- 1Department of Physiology Case Western Reserve University, Cleveland, OH, United States
- 2FloTBI Inc., Cleveland, OH, United States
- 3Neurovascular Research Laboratory, Faculty of Life Sciences and Education, University of South Wales, Wales, United Kingdom
- 4IRMB, INM, UFR Odontology, University Montpellier, INSERM, CHU Montpellier, CNRS, Montpellier, France
- 5Brain Molecular Imaging Lab, CNRS UMR 5287, INCIA, University of Bordeaux, Bordeaux, France
- 6Cerebrovascular and Glia Research, Department of Neuroscience, Institute of Functional Genomics (UMR 5203 CNRS—U 1191 INSERM, University of Montpellier), Montpellier, France
Within the neurovascular unit (NVU), the blood–brain barrier (BBB) operates as a key cerebrovascular interface, dynamically insulating the brain parenchyma from peripheral blood and compartments. Increased BBB permeability is clinically relevant for at least two reasons: it actively participates to the etiology of central nervous system (CNS) diseases, and it enables the diagnosis of neurological disorders based on the detection of CNS molecules in peripheral body fluids. In pathological conditions, a suite of glial, neuronal, and pericyte biomarkers can exit the brain reaching the peripheral blood and, after a process of filtration, may also appear in saliva or urine according to varying temporal trajectories. Here, we specifically examine the evidence in favor of or against the use of protein biomarkers of NVU damage and BBB permeability in traumatic head injury, including sport (sub)concussive impacts, seizure disorders, and neurodegenerative processes such as Alzheimer's disease. We further extend this analysis by focusing on the correlates of human extreme physiology applied to the NVU and its biomarkers. To this end, we report NVU changes after prolonged exercise, freediving, and gravitational stress, focusing on the presence of peripheral biomarkers in these conditions. The development of a biomarker toolkit will enable minimally invasive routines for the assessment of brain health in a broad spectrum of clinical, emergency, and sport settings.
Introduction: From Blood–Brain Barrier to Blood–Brain Dynamic Interface
The blood–brain barrier (BBB) is the complex and finely tuned network of brain capillaries governing the homeostatic exchange of ions, molecules, and cells between the brain and the peripheral blood (1–3). The importance of the BBB in the understanding and diagnosis of neurological disorders and brain health is recognized (4). The notion of BBB has evolved from that of a static brain shield to that of a dynamic blood–brain interface where endothelial cells continuously communicate with mural cells (pericytes and smooth muscle) and glia (astrocytes and microglia), located near neurons and spatially assembled to constitute the neurovascular unit (NVU) (Figure 1) (2). A precise layering of cells and extracellular matrixes forms an impermeable wall (Figure 1B). BBB dysfunction has etiologic and diagnostic significance (4), and BBB permeability is a key element of perivascular and neuroinflammation (Figure 1B1) (5, 6). Increased BBB permeability provokes an immediate loss of homeostatic control of ions, ATP, and neurotransmitters levels in the brain, promoting abnormal synaptic transmission or neuronal firing, possibly leading to neurological sequelae (6–13). On the other hand, neuronal activity significantly influences cerebrovascular functions in health and disease conditions (14, 15). Diagnostically and because of increased BBB permeability, peripherally injected imaging contrast agents can access the brain parenchyma while a suite of central nervous system (CNS) proteins (see Table 1) or nucleic acids [circulating free DNA and microRNA; for a review see (44, 45)] can exit into the peripheral blood (Figures 2A–C, 3A–C). Contrast MRI and CT scans are common clinical tools, while monitoring the levels of CNS proteins in peripheral body fluids represents a novel strategy for identifying BBB and neuronal damage (46). Importantly, the NVU connects with specialized brain acellular spaces through which the cerebrospinal and interstitial fluids carry ions, molecules, and proteins across the parenchyma or toward waste clearance pathways (Figure 2B) (47–50). This spatial perivascular and interstitial connectivity is important in the context of contrast-based brain imaging, possibly influencing the availability of biomarkers and their exit trajectories from the CNS [Figure 2; see (46, 51, 52) for a review]. Starting from these fundamental concepts, we here examine the evidence supporting the development and the use of specific peripheral biomarker proteins to detect glioneuronal damage and BBB permeability in a plethora of clinical, emergency and sport-related settings.
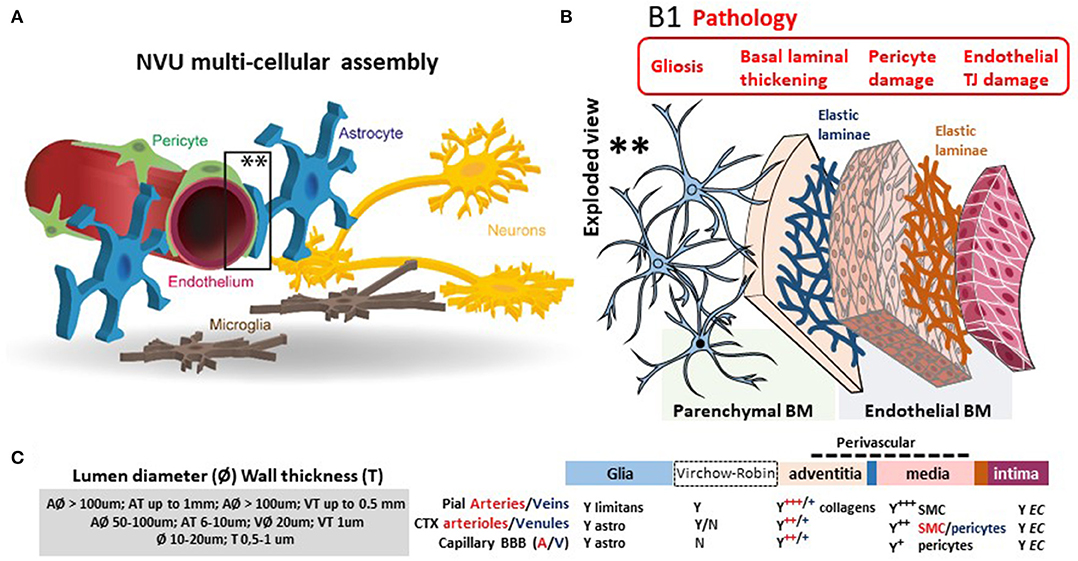
Figure 1. The dynamic NVU multicellular layout. (A) Within the NVU, the BBB encapsulates a set of unique properties of the microvascular capillary and post-capillary venules. The BBB endothelium lacks fenestrations, is assembled by structured tight junctions (TJs), and expresses luminal or abluminal transporters, altogether finely regulating brain homeostasis for proper neuronal physiology. These endothelial specializations are generated and controlled by precise interactions with pericytes, astrocyte end-feet, and microglial cells, all participating to the NVU. (B) Exploded view to illustrate the varying cellular composition and wall thickness of the intima, media, and adventitia layers. The endothelial basement membrane (BM) embeds pericytes. A second basement membrane is deposited by astrocytes and surrounds the end-feet. At the capillary level, the endothelial and parenchymal basement membranes merge. At the post-capillary venules, the two basement membranes separate to provide a perivascular space that allows for immune cells homing. (B1) Commonly reported pathological modifications leading to BBB permeability or NVU damage. (C) The cerebrovasculature in numbers (A, arteries; V, veins; CTX, cortex; SMC, smooth muscles cells). Proper BBB commences as the deepening cortical arteries (diameter > 100 μm in mice) branch into arterioles (diameter 15–50 μm; wall thickness 5–10 μm) and capillaries (diameter <10 μm; wall thickness a few μm). Pial vessels have glia limitans and an anatomically distinguishable Virchow-Robin space (B). Blood flow velocity rates in cortical mouse arterioles and capillaries are 3 and <0.5 mm/s, respectively. Capillary blood flow decreases with cortical depth (down to 0.1 mm/s). Diameter ranges (rodent) and anatomical abundance of glia, Virchow-Robin space, collagens, and mural cells (smooth muscles or pericytes) is provided. The multicellular layering within the tunica media (sign +++ indicates more than three smooth muscle cells at the arteries; + indicates one layer of pericytes at the BBB) is the major determinant of vascular thickness and elasticity. Original images by NM and IGF graphical service.
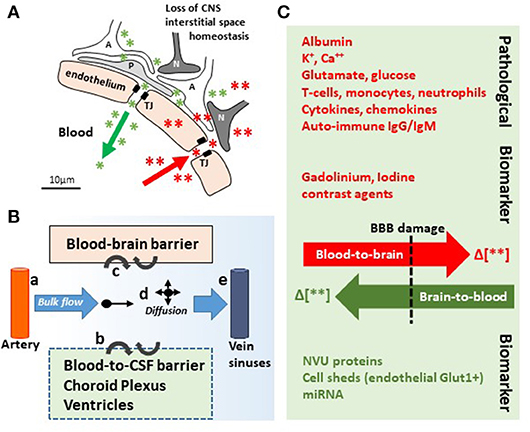
Figure 2. In and out the brain: plausible biomarker exit routes and transport. (A) NVU cell disassembly causes disruption of brain homeostasis, allowing blood (red asterisks and arrow) and brain (green asterisks and arrow) components directly crossing the permeable BBB (TJ, tight junctions; A, astrocytes; P, pericytes; N, neurons). (B) Brain fluid paths regulate the movement of molecules (e.g., biomarkers) within the brain parenchyma into the blood and the CSF: (a) the CSF is produced by subarachnoid arteries and (b) the choroid plexus (blood-to-CSF barrier). By bulk flow mechanisms, the CSF diffuses at the cortical levels and in periventricular organs, constituting a possible vehicle for biomarker transport. (c) BBB damage allows biomarkers exiting (or entering) the brain. (d,e) Parenchymal bulk flow and CSF reabsorption occurs at larger veins, dural venous sinuses, and dural lymphatic vessels, sites where biomarkers could accumulate. (C) Concentration gradients (green/red Δ or arrows) between the peripheral blood and the brain parenchyma are the underpinning for pathological modifications and the driving force for biomarkers (e.g., MR contrast agent brain entry, red; protein biomarkers brain exit, green). Original images by NM.
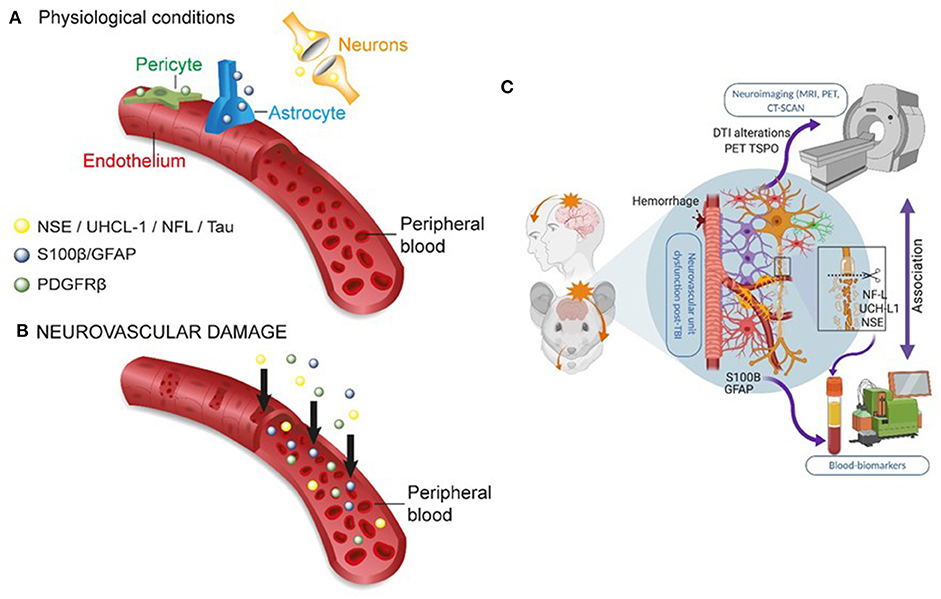
Figure 3. Peripheral biomarkers of BBB permeability and brain damage. (A) Under physiological conditions, BBB tightness within a healthy NVU limits proteins from exiting the brain into the peripheral blood. (B) In conditions of brain damage, each neurovascular cell acts as a source of specific biomarker(s) (color coded), accessing the peripheral blood across a leaky BBB. The production or secretion of biomarkers at each NVU cell type depends on the severity, time, and the progression of disease states (see Table 1). (C) Integrating the use of brain imaging and peripheral biomarkers is a developing strategy to detect brain damage and to validate the usefulness of specific biomarker proteins in peripheral fluids. Original images by NM, JB, and IGF graphical service.
Peripheral Biomarkers: Basic Concepts and Focus on Traumatic Brain Injury
Elevated BBB permeability, or dysfunction, occurs in response to an acute injury (e.g., head trauma, stroke, and status epilepticus) and may be present throughout CNS disease progression (e.g., neurodegeneration, epileptogenesis, and multiple sclerosis), often due to inflammation (5–7, 13). Peripheral biological fluids represent suitable matrices to detect and quantify brain-derived proteins reporting BBB permeability and susceptibility to glio-neuronal damage (27–29, 53). Table 1 provides a list of protein biomarkers and their characteristics, properties, and proposed use in diagnostics. In general, peripheral biomarker proteins must (i) be present in brain interstitial fluids or be released by neurovascular cells into the interstitial or perivascular spaces, reaching the peripheral blood across a leaky BBB or by cerebrospinal fluid (CSF)–blood exchange (Figures 2A, 3A,B); (ii) have a concentration gradient driving passive diffusion [Figure 2C; see (29)]; (iii) have a known and appropriate half-life to allow diagnostic interpretation (29) (biomarker half-life in peripheral fluids may impact usefulness in acute vs. long-term settings; see Table 1); and (iv) have a low molecular weight to allow a rapid egress across the damaged barriers or interfaces (19, 25, 27).
The bulk of neurological clinical biomarker literature has often focused on traumatic brain injury (TBI), with a recent emphasis on mild TBI (mTBI) (18, 54). Within this framework, the astrocytic protein S100B (55) has been examined as a peripheral biomarker of BBB permeability and gliosis (Table 1 and Figures 3A,B). Early proof-of-principle studies showed serum S100B levels to rapidly increase in response to a sudden BBB permeability, supporting the hypothesis that perivascular S100B can readily exit the brain (27, 28, 56). S100B was reported to rule out mTBI sequelae in emergency room settings (57), and measurement of blood S100B levels displayed a 99.7% negative predictive value (NPV) (57–60). Further evidence indicated that monitoring S100B after a mTBI could override the need for a CT scan for the identification of intracranial injury, with an excellent NPV (61). However, another study reported no relationship between serum S100B concentration and mTBI severity (62). In sports, S100B blood levels increased immediately after football games as compared to pregame baselines in players experiencing repeated head hits (25, 63). The evidence of a rapid S100B surge in blood after sub concussive hits was confirmed in follow-up studies (63–65). Importantly, extra-CNS sources of S100B were reported, representing a potential confounding factor if timing of blood draws in relation to injury is not adequately controlled and standardized (18, 66). These concerns have been discussed in (23, 67, 68).
The astrocytic glial fibrillary actin protein (GFAP) and the neuronal ubiquitin carboxyl-terminal hydrolase isoenzyme L1 (UCH-L1) are important biomarker candidates for glioneuronal damage (Table 1 and Figures 3A,B). UCH-L1 is also expressed at the neuromuscular junction (69, 70) while the contribution of extracranial sources of GFAP is debated (20, 71, 72). Monitoring of blood GFAP and UCH-L1 levels was used to grade brain injury after TBI. GFAP and UCH-L1 levels were increased in non-concussive and concussive head trauma as compared to body trauma (73, 74). The analysis of blood GFAP (or S100B) levels within 24 h from the head injury was proposed as a means to improve the detection of TBI and to identify patients in need of a subsequent MRI, in addition to routine CT surveillance (75, 76). GFAP and UCH-L1 blood levels were used to rule out intracranial injuries and the need for CT scans, showing high test sensitivity and NPV (21). One study reported no significant difference in blood UCH-L1 between control and players who sustained repetitive head hits (77). Collectively, this evidence points to GFAP as a diagnostic candidate to be used in TBI (33, 54, 71, 72). In two studies (78, 79), however, GFAP and UCH-L1 levels were below the lower limits of quantification or detection (LLOQ or LLOD, respectively) in a percentage of both TBI and trauma control groups, representing a possible concern for estimating NPV (20, 80).
Important biomarkers detecting neuronal damage are myelin basic protein (MBP), neuron-specific enolase (NSE), tau, and neurofilament light chain [NfL; Table 1 and Figures 3A,B; see (43)]. Blood MBP levels were unchanged in a pediatric mTBI population as compared to controls. Interestingly, MBP levels remained elevated for up to 2 weeks in case of intracranial hemorrhage (81). NfLs are found in axons and have been proposed as biomarkers of axonal damage triggered by mTBI, for example, after an amateur boxing bout (82–84). S100B levels were also increased following amateur boxing (85). Further evidence indicated neurofilament heavy chain increase after mTBI (82). Finally, NSE levels in CSF were shown to be proportional to TBI severity, in the setting of moderate or severe TBI (86–88). NSE in the blood is less investigated due to its presence in erythrocytes (89, 90). Collectively, these data support the further development of blood biomarker toolkits of TBI, with a special relevance to mild head injury and sport-related (sub)concussions, when emergency and sideline diagnostic solutions need to be readily accessible.
Phosphorylated TAU as an Emerging Blood Biomarker of Alzheimer's and Neurodegenerative Diseases
Accumulating evidence points to blood phosphorylated tau as a promising biomarker to improve the diagnosis and staging of and to enable trials in Alzheimer's disease (AD) subjects. In a cross-sectional study performed in AD patients, phosphorylated tau isoforms were used as diagnostic biomarkers to track disease progression (91). A method measuring attomolar concentrations of tau isoforms in plasma was implemented using stable isotope labeling kinetics and mass spectroscopy. Changes in plasma p-tau, particularly p-tau217, mirrored specific changes in CSF to detect phosphorylation of soluble tau and amyloidosis. No correlation was found between CSF and plasma p-tau202 levels. Plasma p-tau217 level distinguished amyloid-negative from amyloid-positive groups regardless of the cognitive status, indicating that p-tau217 in plasma may be an accurate biomarker of abnormal brain tau metabolism. Furthermore, a longitudinal study of familial AD (presenting pathogenic mutations in PSEN1 or APP genes) included 19 symptomatic and 51 asymptomatic participants where plasma p-tau181 levels were quantified by using a single-molecule array (Simoa) method (92). Elevated plasma p-tau181 concentrations segregated symptomatic mutation carriers from non-carriers. In another cross-sectional study including the Arizona-based neuropathology cohort (37 AD and 47 without AD), the Swedish BioFINDER 2 cohort [121 AD, 178 mild cognitive impairment [MCI], 301 without AD, and 99 other neurological disorders], and a Columbian autosomal-dominant AD kindred (365 PSEN1 E280A mutation carriers and 257 mutation non-carriers), plasma tau phosphorylated at the threonine 217 (p-tau217) was quantified by the Meso Scale Discovery (MSD) assay as a diagnostic AD biomarker (93). Among 1,402 participants from the three cohorts, plasma p-tau217 discriminated AD from other neurological disorders with higher accuracy compared with plasma p-tau181, plasma Nfl, CSF p-tau181, and CSF Aβ42:Aβ40 ratio. A positive correlation between CSF and plasma p-tau217 was found in the Swedish BioFINDER 2 cohort. Finally, a high-sensitivity immunoassay measuring p-tau181 in plasma and serum was developed (94). A positive correlation was reported between plasma and CSF p-tau181 levels, distinguishing Aβ-negative cognitively unimpaired older adults from Aβ-positive older adults and Aβ-positive individuals with MCI.
Furthermore, at the BBB, the low-density receptor-related protein 1 (LRP1) plays an important role in regulating cerebrovascular permeability (95). sLRP1, a truncated soluble form of LRP1, freely circulates in plasma, and it sequesters unbound Aβ in the peripheral circulation (96). Plasma sLRP1 levels are significantly reduced in AD patients, and sLRP1 binding to Aβ is disrupted by oxidation (96, 97). Impaired sLRP1-mediated binding of plasma Aβ was suggested as an early biomarker for MCI preceding AD-type dementia (97). In summary, this evidence supports the further development of tau-based blood biomarkers as an accessible test for the screening and diagnosis of AD within the spectrum of cognitive impairments and dementia.
Peripheral Biomarkers of BBB Permeability and Seizure Conditions
The use of blood biomarkers extends to epilepsies, a cluster of diseases where BBB damage represents an etiological or a contributing pathophysiological player (98–100). A first study (67) demonstrated that blood S100B is elevated at seizure onset and after seizures, in support of the hypothesis that BBB damage may trigger a seizure (7, 101–103). A systematic review analyzed 18 studies and a total of 1,057 subjects, indicating that epileptic patients displayed elevated S100B blood levels as compared to controls (104). Meta-regression analyses showed that gender and mean age can impact serum S100B levels (104). Another study correlated MRI T1 peri-ictal imaging to blood S100B in drug-resistant epileptic patients, confirming the increase in BBB permeability during a seizure (105). Increased S100B blood levels were reported in pediatric temporal lobe epilepsy, with blood samples obtained 30 min after a complex partial seizure (106).
Children suffering from intractable focal epilepsy displayed elevated blood S100B levels as compared to controls (107). One study included 39 patients suffering from simple febrile seizures and age- and sex-matched controls, showing no S100B differences between groups when assessed immediately after seizures (108). These findings were corroborated in a follow-up study, (109) with the conclusion that febrile seizures are relatively harmless to the developing brain. Currently, a clinical trial is investigating whether S100B, as well as other protein biomarkers, increase in blood after a first generalized seizure could be used to predict first-to-chronic seizure conversion in adult subjects (https://clinicaltrials.gov/ct2/show/NCT02424123). Moreover, NSE elevations were reported in blood over time in patients affected by temporal lobe and extratemporal lobe epilepsies (110). Finally, recent evidence indicates miRNA in blood, or body fluids, as potential biomarkers indicating neurovascular and neuroinflammatory modifications occurring in specific forms of epilepsies [see (111–113) for comprehensive topic reviews]. In summary, blood biomarkers could represent a surrogate method of clinical electroencephalographic explorations to examine damage and brain neurophysiology in epileptic patients.
Imaging BBB Permeability and Brain Damage: Is the Integration With Blood Biomarkers Possible?
Available evidence supports the prospective use of blood biomarkers to detect NVU damage in acute and chronic neurological conditions. In this context, can peripheral biomarkers replace brain imaging? This is an important question especially if one considers the logistics (scarce imaging availability in rural areas and emergency, sport, and combat settings) and economic advantages that come with peripheral biomarkers, notwithstanding the complications associated with radiation exposure (e.g., CT scan). As a result, the diagnostic equivalence of blood biomarkers and enhanced MRI or CT scans (114–119) is being investigated. Accumulating evidence has shown that mTBI represents an optimal clinical arena to study the usefulness of imaging and peripheral biomarkers, also fulfilling an urgent clinical need (120–122). Neuroimaging techniques [CT scan (61)] show limitations for the diagnosis of mTBI patients (122, 123). Importantly, blood levels of GFAP, tau, and NfL were higher in patients with TBI-related findings on CT as compared to subjects presenting with normal CT, where the only significant predictor of damage was GFAP (124). Combining the biomarkers tau, NfL, and GFAP showed a good discriminatory power for detecting MRI abnormalities, even in mTBI patients with a normal CT (124). Furthermore, peak serum S100B levels negatively correlated with resting-state brain connectivity and behavioral outcomes in mTBI to severe TBI cases (125). S100B has proven its high NPV to rule out intracranial bleeding in patients after mTBI. However, its specificity for brain parenchyma structural lesions remains debated, and MRI is required for a specific explanation of clinical symptoms (76, 126, 127). Positron emission tomography (PET) and radiolabeled biomarkers were tested along with blood biomarkers. The [18F]AV1451 (flortaucipir) tau ligand was detected at the white/gray matter junction in frontal, parietal, and temporal brain regions, a typical localization of chronic traumatic encephalopathy (CTE) and tauopathy in veterans. Elevated levels of Nfl were also reported in plasma (43). Finally, TBI is associated with inflammation as blood levels of IL6, TNFα, and VEGF were increased in CT- and MRI-positive patients as compared to controls (126).
Importantly, newer brain imaging approaches are being tested. Proton magnetic resonance spectroscopy (1H-MRS) represents an emerging neuroimaging modality to track the metabolic changes occurring after TBI (128, 129). Spectroscopy can predict changes of key metabolites such N-acetylaspartate (NAA), a marker of neuronal loss (130), and its early decrease associates with long-term poor outcomes in clinical pediatric mTBI and moderate TBI (130). Experimentally, spectroscopy modifications post injury were linked to altered astrocyte metabolism (131). Brain structural changes observed using diffusion tensor imaging were correlated to astrocyte dysfunction and astrogliosis at early (1–7 days) and late (60 days) time points after injury (132, 133). Tractography provides an opportunity for measuring structural alterations in the white matter that are not detected by conventional structural MRI (134). Magnetic encephalography has also been proposed to study mTBI damage, in addition to being used for post-traumatic stress disorders (135, 136). Collectively, these data underscore the need for integrating the temporal and quantitative profiles of emerging imaging read-outs with the dynamics of peripheral biomarker of NVU damage. These studies will allow us to fully understand whether blood biomarkers can reliably act as surrogates for brain imaging.
Saliva as a Biomarker Matrix: General Concepts
Another key cellular “barrier” can be exploited for diagnostic purposes, namely, the salivary glands and gingival vessels, both interfacing with the peripheral blood (Figures 4A,B) (53, 137–144). While plasma and serum are considered as classic biofluids for assessment of systemic biomarkers, saliva is being increasingly viewed as a matrix with a high diagnostic value (141, 145). Saliva collection is economical, safe and can be performed without the assistance of specialized health care personnel, allowing for point-of-injury (POI) sampling. Saliva lacks cellular and soluble components (e.g., coagulation cascade). As the leakage of brain-derived biomarkers in saliva undergoes a process of biological filtration (53, 137, 146), the use of saliva does not require separation steps that are an obstacle to the development of POI blood tests (138, 139). Human saliva is a clear, slightly acidic (pH 6.0–7.0) heterogeneous biofluid composed of water (99%), proteins (0.3%), and inorganic substances (0.2%) (147). Saliva contains enzymes, hormones, antibodies, nucleic acids, antimicrobial constituents, and cytokines (148), which accumulate in salivary glands and are secreted into the oral cavity through acinar cell ducts (149). Available protocols indicate that saliva samples can be stored short term at room temperature and long term at −20°C or −80°C without significant protein degradation, similar to serum or plasma samples (150, 151). Relevant information inherent to the preparation and the technical handling of saliva samples can be found in (150, 152–154).
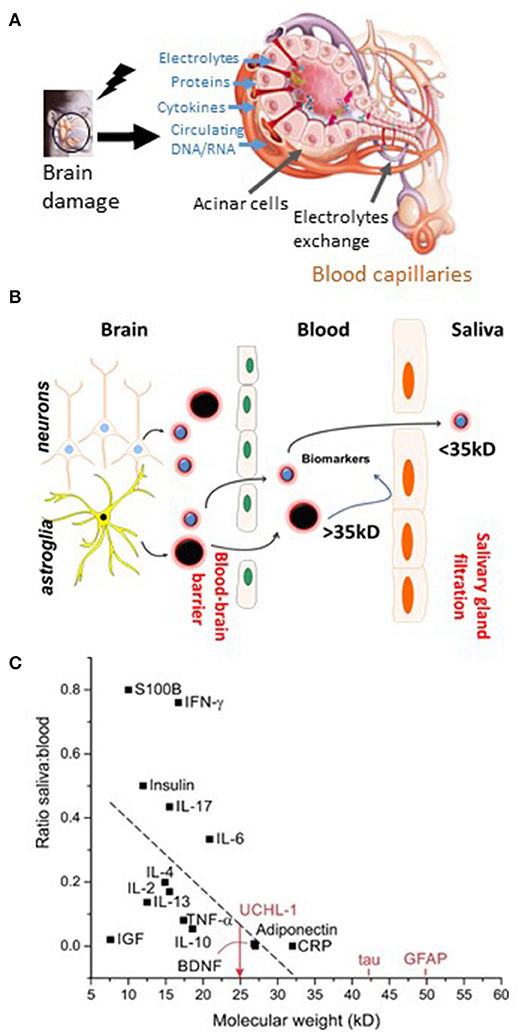
Figure 4. The blood-to-saliva interface and proposed salivary biomarkers. (A) Schematic representation of molecular transport or passage from blood into salivary glands. Salivary glands are highly vascularized, enabling exchange of blood-based constituents (ions, proteins, etc.). Alterations in the molecular composition of the blood may lead to modifications of the composition of saliva. (B) Biomarker extravasation from brain to blood depends on the permeability of the BBB to a given biomarker. Under normal conditions and when the BBB is intact, endothelial tight junctions restrict the passage of polar or large (>~300 Da) molecules. When the BBB is breached, appearance in the blood of brain-derived protein biomarkers occur. Next, the passage of protein from blood to saliva is proposed. (C) Possible protein ratio of saliva to blood for biomarkers and pro-inflammatory factors (see text for details and references). Original images by DJ and CH.
The whole saliva (WS) proteome, when compared with the plasma proteome, displays a larger proportion (14.5%) of low-molecular-weight proteins (<20 kDa), in contrast to only 7% for the plasma proteome (154). The highest fraction of proteins found in WS ranges from 20 to 40 kDa, whereas the 40–60 kDa range is the largest fraction for plasma. This is consistent with selective permeability between blood and saliva for low-molecular-weight proteins. Five diagnostic alphabets are outlined in saliva, including proteome (153, 155), transcriptome (156, 157), microRNA (158), metabolome (159), and microbiome (160). Saliva is used by clinical laboratories for the detection of secretory IgA antibodies, for the analysis of salivary cortisol and hormones, and for genetic purposes (161–163).
Salivary Biomarkers of NVU Damage: a New Diagnostic Opportunity?
The salivary proteome has been characterized in CNS disease conditions, such as schizophrenia, bipolar disorders, and genetic disorders including Down's syndrome and Wilson disease (164). An overview of biomarkers identified in saliva for the diagnosis of neurodegenerative diseases such as AD, Parkinson's disease, amyotrophic lateral sclerosis, and multiple sclerosis is provided in (165). Inflammatory biomarkers (e.g., IL-1β, TNF-α, and IL-6) have been quantified in saliva (166).
The deployment of POI salivary tests represents an opportunity for the detection of time-sensitive brain injuries (139–141, 167, 168). NSE was shown as a possible diagnostic salivary biomarker for neuronal damage in patients post stroke (169). Saliva samples have been analyzed for S100B levels, pro-inflammatory factors, and microRNAs in the settings of TBI (168, 170, 171). In particular, S100B levels in saliva were elevated in children post TBI (171). In another pilot study, 15 adult patients with suspected TBI and 15 control subjects were studied. Average salivary S100B level was 3.9-fold higher than blood S100B level, regardless of the presence of pathology [S100B]saliva correlated positively with [S100B]serum, and salivary S100B levels were as effective in differentiating TBI patients from control subjects as serum levels (172).
In an attempt to further accentuate the diagnostic significance of salivary testing, we reviewed the literature to obtain potential blood-to-saliva ratios for a number of proteins (Figure 4C). This search was directed to proteins that are not secreted by salivary glands. These proteins can access the salivary fluid by pericellular capillary leak, primarily the crevicular fluid. Importantly, it is currently unknown whether the steady-state permeability of the blood-to-saliva protein diffusion is preserved even at times when the BBB is breached due to brain insults. Literature references were used to examine insulin (173, 174), EGF (175), HGH (19, 176), S100B (18, 54–56, 177–180), adiponectin (181), prostate-specific antigen (PSA) (182), and cytokines (183). To our knowledge, there are no reports of salivary BDNF or NFL levels. All retrieved values were plotted to outline the theoretical cutoff properties of salivary filtration (Figure 4C). Large molecules (e.g., IgG) can be present in saliva owing to active secretion or local production.
Finally, we examined whether blood-to-saliva biomarkers' passage could be empirically predicted or modeled (153). Available data indicate that saliva is not a diluted substitute for the determination of plasma protein levels, as indicated by the incoherent plasma and saliva proteomes (152). Therefore, understanding the kinetic protein passage from blood to saliva is difficult. In the past, a model describing the passage of biomarkers from the brain into the peripheral blood was proposed (27–29). A physiologically based pharmacokinetic model can be used to describe the distribution of drugs and small molecules in body fluids (184). This computational approach can estimate the extent and time course of salivary biomarkers originating from the brain, offering the likelihood of a protein in saliva to be blood-borne (185). The physiologically based pharmacokinetic model used to describe the distribution of brain-derived biomarkers in blood was expanded to include an idealized salivary gland receiving its vascular supply from the external carotid. The venous output was mimicked according to the properties of jugular vein branches. To approximate the combined contribution of transcellular and paracellular pathways of protein extravasation across capillary endothelial cells and salivary gland epithelia, the following equation was used to calculate Js, the transfer of protein from blood to saliva:
where Js (mol/min) is the mass transfer from blood to saliva, Jv (ml/min) is the blood flow to the salivary gland, R is the reflectance of the vascular wall, Cp (mol/L) is the concentration of biomarker in the serum, Ci (mol/L) is the concentration of biomarker in the saliva, and P and S refer to permeability (cm/s) and surface of exchange (cm2), respectively. The value of reflectance has no dimension and has a range from one (no passage of protein) to 0 (protein passage dictated by diffusion alone). The value of reflectance is derived from pore radius and molecular radius. To estimate PS, we used PS = Jv * Ci/(Cp – Ci) with salivary flow at 1 ml/min and Ci and Cp at 2.5 and 61.5 mg/ml, respectively. These values were derived by measurements and transfer of albumin levels from blood and saliva. The equation can be greatly simplified by fitting experimental data to confirm their accuracy. Once this is done, the predictors of passage of a given protein are primarily related to its molecular size (vascular wall reflectance) and the presence of a gradient for passage from blood to crevicular fluid. For (1), note that if the reflectance tends toward 1 (large molecular weight), the first term equals zero, thus leaving only the permeability of the capillary wall and the osmotic gradient as variables. Considering that permeability also depends on molecular size, a cutoff for extravasation seems to be mostly related to the size of the permeating protein. By using other computational models, it was shown that the physicochemical properties of proteins were the main predictors of presence in saliva. Among several properties, molecular size was the most relevant (185, 186).
It is important to underscore that the use of saliva samples comes with confounding factors. For instance, gingivitis or periodontal disease can affect the identification and quantification of proteins. It has been shown that submandibular saliva flow rates are lower in AD patients as compared to controls (187), possibly impacting the proportion of proteins detectable (188). In summary, fully defining the qualitative and quantitative characteristics of salivary biomarkers in physiological and neuropathological conditions is important to develop non-invasive point of care applicable to NVU screening.
Pushing the BBB Limits: Relevance of Peripheral Blood Biomarkers in Human Models of Extreme Brain Physiology
Here, we focus on extreme sport settings that can be exploited as ‘human' models to study BBB permeability, neuronal damage, and hemodynamic modifications in a controlled spatiotemporal manner. We review the evidence supporting the use of blood biomarkers to detect neurovascular modifications associated with extremes of cerebral blood flow (Figure 5A). These models share similar pathophysiological features unified by the cerebral formation of free radicals, associated reactive oxygen/nitrogen species (ROS/RNS), and impaired cerebral autoregulation (CA).
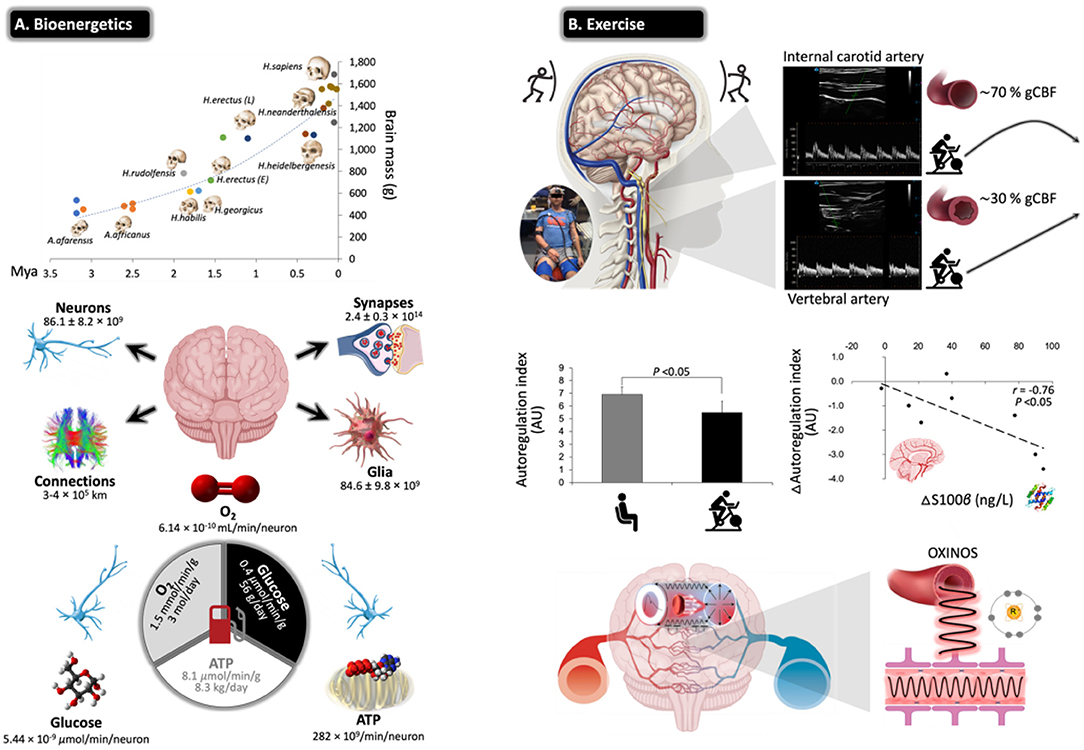
Figure 5. Challenges for the exercising human brain: applicability of NVU biomarkers. (A) Evolutionary ‘drive-for-size' with exponential increases in estimated brain mass observed in fossil hominids. Note the structural complexities and corresponding bioenergetic demands that define the ‘modern' human brain, highlighting its limited energy reserves in the form of oxygen (O2), glucose, and adenosine triphosphate (ATP) in the face of extraordinarily high rates of neuronal metabolism. This renders the human brain exquisitely sensitive to anoxia and ischemia, and thus, it has developed a sophisticated armory of mechanisms that collectively defend O2 homeostasis. Calculations cited and figures modified from (189). (B) Physical exercise poses unique challenges for the human brain with perfusion typically characterized by preferential redistribution to the phylogenetically ‘older' regions subserved by the posterior circulation (typical B-mode Doppler images illustrated). This makes teleological sense given that it is one of the most primitive neuroanatomical regions of the human brain, which has remained highly conserved across vertebrate evolution housing (almost exclusively) all the major cardiovascular and respiratory control centers essential for the integrated regulation of autonomic nervous control (190). However, this can come at a cost, with emerging evidence indicating that high flow/pressure and systemic/cerebral formation of free radicals and oxidative inactivation of nitric oxide [oxidative–nitrosative (OXINOS) stress] contribute to impaired cerebral autoregulation and BBB disruption. The latter is confirmed through proportional extravasation of brain-specific proteins, including S100B, in the absence of structural tissue damage. BBB permeability can cause extracellular vasogenic edema resulting in a regional O2 diffusion limitation, with the potential to adversely affect cerebral bioenergetics and cognition. This is relevant to patients already suffering from impaired cerebral autoregulation/autonomic dysfunction, including older adults, notwithstanding patients diagnosed with diabetes, hypertension, stroke, and AD (191) (original images by DMB).
Exercise, Cerebrovascular Regulation, and Blood Biomarkers
Evidence indicates that moderate-intensity continuous training (MICT) and corresponding improvements in cardiorespiratory fitness (CRF) can increase cerebral perfusion and vasoreactivity across the human life span (192, 193), translating into a lower risk of stroke mortality and dementia (194, 195). The primary mechanisms include accelerated neurogenesis, in particular of the hippocampal dentate gyrus (196); reduction in β-amyloid (197); neuro-oxidative inflammatory nitrosative stress (198); proprioceptive adaptations incurred by movements that require sustained mental effort (199); increased brain-derived neurotrophic factor that modulates brain plasticity by promoting neuritic outgrowth and synaptic function (200); and improved BBB integrity and bolstering of tight junctions (201). More recently, high-intensity interval training (HIIT) has emerged as a more time-efficient model of exercise that can potentially promote superior improvements in CRF and cerebrovascular adaptation (191). However, this type of exercise characterized by high-flow/high-arterial-pressure transmission poses unique challenges for the brain with emerging evidence suggesting that an acute bout of HIIT could increase BBB permeability in the absence of neuronal injury (e.g., increased blood S100B and no NSE changes), subsequent to a free radical-mediated impairment in dynamic CA that persists into the recovery period (202) (Figure 5B).
High-Altitude Mountaineering, Freediving, NVU Dynamics, and Blood Biomarkers
High-altitude (HA) mountaineering (Figure 6A) and freediving (Figure 6B) represent unique physiological models to study severe arterial hypoxemia (O2 lack) and hypocapnia/hypercapnia (CO2 lack/excess) in ‘extreme' athletes who consistently operate at, or very close to, the limits of human consciousness (189, 212). Diffusion-weighted magnetic resonance imaging has identified increases in brain volume, T2 relaxation time (T2-rt), and apparent diffusion coefficients (ADCs) in healthy participants acutely exposed to hypoxia, taken to reflect extracellular vasogenic edematous brain swelling (205, 206). These changes were pronounced in the splenium and genu of the corpus callosum, the likely consequence of a unique vascular constitution. Densely packed horizontal fibers characterized by short arterioles that lack adrenergic tone likely render it more susceptible to hyperperfusion edema in the setting of hypoxic cerebral vasodilatation and/or autoregulatory impairment (205, 206). Local sampling of CSF and arterial–jugular venous blood concentration gradients of biomarkers including S100B indicated that BBB disruption is likely minor and linked to increased free radical formation (207, 216).
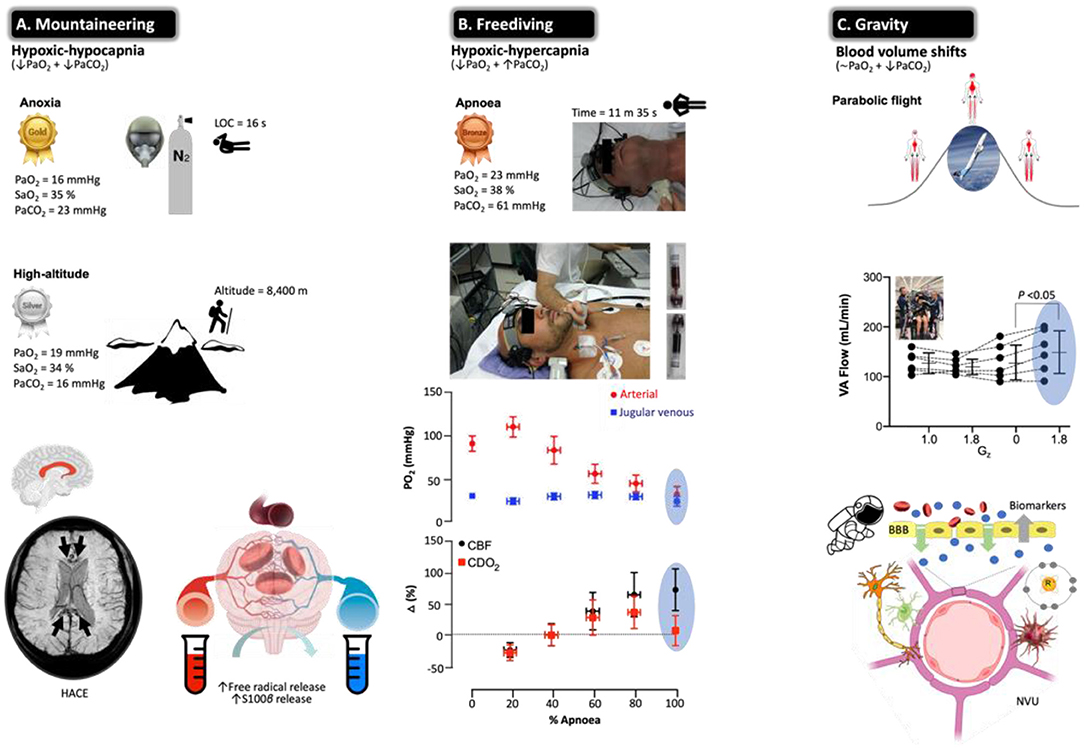
Figure 6. Beyond barriers: the brain under pressure and NVU blood biomarkers. Extreme examples highlighting how the human brain adapts to the most severe swings in circulating oxygen, carbon dioxide, and blood volume recorded in the published literature with data obtained from apparently healthy participants. All examples are unified by a general increase in CBF to ensure preservation of O2 and glucose substrate supply. Note the most hypoxemic (notwithstanding hypocapnic/hypercapnic) measurements documented in humans (gold, silver, and bronze awarded according to severity of hypoxemia) who regularly operate at the ‘cusp' of consciousness or indeed beyond. (A) Acute exposure to anoxia (pure nitrogen) resulted in an arterial partial pressure of O2 (PaO2) of 16 mmHg (lowest ever recorded) that resulted in unconsciousness within 16 s (203). The highest recorded femoral arterial puncture performed on an acclimatized mountaineer at 8,400 m on Mt. Everest revealed the second lowest (silver award) PaO2 (and lowest PaCO2) of 19 mmHg (204). Prolonged exposure to the severe hypoxia of terrestrial HA can result in HACE, a rare albeit deadly syndrome characterized by ataxia resulting in rapid progression to coma. Susceptibility-weighted MRI has revealed hemosiderin deposits (insoluble iron(III) oxide-hydroxide, reflecting micro-hemorrhages) in nonlethal HACE confined to the genu and splenium of the corpus callosum. In combination with vasogenic edematous brain swelling previously documented by MRI studies in healthy participants exposed to acute hypoxia (205, 206) and net trans-cerebral (arterio-jugular venous) outflow of free radicals and S100B (207–209) in the face of impaired dynamic cerebral autoregulation (210) has led to the suggestion that severe hypoxia results in permeability of the BBB subsequent to molecular (free radical-mediated increase in permeability) and hemodynamic (cerebral vasodilatation) stress (211). (B) The third lowest PaO2 (and highest PaCO2) recorded in a single freediver during the course of a static breath-hold (apnea) (212, 213). Note that the level of hypoxemia was so marked there was no observable arterio-jugular venous O2 gradient across the brain (marked cyanosis and ‘black blood' visible toward the end of apnea). Hypoxic-hypercapnic increase in CBF was sufficient to offset arterial hypoxemia and preserve the cerebral delivery of O2 (CDO2) (213), though a mild net trans-cerebral outflow in S100B was observed in a follow-up study taken to reflect mild, diffuse BBB disruption (214). (C) Gravitational stress and reciprocal changes in central blood volume were recently shown to increase blood flow to the posterior region of the brain (VA, vertebral arteries) arguably more prone to hyperperfusion injury. This was associated with a free radical-mediated reduction in nitric oxide (NO) bioavailability and mild damage of the NVU reflected by the extravasation of S100B and GFAP (215). Image credits: mountaineering (T*MRI of a 65-year-old woman 7 weeks after having developed HACE at 3,580 m, courtesy of Professor M Knauth, University Hospital Gottingen, Germany); freediver [photographs courtesy of Prof. PN Ainslie and the late Dr. CK Willie, University of British Columbia, Canada (213) with permission]; Gravity (Novespace's ZERO-G Airbus A300 credit: Novespace/CNES/DLR/ESA).
Some mountaineers, notably those who ascend (too) rapidly to altitudes above 2,500 m and thus not adequately acclimatized, can develop acute mountain sickness (AMS), a primary disorder of the CNS characterized by headache that is associated with, if not the primary trigger for, other vegetative symptoms (217). Traditionally, AMS has been considered a mild form of HA cerebral edema (HACE, the most malignant of all HA illnesses, oftentimes proving fatal) with a common pathophysiology of intracranial hypertension subsequent to vasogenic edematous brain swelling at opposing ends of a clinical continuum. An increase in intracranial pressure (ICP) could potentially result in the mechanical stimulation of pain-sensitive unmyelinated fibers that reside within the trigeminal–vascular system, triggering the symptoms of a headache (218). This makes intuitive sense in light of an early study that identified an increased T2 signal in the white matter of mountaineers with moderate to severe AMS in whom clinical HACE had not yet developed (no ataxia or altered consciousness) (219). However, follow-up MRI studies consistently failed to support this concept, with no clear relationships observed between hypoxia-induced increases in brain volume or T2-rt and cerebral AMS scores (206, 220). Indeed, the only defining morphological feature that distinguishes the AMS brain from its healthy counterpart is a selective attenuation in the ADC signal taken to reflect intracellular (cytotoxic) edema that likely coexists with extracellular vasogenic edema (206, 220). Attenuation of the ADC signal likely reflects fluid redistribution from within the extracellular space, as intracellular (astrocytic) swelling proceeds without any additional increment in brain volume, edema, or ICP (221). The underlying causes and temporal sequence are unknown, perhaps a reflection of ion pump suppression subsequent to (free radical-mediated) downregulation of Na+/K+-ATPase activity (211). More recent evidence suggests that a functional impairment in cerebral ‘venous outflow' at the level of the transverse venous sinus may prove the unifying risk factor for AMS (222).
Freediving (Figure 6B) offers yet another remarkable model of severe arterial hypoxemia (189, 212). The static apnea world record currently stands at an impressive 11 min 35 s held by Stéphane Mifsud. However, unlike mountaineers, apnea results in severe hypercapnia, further compounding the cerebral hyperemic stimulus (Figure 6B), with freedivers also having to contend with the additional challenge of elevated hydrostatic pressure when competing ‘at depth' in select disciplines and complications associated with pulmonary barotrauma, nitrogen narcosis, decompression sickness, and high-pressure neurologic syndrome (212). Competitive freedivers oftentimes experience shallow-water blackout due to severe cerebral hypoxia and loss of motor control, clinical signs that are the frustrating cause for disqualification from competition, notwithstanding immunochemical evidence for structural NVU damage, e.g., increased peripheral blood S100B and NSE after a maximal apnea, with potential long-term neuropsychological consequences (223, 224).
More recent, direct approaches have taken advantage of sampling arterial–jugular venous blood and combining regional measurements of CBF during the course of an apnea in champion freedivers (213, 214). Despite no detectable O2 gradient across the brain, a truly remarkable observation, CDO2 subsequent to increased perfusion was well maintained even at PaO2s as low as 23 mmHg (Figure 6B). Similar to the aforementioned acute hypoxia study (207), apnea was associated with a net trans-cerebral outflow of free radicals and S100B (in the absence of any local gradients in NSE or MBP) that may reflect minor BBB permeability due to the combination of hemodynamic (increased intracranial pressure) and molecular (increased free radical formation) stress in the absence of neuronal damage (214). Rather than consider this simply as a damaging maladaptive response, vasogenic edematous brain swelling may prove the adaptive phenotypical response in the hypoxia-tolerant human brain (211, 225).
Gravitational Stress, Cerebrovascular Regulation, and Blood Biomarkers
Alterations in gravitational fluid pressure gradients caused by the microgravity of orbital spaceflight and hypergravity associated with takeoff and landing pose unique physiological challenges for the astronaut brain. Recent interest has focused on the complex pathophysiology underlying a constellation of debilitating neurological, ophthalmological, and neurovestibular symptoms, known collectively as spaceflight-associated neuro-ocular syndrome (SANS) (226). At the cellular level, microgravity has been associated with a loss of cytoskeletal integrity through dissociation of actin and tubulin bundles (227), and evidence obtained using animal models suggests that BBB disruption may occur during the early phases of unloading induced by suspension or microgravity (228) and during hypergravity induced by prolonged centrifugation (229, 230). In a recent study (215), parabolic flight (PF), a ground-based spaceflight analog, was used as a human model to induce rapidly alternating shifts in central blood volume during repeated exposures to microgravity (0 Gz) interspersed with hypergravity (1.8 Gz) (231) to explore how altered CBF impacts the NVU (232) (Figure 6C). Blood flow to the posterior cerebral circulation (vertebral arteries) was selectively elevated during the most marked gravitational differential from microgravity to hypergravity. Posterior hyperperfusion was associated with a free radical-mediated reduction in nitric oxide bioavailability (oxidative–nitrosative stress) and selective increases in blood S100B and GFAP that persisted following return to microgravity, whereas blood biomarkers of neuronal–axonal damage (NSE, NFL, UCH-L1, and tau) remained stable (215). These findings suggest that the cumulative effects of repeated gravitational transitions may promote minor BBB damage due to the combined effects of hemodynamic-molecular stress. While we appreciate that PF is an entirely different stimulus dominated by hypergravity, these findings provide important mechanistic insight to help understand the neurological risks associated with prolonged microgravity during spaceflight, given that increased BBB permeability directly impacts neuronal function, predisposing to neurological sequelae and brain disease (6).
Blood Biomarkers of NVU Damage: Available Analytical Tools, Limitations, and Controversies
No single ideal peripheral biomarker exists; rather, a suite of biomarkers could have a significant diagnostic impact. In recent years, innovative methods for biomarker detection have been implemented (Table 2). Reaching high sensitivity has several advantages, particularly in the context of neurological settings. Foremost is the ability to detect biomarkers such as NfL, Tau, or GFAP that are readily present in the CSF and in low concentrations in the blood. New technology has enabled the quantification of brain-derived protein biomarkers in blood, getting one step closer to a minimally invasive diagnosis of brain damage and neurodegenerative processes. Furthermore, high-sensitivity methods use microliter quantities of biofluid, allowing the quantification of several analytes and multiplex measurement. As an example, we here provide NfL, GFAP, and tau serum baseline levels as measured in our laboratory using Simoa (Table 2). We include specific LLOD and LLOQ values relative to our particular experience. Obviously, this new technology presents limitations. A shortcoming of high-sensitivity assay resides in the fact that Research Use Only (RUO) kits are not able to provide, yet, a level of robustness and precision that one would expect for a clinical in vitro diagnostics (IVD) use. To date, the impact of analytic interference is not sufficiently investigated. Therefore, the expectations formulated following cohort-based studies need confirmations in large preclinical studies and multicentric clinical trials.
Although the use of blood biomarkers of BBB or neuronal damage is appealing, a number of clinical stumbling blocks currently limit full applicability. The usefulness of blood biomarkers in a given human depends on the availability of reference values, correcting for age, ethnicity, kidney function, and body mass index (29). Adequateness of the blood sampling schedule and availability of baseline controls are crucial for a reliable biomarker outcome. Sample readiness before and after pathological events (e.g., inpatient seizure monitoring and head trauma as in contact sports) provides the optimal framework to calculate biomarker differential in the same individual and within a controlled time frame (24, 25). Availability of ad hoc baseline samples (e.g., specific enrollments for sport events and military personnel) represents a robust method enabling personalized medicine.
As examined so far, the appearance of NVU proteins in blood is reported for neurodegenerative diseases (91, 94), brain tumors (115, 117), TBI (25, 233), neurologic manifestations of systemic disease (234), psychiatric diseases, and seizures (21, 53, 235). Peripheral biomarkers have an excellent NPV to rule out disease(s) but have a poor positive predictive value (PPV) to identify a specific pathological condition (27, 28, 46, 53, 236–238). Another concern is the potential contamination related to extra-CNS sources of protein biomarkers. For example, S100B could be derived from adipose tissue with levels directly depending on body mass index (239). A study excluded the impact of adipose tissue on S100B serum levels (23). Elevated serum S100B was reported in patients presenting with extracranial pathology (240), such as polytrauma and burns (66).
Another important question is whether peripheral biomarkers have a prognostic value for the development of long-term brain pathology. Currently, there is no collective agreement on whether an unhealthy BBB may already exist, and could be diagnosed, in an otherwise apparently healthy brain (241, 242). However, recent evidence indicates that subjects presenting early cognitive impairment had preexisting BBB damage. The platelet-derived growth factor receptor beta (PDGFRβ; Table 1) (243, 244) shedding from perivascular pericytes was proposed as a biomarker of BBB integrity anticipating and predicting neurodegeneration (39, 243). A high-sensitivity method for detecting pericyte injury quantifying PDGFRβ in CSF was recently proposed (245). This method could be extended to study brain pericyte–endothelial damage in neurodegenerative disorders. Moreover, repetitive head hits during contact sports [American football (25)] were shown to associate with recurrent BBB permeability and S100B increases in blood. Players experiencing recurrent BBB permeability presented higher serum reactive autoantibodies, with a possible correlation with cognitive defects (25). The clinical significance of repeated BBB damage in sports is currently debated, with evidence pointing to a role in accelerated neurodegeneration (73). Moreover, total tau in blood was reported as a biomarker of axonal damage in hockey (24). Tau and amyloid monitoring in CSF is undergoing validation processes for dementia and AD (246, 247).
Outlook and Final Remarks
Using peripheral biomarkers to monitor BBB permeability could extend to clinical cases where opening of the BBB is necessary to enhance drug penetration into the brain (13) or when re-establishment of physiological BBB tightness is justified to treat brain diseases (248, 249). Emerging evidence supports a holistic approach to tackle CNS diseases, where neuronal and cerebrovascular contributors of diseases are synchronously targeted. An increasing number of BBB-repairing molecules are currently being tested [for review, see (5, 6)], targeting NVU cells and neuroinflammation. Importantly, BBB biomarker and repairing strategies could become important in the settings of acute or chronic peripheral diseases (infections, metabolic or inflammatory) where immunity and inflammation negatively impact BBB permeability and, consequentially, synaptic transmission (5, 7, 250).
In conclusion, the NVU represents a modern and integrated entry point for the investigations of brain functions, and a continuous technological advancement will be instrumental to improve our ability to link NVU damage with diagnostics. The field of biomarkers of NVU damage, or dysfunction, is expanding together with the use of omic techniques and machine-learning routines for the discovery of signatures of acute or chronic disease conditions.
Data Availability Statement
The original contributions presented in the study are included in the article/supplementary material, further inquiries can be directed to the corresponding author/s.
Author Contributions
NM coordinated this effort, generated most of the figures, table, and contributed or wrote all parts. DJ focused on salivary biomarkers and provided parts of figures. DB focused on extreme conditions and biomarkers and providing relevant figures. JB and NM focused on imaging. SL, RO'F, and CH focused on salivary biomarkers and revised applicability of biomarkers. All authors contributed to the article and approved the submitted version.
Funding
NM was supported by ANR-Hepatobrain, ANR-Epicyte, ERaNet Neu-Vasc, ANSES Epidemicmac, MUSE-iSite University of Montpellier. DB was supported by a Royal Society Wolfson Research Fellowship (#WM170007), Royal Society International Exchanges Award (IES/R2/192137), and Japan Society for the Promotion of Science Research Fellowship (#JSPS/OF317).
Conflict of Interest
DJ was affiliated to the company FloTBI Inc.
The remaining authors declare that the research was conducted in the absence of any commercial or financial relationships that could be construed as a potential conflict of interest.
References
1. Liebner S, Dijkhuizen RM, Reiss Y, Plate KH, Agalliu D, Constantin G. Functional morphology of the blood-brain barrier in health and disease. Acta Neuropathol. (2018) 135:311–36. doi: 10.1007/s00401-018-1815-1
2. Abbott NJ, Patabendige AA, Dolman DE, Yusof SR, Begley DJ. Structure and function of the blood-brain barrier. Neurobiol Dis. (2010) 37:13–25. doi: 10.1016/j.nbd.2009.07.030
3. Abbott NJ, Ronnback L, Hansson E. Astrocyte-endothelial interactions at the blood-brain barrier. Nat Rev Neurosci. (2006) 7:41–53. doi: 10.1038/nrn1824
4. Banks WA. From blood-brain barrier to blood-brain interface: new opportunities for CNS drug delivery. Nat Rev Drug Discov. (2016) 15:275–92. doi: 10.1038/nrd.2015.21
5. Giannoni P, Claeysen S, Noe F, Marchi N. Peripheral routes to neurodegeneration: passing through the blood-brain barrier. Front Aging Neurosci. (2020) 12:3. doi: 10.3389/fnagi.2020.00003
6. Sweeney MD, Zhao Z, Montagne A, Nelson AR, Zlokovic BV. Blood-brain barrier: from physiology to disease and back. Physiol Rev. (2019) 99:21–78. doi: 10.1152/physrev.00050.2017
7. Marchi N, Granata T, Janigro D. Inflammatory pathways of seizure disorders. Trends Neurosci. (2014) 37:55–65. doi: 10.1016/j.tins.2013.11.002
8. Obermeier B, Verma A, Ransohoff RM. The blood-brain barrier. Handb Clin Neurol. (2016) 133:39–59. doi: 10.1016/B978-0-444-63432-0.00003-7
9. Arango-Lievano M, Boussadia B, De Terdonck LDT, Gault C, Fontanaud P, Lafont C, et al. Topographic reorganization of cerebrovascular mural cells under seizure conditions. Cell Rep. (2018) 23:1045–59. doi: 10.1016/j.celrep.2018.03.110
10. Giannoni P, Badaut J, Dargazanli C, De Maudave AF, Klement W, Costalat V, et al. The pericyte-glia interface at the blood-brain barrier. Clin Sci (Lond). (2018) 132:361–74. doi: 10.1042/CS20171634
11. Librizzi L, de Cutis M, Janigro D, Runtz L, de Bock F, Barbier EL, et al. Cerebrovascular heterogeneity and neuronal excitability. Neurosci Lett. (2018) 667:75–83. doi: 10.1016/j.neulet.2017.01.013
12. Pollak TA, Drndarski S, Stone JM, David AS, McGuire P, Abbott NJ. The blood-brain barrier in psychosis. Lancet Psychiatry. (2018) 5:79–92. doi: 10.1016/S2215-0366(17)30293-6
13. Sweeney MD, Sagare AP, Zlokovic BV. Blood-brain barrier breakdown in Alzheimer disease and other neurodegenerative disorders. Nat Rev Neurol. (2018) 14:133–50. doi: 10.1038/nrneurol.2017.188
14. Marchi N, Hallene KL, Kight KM, Cucullo L, Moddel G, Bingaman W, et al. Significance of MDR1 and multiple drug resistance in refractory human epileptic brain. BMC Med. (2004) 2:37. doi: 10.1186/1741-7015-2-37
15. Pulido RS, Munji RN, Chan TC, Quirk CR, Weiner GA, Weger BD, et al. Neuronal activity regulates blood-brain barrier efflux transport through endothelial circadian genes. Neuron. (2020) 108:937–52.e937. doi: 10.1016/j.neuron.2020.09.002
16. Diaz-Arrastia R, Wang KK, Papa L, Sorani MD, Yue JK, Puccio AM, et al. Acute biomarkers of traumatic brain injury: relationship between plasma levels of ubiquitin C-terminal hydrolase-L1 and glial fibrillary acidic protein. J Neurotrauma. (2014) 31:19–25. doi: 10.1089/neu.2013.3040
17. Wang KK, Yang Z, Zhu T, Shi Y, Rubenstein R, Tyndall JA, et al. An update on diagnostic and prognostic biomarkers for traumatic brain injury. Expert Rev Mol Diagn. (2018) 18:165–80. doi: 10.1080/14737159.2018.1428089
18. Kawata K, Liu CY, Merkel SF, Ramirez SH, Tierney RT, Langford D. Blood biomarkers for brain injury: what are we measuring? Neurosci Biobehav Rev. (2016) 68:460–73. doi: 10.1016/j.neubiorev.2016.05.009
19. Mondello S, Kobeissy F, Vestri A, Hayes RL, Kochanek PM, Berger RP. Serum concentrations of ubiquitin C-terminal hydrolase-L1 and glial fibrillary acidic protein after pediatric traumatic brain Injury. Sci Rep. (2016) 6:28203. doi: 10.1038/srep28203
20. Posti JP, Hossain I, Takala RS, Liedes H, Newcombe V, Outtrim J, et al. Glial fibrillary acidic protein and ubiquitin c-terminal hydrolase-l1 are not specific biomarkers for Mild CT-negative traumatic brain injury. J Neurotrauma. (2017). doi: 10.1089/neu.2016.4442
21. Bazarian JJ, Biberthaler P, Welch RD, Lewis LM, Barzo P, Bogner-Flatz V, et al. Serum GFAP and UCH-L1 for prediction of absence of intracranial injuries on head CT (ALERT-TBI): a multicentre observational study. Lancet Neurol. (2018) 17:782–9. doi: 10.1016/S1474-4422(18)30231-X
22. Thelin EP, Nelson DW, Bellander B-M. A review of the clinical utility of serum S100B protein levels in the assessment of traumatic brain injury. Acta Neurochirurgica. (2017) 159:209–25. doi: 10.1007/s00701-016-3046-3
23. Pham N, Fazio V, Cucullo L, Teng Q, Biberthaler P, Bazarian JJ, et al. sources of S100B do not affect serum levels. PLoS ONE. (2010) 5:2010. doi: 10.1371/annotation/bdcb41f2-a320-4401-a6ab-86e71738597e
24. Shahim P, Tegner Y, Wilson DH, Randall J, Skillback T, Pazooki D, et al. Blood biomarkers for brain injury in concussed professional ice hockey players. JAMA Neurol. (2014) 71:684–92. doi: 10.1001/jamaneurol.2014.367
25. Marchi N, Bazarian JJ, Puvenna V, Janigro M, Ghosh C, Zhong J, et al. Consequences of repeated blood-brain barrier disruption in football players. PLoS ONE. (2013) 8:e56805. doi: 10.1371/journal.pone.0056805
26. Kiechle K, Bazarian JJ, Merchant-Borna K, Stoecklein V, Rozen E, Blyth B, et al. Subject-specific increases in serum S-100B distinguish sports-related concussion from sports-related exertion. PLoS ONE. (2014) 9:e84977. doi: 10.1371/journal.pone.0084977
27. Marchi N, Rasmussen P, Kapural M, Fazio V, Kight K, Mayberg MR, et al. Peripheral markers of brain damage and blood-brain barrier dysfunction. Restor Neurol Neurosci. (2003) 21:109–21.
28. Marchi N, Cavaglia M, Fazio V, Bhudia S, Hallene K, Janigro D. Peripheral markers of blood-brain barrier damage. Clin Chim Acta. (2004) 342:1–12. doi: 10.1016/j.cccn.2003.12.008
29. Dadas A, Washington J, Marchi N, Janigro D. Improving the clinical management of traumatic brain injury through the pharmacokinetic modeling of peripheral blood biomarkers. Fluids Barriers CNS. (2016) 13:21. doi: 10.1186/s12987-016-0045-y
30. Bishop P, Rocca D, Henley JM. Ubiquitin C-terminal hydrolase L1 (UCH-L1): structure, distribution and roles in brain function and dysfunction. Biochem J. (2016) 473:2453–62. doi: 10.1042/BCJ20160082
31. Brophy GM, Mondello S, Papa L, Robicsek SA, Gabrielli A, Tepas III J, et al. Biokinetic analysis of ubiquitin C-terminal hydrolase-L1 (UCH-L1) in severe traumatic brain injury patient biofluids. J Neurotr. (2011) 28:861–70. doi: 10.1089/neu.2010.1564
32. Rundgren M, Cronberg T, Friberg H, Isaksson A. Serum neuron specific enolase–impact of storage and measuring method. BMC Research Notes. (2014) 7:726. doi: 10.1186/1756-0500-7-726
33. Bogoslovsky T, Gill J, Jeromin A, Davis C, Diaz-Arrastia R. Fluid biomarkers of traumatic brain injury and intended context of use. Diagnostics (Basel). (2016) 6. doi: 10.3390/diagnostics6040037
34. Gillick K, Rooney K. Serial NSE measurement identifies non-survivors following out of hospital cardiac arrest. Resuscitation. (2018) 128:24–30. doi: 10.1016/j.resuscitation.2018.04.010
35. Shahim P, Zetterberg H, Tegner Y, Blennow K. Serum neurofilament light as a biomarker for mild traumatic brain injury in contact sports. Neurology. (2017) 88:1788–94. doi: 10.1212/WNL.0000000000003912
36. Gresle MM, Butzkueven H, Shaw G. Neurofilament proteins as body fluid biomarkers of neurodegeneration in multiple sclerosis. Mult Scler Int. (2011) 2011:315406. doi: 10.1155/2011/315406
37. Yuan A, Rao MV, Veeranna Nixon RA. Neurofilaments and Neurofilament Proteins in Health and Disease. Cold Spring Harb Perspect Biol. (2017) 9. doi: 10.1101/cshperspect.a018309
38. Lee Y, Lee BH, Yip W, Chou P, Yip BS. Neurofilament proteins as prognostic biomarkers in neurological disorders. Curr Pharm Des. (2020) 25:4560–9. doi: 10.2174/1381612825666191210154535
39. Nation DA, Sweeney MD, Montagne A, Sagare AP, D'Orazio LM, Pachicano M, et al. Blood-brain barrier breakdown is an early biomarker of human cognitive dysfunction. Nat Med. (2019) 25:270–6. doi: 10.1038/s41591-018-0297-y
40. Montagne A, Barnes SR, Sweeney MD, Halliday MR, Sagare AP, Zhao Z, et al. Blood-brain barrier breakdown in the aging human hippocampus. Neuron. (2015) 85:296–302. doi: 10.1016/j.neuron.2014.12.032
41. Randall J, Mörtberg E, Provuncher GK, Fournier DR, Duffy DC, Rubertsson S, et al. Tau proteins in serum predict neurological outcome after hypoxic brain injury from cardiac arrest: results of a pilot study. Resuscitation. (2013) 84:351–6. doi: 10.1016/j.resuscitation.2012.07.027
42. Rubenstein R, Chang B, Yue JK, Chiu A, Winkler EA, Puccio AM, et al. Comparing plasma phospho tau, total tau, and phospho tau-total tau ratio as acute and chronic traumatic brain injury biomarkers. JAMA Neurol. (2017) 74:1063–72. doi: 10.1001/jamaneurol.2017.0655
43. Dickstein DL, De Gasperi R, Gama Sosa MA, Perez-Garcia G, Short JA, Sosa H, et al. Brain and blood biomarkers of tauopathy and neuronal injury in humans and rats with neurobehavioral syndromes following blast exposure. Mol Psychiatry. (2020) doi: 10.1038/s41380-020-0674-z
44. Bhomia M, Balakathiresan NS, Wang KK, Papa L, Maheshwari RK. A Panel of Serum MiRNA Biomarkers for the Diagnosis of Severe to Mild Traumatic Brain Injury in Humans. Sci Rep. (2016) 6:28148. doi: 10.1038/srep28148
45. Papa L, Slobounov SM, Breiter HC, Walter A, Bream T, Seidenberg P, et al. Elevations in MicroRNA biomarkers in serum are associated with measures of concussion, neurocognitive function, and subconcussive trauma over a single national collegiate athletic association division i season in collegiate football players. J Neurotrauma. (2019) 36:1343–51. doi: 10.1089/neu.2018.6072
46. Zhang J, Puvenna V, Janigro D. Biomarkers of traumatic brain injury and their relationship to pathology. In: Laskowitz D, Grant G, editors. Translational Research in Traumatic Brain Injury. Boca Raton, FL: CRC Press/Taylor and Francis Group (2016).
47. Abbott NJ, Pizzo ME, Preston JE, Janigro D, Thorne RG. The role of brain barriers in fluid movement in the CNS: is there a 'glymphatic' system? Acta Neuropathol. (2018) 135:387–407. doi: 10.1007/s00401-018-1812-4
48. Hladky SB, Barrand MA. Mechanisms of fluid movement into, through and out of the brain: evaluation of the evidence. Fluids Barriers CNS. (2014) 11:26. doi: 10.1186/2045-8118-11-26
49. Nicholson C, Hrabetova S. Brain extracellular space: the final frontier of neuroscience. Biophys J. (2017) 113:2133–42. doi: 10.1016/j.bpj.2017.06.052
50. Plog BA, Nedergaard M. The glymphatic system in central nervous system health and disease: past, present, and future. Annu Rev Pathol. (2018) 13:379–94. doi: 10.1146/annurev-pathol-051217-111018
51. Lindblad C, Nelson DW, Zeiler FA, Ercole A, Ghatan PH, von Horn H, et al. Influence of blood-brain barrier integrity on brain protein biomarker clearance in severe traumatic brain injury: a longitudinal prospective study. J Neurotrauma. (2020) 37:1381–91. doi: 10.1089/neu.2019.6741
52. Noe FM, Marchi N. Central nervous system lymphatic unit, immunity, and epilepsy: is there a link? Epilepsia Open. (2019) 4:30–9.
53. Dadas A, Washington J, Diaz-Arrastia R, Janigro D. Biomarkers in traumatic brain injury (TBI): a review. Neuropsychiatr Dis Treat. (2018) 14:2989–3000. doi: 10.2147/NDT.S125620
54. Kim HJ, Tsao JW, Stanfill AG. The current state of biomarkers of mild traumatic brain injury. JCI Insight. (2018) 3. doi: 10.1172/jci.insight.97105
55. Donato R, Cannon BR, Sorci G, Riuzzi F, Hsu K, Weber DJ, et al. Functions of S100 proteins. Curr Mol Med. (2013) 13:24–57. doi: 10.2174/156652413804486214
56. Marchi N, Fazio V, Cucullo L, Kight K, Masaryk T, Barnett G, et al. Serum transthyretin monomer as a possible marker of blood-to-CSF barrier disruption. J Neurosci. (2003) 23:1949–55. doi: 10.1523/JNEUROSCI.23-05-01949.2003
57. Zongo D, Ribereau-Gayon R, Masson F, Laborey M, Contrand B, Salmi LR, et al. S100-B protein as a screening tool for the early assessment of minor head injury. Ann Emerg Med. (2012) 59:209–18. doi: 10.1016/j.annemergmed.2011.07.027
58. Ananthaharan A, Kravdal G, Straume-Naesheim TM. Utility and effectiveness of the Scandinavian guidelines to exclude computerized tomography scanning in mild traumatic brain injury - a prospective cohort study. BMC Emerg Med. (2018) 18:44. doi: 10.1186/s12873-018-0193-2
59. Unden J, Ingebrigtsen T, Romner B. Scandinavian guidelines for initial management of minimal, mild and moderate head injuries in adults: an evidence and consensus-based update. BMC Med. (2013) 11:50. doi: 10.1186/1741-7015-11-50
60. Unden L, Calcagnile O, Unden J, Reinstrup P, Bazarian J. Validation of the Scandinavian guidelines for initial management of minimal, mild and moderate traumatic brain injury in adults. BMC Med. (2015) 13:292. doi: 10.1186/s12916-015-0533-y
61. Jones CMC, Harmon C, McCann M, Gunyan H, Bazarian JJ. S100B outperforms clinical decision rules for the identification of intracranial injury on head CT scan after mild traumatic brain injury. Brain Inj. (2020) 34:407–14. doi: 10.1080/02699052.2020.1725123
62. Metting Z, Wilczak N, Rodiger LA, Schaaf JM, van der Naalt J. GFAP and S100B in the acute phase of mild traumatic brain injury. Neurology. (2012) 78:1428–33. doi: 10.1212/WNL.0b013e318253d5c7
63. Kawata K, Rubin LH, Takahagi M, Lee JH, Sim T, Szwanki V, et al. Subconcussive impact-dependent increase in plasma s100beta levels in collegiate football players. J Neurotrauma. (2017) 34:2254–60. doi: 10.1089/neu.2016.4786
64. Meier TB, Huber DL, Bohorquez-Montoya L, Nitta ME, Savitz J, Teague TK, et al. A prospective study of acute blood-based biomarkers for sport-related concussion. Ann Neurol. (2020) 87:907–20. doi: 10.1002/ana.25725
65. Zonner SW, Ejima K, Bevilacqua ZW, Huibregtse ME, Charleston C, Fulgar C, et al. Association of increased serum s100b levels with high school football subconcussive head impacts. Front Neurol. (2019) 10:327. doi: 10.3389/fneur.2019.00327
66. Neher MD, Keene CN, Rich MC, Moore HB, Stahel PF. Serum biomarkers for traumatic brain injury. South Med J. (2014) 107:248–55. doi: 10.1097/SMJ.0000000000000086
67. Bargerstock E, Puvenna V, Iffland P, Falcone T, Hossain M, Vetter S, et al. Is peripheral immunity regulated by blood-brain barrier permeability changes? PLoS ONE. (2014) 9:e101477. doi: 10.1371/journal.pone.0101477
68. Fazio V, Bhudia SK, Marchi N, Aumayr B, Janigro D. Peripheral detection of S100beta during cardiothoracic surgery: what are we really measuring? Ann Thorac Surg. (2004) 78:46–52. doi: 10.1016/j.athoracsur.2003.11.042
69. Chen F, Sugiura Y, Myers KG, Liu Y, Lin W. Ubiquitin carboxyl-terminal hydrolase L1 is required for maintaining the structure and function of the neuromuscular junction. Proc Natl Acad Sci USA. (2010) 107:1636–41. doi: 10.1073/pnas.0911516107
70. Jeter CB, Hergenroeder GW, Hylin MJ, Redell JB, Moore AN, Dash PK. Biomarkers for the diagnosis and prognosis of mild traumatic brain injury/concussion. J Neurotrauma. (2013) 30:657–70. doi: 10.1089/neu.2012.2439
71. McMahon PJ, Panczykowski DM, Yue JK, Puccio AM, Inoue T, Sorani MD, et al. Measurement of the glial fibrillary acidic protein and its breakdown products GFAP-BDP biomarker for the detection of traumatic brain injury compared to computed tomography and magnetic resonance imaging. J Neurotrauma. (2015) 32:527–33. doi: 10.1089/neu.2014.3635
72. Bogoslovsky T, Wilson D, Chen Y, Hanlon D, Gill J, Jeromin A, et al. Increases of plasma levels of glial fibrillary acidic protein, tau, and amyloid beta up to 90 days after traumatic brain injury. J Neurotrauma. (2017) 34:66–73. doi: 10.1089/neu.2015.4333
73. Papa L, Zonfrillo MR, Welch RD, Lewis LM, Braga CF, Tan CN, et al. Evaluating glial and neuronal blood biomarkers GFAP and UCH-L1 as gradients of brain injury in concussive, subconcussive and non-concussive trauma: a prospective cohort study. BMJ Paediatr Open. (2019) 3:e000473. doi: 10.1136/bmjpo-2019-000473
74. Papa L, Lewis LM, Silvestri S, Falk JL, Giordano P, Brophy GM, et al. Serum levels of ubiquitin C-terminal hydrolase distinguish mild traumatic brain injury from trauma controls and are elevated in mild and moderate traumatic brain injury patients with intracranial lesions and neurosurgical intervention. J Trauma Acute Care Surg. (2012) 72:1335–44. doi: 10.1097/TA.0b013e3182491e3d
75. Yue JK, Yuh EL, Korley FK, Winkler EA, Sun X, Puffer RC, et al. Association between plasma GFAP concentrations and MRI abnormalities in patients with CT-negative traumatic brain injury in the TRACK-TBI cohort: a prospective multicentre study. Lancet Neurol. (2019) 18:953–61. doi: 10.1016/S1474-4422(19)30282-0
76. Linsenmaier U, Wirth S, Kanz KG, Geyer LL. Imaging minor head injury (MHI) in emergency radiology: MRI highlights additional intracranial findings after measurement of trauma biomarker S-100B in patients with normal CCT. Br J Radiol. (2016) 89:20150827. doi: 10.1259/bjr.20150827
77. Puvenna V, Brennan C, Shaw G, Yang C, Marchi N, Bazarian JJ, et al. Significance of ubiquitin carboxy-terminal hydrolase L1 elevations in athletes after sub-concussive head hits. PLoS ONE. (2014) 9:e96296. doi: 10.1371/journal.pone.0096296
78. Papa L, Brophy GM, Welch RD, Lewis LM, Braga CF, Tan CN, et al. Time course and diagnostic accuracy of glial and neuronal blood biomarkers GFAP and UCH-L1 in a large cohort of trauma patients with and without mild traumatic brain injury. JAMA Neurol. (2016) 73:551–60. doi: 10.1001/jamaneurol.2016.0039
79. Welch RD, Ellis M, Lewis LM, Ayaz SI, Mika VH, Millis SR, et al. Modeling the kinetics of serum glial fibrillary acidic protein, ubiquitin carboxyl-terminal hydrolase-L1, and S100B concentrations in patients with traumatic brain injury. J Neurotrauma. (2016) doi: 10.1089/neu.2015.4149
80. Maas AIR, Lingsma HF. ALERT-TBI study on biomarkers for TBI: has science suffered? Lancet Neurol. (2018) 17:737–8. doi: 10.1016/S1474-4422(18)30275-8
81. Berger RP, Adelson PD, Pierce MC, Dulani T, Cassidy LD, Kochanek PM. Serum neuron-specific enolase, S100B, and myelin basic protein concentrations after inflicted and noninflicted traumatic brain injury in children. J Neurosurg. (2005) 103:61–8. doi: 10.3171/ped.2005.103.1.0061
82. Gatson JW, Barillas J, Hynan LS, Diaz-Arrastia R, Wolf SE, Minei JP. Detection of neurofilament-H in serum as a diagnostic tool to predict injury severity in patients who have suffered mild traumatic brain injury. J Neurosurg. (2014) 121:1232–8. doi: 10.3171/2014.7.JNS132474
83. Johnson VE, Stewart W, Smith DH. Axonal pathology in traumatic brain injury. Exp Neurol. (2013) 246:35–43. doi: 10.1016/j.expneurol.2012.01.013
84. Neselius S, Zetterberg H, Blennow K, Marcusson J, Brisby H. Increased CSF levels of phosphorylated neurofilament heavy protein following bout in amateur boxers. PLoS ONE. (2013) 8:e81249. doi: 10.1371/journal.pone.0081249
85. Otto M, Holthusen S, Bahn E, Sohnchen N, Wiltfang J, Geese R, et al. Boxing and running lead to a rise in serum levels of S-100B protein. Int J Sports Med. (2000) 21:551–5. doi: 10.1055/s-2000-8480
86. Bohmer AE, Oses JP, Schmidt AP, Peron CS, Krebs CL, Oppitz PP, et al. Neuron-specific enolase, S100B, and glial fibrillary acidic protein levels as outcome predictors in patients with severe traumatic brain injury. Neurosurgery. (2011) 68:1624–30; discussion 1630-1621. doi: 10.1227/NEU.0b013e318214a81f
87. Chiaretti A, Barone G, Riccardi R, Antonelli A, Pezzotti P, Genovese O, et al. NGF, DCX, and NSE upregulation correlates with severity and outcome of head trauma in children. Neurology. (2009) 72:609–16. doi: 10.1212/01.wnl.0000342462.51073.06
88. Ross SA, Cunningham RT, Johnston CF, Rowlands BJ. Neuron-specific enolase as an aid to outcome prediction in head injury. Br J Neurosurg. (1996) 10:471–6. doi: 10.1080/02688699647104
89. Olsson B, Zetterberg H, Hampel H, Blennow K. Biomarker-based dissection of neurodegenerative diseases. Prog Neurobiol. (2011) 95:520–34. doi: 10.1016/j.pneurobio.2011.04.006
90. Ramont L, Thoannes H, Volondat A, Chastang F, Millet MC, Maquart FX. Effects of hemolysis and storage condition on neuron-specific enolase (NSE) in cerebrospinal fluid and serum: implications in clinical practice. Clin Chem Lab Med. (2005) 43:1215–7. doi: 10.1515/CCLM.2005.210
91. Barthelemy NR, Horie K, Sato C, Bateman RJ. Blood plasma phosphorylated-tau isoforms track CNS change in Alzheimer's disease. J Exp Med. (2020) 217. doi: 10.1084/jem.20200861
92. O'Connor A, Karikari TK, Poole T, Ashton NJ, Lantero Rodriguez J, Khatun A, et al. Plasma phospho-tau181 in presymptomatic and symptomatic familial Alzheimer's disease: a longitudinal cohort study. Mol Psychiatry. (2020) doi: 10.1038/s41380-020-0838-x
93. Palmqvist S, Janelidze S, Quiroz YT, Zetterberg H, Lopera F, Stomrud E, et al. Discriminative accuracy of plasma phospho-tau217 for alzheimer disease vs other neurodegenerative disorders. JAMA. (2020) 324:772–81. doi: 10.1001/jama.2020.12134
94. Karikari TK, Pascoal TA, Ashton NJ, Janelidze S, Benedet AL, Rodriguez JL, et al. Blood phosphorylated tau 181 as a biomarker for Alzheimer's disease: a diagnostic performance and prediction modelling study using data from four prospective cohorts. Lancet Neurol. (2020) 19:422–33. doi: 10.1016/S1474-4422(20)30071-5
95. Polavarapu R, Gongora MC, Yi H, Ranganthan S, Lawrence DA, Strickland D, et al. Tissue-type plasminogen activator-mediated shedding of astrocytic low-density lipoprotein receptor-related protein increases the permeability of the neurovascular unit. Blood. (2007) 109:3270–8. doi: 10.1182/blood-2006-08-043125
96. Sagare A, Deane R, Bell RD, Johnson B, Hamm K, Pendu R, et al. Clearance of amyloid-beta by circulating lipoprotein receptors. Nat Med. (2007) 13:1029–31. doi: 10.1038/nm1635
97. Sagare AP, Deane R, Zetterberg H, Wallin A, Blennow K, Zlokovic BV. Impaired lipoprotein receptor-mediated peripheral binding of plasma amyloid-beta is an early biomarker for mild cognitive impairment preceding Alzheimer's disease. J Alzheimers Dis. (2011) 24:25–34. doi: 10.3233/JAD-2010-101248
98. Friedman A, Kaufer D. Blood-brain barrier breakdown and blood-brain communication in neurological and psychiatric diseases. Cardiovasc Psychiatry Neurol. (2011) 2011:431470. doi: 10.1155/2011/431470
99. Klement W, Blaquiere M, Zub E, deBock F, Boux F, Barbier E, et al. A pericyte-glia scarring develops at the leaky capillaries in the hippocampus during seizure activity. Epilepsia. (2019) 60:1399–1411. doi: 10.1111/epi.16019
100. Marchi N, Tierney W, Alexopoulos AV, Puvenna V, Granata T, Janigro D. The etiological role of blood-brain barrier dysfunction in seizure disorders. Cardiovasc Psychiatry Neurol. (2011) 2011:482415. doi: 10.1155/2011/482415
101. Oby E, Janigro D. The blood-brain barrier and epilepsy. Epilepsia. (2006) 47:1761–74. doi: 10.1111/j.1528-1167.2006.00817.x
102. Marchi N, Angelov L, Masaryk T, Fazio V, Granata T, Hernandez N, et al. Seizure-promoting effect of blood-brain barrier disruption. Epilepsia. (2007) 48:732–42. doi: 10.1111/j.1528-1167.2007.00988.x
103. Marchi N, Granata T, Ghosh C, Janigro D. Blood-brain barrier dysfunction and epilepsy: pathophysiologic role and therapeutic approaches. Epilepsia. (2012) 53:1877–86. doi: 10.1111/j.1528-1167.2012.03637.x
104. Liang KG, Mu RZ, Liu Y, Jiang D, Jia TT, Huang YJ. Increased serum S100B levels in patients with epilepsy: a systematic review and meta-analysis study. Front Neurosci. (2019) 13:456. doi: 10.3389/fnins.2019.00456
105. Ruber T, David B, Luchters G, Nass RD, Friedman A, Surges R, et al. Evidence for peri-ictal blood-brain barrier dysfunction in patients with epilepsy. Brain. (2018) 141:2952–65. doi: 10.1093/brain/awy242
106. Calik M, Abuhandan M, Sonmezler A, Kandemir H, Oz I, Taskin A, et al. Elevated serum S-100B levels in children with temporal lobe epilepsy. Seizure. (2013) 22:99–102. doi: 10.1016/j.seizure.2012.10.012
107. Calik M, Abuhandan M, Kandemir H, Guzel B, Solmaz A, Celik H, et al. Interictal serum S-100B protein levels in intractable epilepsy: a case-control study. Neurosci Lett. (2014) 558:58–61. doi: 10.1016/j.neulet.2013.10.040
108. Atici Y, Alehan F, Sezer T, Tuygun N, Haberal A, Yazici AC, et al. Serum S100B levels in children with simple febrile seizures. Seizure. (2012) 21:175–7. doi: 10.1016/j.seizure.2011.11.003
109. Mikkonen K, Pekkala N, Pokka T, Romner B, Uhari M, Rantala H. S100B proteins in febrile seizures. Seizure. (2012) 21:144–6. doi: 10.1016/j.seizure.2011.10.006
110. Palmio J, Keranen T, Alapirtti T, Hulkkonen J, Makinen R, Holm P, et al. Elevated serum neuron-specific enolase in patients with temporal lobe epilepsy: a video-EEG study. Epilepsy Res. (2008) 81:155–60. doi: 10.1016/j.eplepsyres.2008.05.006
111. Enright N, Simonato M, Henshall DC. Discovery and validation of blood microRNAs as molecular biomarkers of epilepsy: Ways to close current knowledge gaps. Epilepsia Open. (2018) 3:427–36. doi: 10.1002/epi4.12275
112. Henshall DC. MicroRNA and epilepsy: profiling, functions and potential clinical applications. Curr Opin Neurol. (2014) 27:199–205. doi: 10.1097/WCO.0000000000000079
113. Henshall DC, Hamer HM, Pasterkamp RJ, Goldstein DB, Kjems J, Prehn JHM, et al. MicroRNAs in epilepsy: pathophysiology and clinical utility. Lancet Neurol. (2016) 15:1368–76. doi: 10.1016/S1474-4422(16)30246-0
114. Korfias S, Stranjalis G, Papadimitriou A, Psachoulia C, Daskalakis G, Antsaklis A, et al. Serum S-100B protein as a biochemical marker of brain injury: a review of current concepts. Curr Med Chem. (2006) 13:3719–31. doi: 10.2174/092986706779026129
115. Kanner AA, Marchi N, Fazio V, Mayberg MR, Koltz MT, Siomin V, et al. Serum S100beta: a noninvasive marker of blood-brain barrier function and brain lesions. Cancer. (2003) 97:2806–13. doi: 10.1002/cncr.11409
116. Nylen K, Ost M, Csajbok LZ, Nilsson I, Hall C, Blennow K, et al. (Serum levels of S100B, S100A1B and S100BB are all related to outcome after severe traumatic brain injury. Acta Neurochir (Wien). 2008) 150:221–227; discussion 227. doi: 10.1007/s00701-007-1489-2
117. Vogelbaum MA, Masaryk T, Mazzone P, Mekhail T, Fazio V, McCartney S, et al. S100beta as a predictor of brain metastases: brain versus cerebrovascular damage. Cancer. (2005) 104:817–24. doi: 10.1002/cncr.21220
118. Ruan S, Noyes K, Bazarian JJ. The economic impact of S-100B as a pre-head CT screening test on emergency department management of adult patients with mild traumatic brain injury. J Neurotrauma. (2009) 26:1655–64. doi: 10.1089/neu.2009.0928
119. Unden J, Romner B. Can low serum levels of S100B predict normal CT findings after minor head injury in adults?: an evidence-based review and meta-analysis. J Head Trauma Rehabil. (2010) 25:228–40. doi: 10.1097/HTR.0b013e3181e57e22
120. McGinn MJ, Povlishock JT. Pathophysiology of traumatic brain injury. Neurosurg Clin N Am. (2016) 27:397–407. doi: 10.1016/j.nec.2016.06.002
121. Prins M, Greco T, Alexander D, Giza CC. The pathophysiology of traumatic brain injury at a glance. Dis Model Mech. (2013) 6:1307–15. doi: 10.1242/dmm.011585
122. Steyerberg EW, Wiegers E, Sewalt C, Buki A, Citerio G, De Keyser V, et al. Case-mix, care pathways, and outcomes in patients with traumatic brain injury in CENTER-TBI: a European prospective, multicentre, longitudinal, cohort study. Lancet Neurol. (2019) 18:923–34. doi: 10.1016/S1474-4422(19)30232-7
123. Eierud C, Craddock RC, Fletcher S, Aulakh M, King-Casas B, Kuehl D, et al. Neuroimaging after mild traumatic brain injury: review and meta-analysis. Neuroimage Clin. (2014) 4:283–94. doi: 10.1016/j.nicl.2013.12.009
124. Gill J, Latour L, Diaz-Arrastia R, Motamedi V, Turtzo C, Shahim P, et al. Glial fibrillary acidic protein elevations relate to neuroimaging abnormalities after mild TBI. Neurology. (2018) 91:e1385–9. doi: 10.1212/WNL.0000000000006321
125. Thompson WH, Thelin EP, Lilja A, Bellander BM, Fransson P. Functional resting-state fMRI connectivity correlates with serum levels of the S100B protein in the acute phase of traumatic brain injury. Neuroimage Clin. (2016) 12:1004–12. doi: 10.1016/j.nicl.2016.05.005
126. Edwards KA, Pattinson CL, Guedes VA, Peyer J, Moore C, Davis T, et al. Inflammatory cytokines associate with neuroimaging after acute mild traumatic brain injury. Front Neurol. (2020) 11:348. doi: 10.3389/fneur.2020.00348
127. Gozt A, Licari M, Halstrom A, Milbourn H, Lydiard S, Black A, et al. Towards the development of an integrative, evidence-based suite of indicators for the prediction of outcome following mild traumatic brain injury: results from a pilot study. Brain Sci. (2020) 10. doi: 10.3390/brainsci10010023
128. Harris JL, Yeh HW, Choi IY, Lee P, Berman NE, Swerdlow RH, et al. Altered neurochemical profile after traumatic brain injury: (1)H-MRS biomarkers of pathological mechanisms. J Cereb Blood Flow Metab. (2012) 32:2122–34. doi: 10.1038/jcbfm.2012.114
129. Xu S, Zhuo J, Racz J, Shi D, Roys S, Fiskum G, et al. Early microstructural and metabolic changes following controlled cortical impact injury in rat: a magnetic resonance imaging and spectroscopy study. J Neurotrauma. (2011) 28:2091–102. doi: 10.1089/neu.2010.1739
130. Holshouser B, Pivonka-Jones J, Nichols JG, Oyoyo U, Tong K, Ghosh N, et al. Longitudinal metabolite changes after traumatic brain injury: a prospective pediatric magnetic resonance spectroscopic imaging study. J Neurotrauma. (2019) 36:1352–60. doi: 10.1089/neu.2018.5919
131. Harris JL, Choi IY, Brooks WM. Probing astrocyte metabolism in vivo: proton magnetic resonance spectroscopy in the injured and aging brain. Front Aging Neurosci. (2015) 7:202. doi: 10.3389/fnagi.2015.00202
132. Budde MD, Janes L, Gold E, Turtzo LC, Frank JA. The contribution of gliosis to diffusion tensor anisotropy and tractography following traumatic brain injury: validation in the rat using Fourier analysis of stained tissue sections. Brain. (2011) 134:2248–60. doi: 10.1093/brain/awr161
133. Rodriguez-Grande B, Obenaus A, Ichkova A, Aussudre J, Bessy T, Barse E, et al. Gliovascular changes precede white matter damage and long-term disorders in juvenile mild closed head injury. Glia. (2018) doi: 10.1002/glia.23336
134. Wright DK, Trezise J, Kamnaksh A, Bekdash R, Johnston LA, Ordidge R, et al. Behavioral, blood, and magnetic resonance imaging biomarkers of experimental mild traumatic brain injury. Sci Rep. (2016) 6:28713. doi: 10.1038/srep28713
135. Huang M, Risling M, Baker DG. The role of biomarkers and MEG-based imaging markers in the diagnosis of post-traumatic stress disorder and blast-induced mild traumatic brain injury. Psychoneuroendocrinology. (2016) 63:398–409. doi: 10.1016/j.psyneuen.2015.02.008
136. World malaria situation. Division of control of tropical diseases. World Health Stat Q. (1990) 43:68–79.
137. Aqrawi LA, Galtung HK, Vestad B, Ovstebo R, Thiede B, Rusthen S, et al. Identification of potential saliva and tear biomarkers in primary Sjogren's syndrome, utilising the extraction of extracellular vesicles and proteomics analysis. Arthritis Res Ther. (2017) 19:14. doi: 10.1186/s13075-017-1228-x
138. Hirtz C, Chevalier F, Centeno D, Egea JC, Rossignol M, Sommerer N, et al. Complexity of the human whole saliva proteome. J Physiol Biochem. (2005) 61:469–80. doi: 10.1007/BF03168453
139. Hirtz C, Vialaret J, Nowak N, Gabelle A, Deville de Periere D, Lehmann S. Absolute quantification of 35 plasma biomarkers in human saliva using targeted MS. Bioanalysis. (2016) 8:43–53. doi: 10.4155/bio.15.228
140. Kaczor-Urbanowicz KE, Kim Y, Li F, Galeev T, Kitchen RR, Gerstein M, et al. Novel approaches for bioinformatic analysis of salivary RNA sequencing data for development. Bioinformatics. (2018) 34:1–8. doi: 10.1093/bioinformatics/btx504
141. Kaczor-Urbanowicz KE, Martin Carreras-Presas C, Aro K, Tu M, Garcia-Godoy F, Wong DT. Saliva diagnostics - current views and directions. Exp Biol Med (Maywood). (2017) 242:459–72. doi: 10.1177/1535370216681550
142. Malon RS, Sadir S, Balakrishnan M, Corcoles EP. Saliva-based biosensors: noninvasive monitoring tool for clinical diagnostics. Biomed Res Int. (2014) 2014:962903. doi: 10.1155/2014/962903
143. Patel N, Belcher J, Thorpe G, Forsyth NR, Spiteri MA. Measurement of C-reactive protein, procalcitonin and neutrophil elastase in saliva of COPD patients and healthy controls: correlation to self-reported wellbeing parameters. Respir Res. (2015) 16:62. doi: 10.1186/s12931-015-0219-1
144. Pay JB, Shaw AM. Towards salivary C-reactive protein as a viable biomarker of systemic inflammation. Clin Biochem. (2019) 68:1–8. doi: 10.1016/j.clinbiochem.2019.04.006
145. Loo JA, Yan W, Ramachandran P, Wong DT. Comparative human salivary and plasma proteomes. J Dent Res. (2010) 89:1016–23. doi: 10.1177/0022034510380414
146. Dezayee ZM, Al-Nimer MS. Saliva C-reactive protein as a biomarker of metabolic syndrome in diabetic patients. Indian J Dent Res. (2016) 27:388–91. doi: 10.4103/0970-9290.191887
147. Humphrey SP, Williamson RT. A review of saliva: normal composition, flow, and function. J Prosthet Dent. (2001) 85:162–9. doi: 10.1067/mpr.2001.113778
148. Humberto JSM, Pavanin JV, Rocha M, Motta ACF. Cytokines, cortisol, and nitric oxide as salivary biomarkers in oral lichen planus: a systematic review. Braz Oral Res. (2018) 32:e82. doi: 10.1590/1807-3107bor-2018.vol32.0082
149. Tiwari M. Science behind human saliva. J Nat Sci Biol Med. (2011) 2:53–8. doi: 10.4103/0976-9668.82322
150. Neyraud E, Sayd T, Morzel M, Dransfield E. Proteomic analysis of human whole and parotid salivas following stimulation by different tastes. J Proteome Res. (2006) 5:2474–80. doi: 10.1021/pr060189z
151. Tuck MK, Chan DW, Chia D, Godwin AK, Grizzle WE, Krueger KE, et al. Standard operating procedures for serum and plasma collection: early detection research network consensus statement standard operating procedure integration working group. J Proteome Res. (2009) 8:113–7. doi: 10.1021/pr800545q
152. Grassl N, Kulak NA, Pichler G, Geyer PE, Jung J, Schubert S, et al. Ultra-deep and quantitative saliva proteome reveals dynamics of the oral microbiome. Genome Med. (2016) 8:44. doi: 10.1186/s13073-016-0293-0
153. Yan W, Apweiler R, Balgley BM, Boontheung P, Bundy JL, Cargile BJ, et al. Systematic comparison of the human saliva and plasma proteomes. Proteomics Clin Appl. (2009) 3:116–34. doi: 10.1002/prca.200800140
154. Lakshmi PV, Sridevi E, Sai Sankar AJ, Manoj Kumar MG, Sridhar M, Sujatha B. Diagnostic perspective of saliva in insulin dependent diabetes mellitus children: An in vivo study. Contemp Clin Dent. (2015) 6:443–7. doi: 10.4103/0976-237X.169844
155. Denny P, Hagen FK, Hardt M, Liao L, Yan W, Arellanno M, et al. The proteomes of human parotid and submandibular/sublingual gland salivas collected as the ductal secretions. J Proteome Res. (2008) 7:1994–2006. doi: 10.1021/pr700764j
156. Hu S, Li Y, Wang J, Xie Y, Tjon K, Wolinsky L, et al. Human saliva proteome and transcriptome. J Dent Res. (2006) 85:1129–33. doi: 10.1177/154405910608501212
157. Li Y, Zhou X, St John MA, Wong DT. RNA profiling of cell-free saliva using microarray technology. J Dent Res. (2004) 83:199–203. doi: 10.1177/154405910408300303
158. Park NJ, Zhou H, Elashoff D, Henson BS, Kastratovic DA, Abemayor E, et al. Salivary microRNA: discovery, characterization, and clinical utility for oral cancer detection. Clin Cancer Res. (2009) 15:5473–7. doi: 10.1158/1078-0432.CCR-09-0736
159. Sugimoto M, Wong DT, Hirayama A, Soga T, Tomita M. Capillary electrophoresis mass spectrometry-based saliva metabolomics identified oral, breast and pancreatic cancer-specific profiles. Metabolomics. (2010) 6:78–95. doi: 10.1007/s11306-009-0178-y
160. Wong DT. Salivaomics. J Am Dent Assoc. (2012) 143:19S−24S. doi: 10.14219/jada.archive.2012.0339
161. Bastin P, Maiter D, Gruson D. Salivary cortisol testing: preanalytic and analytic aspects. Ann Biol Clin (Paris). (2018) 76:393–405. doi: 10.1684/abc.2018.1355
162. Sonesson M, Hamberg K, Wallengren ML, Matsson L, Ericson D. Salivary IgA in minor-gland saliva of children, adolescents, and young adults. Eur J Oral Sci. (2011) 119:15–20. doi: 10.1111/j.1600-0722.2010.00794.x
163. Khurshid Z, Zafar MS, Khan RS, Najeeb S, Slowey PD, Rehman IU. Role of salivary biomarkers in oral cancer detection. Adv Clin Chem. (2018) 86:23–70. doi: 10.1016/bs.acc.2018.05.002
164. Castagnola M, Scarano E, Passali GC, Messana I, Cabras T, Iavarone F, et al. Salivary biomarkers and proteomics: future diagnostic and clinical utilities. Acta Otorhinolaryngol Ital. (2017) 37:94–101.
165. Schepici G, Silvestro S, Trubiani O, Bramanti P, Mazzon E. Salivary biomarkers: future approaches for early diagnosis of neurodegenerative diseases. Brain Sci. (2020) 10. doi: 10.3390/brainsci10040245
166. Slavish DC, Graham-Engeland JE, Smyth JM, Engeland CG. Salivary markers of inflammation in response to acute stress. Brain Behav Immun. (2015) 44:253–69. doi: 10.1016/j.bbi.2014.08.008
167. Wei F, Wong DT. Point-of-care platforms for salivary diagnostics. Chin J Dent Res. (2012) 15:7–15.
168. Di Pietro V, Porto E, Ragusa M, Barbagallo C, Davies D, Forcione M, et al. Salivary MicroRNAs: diagnostic markers of mild traumatic brain injury in contact-sport. Front Mol Neurosci. (2018) 11:290. doi: 10.3389/fnmol.2018.00290
169. Al-Rawi NH, Atiyah KM. Salivary neuron specific enolase: an indicator for neuronal damage in patients with ischemic stroke and stroke-prone patients. Clin Chem Lab Med. (2009) 47:1519–24. doi: 10.1515/CCLM.2009.345
170. Johnson JJ, Loeffert AC, Stokes J, Olympia RP, Bramley H, Hicks SD. Association of salivary microrna changes with prolonged concussion symptoms. JAMA Pediatr. (2018) 172:65–73. doi: 10.1001/jamapediatrics.2017.3884
171. Yeung C, Bhatia R, Bhattarai B, Sinha M. Role of salivary biomarkers in predicting significant traumatic brain injury: an exploratory study. Pediatr Emerg Care. (2020) doi: 10.1097/PEC.0000000000002050
172. Janigro D, Kawata K, Silverman E, Marchi N, Diaz-Arrastia R. Is salivary S100B a biomarker of traumatic brain injury? A Pilot Study Front Neurol. (2020) 11:528. doi: 10.3389/fneur.2020.00528
173. Markopoulos AK, Belazi M, Drakoulakos D, Petrou-Americanou C, Sioulis A, Sakellari D, et al. Epidermal growth factor in saliva and serum of patients with cyclosporin-induced gingival overgrowth. J Periodontal Res. (2001) 36:88–91. doi: 10.1034/j.1600-0765.2001.360204.x
174. Sanchez Garcia P de Portugal Alvarez J Alonso Gutierrez D Cruz Hernandez JJ [Determination of insulin in saliva and its correlation with plasma insulin. Assessment of the possible participation++ of the salivary glands in the production of the hormone]. An Med Interna. (1989) 6:5–9.
175. Rantonen PJ, Penttila I, Meurman JH, Savolainen K, Narvanen S, Helenius T. Growth hormone and cortisol in serum and saliva. Acta Odontol Scand. (2000) 58:299–303. doi: 10.1080/00016350050217163
176. Gazzolo D, Pluchinotta F, Bashir M, Aboulgar H, Said HM, Iman I, et al. Neurological abnormalities in full-term asphyxiated newborns and salivary S100B testing: the “Cooperative Multitask against Brain Injury of Neonates” (CoMBINe) international study. PLoS ONE. (2015) 10:e0115194. doi: 10.1371/journal.pone.0115194
177. Ebersole JL, Kryscio RJ, Campbell C, Kinane DF, McDevitt J, Christodoulides N, et al. Salivary and serum adiponectin and C-reactive protein levels in acute myocardial infarction related to body mass index and oral health. J Periodontal Res. (2017) 52:419–27. doi: 10.1111/jre.12406
178. Gazzolo D, Lituania M, Bruschettini M, Ciotti S, Sacchi R, Serra G, et al. S100B protein levels in saliva: correlation with gestational age in normal term and preterm newborns. Clin Biochem. (2005) 38:229–33. doi: 10.1016/j.clinbiochem.2004.12.006
179. Michetti F, Bruschettini M, Frigiola A, Abella R, Giamberti A, Marchese N, et al. Saliva S100B in professional sportsmen: High levels at resting conditions and increased after vigorous physical activity. Clin Biochem. (2011) 44:245–7. doi: 10.1016/j.clinbiochem.2010.10.007
180. Risso FM, Sannia A, Gavilanes DA, Vles HJ, Colivicchi M, Ricotti A, et al. Biomarkers of brain damage in preterm infants. J Matern Fetal Neonatal Med. (2012) 25 (Suppl. 4):101–4. doi: 10.3109/14767058.2012.715024
181. Ayatollahi H, Darabi Mahboub MR, Mohammadian N, Parizadeh MR, Kianoosh T, Khabbaz Khoob M, et al. Ratios of free to total prostate-specific antigen and total prostate specific antigen to protein concentrations in saliva and serum of healthy men. Urol J. (2007) 4:238–41.
182. Byrne ML, O'Brien-Simpson NM, Reynolds EC, Walsh KA, Laughton K, Waloszek JM, et al. Acute phase protein and cytokine levels in serum and saliva: a comparison of detectable levels and correlations in a depressed and healthy adolescent sample. Brain Behav Immun. (2013) 34:164–75. doi: 10.1016/j.bbi.2013.08.010
183. La Fratta I, Tatangelo R, Campagna G, Rizzuto A, Franceschelli S, Ferrone A, et al. The plasmatic and salivary levels of IL-1beta, IL-18 and IL-6 are associated to emotional difference during stress in young male. Sci Rep. (2018) 8:3031. doi: 10.1038/s41598-018-21474-y
184. Peters SA. Evaluation of a generic physiologically based pharmacokinetic model for lineshape analysis. Clin Pharmacokinet. (2008) 47:261–75. doi: 10.2165/00003088-200847040-00004
185. Wang J, Liang Y, Wang Y, Cui J, Liu M, Du W, et al. Computational prediction of human salivary proteins from blood circulation and application to diagnostic biomarker identification. PLoS ONE. (2013) 8:e80211. doi: 10.1371/journal.pone.0080211
186. Sun Y, Du W, Zhou C, Zhou Y, Cao Z, Tian Y, et al. A computational method for prediction of saliva-secretory proteins and its application to identification of head and neck cancer biomarkers for salivary diagnosis. IEEE Trans Nanobioscience. (2015) 14:167–74. doi: 10.1109/TNB.2015.2395143
187. Ship JA, DeCarli C, Friedland RP, Baum BJ. Diminished submandibular salivary flow in dementia of the Alzheimer type. J Gerontol. (1990) 45:M61–66. doi: 10.1093/geronj/45.2.M61
188. Siqueira WL, Dawes C. The salivary proteome: challenges and perspectives. Proteomics Clin Appl. (2011) 5:575–9. doi: 10.1002/prca.201100046
189. Bailey DM. Oxygen and brain death; back from the brink. Exp Physiol. (2019) 104:1769–79. doi: 10.1113/EP088005
190. Northcutt RG. Understanding vertebrate brain evolution. Integr Comp Biol. (2002) 42:743–56. doi: 10.1093/icb/42.4.743
191. Calverley TA, Ogoh S, Marley CJ, Steggall M, Marchi N, Brassard P, et al. HIITing the brain with exercise; mechanisms, consequences and practical recommendations. J Physiol. (2020) doi: 10.1113/JP275021
192. Bailey DM, Marley CJ, Brugniaux JV, Hodson D, New KJ, Ogoh S, et al. Elevated aerobic fitness sustained throughout the adult lifespan is associated with improved cerebral hemodynamics. Stroke. (2013) 44:3235–8. doi: 10.1161/STROKEAHA.113.002589
193. Ainslie PN, Cotter JD, George KP, Lucas S, Murrell C, Shave R, et al. Elevation in cerebral blood flow velocity with aerobic fitness throughout healthy human ageing. J. Physiol. (2008) 586:4005–10. doi: 10.1113/jphysiol.2008.158279
194. Prestgaard E, Mariampillai J, Engeseth K, Erikssen J, Bodegård J, Liestøl K, et al. Change in cardiorespiratory fitness and risk of stroke and death: long-term follow-up of healthy middle-aged men. Stroke. (2019) 50:155–61. doi: 10.1161/STROKEAHA.118.021798
195. Tari AR, Nauman J, Zisko N, Skjellegrind HK, Bosnes I, Bergh S, et al. Temporal changes in cardiorespiratory fitness and risk of dementia incidence and mortality: a population-based prospective cohort study. Lancet Public Health. (2019) 4:e565–e574. doi: 10.1016/S2468-2667(19)30183-5
196. Marlatt MW, Potter MC, Lucassen PJ, van Praag H. Running throughout middle-age improves memory function, hippocampal neurogenesis, and BDNF levels in female C57BL/6J mice. Dev Neurob. (2012) 72:943–52. doi: 10.1002/dneu.22009
197. Brown BM, Peiffer J, Taddei K, Lui J, Laws SM, Gupta VB, et al. Physical activity and amyloid-β plasma and brain levels: results from the Australian imaging, biomarkers and lifestyle study of ageing. Mol Psychiatry. (2013) 18:875. doi: 10.1038/mp.2012.107
198. Parachikova A, Nichol KE, Cotman CW. Short-term exercise in aged Tg2576 mice alters neuroinflammation and improves cognition. Neurob Dis. (2008) 30:121–9. doi: 10.1016/j.nbd.2007.12.008
199. Bak TH. Movement disorders: why movement and cognition belong together. Nat Rev Neurol. (2011) 7:10–2. doi: 10.1038/nrneurol.2010.177
200. Berchtold NC, Castello N, Cotman CW. Exercise and time-dependent benefits to learning and memory. Neuroscience. (2010) 167:588–97. doi: 10.1016/j.neuroscience.2010.02.050
201. Malkiewicz MA, Szarmach A, Sabisz A, Cubala WJ, Szurowska E, Winklewski PJ. Blood-brain barrier permeability and physical exercise. J Neuroinfl. (2019) 16:15. doi: 10.1186/s12974-019-1403-x
202. Bailey DM Evans KA McEneny J Young IS Hullin DA James PE . Exercise-induced oxidative-nitrosative stress is associated with impaired dynamic cerebral autoregulation and blood-brain barrier leakage. Exp Physiol. (2011) 96:1196–207. doi: 10.1113/expphysiol.2011.060178
203. Ernsting J. The effect of brief profound hypoxia upon the arterial and venous oxygen tensions in man. J Physiol. (1963) 169:292–311. doi: 10.1113/jphysiol.1963.sp007257
204. Grocott MP, Martin DS, Levett DZ, McMorrow R, Windsor J, Montgomery HE, et al. Arterial blood gases and oxygen content in climbers on Mount Everest. N Engl J Med. (2009) 360:140–9. doi: 10.1056/NEJMoa0801581
205. Bailey DM, Roukens R, Knauth M, Kallenberg K, Christ S, Mohr A, et al. Free radical-mediated damage to barrier function is not associated with altered brain morphology in high-altitude headache. J Cereb Blood Flow Metab. (2006) 26:99–111. doi: 10.1038/sj.jcbfm.9600169
206. Kallenberg K, Bailey DM, Christ S, Mohr A, Roukens R, Menold E, et al. Magnetic resonance imaging evidence of cytotoxic cerebral edema in acute mountain sickness. J Cereb Blood Flow Metab. (2007) 27:1064–71. doi: 10.1038/sj.jcbfm.9600404
207. Bailey DM Taudorf S Berg RMG Lundby C McEneny J Young IS . Increased cerebral output of free radicals during hypoxia: implications for acute mountain sickness? Am J Physiol. (2009) 297:R1283–1292. doi: 10.1152/ajpregu.00366.2009
208. Bailey DM, Rasmussen P, Evans KA, Bohm AM, Zaar M, Nielsen HB, et al. Hypoxia compounds exercise-induced free radical formation in humans; partitioning contributions from the cerebral and femoral circulation. Free Rad Biol Med. (2018) 124:104–13. doi: 10.1016/j.freeradbiomed.2018.05.090
209. Bailey DM, Rasmussen P, Overgaard M, Evans KA, Bohm AM, Seifert T, et al. Nitrite and S-Nitrosohemoglobin exchange across the human cerebral and femoral circulation: relationship to basal and exercise blood flow responses to hypoxia. Circulation. (2017) 135:166–76. doi: 10.1161/CIRCULATIONAHA.116.024226
210. Bailey DM Evans KA James PE McEneny J Young IS Fall L . Altered free radical metabolism in acute mountain sickness: implications for dynamic cerebral autoregulation and blood-brain barrier function. J Physiol. (2009) 587:73–85. doi: 10.1113/jphysiol.2008.159855
211. Bailey DM, Bartsch P, Knauth M, Baumgartner RW. Emerging concepts in acute mountain sickness and high-altitude cerebral edema: from the molecular to the morphological. Cellular and Molecular Life Sciences. (2009) 66:3583–94. doi: 10.1007/s00018-009-0145-9
212. Bailey DM, Willie CK, Hoiland RL, Bain AR, MacLeod DB, Santoro MA, et al. Surviving without oxygen: how low can the human brain go? High Altitude Med Biol. (2017) 18:73–9. doi: 10.1089/ham.2016.0081
213. Willie CK, Ainslie PN, Drvis I, MacLeod DB, Bain AR, Madden D, et al. Regulation of brain blood flow and oxygen delivery in elite breath-hold divers. J Cerebral Blood Flow Metab. (2015) 35:66–73. doi: 10.1038/jcbfm.2014.170
214. Bain AR Ainslie PN Hoiland RL Barak OF Drvis I Stembridge M . Competitive apnea and its effect on the human brain: focus on the redox regulation of blood-brain barrier permeability and neuronal-parenchymal integrity. FASEB J. (2018) 32:2305–14. doi: 10.1096/fj.201701031R
215. Bailey DM, Laneelle D, Trihan JE, Marchi N, Stacey BS, Tamiya K, et al. Gravitational transitions increase posterior cerebral perfusion and systemic oxidative-nitrosative stress: implications for neurovascular unit integrity. Neuroscience. (2020). doi: 10.1016/j.neuroscience.2020.05.048
216. Kallenberg K, Dehnert C, Dorfler A, Schellinger PD, Bailey DM, Knauth M, et al. Microhemorrhages in nonfatal high-altitude cerebral edema. J Cereb Blood Flow Metab. (2008) doi: 10.1038/jcbfm.2008.55
217. Hackett PH, Roach RC. High-altitude illness. N Engl J Med. (2001) 345:107–14. doi: 10.1056/NEJM200107123450206
218. Sanchez del Rio M and Moskowitz MA. High altitude headache. Lessons from headaches at sea level. Adv Exp Med Biol. (1999) 474:145–53. doi: 10.1007/978-1-4615-4711-2_13
219. Matsuzawa Y, Kobayashi T, Fujimoto K, Shinozaki SY. Cerebral edema in acute mountain sickness. In: Ueda G, Reeves JT, Sekiguchi M, editors. High Altitude Med. Matsumoto: Shinshu University Press. (1992). p. 300–4.
220. Schoonman GG, Sandor PS, Nirkko AC, Lange T, Jaermann T, Dydak U, et al. Hypoxia-induced acute mountain sickness is associated with intracellular cerebral edema: a 3 T magnetic resonance imaging study. J Cerebral Blood Flow Metab. (2008) 28:198–206. doi: 10.1038/sj.jcbfm.9600513
221. Rosenblum W. Cytotoxic edema: monitoring its magnitude and contribution to brain swelling. J Neuropathol Exp Neurol. (2007) 66:771–8. doi: 10.1097/nen.0b013e3181461965
222. Wilson MH, Davagnanam I, Holland G, Dattani RS, Tamm A, Hirani SP, et al. Cerebral venous system and anatomical predisposition to high-altitude headache. Ann Neurol. (2013) 73:381–9. doi: 10.1002/ana.23796
223. Andersson JP, Liner MH, Jonsson H. Increased serum levels of the brain damage marker S100B after apnea in trained breath-hold divers: a study including respiratory and cardiovascular observations. J Appl Physiol. (2009) 107:809–15. doi: 10.1152/japplphysiol.91434.2008
224. Kjeld T, Jattu T, Nielsen HB, Goetze JP, Secher NH, Olsen NV. Release of erythropoietin and neuron-specific enolase after breath holding in competing free divers. Scand J Med Sci Sports. (2015) 25:e253–7. doi: 10.1111/sms.12309
225. Hansen AJ. Effect of anoxia on ion distribution in the brain. Physiol Rev. (1985) 65:101–48. doi: 10.1152/physrev.1985.65.1.101
226. Lee AG, Mader TH, Gibson CR, Tarver W, Rabiei P, Riascos RF, et al. Spaceflight associated neuro-ocular syndrome (SANS) and the neuro-ophthalmologic effects of microgravity: a review and an update. NPJ Microgravity. (2020) 6:7. doi: 10.1038/s41526-020-00114-8
227. Carlsson SI, Bertilaccio MT, Ballabio E, Maier JA. Endothelial stress by gravitational unloading: effects on cell growth and cytoskeletal organization. Biochim Biophy Acta. (2003) 1642:173–9. doi: 10.1016/j.bbamcr.2003.08.003
228. Porte Y, Morel JL. Learning on Jupiter, learning on the Moon: the dark side of the G-force. Effects of gravity changes on neurovascular unit and modulation of learning and memory. Front Behav Neurosci. (2012) 6:64. doi: 10.3389/fnbeh.2012.00064
229. Dubayle D, Vanden-Bossche A, Beraneck M, Vico L, Morel JL. Effects of centrifugation and whole-body vibrations on blood-brain barrier permeability in mice. NPJ Microgravity. (2020) 6:1. doi: 10.1038/s41526-019-0094-z
230. Lakin WD, Stevens SA, Penar PL. Modeling intracranial pressures in microgravity: the influence of the blood-brain barrier. Aviat Space Environ Med. (2007) 78:932–6. doi: 10.3357/ASEM.2060.2007
231. Shelhamer M. Parabolic flight as a spaceflight analog. J Appl Physiol. (2016) 120:1442–8. doi: 10.1152/japplphysiol.01046.2015
232. Stein TP. Space flight and oxidative stress. Nutrition. (2002) 18:867–71. doi: 10.1016/S0899-9007(02)00938-3
233. Blyth BJ, Farhavar A, Gee C, Hawthorn B, He H, Nayak A, et al. Validation of serum markers for blood-brain barrier disruption in traumatic brain injury. J Neurotrauma. (2009) 26:1497–507. doi: 10.1089/neu.2008.0738
234. Gulati G, Iffland PH 2nd, Janigro D, Zhang B, Luggen ME. Anti-NR2 antibodies, blood-brain barrier, and cognitive dysfunction. Clin Rheumatol. (2016) 35:2989–97. doi: 10.1007/s10067-016-3339-1
235. Falcone T, Carlton E, Lee C, Janigro M, Fazio V, Forcen FE, et al. Does systemic inflammation play a role in pediatric psychosis? Clin Schizophr Relat Psychoses. (2015) 9:65–78B. doi: 10.3371/CSRP.FACA.030813
236. Jickling GC, Sharp FR. Blood biomarkers of ischemic stroke. Neurotherapeutics. (2011) 8:349–60. doi: 10.1007/s13311-011-0050-4
237. Monbailliu T, Goossens J, Hachimi-Idrissi S. Blood protein biomarkers as diagnostic tool for ischemic stroke: a systematic review. Biomark Med. (2017) 11:503–12. doi: 10.2217/bmm-2016-0232
238. Li Y, Li M, Zuo L, Shi Q, Qin W, Yang L, et al. Compromised blood-brain barrier integrity is associated with total magnetic resonance imaging burden of cerebral small vessel disease. Front Neurol. (2018) 9:221. doi: 10.3389/fneur.2018.00221
239. Steiner J, Schiltz K, Walter M, Wunderlich MT, Keilhoff G, Brisch R, et al. S100B serum levels are closely correlated with body mass index: an important caveat in neuropsychiatric research. Psychoneuroendocrinology. (2010) 35:321–4. doi: 10.1016/j.psyneuen.2009.07.012
240. Korfias S, Stranjalis G, Psachoulia C, Vasiliadis C, Pitaridis M, Boviatsis E, et al. Slight and short-lasting increase of serum S-100B protein in extra-cranial trauma. Brain Inj. (2006) 20:867–72. doi: 10.1080/02699050600832395
241. Reitz C, Mayeux R. Alzheimer disease: epidemiology, diagnostic criteria, risk factors and biomarkers. Biochem Pharmacol. (2014) 88:640–51. doi: 10.1016/j.bcp.2013.12.024
242. Shaw LM, Arias J, Blennow K, Galasko D, Molinuevo JL, Salloway S, et al. Appropriate use criteria for lumbar puncture and cerebrospinal fluid testing in the diagnosis of Alzheimer's disease. Alzheimers Dement. (2018) 14:1505–21. doi: 10.1016/j.jalz.2018.07.220
243. Montagne A, Huuskonen MT, Rajagopal G, Sweeney MD, Nation DA, Sepehrband F, et al. Undetectable gadolinium brain retention in individuals with an age-dependent blood-brain barrier breakdown in the hippocampus and mild cognitive impairment. Alzheimers Dement. (2019) 15:1568–75. doi: 10.1016/j.jalz.2019.07.012
244. Montagne A, Nation DA, Sagare AP, Barisano G, Sweeney MD, Chakhoyan A, et al. APOE4 leads to blood-brain barrier dysfunction predicting cognitive decline. Nature. (2020) 581:71–6. doi: 10.1038/s41586-020-2247-3
245. Sweeney MD, Sagare AP, Pachicano M, Harrington MG, Joe E, Chui HC, et al. A novel sensitive assay for detection of a biomarker of pericyte injury in cerebrospinal fluid. Alzheimers Dement. (2020) 16:821–30. doi: 10.1002/alz.12061
246. Leuzy A, Chiotis K, Lemoine L, Gillberg PG, Almkvist O, Rodriguez-Vieitez E, et al. Tau PET imaging in neurodegenerative tauopathies-still a challenge. Mol Psychiatry. (2019) doi: 10.1038/s41380-018-0342-8
247. Cohen AD, Landau SM, Snitz BE, Klunk WE, Blennow K, Zetterberg H. Fluid and PET biomarkers for amyloid pathology in Alzheimer's disease. Mol Cell Neurosci. (2018) doi: 10.1016/j.mcn.2018.12.004
248. Zetterberg H, Smith DH, Blennow K. Biomarkers of mild traumatic brain injury in cerebrospinal fluid and blood. Nat Rev Neurol. (2013) 9:201–10. doi: 10.1038/nrneurol.2013.9
249. Mayeux R. Biomarkers: potential uses and limitations. NeuroRx. (2004) 1:182–8. doi: 10.1602/neurorx.1.2.182
Keywords: neurovascular unit, blood biomarkers, saliva, concussion, epilepsy, neurodegeneration, traumatic brain injury, extreme sports
Citation: Janigro D, Bailey DM, Lehmann S, Badaut J, O'Flynn R, Hirtz C and Marchi N (2021) Peripheral Blood and Salivary Biomarkers of Blood–Brain Barrier Permeability and Neuronal Damage: Clinical and Applied Concepts. Front. Neurol. 11:577312. doi: 10.3389/fneur.2020.577312
Received: 29 June 2020; Accepted: 01 December 2020;
Published: 04 February 2021.
Edited by:
Denes V. Agoston, Karolinska Institutet (KI), SwedenReviewed by:
Berislav Zlokovic, University of Southern California, United StatesAndrew K. Ottens, Virginia Commonwealth University, United States
Copyright © 2021 Janigro, Bailey, Lehmann, Badaut, O'Flynn, Hirtz and Marchi. This is an open-access article distributed under the terms of the Creative Commons Attribution License (CC BY). The use, distribution or reproduction in other forums is permitted, provided the original author(s) and the copyright owner(s) are credited and that the original publication in this journal is cited, in accordance with accepted academic practice. No use, distribution or reproduction is permitted which does not comply with these terms.
*Correspondence: Nicola Marchi, bmljb2xhLm1hcmNoaUBpZ2YuY25ycy5mcg==