- 1Department of Human Neurosciences, “Sapienza” University of Rome, Rome, Italy
- 2Department of Neurology, Fatebenefratelli Hospital, Isola Tiberina, Rome, Italy
- 3Department of Biomedicine and Prevention, University of Rome Tor Vergata, Rome, Italy
- 4Physical Medicine and Rehabilitation Division, Umberto I Hospital, Rome, Italy
- 5Department of Physical Medicine and Rehabilitation, “Sapienza” University of Rome, Rome, Italy
- 6IRCCS Fondazione Don Carlo Gnocchi, Milan, Italy
- 7Department of Systems Medicine, University of Rome Tor Vergata, Rome, Italy
- 8Department of Radiological, oncological and pathological Sciences - Radiometabolic Division, Umberto I Hospital, Rome, Italy
Focal repetitive muscle vibration (fMV) is a safe and well-tolerated non-invasive brain and peripheral stimulation (NIBS) technique, easy to perform at the bedside, and able to promote the post-stroke motor recovery through conditioning the stroke-related dysfunctional structures and pathways. Here we describe the concurrent cortical and spinal plasticity induced by fMV in a chronic stroke survivor, as assessed with 99mTc-HMPAO SPECT, peripheral nerve stimulation, and gait analysis. A 72-years-old patient was referred to our stroke clinic for a right leg hemiparesis and spasticity resulting from a previous (4 years before) hemorrhagic stroke. He reported a subjective improvement of his right leg's spasticity and dysesthesia that occurred after a30-min ride on a Vespa scooter as a passenger over the Roman Sampietrini (i.e., cubic-shaped cobblestones). Taking into account both the patient's anecdote and the current guidelines that recommend fMV for the treatment of post-stroke spasticity, we then decided to start fMV treatment. 12 fMV sessions (frequency 100 Hz; amplitude range 0.2–0.5 mm, three 10-min daily sessions per week for 4 consecutive weeks) were applied over the quadriceps femoris, triceps surae, and hamstring muscles through a specific commercial device (Cro®System, NEMOCOsrl). A standardized clinical and instrumental evaluation was performed before (T0) the first fMV session and after (T1) the last one. After fMV treatment, we observed a clinically relevant motor and functional improvement, as assessed by comparing the post-treatment changes in the score of the Fugl-Meyer assessment, the Motricity Index score, the gait analysis, and the Ashworth modified scale, with the respective minimal detectable change at the 95% confidence level (MDC95). Data from SPECT and peripheral nerve stimulation supported the evidence of a concurrent brain and spinal plasticity promoted by fMV treatment trough activity-dependent changes in cortical perfusion and motoneuron excitability, respectively. In conclusion, the substrate of post-stroke motor recovery induced by fMV involves a concurrently acting multisite plasticity (i.e., cortical and spinal plasticity). In our patient, operant conditioning of both cortical perfusion and motoneuron excitability throughout a month of fMV treatment was related to a clinically relevant improvement in his strength, step symmetry (with reduced limping), and spasticity.
Introduction
Stroke is the leading cause of long-term disability (1) with reduced mobility in more than half of stroke survivors aged 65 and over (2).
A focal brain lesion resulting from stroke may trigger maladaptive structural and functional changes in perilesional and remote regions (3–5), thereby resulting in incomplete motor recovery. Thus, to evaluate which could be the most effective training protocol for the rehabilitation of a paretic limb, there is a growing interest toward the attempts to condition the dysfunctional cerebral structures and pathways throughout non-invasive central and peripheral stimulation techniques (NIBS), as seen for others neurological diseases (6–10).
Focal repetitive muscle vibration (fMV) is a safe and well-tolerated intervention, easy to perform at the bedside, and able to promote motor recovery both in acute (11) and chronic stroke patients (12–15).
Despite the great number of recent works, the mechanism underlying motor improvement after fMV in stroke patients is not completely understood yet. Given that a repeated sensory input is one of the most effective modulators of cortical motor and somatosensory structures (16), it has been suggested that fMV can produce substantial neurophysiological changes both at a cortical and at a peripheral level (12, 17), probably throughout long-term depression-like plasticity mechanisms (18).
Here we describe the concurrent cortical and spinal plasticity induced by fMV in a chronic stroke survivor, as assessed with 99mTc-HMPAO SPECT, peripheral nerve stimulation, and gait analysis. We also focused on the relationship between the observed multisite plasticity and patient's improvement in different motor aspects (i.e., strength, gait, and spasticity) after fMV treatment.
Case Study—From a Vespa Scooter Ride Over the Roman Sampietrini to Focal Muscle Vibration (fMV)
A 72-years-old patient was referred to our stroke clinic for a right leg hemiparesis and spasticity resulting from a previous (4 years before) hemorrhagic stroke. The neurological examination also showed right-sided severe hypoesthesia and dysesthesia associated with tactile extinction.
He has been treated with Tizanidine (up to 36 mg/day) and physiokinesitherapy (1-h daily session, 3 days a week) with no further clinical improvements in the past year. No other vascular risk factors were known but hypertension. A computer tomography (CT) scan showed the results of the known cerebral hemorrhage involving the left thalamus and the internal capsule.
In a sort of “Roman Holidays” movie set, our patient anecdotally reported subjective clinical improvement in spasticity and dysesthesia of the right leg that occurred after a 30-min ride as a passenger on a Vespa scooter. The ride had taken place on a paved road by Roman Sampietrini (i.e., cubic-shaped cobblestones) which produced many vibrations.
Taking into account both the patient's anecdote and the current guidelines which recommend focal muscle vibration (fMV) for the treatment of post-stroke spasticity (14), we then decided to start fMV treatment.
fMV Treatment
A total of 12 fMV sessions (3 sessions per week for 4 consecutive weeks) were carried out by a trained physiatrist. Each daily session consisted of three 10-min treatment, interspersed with a 1-min break (11).
Low-amplitude fMV (frequency 100 Hz; amplitude range 0.2–0.5 mm) was applied over the quadriceps femoris, triceps surae, and hamstring muscles through a specific commercial device (Cro®System, NEMOCOsrl). During the fMV treatment, the subject was required to maintain a voluntary steady contraction of the target muscle at 20% of the maximal voluntary contraction (MVC), as assessed by visual EMG feedback (12, 19). During the intervals (1 min), fMV was interrupted and the subject was requested to relax the muscle. fMV was delivered during mild voluntary contraction because it was shown that voluntary muscle activity increases response to vibration, probably through fusimotor co-activation and subsequent increase in spindle discharge (18, 20). Moreover, the prolonged activation of the descending volley, induced by the ongoing muscle contraction with ascending volleys, might explain the changes in corticomotor excitability of the target muscle (19).
Clinical Assessment
To examine fMV effects, a standardized clinical and instrumental evaluation was performed before (T0) the first fMV session and 7 days after (T1) the last one as follows (see Figure 1 for the study flow chart).
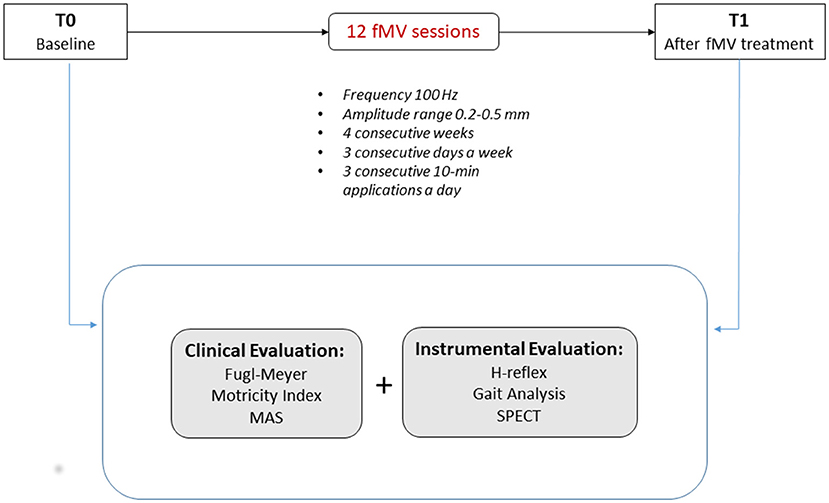
Figure 1. Study flow chart—clinical and instrumental evaluation was performed before (T0) the first fMV session and 7 days after the last one (T1).
Motor and functional performance were evaluated by using both the lower limb subscale of Fugl-Meyer assessment (FMA) (21–23) and the Motricity Index (MI) (24). FMA subscale for the lower limb examines movement, coordination, and reflex action of the hip, knee, and ankle in the supine, sitting, and standing positions. Each item is scored on a 3-point scale (0, cannot perform; 1, partially performs; 2, performs fully). The score range is 0 to 34, with higher scores indicating better lower limb motor performance. The minimal detectable change at the 95% confidence level (MDC95) is 4-point (25).
MI examines hip flexion, knee extension, and ankle dorsiflexion (3 items for each side). The total score ranges from 0 (complete paresis) to 100 (normal strength), with an MDC95 is 12.92 (26).
Spasticity was assessed with the Ashworth scale modified by Bohannon and Smith (27) (MAS), which measures resistance during passive soft-tissue stretching scoring from 0 (no increase in muscle tone) to 4 (affected part(s) rigid in flexion or extension). A one-point decrease reflects a clinically significant improvement at the 95% confidence level MDC95 (28).
Gait Analysis
As regards the instrumental evaluation, we performed a gait analysis to evaluate cadence (step/min), velocity, right, and left stride length. We adopted the inertial measurement unit (IMU), which includes a triaxial accelerometer (16 bits/axis, up to 1,000 Hz) with multiple sensitivity (± 2, ± 4, ± 8, ± 16 g), a triaxial 13-bit magnetometer (± 1,200 μ T, up to 100 Hz), and a triaxial gyroscope (16 bits/axis, up to 8,000 Hz) with multiple sensitivity (± 250, ± 500, ± 1,000, ± 2,000 °/s). The MDC95 for gait analysis' spatiotemporal, kinematic, and kinetic measurements can be found in Geiger et al. (29).
Peripheral Nerve Stimulation
Electromyography (EMG) was performed on the tibial nerve (mixed nerve), using stimuli of increased intensity to evaluate H-reflex, M-reflex, and their ratio H/M. The size of the H-reflex and M-response was defined as the peak-to-peak amplitude of the EMG record. To record the electrical activity of the soleus muscle, both surface and intramuscular EMG were used according to the standardized registration technique proposed by Gorkem et al. (30).
SPECT Imaging
99mTc-HMPAO Single Photon Emission Computed Tomography (SPECT) was performed to evaluate functional cortical reorganization and perfusion, assessed by the evaluation of 99mTc-HMPAO distribution. The blood perfusion in the left and right cerebral hemispheres was assessed in rest status both at baseline and after treatment (Figure 2). The patient was asked to avoid caffeine, smoke, alcohol, and psychotropic drug that can affect the cerebral blood flow.
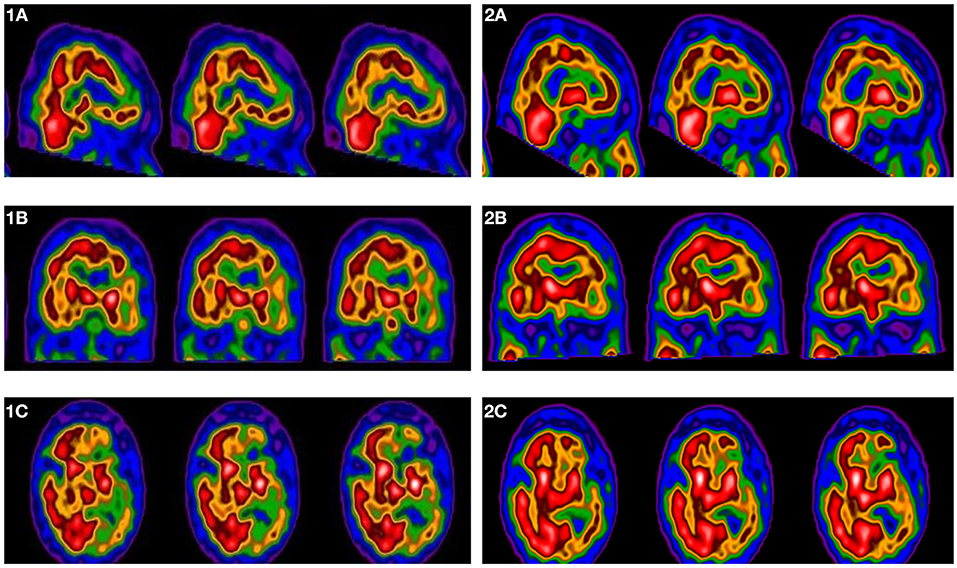
Figure 2. 99mTc-HMPAO brain perfusion SPECT scan at baseline in sagittal (1a), coronal (1b), and transaxial (1c) reconstruction; 99mTc-HMPAO perfusion brain SPECT scan after fMV treatment in sagittal (2a), coronal (2b) and transaxial (2c) reconstruction. SPECT images show an increased 99mTc-HMPAO distribution rate after fMV treatment in left frontal region (1a vs. 2a), in left parietal region (1b vs. 2b), in left occipital region (1c vs. 2c).
The imaging data were evaluated by 2 experienced operators that performed a qualitative analysis. The semi-quantitative analysis was carried out using the NeuroGam software. NeuroGam software (Segami-Corporation, http://www.segamicorp.com/) allows statistical analysis methods for automated diagnosis of brain perfusion SPECT images and can be used to investigate brain perfusion objectively and easily. This software applies an affine anatomical co-registration by blocks of data defined in the Talairach space. The Talairach technique normalized brain cortical perfusion measurements to the perfusion of the cerebellum (31).
NeuroGam was applied to the reoriented and reconstructed data as follows: we reoriented the three-dimensional volume of the brain study defining a line that fits the inferior pole of the occipital lobe and the inferior edge of the frontal lobe. Then, we defined a line above the interhemispheric fissure and automatically orienting this line in the vertical plane to avoid the lateral deviations. After the reorientation of the image, we defined the intermediate level of the pons and anterior plane of the temporal lobes. Therefore, data were processed analyzing ten regions of interest (ROI), corresponding to the entire cortex of the hemisphere, to the parietal, temporal, frontal, and occipital regions, all analyzed with respect to the contralateral region. Data were expressed as maximum, minimum, and mean standard deviation of count rate registered.
The semiquantitative evaluation of brain perfusion in cortical areas of both hemispheres has been automatically performed by the software which automatically calculates the mean and standard deviation of each cortical region's perfusion level (32). The reconstructed data have been also compared to the normal database available for the NeuroGam analysis. Further SPECT imaging's technical details can be found in Frantellizzi et al. (33) Mancini et al. (34).
Ethics Statement
Written informed consent was obtained from the patient both to participate in the study and for the publication of any potentially identifiable images or data included in this article. The study was conducted in accordance with the Declaration of Helsinki Ethical Principles and Good Clinical Practices and was approved by the local ethics committee (Ethics Committee of “Sapienza,” University of Rome—Ref. No. 3661).
Results
After fMV treatment (T1), the patient reported a substantial clinical improvement in his leg's spasticity and dysesthesia, as well as a subjective feeling of “more fluent walking,” that gradually started within the first week of fMV treatment and lasted until T1 evaluation (i.e., 7 days after the end of fMV treatment).
The neurological examination performed at T1 showed an increase in the FMA global score compared to baseline (27 vs. 23, respectively). Namely, we found an improvement in the hip flexion, knee flexion/extension, and ankle dorsiflexion FMA's items. The MI score increased from 76 at T0 to 91 at T1. After treatment, we also found a reduction of the hypertonus as assessed with MAS (T0 vs. T1 MAS score: 2 vs. 1).
The gait analysis was performed by assessing the cadence, speed, and length of the step. When comparing left and right stride length, we found a higher right-left symmetry after treatment (T1 left stride length vs. right stride length: 0.49 ± 0.08 m vs. 0.5 ± 0.09 m) when compared to baseline (T0 left stride length vs. right stride length: 0.43 ± 0.15 m vs. 0.56 ± 0.11 m). We also found a slight decrease in both step cadence (T0 vs. T1: 75.84 ± 16.19 vs. 72.86 ± 16.31 step/min) and mean velocity (T0 vs. T1: 0.66 ± 0.14 vs. 0.61 m/s).
H-reflex amplitude decreased from 1 mv (T0 value) to 0.1 mv (T1 value). The H/M ratio expressed as a percentage was 81.9% at T0, whereas at the end of fMV treatment was 25.9%. Complete data from H-reflex analysis are reported in Table 1.
SPECT data comparing T0 and T1 assessments of cerebral perfusion are shown in Table 2. The qualitative analysis showed a more homogenous perfusion pattern after treatment, with a global improvement of perfusion in the affected hemisphere. In the semiquantitative analysis, the mean standard deviation count rate of 99mTc-HMPAO distribution in the whole brain was higher after treatment compared to baseline (T0 vs. T1: −1.9 vs. −1.6) suggesting a global improvement of blood perfusion.
The distribution of 99mTc-HMPAO after fMV also suggested a global improvement of the mean rate of perfusion reported in areas affected by stroke. Namely, the distribution of 99mTc-HMPAO was higher at T1 in comparison to baseline both in the entire left cortex (T0 vs. T1: −3.3 vs. −2.8), and in single brain regions as the left frontal lobe (T0 vs. T1: −3.1 vs. −2.9), left occipital lobe (T0 vs. T1: −2.7 vs. −1.6), left parietal lobe (T0 vs. T1: −2.6 vs. −1.7), and left temporal lobe (T0 vs. T1: −3.6 vs. −3.2). The distribution of the radiolabeled compound after treatment, considering the semiquantitative nature of Neurogam analysis, was reduced in the right cortex compared to baseline (T0 vs. T1: −1.2 vs. −1.5).
Discussion
Since the last two decades, the attempt to condition the mechanisms underlying plasticity throughout non-invasive central and peripheral stimulation techniques has been pivotal to enhance recovery of locomotion in many neuromuscular disorders and stroke as well.
To test the hypothesis that fMV can produce changes at a cortical and spinal level simultaneously and that these changes are concurrently responsible for the post-stroke motor improvement, we analyzed in the same patient the changes in brain perfusion elicited by spinal H-reflex open conditioning throughout a month of fMV treatment.
In this regard, there is no agreement in the literature about the number of fMV sessions required for motor improvement to be very effective. Since the amount of the induced change grows gradually as conditioning trials continue over subsequent days and weeks (35, 36) we decided to carry out a total of 12 fMV sessions (3 sessions per week for 4 consecutive weeks), thereby extending the classic one-week treatment (overall 3 sessions) (13, 19, 37).
fMV-Induced Brain Plasticity
Our data showed an fMV-induced increase of the 99mTc-HMPAO distribution rate in the left hemisphere, suggesting a global improvement of the mean rate of perfusion in the affected hemisphere after treatment. This confirms that the repeated muscle vibration produces a repeated sensory input that reaches, via Ia fiber afferent input, both the SI and M1 directly (38–41), thereby leading to an improvement of affected limb's motor and functional performance through an intrinsic plasticity-related mechanism (13, 42, 43).
Interestingly, we also found a post-treatment reduction in the distribution of the radiolabeled compound in the right cortex compared to baseline. A possible explanation is that fMV can reduce abnormalities of both the corticospinal excitability and the intracortical inhibitory systems in chronic stroke patients (12), trough modulating the post-stroke altered balance of excitatory and inhibitory influences within the motor network.
Due to the semiquantitative nature of the analysis performed, the 99mTc-HMPAO distribution in the right cortex is influenced by the contralateral compound distribution and, therefore, the reduction of 99mTc-HMPAO distribution in the unaffected hemisphere may reflect the perfusion improvement of the affected one.
Thus, we cannot confirm that the improvement of the mean rate of perfusion in the affected hemisphere directly caused the decrease of perfusion in the unaffected hemisphere through the modulation of the transcallosal inhibition. However, the fMV treatment showed a plasticity-dependent increase of perfusion as a marker of reactivation of functionally impaired circuits within the motor network (44).
fMV-Induced Spinal Cord Plasticity
Operant conditioning of the H-reflex (i.e., the electric analog of the spinal stretch reflex) has widely considered as a valid paradigm for investigating the plasticity induced in the human spinal cord (36). Evidence from studies on healthy subjects (17, 18) suggests that fMV is able to promote spinal plasticity by inducing a decrease of the H-reflex. Thus, H-reflex operant conditioning techniques, as fMV, may represent a new approach for enhancing functional recovery.
In our patient, we observed a reduction in the amplitude of H-reflex from T0 to T1. The change in H-reflex was strongly related to fMV treatment (i.e., activity-dependent) since it has been demonstrated that a sustained voluntary muscle contraction is not able to induce changes of the H-reflex (18). Thus, fMV induces a change in the intrinsic spinal cord properties, probably consisting of an activity-dependent decrease of the intrinsic motoneuron excitability (17) throughout long-term depression-like (LTD-like) plasticity.
A decreased motoneuronal excitability was further confirmed in our patient by the reduction in the H/M ratio from 81.9% at T0 to 25.9% at T1. Given that H max represents an indirect estimate of the number of motoneurons (MN) being recruited, and the M max represents the entire motoneuron pool, the H/M ratio can be interpreted as the proportion of the entire MN pool capable of being recruited (45).
Taken together, data from SPECT and peripheral nerve stimulation support the evidence of a concurrent brain and spinal plasticity promoted by fMV treatment trough activity-dependent changes in cortical perfusion and motoneuron excitability, respectively.
Motor Recovery
After describing the intrinsic changes in the brain and the spine induced by fMV, we aimed to test if these changes were related to an improvement in our patient's motor performance. Thus, we analyzed his strength, gait, and spasticity at 7 days after the end of fMV treatment.
To understand if the patient experienced a clinically relevant change after fMV treatment, we compared the post-treatment changes in the score of each scale with the respective MDC95, which is the smallest real difference that can be detected by a scale at the 95% confidence level beyond measurement error. MDC95 represents the minimal amount of change in the score that must occur to be sure that the change in score is not simply attributable to measurement error and helps clinicians to understand data in the clinical setting also at the individual level (28).
Overall, we observed a clinically relevant motor and functional improvement after fMV treatment. We found an increase in the T1 FMA global score compared to baseline (27 vs. 23, respectively, MDC95:4), with an improvement in the hip flexion, knee flexion/extension, and ankle dorsiflexion. MI score increased from 76 at T0 to 91 at T1 (MDC95:12.92).
Data from gait analysis showed that, beyond a clinically relevant change in both the right and left stride length (T0/T1 right stride length: 0.56/0.5 m; T0/T1 left stride length: 0.43/0.49 m. MDC95:0.06 m), right and left values became similar after treatment, that indicates a recovery of the step symmetry with reduced limping. Although operant conditioning can be a useful therapeutic tool for enhancing locomotion in patients with spinal cord injuries, the mechanism underlying the fMV-induced effects on the symmetry of the step is still debated.
It is well-known the role of fMV in reducing spasticity when applied to the spastic muscles of hemiplegic limb in post-stroke patients (13, 46, 47). Thus, we hypothesized that the observed findings are ascribable to the effects of fMV stimulation, which would result in better motor control of the affected limb and inhibition of pathological motor patterns through a reduction of the hypertonicity degree. This effect was confirmed in our patient, since we found a clinically relevant activity-dependent reduction of the quadriceps femoral muscle's hypertonus, as assessed with the MAS (MDC95:1).
A limitation of the present study is that we use MAS to assess spasticity without considering the muscular viscoelasticity, which is another component of passive resistance to stretch of muscles. Anyway, MAS is widely considered a standard method for the assessment of spasticity in clinical settings. Moreover, several factors may influence the distribution of 99mTc-HMPAO, the reproducibility of the SPECT studies, and the semi-quantitative analysis of the data. Further studies using higher-resolution techniques (i.e., fMRI or quantitative EEG) are needed to confirm this datum.
Conclusions
The substrate of post-stroke motor recovery induced by fMV involves a concurrently acting multisite plasticity (i.e., cortical and spinal plasticity). In our patient, operant conditioning of both cortical perfusion and motoneuron excitability throughout a month of fMV treatment was related to an clinically relevant improvement in his strength, step symmetry (with reduced limping), and spasticity.
Data Availability Statement
The original contributions presented in the study are included in the article/supplementary materials, further inquiries can be directed to the corresponding author/s.
Ethics Statement
The study involving human participants was conducted in accordance with the Declaration of Helsinki Ethical Principles and Good Clinical Practices and was approved by the local ethics committee (Ethics Committee of “Sapienza”, University of Rome). Written informed consent was obtained from the patient both to participate in the study and for the publication of any potentially identifiable images or data included in this article.
Author Contributions
MT: study design and overview and manuscript preparation. MRi: SPECT data analysis and manuscript preparation. CC: fMV execution and data analysis. MP: peripheral nerve stimulation and gait analysis. MRu: clinical evaluation and data analysis. AV: literature review and manuscript review. TJ: literature review and ethics committee. AA: clinical scales' implementation and review. ML: SPECT overview and manuscript review. FC: fMV overview and manuscript review. VD: data interpretation and manuscript review. All authors: contributed to the article and approved the submitted version.
Conflict of Interest
The authors declare that the research was conducted in the absence of any commercial or financial relationships that could be construed as a potential conflict of interest.
References
1. Stroke Unit Trialists' Collaboration. Organised inpatient (stroke unit) care for stroke. Cochrane Datab Syst Rev. (2013) 2013:CD000197. doi: 10.1002/14651858.CD000197.pub3
2. Langhorne P, Coupar F, Pollock A. Motor recovery after stroke: a systematic review. Lancet Neurol. (2009) 8:741–54. doi: 10.1016/S1474-4422(09)70150-4
3. Talelli P, Greenwood RJ, Rothwell JC. Arm function after stroke: neurophysiological correlates and recovery mechanisms assessed by transcranial magnetic stimulation. Clin Neurophysiol. (2006) 117:1641–59. doi: 10.1016/j.clinph.2006.01.016
4. Grefkes C, Fink GR. Reorganization of cerebral networks after stroke: new insights from neuroimaging with connectivity approaches. Brain. (2011) 134:1264–76. doi: 10.1093/brain/awr033
5. Jang SH. Motor function-related maladaptive plasticity in stroke: a review. NeuroRehabilitation. (2013) 32:311–6. doi: 10.3233/NRE-130849
6. Gunduz A, Rothwell J, Vidal J, Kumru H. Non-invasive brain stimulation to promote motor and functional recovery following spinal cord injury. Neural Regen Res. (2017) 12:1933–8. doi: 10.4103/1673-5374.221143
7. Li Y, Luo X, Wan M, Li J, Wang H, Wei D, et al. The effectiveness of non-invasive brain stimulation on arousal and alertness in patients in coma or persistent vegetative state after traumatic brain injury: protocol of systematic review and network meta-analysis. Medicine (Baltimore). (2018) 97:e12321. doi: 10.1097/MD.0000000000012321
8. Liu M, Fan S, Xu Y, Cui L. Non-invasive brain stimulation for fatigue in multiple sclerosis patients: a systematic review and meta-analysis. Mult Scler Relat Disord. (2019) 36:101375. doi: 10.1016/j.msard.2019.08.017
9. Rektorova I, Biundo R. Non-invasive brain stimulation to treat cognitive symptoms of Parkinson's disease. Parkinsonism Relat Disord. (2019) 66:1–2. doi: 10.1016/j.parkreldis.2019.09.012
10. Viganò A, Toscano M, Puledda F, Di Piero V. Treating chronic migraine with neuromodulation: the role of neurophysiological abnormalities and maladaptive plasticity. Front Pharmacol. (2019) 10:32. doi: 10.3389/fphar.2019.00032
11. Toscano M, Celletti C, Viganò A, Altarocca A, Giuliani G, Jannini TB, et al. Short-term effects of focal muscle vibration on motor recovery after acute stroke: a pilot randomized sham-controlled study. Front Neurol. (2019) 10:115. doi: 10.3389/fneur.2019.00115
12. Marconi B, Filippi GM, Koch G, Giacobbe V, Pecchioli C, Versace V, et al. Long-term effects on cortical excitability and motor recovery induced by repeated muscle vibration in chronic stroke patients. Neurorehabil Neural Repair. (2011) 25:48–60. doi: 10.1177/1545968310376757
13. Caliandro P, Celletti C, Padua L, Minciotti I, Russo G, Granata G, et al. Focal muscle vibration in the treatment of upper limb spasticity: a pilot randomized controlled trial in patients with chronic stroke. Arch Phys Med Rehabil. (2012) 93:1656–61. doi: 10.1016/j.apmr.2012.04.002
14. Winstein CJ, Stein J, Arena R, Bates B, Cherney LR, Cramer SC, et al. Guidelines for adult stroke rehabilitation and recovery: a guideline for healthcare professionals from the American Heart Association/American Stroke Association. Stroke. (2016) 47:e98–169. doi: 10.1161/STR.0000000000000098
15. Celletti C, Suppa A, Bianchini E, Lakin S, Toscano M, La Torre G, et al. Promoting post-stroke recovery through focal or whole body vibration: criticisms and prospects from a narrative review. Neurol Sci. (2019) 41:11–24. doi: 10.1007/s10072-019-04047-3
16. Ward NS, Cohen LG. Mechanisms underlying recovery of motor function after stroke. Arch Neurol. (2004) 61:1844–8. doi: 10.1001/archneur.61.12.1844
17. Souron R, Baudry S, Millet GY, Lapole T. Vibration-induced depression in spinal loop excitability revisited. J Physiol. (2019) 597:5179–93. doi: 10.1113/JP278469
18. Rocchi L, Suppa A, Leodori G, Celletti C, Camerota F, Rothwell J, et al. Plasticity induced in the human spinal cord by focal muscle vibration. Front Neurol. (2018) 2:935. doi: 10.3389/fneur.2018.00935
19. Marconi B, Filippi GM, Koch G, Pecchioli C, Salerno S, Don R, et al. Long-term effects on motor cortical excitability induced by repeated muscle vibration during contraction in healthy subjects. J Neurol Sci. (2008) 275:51–9. doi: 10.1016/j.jns.2008.07.025
20. Fattorini L, Ferraresi A, Rodio A, Azzena GB, Filippi GM. Motor performance changes induced by muscle vibration. Eur J Appl Physiol. (2006) 98:79–87. doi: 10.1007/s00421-006-0250-5
21. Fugl-Meyer AR, Jääskö L, Leyman I, Olsson S, Steglind S. The post-stroke hemiplegic patient, I: a method for evaluation of physical performance. Scand J Rehabil Med. (1975) 7:13–31.
22. Sanford J, Moreland J, Swanson LR, Stratford PW, Gowland C. Reliability of the Fugl-Meyer Assessment for Testing Motor Performance in Patients Following Stroke. Phys Therapy. (1993) 73:447–54. doi: 10.1093/ptj/73.7.447
23. Rödén-Jüllig A, Britton M, Gustafsson C, Fugl-Meyer A. Validation of four scales for the acute stage of stroke. J Intern Med. (1994) 236:125–36. doi: 10.1111/j.1365-2796.1994.tb01274.x
24. Demeurisse G, Demol O, Robaye E. Motor evaluation in vascular hemiplegia. Eur Neurol. (1980) 19:382–9. doi: 10.1159/000115178
25. Hiengkaew V, Jitaree K, Chaiyawat P. Minimal detectable changes of the Berg Balance Scale, Fugl-Meyer Assessment Scale, Timed “Up & Go” Test, gait speeds, and 2-minute walk test in individuals with chronic stroke with different degrees of ankle plantarflexor tone. Arch Phys Med Rehabil. (2012) 93:1201–8. doi: 10.1016/j.apmr.2012.01.014
26. Fayazi M, Dehkordi SN, Dadgoo M, Salehi M. Test-retest reliability of Motricity Index strength assessments for lower extremity in post stroke hemiparesis. Med J Islam Repub Iran. (2012) 26:27–30.
27. Bohannon RW, Smith MB. Interrater reliability of a modified Ashworth scale of muscle spasticity. Phys Ther. (1987) 67:206–7. doi: 10.1093/ptj/67.2.206
28. Chen CL, Chen CY, Chen HC, Wu CY, Lin KC, Hsieh YW, et al. Responsiveness and minimal clinically important difference of Modified Ashworth Scale in patients with stroke. Eur J Phys Rehabil Med. (2019) 55:754–60. doi: 10.23736/S1973-9087.19.05545-X
29. Geiger M, Supiot A, Pradon D, Do MC, Zory R, Roche N. Minimal detectable change of kinematic and spatiotemporal parameters in patients with chronic stroke across three sessions of gait analysis. Hum Mov Sci. (2019) 64:101–7. doi: 10.1016/j.humov.2019.01.011
30. Özyurt MG, Shabsog M, Dursun M, Türker KS. Optimal location for eliciting the tibial H-reflex and motor response. Muscle Nerve. (2018) 58:828–33. doi: 10.1002/mus.26308
31. Valotassiou V, Papatriantafyllou J, Sifakis N, Tzavara C, Tsougos I, Psimadas D, Kapsalaki E, et al. Brain perfusion SPECT with Brodmann areas analysis in differentiating frontotemporal dementia subtypes. Curr Alzheimer Res. (2014) 11:941–54. doi: 10.2174/1567205011666141107125104
32. Valotassiou V, Papatriantafyllou J, Sifakis N, Tzavara C, Tsougos I, Psimadas D, et al. Clinical evaluation of brain perfusion SPECT with brodmann areas mapping in early diagnosis of Alzheimer's disease. J Alzheimers Dis. (2015) 47:773–85. doi: 10.3233/JAD-150068
33. Frantellizzi V, Morreale M, Pontico M, Francia A, Drudi FM, Farcomeni A, et al. 99mTc-HMPAO brain SPECT in the monitoring of cerebral vasculitis therapy. Rev Esp Med Nucl Imagen Mol. (2018) 37:211–7. doi: 10.1016/j.remn.2017.10.009
34. Mancini V, Mastria G, Frantellizzi VPT, Zampatti S, Carboni S, Iardina E, et al. Migrainous infarction in a patient with sporadic hemiplegic migraine and cystic fibrosis: a 99mTc-HMPAO brain SPECT study. Headache. (2019) 59:253–8. doi: 10.1111/head.13472
35. Thompson AK, Wolpaw JR. Targeted neuroplasticity for rehabilitation. Prog Brain Res. (2015) 218:157–72. doi: 10.1016/bs.pbr.2015.02.002
36. Norton JJS, Wolpaw JR. Acquisition, maintenance, and therapeutic use of a simple motor skill. Curr Opin Behav Sci. (2018) 20:138–44. doi: 10.1016/j.cobeha.2017.12.021
37. Celletti C, Sinibaldi E, Pierelli F, Monari G, Camerota F. Focal muscle vibration and progressive modular rebalancing with neurokinetic facilitations in post- stroke recovery of upper limb. Clin Ter. (2017) 168:e33–6. doi: 10.7417/CT.2017.1979
38. Heath CJ, Hore J, Phillips CG. Inputs from low threshold muscle and cutaneous afferents of hand and forearm to areas 3a and 3b of baboon's cerebral cortex. J Physiol. (1976) 257:199–227. doi: 10.1113/jphysiol.1976.sp011364
40. Ghosh S, Brinkman C, Porter R. A quantitative study of the distribution of neurons projecting to the precentral motor cortex in the monkey (M. fascicularis). J Comp Neurol. (1987) 259:424–44. doi: 10.1002/cne.902590309
41. Huerta MF, Pons TP. Primary motor cortex receives input from area 3a in macaques. Brain Res. (1990) 537:367–71. doi: 10.1016/0006-8993(90)90388-r
42. Brunetti O, Filippi GM, Lorenzini M, Liti A, Panichi R, Roscini M, et al. Improvement of posture stability by vibratory stimulation following anterior cruciate ligament reconstruction. Knee Surg Sports Traumatol Arthrosc. (2006) 14:1180–7. doi: 10.1007/s00167-006-0101-2
43. Lopez S, Bini F, Del Percio C, Marinozzi F, Celletti C, Suppa A, et al. Electroencephalographic sensorimotor rhythms are modulated in the acute phase following focal vibration in healthy subjects. Neuroscience. (2017) 352:236–48. doi: 10.1016/j.neuroscience.2017.03.015
44. Heiss WD. Radionuclide imaging in ischemic stroke. J Nucl Med. (2014) 55:1831–41. doi: 10.2967/jnumed.114.145003
45. Palmieri RM, Ingersoll CD, Hoffman MA. The hoffmann reflex: methodologic considerations and applications for use in sports medicine and athletic training research. J Athl Train. (2004) 39:268–77.
46. Noma T, Matsumoto S, Etoh S, Shimodozono M, Kawahira K. Anti-spastic effects of the direct application of vibratory stimuli to the spastic muscles of hemiplegic limbs in post-stroke patients. Brain Inj. (2009) 23:623–31. doi: 10.1080/02699050902997896
Keywords: stroke, focal muscle vibration, brain plasticity, spinal cord plasticity, motor recovery
Citation: Toscano M, Ricci M, Celletti C, Paoloni M, Ruggiero M, Viganò A, Jannini TB, Altarocca A, Liberatore M, Camerota F and Di Piero V (2020) Motor Recovery After Stroke: From a Vespa Scooter Ride Over the Roman Sampietrini to Focal Muscle Vibration (fMV) Treatment. A 99mTc-HMPAO SPECT and Neurophysiological Case Study. Front. Neurol. 11:567833. doi: 10.3389/fneur.2020.567833
Received: 30 May 2020; Accepted: 19 October 2020;
Published: 12 November 2020.
Edited by:
Paolo Tonin, Sant'Anna Institute, ItalyReviewed by:
Birgitta Langhammer, Oslo Metropolitan University, NorwayAntonio Cerasa, Consiglio Nazionale delle Ricerche (CNR), Italy
Copyright © 2020 Toscano, Ricci, Celletti, Paoloni, Ruggiero, Viganò, Jannini, Altarocca, Liberatore, Camerota and Di Piero. This is an open-access article distributed under the terms of the Creative Commons Attribution License (CC BY). The use, distribution or reproduction in other forums is permitted, provided the original author(s) and the copyright owner(s) are credited and that the original publication in this journal is cited, in accordance with accepted academic practice. No use, distribution or reproduction is permitted which does not comply with these terms.
*Correspondence: Massimiliano Toscano, bWFzc2ltaWxpYW5vLnRvc2Nhbm9AdW5pcm9tYTEuaXQ=
†These authors have contributed equally to this work