- 1Department of Translational Medicine, University of Campinas, Campinas, Brazil
- 2Brazilian Institute of Neuroscience and Neurotechnology, Campinas, Brazil
- 3Department of Neurology, University of Campinas, Campinas, Brazil
- 4Department of Pathology, University of Campinas, Campinas, Brazil
- 5Department of Neuroscience and Behavioral Science, Ribeirão Preto Medical School, University of São Paulo, Ribeirão Preto, Brazil
- 6Department of Internal Medicine, Federal University of Minas Gerais, Belo Horizonte, Brazil
We aimed to investigate the role of interleukin-1 beta (IL-1β) in the mechanisms underlying mesial temporal lobe epilepsy with hippocampal sclerosis (MTLE+HS). We assessed a cohort of 194 patients with MTLE+HS and 199 healthy controls. Patients were divided into those with positive and negative antecedent febrile seizures (FS). We used a multidimensional approach, including (i) genetic association with single nucleotide polymorphisms (SNPs) in the IL1B gene; (ii) quantification of the IL1B transcript in the hippocampal tissue of patients with refractory seizures; and (iii) quantification of the IL-1β protein in the plasma. We found a genetic association signal for two SNPs, rs2708928 and rs3730364*C in the IL1B gene, regardless of the presence of FS (adjusted p = 9.62e–11 and 5.14e–07, respectively). We found no difference between IL1B transcript levels when comparing sclerotic hippocampal tissue from patients with MTLE+HS, without FS, and hippocampi from autopsy controls (p > 0.05). Nevertheless, we found increased IL-1β in the plasma of patients with MTLE+HS with FS compared with controls (p = 0.0195). Our results support the hypothesis of a genetic association between MTLE+HS and the IL1B gene
Introduction
Mesial temporal lobe epilepsy with hippocampal sclerosis (MTLE+HS) is the most common and usually pharmacoresistant form of focal epilepsy in the adult population (1). In addition, MTLE+HS has been associated with the presence of an initial precipitating injury (IPI) in some patients, including trauma, status epilepticus, infections, and childhood febrile seizures (FS) (2).
Clinical and experimental evidence indicates a link between initial precipitating injuries (IPIs) and neuroinflammation, leading to the development of epilepsy (3–7). Furthermore, it has been suggested that the interleukin-1 family may play a role in the development of FS and subsequently in MTLE+HS (8, 9). Among the interleukin-1 family, interleukin-1 beta (IL-1β) is the primary cytokine responsible for intermediating human febrile responses (7, 8). Indeed, many studies have shown a link between IL-1β and MTLE (10–16). The use of an anticytokine compound to block IL-1β synthesis in the kindling animal model suggests that IL-1β is involved in kindling progression (17). In addition, a subtle increase in the levels of IL-1β was observed in plasma from children with febrile status epilepticus, as part of the FEBSTAT study (14).
Altogether, these findings raise the hypothesis that genetic variation in the IL1B gene could lead to abnormal levels of IL1B RNA transcripts and consequently of IL-1β protein levels, which, in turn, could influence the development of MTLE+HS (18). In fact, a previous study has identified a genetic association between the single nucleotide polymorphism (SNP) rs16944 and patients with MTLE+HS in the Japanese population. This SNP is located in the promoter region of the IL1B (c.-511C>T), suggesting that this change may be associated with abnormal levels of IL-1β (10, 19). However, these results were not replicated in subsequent studies in patients of European and Chinese origins (19–23). More recently, a systematic meta-analysis including 17 studies of European and Chinese children with FS suggested that the SNP rs16944 on IL1B could be a risk factor for FS and the subsequent development of MTLE+HS (24).
In this context, we aimed to assess a cohort of patients with MTLE+HS to explore the role of IL-1β at three different molecular domains: (i) a genetic association study with polymorphisms covering the IL1B gene; (ii) quantification of the IL1B transcript in the hippocampal tissue from patients with pharmacoresistant MTLE+HS; and (iii) IL-1β protein quantification in the plasma of patients with MTLE+HS.
Materials and Methods
We included 194 unrelated patients with MTLE+HS from two clinical centers. One hundred and seventy patients were followed at the epilepsy clinic of the University of Campinas (UNICAMP) University Hospital and 24 at the epilepsy clinic of the University of São Paulo at Ribeirão Preto (USP-RP) University Hospital. This study was approved by the research ethics committee of the UNICAMP and USP-RP, and written informed consent was obtained from all participants.
Characterization of the Patients
Only adults were included in this study; the mean age of the patients was 45.7 years old, ranging from 23 to 90 years of age. MTLE+HS was confirmed in all the patients according to the International League Against Epilepsy (ILAE) criteria (25). Clinical evaluation was performed by experienced neurologists in the management of the patients with epilepsy. All the patients were interviewed using a structured questionnaire to collect information about age, the onset of epilepsy, detailed seizure semiology, history of FS, other IPIs, family history of epilepsy, and the number of antiseizure medications used. A positive antecedent of FS was registered if confirmed by a relative or in the presence of medical records. In addition, all the patients had a neurological examination, serial interictal electroencephalograms, and high-resolution magnetic resonance imaging (MRI) with a specific epilepsy protocol to identify hippocampal atrophy or other MRI signs of HS (26).
Patients with dual pathology (HS associated with other structural lesions on MRI), mental retardation, associated progressive neurologic diseases, autoimmune diseases, cancer, or major chronic debilitating conditions such as renal failure or hepatic failure were not included in this study.
Characterization of the Control Group
The control group included 199 unrelated healthy individuals, mainly unrelated spouses of the patients. None of the control subjects had an antecedent of epilepsy or FS in their own family (blood relatives). In addition, 41 control individuals had an MRI to confirm the absence of hippocampal abnormalities.
MRI Acquisition
The MRIs were acquired using a 3T scanner (Philips Achieva) in coronal, sagittal, and axial planes, as well as 3D T1 high-resolution with 1-mm3 voxel size. The coronal images for visual analysis were obtained perpendicular to the long axis of the hippocampus and included 1-mm T1-weighted, 3-mm T1-inversion recovery, 3-mm fast spin-echo T2-weighted, 3-mm T2-weighted multiecho, and 1- or 3-mm FLAIR (fluid-attenuated inversion recovery). MRI signs of HS include hippocampal atrophy, increased T2 and FLAIR signal intensity, and abnormal shape and internal structure of the hippocampus (27), which were defined by visual analysis and confirmed by hippocampal volumetry and T2 relaxometry as described previously (26).
SNP Genotyping
Genomic DNA was isolated from peripheral blood from 194 patients with MTLE+HS and 199 controls using the phenol-chloroform method (28). DNA samples were quantified using a spectrophotometer (NanoVue, GE Healthcare, Pittsburgh, PA, USA), and samples with a 260/280 ratio ≥ 1.7 were used in the study.
We selected SNPs located within the IL1B gene by the SNPBROWSERTM 4.0 software (Applied Biosystems, Foster City, CA, USA) based on the SNPs from the HapMap project (29), a minimum allele frequency (MAF) > 0.01, and linkage disequilibrium (r2) > 0.8. To verify the presence of population stratification, we genotyped additional 23 SNPs as a genomic control on chromosome 2 (Supplementary Table 1). These were randomly selected using an in-house algorithm developed in the R software (30). These SNPs were located outside the IL1B region, with a minimum distance of 0.3 cM (300 kb) between each other, to avoid possible linkage disequilibrium (31). We focused on using SNPs on chromosome 2 for the genomic control because previous studies showed differences in fixation index (Fst) values among chromosomes, and the presence of natural selection imprint observed in many regions of the genome could create significant variation in Fst, a phenomenon that could lead to biased global Fst values (32).
SNPs were genotyped using the SNPlexTM Genotyping System 48-plex technology (Applied Biosystems). Allelic discrimination was performed through a capillary electrophoresis analysis with the Applied Biosystems 3130xl DNA Analyzer and the GeneMapperTM 4.0 software. Also, we genotyped the rs16944 allele by polymerase chain reaction (PCR) amplification of a 204-bp fragment and restriction fragment length polymorphism (RFLP) using the following primers: 5′-GGCTAGGGTAACAGCACCTG-3′ and 5′-TGAGGGTGTGGGTCTCTACC-3′. PCR conditions were as follows: a denaturing step of 94°C for 10 min, then 35 cycles of 94°C for 20 s, 55°C for 20 s, 72°C for 40 s, and a final incubation at 72°C for 10 min. RFLP analysis was performed using 5 U DdeI (Thermo Scientific, San Jose, CA, USA) at 37°C overnight, and PCR products were run on a 12% polyacrylamide gel followed by ethidium bromide staining. PCR products were cut in three fragments of 131, 42, and 31 bp for the rs16944*A allele or two fragments of 131 and 73 bp for the rs16944*G allele.
IL1B RNA Quantification in Hippocampal Tissue
To investigate the IL1B RNA levels in sclerotic tissue, we used hippocampal specimens from four patients with MTLE+HS, who underwent a standard anteromedial temporal resection to treat pharmacoresistant seizures at the UNICAMP University Hospital. None of the four patients with MTLE+HS presented antecedent FS. We compared these samples with six autopsy controls, whose cause of death was other than central nervous system diseases. All tissues were evaluated by a neuropathologist, who confirmed hippocampal sclerosis in samples from patients, and no histopathological abnormalities in autopsy tissue used as a control.
The hippocampal specimens were frozen in liquid nitrogen immediately after resection and stored at −80°C until processing. Total RNA was obtained using a TRIzol® Reagent (Invitrogen, Carlsbad, CA, USA). RNA samples were quantified by a spectrophotometer (NanoVue GE Health Care, Chicago, IL, USA), and samples that met the requirement of a 260/280 ratio ≥ 1.9 were used in the study. The RNA integrity was checked on a 1% agarose gel. According to the instructions of the manufacturer, 1 μg of total RNA was reverse-transcribed into complementary DNA (cDNA) using SuperScript IIITM Reverse Transcriptase (Invitrogen, Carlsbad, CA, USA).
IL1B transcript quantification was carried out using a TaqMan® Gene Expression Assay (Hs01555410_m1) in an ABI 7500 Real-Time PCR system (Applied Biosystems, Waltham, MA, USA) using SYP (synaptophysin, Hs00300531_m1) and SDHA (succinate dehydrogenase, subunit A, Hs00417200_m1) as endogenous control genes because they were previously validated for the normalization of target genes in human hippocampal samples obtained from patients with MTLE (33). Reactions were performed in triplicate, and the relative quantification (RQ) was calculated according to the equation RQ = 2−ΔΔCT (34). Data were analyzed using the SDS 7500 software (Applied Biosystems, Waltham, MA, USA).
IL-1β Protein Quantification in Plasma
We obtained plasma samples from 108 patients with MTLE+HS and 75 controls. Of these, 90 patients had an antecedent of FS. Samples were collected and analyzed blindly regarding clinical status. Also, all samples were collected in the morning to avoid influences from the circadian cycle. We did not collect the blood in patients presenting any symptoms of infectious disease. Blood samples were centrifuged for 10 min at 3,000 rpm at 4°C, and plasma was collected and stored in a −80°C freezer until analyzed. IL-1β levels in plasma were measured by enzyme-linked immunosorbent assay (ELISA) using a high sensitivity immunoassay kit (Human IL-1Beta Quantikine High Sensitivity ELISA, R&D Systems, Minneapolis, MN, USA). Samples were analyzed in duplicate, and concentrations are expressed as pg/ml. The lower detection limit for IL-1β is 0.023 pg/ml.
We compared the proportion of patients who had seizures <24 h and more than 24 h before the blood collection and the proportion of patients with depressive symptoms in the patients' subgroups with and without FS using Fisher's exact test; these variables could affect the IL-1β plasma levels. In addition, we assessed depressive symptoms by the Beck Depression Inventory (BDI-II) scores.
Statistical Analysis
We compared patients and controls for sex distribution using Fisher's exact test and mean age using Student's t-test, both performed in the R software (www.r-project.org). The SNPs were filtered by minimum allele frequency (MAF > 0.01) and Hardy–Weinberg disequilibrium (p < 0.01) by the PLINK v1.9 software (35). We also evaluated the linkage disequilibrium in terms of r2 among SNPs by the HAPLOVIEW software (36).
Population stratification is a known phenomenon that could lead to biased genetic association results (37, 38). Therefore, to assess whether our sample of patients and controls presents population stratification, we performed an analysis of molecular variance (AMOVA) using the ARLEQUIN software (39), based on the 23 genomic control SNPs. The AMOVA approach divides the source of genetic variance (σ2) into two components: within-groups and between-groups. Under a null hypothesis, the samples were obtained from a global population, and the variation is due to random sampling in the construction of populations. Thus, we would expect a high variation within-groups (σ2 = 100%) and no variation between-groups (σ2 = 0%). On the other hand, under the alternative hypothesis of population stratification, each group was obtained from different populations, and we would expect a low variation within-groups (σ2 <100%) and high variation between-groups (σ2 > 0%) (40).
The genetic association between SNPs in IL1B and MTLE+HS was estimated using an additive logistic regression model by the PLINK v1.9 software (35). Because we performed two comparisons (controls vs. MTLE+HS with FS and controls vs. MTLE+HS without FS), we adjusted p-values with the Bonferroni correction to account for multiple comparisons. We also estimated the effect size in terms of an odds ratio (OR) with a 95% confidence interval (CI). To evaluate the sensitivity of our sample to detect an association, we estimated the effect size in terms of OR using the G*POWER software (41), including the following parameters: logistic regression model; two-sided analysis; a level of significance α = 0.00625 (adjusted by Bonferroni correction); statistical power 1–β = 0.80; and total sample = 393.
We used the Wilcoxon rank-sum test, using the Wilcox.test function in the R software, to compare the RQ of the IL1B RNA transcript from the hippocampal tissue between patients and controls for both endogenous controls (SYP and SDHA). We also used the Wilcoxon rank-sum test to compare the IL-1β plasma levels in two scenarios: MTLE+HS with FS and controls and between MTLE+HS without FS and controls. Because we performed two comparisons, p-values were adjusted by the Bonferroni correction. The effect size was also calculated in R, including the 95% CI, using the Wilcox.test function.
Results
Subjects
Demographic and clinical data are shown in Table 1. Among the patients, the mean age was 45.7 years old, ranging from 23 to 90 years old (standard deviation [SD] = 11.2), and the mean age at seizure onset was 11.14 years old, ranging from 1 to 53 years old (standard deviation [SD] = 9.53). Patients with MTLE+HS were found to be older than controls (Student's t-test p = 0.011). Although we did not observe differences in the sex distribution between the two groups (Fisher's exact test p = 0.361), we observe an imbalance in the female/male ratio between patients with MTLE+HS (ratio = 1.18) and control individuals (ratio = 0.7). Therefore, we included age and sex as covariates to adjust the logistic regression model. We also identified 40 patients with an antecedent of FS (20.6%). All 194 patients presented hippocampal sclerosis, and all 41 normal controls who had MRIs did not present any signs of hippocampal sclerosis.
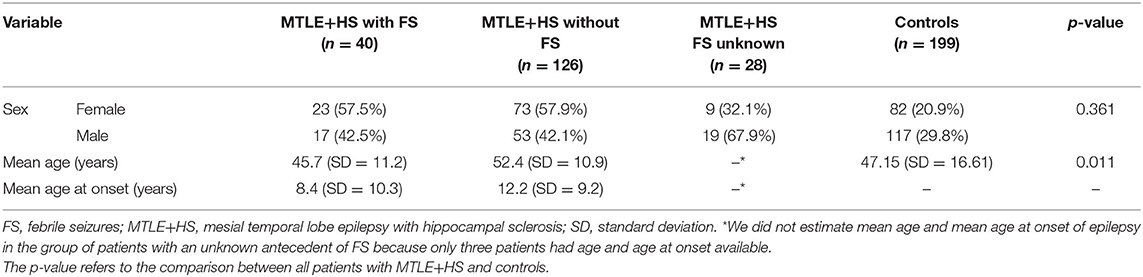
Table 1. Descriptive statistics of patients with MTLE+HS and controls ascertained for the genetic association study.
IL1B Candidate Gene Association Analysis
We found that our cohort can detect a genetic association with 80% statistical power with an OR <0.63 (protective allele effect) or an OR > 1.58 (increased risk allele effect). Furthermore, the AMOVA results also showed that 99.35% of the variation component was observed within groups, and 0.65% was observed between groups (Table 2), indicating that the sample does not present population stratification. Hence, the sample provides unbiased genetic association results.
The eight SNPs genotyped in the IL1B gene (Table 3) presented a mean genotype call rate of 90.87%. Two SNPs presented Hardy–Weinberg disequilibrium (rs3917365, p = 8.34e-07; rs1143633, p = 2.07e-24) and were excluded from the logistic regression analysis. In addition, SNPs rs3917368 and rs1143646 presented linkage disequilibrium (r2 = 0.96). As shown in Table 3, the SNP rs16944—located in the promoter region of the IL1B gene—showed no association with MTLE+HS and a small effect for both MTLE+HS with FS (rs16944*T: OR = 1.06, 95% CI = 0.76–1.46, p = 1.000) and MTLE+HS without FS (rs16944*T: OR = 0.98, 95% CI = 0.70–1.39, p = 1.000). By contrast, two other SNPs showed evidence of association with the MTLE+HS with the FS phenotype (rs2708928, adjusted p = 9.62e−11; rs3730364, adjusted p = 5.14e−07). These two SNPs presented a protective effect (rs2708928*G: OR = 0.10, 95% CI = 0.05–0.20; rs3730364*T: OR = 0.38, 95% CI = 0.26–0.54), while other alleles were associated with an increased risk effect (rs2708928*C: OR = 9.66, 95% CI = 5.01–18.60; rs3730364*C: OR = 2.66, 95% CI = 1.86–3.79). Additional analyses, in which patients with MTLE+HS with FS were removed, showed similar results for the SNPs rs2708928 (adjusted p = 2.05e−10) and rs3730364 (adjusted p = 1.34e−05), including similar estimates for the OR (Table 3).
Even though rs2708928 and rs3730364 are located in intronic regions, we decided to investigate possible functional implication by predicting the impact on protein function using six algorithms present in the Ensembl Variant Effect Predictor (VEP) (42), which included PolyPhen2 (43), Sort Intolerant from Tolerant (SIFT) (44), MutationTaster (45), PROVEAN (46), Combined Annotation Dependent Depletion (CADD) (47), and Functional Analysis through Hidden Markov Models (FATHMM) (48). However, we did not find evidence of a functional effect for either rs2708928 or rs3730364.
IL1B RNA Quantification in Hippocampal Tissue
We did not find differences in the IL1B RNA transcript levels in the hippocampal tissue from patients with MTLE+HS, none of whom had an antecedent of FS, compared with control tissue using the two different endogenous control genes: SYP (Wilcoxon rank-sum test adjusted p = 0.0762; Figure 1A) and SDHA (Wilcoxon rank-sum test adjusted p = 0.1334; Figure 1B). For the transcript analysis, we used two different reference genes, SYP and SDHA, which have been validated specifically for this type of analysis in human hippocampal tissue (Figure 1) (33).
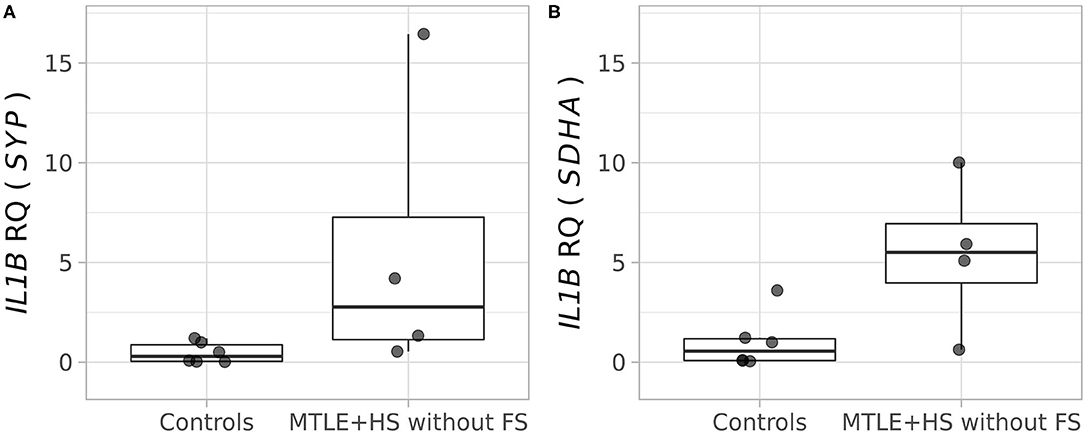
Figure 1. Relative quantification of the IL1B (interleukin 1 beta) transcript in the hippocampal tissue of four patients with pharmacoresistant mesial temporal lobe epilepsy, who were operated on to control refractory seizures, and autopsy controls (n = 6). (A) Analysis performed using the SYP (synaptophysin) gene as an endogenous control (Wilcoxon rank-sum test, adjusted p = 0.0762). (B) Analysis performed using the SDHA (succinate dehydrogenase, subunit A) gene as an endogenous control (Wilcoxon rank-sum test, adjusted p = 0.1334).
IL-1β Protein Quantification in Plasma
We obtained information regarding the time of the last seizure before the blood collection in 52 patients and depressive symptoms in 87 patients. The proportion of patients who had seizures <24 h and more than 24 h before the blood collection was similar in patients with and without FS (Fisher's exact test p = 1.000; Supplementary Table 2). Also, the proportion of patients with depressive symptoms was similar in patients with and without FS (Fisher's exact test p = 1.000; Supplementary Table 3).
As shown in Figure 2, the median IL-1β plasma concentration in controls was 0.997 pg/ml (25% quantile = 0.713 pg/ml, 75% quantile = 1.924 pg/ml). A similar concentration was observed in patients with MTLE+HS without FS (median = 0.728 pg/ml, 25% quantile = 0.214 pg/ml, 75% quantile = 1.973 pg/ml; Wilcoxon rank sum test p = 0.4539), including a small effect size (r = 0.129, 95% CI = 0.00–0.283). However, we observed a significant increase in the IL-1β plasma concentration in patients with MTLE+HS with an antecedent of FS (median = 2.063 pg/ml, 25% quantile = 1.466 pg/ml, 75% quantile = 3.420 pg/ml; Wilcoxon rank sum test p = 0.0097), including an increase in the effect size (r = 0.202, 95% CI = 0.052–0.347).
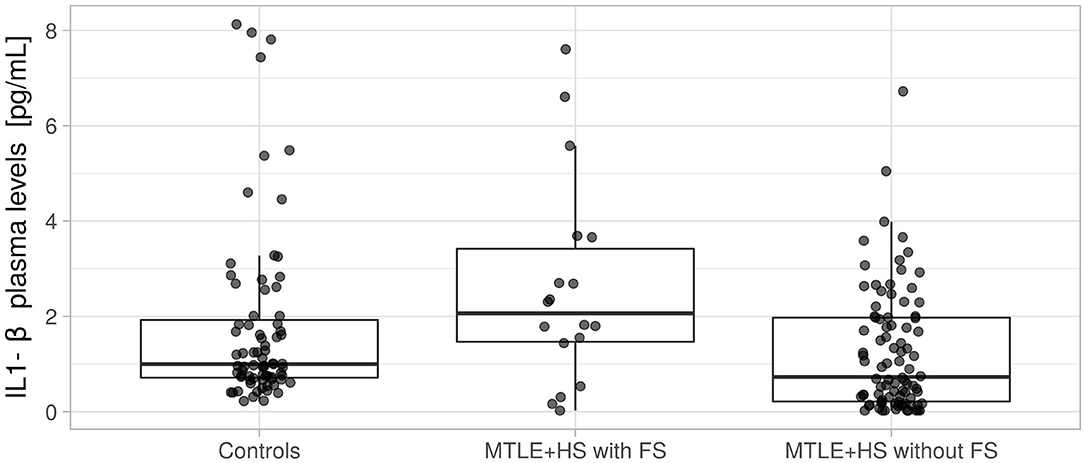
Figure 2. Quantification of interleukin 1 beta (IL-1β) protein in plasma from controls, patients with mesial temporal lobe epilepsy with an antecedent of febrile seizure (MTLE+HS with FS), and patients with mesial temporal lobe epilepsy with no antecedent of febrile seizure (MTLE+HS without FS). We used the Wilcoxon rank-sum test to compare patients with MTLE+HS with FS and controls (p = 0.1639) and compare patients with MTLE+HS without FS and controls (p = 0.0195).
Discussion
IL-1β is a powerful pro-convulsant and may have implications in epileptogenesis (8). In the last two decades, many studies have contributed to our knowledge about the role of the proinflammatory cytokine IL-1β in MTLE+HS and FS. However, there are still many controversies, and the subject is far from being settled (11–13, 15, 49, 50). Noteworthy, most previous studies investigating the role of IL-1β have not addressed the specific questions of whether IL-1β may be involved in the pathogenesis of MTLE=HS (10–16). Also, the work presented here can only indicate a link between IL-1β and MTLE+HS, and it was not explicitly designed to investigate causality.
We performed a candidate gene association study using SNPs encompassing the entire IL1B, including the still disputed associated functional variant−511C>T, present in the promoter region (20). This variant was first associated with MTLE+HS in a Japanese cohort (20) and Finnish children with FS (21). However, the association was not confirmed in other studies, for example, by Buono et al. (22) in a cohort with European ancestry, by Jin et al. (17) in Chinese individuals, and by Chou et al. (44) in Taiwanese children with FS. In addition, a meta-analysis revealed a modest association and effect size for the c.-511C>T polymorphism in patients with MTLE+HS (51). In the present study, we found no association between the c.-511C>T (rs16944) promoter variant in patients with MTLE+HS, with or without an antecedent of FS (Table 3). Of note, Kanemoto and coworkers reported that the rs16944*T allele might be a risk factor for prolonged febrile seizures (PFS) (52). Furthermore, several authors have hypothesized that c.-511C>T (rs16944) may increase IL1B transcription and the protein levels during PFS, elevating the risk of hippocampal injuries (14, 53, 54), which, in turn, could lead to the future development of MTLE+HS. Unlike FS, which are seizures that last <10–15 min in infants and young children and are believed not to be associated with an increased risk of epilepsy later in life, a history of PFS (lasting > 15 min) has been associated with subsequent MTLE+HS (55–57). However, determining the exact duration of an event that occurred decades earlier is either impossible or imprecise in retrospective studies, which explains, at least in part, the high variability in the proportion of PFS reported in the MTLE series (55–57). We could not unequivocally distinguish between FS and PFS in our cohort, which is a limitation of the present study. Thus, the absence of genetic association with the c.-511C>T (rs16944) variant found in the current study may reflect phenotypic differences in the cohorts.
Nevertheless, we found a genetic association signal between MTLE+HS and the SNPs rs2708928 and rs3730364 (Table 3). Interestingly, the SNP rs3730364*C, associated with an increased risk effect for MTLE+HS, appeared at a frequency of 0.673 in patients with MTLE+HS with FS and 0.414 in the control group. These frequency estimates are much higher than what was reported among 257 Brazilian exomes by the BIPMed project (rs3730364*C = 0.002; https://bipmed.org/) (58), as well as in the 1,000 Genomes (rs3730364*C = 0.008) and GnomAD (rs3730364*C = 0.007) databases (59, 60). We did not find the reported frequency for the SNP rs2708928 in public databases. SNPs rs3730364 and rs2708928 are located on the second and third introns of the IL1B gene, respectively, and according to prediction algorithms, there is no evidence that they have functional implications (61). Therefore, it is more plausible that both SNPs are in linkage disequilibrium with another functional variant, still unidentified, located within the IL1B gene.
In genetic association studies, addressing population stratification is a pivotal step to avoid spurious results due to genetic diversity between the compared groups (37, 38). Our AMOVA results showed a high variation component within groups, patients, and controls and a low variation component between the two groups (Table 2). Therefore, the two groups are most likely derived from the same population and do not present population stratification, allowing unbiased comparisons.
We also investigated the transcript levels of the IL1B gene in hippocampal tissue from four patients with pharmacoresistant MTLE+HS, who had no antecedent of FS, and we found no difference when comparing with tissue from autopsy controls (Figure 1). These results are somewhat different from previous studies investigating gene expression in resected hippocampal tissue from pharmacoresistant MTLE+HS and experimental models of temporal lobe epilepsy, which have shown an increase in IL1B mRNA levels (16, 62–67). However, all previous studies mentioned above have a critical limitation since they did not evaluate patients based on the antecedent of FS (16, 62–67). Thus, our results bring a novel and important finding regarding the relevance of considering the antecedent of FS when analyzing the pattern of transcript expression in the hippocampal tissue of patients with MTLE. Noteworthy, we also did not find differences in IL-1β levels in the plasma of patients with MTLE+HS without FS (Figure 2), which agrees with our transcript results. Thus, taking all the evidence presented here, we may suggest that the difference between our results and previous studies is because our IL1B mRNA quantification was carried out exclusively in patients with MTLE+HS without FS. However, we also recognize that we could not obtain hippocampal tissues from patients who presented antecedent of FS, and further studies comparing hippocampal tissue from patients with MTLE+HS with and without FS are essential to investigate this issue further.
It is also worth mentioning that our gene expression study in hippocampal tissue was carried out using two different reference genes, which have been validated specifically for this type of analysis in human hippocampal tissue (33). This technical aspect is also a key feature of the present study since it considerably increases the reliability of the tissue transcript analysis. Indeed, a previous study of protein expression in hippocampal tissue showed different results when using two different antibodies for the same protein, pointing to the lack of reliability of the western blot experiment in detecting the tissue expression of IL-1β and suggesting that RT-PCR is the method of choice to evaluate gene expression (68).
Finally, we quantified IL-1β protein in the plasma of patients with MTLE+HS and controls. We found similar IL-1β levels when comparing patients with no antecedent FS and controls, which agrees with our IL1B mRNA quantification findings in the hippocampal tissue. Also, we observed increased IL-1β plasma levels in patients with MTLE+HS with FS (Figure 2). The IL-1β plasma level has been reported to be increased in the postictal period in patients with MTLE and associated with depression in these patients (69). However, the proportion of patients with depressive symptoms and the intervals between the last seizure and blood collection were similar in patients with and without FS. Thus, our results might indicate a chronic inflammatory state in patients with MTLE+HS with an antecedent of FS, which may be less intense, or even not present in patients with MTLE+HS without antecedent FS (70). Furthermore, it has been demonstrated that IL-1β levels may be significantly different in the cerebrospinal fluid, blood, and central nervous system tissue of the same individual (18, 68), emphasizing the relevance of studying these different biological compartments. In addition, we recognize that upstream and downstream regulatory targets of IL-1β should also be investigated in further studies, including in the pediatric population (8, 9, 21, 23, 24).
In conclusion, we sought to assess the role of the IL-1β in a cohort of patients with an in-depth phenotypic characterization and using a multidimensional approach to access genetic association, transcript, and protein quantification of IL-1β. Not surprisingly, our results support a complex relationship for IL-1β in the context of MTLE+HS, as demonstrated by the positive genetic association with two SNPs in the IL1B gene in patients with MTLE+HS independently of the presence of FS. Also, there was an increase of plasmatic IL-1β levels only in MTLE+HS patients with antecedent FS, suggesting that they may have an immune system more prone to overexpress inflammation. Overall, our results support the hypothesis of a genetic association between MTLE+HS with the IL1B gene.
Data Availability Statement
The datasets presented in this study can be found in online repositories. The name of the repository and accession numbers can be found below: The European Molecular Biology Laboratory's European Bioinformatics Institute (EMBL-EBI) European Nucleotide Archive(ENA), https://www.ebi.ac.uk/ena/browser/home, acession numbers PRJEB44301 and PRJEB39251.
Ethics Statement
The studies involving human participants were reviewed and approved by the research ethics committee of the UNICAMP and USP-RP, and written informed consent was obtained from all participants.
Author Contributions
ROS and RS created the study design, conceptualized the work, and performed data acquisition and analysis. PB, MS-A, MA, CY, FR, TV, AS, AT, and FC participated in data acquisition and analysis. CM-M and IL-C conceptualized the work and served as principal investigators. All authors reviewed and approved the final version of the manuscript.
Funding
This work was supported by the Fundação de Amparo à Pesquisa do Estado de São Paulo, Brazil (FAPESP, grant number 2013/07559-3 and 2008/52590-8) and Coordenação de Aperfeiçoamento de Pessoal de Nível Superior, Brazil (CAPES, grant number 001). RS was supported by FAPESP (grant number 2019/08526-8). IL-C was supported by Conselho Nacional de Pesquisa, Brazil (CNPq, grant number 311923/2019-4).
Conflict of Interest
The authors declare that the research was conducted in the absence of any commercial or financial relationships that could be construed as a potential conflict of interest.
The Handling Editor KV declared a shared affiliation, though no other collaboration, with one or more authors TV and AS at the time of the review.
Publisher's Note
All claims expressed in this article are solely those of the authors and do not necessarily represent those of their affiliated organizations, or those of the publisher, the editors and the reviewers. Any product that may be evaluated in this article, or claim that may be made by its manufacturer, is not guaranteed or endorsed by the publisher.
Supplementary Material
The Supplementary Material for this article can be found online at: https://www.frontiersin.org/articles/10.3389/fneur.2021.690847/full#supplementary-material
References
1. Kwan P, Arzimanoglou A, Berg AT, Brodie MJ, Allen Hauser W, Mathern G, et al. Definition of drug resistant epilepsy: consensus proposal by the ad hoc Task Force of the ILAE Commission on Therapeutic Strategies. Epilepsia. (2009) 51:1069–77. doi: 10.1111/j.1528-1167.2009.02397.x
2. O'Dell CM, Das A, Wallace G, Ray SK, Banik NL. Understanding the basic mechanisms underlying seizures in mesial temporal lobe epilepsy and possible therapeutic targets: a review. J Neurosci Res. (2012) 90:913–24. doi: 10.1002/jnr.22829
3. Webster KM, Sun M, Crack P, O'Brien TJ, Shultz SR, Semple BD. Inflammation in epileptogenesis after traumatic brain injury. J Neuroinflammation. (2017) 14:10. doi: 10.1186/s12974-016-0786-1
4. Feng B, Chen Z. Generation of febrile seizures and subsequent epileptogenesis. Neurosci Bull. (2016) 32:481–92. doi: 10.1007/s12264-016-0054-5
5. Fukuda M, Ito M, Yano Y, Takahashi H, Motoie R, Yano A, et al. Postnatal interleukin-1β administration after experimental prolonged febrile seizures enhances epileptogenesis in adulthood. Metab Brain Dis. (2015) 30:813–9. doi: 10.1007/s11011-014-9648-7
6. Vezzani A, Aronica E, Mazarati A, Pittman QJ. Epilepsy and brain inflammation. Exp Neurol. (2013) 244:11–21. doi: 10.1016/j.expneurol.2011.09.033
7. Dubé CM, Ravizza T, Hamamura M, Zha Q, Keebaugh A, Fok K, et al. Epileptogenesis provoked by prolonged experimental febrile seizures: mechanisms and biomarkers. J Neurosci. (2010) 30:7484–94. doi: 10.1523/JNEUROSCI.0551-10.2010
8. Dubé C, Vezzani A, Behrens M, Bartfai T, Baram TZ. Interleukin-1β contributes to the generation of experimental febrile seizures. Ann Neurol. (2005) 57:152–5. doi: 10.1002/ana.20358
9. Heida JG, Moshé SL, Pittman QJ. The role of interleukin-1β in febrile seizures. Brain Dev. (2009) 31:388–93. doi: 10.1016/j.braindev.2008.11.013
10. Choi J, Koh S. Role of brain inflammation in epileptogenesis. Yonsei Med J. (2008) 49:1. doi: 10.3349/ymj.2008.49.1.1
11. Vezzani A. Epilepsy and inflammation in the brain: overview and pathophysiology. Epilepsy Curr. (2014) 14:3–7. doi: 10.5698/1535-7511-14.s2.3
12. Vezzani A, French J, Bartfai T, Baram TZ. The role of inflammation in epilepsy. Nat Rev Neurol. (2011) 7:31–40. doi: 10.1038/nrneurol.2010.178
13. Feng ZH, Hao J, Ye L, Dayao C, Yan N, Yan Y, et al. Overexpression of μ-calpain in the anterior temporal neocortex of patients with intractable epilepsy correlates with clinicopathological characteristics. Seizure. (2011) 20:395–401. doi: 10.1016/j.seizure.2011.01.010
14. Gallentine WB, Shinnar S, Hesdorffer DC, Epstein L, Nordli DR, Lewis DV, et al. Plasma cytokines associated with febrile status epilepticus in children: a potential biomarker for acute hippocampal injury. Epilepsia. (2017) 58:1102–11. doi: 10.1111/epi.13750
15. Maroso M, Balosso S, Ravizza T, Liu J, Bianchi ME, Vezzani A. Interleukin-1 type 1 receptor/Toll-like receptor signalling in epilepsy: the importance of IL-1beta and high-mobility group box 1. J Intern Med. (2011) 270:319–26. doi: 10.1111/j.1365-2796.2011.02431.x
16. Ravizza T, Gagliardi B, Noe F, Boer K, Aronica E, Vezzani A. Innate and adaptive immunity during epileptogenesis and spontaneous seizures: evidence from experimental models and human temporal lobe epilepsy. Neurobiol Dis. (2008) 29:142–60. doi: 10.1016/j.nbd.2007.08.012
17. Ravizza T, Balosso S, Vezzani A. Inflammation and prevention of epileptogenesis. Neurosci Lett. (2011) 497:223–30. doi: 10.1016/j.neulet.2011.02.040
18. Li G, Bauer S, Nowak M, Norwood B, Tackenberg B, Rosenow F, et al. Cytokines and epilepsy. Seizure. (2011) 20:249–56. doi: 10.1016/j.seizure.2010.12.005
19. Jin L, Jia Y, Zhang B, Xu Q, Fan Y, Wu L, et al. Association analysis of a polymorphism of interleukin 1β (IL-1β) gene with temporal lobe epilepsy in a Chinese population. Epilepsia. (2003) 44:1306–9. doi: 10.1046/j.1528-1157.2003.11003.x
20. Kanemoto K, Kawasaki J, Miyamoto T, Obayashi H, Nishimura M. Interleukin (IL)-1β, IL-1α, and IL-1 receptor antagonist gene polymorphisms in patients with temporal lobe epilepsy. Ann Neurol. (2000) 47:571–4. doi: 10.1002/1531-8249(200005)47:5 <571::AID-ANA3>3.0.CO;2-A
21. Virta M, Hurme M, Helminen M. Increased frequency of interleukin-1β (-511) allele 2 in febrile seizures. Pediatr Neurol. (2002) 26:192–5. doi: 10.1016/S0887-8994(01)00380-0
22. Buono RJ, Ferraro TN, O'Connor MJ, Sperling MR, Ryan SG, Scattergood T, et al. Lack of association between an interleukin 1 beta (IL-1β gene variation and refractory temporal lobe epilepsy. Epilepsia. (2001) 42:782–4. doi: 10.1046/j.1528-1157.2001.42900.x
23. Chou IC, Tsai CH, Hsieh YY, Peng CT, Tsai FJ. Association between polymorphism of interleukin-1 β-511 promoter and susceptibility to febrile convulsions in Taiwanese children. Acta Paediatr Int J Paediatr. (2003) 92:1356. doi: 10.1111/j.1651-2227.2003.tb00513.x
24. Yu X, Zhang N, Liu S, Xi Z, Zhang Y. Polymorphisms in the interleukin-1β (IL-1B) and interleukin-1α (IL-1A) genes on risk of febrile seizures: a meta-analysis. Neurol Sci. (2018) 39:1529–36. doi: 10.1007/s10072-018-3449-4
25. Scheffer IE, Berkovic S, Capovilla G, Connolly MB, French J, Guilhoto L, et al. ILAE classification of the epilepsies: position paper of the ILAE Commission for Classification and Terminology. Epilepsia. (2017) 58:512–21. doi: 10.1111/epi.13709
26. Coan AC, Kubota B, Bergo FPG, Campos BM, Cendes F. 3T MRI quantification of hippocampal volume and signal in mesial temporal lobe epilepsy improves detection of hippocampal sclerosis. Am J Neuroradiol. (2014) 35:77–83. doi: 10.3174/ajnr.A3640
27. Cendes F, Sakamoto AC, Spreafico R, Bingaman W, Becker AJ. Epilepsies associated with hippocampal sclerosis. Acta Neuropathol. (2014) 128:21–37. doi: 10.1007/s00401-014-1292-0
28. Sambrook J, Fritsch EF, Maniatis T. Molecular Cloning: A Laboratory Manual. New York, NY: Cold Spring Harbor Laboratory Press (1982).
29. The International HapMap Consortium. The International HapMap Project. Nature. (2003) 426:789–96. doi: 10.1038/nature02168
30. Silva-alves MS, Secolin R, Carvalho BS, Yasuda CL, Bilevicius E, Alvim MKM, et al. A prediction algorithm for drug response in patients with mesial temporal lobe epilepsy based on clinical and genetic information. PLoS One. (2017) 12:e0169214. doi: 10.1371/journal.pone.0169214
31. Baye TM, Tiwari HK, Allison DB, Go RC. Database mining for selection of SNP markers useful in admixture mapping. BioData Min. (2009) 2:1. doi: 10.1186/1756-0381-2-1
32. Baye TM. Inter-chromosomal variation in the pattern of human population genetic structure. Hum Genomics. (2011) 5:220–40. doi: 10.1186/1479-7364-5-4-220
33. Maurer-Morelli CV, de Vasconcellos JF, Reis-Pinto FC, Rocha CS, Domingues RR, Yasuda CL, et al. A comparison between different reference genes for expression studies in human hippocampal tissue. J Neurosci Methods. (2012) 208:44–7. doi: 10.1016/j.jneumeth.2012.04.020
34. Livak KJ, Schmittgen TD. Analysis of relative gene expression data using real-time quantitative PCR and the 2(-Delta Delta C(T)) Method. Methods. (2001) 25:402–8. doi: 10.1006/meth.2001.1262
35. Purcell S, Neale B, Todd-Brown K, Thomas L, Ferreira MAR, Bender D, et al. PLINK: A tool set for whole-genome association and population-based linkage analyses. Am J Hum Genet. (2007) 81:559–75. doi: 10.1086/519795
36. Barrett JC, Fry B, Maller J, Daly MJ. Haploview: analysis and visualization of LD and haplotype maps. Bioinformatics. (2005) 21:263–5. doi: 10.1093/bioinformatics/bth457
37. Price AL, Zaitlen NA, Reich D, Patterson N. New approaches to population stratification in genome-wide association studies. Nat Rev Genet. (2010) 11:459–63. doi: 10.1038/nrg2813
38. Marchini J, Cardon LR, Phillips MS, Donnelly P. The effects of human population structure on large genetic association studies. Nat Genet. (2004) 36:512–7. doi: 10.1038/ng1337
39. Excoffier L, Lischer HEL. Arlequin suite ver 3.5: a new series of programs to perform population genetics analyses under Linux and Windows. Mol Ecol Resour. (2010) 10:564–7. doi: 10.1111/j.1755-0998.2010.02847.x
40. Excoffier L, Smouse PE, Quattro JM. Analysis of molecular variance inferred from metric distances among DNA haplotypes: application to human mitochondrial DNA restriction data. Genetics. (1992) 131:479–91. doi: 10.1093/genetics/131.2.479
41. Faul F, Erdfelder E, Lang A-G, Buchner A. G*Power 3: a flexible statistical power analysis program for the social, behavioral, and biomedical sciences. Behav Res Methods. (2007) 39:175–91. doi: 10.3758/BF03193146
42. McLaren W, Gil L, Hunt SE, Riat HS, Ritchie GRS, Thormann A, et al. The ensembl variant effect predictor. Genome Biol. (2016) 17:122. doi: 10.1186/s13059-016-0974-4
43. Adzhubei I, Jordan DM, Sunyaev SR. Predicting functional effect of human missense mutations using PolyPhen-2. Curr Protoc Hum Genet. (2013) 76:7.20.1–41. doi: 10.1002/0471142905.hg0720s76
44. Sim NL, Kumar P, Hu J, Henikoff S, Schneider G, Ng PC. SIFT web server: predicting effects of amino acid substitutions on proteins. Nucleic Acids Res. (2012) 40:W452–W7. doi: 10.1093/nar/gks539
45. Schwarz JM, Rödelsperger C, Schuelke M, Seelow D. MutationTaster evaluates disease-causing potential of sequence alterations. Nat Methods. (2010) 7:575–6. doi: 10.1038/nmeth0810-575
46. Choi Y, Sims GE, Murphy S, Miller JR, Chan AP. Predicting the functional effect of amino acid substitutions and indels. PLoS One. (2012) 7:e46688. doi: 10.1371/journal.pone.0046688
47. Rentzsch P, Witten D, Cooper GM, Shendure J, Kircher M. CADD: predicting the deleteriousness of variants throughout the human genome. Nucleic Acids Res. (2019) 47:D886–D94. doi: 10.1093/nar/gky1016
48. Shihab HA, Rogers MF, Gough J, Mort M, Cooper DN, Day INM, et al. An integrative approach to predicting the functional effects of non-coding and coding sequence variation. Bioinformatics. (2015) 31:1536–43. doi: 10.1093/bioinformatics/btv009
50. Rana A, Musto AE. The role of inflammation in the development of epilepsy. J Neuroinflammation. (2018) 15:144. doi: 10.1186/s12974-018-1192-7
51. Kauffman MA, Moron DG, Consalvo D, Bello R, Kochen S. Association study between interleukin 1β gene and epileptic disorders: a HuGe review and meta-analysis. Genet Med. (2008) 10:83–88. doi: 10.1097/GIM.0b013e318161317c
52. Kanemoto K, Kawasaki J, Yuasa S, Kumaki T, Tomohiro O, Kaji R, et al. Increased frequency of interleukin-1β-511T allele in patients with temporal lobe epilepsy, hippocampal sclerosis, and prolonged febrile convulsion. Epilepsia. (2003) 44:796–9. doi: 10.1046/j.1528-1157.2003.43302.x
53. Shinnar S, Bello JA, Chan S, Hesdorffer DC, Lewis DV, MacFall J, et al. MRI abnormalities following febrile status epilepticus in children: the FEBSTAT study. Neurology. (2012) 79:871–7. doi: 10.1212/WNL.0b013e318266fcc5
54. Lewis D V, Shinnar S, Hesdorffer DC, Bagiella E, Bello JA, Chan S, et al. Hippocampal sclerosis after febrile status epilepticus: the FEBSTAT study. Ann Neurol. (2014) 74:178–85. doi: 10.1002/ana.24081
55. Shinnar S, Hesdorffer DC, Nordli DR, Pellock JM, O'Dell C, Lewis DV, et al. Phenomenology of prolonged febrile seizures: results of the FEBSTAT study. Neurology. (2008) 71:170–6. doi: 10.1212/01.wnl.0000310774.01185.97
56. Bassan H, Barzilay M, Shinnar S, Shorer Z, Matoth I, Gross-Tsur V. Prolonged febrile seizures, clinical characteristics, and acute management. Epilepsia. (2013) 54:1092–8. doi: 10.1111/epi.12164
57. Dubé CM, Brewster AL, Baram TZ. Febrile seizures: mechanisms and relationship to epilepsy. Brain Dev. (2009) 31:366–71. doi: 10.1016/j.braindev.2008.11.010
58. Rocha CS, Secolin R, Rodrigues MR, Carvalho BS, Lopes-Cendes I. The Brazilian Initiative on Precision Medicine (BIPMed): fostering genomic data-sharing of underrepresented populations. npj Genomic Med. (2020) 5:42. doi: 10.1038/s41525-020-00149-6
59. Genomes Project Consortium, Auton A, Brooks LD, Durbin RM, Garrison EP, Kang HM, et al. A global reference for human genetic variation. Nature. (2015) 526:68–74. doi: 10.1038/nature15393
60. Karczewski KJ, Francioli LC, Tiao G, Cummings BB, Alföldi J, Wang Q, et al. The mutational constraint spectrum quantified from variation in 141,456 humans. Nature. (2020) 581:434–43. doi: 10.1530/ey.17.14.3
61. Wong N, Wang X. miRDB: an online resource for microRNA target prediction and functional annotations. Nucleic Acids Res. (2015) 43:D146–D52. doi: 10.1093/nar/gku1104
62. Lachos J, Zattoni M, Wieser H-G, Fritschy J-M, Langmann T, Schmitz G, et al. Characterization of the gene expression profile of human hippocampus in mesial temporal lobe epilepsy with hippocampal sclerosis. Epilepsy Res Treat. (2011) 2011:758407. doi: 10.1155/2011/758407
63. Pernot F, Heinrich C, Barbier L, Peinnequin A, Carpentier P, Dhote F, et al. Inflammatory changes during epileptogenesis and spontaneous seizures in a mouse model of mesiotemporal lobe epilepsy. Epilepsia. (2011) 52:2315–25. doi: 10.1111/j.1528-1167.2011.03273.x
64. Rijkers K, Majoie HJ, Hoogland G, Kenis G, De Baets M, Vles JS. The role of interleukin-1 in seizures and epilepsy: a critical review. Exp Neurol. (2009) 216:258–71. doi: 10.1016/j.expneurol.2008.12.014
65. Van Gassen KLI, De Wit M, Koerkamp MJAG, Rensen MGA, Van Rijen PC, Holstege FCP, et al. Possible role of the innate immunity in temporal lobe epilepsy. Epilepsia. (2008) 49:1055–65. doi: 10.1111/j.1528-1167.2007.01470.x
66. Leal B, Chaves J, Carvalho C, Rangel R, Santos A, Bettencourt A, et al. Brain expression of inflammatory mediators in Mesial Temporal Lobe Epilepsy patients. J Neuroimmunol. (2017) 313:82–8. doi: 10.1016/j.jneuroim.2017.10.014
67. Mills JD, van Vliet EA, Chen BJ, Janitz M, Anink JJ, Baayen JC, et al. Coding and non-coding transcriptome of mesial temporal lobe epilepsy: critical role of small non-coding RNAs. Neurobiol Dis. (2020) 134:104612. doi: 10.1016/j.nbd.2019.104612
68. Gasmi N, Navarro FP, Ogier M, Belmeguenaï A, Lieutaud T, Georges B, et al. Low grade inflammation in the epileptic hippocampus contrasts with explosive inflammation occurring in the acute phase following status epilepticus in rats: translation to patients with epilepsy. bioRxiv [preprint]. (2021). doi: 10.1101/2021.03.25.436701
69. Vieira ELM, de Oliveira GNM, Lessa JMK, Goncalves AP, Sander JW, Cendes F, et al. Interleukin-1 beta plasma levels are associated with depression in temporal lobe epilepsy. Epilepsy Behav. (2015) 53:131–4. doi: 10.1016/j.yebeh.2015.09.035
Keywords: mesial temporal sclerosis, hippocampal atrophy, association study, gene expression, neuroinflammation
Citation: Santos RO, Secolin R, Barbalho PG, Silva-Alves MS, Alvim MKM, Yasuda CL, Rogerio F, Velasco TR, Sakamoto AC, Teixeira AL, Cendes F, Maurer-Morelli CV and Lopes-Cendes I (2021) Multidimensional Approach Assessing the Role of Interleukin 1 Beta in Mesial Temporal Lobe Epilepsy. Front. Neurol. 12:690847. doi: 10.3389/fneur.2021.690847
Received: 04 April 2021; Accepted: 28 June 2021;
Published: 05 August 2021.
Edited by:
Kette D. Valente, Hospital das Clínicas, Faculdade de Medicina, Universidade de São Paulo, BrazilReviewed by:
Liwen Wu, Central South University, ChinaFrancesca Felicia Operto, University of Salerno, Italy
Copyright © 2021 Santos, Secolin, Barbalho, Silva-Alves, Alvim, Yasuda, Rogerio, Velasco, Sakamoto, Teixeira, Cendes, Maurer-Morelli and Lopes-Cendes. This is an open-access article distributed under the terms of the Creative Commons Attribution License (CC BY). The use, distribution or reproduction in other forums is permitted, provided the original author(s) and the copyright owner(s) are credited and that the original publication in this journal is cited, in accordance with accepted academic practice. No use, distribution or reproduction is permitted which does not comply with these terms.
*Correspondence: Iscia Lopes-Cendes, aWNlbmRlc0B1bmljYW1wLmJy
†These authors have contributed equally to this work