on 10 PD patients with LID over SMA after levodopa intake (6). Unlike the pilot study, besides 15-min, single-day stimulation, this study also observed the effect of repeated, 5-day stimulation (6). This study found that mean (average of two raters) dyskinesia were significantly lower 15 and 30 min but not 45 and 60 min after the single-day and 5-day LF-rTMS sessions (6). Also, both single- and multiple-session LF-rTMS increased dyskinesia onset latency to the same degree comparing with sham control and no rTMS condition (6).
A later study conducted a 10-day LF-rTMS protocol over the primary motor cortex (MC) for 6 PD patients with LID after levodopa intake (7). Peak (during peak ON) and mean (average of early ON, peak ON, and late ON) dyskinesia were significantly lower for up to 1 day but not 2 weeks, whereas cortical excitability remained no change for all these time points (7). Although this study did not conduct sham control, we still could make some preliminary conclusions from it. Firstly, alterations of cortical excitability might not be the only mechanism involving the efficiency of LF-rTMS since cortical excitability did not correlate with the observed improvements in LID in this study. Secondly, more prolonged stimulation after-effects could be attained by extending stimulation days appropriately, such as 10 days rather than only 5 days. Positive relations between longer-lasting reduction of LID and longer sessions could be affirmed if further studies could apply longer-session LF-rTMS, such as a 3-week or even 4-week course.
A sham-controlled study later applied 4 consecutive days of LF-rTMS on 10 PD patients with LID during levodopa intake (8). In this study, a single session per day was increased from the previous 15 to 32 min (8). One-day reduction of LID severity was observed (8). However, it was a pity that this study did not record when the efficacy of LF-rTMS disappeared. Comparing with the outcome from Wagle-Shukla et al. (7) this finding suggests prolonged after-effects might also be obtained by increasing daily stimulation duration besides stimulation days (8). Also, this study firstly found that the major effect of LF-rTMS on LID improvement was on dystonia subscores (8).
Sayin et al. performed 10 consecutive days (30 min daily) of LF-rTMS over SMA on 17 PD patients with LID during levodopa intake (9). The study replicated 1 day of alleviation for LID, but the efficacy disappeared up to 120 min on the second day (9). Since this is a parallel sham controlled study, discrepancies of baseline dyskinesia severity between two groups might bring bias to outcomes (9). All aforementioned studies showed LF-rTMS had beneficial effects on LID improvement.
However, a later study showed adverse outcomes both after single-session and 5-day-multiple-session LF-rTMS (10). This was the first study to use two separate coils on bilateral MC; such an unexpected outcome might result from an offset of bilateral stimulation (10). It is speculated that a positive ipsilateral effect could be counterbalanced by a subsequent contralateral LF-rTMS session influencing more distant areas because previous studies have shown LF-rTMS ability to induce changes in areas distant from the stimulated area (11).
Lohse et al. firstly applied LF-rTMS over the pre-supplementary motor area (pre-SMA) on 17 PD patients with LID before levodopa intake (12). They found LF-rTMS utilization could help improve LID symptoms transiently (12). This was also the sole study regarding the relationship between stimulation intensity of LF-rTMS and its clinical impact on LID. Stimulation intensity is documented as the percentage of maximum stimulator output (MSO). With MSO of LF-rTMS increasing up to 60%, Lohse et al. found a significant linear correlation between stimulation intensity and individual prolongation of the time to onset of dyskinesia after levodopa intake (12). They also found a similar trend between MSO and individual reduction in dyskinesia severity, but it did not reach statistical significance (12). Recently, Flamez et al. conducted single-session LF-rTMS (16 min daily) over pre-SMA on 17 PD patients with LID before levodopa intake but failed to replicate the therapeutic effect on LID (13).
Overall, most of these studies validated the short-term beneficial effect of LF-rTMS, but long-term therapeutic effects still needed to be explored. Among these studies, no adverse event was reported. Moreover, these beneficial effects are less likely to be induced by placebo effects. LF-rTMS seems to be a potential approach to treat LID. However, the conclusions from these studies are limited by the small sample sizes used. Also, differences in pharmacological status, dyskinesia assessment scales, and stimulation parameters (Table 1, Figures 1, 2) can confound outcomes of these LF-rTMS studies. Thus, once a mode of LF-rTMS with definite, reproducible, and sustained improvement on LID is established, LF-rTMS might be one of the most valuable approaches to alleviate LID in clinical settings.
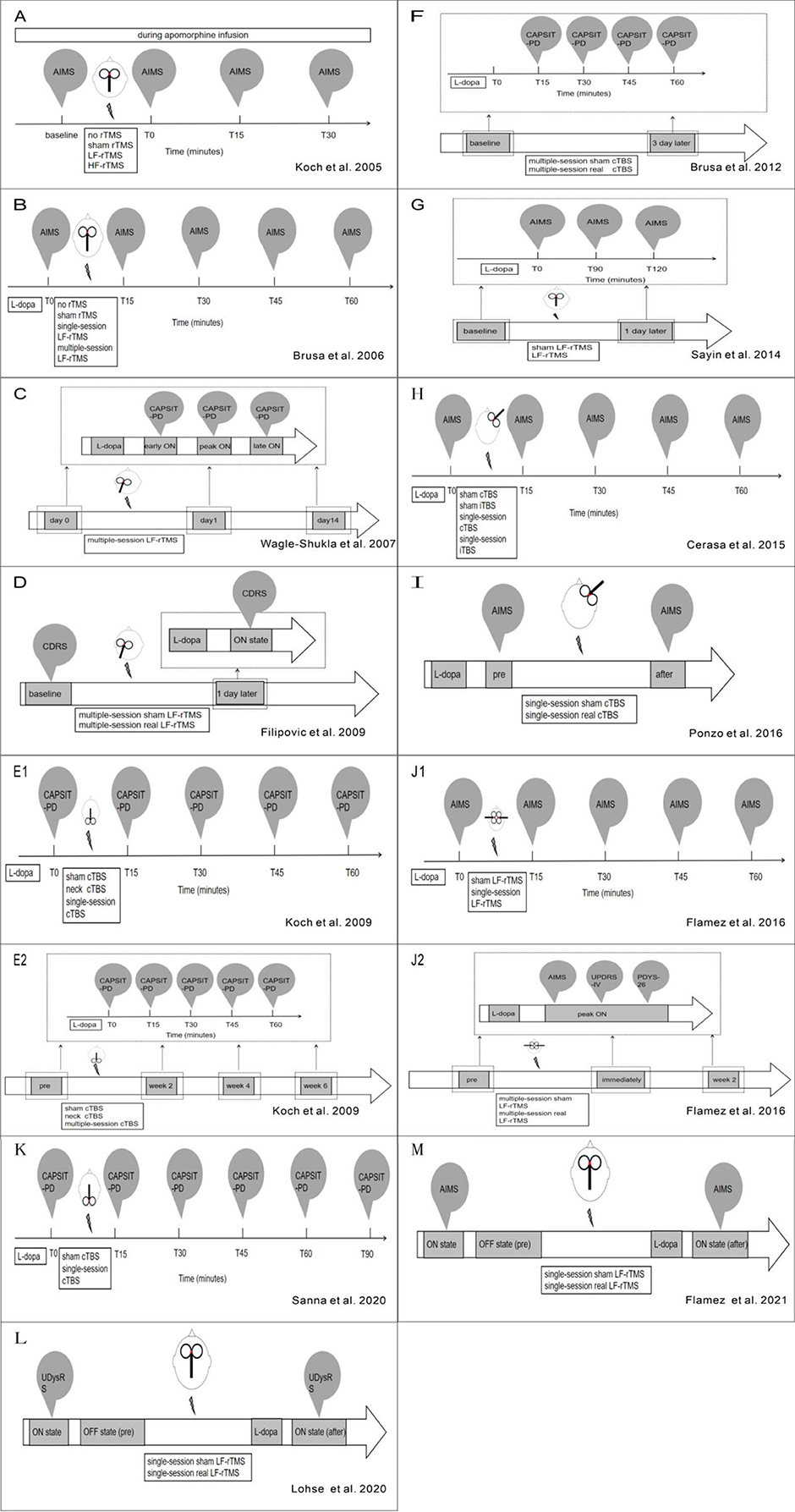
Figure 2. (A–L) Refers to overview of stimulation workflow in studies mentioned in Table 1.
On the other hand, it was shown that all high frequency (5 and 10 Hz) rTMS (HF-rTMS) studies (Table 2, Figure 1) have no effect on LID.
Studies Utilizing Theta Burst Stimulation
Unlike rTMS, the protocol of TBS is comparatively more consistent among studies (Table 1, Figures 1, 2). For all five studies utilizing continuous TBS (cTBS), cTBS consists of three-pulse bursts at 50 Hz repeated every 200 ms for 40 s (20) and was administered after levodopa intake.
In Koch's study, they firstly applied single-session cTBS on 10 PD patients with LID over the cerebellum, and a 45-min reduction was observed (14). In this study, a 10-day course of cTBS was further conducted and induced persistent clinical beneficial effects up to 4 weeks (14). However, a later study applied a 5-day course of cTBS on 8 PD patients with LID over the cerebellum only reduced LID up to 45 min (15).
A study applied single-session cTBS over the inferior frontal cortex (IFC) and MC on 8 PD patients with LID, respectively (16). Stimulation over the right IFC induced improvement of LID only up to 30 min, while stimulation over MC did not exhibit any change (16). Although efficacy duration was not mentioned, Ponza et al. also observed the beneficial effect of cTBS on LID symptoms after single-session stimulation over the right IFC (17). A recent study targeting cerebellum also displayed 60-min alleviation for LID after cTBS stimulation (18).
Among these cTBS studies, two have mentioned specific stimulation intensity. In Koch's and Cerasa's studies (14, 16), 46.2 ± 8.5% MSO applied over the right IFC and cerebellum alleviated LID symptoms, while the same stimulation intensity over MC failed to improve LID symptoms. Since Cerasa et al. did not conduct further study to see whether higher stimulation intensity over MC would change the result or not, it could be early to deny the role of stimulation intensity for cTBS efficacy.
Like LF-rTMS, the short-term benefits of cTBS have been corroborated in several studies and are patient-tolerable. Although a remarkably longer after effect of cTBS than of LF-rTMS was exhibited only in one study, such prolonged effect did not replicate in other studies.
When it comes to intermittent theta-burst stimulation (iTBS) mode applied on IFC or MC (Table 2, Figure 1), no change has occurred to LID symptoms at both regions.
An Outline for Stimulation Targets in TMS Protocols From the Studies Above
Brain Regions in Motor Basal Ganglia Loop
MC is a crucial brain region involving in the development of LID. Alterations of potentials recorded from MC shed light on possible mechanisms underlying the benefits of LF-rTMS and cTBS for LID.
Short-interval intracortical inhibitions (SICI) and long-latency intracortical inhibition (LICI) reflect suppression of MC excitability (21, 22). In off therapy, SICI and LICI were decreased in PD patients with and without LID (23). Unlike PD patients without LID, administration of levodopa could not reverse decreased SICI and LICI in PD patients with LID (23). In off therapy, γ-Aminobutyric acid (GABAergic) agonist increased SICI in PD patients (24). Administration of GABAergic agonist could also alleviate LID (25). It is believed that SICI is likely to be mediated by GABA-A-ergic receptors (26) and LICI by GABA-B-ergic receptors (27, 28).
On the contrary, intracortical facilitation (ICF) and short-interval intracortical facilitation (SICF) reflect the facilitation of MC excitability (21, 29). Regardless of drug condition, ICF was found to decrease or remain normal in PD patients with LID (23, 30). Unlike ICF in dyskinetic patients, SICF kept increased in off and on the state (30). Such increase was positively correlated with the severity of LID (30). Increased SICF in LID patients could be alleviated by anti-glutamatergic drugs (30). Improvement of LID did not come with restoration of SICF (30), which suggests additional pathophysiological mechanisms might contribute to LID.
Findings of the two opposite types of potentials both indicated overexcitability of MC renders occurrence of LID. HF-rTMS (31) and iTBS (20) increases cortical excitability, whereas LF-rTMS (31, 32) and cTBS (20) decreases cortical excitability, which conforms to their opposite effects on LID symptoms. Apart from alterations of these potentials, dendritic spines in intratelencephalic-type corticostriatal neurons in MC became enlarged of rats with LID (33).
Although SMA did not show any structural modifification in PD patients with LID (34), neuroimaging has linked overactive SMA with the occurrence of LID (35, 36). Indeed, inhibitory LF-rTMS over SMA improved LID symptoms (5, 6, 9).
Brain Regions in Associative and Limbic Basal Ganglia Loop
Voxel based morphometry reveals increased gray matter volume of the bilateral IFC in dyskinetic patients (34). Right IFC engages in suppressing an already initiated manual response (37). One study further revealed dyskinetic PD patients have a weaker inhibitory interaction between the right IFC and contralateral MC (17). This finding conforms with beneficial effects of inhibitory cTBS over right IFC on LID (17). Another study revealed that connectivity of the right IFC with the left MC was decreased in patients with LID (16). Nevertheless, inhibitory cTBS over right IFC improved LID symptoms in this study as well (16). Authors speculated that the increased communication between the right IFC and the putamen observed in this study in patients with LID might interfere with the motor inhibition network (16).
Task-based functional magnetic resonance imaging revealed activation of pre-SMA after intake of levodopa in LID patients (38). The pre-SMA has been implicated in both the suppression and initiation of movements (39). This might partly explain the contradictory outcomes of two LF-rTMS studies over pre-SMA on LID (12, 13).
Activation of the dorsolateral prefrontal cortex (DLPFC) was also observed in PD patients with LID (40). However, it was bewildering that HF-rTMS Stimulation of the left DLPFC induced a significant MC depression (19). Moreover, such MC depression did not reach a significant reduction of LID symptoms (19).
Cerebellum
Increased metabolic activity in the dentate nucleus (15) and in the red nucleus (41) indicated cerebellar involvement in the development of LID. Further studies revealed cerebellar-cortical interaction in dyskinetic patients. After delivery of inhibitory cTBS over cerebellum, alleviated LID symptoms concurrently accompanied by enhancement of MC plasticity (42). Also resting-state functional connectivity was found to increase between cerebellum and left IFC: the greater the enhancement of cerebellar-IFC functional connectivity, the shorter was the latency of dyskinesia onset (43).
Since many circuits take part in the occurrence of LID, identification of the critical brain region (Figure 3) involved in all LID mechanisms as the stimulation target or combination of different regions might prolong treatment efficacy.
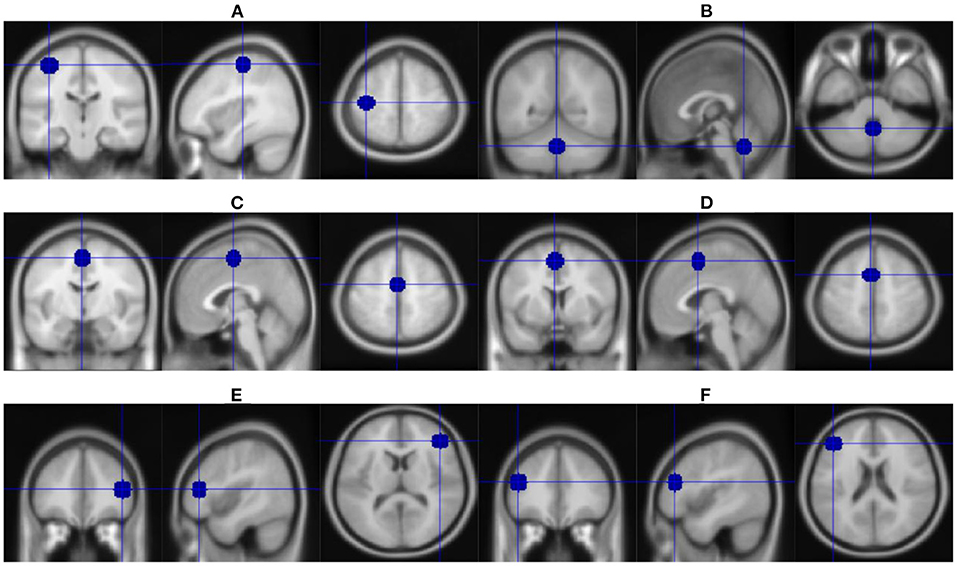
Figure 3. Overview of stimulation brain regions in studies mentioned in Tables 1, 2. (A) Left motor cortex. (B) Cerebellum. (C) Supplementary motor area. (D) Pre-supplementary motor area. (E) Right inferior frontal cortex. (F) Left dorsolateral prefrontal cortex.
The Therapeutic Mechanism of TMS in the Clinical Management of LID
Brain Connectivity
Electrophysiology and functional imaging are helpful to explore the role of brain connectivity in the occurrence of LID. Cross-frequency coupling (CFC) refers to a phenomenon that oscillations recorded by microelectrodes in various brain regions interact with each other (44). Such CFC is presented as a quantitative value to show inter-brain synchrony (44). It is revealed that CFC between MC and dorsolateral striatum was decreased in LID rats (44). After delivery of HF-rTMS, hippocampus-prefrontal CFC in patients with major depression was enhanced (45). How LF-rTMS and cTBS alleviate LID symptoms by the change of CFC remains unknown.
Neuroplasticity
MC lacks long-term potentiation (LTP) -like synaptic plasticity when levodopa is not being administered (46). LTP can be reversed in non-dyskinesia patients by administering levodopa (46). In LID patients, this therapeutic option may fail to reverse LTP (46). Deficiency of depotentiation exists in PD patients with LID (47). Theta and gamma wave patterns recorded by electroencephalography were found to be potent inducers of neuroplasticity (48). HF-rTMS was found to induce theta wave, and cTBS was found to induce theta and gamma in physiological conditions (49), which shows the capability of TMS to change neuroplasticity. Nevertheless, how rTMS-evoked neuroplasticity reverses dysfunctional neuroplasticity (that refers to lack of depotentiation) in the occurrence of LID and shows beneficial improvement on LID remains unknown.
Neurotransmitter and Receptor Modulation
Imbalanced neurotransmitters are the major pathological mechanisms for LID. Studies have explored the roles of neurotransmitters and their receptors in TMS. Elevated GABA receptor levels have been found in postmortem samples of LID patients (50). The N-methyl-D-aspartate receptor antagonist, dextrorphan hydrochloride, has been shown to improve LID clinical outcomes (51). HF-rTMS increases the expression of amino acids (taurine, aspartate, and serine) and dopamine in the hypothalamic paraventricular nucleus and dorsal hippocampus, respectively, and decreases expression of arginine vasopressin in the hypothalamic paraventricular nucleus in healthy brains of rats and mice (52). LF-rTMS was, however, not capable of exhibiting any changes in neurotransmitters (53). Both LF-rTMS (54) and HF-rTMS (55) bring about an imbalance between glutamate and glutamine in healthy human brains. It has been shown that cTBS decreases vesicular glutamate transporters one and increases plasmatic glutamate transporters one in healthy rat brains (56). Upregulation of glutamate transporter and GABA transporter mRNAs have been reported in TMS-treated mice (57). Studies have confirmed the pivotal roles of glutamatergic and GABAergic neurotransmitters during TBS (58). More studies are needed to evaluate the role of neurotransmitters and receptors in LID patients during TMS therapy (59).
Neurorestoration
Administration of glial cell line-derived neurotrophic factor (GDNF) improved LID both in patients and marmosets (60, 61). GDNF-mediated neurorestoration was revealed to selectively induce sprouting of dopaminergic cells without affecting GABAergic or serotonergic cells (62). In 50-sample animal research, rTMS alleviated LID with remarkably increased GDNF (63). However, cTBS alleviate LID with decreased brain-derived neurotrophic factor (BDNF) levels (18). Over-expression of BDNF was found to induce striatal serotonin fiber sprouting and lead to LID in 6-OHDA-lesioned rats (64). These findings suggest TMS might alleviate LID by sparing dopaminergic innervation and promoting serotonergic denervation. It is intriguing to note that different BDNF genotypes have a variable response to cTBS treatment (18). Val66Val carriers exhibited improvement of LID symptoms with decreased BDNF level after receiving cTBS treatment, while the Val66Met group showed no change for LID symptoms and the amount of BDNF as well (18).
Blood Flow and Glucose Metabolism
Blood flow and glucose metabolism dissociation in subcortical regions, especially putamen, has been found implicated in the occurrence of LID (65, 66). Blood flow increased while glucose metabolism decreased in the putamen, be it in the medicated or unmedicated state (65, 66). Bilateral cerebellum cTBS alleviates LID by reducing [18F]-fluorodeoxyglucose positron emission tomography metabolism in bilateral cerebellar hemispheres and dentate nucleus (15). This study suggests that metabolic changes might mediate the efficacy of TMS (15). Till now, none studies have unraveled the relation of blood flow with TMS in the management of LID. Nevertheless, many studies indeed identified blood flow alteration after TMS in a wide range of brain regions and various diseases. Blood flow and glucose metabolism may imply some beneficial effects of TMS on LID.
Prospects
To sum up, TMS has a neuromodulatory potential that might be successfully used in the clinical management of LID. However, more large randomized controlled studies of TMS application in LID are needed to understand better the underlying mechanisms, the efficacy evaluation, and optimization of stimulation protocols.
Author Contributions
YW: writing—original draft preparation. X-bC: conceptualization. W-qZ, HZ, X-qZ, X-mY, CC, J-lW, and X-mY: resources. YX: writing—reviewing and editing. All authors contributed to the article and approved the submitted version.
Funding
This work was supported by the National Key R&D Program of China (2017YFC1310300) and the National Natural Science Foundation of China (81873734, 81974200, and 81671108).
Conflict of Interest
The authors declare that the research was conducted in the absence of any commercial or financial relationships that could be construed as a potential conflict of interest.
Publisher's Note
All claims expressed in this article are solely those of the authors and do not necessarily represent those of their affiliated organizations, or those of the publisher, the editors and the reviewers. Any product that may be evaluated in this article, or claim that may be made by its manufacturer, is not guaranteed or endorsed by the publisher.
Acknowledgments
Thanks for free science providing language help.
Abbreviations
PD, Parkinson's disease; LID, levodopa-induced dyskinesia; TMS, transcranial magnetic stimulation; rTMS, repetitive TMS; HF-rTMS, high frequency rTMS; LF-rTMS, low frequency rTMS; TBS, theta burst stimulation; cTBS, continuous TBS; iTBS, intermittent TBS; MC, primary motor cortex; SMA, supplementary motor area; preSMA, pre-supplementary motor area; DLPFC, dorsolateral prefrontal cortex; IFC, inferior frontal cortex; SMA, supplementary motor area; SICI, short-interval intracortical inhibitions; LICI, long-latency intracortical inhibition; ICF, intracortical facilitation; SICF, short-interval intracortical facilitation; BDNF, brain-derived neurotrophic factor; GDNF, glial cell line-derived neurotrophic factor; LTP, long-term potentiation; MSO, maximum stimulator output; CFC, cross-frequency coupling; GABA, γ-Aminobutyric acid.
References
1. Tran TN, Vo TNN, Frei K, Truong DD. Levodopa-induced dyskinesia: clinical features, incidence, and risk factors. J Neural Transm. (2018) 125:1109–117. doi: 10.1007/s00702-018-1900-6
2. Aradi SD, Hauser RA. Medical management and prevention of motor complications in Parkinson's disease. Neurotherapeutics. (2020) 17:1339–65. doi: 10.1007/s13311-020-00889-4
3. Dragasevic-Miskovic N, Petrovic I, Stankovic I, Kostic VS. Chemical management of levodopa-induced dyskinesia in Parkinson's disease patients. Expert Opin Pharmacother. (2019) 20:219–30. doi: 10.1080/14656566.2018.1543407
4. Martini ML, Mocco J, Panov F. Neurosurgical approaches to levodopa-induced dyskinesia. World Neurosurg. (2019) 126:376–82. doi: 10.1016/j.wneu.2019.03.056
5. Koch G, Brusa L, Caltagirone C, Peppe A, Oliveri M, Stanzione P, et al. rTMS of supplementary motor area modulates therapy-induced dyskinesias in Parkinson disease. Neurology. (2005) 65:623–5. doi: 10.1212/01.wnl.0000172861.36430.95
6. Brusa L, Versace V, Koch G, Iani C, Stanzione P, Bernardi G, et al. Low frequency rTMS of the SMA transiently ameliorates peak-dose LID in Parkinson's disease. Clin Neurophysiol. (2006) 117:1917–21. doi: 10.1016/j.clinph.2006.03.033
7. Wagle-Shukla A, Angel MJ, Zadikoff C, Enjati M, Gunraj C, Lang AE, et al. Low-frequency repetitive transcranial magnetic stimulation for treatment of levodopa-induced dyskinesias. Neurology. (2007) 68:704–5. doi: 10.1212/01.wnl.0000256036.20927.a5
8. Filipovic SR, Rothwell JC, van de Warrenburg BP, Bhatia K. Repetitive transcranial magnetic stimulation for levodopa-induced dyskinesias in Parkinson's disease. Mov Disord. (2009) 24:246–53. doi: 10.1002/mds.22348
9. Sayin S, Cakmur R, Yener GG, Yaka E, Ugurel B, Uzunel F. Low-frequency repetitive transcranial magnetic stimulation for dyskinesia and motor performance in Parkinson's disease. J Clin Neurosci. (2014) 21:1373–6. doi: 10.1016/j.jocn.2013.11.025
10. Anja F, Ann CA, Sylvie DRA, Veronique MA, Sara S, Annick VMA, et al. Bilateral low frequency rTMS of the primary motor cortex may not be a suitable treatment for levodopa-induced dyskinesias in late stage Parkinson's disease. Parkinsonism Relat Disord. (2016) 22:54–61. doi: 10.1016/j.parkreldis.2015.11.009
11. Min Y, Park JW, Jin SU, Jang KE, Lee B, Lee HJ, et al. Neuromodulatory effects of offline low-frequency repetitive transcranial magnetic stimulation of the motor cortex: a functional magnetic resonance imaging study. Sci Rep. (2016) 6:36058. doi: 10.1038/srep36058
12. Lohse A, Meder D, Nielsen S, Lund AE, Herz DM, Løkkegaard A, et al. Low-frequency transcranial stimulation of pre-supplementary motor area alleviates levodopa-induced dyskinesia in Parkinson's disease: a randomized cross-over trial. Brain Commun. (2020) 2:fcaa147. doi: 10.1093/braincomms/fcaa147
13. Flamez A, Wu G, Wiels W, Van Schuerbeek P, De Mey J, De Keyser J, et al. Opposite effects of one session of 1 Hz rTMS on functional connectivity between pre-supplementary motor area and putamen depending on the dyskinesia state in Parkinson's disease. Clin Neurophysiol. (2021) 132:851–856. doi: 10.1016/j.clinph.2020.12.024
14. Koch G, Brusa L, Carrillo F, Lo Gerfo E, Torriero S, Oliveri M, et al. Cerebellar magnetic stimulation decreases levodopa-induced dyskinesias in Parkinson disease. Neurology. (2009) 73:113–9. doi: 10.1212/WNL.0b013e3181ad5387
15. Brusa L, Ceravolo R, Kiferle L, Monteleone F, Iani C, Schillaci O, et al. Metabolic changes induced by theta burst stimulation of the cerebellum in dyskinetic Parkinson's disease patients. Parkinsonism Relat Disord. (2012) 18:59–62. doi: 10.1016/j.parkreldis.2011.08.019
16. Cerasa A, Koch G, Donzuso G, Mangone G, Morelli M, Brusa L, et al. A network centred on the inferior frontal cortex is critically involved in levodopa-induced dyskinesias. Brain. (2015) 138:414–27. doi: 10.1093/brain/awu329
17. Ponzo V, Picazio S, Benussi A, Di Lorenzo F, Brusa L, Caltagirone C, et al. Altered inhibitory interaction among inferior frontal and motor cortex in l-dopa-induced dyskinesias. Mov Disord. (2016) 31:755–9. doi: 10.1002/mds.26520
18. Sanna A, Follesa P, Puligheddu M, Cannas A, Serra M, Pisu MG, et al. Cerebellar continuous theta burst stimulation reduces levodopa-induced dyskinesias and decreases serum BDNF levels. Neurosci Lett. (2020) 716:134653. doi: 10.1016/j.neulet.2019.134653
19. Rektorova I, Sedlackova S, Telecka S, Hlubocky A, Rektor I. Dorsolateral prefrontal cortex: a possible target for modulating dyskinesias in Parkinson's disease by repetitive transcranial magnetic stimulation. Int J Biomed Imaging. (2008) 2008:372125. doi: 10.1155/2008/372125
20. Huang Y, Edwards MJ, Rounis E, Bhatia KP, Rothwell JC. Theta burst stimulation of the human motor cortex. Neuron. (2005) 45:201–6. doi: 10.1016/j.neuron.2004.12.033
21. Kujirai T, Caramia MD, Rothwell JC, Day BL, Thompson PD, Ferbert A, et al. Corticocortical inhibition in human motor cortex. The Journal of physiology. (1993) 471:501–19. doi: 10.1113/jphysiol.1993.sp019912
22. Chen R, Cros D, Curra A, Di Lazzaro V, Lefaucheur J, Magistris MR, et al. The clinical diagnostic utility of transcranial magnetic stimulation: report of an IFCN committee. Clin Neurophysiol. (2008) 119:504–32. doi: 10.1016/j.clinph.2007.10.014
23. Barbin L, Leux C, Sauleau P, Meyniel C, Nguyen J, Pereon Y, et al. Non-homogeneous effect of levodopa on inhibitory circuits in Parkinson's disease and dyskinesia. Parkinsonism Relat D. (2013) 19:165–70. doi: 10.1016/j.parkreldis.2012.08.012
24. Pierantozzi M, Palmieri MG, Marciani MG, Bernardi G, Giacomini P, Stanzione P. Effect of apomorphine on cortical inhibition in Parkinson's disease patients: a transcranial magnetic stimulation study. Exp Brain Res. (2001) 141:52–62. doi: 10.1007/s002210100839
25. Turjanski N, Lees AJ. Gamma vinyl GABA in the treatment of levodopa-induced dyskinesias in Parkinson's disease. J Neurol Neurosurg Psychiatry. (1992) 55:413. doi: 10.1136/jnnp.55.5.413
26. Berardelli A, Abbruzzese G, Chen R, Orth M, Ridding MC, Stinear C, et al. Consensus paper on short-interval intracortical inhibition and other transcranial magnetic stimulation intracortical paradigms in movement disorders. Brain Stimul. (2008) 1:183–91. doi: 10.1016/j.brs.2008.06.005
27. Nakamura H, Kitagawa H, Kawaguchi Y, Tsuji H. Intracortical facilitation and inhibition after transcranial magnetic stimulation in conscious humans. J Physiol. (1997) 498:817–23. doi: 10.1113/jphysiol.1997.sp021905
28. Sanger TD, Garg RR, Chen R. Interactions between two different inhibitory systems in the human motor cortex. J Physiol. (2001) 530:307–17. doi: 10.1111/j.1469-7793.2001.0307l.x
29. Ziemann U, Tergau F, Wassermann EM, Wischer S, Hildebrandt J, Paulus W. Demonstration of facilitatory I wave interaction in the human motor cortex by paired transcranial magnetic stimulation. J Physiol. (1998) 511:181–90. doi: 10.1111/j.1469-7793.1998.181bi.x
30. Guerra A, Suppa A, D'Onofrio V, Di Stasio F, Asci F, Fabbrini G, et al. Abnormal cortical facilitation and L-dopa-induced dyskinesia in Parkinson's disease. Brain Stimul. (2019) 12:1517–25. doi: 10.1016/j.brs.2019.06.012
31. Pascual-Leone A, Valls-Solé J, Wassermann EM, Hallett M. Responses to rapid-rate transcranial magnetic stimulation of the human motor cortex. Brain. (1994) 117 (Pt 4):847–58. doi: 10.1093/brain/117.4.847
32. Chen R, Classen J, Gerloff C, Celnik P, Wassermann EM, Hallett M, et al. Depression of motor cortex excitability by low-frequency transcranial magnetic stimulation. Neurology. (1997) 48:1398–403. doi: 10.1212/WNL.48.5.1398
33. Ueno T, Yamada J, Nishijima H, Arai A, Migita K, Baba M, et al. Morphological and electrophysiological changes in intratelencephalic-type pyramidal neurons in the motor cortex of a rat model of levodopa-induced dyskinesia. Neurobiol Dis. (2014) 64:142–9. doi: 10.1016/j.nbd.2013.12.014
34. Cerasa A, Messina D, Pugliese P, Morelli M, Lanza P, Salsone M, et al. Increased prefrontal volume in PD with levodopa-induced dyskinesias: a voxel-based morphometry study. Movement Disord. (2011) 26:807–12. doi: 10.1002/mds.23660
35. Rascol O, Sabatini U, Brefel C, Fabre N, Rai S, Senard JM, et al. Cortical motor overactivation in parkinsonian patients with L-dopa-induced peak-dose dyskinesia. Brain. (1998) 121 (Pt 3):527–33. doi: 10.1093/brain/121.3.527
36. Cerasa A, Pugliese P, Messina D, Morelli M, Cecilia Gioia M, Salsone M, et al. Prefrontal alterations in Parkinson's disease with levodopa-induced dyskinesia during fMRI motor task. Movement Disord. (2012) 27:364–71. doi: 10.1002/mds.24017
37. Aron AR. Cortical and subcortical contributions to stop signal response inhibition: role of the subthalamic nucleus. J Neurosci. (2006) 26:2424–33. doi: 10.1523/JNEUROSCI.4682-05.2006
38. Herz DM, Haagensen BN, Christensen MS, Madsen KH, Rowe JB, Lokkegaard A, et al. The acute brain response to levodopa heralds dyskinesias in Parkinson disease. Ann Neurol. (2014) 75:829–36. doi: 10.1002/ana.24138
39. Ikeda A, Yazawa S, Kunieda T, Ohara S, Terada K, Mikuni N, et al. Cognitive motor control in human pre-supplementary motor area studied by subdural recording of discrimination/selection-related potentials. Brain. (1999) 122:915–31. doi: 10.1093/brain/122.5.915
40. Brooks DJ, Piccini P, Turjanski N, Samuel M. Neuroimaging of dyskinesia. Ann Neurol. (2000) 47:S154–8; discussion S158–9.
41. Lewis MM, Du G, Kidacki M, Patel N, Shaffer ML, Mailman RB, et al. Higher iron in the red nucleus marks Parkinson's dyskinesia. Neurobiol Aging. (2013) 34:1497–503. doi: 10.1016/j.neurobiolaging.2012.10.025
42. Kishore A, Popa T, Balachandran A, Chandran S, Pradeep S, Backer F, et al. Cerebellar sensory processing alterations impact motor cortical plasticity in Parkinson's disease: clues from dyskinetic patients. Cereb Cortex. (2014) 24:2055–67. doi: 10.1093/cercor/bht058
43. Yoo HS, Choi YH, Chung SJ, Lee YH, Ye BS, Sohn YH, et al. Cerebellar connectivity in Parkinson's disease with levodopa-induced dyskinesia. Ann Clin Transl Neurol. (2019) 6:2251–60. doi: 10.1002/acn3.50918
44. Belić JJ, Halje P, Richter U, Petersson P, Hellgren Kotaleski J. Untangling Cortico-striatal connectivity and cross-frequency coupling in L-DOPA-induced dyskinesia. Front Syst Neurosci. (2016) 10:26. doi: 10.3389/fnsys.2016.00026
45. Noda Y, Zomorrodi R, Daskalakis ZJ, Blumberger DM, Nakamura M. Enhanced theta-gamma coupling associated with hippocampal volume increase following high-frequency left prefrontal repetitive transcranial magnetic stimulation in patients with major depression. Int J Psychophysiol. (2018) 133:169–74. doi: 10.1016/j.ijpsycho.2018.07.004
46. Morgante F, Espay AJ, Gunraj C, Lang AE, Chen R. Motor cortex plasticity in Parkinson's disease and levodopa-induced dyskinesias. Brain. (2006) 129:1059–69. doi: 10.1093/brain/awl031
47. Lago-Rodriguez A, Ponzo V, Jenkinson N, Benitez-Rivero S, Del-Olmo MF, Hu M, et al. Paradoxical facilitation after depotentiation protocol can precede dyskinesia onset in early Parkinson's disease. Exp Brain Res. (2016) 234:3659–67. doi: 10.1007/s00221-016-4759-5
48. Larson J, Lynch G. Induction of synaptic potentiation in hippocampus by patterned stimulation involves two events. Science. (1986) 232:985–8. doi: 10.1126/science.3704635
49. Bergmann TO, Karabanov A, Hartwigsen G, Thielscher A, Siebner HR. Combining non-invasive transcranial brain stimulation with neuroimaging and electrophysiology: current approaches and future perspectives. Neuroimage. (2016) 140:4–19. doi: 10.1016/j.neuroimage.2016.02.012
50. Calon F, Morissette M, Rajput AH, Hornykiewicz O, Bedard PJ, Di Paolo T. Changes of GABA receptors and dopamine turnover in the postmortem brains of parkinsonians with levodopa-induced motor complications. Mov Disord. (2003) 18:241–53. doi: 10.1002/mds.10343
51. Blanchet PJ, Metman LV, Mouradian MM, Chase TN. Acute pharmacologic blockade of dyskinesias in Parkinson's disease. Mov Disord. (1996) 11:580–1. doi: 10.1002/mds.870110516
52. Keck ME, Sillaber I, Ebner K, Welt T, Toschi N, Kaehler ST, et al. Acute transcranial magnetic stimulation of frontal brain regions selectively modulates the release of vasopressin, biogenic amines and amino acids in the rat brain. Eur J Neurosci. (2000) 12:3713–20. doi: 10.1046/j.1460-9568.2000.00243.x
53. Poh EZ, Hahne D, Moretti J, Harvey AR, Clarke MW, Rodger J. Simultaneous quantification of dopamine, serotonin, their metabolites and amino acids by LC-MS/MS in mouse brain following repetitive transcranial magnetic stimulation. Neurochem Int. (2019) 131:104546. doi: 10.1016/j.neuint.2019.104546
54. Tremblay S, Beaulé V, Proulx S, de Beaumont L, Marjańska M, Doyon J, et al. Relationship between transcranial magnetic stimulation measures of intracortical inhibition and spectroscopy measures of GABA and glutamate+glutamine. J Neurophysiol. (2013) 109:1343–9. doi: 10.1152/jn.00704.2012
55. Michael N, Gösling M, Reutemann M, Kersting A, Heindel W, Arolt V, et al. Metabolic changes after repetitive transcranial magnetic stimulation (rTMS) of the left prefrontal cortex: a sham-controlled proton magnetic resonance spectroscopy (1 H MRS) study of healthy brain. Eur J Neurosci. (2003) 17:2462–8. doi: 10.1046/j.1460-9568.2003.02683.x
56. Mancic B, Stevanovic I, Ilic TV, Djuric A, Stojanovic I, Milanovic S, et al. Transcranial theta-burst stimulation alters GLT-1 and vGluT1 expression in rat cerebellar cortex. Neurochem Int. (2016) 100:120–7. doi: 10.1016/j.neuint.2016.09.009
57. Ikeda T, Kobayashi S, Morimoto C. Effects of repetitive transcranial magnetic stimulation on ER stress-related genes and glutamate, γ-aminobutyric acid and glycine transporter genes in mouse brain. Biochem Biophys Rep. (2019) 17:10–16. doi: 10.1016/j.bbrep.2018.10.015
58. Li CT, Huang YZ, Bai YM, Tsai SJ, Su TP, Cheng CM. Critical role of glutamatergic and GABAergic neurotransmission in the central mechanisms of theta-burst stimulation. Hum Brain Mapp. (2018) 40:2001–9. doi: 10.1002/hbm.24485
59. Wei H, Zhou Y, Zhao J, Zhan L. Risk factors and metabolism of different brain regions by positron emission tomography in Parkinson disease with disabling dyskinesia. Curr Neurovasc Res. (2019) 16:310–20. doi: 10.2174/1567202616666191009102112
60. Jenner P. Pathophysiology and biochemistry of dyskinesia: clues for the development of non-dopaminergic treatments. J Neurol. (2000) 247(Suppl. 2):II43–50. doi: 10.1007/pl00007760
61. Gill SS, Patel NK, Hotton GR, O'Sullivan K, McCarter R, Bunnage M, et al. Direct brain infusion of glial cell line-derived neurotrophic factor in Parkinson disease. Nat Med. (2003) 9:589–95. doi: 10.1038/nm850
62. Wu SS, Frucht SJ. Treatment of Parkinson's disease: what's on the horizon? CNS Drugs. (2005) 19:723–43. doi: 10.2165/00023210-200519090-00001
63. Ba M, Kong M, Guan L, Yi M, Zhang H. Repetitive transcranial magnetic stimulation (rTMS) improves behavioral and biochemical deficits in levodopa-induced dyskinetic rats model. Oncotarget. (2016) 7:58802–812. doi: 10.18632/oncotarget.11587
64. Tronci E, Napolitano F, Munoz A, Fidalgo C, Rossi F, Bjorklund A, et al. BDNF over-expression induces striatal serotonin fiber sprouting and increases the susceptibility to l-DOPA-induced dyskinesia in 6-OHDA-lesioned rats. Exp Neurol. (2017) 297:73–81. doi: 10.1016/j.expneurol.2017.07.017
65. Jourdain VA, Tang CC, Holtbernd F, Dresel C, Choi YY, Ma Y, et al. Flow-metabolism dissociation in the pathogenesis of levodopa-induced dyskinesia. JCI Insight. (2016) 1:e86615. doi: 10.1172/jci.insight.86615
Keywords: transcranial magnetic stimulation, Parkinson's disease, dyskinesia, mechanism, treatment
Citation: Wu Y, Cao X-b, Zeng W-q, Zhai H, Zhang X-q, Yang X-m, Cheng C, Wang J-l, Yang X-m and Xu Y (2021) Transcranial Magnetic Stimulation Alleviates Levodopa-Induced Dyskinesia in Parkinson's Disease and the Related Mechanisms: A Mini-Review. Front. Neurol. 12:758345. doi: 10.3389/fneur.2021.758345
Received: 13 August 2021; Accepted: 04 October 2021;
Published: 08 November 2021.
Edited by:
Marina Picillo, University of Salerno, ItalyReviewed by:
Francesco Di Lorenzo, Santa Lucia Foundation (IRCCS), ItalyKaviraja Udupa, National Institute of Mental Health and Neurosciences, India
Kai-Hsiang Stanley Chen, National Taiwan University Hospital Hsin-Chu Branch, Taiwan
Copyright © 2021 Wu, Cao, Zeng, Zhai, Zhang, Yang, Cheng, Wang, Yang and Xu. This is an open-access article distributed under the terms of the Creative Commons Attribution License (CC BY). The use, distribution or reproduction in other forums is permitted, provided the original author(s) and the copyright owner(s) are credited and that the original publication in this journal is cited, in accordance with accepted academic practice. No use, distribution or reproduction is permitted which does not comply with these terms.
*Correspondence: Yan Xu, xuyanwxf@126.com
†These authors have contributed equally to this work and share first authorship