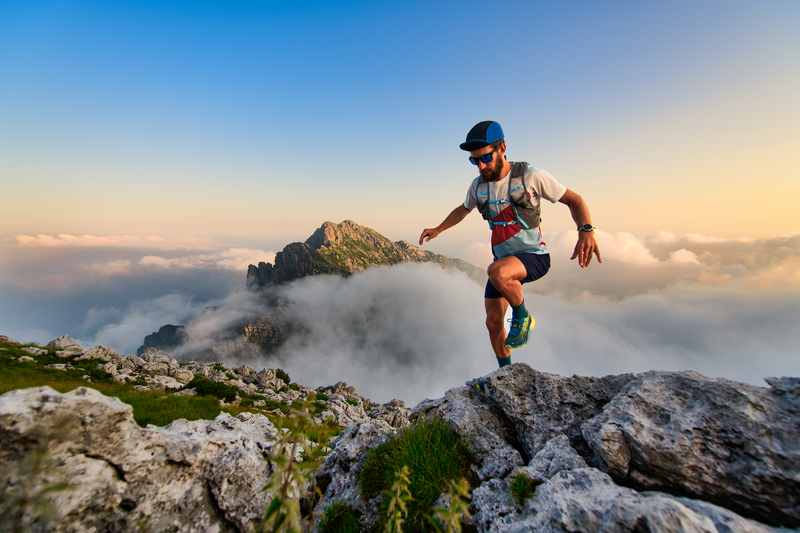
95% of researchers rate our articles as excellent or good
Learn more about the work of our research integrity team to safeguard the quality of each article we publish.
Find out more
BRIEF RESEARCH REPORT article
Front. Neurol. , 22 June 2021
Sec. Movement Disorders
Volume 12 - 2021 | https://doi.org/10.3389/fneur.2021.681413
Background: Vagal parasympathetic neurons are prone to degeneration in Parkinson's disease (PD). High-resolution ultrasound can precisely estimate the cross-sectional (CSA) area of peripheral nerves. Here, we tested the hypothesis that vagus CSA is reduced in PD.
Methods: We included 56 healthy controls (HCs) and 63 patients with PD. Using a high-end ultrasound system equipped with a high-frequency transducer, five images were obtained of each nerve. The hypoechoic neuronal tissue was delineated offline with dedicated software and the CSA extracted.
Results: In the initial PD vs. HC comparison, no statistically significant differences were observed in mean left vagus CSA (HC: 1.97 mm2, PD: 1.89 mm2, P = 0.36) nor in mean right vagus CSA (HC: 2.37 mm2, PD: 2.23 mm2, P = 0.17). The right vagus CSA was significantly larger than the left vagus CSA in both groups (P < 0.0001). Females were overrepresented in the HC group and presented with generally smaller vagus CSAs. Consequently, sex-adjusted CSA was significantly smaller for the right vagus nerve of the PD group (P = 0.041), but not for the left.
Conclusion: A small but significant reduction in sex-adjusted right vagus CSA was observed in patients with PD. The left vagus CSA was not significantly reduced in patients with PD. Ultrasound may not be a suitable method to detecting vagal axonal loss in individual patients.
The vagus nerve may play a crucial role in the pathogenesis of Parkinson's disease (PD). It has been hypothesized that α-synuclein aggregates form in the enteric nervous system, and spread via the autonomic nervous system to the central nervous system (1). In this context, vagal parasympathetic neurons that project to most of the gastrointestinal tract serve as entrance to the dorsal motor nucleus of the vagus (DMV). In support, truncal vagotomy decrease the risk of developing PD (2, 3). The DMV and the vagus nerve itself are among the most frequently affected structures in postmortem PD studies (4–7). This is accompanied by up to 50% DMV motor neuron loss in deceased patients (8–10).
Using 11C-donepezil PET/CT, we have previously demonstrated decreased gastrointestinal acetylcholinesterase density in patients with PD (11–13). This is believed to reflect parasympathetic denervation, mainly derived from the vagus nerve. Furthermore, subjects with isolated REM-sleep behavior disorder, the strongest marker of prodromal PD (14), also exhibit decreased gastrointestinal acetylcholinesterase density (15). Hence, the vagal parasympathetic system is affected years before diagnosis in some patients. Therefore, precise evaluation of vagal degeneration could serve as tool to diagnose PD patients, even during the premotor phase.
High-resolution ultrasound is a non-invasive, radiation-free alternative to assess vagal degeneration. The method can precisely measure the caliber of superficial nerves. Six previous studies tested the hypothesis that vagal neuron loss is detectable by ultrasound, but reported conflicting results. Four studies found significant atrophy of both left and right vagus nerves in patients with PD (16–19). In contrast, three studies reported no difference between patients with PD and healthy controls (HCs) (20–22). Thus, whether ultrasound can detect vagal neuronal loss in patients with PD remains unresolved.
In the present study we determined the vagus cross-sectional area (CSA) in the largest cohort of PD patients studied so far and compared to HCs. Five images of each nerve were obtained to optimize accuracy, and all analyses were performed offline for optimal precision. We compared the vagus CSA with measures of gastrointestinal acetylcholinesterase density (parasympathetic innervation) and retained radiopaque markers (estimate of gastrointestinal transit time) in subgroups. These measures should theoretically be altered in parallel to vagal parasympathetic depletion.
We included 63 early-to-moderate stage PD patients and 56 HCs. Participants were enrolled between April 2019 and November 2020 from ongoing studies and one published study (13) at Aarhus University Hospital, Denmark. Demographic and clinical information is listed in Table 1. All participants provided written informed consent. The study was approved by the Science Ethical Committees of the Central Denmark Region.
All images were obtained with a high-end ultrasound system (Philips EPIQ 7, Andover, MA, USA) equipped with a linear array 12.0-MHz transducer (L12-3, Phillips, Bothell, VA, USA). Participants were examined in the supine position by the same investigator (JH), who was not blinded to clinical status at ultrasound examination. The vagus nerve was identified midcervically at the level of the thyroid cartilage. On approximately the same location (within a few centimeters), five individual images were obtained bilaterally, that is, 10 images of each participant. All images were exported to offline analysis. In PMOD 4.0 (PMOD, Zürich, Switzerland), the intensity scale of each image was adjusted to the maximum intensity of the vagus nerve rim. This was performed to ensure optimal contrast between hypoechoic neuronal tissue and the hyperechoic epineurium. Next, the inner border of the hyperechoic epineural tissue was delineated, and CSA was extracted in mm2 with two decimals, that is, a precision of 0.01 mm2 (Figure 1). Of the five images of each nerve, the largest and smallest CSAs were discarded to prevent influence of potential outliers and the average of the three middle values was used for statistical analyses. All offline image analyses were performed blinded to clinical status.
Figure 1. Representative ultrasound image of the vagus nerve. (A) The vagus nerve (white arrow) of a patient with PD. (B) Delineation of the same vagus nerve following the hyperechoic epineurium. IJV, internal jugular vein; CCA, common carotid artery; CSA, cross-sectional area.
Radiopaque marker (ROM) data was available for 74 subjects (PD = 53, HC = 21) from two ongoing studies and one published study (13). In short, 10 ROMs were ingested every morning, 6 days prior to an abdominal CT scan. The number of retained ROMs estimates the colonic transit time. Colonic acetylcholinesterase density (measure of parasympathetic innervation) assessed by 11C-donepezil PET/CT was available in 41 patients with PD (13). These objective measures of gastrointestinal function and innervation was used to explore possible correlations with vagus CSA in subgroup analyses. Gastrointestinal symptoms were evaluated with ROME constipation questionnaire in 54 patients with PD and 21 HCs. Furthermore, 47 patients with PD and 14 HCs were evaluated with Non-Motor Symptoms Scale (NMSS) (here only the gastrointestinal section is presented).
Statistical analyses were performed with Stata 13.1 and GraphPad Prism 7.0. Normality in distribution was assessed by Q-Q plots and histograms. Group differences of sex were investigated using Fisher's exact tests. Group differences of age and BMI were tested with student's t-test. Differences between right and left side were assessed with paired t-tests. Differences in symmetry index and gastrointestinal symptom questionnaires were assessed with Mann-Whitney-U test. Pearson correlation coefficients were calculated to explore correlations between vagus CSA and other variables [age, body mass index (BMI), time since diagnosis, disease severity defined by Hoehn and Yahr stage (H&Y), ROM, and colon acetylcholinesterase density measured by 11C-donepezil PET]. The unmatched composition of our two cohorts regarding sex was addressed with a multiple linear regression model to calculate sex-adjusted differences in CSA. This model was checked by diagnostic plots of residuals. P < 0.05 were considered significant. Method precision was ascertained with coefficient of variation (SD/mean) for each nerve using the three middle CSA values.
Mean vagus nerve CSAs are presented in Table 1, and depicted graphically in Figure 2. There was no significant difference in the crude comparison between patients with PD and HCs. We observed a tendency toward higher CSAs in males than females, most pronounced in the right vagus (Table 1). Thus, we used multiple linear regression to determine sex-adjusted CSA of the vagus. First, we applied a model allowing different slopes in each group. We found no significant slope interactions (P > 0.5). Thus, we applied a model with one common slope. Sex-adjusted difference in mean left vagus CSA was 0.12 mm2 (95% CI: −0.06 to 0.29 mm2, P = 0.19) and right vagus was 0.21 mm2 (95% CI: 0.01–0.41 mm2, P = 0.041). In this model, sex was a significant predictor of right vagus CSA (P = 0.003), but not of the left vagus CSA (P = 0.06). No difference in symmetry index was observed (P = 0.69).
Figure 2. Left and right vagus nerve cross-sectional area (CSA) is presented for patients with Parkinson's disease (PD, black circles) and healthy controls (HC, white circles). Mean and standard deviation is depicted with black error bars.
In the HC group, mean right vagus CSA was 0.4 mm2 larger than mean left vagus CSA (95% CI: 0.24–0.57 mm2, P < 0.0001). In the PD group, mean right vagus CSA was 0.34 mm2 larger than left vagus CSA (95% CI: 0.20–0.48 mm2, P < 0.0001). Left and right vagus CSA did not correlate with age, BMI, time since diagnosis, disease severity (H&Y), ROM (estimate of colonic transit time), nor colon acetylcholinesterase density measured by 11C-donepezil PET (P > 0.05).
Mean coefficient of variation of vagus CSAs was 6.7%.
In the present study, we found no difference in vagus nerve CSA between patients with PD and HCs, but female vagus CSA was smaller than male vagus CSA in both groups. One previous report found the opposite pattern; vagus CSA in females were larger than males (23). More females were included in our HC group compared to the PD group so we determined the sex-adjusted differences between patients with PD and HCs. We found a statistically significant reduction of the right vagus CSA (P = 0.041) but not of the left vagus CSA (P = 0.19).
Although the mean right vagus CSA was significantly reduced in PD, the effects size of ~10% is small. The effect size in other studies, which reported statistically significant CSA reduction, range from ~10–30% (16–18). Neuronal loss in DMV has been reported to be up to 50% in postmortem studies (8–10). Other nuclei related to the vagus nerve (nucleus ambiguus, solitary nucleus, and spinal trigeminal nucleus) are less affected by α-synuclein aggregates (24). Also, no motor neurons are lost in nucleus ambiguus (9, 25). The axonal diameter of somato-motor and somato-sensory myelinated fibers is generally much larger than that of autonomic neurons, and must necessarily occupy a substantial fraction of the midcervical vagus CSA. Cardiac sympathetic neurons also reside in the vagus nerve and occupy up to 5% of the CSA (26, 27). Cardiac sympathetic neurons are particularly vulnerable in PD (28) and may also contribute to slight vagal atrophy. However, if vagal atrophy is mainly caused by sympathetic and parasympathetic (DMV) axonal loss, the reduction in CSA is probably very small and could explain why three studies failed to show vagal atrophy in PD (20–22). Additionally, the vagus nerve shows frequent branching (up to 29%) (29). Branched nerves have smaller CSA at the midcervical level (29). Although we have no reasons to believe that branching should differ between patients with PD and HC, it may have contributed to increased data variance.
Time since the diagnosis could be another factor explaining some incongruence between studies. In the present study, the median duration was short (8 months), as many of the patients were newly diagnosed. The four previous studies that demonstrated significant CSA reduction included patients 5–10 years post-diagnosis (16–19) (Table 2). Thus, the rather small difference demonstrated in the present study might be partly caused by the shorter duration of motor symptoms. However, we found no correlation between vagus CSA and time since diagnosis in the present data, and the three studies that failed to show a significant difference also investigated moderately affected patients, 4–8 years postdiagnosis (20–22) (Table 2).
If a biological difference in right vagus CSA truly exists between patients with PD and HCs, two aspects may have enabled us to identify this small difference in the present study: (1) our two- to three-times larger PD cohort than previous studies and (2) offline measurements as advised for optimal precision (30). However, our study does not support that this method can be applied for meaningful interpretation at the individual level, because the average between-group difference of 0.21 mm2 is hardly discerned, given that the normal vagal CSA ranges from 1 mm2 to nearly 4 mm2 (Figure 2).
Right vagus CSA was significantly larger than left vagus CSA as previously reported (16–18, 20, 22, 30). This also corresponds to the side-to-side difference of the cervical vagus neuronal area defined on postmortem (31). Further, the right midcervical vagus contains ~20% more nerve fibers than the left (32). We believe this side difference may be the main reason for the larger CSA reduction of the right vagus, that is, the potential absolute reduction must increase with larger nerve CSA. The mean CSA values were in quite close agreement with six previous studies that estimated vagus CSA by tracing the epineural rim, as in the present study (16, 18, 20, 22, 23, 30). Thus, in studies using the tracing method, a reasonable interstudy reproducibility of the vagus CSA seems to exist.
Vagus CSA did not correlate with the number of retained ROM, nor with colon acetylcholinesterase density measured by 11C-donepezil PET. If vagus CSA atrophy occurs in parallel to parasympathetic denervation, we would expect these parameters to be correlated. One explanation could be that ROM and colon acetylcholinesterase density reflect global colonic function/innervation, and vagal parasympathetic projections end close to the left colonic flexure. However, α-synuclein aggregates are also found in spinal parasympathetic neurons that innervate the descending and sigmoid colon and the rectum (33). Therefore, it is likely that these parasympathetic neurons degenerate in parallel to those in the vagus, and the correlation between vagus CSA and these global colonic measurements should still be apparent. Thus, we argue that the most likely cause for the lack of correlation is the inability of the ultrasound method to detect vagal parasympathetic loss.
The precision of the three middle values was evaluated for each nerve by calculating the coefficient of variation. The average coefficient of variation was acceptable (6.7%). We also calculated the average distance from the three middle values to the mean. The average distance was 4.9% of the mean, for example, values defining a CSA of 2 mm2 on average were 0.1 mm2 from the “exact” value. Therefore, we argue that obtaining five ultrasound images of each nerve and using the three middle values to determine the CSA yields precise results.
This study has some limitations. First, the ultrasound investigator was not blinded to clinical status during examination. However, the offline CSA estimation was performed blinded. Second, our two groups were matched on age but not sex, forcing us to do linear adjustment in the comparison analyses.
In conclusion, we found a small but significant reduction in sex-adjusted right vagus CSA in patients with PD. No difference was found in left vagus CSA. Sex was a significant predictor for the right but not left vagus caliber. This study does not support the use of vagus ultrasound as a diagnostic marker for PD, but there may be applications for the method in a research setting. Other methods to assess vagal atrophy, including MR neurography, should be explored in the future.
The raw data supporting the conclusions of this article will be made available by the authors, without undue reservation.
The studies involving human participants were reviewed and approved by Science Ethical Committee of Central Denmark Region. The patients/participants provided their written informed consent to participate in this study.
JH: acquisition, analysis, interpretation of the data, and drafted the manuscript. TF: analysis and interpretation of the data and critical revision the manuscript. KA, CS, KK, NO, PvW-M, SD, and BB: acquisition of the data and critical revision the manuscript. UW: design and concept of the study, analysis and interpretation of data, and critical revision the manuscript. PB: design and concept of the study, interpretation of data, and critical revision the manuscript. All authors have approved the final manuscript and agree to be accountable for the content of the work and made substantial contribution to the following parts of the study.
This study was supported by the Lundbeck Foundation [Grant No: R190-2014-4183].
The authors declare that the research was conducted in the absence of any commercial or financial relationships that could be construed as a potential conflict of interest.
1. Hawkes CH, Del Tredici K, Braak H. Parkinson's disease: a dual-hit hypothesis. Neuropathol Appl Neurobiol. (2007) 33:599–614. doi: 10.1111/j.1365-2990.2007.00874.x
2. Svensson E, Horvath-Puho E, Thomsen RW, Djurhuus JC, Pedersen L, Borghammer P, et al. Vagotomy and subsequent risk of Parkinson's disease. Ann Neurol. (2015) 78:522–9. doi: 10.1002/ana.24448
3. Liu B, Fang F, Pedersen NL, Tillander A, Ludvigsson JF, Ekbom A, et al. Vagotomy and Parkinson disease: a Swedish register-based matched-cohort study. Neurology. (2017) 88:1996–2002. doi: 10.1212/WNL.0000000000003961
4. Braak H, Del Tredici K, Rub U, de Vos RA, Jansen Steur EN, Braak E. Staging of brain pathology related to sporadic Parkinson's disease. Neurobiol Aging. (2003) 24:197–211. doi: 10.1016/S0197-4580(02)00065-9
5. Beach TG, Adler CH, Sue LI, Vedders L, Lue L, White CL, et al. Multi-organ distribution of phosphorylated alpha-synuclein histopathology in subjects with Lewy body disorders. Acta Neuropathol. (2010) 119:689–702. doi: 10.1007/s00401-010-0664-3
6. Gelpi E, Navarro-Otano J, Tolosa E, Gaig C, Compta Y, Rey MJ, et al. Multiple organ involvement by alpha-synuclein pathology in Lewy body disorders. Mov Disord. (2014) 29:1010–8. doi: 10.1002/mds.25776
7. Kalaitzakis ME, Graeber MB, Gentleman SM, Pearce RK. The dorsal motor nucleus of the vagus is not an obligatory trigger site of Parkinson's disease: a critical analysis of alpha-synuclein staging. Neuropathol Appl Neurobiol. (2008) 34:284–95. doi: 10.1111/j.1365-2990.2007.00923.x
8. Gai WP, Blumbergs PC, Geffen LB, Blessing WW. Age-related loss of dorsal vagal neurons in Parkinson's disease. Neurology. (1992) 42:2106–11. doi: 10.1212/WNL.42.11.2106
9. Eadie MJ. The Pathology of certain medullary nuclei in Parkinsonism. Brain. (1963) 86:781–92. doi: 10.1093/brain/86.4.781
10. Greene JG. Causes and consequences of degeneration of the dorsal motor nucleus of the vagus nerve in Parkinson's disease. Antioxid Redox Signal. (2014) 21:649–67. doi: 10.1089/ars.2014.5859
11. Gjerloff T, Fedorova T, Knudsen K, Munk OL, Nahimi A, Jacobsen S, et al. Imaging acetylcholinesterase density in peripheral organs in Parkinson's disease with 11C-donepezil PET. Brain. (2015) 138(Pt 3):653–63. doi: 10.1093/brain/awu369
12. Fedorova TD, Seidelin LB, Knudsen K, Schacht AC, Geday J, Pavese N, et al. Decreased intestinal acetylcholinesterase in early Parkinson disease: An (11)C-donepezil PET study. Neurology. (2017) 88:775–81. doi: 10.1212/WNL.0000000000003633
13. Horsager J, Andersen KB, Knudsen K, Skjaerbaek C, Fedorova TD, Okkels N, et al. Brain-first versus body-first Parkinson's disease: a multimodal imaging case-control study. Brain. (2020) 143:3077–88. doi: 10.1093/brain/awaa238
14. Heinzel S, Berg D, Gasser T, Chen H, Yao C, Postuma RB, et al. Update of the MDS research criteria for prodromal Parkinson's disease. Mov Disord. (2019) 34:1464–70. doi: 10.1002/mds.27802
15. Knudsen K, Fedorova TD, Hansen AK, Sommerauer M, Otto M, Svendsen KB, et al. In-vivo staging of pathology in REM sleep behaviour disorder: a multimodality imaging case-control study. Lancet Neurol. (2018) 17:618–28. doi: 10.1016/S1474-4422(18)30162-5
16. Tsukita K, Taguchi T, Sakamaki-Tsukita H, Tanaka K, Suenaga T. The vagus nerve becomes smaller in patients with Parkinson's disease: a preliminary cross-sectional study using ultrasonography. Parkinsonism Relat Disord. (2018) 55:148–9. doi: 10.1016/j.parkreldis.2018.06.002
17. Walter U, Tsiberidou P, Kersten M, Storch A, Lohle M. Atrophy of the vagus nerve in Parkinson's Disease revealed by high-resolution ultrasonography. Front Neurol. (2018) 9:805. doi: 10.3389/fneur.2018.00805
18. Pelz JO, Belau E, Fricke C, Classen J, Weise D. Axonal degeneration of the vagus nerve in Parkinson's disease-a high-resolution ultrasound study. Front Neurol. (2018) 9:951. doi: 10.3389/fneur.2018.00951
19. Sartucci F, Bocci T, Santin M, Bongioanni P, Orlandi G. High-resolution ultrasound changes of the vagus nerve in idiopathic Parkinson's disease (IPD): a possible additional index of disease. Neurol Sci. (2021). doi: 10.1007/s10072-021-05183-5. [Epub ahead of print].
20. Fedtke N, Witte OW, Prell T. Ultrasonography of the vagus nerve in Parkinson's disease. Front Neurol. (2018) 9:525. doi: 10.3389/fneur.2018.00525
21. Laucius O, Balnyte R, Petrikonis K, Matijosaitis V, Juceviciute N, Vanagas T, et al. Ultrasonography of the vagus nerve in the diagnosis of Parkinson's disease. Parkinsons Dis. (2020) 2020:2627471. doi: 10.1155/2020/2627471
22. Sijben LCJ, Mess WH, Walter U, Janssen AML, Kuijf M, Oosterloo M, et al. The cross-sectional area of the vagus nerve is not reduced in Parkinson's Disease patients. medRxiv [Preprint]. (2020). doi: 10.1101/2020.10.19.20214973
23. Walter U, Tsiberidou P. Differential age-, gender-, and side-dependency of vagus, spinal accessory, and phrenic nerve calibers detected with precise ultrasonography measures. Muscle Nerve. (2019) 59:486–91. doi: 10.1002/mus.26412
24. Del Tredici K, Rub U, De Vos RA, Bohl JR, Braak H. Where does parkinson disease pathology begin in the brain? J Neuropathol Exp Neurol. (2002) 61:413–26. doi: 10.1093/jnen/61.5.413
25. Benarroch EE, Schmeichel AM, Sandroni P, Low PA, Parisi JE. Involvement of vagal autonomic nuclei in multiple system atrophy and Lewy body disease. Neurology. (2006) 66:378–83. doi: 10.1212/01.wnl.0000196638.98781.bb
26. Seki A, Green HR, Lee TD, Hong L, Tan J, Vinters HV, et al. Sympathetic nerve fibers in human cervical and thoracic vagus nerves. Heart Rhythm. (2014) 11:1411–7. doi: 10.1016/j.hrthm.2014.04.032
27. Seki A, Chen PS, Fishbein MC. Reply to the editor-does the cervical vagus contain sympathetic fibers that act on the heart? Heart Rhythm. (2014) 11:e79–80. doi: 10.1016/j.hrthm.2014.07.009
28. Lamotte G, Holmes C, Sullivan P, Lenka A, Goldstein DS. Cardioselective peripheral noradrenergic deficiency in Lewy body synucleinopathies. Ann Clin Transl Neurol. (2020) 7:2450–60. doi: 10.1002/acn3.51243
29. Hammer N, Glatzner J, Feja C, Kuhne C, Meixensberger J, Planitzer U, et al. Human vagus nerve branching in the cervical region. PLoS ONE. (2015) 10:e0118006. doi: 10.1371/journal.pone.0118006
30. Pelz JO, Belau E, Henn P, Hammer N, Classen J, Weise D. Sonographic evaluation of the vagus nerves: protocol, reference values, and side-to-side differences. Muscle Nerve. (2018) 57:766–71. doi: 10.1002/mus.25993
31. Verlinden TJ, Rijkers K, Hoogland G, Herrler A. Morphology of the human cervical vagus nerve: implications for vagus nerve stimulation treatment. Acta Neurol Scand. (2016) 133:173–82. doi: 10.1111/ane.12462
32. Hoffman HH, Schnitzlein HN. The numbers of nerve fibers in the vagus nerve of man. Anat Rec. (1961) 139:429–35. doi: 10.1002/ar.1091390312
Keywords: parkinson's disease, vagus nerve, ultrasound, parasympathetic, cross-sectional area
Citation: Horsager J, Walter U, Fedorova TD, Andersen KB, Skjærbæk C, Knudsen K, Okkels N, von Weitzel-Mudersbach P, Dyrskog SE, Bergholt B and Borghammer P (2021) Vagus Nerve Cross-Sectional Area in Patients With Parkinson's Disease—An Ultrasound Case-Control Study. Front. Neurol. 12:681413. doi: 10.3389/fneur.2021.681413
Received: 16 March 2021; Accepted: 03 May 2021;
Published: 22 June 2021.
Edited by:
Alain Kaelin-Lang, University of Italian Switzerland, SwitzerlandReviewed by:
Nico Diederich, Centre Hospitalier de Luxembourg, LuxembourgCopyright © 2021 Horsager, Walter, Fedorova, Andersen, Skjærbæk, Knudsen, Okkels, von Weitzel-Mudersbach, Dyrskog, Bergholt and Borghammer. This is an open-access article distributed under the terms of the Creative Commons Attribution License (CC BY). The use, distribution or reproduction in other forums is permitted, provided the original author(s) and the copyright owner(s) are credited and that the original publication in this journal is cited, in accordance with accepted academic practice. No use, distribution or reproduction is permitted which does not comply with these terms.
*Correspondence: Jacob Horsager, amFjb2JubHNAcm0uZGs=
Disclaimer: All claims expressed in this article are solely those of the authors and do not necessarily represent those of their affiliated organizations, or those of the publisher, the editors and the reviewers. Any product that may be evaluated in this article or claim that may be made by its manufacturer is not guaranteed or endorsed by the publisher.
Research integrity at Frontiers
Learn more about the work of our research integrity team to safeguard the quality of each article we publish.