- 1Neurovascular Center, Neurological Surgery, P.C., Lake Success, NY, United States
- 2Hybernia Medical, LLC, New Rochelle, NY, United States
- 3Department of Neurology & Stroke, Hertie-Institute for Clinical Brain Research, Eberhard-Karls University of Tübingen, Tübingen, Germany
- 4Stroke Center, Department of Neurosurgery, Rush University Medical Center, Chicago, IL, United States
- 5Interventional Neurology/Neuroradiology, Boston University School of Medicine, Boston, MA, United States
- 6Comprehensive Stroke Center and Department of Neurology, University of California, Los Angeles (UCLA), Los Angeles, CA, United States
- 7Neurovascular Surgery, Department of Neurosurgery, Yale University-New Haven Hospital, New Haven, CT, United States
In acute ischemic stroke, early recanalization of the occluded artery is crucial for best outcome to be achieved. Recanalization aims at restoring blood flow to the ischemic tissue (reperfusion) and is achieved with pharmacological thrombolytic drugs, endovascular thrombectomy (EVT) devices, or both. The introduction of modern endovascular devices has led to tremendous anatomical and clinical success with rates of substantial reperfusion exceeding 80% and proven clinical benefit in patients with anterior circulation large vessel occlusions (LVOs). However, not every successful reperfusion procedure leads to the desired clinical outcome. In fact, the rate of non-disabled outcome at 3 months with current EVT treatment is ~1 out of 4. A constraint upon better outcomes is that reperfusion, though resolving ischemic stress, may not restore the anatomic structures and metabolic functions of ischemic tissue to their baseline states. In fact, ischemia triggers a complex cascade of destructive mechanisms that can sometimes be exacerbated rather than alleviated by reperfusion therapy. Such reperfusion injury may cause infarct progression, intracranial hemorrhage, and unfavorable outcome. Therapeutic hypothermia has been shown to have a favorable impact on the molecular elaboration of ischemic injury, but systemic hypothermia is limited by slow speed of attaining target temperatures and clinical complications. A novel approach is endovascular delivery of hypothermia to cool the affected brain tissue selectively and rapidly with tight local temperature control, features not available with systemic hypothermia devices. In this perspective article, we discuss the possible benefits of adjunctive selective endovascular brain hypothermia during interventional stroke treatment.
Introduction
Several randomized controlled clinical trials have demonstrated the clinical benefit of endovascular thrombectomy (EVT) in selected patients with acute ischemic stroke due to large vessel occlusions (LVO-AIS) (1–7). The introduction of endovascular clot extraction has not only led to tremendous improvements in reperfusion rates (often exceeding 80%) and clinical outcome (compared to intravenous rtPA alone), but also offered opportunities to further refine patient selection criteria and extend the treatment time window (8–10). Although a significant improvement in clinical outcome after LVO-AIS could be achieved, a wide gap remains between the success in restoring perfusion through the occluded artery (>4 out of 5) and the rate of excellent, non-disabled (modified Rankin Scale score 0-1) functional outcome achieved in only ~1 out of 4 treated patients (11). Recently, Van Horn and colleagues evaluated 123 consecutive patients at a single German center from 2015 to 2019 who had complete TICI (Thrombolysis in Cerebral Infarction score) 3 reperfusion and still found 54.5% to have poor clinical outcomes at 90 days (12).
Brain ischemia triggers a cascade of molecular and cellular mechanisms many of which have been identified (13). Following the quick depletion of oxygen and energy carriers from brain tissue it comes to progressive failure of cellular ion pumps, NMDA (N-Methyl- d-aspartate) receptor activation, and anoxic depolarization that further lead to disturbance of ion homeostasis, excitotoxicity, acidification, and increasing cellular influx of Ca2+ (14–17). Activation of nitric oxide synthase and cyclooxygenase-2, generation of free radicals, upregulation of cell adhesion molecules, and increase in the production of proinflammatory cytokines follow (18–22). The resulting inflammatory reactions include recruitment of cell-mediated immunity, activation of protein kinases and matrix zinc-metalloproteinases, and neutrophil transmigration, among others (22–28). In addition, apoptosis is promoted by up-regulation of the BAX (Bcl-2 Associated X-protein) and calpain genes (29, 30).
As a result of these molecular pathways, functional and structural changes follow, such as impaired vasomotor regulation (31, 32), cytotoxic and vasogenic edema (33, 34), and breakdown of the blood-brain-barrier (35–37). With sustained activation of these pathways the risk for extensive neuronal cell death, infarct progression, and intracranial hemorrhage increases (38, 39).
Paradoxically, reperfusion of the ischemic brain tissue can exacerbate these destructive processes that have been triggered by stroke. This is called reperfusion injury and is thought to be the result of multiple pathways of tissue insult, oxidative stress, leukocyte infiltration, complement activation, mitochondrial dysfunction, platelet activation and aggregation, and blood-brain-barrier disruption, culminating in neuron death, brain edema or hemorrhagic transformation (13, 40–42). Reperfusion injury is a common biologic phenomenon across multiple organs and not limited to reperfusion procedures of the neurovasculature, also occurring following treatment of ischemic conditions of the limbs, gastrointestinal tract, and the heart (43–45). The most feared consequence of cerebral reperfusion injury is intracerebral hemorrhage (ICH) (46, 47).
EVT devices are well-suited to remove the target thrombus and anatomically clear the artery to restore blood flow, but do not offer direct therapy of metabolic consequences of ischema. For ameliorating metabolic disruptions, therapeutic hypothermia has been one of the most promising concepts based on its pleiotropic mechanisms of action (48, 49). In this perspective article we present the possible benefits of a novel form of therapeutic hypothermia: endovascular selective brain cooling, and how its adjunct application during endovascular stroke treatment could improve the outcome in LVO-AIS patients by reducing the deleterious impact of ischemia and reperfusion injury.
Subsections
The Physiological Limits of Endovascular Clot Extraction
Although there are numerous endovascular devices available to remove a clot from the neurovasculature, in principle, they are of two main types (1–7). One has a tip with a stent-like mesh that lodges into the clot and allows its retrieval and the other, is an aspiration catheter that applies a suction force to the clot while it is removed from the vasculature. The success rate to clear the artery from a clot with endovascular devices is high (Thrombolysis in Cerebral Infarction Scale score 2b and 3) and ranges between 59 to 88% (1–7).
The clinical benefit of the combined treatment approach, i.e., systemic pharmacological therapy and endovascular clot extraction, over pharmacological therapy alone, is due to its high effectiveness to anatomically revascularize the occluded artery. In addition, endovascular catheters may be used to locally infuse fluids and various drugs. However, drugs that are considered neuroprotective and for intra-arterial use are rather limited (50), and currently, there are no intra-arterial agents with the FDA-approved indication to be used for the treatment of acute ischemic stroke.
The rate for symptomatic ICH, often seen with parenchymal hemorrhage, following the combined treatment of acute ischemic stroke varies and may be as high as 10% (1–10). The rate for asymptomatic ICH, often associated with hemorrhagic infarction-type hemorrhagic transformation, is generally higher and may involve as many as 1 out of 3 treated patients (36–39). Clinical factors, such as thrombolytic therapy, thromboembolism, and specific imaging markers, comorbid factors, and clinical work-flow performance markers are often considered risk factors for post-treatment ICH (13, 36–39, 51). As such, these factors represent parts of the puzzle that complete the picture to understanding how stroke evolves toward a critical level of impairment of cerebral autoregulation, edema, blood-brain-barrier disruption, and post-treatment reperfusion injury. Recanalization therapy, albeit necessary and often successful to restore the ischemic brain to baseline condition, does not directly modify these pathophysiologic mechanisms of stroke and reperfusion injury. Furthermore, almost all drugs with mechanisms thought to counter a specific part of the stroke injury cascade have failed to provide a conclusive neuroprotective effect in clinical studies (50, 52). Certainly, a treatment concept that can attenuate or prevent these pathophysiologic processes is desirable and would be an ideal candidate for adjunctive application.
The Physiological Limits of Systemic Hypothermia
Therapeutic hypothermia has been identified as one of the most promising neuroprotective methods. Systemic cooling experiments in stroke models involving animals across various species have shown that hypothermia is neuroprotective in terms of reduction in infarct size and improvement in neuro-behavioral testing scores (average effect size 44% [95% confidence interval, 40−47%]) without increasing the risk for ICH (48). The clinical translation of systemic hypothermia (mild to moderate hypothermia, reduction of body core temperature to ~33°C −35°C) has been successful in comatose patients with out-of-hospital cardiac arrest in both shockable and non-shockable rhythms (53, 54) as well as in neonates with ischemic encephalopathy (55). In addition, cardiac surgery has been routinely performed with extracorporeal blood cooling or total exchange with cold fluids (profound hypothermia, reduction of body core temperature to ≤25°C) to protect the brain from ischemic injury during circulatory arrest or vascular clamping (56).
The neuroprotective effect of cooling is the result of hypothermia's pleiotropic mechanisms of action. Among a plethora of demonstrated effects that help to produce a physiological state of ischemic tolerance, cooling causes metabolic depression reducing the cellular demand for oxygen and energy, has anti-excitotoxic, anti-inflammatory, anti-edematous, and anti-apoptotic properties, and suppresses the breakdown of the blood-brain-barrier (49, 57, 58). Perhaps the most known endogenous feature of hypothermia is found in hibernating mammals that are able to survive prolonged periods of hypometabolism and reduced tissue perfusion under extreme hypothermic conditions (body temperature decreases even below the freezing point of water) (59).
What appears to be simply natural and is repeated year after year in hibernating mammals, in humans the process of inducing and maintaining systemic hypothermia are extremely difficult, complicated, prolonged, and painful due to the strong physiological counter mechanisms and frequent adverse events (60, 61) (Figures 1A–C). As such, this clinical process requires specialized intensive care resources, sedation, and muscle relaxation, machine ventilation, and co-treatment of the frequent adverse effects of body hypothermia. Despite the successes achieved in the clinical settings of out-of-hospital cardiac arrest and neonatal asphyxia, systemic hypothermia has been difficult to realize in acute stroke patients (62).
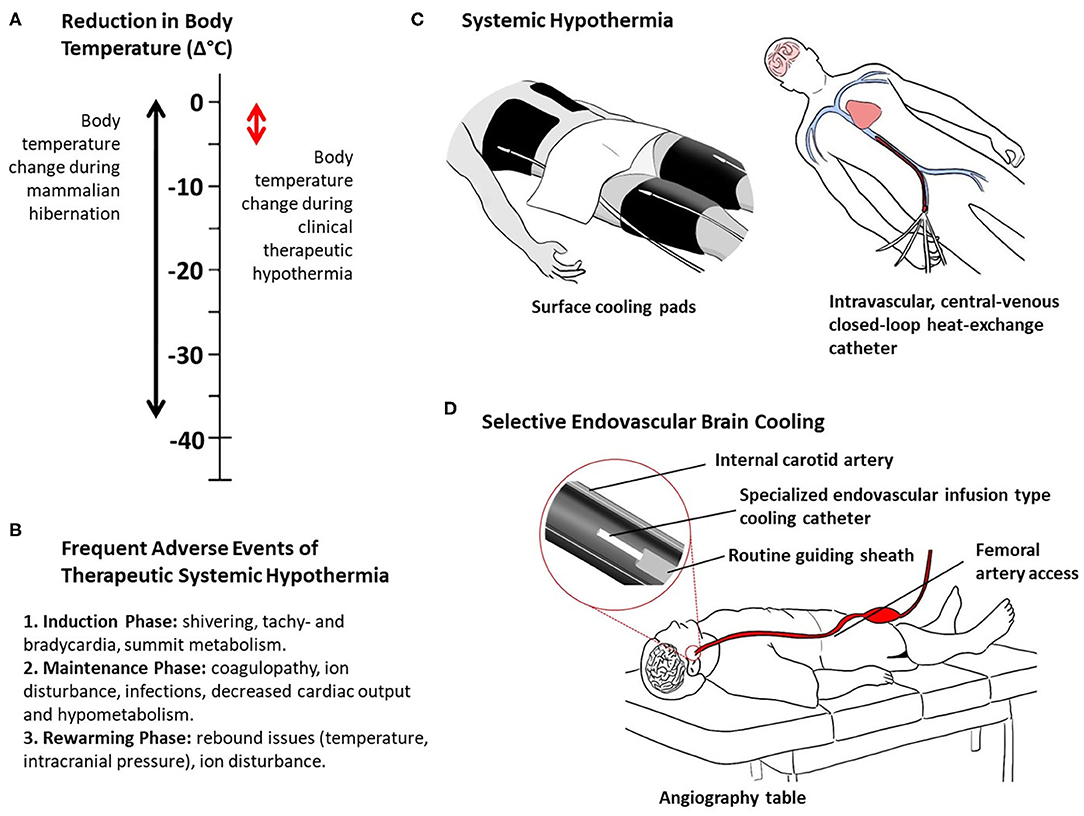
Figure 1. Thermal characteristics of mammalian hibernation and clinical hypothermia (A), frequent adverse events of systemic therapeutic hypothermia (B), and methods of clinical therapeutic hypothermia (C, D). Systemic hypothermia, examples of surface and intravascular conductive cooling (C). Selective endovascular brain cooling, example of direct intra-arterial cold infusion (D).
The Promises of Adjunct Endovascular Brain Hypothermia
Selective brain hypothermia is an attractive alternative to systemic hypothermia, providing focused cooling of the injured organ and avoiding the complications of systemic hypothermia, including intubation, shivering, pneumonia, altered coagulability, and cold-induced stress reactions (58). A variety of devices and strategies have been developed to induce selective brain hypothermia and tested in preclinical models and early human clinical trials, including intranasal selective hypothermia, transvenous endovascular cooling, extraluminal vascular cooling, and epidural cerebral cooling (63–72). However, advance of these devices in clinical development has been constrained by slow onset of cooling induced by external cerebral cooling techniques and procedural time delay needed to place internal nasopharyngeal cooling devices. A promising emerging approach that overcomes these limitations is selective endovascular brain cooling (SEBC; Supplement Table) through an interventional catheter system that is navigated to the common or internal carotid artery to perform an intravascular heat-exchange with the arterial blood before it enters the cerebral vasculature (Figure 1D). Different endovascular local heat-exchange concepts exist exploiting the physical forces of conduction or direct mixing of cold fluids with blood, and intravascular vs. extracorporeal heat-exchange methods (63, 72).
Experiments in animal stroke models, commonly with transient occlusion of the middle cerebral artery, have found a significant improvement in outcome (stroke volume, edema, behavioral scores) with brief endovascular selective brain cooling [average effect size 51% (95% CI, 38–64%)] (73, 74). Hereby, the risk for ICH was not increased in the cooling groups compared to control groups. The preferred method is direct intra-arterial infusion of cold fluids to cool the blood that enters the brain either during ischemia, post reperfusion, or during both phases. Although brain cooling was performed for only a short duration, the rapid induction of brain hypothermia led to the suppression of the pathophysiological mechanisms of ischemia and reperfusion injury to levels that allowed a significantly better restoration of the affected brain tissue when compared to the normothermia group.
Speed of brain cooling is one of the unique features of SEBC that distinguishes itself from any form of systemic hypothermia. This is because only a selected vascular territory of the brain is cooled, rather than the whole body, and a direct modification of the arterial input temperature is performed (63). The cooling performance with SEBC is in the range of minutes with the direct infusion method, not hours (Figure 1D). For instance, a brain temperature reduction of more than 3.5°C in 10 min was shown in a large pig model with SEBC using the direct intra-arterial cold infusion (IACI) method (75). In a canine transient occlusion model, a reduction in brain temperature of >10°C within 10 min was found using SEBC, with direct IACI during the phases of ischemia and post-reperfusion (76). The animals with brain cooling had smaller infarct sizes compared to controls. Extracorporeal blood cooling is a more invasive cooling concept primarily performed in the clinical setting of cardiac surgery. Blood from the femoral artery, aorta, or carotid artery is passed through an extracorporeal heat-exchanger where it is cooled to the desired temperature. This cooled and medically processed blood is infused back into the cerebral circulation, often via the carotid artery. In another swine stroke model experiment, extracorporeal blood cooling led to a brain temperature decrease of >6°C in ~30 min (77). In contrast to SEBC with IACI (cold fluid or cold blood), local blood cooling via conduction, i.e., with a closed-loop intra-carotid catheter in which cold fluids circulate, is far less effective due to the limited dimensions of the parent artery and resulting limitations to the size and surface area of the heat-exchange catheter (78).
SEBC has also been studied in humans (Table 1). The feasibility and safety of SEBC with a brief intra-carotid infusion of cold fluid was demonstrated in a first-in-human study in 18 elective cerebrovascular patients undergoing follow-up cerebral angiograms (79). Blood temperature of the ipsilateral jugular venous bulb was monitored as a surrogate for local brain temperature. Clinical testing during the procedure in awake patients, and after the procedure in all patients, found that this selective cooling process was painless and produced no serious adverse effects. Transcranial Doppler monitoring and serial blood sample analyses showed stable parameters. In three following clinical studies, this direct infusion method of SEBC was applied, during ischemia and post-reperfusion, in acute ischemic stroke LVO patients (80–82). However, brain temperature or surrogates were not measured. Due to the limited number of patients and study design, the efficacy of SEBC in LVO patients remains to be determined. Nevertheless, SEBC with brief cold fluid infusion was found to be safe in LVO patients undergoing endovascular therapy and was not associated with serious adverse events. A phase II randomized controlled trial is currently being conducted to study brief SEBC with IACI in a larger group of LVO acute ischemic stroke patients undergoing clot extraction (83). In 2018, a single-arm explorative study investigating the safety of brief SEBC-IACI-induced brain cooling in anterior circulation acute ischemic stroke patients undergoing clot extraction (and refractory to tissue plasminogen activator therapy) was completed (84). The results are pending publication.
Discussion
The advantages of endovascular selective brain cooling for the treatment of LVO stroke are mani-fold. One, with significantly reduced time to target temperature of the organ of interest, the brain, hypothermic neuroprotection can be achieved quickly. Hereby, the physical concept of SEBC is ideally suited to achieve brain cooling quickly (10–30 times faster than traditional systemic hypothermia) and with minimal invasiveness because it directly modifies the cerebral arterial input temperature and would be administered via the routine endovascular route. Two, selective brain cooling allows to reduce the impact of cooling on the body, thus minimizing or avoiding systemic hypothermia and its adverse consequences. Three, due to the endovascular route, SEBC is ideally suited to be applied as an adjunct treatment to endovascular recanalization procedures in LVO stroke. Four, due to its selective and immediate cooling features and endovascular route, SEBC would be capable to attenuate the destructive forces of reperfusion injury, locally and directly, following endovascular clot-extraction.
In practice, and taking the direct infusion method as an example, the SEBC catheter would be placed in the ipsilateral (ischemic hemisphere) internal carotid artery through the same intra-arterial access as used for clot extraction (Figure 1D). The catheter diameter would be small enough to fit through the regular guiding sheath. Selective brain cooling would be performed immediately following thrombectomy via exchange of catheters. Parallel use of thrombectomy and SEBC catheters is a possibility. In order to achieve brain hypothermia quickly, one could even attempt to cool the ischemic brain before thrombectomy is performed (intra-ischemic), as done in the explorative clinical studies (Table 1). However, this would undoubtedly delay reperfusion therapy and pose additional risks for distal embolization and cerebral vascular injury due to manipulations of the cooling catheter as it is pushed past the clot.
The key to reducing the impact of ischemia and reperfusion injury is the suppression of their pathophysiological mechanisms. While it is clear that SEBC-induced hypothermic neuroprotection would be delayed in LVO patients when they finally undergo endovascular clot-extraction, regardless of how quickly brain cooling can be achieved, the results from animal stroke models and our understanding of the molecular and cellular evolution of stroke suggest that brain cooling is neuroprotective, even when delayed for several hours. This is a plausible assumption. If the early stage excitotoxicity cannot be prevented, brain hypothermia may still suppress the later-stage inflammatory processes, reduce edema, and prevent vessels from becoming too “leaky.” Disturbance of the structural integrity and leaky vessels are considered the basic preconditions for developing post stroke ICH.
The beneficial impact of SEBC on the potential harms of sudden reperfusion is more evident when considering SEBC is applied as an adjunct to endovascular clot-extraction. Furthermore, SEBC induced via the direct mixing method with IACI could offer additional benefit as the incoming blood would be diluted, therefore reducing the impact of inflammatory promoters and immune cells on the ischemic and reperfused brain tissue. Another important advantage of the infusion method is that the dimensions of the infusion-type cooling catheter would be small enough to fit through the guiding sheath that has already been placed to navigate the EVT catheter to remove the clot, requiring only an exchange of catheters or even allowing concomitant use of both catheter systems (Figure 1D).
There are limitations of SEBC. First, although hypothermia induction would be rapid, the duration of cooling would be limited by the routine times allowed for indwelling endovascular catheters to remain in the arterial system. Second, the direct mixing method with IACI, albeit the fastest cooling method in physical terms, would be limited by the volume that could be infused within a certain period. In contrast, the extracorporeal blood cooling concept would theoretically address the issue of hypervolemia as practically the same amount of blood is re-introduced into the cerebral circulation as it was removed from the system before exposing the blood to the external heat-exchanger. With this isovolemic cooling concept, long-term brain hypothermia would become feasible. However, this isovolemic method adds layers of invasiveness and complexity to the hyperacute workflow, such as a second arterial puncture (blood outflow), vascular reconstruction (e.g., temporary arterial occlusion), necessity of a perfusion specialist, and heparinization of blood in the external circulation. Third, potential adverse effects may occur from local exposure to cold, additional fluid volume (local hemodilution) and mechanical stress from the endovascular cooling catheter. Thus, careful monitoring of vitals, dilution, local temperature, and potential vascular injury and spasm would be necessary. Lasty, there are yet no devices on the market for SEBC. Only routine catheters have been used to explore and investigate the safety and feasibility of brief SEBC (10–15 min) in elective and acute cerebrovascular patients. While the results have been promising and larger trials are underway, it is questionable whether brief and uncontrolled IACI will deliver the answers to the ideal depth, duration, and timing of brain hypothermia the determination of which should be based on physiological parameters and cerebral metabolic demand, such as changes in brain metabolism, cerebral blood flow, and infarct evolution. This could become more critical when infusing fluids distally to a cerebral artery occlusion (intra-ischemic brain cooling) without any information about the metabolic and hemodynamic condition of the ischemic tissue bed. As such, we believe that to provide a safe and most efficient SEBC, specialized catheter systems are necessary that offer excellent heat-exchange, mixing, and embedded safety and control mechanisms.
Given the growing and widespread utilization of endovascular clot-extraction in LVO stroke and lack of additional means to counter the deleterious mechanisms of ischemia and reperfusion injury (12, 85), SEBC is an appealing concept to reap the benefits of therapeutic hypothermia while minimizing the adverse effects of systemic hypothermia. Based on our current understanding of the mechanisms of stroke, reperfusion, and therapeutic (brain) hypothermia, it is reasonable to consider an improvement in outcome and reduction in the occurrence of post-stroke ICH may occur. Currently, technologies that would enable safe and controlled SEBC in acute ischemic stroke patients are in pre-clinical and explorative clinical development (Supplement Table). However, it is foreseeable that specialized medical devices for SEBC will be available in the near future. Ultimately, clinical investigations will show whether SEBC will be a safe, practical, and effective tool in the armamentarium of stroke treatment.
Data Availability Statement
The original contributions presented in the study are included in the article/Supplementary Material, further inquiries can be directed to the corresponding author/s.
Author Contributions
JC and JP-S contributed conception of the article. JC wrote the first draft of the article. All authors have approved the final version of the manuscript, contributed critical review and revision of the manuscript.
Conflict of Interest
JC and JS are co-founders of Hybernia Medical, LLC. SP and JS are consultants to Hybernia Medical, LLC.
The remaining authors declare that the research was conducted in the absence of any commercial or financial relationships that could be construed as a potential conflict of interest.
Supplementary Material
The Supplementary Material for this article can be found online at: https://www.frontiersin.org/articles/10.3389/fneur.2020.594289/full#supplementary-material
References
1. Berkhemer OA, Fransen PS, Beumer D, van den Berg LA, Lingsma HF, Yoo AJ, et al. A randomized trial of intraarterial treatment for acute ischemic stroke. N Engl J Med. (2015) 372:11–20. doi: 10.1056/NEJMoa1411587
2. Jovin TG, Chamorro A, Cobo E, de Miquel MA, Molina CA, Rovira A, et al. Thrombectomy within 8 hours after symptom onset in ischemic stroke. N Engl J Med. (2015) 372:2296–306. doi: 10.1056/NEJMoa1503780
3. Saver JL, Goyal M, Bonafe A, Diener HC, Levy EI, Pereira VM, et al. Stent-retriever thrombectomy after intravenous t-PA vs. t-PA alone in stroke. N Engl J Med. (2015) 372:2285–95. doi: 10.1056/NEJMoa1415061
4. Goyal M, Demchuk AM, Menon BK, Eesa M, Rempel JL, Thornton J, et al. Randomized assessment of rapid endovascular treatment of ischemic stroke. N Engl J Med. (2015) 372:1019–30. doi: 10.1056/NEJMoa1414905
5. Campbell BC, Mitchell PJ, Kleinig TJ, Dewey HM, Churilov L, Yassi N, et al. Endovascular therapy for ischemic stroke with perfusion-imaging selection. N Engl J Med. (2015) 372:1009–18. doi: 10.1056/NEJMoa1414792
6. Mocco J, Zaidat OO, von Kummer R, Yoo AJ, Gupta R, Lopes D, et al. Aspiration thrombectomy after intravenous alteplase vs. intravenous alteplase alone. Stroke. (2016) 47:2331–8. doi: 10.1161/STROKEAHA.116.013372
7. Martins SO, Mont'Alverne F, Rebello LC, Abud DG, Silva GS, Lima FO, et al. Thrombectomy for stroke in the public health care system of Brazil. N Engl J Med. (2020) 382:2316–26. doi: 10.1056/NEJMoa2000120
8. Saver JL, Goyal M, van der Lugt A, Menon BK, Majoie CBLM, Dippel DW, et al. Time to treatment with endovascular thrombectomy and outcomes from ischemic stroke: a meta-analysis. JAMA. (2016) 316:1279–88. doi: 10.1001/jama.2016.13647
9. Albers GW, Marks MP, Kemp S, Christensen S, Tsai JP, Ortega-Gutierrez S, et al. Thrombectomy for stroke at 6 to 16 hours with selection by perfusion imaging. N Engl J Med. (2018) 378:708–18. doi: 10.1056/NEJMoa1713973
10. Nogueira RG, Jadhav AP, Haussen DC, Bonafe A, Budzik RF, Bhuva P, et al. Thrombectomy 6 to 24 hours after stroke with a mismatch between deficit and infarct. N Engl J Med. (2018) 378:11–21. doi: 10.1056/NEJMoa1706442
11. Badhiwala JH, Nassiri F, Alhazzani W, Selim MH, Farrokhyar F, Spears, et al. Endovascular thrombectomy for acute ischemic stroke: a meta-analysis. JAMA. (2015) 314:1832–43. doi: 10.1001/jama.2015.13767
12. van Horn N, Kniep H, Leischner H, McDonough R, Deb-Chatterji M, Broocks G, et al. Predictors of poor clinical outcome despite complete reperfusion in acute ischemic stroke patients. J NeuroInterv Surg. (2020). doi: 10.1136/neurintsurg-2020-015889. [Epub ahead of print].
13. Choi JH, Pile-Spellman J. Reperfusion changes after stroke and practical approaches for neuroprotection. Neuroimag Clin N Am. (2018) 28:663–82. doi: 10.1016/j.nic.2018.06.008
14. Lipton P, Raley PKM, Lobner D. Long-term inhibition of synaptic transmission and macromolecular synthesis following anoxia in the rat hippocampal slice: interaction between Ca and NMDA receptors. In: G. Somjen, editor. Mechanisms of Cerebral Hypoxia and Stroke. Boston, MA: Springer (1998). p. 229–49.
15. Lauritzen M, Hansen AJ. The effect of glutamate receptor blockade on anoxic depolarization and cortical spreading depression. J Cerebral Blood Flow Metab. (1992) 12:223–9. doi: 10.1038/jcbfm.1992.32
16. Grigg JJ, Anderson EG. Competitive and non-competitive N-methyl-D-aspartate antagonists modify hypoxia-induced membrane potential changes and protect rat hippocampal slices from functional failure: a quantitative comparison. J Pharmacol Exp Ther. (1990) 253:130–5.
17. Benveniste H, Drejer J, Schousboe A, Diemer N. Elevation of the extracellular concentrations of glutamate and aspartate in rat hippocampus during transient cerebral ischemia monitored by intracerebral microdialysis. J Neurochem. (1984) 43:1369–74. doi: 10.1111/j.1471-4159.1984.tb05396.x
18. Iadecola C, Xu X, Zhang F, El-Fakahany EE, Ross ME. Marked induction of calcium-independent nitric oxide synthase activity after focal ischemia. J Cerebral Blood Flow Metabol. (1995) 15:52–9. doi: 10.1038/jcbfm.1995.6
19. Nogawa S, Zhang F, Ross ME, Iadecola C. Cyclooxygenase-2 gene expression in neurons contributes to ischemic brain damage. J Neurosci. (1997) 17:2746–55. doi: 10.1523/JNEUROSCI.17-08-02746.1997
20. Degraba TJ. The role of inflammation after acute stroke. utility of pursuing anti-adhesion molecule therapy. Neurology. (1998) 51(Suppl. 3):S62–8. doi: 10.1212/WNL.51.3_Suppl_3.S62
21. Chamorro A, Hallenbeck J. The harms and benefits of inflammatory and immune responses in vascular disease. Stroke. (2006) 37:291–3. doi: 10.1161/01.STR.0000200561.69611.f8
22. Zheng Z, Yenari MA. Post-ischemic inflammation: molecular mechanisms and therapeutic implications. Neurol Res. (2004) 26:884–92. doi: 10.1179/016164104X2357
23. Nawashiro H, Tasaki K, Ruetzler A, Hallenbeck J. TNF-alpha pretreatment induces protective effects against focal cerebral ischemia in mice. J Cerebral Blood Flow Metab. (1997) 17:483–90. doi: 10.1097/00004647-199705000-00001
24. Allan SM, Rothwell NJ. Cytokines and acute neurodegeneration. Nat Rev Neurosci. (2001) 2:734–44. doi: 10.1038/35094583
25. Baeuerle PA, Henkel T. Function and activation of NF-kappa B in the immune system. Annu Rev Immunol. (1994) 12:141–79. doi: 10.1146/annurev.iy.12.040194.001041
26. Schneider A, Martin-Villalba A, Weih F, Vogel J, Wirth T, Schwaninger M. NF-kappaB is activated and promotes cell death in focal cerebral ischemia. Nat Med. (1999) 5:554–9. doi: 10.1038/8432
27. Irving EA, Bamford M. Role of mitogen- and stress-activated kinases in ischemic injury. J Cerebral Blood Flow Metab. (2002) 22:631–47. doi: 10.1097/00004647-200206000-00001
28. Rosenberg GA. Matrix metalloproteinases in neuroinflammation. Glia. (2002) 39:279–91. doi: 10.1002/glia.10108
29. Chen J, Zhu RL, Nakayama M. Expression of the apoptosis-effector gene, BAX, is upregulated in vulnerable hippocampal CA1 neurons following global ischemia. J Neurochem. (1996) 67:64–71. doi: 10.1046/j.1471-4159.1996.67010064.x
30. Squier MKT, Miller ACK, Malkinson AM, Cohen JJ. Calpain activation in apoptosis. J Cell Physiol. (1994) 159:229–37. doi: 10.1002/jcp.1041590206
31. Marchal G, Furlan M, Beaudouin V, Rioux P, Hauttement JL, Serrati C, et al. Early spontaneous hyperperfusion after stroke. a marker of favourable tissue outcome? Brain. (1996) 119:409–19. doi: 10.1093/brain/119.2.409
32. Macfarlane R, Moskowitz MA, Sakas DE, Tasdemiroglu E, Wei EP, Kontos HA. The role of neuroeffector mechanisms in cerebral hyperperfusion syndromes. J Neurosurg. (1991) 75:845–55. doi: 10.3171/jns.1991.75.6.0845
33. Schaefer PW, Gonzalez RG, Hunter G, Wang B, Koroshetz WJ, Schwamm LH. Diagnostic value of apparent diffusion coefficient hyperintensity in selected patients with acute neurologic deficits. J Neuroimaging. (2001) 11:369–80. doi: 10.1111/j.1552-6569.2001.tb00065.x
34. Ay H, Buonanno FS, Schaefer PW, Le DA, Wang B, Gonzalez RG, et al. Posterior leukoencephalopathy without severe hypertension: utility of diffusion-weighted MRI. Neurology. (1998) 51:1369–76. doi: 10.1212/WNL.51.5.1369
35. Wang X, Tsuji K, Lee SR, Ning M, Furie KL, Buchan AM, et al. Mechanisms of hemorrhagic transformation after tissue plasminogen activator reperfusion therapy for ischemic stroke. Stroke. (2004) 35:2726–30. doi: 10.1161/01.STR.0000143219.16695.af
36. Warach S, Latour LL. Evidence of reperfusion injury, exacerbated by thrombolytic therapy, in human focal brain ischemia using a novel imaging marker of early blood–brain barrier disruption. Stroke. (2004) 35(Suppl. I):2659–61. doi: 10.1161/01.STR.0000144051.32131.09
37. Leigh R, Christensen S, Campbell BCV, Marks MP, Albers GW, Lansberg MG. Pretreatment blood–brain barrier disruption and post-endovascular intracranial hemorrhage. Neurology. (2016) 87:263–9. doi: 10.1212/WNL.0000000000002862
38. Fiehler J, Remmele C, Kucinski T, Rosenkranz M, Thomalla G, Weiller C, et al. Reperfusion after severe local perfusion deficit precedes hemorrhagic transformation: An MRI study in acute stroke patient. Cerebrovasc Dis. (2005) 19:117–24. doi: 10.1159/000083180
39. Renu A, Laredo C, Tudela R, Urra X, Lopes-Rueda A, Llull L, et al. Brain hemorrhage after endovascular reperfusion therapy of ischemic stroke: a threshold-finding whole-brain perfusion CT study. J Cerebral Blood Flow Metabol. (2017) 37:153–65. doi: 10.1177/0271678X15621704
40. Pan J, Konstas AA, Bateman B, Ortolano G, Pile-Spellman J. Reperfusion injury following cerebral ischemia: pathophysiology, MR imaging, and potential therapies. Neuroradiology. (2007) 49:93–102. doi: 10.1007/s00234-006-0183-z
41. Karapanayioitides T, Meuli R, Devuyst G, Piechowski-Jozwiak B, Dewarrat A, Ruchat P, et al. Postcarotid endarterectomy hyperperfusion or reperfusion syndrome. Stroke. (2005) 36:21–6. doi: 10.1161/01.STR.0000149946.86087.e5
42. Lin L, Wang X, Yu Z. Ishemia-reperfusion injury in the brain: Mechanisms and potential therapeutic strategies. Biochem Pharmacol. (2016) 5:213. doi: 10.4172/2167-0501.1000213
43. Jennings RB, Sommers HM, Smyth GA, Flack HA, Linn H. Myocardial necrosis induced by temporary occlusion of a coronary artery in the dog. Arch Pathol. (1960) 70:68–78.
44. Beyersdorf F, Matheis G, Kruger S, Hanselmann A, Freisleben HG, Zimmer G, et al. Avoiding reperfusion injury after limb revascularization: experimental observations and recommendations for clinical application. J Vase Surg. (1989) 9:757–66. doi: 10.1016/0741-5214(89)90081-5
45. Gonzalez LM, Moeser AJ, Blikslager AT. Animal models of ischemia-reperfusion-induced intestinal injury: progress and promise for translational research. Am J Physiol Gastrointest Liver Physiol. (2015) 308:63–75. doi: 10.1152/ajpgi.00112.2013
46. Khatri P, Wechsler LR, Broderick JP. Intracranial hemorrhage associated with revascularization therapies. Stroke. (2007) 38:431–40. doi: 10.1161/01.STR.0000254524.23708.c9
47. Khatri R, McKinney AM, Swenson B, Janardhan V. Blood-brain barrier, reperfusion injury, and hemorrhagic transformation in acute ischemic stroke. Neurology. (2012) 79(13 Suppl. 1):S52–7. doi: 10.1212/WNL.0b013e3182697e70
48. van der Worp HB, Sena ES, Donnan GA, Howells DW, Macleod MR. Hypothermia in animal models of acute ischaemic stroke: a systematic review and meta-analysis. Brain. (2007) 130:3063–74. doi: 10.1093/brain/awm083
49. Polderman KH. Induced hypothermia and fever control for prevention and treatment of neurological injuries. Lancet. (2008) 371:1955–69. doi: 10.1016/S0140-6736(08)60837-5
50. Hill MD, Goyal MG, Menon BK, Nogueira RG, McTaggart RA, Demchuk AM, et al. Efficacy and safety or nerinetide for the treatment of acute ischaemic stroke (ESCAPE-NA1): a multicentre, double-blind, randomised controlled trial. Lancet. (2020) 395:878–87. doi: 10.1016/S0140-6736(20)30258-0
51. Nogueira RG, Gupta R, Jovin TG, Levy EI, Liebeskind DS, Zaidat OO, et al. Predictors and clinical relevance of hemorrhagic transformation after endovascular therapy for anterior circulation large vessel occlusion strokes: a multicenter retrospective analysis of 1122 patients. J Neurointerv Surg. (2015) 7:16–21. doi: 10.1136/neurintsurg-2013-010743
52. O'Collins VE, Macleod MR, Donnan GA, Horky LL, van der Worp BH, Howells DW. 1,026 experimental treatments in acute stroke. Ann Neurol. (2006) 59:467–77. doi: 10.1002/ana.20741
53. Bernard SA, Gray TW, Buist MD, Jones BM, Silvester W, Gutteridge G, et al. Treatment of comatose survivors of out-of-hospital cardiac arrest with induced hypothermia. N Engl J Med. (2002) 346:557–63. doi: 10.1056/NEJMoa003289
54. Lascarrou JB, Merdji H, Le Gouge A, Colin G, Grillet G, Girardie P, et al. Targeted temperature management for cardiac arrest with nonshockable rhythm. N Engl J Med. (2019) 381:2327–37. doi: 10.1056/NEJMoa1906661
55. Shankaran S, Laptook AR, Ehrenkranz RA, Tyson JE, McDonald SA, Donovan EF, et al. Whole-body hypothermia for neonates with hypoxic-ischemic encephalopathy. N Engl J Med. (2005) 353:1574–84. doi: 10.1056/NEJMcps050929
56. Harrington DK, Walker AS, Kukuntla H, Bracewell RM, Clutton-Brock TH, Faroqui M, et al. Selective antegrade cerebral perfusion attenuates brain metabolic deficit in aortic arch surgery. a prospective randomized trial. Circulation. (2004) 110(Suppl. II): 231–6. doi: 10.1161/01.CIR.0000138945.78346.9c
57. Erecinska M, Thoresen M, Silver IA. Effects of hypothermia on energy metabolism in mammalian central nervous system. J Cerebral Blood Flow Metab. (2003) 23:513–30. doi: 10.1097/01.WCB.0000066287.21705.21
58. Zhao H, Steinberg G, Sapolsky RM. General vs. specific actions of mild-moderate hypothermia in attenuating cerebral ischemic damage. J Cerebral Blood Flow Metab. (2007) 27:1879–94. doi: 10.1038/sj.jcbfm.9600540
59. Drew KL, Buck CL, Barnes BM, Christian SL, Rasley BT, Harris MB. Central nervous system regulation of mammalian hibernation: implications for metabolic suppression and ischemia tolerance. J Neurochem. (2007) 102:1713–26. doi: 10.1111/j.1471-4159.2007.04675.x
60. Lyden P, Hemmen T, Grotta J, Rapp K, Ernstrom K, Rzesiewicz T, et al. Results of the ICTuS 2 Trial. Stroke. (2016) 47:2888–95.
61. Andresen M, Gazmuri JT, Marin A, Regueira T, Rovegno M. Therapeutic hypothermia for acute brain injuries. Scand J Trauma Resusc. (2015) 23:42. doi: 10.1186/s13049-015-0121-3
62. van der Worp HB, Macleod MR, Bath PMW, Bathula R, Christensen H, Colam B, et al. Therapeutic hypothermia for acute ischaemic stroke. results of a European multicentre, randomised, phase III clinical trial. J Cerebral Blood Flow Metab. (2019) 4:254–62. doi: 10.1177/2396987319844690
63. Choi JH, Pile-Spellman J. Selective brain hypothermia. In: AA. Romanovsky, editor. Handbook of Clinical Neurology, Vol. 157 (3rd series) Thermoregulation: From Basic Neuroscience to Clinical Neurology, Part II. (2018). p. 839–52.
64. Christian E, Zada G, Sung G, Giannotta SL. A review of selective hypothermia in the management of traumatic brain injury. Neurosurg Focus. (2008) 25:E9. doi: 10.3171/FOC.2008.25.10.E9
65. Hachimi-Idrissi S, Corne L, Ebinger G, Michotte Y, Huyghens L. Mild hypothermia induced by a helmet device: a clinical feasibility study. Resuscitation. (2001) 51:275–81. doi: 10.1016/S0300-9572(01)00412-9
66. Bayegan K, Janata A, Frossard M, Holzer M, Sterz F, Losert UM, et al. Rapid non-invasive external cooling to induce mild therapeutic hypothermia in adult human-sized swine. Resuscitation. (2007) 76:291–8. doi: 10.1016/j.resuscitation.2007.07.006
67. Gladen A, Iaizzo PA, Bischof JC, Erdman AG, Divani AA. A head and neck support device for inducing local hypothermia. J Med Dev. (2013) 8:110021–9. doi: 10.1115/1.4025448
68. Islam S, Hampton-Till J, Watson N, Mannakkara NN, Hamarneh A, Webber T, et al. Early targeted brain COOLing in the cardiac CATHeterisation laboratory following cardiac arrest (COOLCATH). Resuscitation. (2015) 95:61–7. doi: 10.1016/j.resuscitation.2015.09.386
69. Poli S, Purrucker J, Priglinger M, Ebner M, Sykora M, Diedler J, et al. Rapid induction of COOLing in stroke patients (iCOOL1): a randomised pilot study comparing cold infusions with nasopharyngeal cooling. Crit Care. (2014) 18:582. doi: 10.1186/s13054-014-0582-1
70. Sayedsaadat SM, Marasco SF, Daly DJ, McEgan R, Anderson J, Rodgers S, et al. Selective brain hypothermia: feasibility and safety study of a novel method in five patients. Perfusion. (2019) 35:96–103. doi: 10.1177/0267659119853950
71. Assis FR, Narasimhan B, Ziai W, Tandri H. From systemic to selective brain cooling – Methods in review. Brain Circ. (2019) 5:179–86. doi: 10.4103/bc.bc_23_19
72. Wu L, Wu D, Yang T, Xu J, Chen J, Wang L, et al. Hypothermic neuroprotection against acute ischemic stroke: The 2019 update. J Cerebral Blood Flow Metab. (2020) 40:461–81. doi: 10.1177/0271678X19894869
73. Esposito E, Ebner M, Ziemann U, Poli S. In cold blood: intraarterial cold infusion for selective brain cooling in stroke. J Cerebral Blood Flow Metab. (2014) 34:743–52. doi: 10.1038/jcbfm.2014.29
74. Dumitrascu OM, Lamb J, Lyden PD. Still cooling after all these years: meta-analysis of pre-clinical trials of therapeutic hypothermia for acute ischemic stroke. J Cerebral Blood Flow Metab. (2016) 36:1157–64. doi: 10.1177/0271678X16645112
75. Choi JH, Mangla S, Barone FC, Novotney C, Pile-Spellman J. Rapid and selective brain cooling and maintenance of selective cooling with intra-carotid cold fluid infusion is feasible and safe. Eur Stroke J. (2016) 1:391. doi: 10.1177/2396987316642909
76. Caroff J, King RM, Mitchell JE, Marosfoi M, Licwinko JR, Gray-Edwards HL, et al. Focal cooling of brain parenchyma in a transient large vessel occlusion model: proo-of-concept. J Neurointervent Surg. (2020) 12:209–13. doi: 10.1136/neurintsurg-2019-015179
77. Solar RJ, Lownie SP. Neuroprotection by selective endovascular brain cooling-the TwinFlo catheter. EuroIntervention. (2020) 15:1291–6. doi: 10.4244/EIJ-D-19-00316
78. Merrill TL, Mitchell JE, Merrill D. Heat transfer analysis of catheters used for localized tissue cooling to attenuate reperfusion injury. Med Engin Physics. (2016) 38:75. doi: 10.1016/j.medengphy.2016.05.007
79. Choi JH, Marshall RS, Neimark MA, Konstas AA, Lin E, Chiang YT, et al. Selective brain cooling with endovascular intracarotid infusion of cold saline: a pilot feasibility study. AJNR Am J Neuroradiol. (2010) 31:928–34. doi: 10.3174/ajnr.A1961
80. Peng X, Wan Y, Liu W, Dan B, Lin L, Tang Z. Protective roles of intra-arterial mild hypothermia and arterial thrombolysis in acute cerebral infarction. SpringerPlus. (2016) 5:1988. doi: 10.1186/s40064-016-3654-7
81. Chen J, Liu L, Zhang H, Geng X, Jiao L, Li G, et al. Endovascular hypothermia in acute ischemic stroke. pilot study of selective intra-arterial cold saline infusion. Stroke. (2016) 47:1933–35. doi: 10.1161/STROKEAHA.116.012727
82. Wu C, Zhao W, An H, Wu L, Chen J, Hussain M, et al. Safety, feasibility, and potential efficacy of intraarterial selective cooling infusion for stroke patients treated with mechanical thrombectomy. J Cerebral Blood Flow Metab. (2018) 38:2251–60. doi: 10.1177/0271678X18790139
83. ClinicalTrials.gov Identifier: NCT03163459. Available online at: https://clinicaltrials.gov/ct2/show/NCT03163459 (accessed September 29, 2020).
84. UMIN-CTR Clinical Trial Identifier: UMIN000018255. Available online at: https://upload.umin.ac.jp/cgi-open-bin/ctr_e/ctr_view.cgi?recptno=R000021101 (accessed September 29, 2020).
Keywords: stroke, reperfusion, reperfusion injury, hypothermia, brain cooling, selective endovascular brain cooling, neuroprotection
Citation: Choi JH, Poli S, Chen M, Nguyen TN, Saver JL, Matouk C and Pile-Spellman J (2020) Selective Brain Hypothermia in Acute Ischemic Stroke: Reperfusion Without Reperfusion Injury. Front. Neurol. 11:594289. doi: 10.3389/fneur.2020.594289
Received: 13 August 2020; Accepted: 15 October 2020;
Published: 13 November 2020.
Edited by:
Richard Leigh, National Institutes of Health (NIH), United StatesReviewed by:
Miao Chen, University of Shanghai for Science and Technology, ChinaYuchuan Ding, Wayne State University, United States
Copyright © 2020 Choi, Poli, Chen, Nguyen, Saver, Matouk and Pile-Spellman. This is an open-access article distributed under the terms of the Creative Commons Attribution License (CC BY). The use, distribution or reproduction in other forums is permitted, provided the original author(s) and the copyright owner(s) are credited and that the original publication in this journal is cited, in accordance with accepted academic practice. No use, distribution or reproduction is permitted which does not comply with these terms.
*Correspondence: Jae H. Choi, amFlaGNob2kwNTI0JiN4MDAwNDA7Z21haWwuY29t