- 1Department of Brain and Behavioural Sciences, University of Pavia, Pavia, Italy
- 2University of Insubria, Varese, Italy
- 3University of Sassari, Sassari, Italy
- 4Department of Molecular Medicine, University of Pavia, Pavia, Italy
- 5Department of Drug Sciences, University of Pavia, Pavia, Italy
Cannabis has been used for centuries for recreational and therapeutic purposes. Whereas, the recreative uses are based on the psychotropic effect of some of its compounds, its therapeutic effects range over a wide spectrum of actions, most of which target the brain or the immune system. Several studies have found cannabinoid receptors in the auditory system, both at peripheral and central levels, thus raising the interest in cannabinoid signaling in hearing, and especially in tinnitus, which is affected also by anxiety, memory, and attention circuits where cannabinoid effects are well described. Available studies on animal models of tinnitus suggest that cannabinoids are not likely to be helpful in tinnitus treatment and could even be harmful. However, the pharmacology of cannabinoids is very complex, and most studies focused on neural CB1R-based responses. Cannabinoid effects on the immune system (where CB2Rs predominate) are increasingly recognized as essential in understanding nervous system pathological responses, and data on immune cannabinoid targets have emerged in the auditory system as well. In addition, nonclassical cannabinoid targets (such as TRP channels) appear to play an important role in the auditory system as well. This review will focus on neuroimmunological mechanisms for cannabinoid effects and their possible use as protective and therapeutic agents in the ear and auditory system, especially in tinnitus.
Introduction
Endocannabinoids (ECs; Figure 1) are a class of ubiquitous endogenous lipids regulating essential processes ranging from energy balance, to pain, to motor control, and involved in pathologies as diverse as (among others) schizophrenia, glaucoma, multiple sclerosis, and obesity (20). In the CNS, ECs influence synaptic plasticity (21, 22), modulate neuroinflammation (23), and affect neurogenesis (24) and may also affect neuronal activity by binding to neurotransmitter receptors and ion channels (25). These cellular effects are reflected in the EC modulation of several brain functions, including fear and anxiety (26), or memory (27). Overall, the standard arrangement in the brain appears to be the presence of multiple EC pathways affecting the same circuits, often with different or even opposing effect.
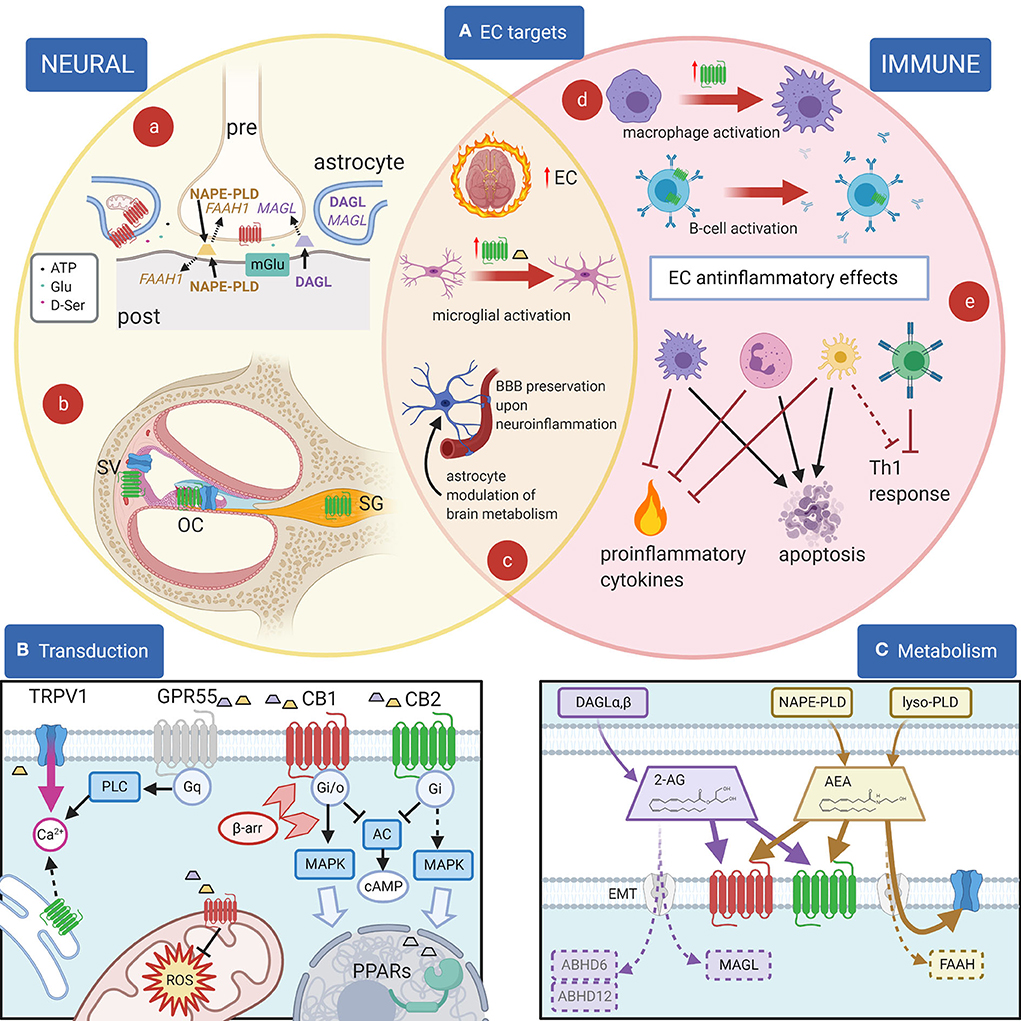
Figure 1. EC and their effects. (A): Principal EC targets in neural and immune systems, and in the cochlea. (a) In most brain areas, 2-AG (purple) is synthetized by DAGL-α in neuronal dendrites and somata and catabolized by MAGL in presynaptic terminals, where CB1R (red) are also present. 2-AG is produced postsynaptically in a Ca2+-dependent way upon activation of metabotropic receptors (blue) and inactivated presynaptically near its target (1). For AEA (yellow), on the other hand, the biosynthetic enzyme NAPE-PLD is both pre- and postsynaptic, and the catabolic enzyme FAAH-1 is predominantly postsynaptic (1). Astrocytes are also involved in synaptic effects through an EC modulation of gliotransmission, and in addition EC effects on astrocyte mitochondria contribute to neuronal metabolism regulation. (b) In the cochlea, CB2R (green) are found in the organ of Corti (OC), basal cells of the stria vascularis (SV) and spiral ganglion (SG), whereas TRPV1 channels (blue) are found in the organ of Corti and marginal cells of the stria vascularis. (c) During neuroinflammation, several changes are seen in the EC system. The overall EC production increases. Activated microglia increases AEA production (yellow trapezoid) and CB2R expression (green). Astrocytes become activated and BBB is affected (both effects are counteracted by EC responses). (d) Cell activation may change CB2R expression as in macrophages (purple) (2) or CB2R subcellular localization as in B lymphocytes (blue) (3). (e) Anti-inflammatory EC responses in immune cells include the block of Th1 responses due to direct effects on T cells (green) and indirect effects on dendritic cells (yellow), apoptosis induction on several cell types, and the inhibition of proinflammatory cytokines and factors. (B): Principal EC receptors and their main intracellular pathways. Both 2-AG (purple trapezoid) and AEA (yellow trapezoid) act on CB1R and CB2R, which are class A GPCRs (4) coupled to Gi/o G-proteins (5, 6), reducing cAMP concentration (7). β-Arrestin binding (light red arrowheads) induces CBR internalization and switches receptor coupling, especially for CB1, also activating MAP kinase pathways (8–10) linked to nuclear effects (large light blue arrow). MAPK pathways are also activated through Gi βγ action (dotted line), both by CB1R and by CB2R (11). Functional CBRs have been also found in intracellular compartments such as the outer mitochondrial membrane (12), where they modulate cell energetic balance (13), and ROS production (14) or endoplasmic reticulum, where they may induce Gq-related Ca2+ release from intracellular stores (3). Moreover, ECs or related lipids activate nuclear PPARs (15). TRPV1 channels are often colocalized with CB1R and/or CB2R and activated by cytoplasmic ECs (16), increasing cytosolic Ca2+. Finally, orphan receptors may activate other pathways, e.g., GPR55 is linked to Gq-PLC and therefore contributes to cytosolic Ca2+ increase. Most cells only express a subset of these pathways. (C): Metabolism of 2-AG and anandamide (AEA). 2-AG (purple) is produced from DAG by DAG lipases (DAGLα and β). Biosynthesis of AEA (yellow) is more complex and may involve hydrolysis of NAPE membrane phospholipids by NAPE-PLD (which directly generates AEA) or sequential action of several enzymes (not shown), followed by a lyso-PLD (17). Although lipophilic, ECs have membrane transport mechanisms (EMT, light gray) (18). EC binding sites on CB1 (red) and CB2 (green) are extracellular, whereas on TRP channels (blue) the site is intracellular. Degradation of AEA is mainly due to FAAH, whereas 2-AG is primarily degraded by MAGL, and less by ABHD6 and ABHD12 (19). Created with Biorender.
In the immune system, ECs affect cell proliferation, migration, differentiation, cytokine production, and apoptosis (28). The two responses, immune and neural, interact in neuroinflammation, where ECs play major roles (29). Earlier studies suggested that neural effects of cannabinoids are mediated by CB1R activation (30) whereas immune effects are mediated by CB2R (31). However, it is important to stress that the separation of these biological actions is not as clear-cut as initially suggested (32), and other receptors can also be activated by ECs. The dizzying complexity of cannabinoid pharmacology (see Supplementary Tables 1, 2) requires a deep knowledge of the precise “fingerprint” of the molecular pathways affected by each compound, in each organ and each species, to dissect its effects.
Cannabinoid Pharmacology
The pharmacology of cannabinoids is very complex, for several reasons. First, more than one hundred phytocannabinoids (33) and at least 13 ECs (25) have been identified. Second, their lipidic nature makes unraveling their molecular interactions more difficult than for conventional transmitters (34). Third, CBRs are connected to several intracellular pathways (Figure 1B) and may produce different (even opposite) results depending on the particular ligand and its concentration (8, 9, 35) and on the cell repertoire of signal transduction molecules (36).
ECs are one of the four families of bioactive lipids (together with classical eicosanoids, SPMs, and lysoglycerophospholipids/sphingolipids), which are generated from PUFA precursors esterified into membrane lipids (37). The EC system includes CBRs, their endogenous ligands, and the proteins involved in EC formation, transport, and degradation.
The first discovered and best-characterized ECs are AEA and 2-AG (38–40). Several other EC lipid mediators (41–43) [and a family of EC peptides, named “pepcans” (44)] have also been described (see also Supplementary Table 1), but their endogenous functions have been less characterized.
ECs (Figure 1C) are produced “on demand” from membrane lipids by several Ca2+-dependent enzymes (17), and metabolic pathways for production, transport, and degradation differ for the various ECs, making it possible for cells to tailor their local EC repertoire (45) by regulating their local concentrations through modulation of their biosynthesis, transport, and degradation (46). Once released, ECs are rapidly deactivated by intracellular enzymes (47): AEA by FAAH1 and 2 [the latter not expressed in rodents (1)], and 2-AG mainly by MAGL, and less by ABHD6 and ABHD12 (19). In addition, ECs may be transformed in non-EC bioactive metabolites [e.g., by COX-2 (48)].
ECs (Figure 1B) bind and activate two specific G-protein-coupled cannabinoid receptors, CB1R and CB2R (49–51), plus additional targets (52), such as TRP channels (53), PPARs (54–57), and “orphan” G-protein coupled receptors such as GPR18 and GPR55 (58, 59). Most EC are able to activate both CB1R and CB2R, although with different potency and effects (60), whereas nonclassical targets may interact with limited EC subsets (Supplementary Table 1) and also non-EC ligands. A clear example is TRPV1, which is activated by AEA binding to a cytoplasmic site (16) but is also sensitive to other stimuli such as heat, vanilloids, protons, N-acyl amides, and arachidonic acid derivatives (61).
CB1R and CB2R are class A (rhodopsin-like) GPCRs (4), and both couple to Gi/o G-proteins (5, 6), reducing cAMP concentration (7). However, the coupling between CBRs and biochemical pathways is complex and context-dependent. First, CB1Rs may form homo- or heterodimers with other GPCRs (62), such as (among others) CB2Rs (63), A2A adenosine receptors (64), D2 dopamine receptors (65), μ opioid receptors (66), and orexin-1 receptors (67), whereas CB2R may dimerize with the CXCR4 chemokine receptor (68) and GPR55 (69). The presence of CB1R/CB2R heteromers makes it impossible to clearly separate the biological responses of CB1R and CB2R in vivo.
Second, CBRs show dimerization- and agonist-biased response, due to conformation-dependent binding by β-arrestins (10). Besides blocking interactions with Gi/o proteins, β-arrestin effects include CBR internalization and ERK pathway/Gs protein activation (9), so that cAMP levels may increase instead of decreasing depending on CBR receptor bias. Receptor coupling flexibility appears more limited (although not absent) for CB2Rs, which mainly activate Gi proteins, whereas CB1Rs may couple to Go, Gs, Gq, and G12/13, thus activating a very diverse network of responses (8). Both receptors are in addition able to activate ER stress pathways linked to autophagy (70).
The β-arrestin-dependent internalization of plasma membrane CBRs is linked to receptor degradation (9); however, functional CBRs have been found in the outer mitochondrial membrane (12), and in the endoplasmic reticulum, endosomes, lysosomes, and nuclear membrane (3). Subcellular localization affect CB-related responses: mitochondrial localization allows CBRs to modulate cell energetic balance (13), and ROS production (14), whereas endolysosomal localization is correlated with inflammation and phagocytosis (71). Moreover, intracellular receptor sites will be inaccessible to membrane-impermeant cannabinoid agonists and antagonists.
Besides G-protein-coupled receptors, TRP nonselective cation channels are being increasingly recognized as an integral part of the EC system (ionotropic EC receptors): six of the 28 TRP channels are sensitive to cannabinoids (53). Among these, TRPV1 is the most studied, mainly due to its expression in nociceptors and role in pain-related processes: TRPV1 channels are colocalized with CB1R and/or CB2R in several types of cells, and TRPV1 block or desensitization underlies analgesia (72); the analgesic and antihyperalgesic effects of phytocannabinoids are, at least in part, mediated by this channel (53).
EC System in the Brain
CBRs are expressed in most tissues of the body (73) and are by far the most abundant type of G-protein-coupled receptors in the mammalian brain (74). CB1R is predominantly expressed in the CNS (75), at comparable levels as glutamate and GABA receptors (74, 76). On the other hand, CB2R was originally thought to be restricted to immune and hematopoietic cells (77, 78), but more precise localization tools have subsequently allowed to assess its expression in other systems, including the nervous system (79) and the inner ear (80). CB2R expression in the healthy brain is in fact hundreds of times less than for CB1R but is strongly upregulated under pathological conditions (81). Localization, splice variants, and physiology of CBRs appear to be highly species-dependent (73), thus complicating result comparisons between animal and human studies.
CB1R neuronal effects are well known and have been extensively covered in several exhaustive reviews (49, 82, 83). Glial responses are less completely characterized but appear important especially in the presence of neuroinflammation (84), where EC tone is elevated (85). Neuroinflammation is a protective brain defense response that can however degenerate into a chronical state involved in the pathophysiology of several neurological and psychiatric disorders (86).
In neurons (Figure 1Aa), the classical EC effect is retrograde inhibition mediated by presynaptic neuronal CB1Rs and postsynaptically produced 2-AG: CB1R activation inhibits the release of the presynaptic transmitter (22), causing short-term DSE on excitatory neurons, or DSI on inhibitory neurons (87). This mechanism has been dissected in the DCN molecular layer, where glutamatergic parallel fibers carrying non-auditory signals contact fusiform cells and glycinergic cartwheel cells (which in turn provide feedforward inhibition to fusiform cells) (88). Fusiform cell output is shaped by plasticity in the molecular layer circuits, which collectively generate “negative images” of expected sounds to be attenuated at fusiform apical dendrites (89). Plasticity changes in this circuit have been correlated with tinnitus onset (90, 91). Cartwheel cells release EC from their dendrites upon stimulation, thus inducing DSE at parallel fibers (92), whereas fusiform cells do not; therefore, activation of cartwheel cells depresses its parallel fiber input, gradually reducing their feedforward inhibition (93). In fusiform cells, ECs are involved in acetylcholine-induced plasticity changes at parallel fiber synapses (94) which have been correlated with tinnitus (95). Prolonged exposure to high doses of salicylate (a well-known tinnitus inducer) increases EC release in the DCN, thus changing molecular layer plasticity (96). Unfortunately, cannabinoid modulation of this circuit has not yielded effective tinnitus treatments [see discussion in (97)].
For AEA, on the other hand, the biosynthetic enzyme NAPE-PLD is both pre- and postsynaptic, and the catabolic enzyme FAAH-1 is predominantly postsynaptic (1). Postsynaptic production of AEA produces a “tonic” retrograde inhibition at some synapses, which is shut down by neuronal inactivity through upregulation of FAAH1 (98); presynaptic production feeds instead into an anterograde mechanism. In addition, in the hippocampus, NAPE-PLD is localized in intracellular membrane cisternae of axonal Ca2+ stores (99) and AEA may act as an intracellular messenger by activating TRPV1 intracellular binding site.
Like neurons, glial cells can synthesize ECs in response to physiological or pathological stimuli (100, 101). In astrocytes, more than 70% of CB1Rs are found at perivascular endfeet, and EC activation has been found to modulate brain energy consumption (102) through EC effects on astrocyte mitochondria (103). At synapses, astrocytes express both DAGLα and MAGL and may display Ca2+-dependent EC release, which modulates synaptic response (104); conversely, astrocytic CB1R activation may induce Ca2+-dependent release of Glu (105), ATP, or D-serine (106) in response to synaptic EC. Astrocyte EC effects have been found to be involved in the regulation of sleep in the PPT (107) and in the regulation of circadian rhythms in the suprachiasmatic nucleus (108). These latter effects may be relevant for tinnitus given its association with sleep disturbances (109) and its circadian modulation (110).
Neuroinflammation is a brain reaction aimed at counteracting acute damage, restoring the homeostasis and limiting brain parenchyma injury, and includes microglial activation, reactive astrogliosis, production of inflammatory mediators, BBB breakdown, and subsequent brain infiltration of circulating immune cells (111). Neuroinflammation dysregulation may turn microglia and astrocytes in uncontrolled sources of inflammatory mediators, which may worsen damage progression.
A growing body of data suggest that EC are able to exert immunoregulatory and anti-inflammatory properties (112–114), by decreasing the production of NO, ROS/RNS, free radicals, and pro-inflammatory cytokines in activated glial cells, while switching microglia toward anti-inflammatory phenotypes (115–118). Remarkably, the increase in EC concentration and microglial CB receptors during neuroinflammation may yield a neuroprotective negative feedback mechanism aimed at limiting inflammatory responses.
The main brain source of ECs in neuroinflammatory conditions is microglia (119, 120), the resident immune cells of the CNS (121–123). Consistently with its immune role and nature, microglia express DAGL-β and (mainly) ABHD12 instead of the neuronal DAGL-α and MAGL (124), and while CB1Rs are expressed at low levels and mostly located intracellularly (120), microglia is the main CB2R-expressing cell in the brain (125). Microglial CB2R expression may increase up to 100 fold upon inflammation or tissue injury (126), and microglial Ca2+ increases [e.g., from P2X7 receptor activation (127)] and directly increases DAGL, thus increasing the production of 2-AG (128), which during neuroinflammation becomes 20-fold higher in microglia than in other brain cells (120). Mounting evidence suggests that the EC system might represent a promising tool to modify (micro)glial activity and profiles in order to achieve benefits for neuroinflammatory diseases (104). Indeed, CB2Rs can downregulate astrocyte and microglial cell overactivation during neuroinflammatory disorders, thus protecting them (129); selective depletion of MAGL in astrocytes attenuates LPS-induced neuroinflammation [(130), and CB2R upregulation and activation of EC signaling pathways have been associated with a restoration of tissue homeostasis in neuroinflammatory conditions (118, 131).
Brain CB2Rs have been less studied than CB1Rs (79), mainly due to the delay in the availability of sensitive genetic and molecular tools (126, 132, 133). In the CNS, CB2Rs are chiefly expressed on microglia (134, 135), and to some extent on astrocytes, oligodendrocytes, progenitor neural cells, and neurons (136–138); neuronal CB2R is mainly postsynaptic, differently from CB1R (137). In human, the brain only expresses one CB2R isoform (CB2RA) whereas a second one (CB2RB) is expressed in the immune system (139); rats express two additional isoforms (CB2RC and CB2RD) present neither in mice nor in humans (126), and their CB2R expression is lower and with a different distribution from mice (140). Lack of CB2R brain expression was incorrectly inferred by methods only evidencing non-brain isoforms or with insufficient sensitivity (126).
Microglial actions range from protection against damaging signals altering CNS homeostasis through phagocytosis, release of proinflammatory cytokines, and recruitment of circulating immune cells [reviewed in (141)], to controlling neuronal proliferation and differentiation [through selective neuronal phagocytosis and release of neurotrophic and neurotoxic factors reviewed in (142)], to modulating neuronal plasticity and memory [through neurotrophin release and selective synaptic pruning reviewed in (141, 142)]. In order to fulfill all these tasks, microglia are extremely plastic cells that readily change their phenotypes on demand; microglial phenotypes, previously crammed into an M1–M2 gradient to fit a classical macrophage activation model (143), are now recognized to be much more diverse (144) and influenced by the brain region (145), species (146), age (147), gender (148), and physiopathological state (149). In particular, neurodegenerative diseases appear to associate with specific microglial phenotypes which release pro-inflammatory mediators, as well as contributing to prolonged oxidative stress, leading to chronic neuroinflammation, which in turn drives neurodegeneration (141, 150, 151).
As regards hearing loss, which is a risk factor for tinnitus, chronic inflammation is seen as a major player in presbycusis [reviewed in (152)] and has been found to be associated with poorer hearing in a population-based cross-sectional study (153). Moreover, in mice, microglial ablation and TNF-alpha antagonism (154) both decrease tinnitus signs, and TNF-alpha KO mice are resilient to noise trauma-induced tinnitus (154). In human, gene polymorphisms in both TNF-alpha (155) and IL-6 (156) have been found to increase tinnitus risk in an elderly population with a history of occupational noise exposure. Neuroinflammation (and its dysregulation) appears therefore as a promising candidate mechanism for tinnitus susceptibility, and its modulation by cannabinoids may provide novel therapeutic targets. A caveat regarding neuroinflammation as a target is the complexity emerging from single-cell studies (157), which could underlie a heterogeneity similar to that observed in most multifactorial inflammatory disorders [e.g., rheumatoid arthritis (158), Menière's disease (159), and IBD (160)].
Besides neurons and glial cells, neuroinflammation involves cells of the immune system, where EC cellular mechanisms differ from neuronal ones. Cannabinoid immunomodulatory effects are complex but appear to be largely mediated through CB2Rs, whose expression on immune cells is usually higher than that of CB1R (161, 162). Moreover, nonclassical cannabinoid targets such as TRP channels (53) and PPARs (15) are well-known as key regulators of the immune response (163–165). It is interesting that EC responses in the cochlea (see below) appear more similar to those observed in the immune system than in the nervous system.
In human immune cells, CB2R is expressed most in B cells, followed by NK cells, monocytes, neutrophils, and finally T cells (134, 166, 167). Peripheral blood T cells, monocytes, and dendritic cells only express intracellular CB2R (168), whereas naïve peripheral blood B cells also express these receptors on the cell surface and lose it upon activation (169). Intracellular CB2Rs in immune cells have been associated with Ca2+ release from stores (3).
CB2R activation in immune cells regulates all three major MAPKs (12) and decreases DNA binding for various nuclear factors (170), which results in the downregulation of critical immunoregulatory genes including IL-2 (171, 172). Overall, these major signaling networks play important roles in CB2R-mediated effects on immune cell functions including migration, proliferation, differentiation, apoptosis, and cytokine production (28). Generally, effects of the EC system on immune cells appear directed toward an anti-inflammatory action, although the context-dependent action of cannabinoids may support different responses in different cell types and states (62, 173–176).
As regards neuroinflammatory responses, a major player is the Toll-like receptor (TLR) system (177). TLRs are able to recognize pathogen-associated and damage-associated molecular patterns (PAMPs and DAMPs), and several of their effects appear to be counteracted by ECs [especially through CB2R-related mechanisms (11)]. Since cochlear damage has also been found to induce TLR4-responses (178), similar protective effects could be expected on the cochlea.
Cannabinoids and Tinnitus
Cannabinoids have been considered as potential treatment for tinnitus percept and/or distress, and with the legalization of light cannabis (L.242/2016 as regards Italy), several tinnitus sufferers are turning to it as a possible DIY remedy. Interest in cannabinoids as possible treatment for tinnitus has been motivated by several reasons. Early models of tinnitus stressed its similarities with neuropathic pain (179) and with epilepsy (180), both of which can be modulated by cannabinoids (181, 182).
The association between tinnitus and marijuana use in humans has been studied with contrasting results. In one study on health problems related to illicit drug use from the NSDUH database (n = 29,195) (183), tinnitus did not show any association to marijuana use (whereas an association was found with hallucinogens and inhalants); in a second, cross-sectional study on the NHANES database (n = 2,705) (184), a correlation was found between tinnitus and cannabis use, although not between cannabis use frequency and tinnitus severity, and the authors concluded that it was not possible to differentiate between causal association (cannabis use increases tinnitus prevalence), reverse causal association (tinnitus sufferers use more cannabis than non-sufferers), and association due to external common cause (i.e., anxiety, which increases both tinnitus risk and cannabis use).
Animal studies [reviewed in (97)] suggest that cannabinoids do not reduce, and may even favor, tinnitus percept. Similarly, tinnitus in humans has been sporadically observed in association with abuse of synthetic cannabinoid mixtures (185, 186).
These seemingly contradictory results arise from two inherent complexities in the problem under study. First, the responses to cannabinoids (even for the same compound mixtures) strongly depend on drug formulation, administration route, and concentration. Second (Figure 2), tinnitus can result from many different mechanisms which are often hard to identify.
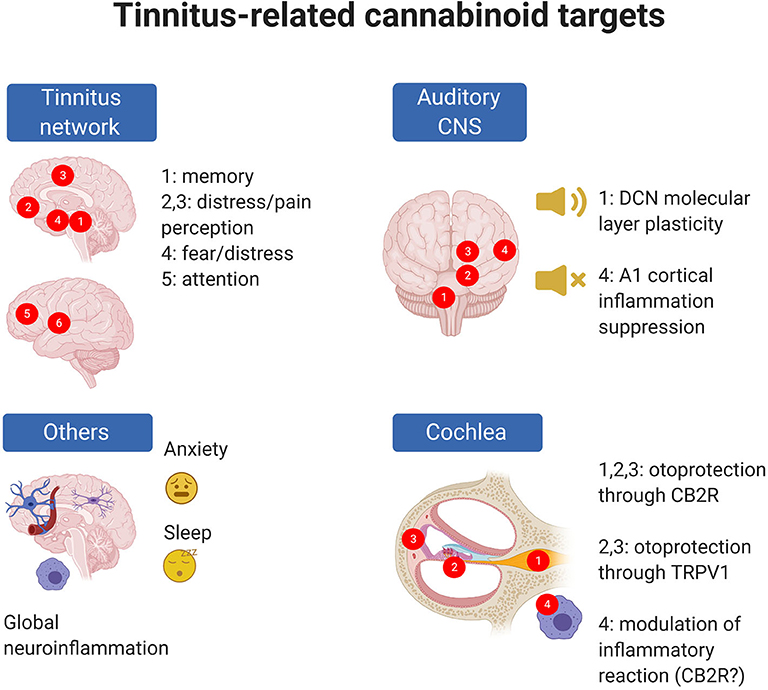
Figure 2. Tinnitus-related EC targets are present in the cochlea and central auditory system but also in CNS circuits altered in tinnitus; moreover, ECs may target phenomena which are known to be associated with tinnitus risk (e.g., anxiety) even though precise cellular mechanisms are uncertain. In panel “Tinnitus network,” numbers indicate as follows: 1: parahippocampal cortex; 2: ventromedial prefrontal cortex; 3: cingulate cortex; 4: amygdala; 5: dorsolateral prefrontal cortex; 6: insula (from 273). In panel “Auditory CNS” numbers indicate as follow: 1: cochlear nuclei; 2: auditory pons and midbrain; 3: medial geniculate body; 4: auditory cortex. In panel “Cochlea,” numbers indicate as follows: 1: spiral ganglion; 2: organ of Corti; 3: stria vascularis; 4: cochlear macrophages. Created with Biorender.
As regards the first complexity, it is important to stress that isolated and characterized phytocannabinoids, present in Cannabis sativa L. and a few other plant species (187, 188), include about 120 molecules (189), the most studied of which are Δ9-THC, mainly responsible for cannabis psychoactive effects (55), and cannabidiol (CBD), the major non-psychotropic component (190).
After the explanation of the structure–activity relationships in the Δ9-THC series (191, 192), a large and heterogeneous array of cannabimimetic compounds (Supplementary Tables 1, 2) have been synthesized (193, 194) including cannabinoid receptor agonists and antagonists (195), as well as drugs acting on EC metabolism (18, 196). Although, for several of them, dangerous health effects and strong potential for abuse and addiction greatly limit therapeutic use (197–199), several synthetic and phyto-cannabinoids are currently under clinical evaluation for different pathological conditions (see Table 1).
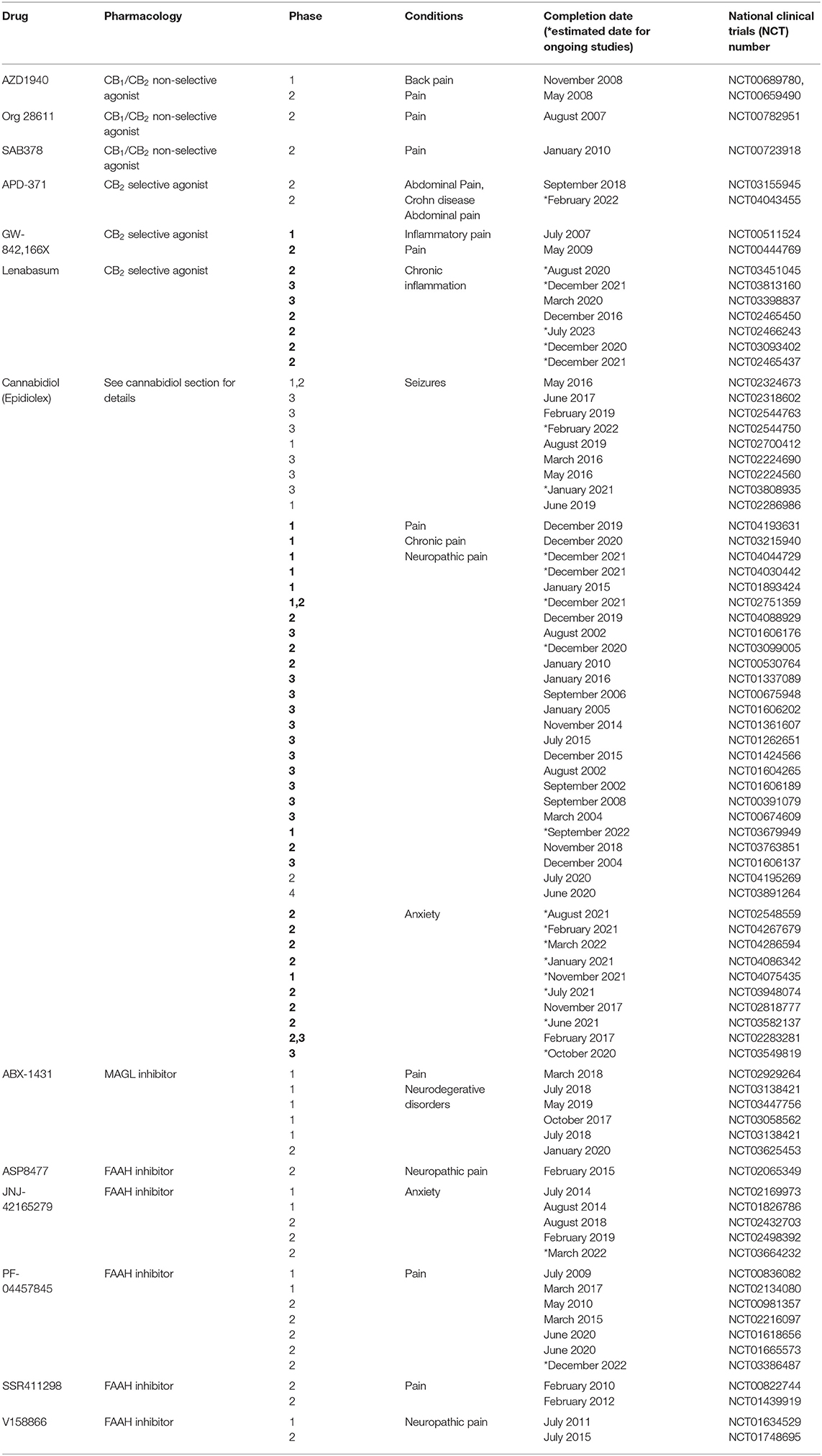
Table 1. Major clinical trials based on pharmacological treatment targeting the endocannabinoid system (updated to July 21, 2020).
Cannabinoid bioavailability varies significantly by their formulation and route of administration (200, 201) and is also affected by poorly controllable factors such as subjective inhalation characteristics (200, 202, 203) or hepatic first-pass metabolism (202, 204–206). This is particularly relevant because the expansion of legal use of cannabinoids, for medical and nonmedical purposes, has substantially increased the types of commercially available preparations (207).
Second, besides the intrinsic complexities of cannabinoid pharmacology, the main problem in attempting a pharmacological approach to tinnitus is the lack of a clear unifying causative hypothesis for this condition (208, 209). Current models of tinnitus include (1) a peripheral trigger [which is assumed to be reduced or altered cochlear input (210), even if transient (211) or “hidden” [but see (212)], or possibly a somatosensory trigger (210, 213)]; (2) an aberrant compensatory response in the brainstem [most likely more complex than a simple “gain increase” (91, 210, 214) as was initially postulated to compensate for reduced input (215)]; and (3) a reconfiguration of cortical pathways including auditory, attentional, salience-related, and emotion-processing networks [which is thought to be necessary for the tinnitus percept to emerge to consciousness (216, 217)]. Given the absence of a causative hypothesis for tinnitus, in this review we will consider cannabinoid effects linked to both tinnitus and its main risk factors such as hearing loss or anxiety.
In animal models, tinnitus may be induced by noise trauma or ototoxic drugs such as salicylate (218). In humans, tinnitus is associated with several risk factors such as hearing loss, head trauma, and endocrine and immune dysregulation (208); however, the association between risk factors and tinnitus is far from linear. For example, although hearing loss is the main risk factor for tinnitus, it is not always accompanied by it, and tinnitus may be present without hearing loss (208). Non-auditory brain circuits also play important roles: in particular, tinnitus shows comorbidity with anxiety and depression (208, 219) and chronic tinnitus is associated with changes in attentional, memory, and limbic circuits (220, 221). The hypothesis explaining the involvement of non-auditory circuits includes a misdirection of attention which stays anomalously focused on the tinnitus percept (216), the involvement of limbic circuits encoding distress (220, 221) and the “replaying” of phantom sounds from memory in the absence of real percepts (220, 221).
At each of the levels thought to be associated with tinnitus onset and chronicization there are both well-known and potential cannabinoid targets. EC mechanisms have been found in the auditory brainstem, and particularly in the DCN, which is thought to be a major site of tinnitus onset (91, 222, 223). These neuronal, CB1R-based mechanisms (see previous section for a discussion of DCN effects) were considered very promising for a cannabinoid-based tinnitus treatment; unfortunately, animal studies displayed no effects, or even tinnitus increase, upon treatment [see discussion in (97)].
In addition to these targets, however, several other EC mechanisms (mainly related to inflammation) are present in the auditory system and in other CNS regions important for tinnitus (Figure 2). A protective EC mechanism is present in the cochlea (224, 225). Moreover, animal studies show inflammatory responses in the auditory cortex after tinnitus induction (154, 226), and inflammatory responses in the cochlea (154, 227, 228) and cochlear nuclei (229–232) after tinnitus-inducing treatments. Neuroinflammation may uncover novel EC-related therapeutic strategies, given the well-known anti-inflammatory effect of several cannabinoid drugs and pathways (see previous section).
In the auditory system, EC receptors and biosynthetic enzymes have been observed in several species and at several levels, and EC system modulation affects hearing at various levels. Moreover, several immune components and mechanisms known to be affected by EC modulation are also present in the auditory system, both peripheral and central. In the mammalian auditory system, EC system components or effects have been found in the cochlea (80), cochlear nuclei (93, 96, 233), MNTB (234), inferior colliculus (235, 236), and auditory cortex (237).
The hearing phenotypes of knockout mice for CB1R (238) and ABHD12 (239) have been characterized. In CB1R KO mice, high-frequency hearing is reduced but gap detection is improved, suggesting a change in auditory processing (238) or attentional modulation of perception, since in humans, chronic cannabis use is associated with attention-modulated deficit in PPI (240). Of relevance for tinnitus, CB1R KO mice also exhibit increased anxiety responses (241).
ABHD12 KO mice (239) and human ABHD12 nonsense mutations (242) display progressive hearing loss within PHARC syndrome. The absence of functional ABHD12 removes a catabolic pathway for 2-AG (see Figure 1); although the causative link between mutation and phenotype is still missing, a pro-inflammatory phenotype displaying microglial activation is observed (243), consistent with the expression of ABHD12 in both resting and activated microglia (242). Moreover, in the ABHD12 KO mouse the AA-related lipidome displays significant brain region-dependent changes (239) and macrophages increase LPS-induced cytokine production (244). On the other hand, the selective block of ABHD12 in adult mice does not induce hearing loss, suggesting developmental effects (245).
KO mice for CB2R (246) and other EC system components (239) are available, but their hearing has not been characterized; CB2R KO mice, on the other hand, display significant memory alterations (247).
In the cochlea, CB1R mRNA has been detected, and it decreases upon tinnitus-inducing salicylate treatment (248). However, the role of CB1Rs in the cochlea is still uncertain. On the other hand, CB2Rs have been found in rodent hair cells and pillar and Deiters' cells, spiral ganglion and nerve, and stria vascularis basal cells (224), and their expression increases upon cisplatin administration (80). Cisplatin is known to be strongly ototoxic by inducing cochlear inflammation (249), and CB2R block or knockdown makes the cochlea more sensitive to cisplatin ototoxicity (224): moreover, treatment with the CB2R antagonist AM630 is in itself proinflammatory, suggesting the presence of a cytoprotective EC tone in the cochlea (224). In addition, EC protective role in the cochlea has been found to involve TRPV1 activation: TRPV1 channels are expressed in hair cells (especially toward the apical pole), pillar, and Deiters' cells and in the marginal cells of the stria vascularis (250). The TRPV1 agonist capsaicin increases cochlear CB2R expression, and a CB2R-dependent mechanism induces the activation of STAT3; on the other hand, cisplatin induces the activation of proapoptotic factor STAT1 (225). The protective effect of capsaicin, which transiently induces STAT1 and TTS (225), is most likely due to the strong desensitization it induces on TRPV1 channels after a transient activation, similar to its effect in pain treatment (251).
CB1Rs are present in both ventral (VCN) and dorsal (DCN) cochlear nuclei of the rat; in the VCN, their role is unclear, but their expression decreases upon salicylate treatment, which induces tinnitus (233). In the DCN, salicylate does not change CB1R expression (233) but alters EC response on cartwheel cells (96). It is interesting to note the presence of CB1R (252) and CB2R (253) in the IV ventricle choroid plexus, especially because CB2R promote neural stem cell proliferation (254) and neurogenesis was observed in cochlear nuclei after deafferentation (255). In both man (256) and rat (257), there is a variable direct contact between the DCN surface and branches of the choroid plexus, where ECs released in the DCN molecular layer (92) could reach the plexus, possibly modulating its immune gate function (258).
As regards cortical effects important for tinnitus treatment, it is well known that anxiety (181) and attention (259) are strongly affected by cannabinoids. A point to be remembered is that, although cannabis use is associated with an acute anti-anxiety effect (260), chronic cannabis use may dramatically worsen anxiety (261, 262), thus exacerbating tinnitus severity. The anxiety-inducing effect of cannabis is correlated with its Δ9-THC content, and Δ9-THC alone may induce anxiety and paranoia (263); on the other hand, CBD appears to have opposite effects on anxiety (264) and is currently under clinical evaluation for the treatment of anxiety, psychosis, and posttraumatic stress disorder (190, 265, 266).
These data show that cannabinoid effects of possible relevance for tinnitus are very diverse and include anti-inflammatory, protective reactions and selective circuit modulation of “auditory context.” Since the anti-inflammatory route is starting to be explored as a possible therapeutic target in hearing loss (152) and tinnitus (154), interest has been raised for cannabinoids as a treatment option, and in particular for CBD, owing to its good toxicological profile in humans and lack of psychotropic effects. The recent availability of CBD preparations underlies anecdotal use reports by tinnitus patients; however, no controlled human studies have been performed yet.
Cannabidiol (CBD) is currently under clinical evaluation for the treatment of pain, anxiety, depression, sleep disorders, PTSD, headaches, and seizures (see Supplementary Table 1), all conditions which display analogies or associations with tinnitus (97, 179, 208). Despite such a wide spectrum of potentially interesting pharmacological properties, the practical effects of CBD on tinnitus are still underexplored.
Indeed, as of today the only study using CBD investigated the effects of a THC-CBD 1:1 mixture on noise trauma-induced tinnitus in the rat, showing no effects of daily treatment on tinnitus animals, and actually suggesting that cannabinoids might favor tinnitus onset, since treatment increased the fraction of animals showing tinnitus signs (267). These results agree with the effects of synthetic CB1R agonists (WIN55, 212-2, CP55,940, and ACEA) which have been tested in animal models of salicylate-induced tinnitus, with negative results [rat: (268); guinea pig: (269)]. It has to be remembered, however, that co-administered CBD and THC interact in a very complex way, and cannabinoid mixtures exert effects which may be very different from the simple combination of the effects of each drug per se (270). One example is CB1R activation in the cerebral cortex and hippocampus, associated with effects on cognition and memory (271): in this model, CBD is able to counteract THC-induced memory impairment (272).
In general, the pharmacodynamic of CBD appears particularly complex, with over 65 identified molecular targets, and different mechanisms proposed to explain its actions (190, 273, 274). Here we summarize only the CBD targets which may bear relevance for tinnitus.
On CB1R/CB2R, CBD has a very low affinity (in the μM range) and shows little agonist activity; on the other hand, it seems to antagonize CB1/CB2 synthetic agonist action with KB values in the nM range (275). It has been suggested that CBD acts as negative allosteric modulator of CB1R and as antagonist/inverse agonist of CB2R (276); in addition, it may indirectly affect CBR function by inhibiting FAAH activity, thus increasing endogenous anandamide levels (277, 278). For example, CBD neuroprotective effect after cerebral hypoxia–ischemia in immature pigs involves CB2R activation (279) and may be therefore due to EC increase rather than to a direct receptor effect.
Besides these effects, CBD acts as antagonist/inverse agonist of GPCR3, GPCR6, GPCR18, and GPCR55 (33, 280) and modulates serotonergic transmission acting as an allosteric agonist of 5HT1A receptor, a partial agonist of 5HT2A, and an allosteric inhibitor of 5HT3A (281–283). CBD protective effects on a BBB permeability model (284) required PPARγ and 5HT1A and were independent of CBRs. Similarly, CBD anti-depressant and anxiolytic effects also appear independent from CB2R (285) and linked to 5HT1A activation.
In the μM range, CBD may also activate adenosine A1 (286) and A2A receptors (287), activate glycine α1 (288) and α3 receptors (289), inhibit α7 nicotinic acetylcholine receptors (290), and allosterically modulate μ and δ opioid receptors (half maximal inhibition was observed at ~10 μM) (291). As a caveat, since CBD concentrations > 20 μM are unlikely to be attained in vivo (292), not all the described CBD pharmacological activities are likely to be physiologically meaningful.
Modulation of α7 nAChRs may be relevant for tinnitus since these receptors are expressed in cortical and hippocampal neurons and affect cognition and memory [reviewed in (293)]; moreover, these receptors are also expressed in microglia (294) and macrophages (295) and are involved in the vagal-mediated cholinergic anti-inflammatory response signaling through the JAK2/STAT3 pathway, decreasing levels of pro-inflammatory cytokines, such as TNF-α, IL-1β, and IL-6 and increasing levels of anti-inflammatory cytokines such as IL-10 (295–298).
Finally, CBD may affect several ion channels including voltage-dependent Na channels (299), T-type Ca channels (300), and TRPV1 and TRPV2 channels (301). In particular, CBD can act on TRPV-1, exhibiting an action similar to capsaicin, both in vitro (302) and in an animal model of acute inflammation (303). This is relevant since capsaicin is able to exert protective effects on cochlear inflammatory damage (225), and therefore, CBD may exert similar otoprotective actions.
Conclusions
1. Cannabinoids are involved in neural processing in the healthy auditory system, in protective reaction to auditory damage, and in most non-auditory circuits known to be associated with tinnitus.
2. Given the availability of a large number of drugs with a wide spectrum of different effects on the EC system, it appears possible that some of them may reduce tinnitus percept or risk factors rather than increase them, similar to what is seen, e.g., for anxiety (where EC-targeting drugs may either worsen or ameliorate it).
3. EC modulation of neuroinflammatory responses in the auditory system, in particular by CBD, which is neuroprotective, is anti-inflammatory, undergoes clinical trial as an anxiolytic, and acts on pathways involved in cochlear damage protection, may represent a novel pharmacological approach to hearing loss and tinnitus, although more data are necessary (especially on humans) to assess the therapeutic value of this or other EC drugs.
Author Contributions
All authors listed have made a substantial, direct and intellectual contribution to the work, and approved it for publication. PP, RP, and PE contributed auditory expertise. AMT, FM, and MC neuroimmunological expertise. PE and CB pharmacological and clinical expertise.
Funding
This work was supported by AIT ONLUS donations to PP and FRG2019 grant to CB.
Conflict of Interest
The authors declare that the research was conducted in the absence of any commercial or financial relationships that could be construed as a potential conflict of interest.
Supplementary Material
The Supplementary Material for this article can be found online at: https://www.frontiersin.org/articles/10.3389/fneur.2020.505995/full#supplementary-material
Abbreviations
2-AG, 2-arachidonoylglycerol; ABHD4, ABHD6, ABHD12, αβ-hydrolase domain 4,6,12; ACEA, arachidonyl-2'-chloroethylamide; AEA, N-arachidonoylethanolamide (anandamide); BDNF, brain-derived nerve factor; BNST, bed nucleus of the stria terminalis; CBR; CB1R; CB2R, cannabinoid receptor, type 1, type 2; CBD, cannabidiol; COX-2, cyclooxygenase type 2; CREB, cAMP response element binding protein; DAG, diacylglycerol; DAGL, DAG lipase; DCN, dorsal cochlear nucleus; DSE, Depolarization-induced suppression of excitation; DSI, Depolarization-induced suppression of inhibition; EC, endocannabinoid; EMT, endocannabinoid membrane transporter; ERK, Extracellular signal-Regulated Kinase; FAAH, fatty acid amide hydrolase; GDE1, glycerophosphodiesterase 1; GPCR, G-protein-coupled receptor; HPA, hypothalamic-pituitary-adrenal; MAGL, monoacylglycerol lipase; NAPE, N-arachidoylphosphatidyletanolamine; NAT, N-acyltransferase; NR3C1, glucocorticoid receptor; PHARC, Polyneuropathy, Hearing loss, Ataxia, Retinitis pigmentosa, and Cataracts; PLA2, phospholipase A2; PLD, phospholipase D; PPAR, Peroxisome proliferator-activated receptors; PUFA, polyunsaturated fatty acids; SPM, specialized pro-resolving mediators; TRP, Transient receptor potential; Δ9-THC, Δ9-tetrahydrocannabinol; VCN, ventral cochlear nucleus.
References
1. Di Marzo V, De Petrocellis L. Why do cannabinoid receptors have more than one endogenous ligand? Philos Trans R Soc Lond B Biol Sci. (2012) 367:3216–28. doi: 10.1098/rstb.2011.0382
2. Carlisle SJ, Marciano-Cabral F, Staab A, Ludwick C, Cabral GA. Differential expression of the CB2 cannabinoid receptor by rodent macrophages and macrophage-like cells in relation to cell activation. Int Immunopharmacol. (2002) 2:69–82. doi: 10.1016/S1567-5769(01)00147-3
3. Brailoiu GC, Deliu E, Marcu J, Hoffman NE, Console-Bram L, Zhao P, et al. Differential activation of intracellular versus plasmalemmal CB2 cannabinoid receptors. Biochemistry. (2014) 53:4990–9. doi: 10.1021/bi500632a
4. Console-Bram L, Marcu J, Abood ME. Cannabinoid receptors: nomenclature and pharmacological principles. Prog Neuropsychopharmacol Biol Psychiatry. (2012) 38:4–15. doi: 10.1016/j.pnpbp.2012.02.009
5. Mackie K, Lai Y, Westenbroek R, Mitchell R. Cannabinoids activate an inwardly rectifying potassium conductance and inhibit Q-type calcium currents in AtT20 cells transfected with rat brain cannabinoid receptor. J Neurosci. (1995) 15:6552–61. doi: 10.1523/JNEUROSCI.15-10-06552.1995
6. Mackie K, Hille B. Cannabinoids inhibit N-type calcium channels in neuroblastoma-glioma cells. Proc Natl Acad Sci USA. (1992) 89:3825–9. doi: 10.1073/pnas.89.9.3825
7. Zou S, Kumar U. Cannabinoid receptors and the endocannabinoid system: signaling and function in the central nervous system. Int J Mol Sci. (2018) 19:833. doi: 10.3390/ijms19030833
8. Ibsen MS, Connor M, Glass M. Cannabinoid CB(1) and CB(2) receptor signaling and bias. Cannabis Cannabinoid Res. (2017) 2:48–60. doi: 10.1089/can.2016.0037
9. Ibsen MS, Finlay DB, Patel M, Javitch JA, Glass M, Grimsey NL. Cannabinoid CB1 and CB2 receptor-mediated arrestin translocation: species, subtype, and agonist-dependence. Front Pharmacol. (2019) 10:350. doi: 10.3389/fphar.2019.00350
10. Smith JS, Rajagopal S. The β-arrestins: multifunctional regulators of G protein-coupled receptors. J Biol Chem. (2016) 291:8969–77. doi: 10.1074/jbc.R115.713313
11. McCoy KL. Interaction between cannabinoid system and toll-like receptors controls inflammation. Mediat Inflamm. (2016) 2016:5831315. doi: 10.1155/2016/5831315
12. Hebert-Chatelain E, Desprez T, Serrat R, Bellocchio L, Soria-Gomez E, Busquets-Garcia A, et al. A cannabinoid link between mitochondria and memory. Nature. (2016) 539:555–9. doi: 10.1038/nature20127
13. Silvestri C, Di Marzo V. The endocannabinoid system in energy homeostasis and the etiopathology of metabolic disorders. Cell Metab. (2013) 17:475–90. doi: 10.1016/j.cmet.2013.03.001
14. Gallelli CA, Calcagnini S, Romano A, Koczwara JB, de Ceglia M, Dante D, et al. Modulation of the oxidative stress and lipid peroxidation by endocannabinoids and their lipid analogues. Antioxidants. (2018) 7:93. doi: 10.3390/antiox7070093
15. Pistis M, O'Sullivan SE. The role of nuclear hormone receptors in cannabinoid function. Adv Pharmacol. (2017) 80:291–328. doi: 10.1016/bs.apha.2017.03.008
16. Starkus J, Jansen C, Shimoda LMN, Stokes AJ, Small-Howard AL, Turner H. Diverse TRPV1 responses to cannabinoids. Channels. (2019) 13:172–91. doi: 10.1080/19336950.2019.1619436
17. Di Marzo V. Targeting the endocannabinoid system: to enhance or reduce? Nat Rev Drug Discov. (2008) 7:438–55. doi: 10.1038/nrd2553
18. Chicca A, Arena C, Manera C. Beyond the direct activation of cannabinoid receptors: new strategies to modulate the endocannabinoid system in CNS-related diseases. Recent Pat CNS Drug Discov. (2016) 10:122–41. doi: 10.2174/1574889810999160603185126
19. Savinainen JR, Saario SM, Laitinen JT. The serine hydrolases MAGL, ABHD6 and ABHD12 as guardians of 2-arachidonoylglycerol signalling through cannabinoid receptors. Acta Physiol. (2012) 204:267–76. doi: 10.1111/j.1748-1716.2011.02280.x
20. Kaur R, Ambwani SR, Singh S. Endocannabinoid system: a multi-facet therapeutic target. Curr Clin Pharmacol. (2016) 11:110–7. doi: 10.2174/1574884711666160418105339
21. Wilson RI, Nicoll RA. Endogenous cannabinoids mediate retrograde signalling at hippocampal synapses. Nature. (2001) 410:588–92. doi: 10.1038/35069076
22. Kano M, Ohno-Shosaku T, Hashimotodani Y, Uchigashima M, Watanabe M. Endocannabinoid-mediated control of synaptic transmission. Physiol Rev. (2009) 89:309–80. doi: 10.1152/physrev.00019.2008
23. Labra VC, Santibáñez CA, Gajardo-Gómez R, Díaz EF, Gómez GI, Orellana JA. The neuroglial dialog between cannabinoids and hemichannels. Front Mol Neurosci. (2018) 11:79. doi: 10.3389/fnmol.2018.00079
24. Oddi S, Scipioni L, Maccarrone M. Endocannabinoid system and adult neurogenesis: a focused review. Curr Opin Pharmacol. (2019) 50:25–32. doi: 10.1016/j.coph.2019.11.002
25. Pertwee RG. Endocannabinoids and their pharmacological actions. Handb Exp Pharmacol. (2015) 231:1–37. doi: 10.1007/978-3-319-20825-1_1
26. Lutz B. Lipid mediators in the regulation of emotions, memory, and cognitive functions. In: Melis M, editor. Endocannabinoids and Lipid Mediators in Brain Functions. Cham: Springer International Publishing, A. G. (2017).
27. Lisboa SF, Vila-Verde C, Rosa J, Uliana DL, Stern CAJ, Bertoglio LJ, et al. Tempering aversive/traumatic memories with cannabinoids: a review of evidence from animal and human studies. Psychopharmacology. (2019) 236:201–26. doi: 10.1007/s00213-018-5127-x
28. Turcotte C, Blanchet MR, Laviolette M, Flamand N. The CB2 receptor and its role as a regulator of inflammation. Cell Mol Life Sci. (2016) 73:4449–70. doi: 10.1007/s00018-016-2300-4
29. Mastinu A, Premoli M, Ferrari-Toninelli G, Tambaro S, Maccarinelli G, Memo M, et al. Cannabinoids in health and disease: pharmacological potential in metabolic syndrome and neuroinflammation. Horm Mol Biol Clin Investig. (2018) 36:1–15. doi: 10.1515/hmbci-2018-0013
30. Marco EM, García-Gutiérrez MS, Bermúdez-Silva FJ, Moreira FA, Guimarães F, Manzanares J, et al. Endocannabinoid system and psychiatry: in search of a neurobiological basis for detrimental and potential therapeutic effects. Front Behav Neurosci. (2011) 5:63. doi: 10.3389/fnbeh.2011.00063
31. Basu S, Dittel BN. Unraveling the complexities of cannabinoid receptor 2 (CB2) immune regulation in health and disease Immunol. Res. (2011) 51:26–38. doi: 10.1007/s12026-011-8210-5
32. Onaivi ES, Ishiguro H, Gu S, Liu QR. CNS effects of CB2 cannabinoid receptors: beyond neuro-immuno-cannabinoid activity. J Psychopharmacol. (2012) 26:92–103. doi: 10.1177/0269881111400652
33. Morales P, Hurst DP, Reggio PH. Molecular targets of the phytocannabinoids: a complex picture. Prog Chem Org Nat Prod. (2017) 103:103–31. doi: 10.1007/978-3-319-45541-9_4
34. Lynch DL, Hurst DP, Shore DM, Pitman MC, Reggio PH. Molecular dynamics methodologies for probing cannabinoid ligand/receptor interaction. Methods Enzymol. (2017) 593:449–90. doi: 10.1016/bs.mie.2017.05.004
35. Di Marzo V, Piscitelli F. The endocannabinoid system and its modulation by phytocannabinoids. Neurotherapeutics. (2015) 12:692–8. doi: 10.1007/s13311-015-0374-6
36. Hryhorowicz S, Walczak M, Zakerska-Banaszak O, Słomski R, Skrzypczak-Zielińska M. Pharmacogenetics of cannabinoids. Eur J Drug Metab Pharmacokin. (2018) 43:1–12. doi: 10.1007/s13318-017-0416-z
37. Chiurchiù V, Leuti A, Maccarrone M. Bioactive lipids and chronic inflammation: managing the fire within. Front Immunol. (2018) 9:38. doi: 10.3389/fimmu.2018.00038
38. Devane WA, Hanus L, Breuer A, Pertwee RG, Stevenson LA, Griffin G, et al. Isolation and structure of a brain constituent that binds to the cannabinoid receptor. Science. (1992) 258:1946–9. doi: 10.1126/science.1470919
39. Mechoulam R, Ben-Shabat S, Hanus L, Ligumsky M, Kaminski NE, Schatz AR, et al. Identification of an endogenous 2-monoglyceride, present in canine gut, that binds to cannabinoid receptors. Biochem Pharmacol. (1995) 50:83–90. doi: 10.1016/0006-2952(95)00109-D
40. Sugiura T, Kishimoto S, Oka S, Gokoh M. Biochemistry, pharmacology and physiology of 2-arachidonoylglycerol, an endogenous cannabinoid receptor ligand. Prog Lipid Res. (2006) 45:405–46. doi: 10.1016/j.plipres.2006.03.003
41. Pagotto U, Marsicano G, Cota D, Lutz B, Pasquali R. The emerging role of the endocannabinoid system in endocrine regulation and energy balance. Endocr Rev. (2006) 27:73–100. doi: 10.1210/er.2005-0009
42. Wang J, Ueda N. Biology of endocannabinoid synthesis system. Prostaglandins Other Lipid Mediat. (2009) 89:112–9. doi: 10.1016/j.prostaglandins.2008.12.002
43. Hanus L, Abu-Lafi S, Fride E, Breuer A, Vogel Z, Shalev DE, et al. 2-arachidonyl glyceryl ether, an endogenous agonist of the cannabinoid CB1 receptor. Proc Natl Acad Sci USA. (2001) 98:3662–5. doi: 10.1073/pnas.061029898
44. Bauer M, Chicca A, Tamborrini M, Eisen D, Lerner R, Lutz B, et al. Identification and quantification of a new family of peptide endocannabinoids (Pepcans) showing negative allosteric modulation at CB1 receptors. J Biol Chem. (2012) 287:36944–67. doi: 10.1074/jbc.M112.382481
45. Leishman E, Cornett B, Spork K, Straiker A, Mackie K, Bradshaw HB. Broad impact of deleting endogenous cannabinoid hydrolyzing enzymes and the CB1 cannabinoid receptor on the endogenous cannabinoid-related lipidome in eight regions of the mouse brain. Pharmacol Res. (2016) 110:159–72. doi: 10.1016/j.phrs.2016.04.020
46. Alger BE, Kim J. Supply and demand for endocannabinoids. Trends Neurosci. (2011) 34:304–15. doi: 10.1016/j.tins.2011.03.003
47. Marrs WR, Horne EA, Ortega-Gutierrez S, Cisneros JA, Xu C, Lin YH, et al. Dual inhibition of alpha/beta-hydrolase domain 6 and fatty acid amide hydrolase increases endocannabinoid levels in neurons. J Biol Chem. (2011) 286:28723–8. doi: 10.1074/jbc.M110.202853
48. Gatta L, Piscitelli F, Giordano C, Boccella S, Lichtman A, Maione S, et al. Discovery of prostamide F2α and its role in inflammatory pain and dorsal horn nociceptive neuron hyperexcitability. PLoS ONE. (2012) 7:e31111. doi: 10.1371/journal.pone.0031111
49. Mechoulam R, Parker LA. The endocannabinoid system and the brain. Ann Rev Psychol. (2013) 64:21–47. doi: 10.1146/annurev-psych-113011-143739
50. Fonseca BM, Costa MA, Almada M, Correia-da-Silva G, Teixeira NA. Endogenous cannabinoids revisited: a biochemistry perspective. Prostaglandins Other Lipid Mediat. (2013) 102–3:13–30. doi: 10.1016/j.prostaglandins.2013.02.002
51. Lu HC, Mackie K. An introduction to the endogenous cannabinoid system. Biol Psychiatry. (2016) 79:516–25. doi: 10.1016/j.biopsych.2015.07.028
52. Begg M, Pacher P, Bátkai S, Osei-Hyiaman D, Offertáler L, Mo FM, et al. Evidence for novel cannabinoid receptors. Pharmacol Ther. (2005) 106:133–45. doi: 10.1016/j.pharmthera.2004.11.005
53. Muller C, Morales P, Reggio PH. Cannabinoid ligands targeting TRP channels. Front Mol Neurosci. (2019) 11:487. doi: 10.3389/fnmol.2018.00487
54. O'Sullivan SE. Cannabinoids go nuclear: evidence for activation of peroxisome proliferator-activated receptors. Br J Pharmacol. (2007) 152:576–82. doi: 10.1038/sj.bjp.0707423
55. Pertwee RG. Cannabinoid pharmacology: the first 66 years. Br J Pharmacol. (2006) 147 (Suppl. 1):S163–71. doi: 10.1038/sj.bjp.0706406
56. Pertwee RG. Cannabinoid receptor ligands: clinical and neuropharmacological considerations, relevant to future drug discovery and development. Expert Opin Investig Drugs. (2000) 9:1553–71. doi: 10.1517/13543784.9.7.1553
57. Pistis M, Melis M. From surface to nuclear receptors: the endocannabinoid family extends its assets. Curr Med Chem. (2010) 17:1450–67. doi: 10.2174/092986710790980014
58. Irving A, Abdulrazzaq G, Chan SLF, Penman J, Harvey J, Alexander SPH. Cannabinoid receptor-related orphan G protein-coupled receptors. Adv Pharmacol. (2017) 80:223–47. doi: 10.1016/bs.apha.2017.04.004
59. Morales P, Reggio PH. An Update on Non-CB1, Non-CB2 cannabinoid related G-protein-coupled receptors. Cannabis Cannabinoid Res. (2017) 2:265–73. doi: 10.1089/can.2017.0036
60. Pertwee RG, Howlett AC, Abood ME, Alexander SPH, Di Marzo V, Elphick MR, et al. International Union of Basic and Clinical Pharmacology. LXXIX. Cannabinoid receptors and their ligands: beyond CB1 and CB2. Pharmacol Rev. (2010) 62:588–631. doi: 10.1124/pr.110.003004
61. De Petrocellis L, Nabissi M, Santoni G, Ligresti A. Actions and regulation of ionotropic cannabinoid receptors. Adv Pharmacol. (2017) 80:249–89. doi: 10.1016/bs.apha.2017.04.001
62. Oláh A, Szekanecz Z, Bíró T. Targeting cannabinoid signaling in the immune system: “high”-ly exciting questions, possibilities, and challenges. Front Immunol. (2017) 8:1487. doi: 10.3389/fimmu.2017.01487
63. Callén L, Moreno E, Barroso-Chinea P, Moreno-Delgado D, Cortés A, Mallol J, et al. Cannabinoid receptors CB1 and CB2 form functional heteromers in brain. J Biol Chem. (2012) 287:20851–65. doi: 10.1074/jbc.M111.335273
64. Moreno E, Chiarlone A, Medrano M, Puigdellívol M, Bibic L, Howell LA, et al. Singular location and signaling profile of adenosine A2A-cannabinoid CB1 receptor heteromers in the dorsal striatum. Neuropsychopharmacology. (2018) 43:964–77. doi: 10.1038/npp.2017.12
65. Kearn CS, Blake-Palmer K, Daniel E, Mackie K, Glass M. Concurrent stimulation of cannabinoid CB1 and dopamine D2 receptors enhances heterodimer formation: a mechanism for receptor cross-talk? Mol Pharmacol. (2005) 67:1697–704. doi: 10.1124/mol.104.006882
66. Hojo M, Sudo Y, Ando Y, Minami K, Takada M, Matsubara T, et al. mu-Opioid receptor forms a functional heterodimer with cannabinoid CB1 receptor: electrophysiological and FRET assay analysis. J Pharmacol Sci. (2008). 108:308–19. doi: 10.1254/jphs.08244fp
67. Ward RJ, Pediani JD, Milligan G. Heteromultimerization of cannabinoid CB(1) receptor and orexin OX(1) receptor generates a unique complex in which both protomers are regulated by orexin, A. J Biol Chem. (2011) 286:37414–28. doi: 10.1074/jbc.M111.287649
68. Coke CJ, Scarlett KA, Chetram MA, Jones KJ, Sandifer BJ, Davis AS, et al. Simultaneous activation of induced heterodimerization between CXCR4 chemokine receptor and cannabinoid receptor 2 (CB2) reveals a mechanism for regulation of tumor progression. J Biol Chem. (2016) 291:9991–10005. doi: 10.1074/jbc.M115.712661
69. Balenga NA, Martínez-Pinilla E, Kargl J, Schröder R, Peinhaupt M, Platzer W, et al. Heteromerization of GPR55 and cannabinoid CB2 receptors modulates signalling. Br J Pharmacol. (2014). 171:5387–406. doi: 10.1111/bph.12850
70. Costa L, Amaral C, Teixeira N, Correia-da-Silva G, Fonseca BM. Cannabinoid-induced autophagy: protective or death role?, prostaglandins & other lipid. Mediators. (2016) 122:54–63. doi: 10.1016/j.prostaglandins.2015.12.006
71. Hiebel C, Behl C. The complex modulation of lysosomal degradation pathways by cannabinoid receptors 1 and 2. Life Sci. (2015) 138:3–7. doi: 10.1016/j.lfs.2015.03.020
72. Premkumar LS, Abooj M. TRP channels and analgesia. Life Sci. (2013) 92:415–24. doi: 10.1016/j.lfs.2012.08.010
73. Joshi N, Onaivi ES. Endocannabinoid system components: overview and tissue distribution. Adv Exp Med Biol. (2019) 1162:1–12. doi: 10.1007/978-3-030-21737-2_1
74. Howlett AC. The cannabinoid receptors. Prostaglandins Other Lipid Mediat. (2002) 68–9:619–31. doi: 10.1016/S0090-6980(02)00060-6
75. Pacher P, Bátkai S, Kunos G. The endocannabinoid system as an emerging target of pharmacotherapy. Pharmacol Rev. (2006) 58:389–462. doi: 10.1124/pr.58.3.2
76. Herkenham M, Lynn AB, Johnson MR, Melvin LS, de Costa BR, Rice KC. Characterization and localization of cannabinoid receptors in rat brain: a quantitative in vitro autoradiographic study. J Neurosci. (1991) 11:563–83. doi: 10.1523/JNEUROSCI.11-02-00563.1991
77. Munro S, Thomas KL, Abu-Shaar M. Molecular characterization of a peripheral receptor for cannabinoids. Nature. (1993). 365:61–5. doi: 10.1038/365061a0
78. Pacher P, Mechoulam R. Is lipid signaling through cannabinoid 2 receptors part of a protective system? Prog Lipid Res. (2011). 50:193–211. doi: 10.1016/j.plipres.2011.01.001
79. Atwood BK, Mackie K. CB2: a cannabinoid receptor with an identity crisis. Br J Pharmacol. (2010) 160:467–79. doi: 10.1111/j.1476-5381.2010.00729.x
80. Martín-Saldaña S, Trinidad A, Ramil E, Sánchez-López AJ, Coronado MJ, Martínez-Martínez E, et al. Spontaneous cannabinoid receptor 2 (CB2) expression in the cochlea of adult albino rat and its up-regulation after cisplatin treatment. PLoS ONE. (2016) 11:e0161954. doi: 10.1371/journal.pone.0161954
81. Yu SJ, Reiner D, Shen H, Wu KJ, Liu QR, Wang Y. Time-dependent protection of CB2 receptor agonist in stroke. PLoS ONE. (2015) 10:e0132487. doi: 10.1371/journal.pone.0132487
82. Katona I, Freund TF. Multiple functions of endocannabinoid signaling in the brain. Annu Rev Neurosci. (2012) 35:529–58. doi: 10.1146/annurev-neuro-062111-150420
83. Busquets-Garcia A, Bains J, Marsicano G. CB(1) receptor signaling in the brain: extracting specificity from ubiquity. Neuropsychopharmacology. (2018) 43:4–20. doi: 10.1038/npp.2017.206
84. Narayanaswami V, Dahl K, Bernard-Gauthier V, Josephson L, Cumming P, Vasdev N. Emerging PET radiotracers and targets for imaging of neuroinflammation in neurodegenerative diseases: outlook beyond TSPO. Mol Imaging. (2018) 17:1536012118792317. doi: 10.1177/1536012118792317
85. Toczek M, Malinowska B. Enhanced endocannabinoid tone as a potential target of pharmacotherapy. Life Sci. (2018) 204:20–45. doi: 10.1016/j.lfs.2018.04.054
86. Chen WW, Zhang X, Huang WJ. Role of neuroinflammation in neurodegenerative diseases. Mol Med Rep. (2016) 13:3391–6. doi: 10.3892/mmr.2016.4948
87. Diana MA, Marty A. Endocannabinoid-mediated short-term synaptic plasticity: depolarization-induced suppression of inhibition (DSI) and depolarization-induced suppression of excitation (DSE). Br J Pharmacol. (2004) 142:9–19. doi: 10.1038/sj.bjp.0705726
88. Oertel D, Young ED. What's a cerebellar circuit doing in the auditory system? Trends Neurosci. (2004) 27:104–10. doi: 10.1016/j.tins.2003.12.001
89. Singla S, Dempsey C, Warren R, Enikolopov AG, Sawtell NB. A cerebellum-like circuit in the auditory system cancels responses to self-generated sounds. Nat Neurosci. (2017) 20:943–50. doi: 10.1038/nn.4567
90. Tzounopoulos T. Mechanisms of synaptic plasticity in the dorsal cochlear nucleus: plasticity-induced changes that could underlie tinnitus. Am J Audiol. (2008). 17:S170–5. doi: 10.1044/1059-0889(2008/07-0030)
91. Shore SE, Wu C. Mechanisms of noise-induced tinnitus: insights from cellular studies. Neuron. (2019) 103:8–20. doi: 10.1016/j.neuron.2019.05.008
92. Sedlacek M, Tipton PW, Brenowitz SD. Sustained firing of cartwheel cells in the dorsal cochlear nucleus evokes endocannabinoid release and retrograde suppression of parallel fiber synapses. J Neurosci. (2011) 31:15807–17. doi: 10.1523/JNEUROSCI.4088-11.2011
93. Zhao Y, Rubio ME, Tzounopoulos T. Distinct functional and anatomical architecture of the endocannabinoid system in the auditory brainstem. J Neurophysiol. (2009) 101:2434–46. doi: 10.1152/jn.00047.2009
94. Zhao Y, Tzounopoulos T. Physiological activation of cholinergic inputs controls associative synaptic plasticity via modulation of endocannabinoid signaling. J Neurosci. (2011) 31:3158–68. doi: 10.1523/JNEUROSCI.5303-10.2011
95. Stefanescu RA, Shore SE. Muscarinic acetylcholine receptors control baseline activity and Hebbian stimulus timing-dependent plasticity in fusiform cells of the dorsal cochlear nucleus. J Neurophysiol. (2017) 117:1229–38. doi: 10.1152/jn.00270.2016
96. Zugaib J, Leão RM. Enhancement of endocannabinoid-dependent depolarization-induced suppression of excitation in glycinergic neurons by prolonged exposure to high doses of salicylate. Neuroscience. (2018) 376:72–9. doi: 10.1016/j.neuroscience.2018.02.016
97. Zheng Y, Smith PF. Cannabinoid drugs: will they relieve or exacerbate tinnitus? Curr Opin Neurol. (2019) 32:131–6. doi: 10.1097/WCO.0000000000000631
98. Kim J, Alger BE. Reduction in endocannabinoid tone is a homeostatic mechanism for specific inhibitory synapses. Nat Neurosci. (2010). 13:592–600. doi: 10.1038/nn.2517
99. Nyilas R, Dudok B, Urbán GM, Mackie K, Watanabe M, Cravatt BF, et al. Enzymatic machinery for endocannabinoid biosynthesis associated with calcium stores in glutamatergic axon terminals. J Neurosci. (2008) 28:1058–63. doi: 10.1523/JNEUROSCI.5102-07.2008
100. Freund TF, Katona I, Piomelli D. Role of endogenous cannabinoids in synaptic signaling. Physiol Rev. (2003) 83:1017–66. doi: 10.1152/physrev.00004.2003
101. Hillard CJ. Biochemistry and pharmacology of the endocannabinoids arachidonylethanolamide and 2-arachidonylglycerol. Prostaglandins Other Lipid Mediat. (2000) 61:3–18. doi: 10.1016/S0090-6980(00)00051-4
102. Stella N. Cannabinoid signaling in glial cells. Glia. (2004) 48:267–77. doi: 10.1002/glia.20084
103. Jimenez-Blasco D, Busquets-Garcia A, Hebert-Chatelain E, Serrat R, Vicente-Gutierrez C, Ioannidou C, et al. Glucose metabolism links astroglial mitochondria to cannabinoid effects. Nature. (2020) 583:603–8. doi: 10.1038/s41586-020-2470-y
104. Viader A, Blankman JL, Zhong P, Liu X, Schlosburg JE, Joslyn CM, et al. Metabolic interplay between astrocytes and neurons regulates endocannabinoid action. Cell Rep. (2015) 12:798–808. doi: 10.1016/j.celrep.2015.06.075
105. Navarrete M, Araque A. Endocannabinoids potentiate synaptic transmission through stimulation of astrocytes. Neuron. (2010) 68:113–26. doi: 10.1016/j.neuron.2010.08.043
106. Rasooli-Nejad S, Palygin O, Lalo U, Pankratov Y. Cannabinoid receptors contribute to astroglial Ca2+-signalling and control of synaptic plasticity in the neocortex Phil. Trans, R Soc B. (2014) 369:20140077. doi: 10.1098/rstb.2014.0077
107. Kovács A, Bordás C, Bíró T, Hegyi Z, Antal M, Szücs P, et al. Direct presynaptic and indirect astrocyte-mediated mechanisms both contribute to endocannabinoid signaling in the pedunculopontine nucleus of mice. Brain Struct Funct. (2017) 222:247–66. doi: 10.1007/s00429-016-1214-0
108. Hablitz LM, Gunesch AN, Cravetchi O, Moldavan M, Allen CN. Cannabinoid signaling recruits astrocytes to modulate presynaptic function in the suprachiasmatic nucleus. eNeuro. (2020) 7:ENEURO.0081-19.2020. doi: 10.1523/ENEURO.0081-19.2020
109. Koning HM. Sleep disturbances associated with tinnitus: reduce the maximal intensity of tinnitus. Int Tinnitus J. (2019) 23:64–8. doi: 10.5935/0946-5448.20190018
110. Probst T, Pryss RC, Langguth B, Rauschecker JP, Schobel J, Reichert M, et al. Does tinnitus depend on time-of-day? An ecological momentary assessment study with the “TrackYourTinnitus” Application Front Aging Neurosci. (2017) 9:253. doi: 10.3389/fnagi.2017.00253
111. Becher B, Spath S, Goverman J. Cytokine networks in neuroinflammation. Nat Rev Immunol. (2017) 17:49–59. doi: 10.1038/nri.2016.123
112. Walter L, Stella N. Endothelin-1 increases 2-arachidonoyl glycerol (2-AG) production in astrocytes. Glia. (2003) 44:85–90. doi: 10.1002/glia.10270
113. Rom S, Persidsky Y. Cannabinoid receptor 2: potential role in immunomodulation and neuroinflammation. J Neuroimmune Pharmacol. (2013) 8:608–20. doi: 10.1007/s11481-013-9445-9
114. Turcotte C, Chouinard F, Lefebvre JS, Flamand N. Regulation of inflammation by cannabinoids, the endocannabinoids 2-arachidonoyl-glycerol and arachidonoyl-ethanolamide and their metabolites. J Leukoc Biol. (2015) 97:1049–70. doi: 10.1189/jlb.3RU0115-021R
115. Waksman Y, Olson JM, Carlisle SJ, Cabral GA. The central cannabinoid receptor (CB1) mediates inhibition of nitric oxide production by rat microglial cells. J Pharmacol Exp Ther. (1999) 288:1357–66.
116. Molina-Holgado F, Molina-Holgado E, Guaza C, Rothwell NJ. Role of CB1 and CB2 receptors in the inhibitory effects of cannabinoids on lipopolysaccharide-induced nitric oxide release in astrocyte cultures. J Neurosci Res. (2002) 67:829–36. doi: 10.1002/jnr.10165
117. Molina-Holgado F, Pinteaux E, Moore JD, Molina-Holgado E, Guaza C, Gibson RM, et al. Endogenous interleukin-1 receptor antagonist mediates anti-inflammatory and neuroprotective actions of cannabinoids in neurons and glia. J Neurosci. (2003) 23:6470–4. doi: 10.1523/JNEUROSCI.23-16-06470.2003
118. Mecha M, Feliú A, Carrillo-Salinas FJ, Rueda-Zubiaurre A, Ortega-Gutiérrez S, de Sola RG, et al. Endocannabinoids drive the acquisition of an alternative phenotype in microglia. Brain Behav Immun. (2015) 49:233–45. doi: 10.1016/j.bbi.2015.06.002
119. Stella N. Endocannabinoid signaling in microglial cells. Neuropharmacology. (2009) 56:244–53. doi: 10.1016/j.neuropharm.2008.07.037
120. Walter L, Franklin A, Witting A, Wade C, Xie Y, Kunos G, et al. Nonpsychotropic cannabinoid receptors regulate microglial cell migration. J Neurosci. (2003) 23:1398–405. doi: 10.1523/JNEUROSCI.23-04-01398.2003
121. Bruttger J, Karram K, Wörtge S, Regen T, Marini F, Hoppmann N, et al. Genetic cell ablation reveals clusters of local self-renewing microglia in the mammalian central nervous system. Immunity. (2015) 43:92–106. doi: 10.1016/j.immuni.2015.06.012
122. Askew K, Li K, Olmos-Alonso A, Garcia-Moreno F, Liang Y, Richardson P, et al. Coupled proliferation and apoptosis maintain the rapid turnover of microglia in the adult brain. Cell Rep. (2017) 18:391–405. doi: 10.1016/j.celrep.2016.12.041
123. Huang Y, Xu Z, Xiong S, Sun F, Qin G, Hu G, et al. Repopulated microglia are solely derived from the proliferation of residual microglia after acute depletion. Nat Neurosci. (2018) 21:530–40. doi: 10.1038/s41593-018-0090-8
124. Viader A, Ogasawara D, Joslyn CM, Sanchez-Alavez M, Mori S, Nguyen W, et al. A chemical proteomic atlas of brain serine hydrolases identifies cell type-specific pathways regulating neuroinflammation. Elife. (2016) 5:e12345. doi: 10.7554/eLife.12345
125. Stella N. Cannabinoid and cannabinoid-like receptors in microglia, astrocytes, and astrocytomas. Glia. (2010) 58:1017–30. doi: 10.1002/glia.20983
126. Jordan CJ, Xi ZX. Progress in brain cannabinoid CB(2) receptor research: From genes to behavior. Neurosci Biobehav Rev. (2019) 98:208–20. doi: 10.1016/j.neubiorev.2018.12.026
127. Lemos JR, Ortiz-Miranda SI, Cuadra AE, Velázquez-Marrero C, Custer EE, Dad T, et al. Modulation/physiology of calcium channel sub-types in neurosecretory terminals. Cell Calcium. (2012) 51:284–92. doi: 10.1016/j.ceca.2012.01.008
128. Witting A, Walter L, Wacker J, Möller T, Stella N. P2X7 receptors control 2-arachidonoylglycerol production by microglial cells. Proc Natl Acad Sci USA. (2004) 101:3214–9. doi: 10.1073/pnas.0306707101
129. Cassano T, Calcagnini S, Pace L, De Marco F, Romano A, Gaetani S. Cannabinoid receptor 2 signaling in neurodegenerative disorders: from pathogenesis to a promising therapeutic target. Front Neurosci. (2017) 11:30. doi: 10.3389/fnins.2017.00030
130. Grabner GF, Eichmann TO, Wagner B, Gao Y, Farzi A, Taschler U, et al. Deletion of monoglyceride lipase in astrocytes attenuates lipopolysaccharide-induced neuroinflammation. J Biol Chem. (2016) 291:913–23. doi: 10.1074/jbc.M115.683615
131. Miller LK, Devi LA. The highs and lows of cannabinoid receptor expression in disease: mechanisms and their therapeutic implications. Pharmacol Rev. (2011) 63:461–70. doi: 10.1124/pr.110.003491
132. Baek JH, Darlington CL, Smith PF, Ashton JC. Antibody testing for brain immunohistochemistry: brain immunolabeling for the cannabinoid CB2 receptor. J Neurosci Methods. (2013) 216:87–95. doi: 10.1016/j.jneumeth.2013.03.021
133. Zhang HY, Shen H, Jordan CJ, Liu QR, Gardner EL, Bonci A, et al. CB2 receptor antibody signal specificity: correlations with the use of partial CB2-knockout mice and anti-rat CB2 receptor antibodies. Acta Pharmacol Sin. (2019) 40:398–409. doi: 10.1038/s41401-018-0037-3
134. Galiègue S, Mary S, Marchand J, Dussossoy D, Carrière D, Carayon P, et al. Expression of central and peripheral cannabinoid receptors in human immune tissues and leukocyte subpopulations. Eur J Biochem. (1995) 232:54–61. doi: 10.1111/j.1432-1033.1995.tb20780.x
135. Ofek O, Karsak M, Leclerc N, Fogel M, Frenkel B, Wright K, et al. Peripheral cannabinoid receptor, CB2, regulates bone mass. Proc Natl Acad Sci USA. (2006) 103:696–701. doi: 10.1073/pnas.0504187103
136. Benito C, Romero JP, Tolón RM, Clemente D, Docagne F, Hillard CJ, et al. Cannabinoid CB1 and CB2 receptors and fatty acid amide hydrolase are specific markers of plaque cell subtypes in human multiple sclerosis. J Neurosci. (2007) 27:2396–402. doi: 10.1523/JNEUROSCI.4814-06.2007
137. Brusco A, Tagliaferro P, Saez T, Onaivi ES. Postsynaptic localization of CB2 cannabinoid receptors in the rat hippocampus. Synapse. (2008) 62:944–9. doi: 10.1002/syn.20569
138. Molina-Holgado E, Vela JM, Arévalo-Martín A, Almazán G, Molina-Holgado F, Borrell J, et al. Cannabinoids promote oligodendrocyte progenitor survival: involvement of cannabinoid receptors and phosphatidylinositol-3 kinase/Akt signaling. J Neurosci. (2002) 22:9742–53. doi: 10.1523/JNEUROSCI.22-22-09742.2002
139. Liu QR, Pan CH, Hishimoto A, Li C-Y, Xi Z-X, Llorente-Berzal A, et al. Species differences in cannabinoid receptor 2 (CNR2 gene): identification of novel human and rodent CB2 isoforms, differential tissue expression and regulation by cannabinoid receptor ligands. Genes Brain Behav. (2009). 8:519–30. doi: 10.1111/j.1601-183X.2009.00498.x
140. Zhang HY, Bi GH, Li X, Li J, Qu H, Zhang S-J, et al. Species differences in cannabinoid receptor 2 and receptor responses to cocaine self-administration in mice and rats. Neuropsychopharmacology. (2015). 40:1037–51. doi: 10.1038/npp.2014.297
141. Colonna M, Butovsky O. Microglia function in the central nervous system during health and neurodegeneration. Annu Rev Immunol. (2017) 35:441–68. doi: 10.1146/annurev-immunol-051116-052358
142. Casano AM, Peri F. Microglia: multitasking specialists of the brain. Dev Cell. (2015) 32:469–77. doi: 10.1016/j.devcel.2015.01.018
143. Ransohoff RM. A polarizing question: do M1 and M2 microglia exist? Nat Neurosci. (2016) 19:987–91. doi: 10.1038/nn.4338
144. Masuda T, Sankowski R, Staszewski O, Prinz M. Microglia heterogeneity in the single-cell era. Cell Rep. (2020) 30:1271–81. doi: 10.1016/j.celrep.2020.01.010
145. De Biase LM, Bonci A. Region-specific phenotypes of microglia: the role of local regulatory cues. Neuroscientist. (2019) 25:314–33. doi: 10.1177/1073858418800996
146. Geirsdottir L, David E, Keren-Shaul H, Weiner A, Bohlen SC, Neuber J, et al. Cross-species single-cell analysis reveals divergence of the primate microglia program. Cell. (2019) 179:1609–22.e16. doi: 10.1016/j.cell.2019.11.010
147. Galatro TF, Holtman IR, Lerario AM, Vainchtein ID, Brouwer N, Sola PR, et al. Transcriptomic analysis of purified human cortical microglia reveals age-associated changes. Nat Neurosci. (2017) 20:1162–71. doi: 10.1038/nn.4597
148. Villa A, Della Torre S, Maggi A. Sexual differentiation of microglia. Front Neuroendocrinol. (2019) 52:156–64. doi: 10.1016/j.yfrne.2018.11.003
149. Butovsky O, Weiner HL. Microglial signatures and their role in health and disease. Nat Rev Neurosci. (2018) 19:622–35. doi: 10.1038/s41583-018-0057-5
150. Rivest S. Regulation of innate immune responses in the brain. Nat Rev Immunol. (2009) 9:429–39. doi: 10.1038/nri2565
151. Tansey MG, McCoy MK, Frank-Cannon TC. Neuroinflammatory mechanisms in Parkinson's disease: potential environmental triggers, pathways, and targets for early therapeutic intervention. Exp Neurol. (2007) 208:1–25. doi: 10.1016/j.expneurol.2007.07.004
152. Watson N, Ding B, Zhu X, Frisina RD. Chronic inflammation - inflammaging - in the ageing cochlea: a novel target for future presbycusis therapy. Ageing Res Rev. (2017) 40:142–8. doi: 10.1016/j.arr.2017.10.002
153. Wang J, Sung V, Carew P, Liu RS, Burgner D, Wake M. Inflammation and hearing status in mid-childhood and mid-life: a population-based cross-sectional study. Int J Epidemiol. (2019) 48:1556–66. doi: 10.1093/ije/dyz023
154. Wang W, Zhang LS, Zinsmaier AK, Patterson G, Leptich EJ, Shoemaker SL, et al. Neuroinflammation mediates noise-induced synaptic imbalance and tinnitus in rodent models. PLoS Biol. (2019) 17:e3000307. doi: 10.1371/journal.pbio.3000307
155. Marchiori LL, Dias AC, Gonçalvez AS, Poly-Frederico RC, doi MY. Association between polymorphism of tumor necrosis factor alpha (tnfα) in the region−308 g/a with tinnitus in the elderly with a history of occupational noise exposure. Noise Health. (2018) 20:37−41. doi: 10.4103/nah.NAH_34_17
156. Doi MY, Dias AC, Poly-Frederico RC, Maria MG, de Oliveira MN, de Moraes Marchiori LL. Association between polymorphism of interleukin-6 in the region−174G/C and tinnitus in the elderly with a history of occupational noise exposure. Noise Health. (2015) 17:406–10. doi: 10.4103/1463-1741.169703
157. Masgrau R, Guaza C, Ransohoff RM, Galea E. Should we stop saying 'glia' and 'neuroinflammation'? Trends Mol Med. (2017) 23:486–500. doi: 10.1016/j.molmed.2017.04.005
158. Petrackova A, Horak P, Radvansky M, Fillerova R, Smotkova Kraiczova V, Kudelka M, et al. Revealed heterogeneity in rheumatoid arthritis based on multivariate innate signature analysis. Clin Exp Rheumatol. (2019) 13:1–7. doi: 10.1155/2019/3575803
159. Lopez-Escamez JA, Batuecas-Caletrio A, Bisdorff A. Towards personalized medicine in Ménière's disease. F1000Res. (2018) 7:F1000. doi: 10.12688/f1000research.14417.1
160. Borg-Bartolo SP, Boyapati RK, Satsangi J, Kalla R. Precision medicine in inflammatory bowel disease: concept, progress and challenges. F1000Res. (2020) 9:F1000. doi: 10.12688/f1000research.20928.1
161. Galve-Roperh I, Chiurchiù V, Díaz-Alonso J, Bari M, Guzmán M, Maccarrone M. Cannabinoid receptor signaling in progenitor/stem cell proliferation and differentiation. Prog Lipid Res. (2013) 52:633–50. doi: 10.1016/j.plipres.2013.05.004
162. Patel KD, Davison JS, Pittman QJ, Sharkey KA. Cannabinoid CB(2) receptors in health and disease. Curr Med Chem. (2010) 17:1393–410. doi: 10.2174/092986710790980041
163. O'Sullivan SE, Kendall DA. Cannabinoid activation of peroxisome proliferator-activated receptors: potential for modulation of inflammatory disease. Immunobiology. (2010) 215:611–6. doi: 10.1016/j.imbio.2009.09.007
164. Parenti A, De Logu F, Geppetti P, Benemei S. What is the evidence for the role of TRP channels in inflammatory and immune cells? Br J Pharmacol. (2016) 173:953–69. doi: 10.1111/bph.13392
165. Santoni G, Cardinali C, Morelli MB, Santoni M, Nabissi M, Amantini C. Danger- and pathogen-associated molecular patterns recognition by pattern-recognition receptors and ion channels of the transient receptor potential family triggers the inflammasome activation in immune cells and sensory neurons. J Neuroinflammation. (2015) 12:21. doi: 10.1186/s12974-015-0239-2
166. Klein TW, Newton C, Zhu W, Daaka Y, Friedman H. delta 9-Tetrahydrocannabinol, cytokines, and immunity to Legionella pneumophila. Proc Soc Exp Biol Med. (1995) 209:205–12. doi: 10.3181/00379727-209-43897b
167. Lee SF, Newton C, Widen R, Friedman H, Klein TW. Differential expression of cannabinoid CB(2) receptor mRNA in mouse immune cell subpopulations and following B cell stimulation. Eur J Pharmacol. (2001) 423:235–41. doi: 10.1016/S0014-2999(01)01122-0
168. Castaneda JT, Harui A, Kiertscher SM, Roth JD, Roth MD. Differential expression of intracellular and extracellular CB(2) cannabinoid receptor protein by human peripheral blood leukocytes. J Neuroimmune Pharmacol. (2013) 8:323–32. doi: 10.1007/s11481-012-9430-8
169. Castaneda JT, Harui A, Roth MD. Regulation of cell surface CB2 receptor during human B cell activation and differentiation. J Neuroimmune Pharmacol. (2017) 12:544–54. doi: 10.1007/s11481-017-9744-7
170. Massi P, Vaccani A, Parolaro D. Cannabinoids, immune system and cytokine network. Curr Pharm Des. (2006) 12:3135–46. doi: 10.2174/138161206777947425
171. Herring AC, Koh WS, Kaminski NE. Inhibition of the cyclic AMP signaling cascade and nuclear factor binding to CRE and κB elements by cannabinol, a minimally CNS-active cannabinoid. Biochem Pharmacol. (1998) 55:1013–23. doi: 10.1016/S0006-2952(97)00630-8
172. Kaminski NE. Immune regulation by cannabinoid compounds through the inhibition of the cyclic AMP signaling cascade and altered gene expression. Biochem Pharmacol. (1996) 52:1133–40. doi: 10.1016/0006-2952(96)00480-7
173. Chiurchiù V. Endocannabinoids and immunity. Cannabis Cannabinoid Res. (2016) 1:59–66. doi: 10.1089/can.2016.0002
174. Chiurchiù V, Battistini L, Maccarrone M. Endocannabinoid signalling in innate and adaptive immunity. Immunology. (2015) 144:352–64. doi: 10.1111/imm.12441
175. Croxford JL, Yamamura T. Cannabinoids and the immune system: potential for the treatment of inflammatory diseases? J Neuroimmunol. (2005) 166:3–18. doi: 10.1016/j.jneuroim.2005.04.023
176. Tanasescu R, Constantinescu CS. Cannabinoids and the immune system: an overview. Immunobiology. (2010) 215:588–97. doi: 10.1016/j.imbio.2009.12.005
177. Kumar V. Toll-like receptors in the pathogenesis of neuroinflammation. J Neuroimmunol. (2019) 332:16–30. doi: 10.1016/j.jneuroim.2019.03.012
178. Zhang G, Zheng H, Pyykko I, Zou J. The TLR-4/NF-κB signaling pathway activation in cochlear inflammation of rats with noise-induced hearing loss. Hear Res. (2019) 379:59–68. doi: 10.1016/j.heares.2019.04.012
179. Møller AR. Tinnitus and pain. Prog Brain Res. (2007) 166:47–53. doi: 10.1016/S0079-6123(07)66004-X
180. Jastreboff PJ, Sasaki CT. An animal model of tinnitus: a decade of development. Am J Otol. (1994) 15:19–27.
181. Donvito G, Nass SR, Wilkerson JL, Curry ZA, Schurman LD, Kinsey SG, et al. The endogenous cannabinoid system: a budding source of targets for treating inflammatory and neuropathic pain. Neuropsychopharmacology. (2018) 43:52–79. doi: 10.1038/npp.2017.204
182. Billakota S, Devinsky O, Marsh E. Cannabinoid therapy in epilepsy. Curr Opin Neurol. (2019) 32:220–6. doi: 10.1097/WCO.0000000000000660
183. Han B, Gfroerer JC, Colliver JD. Associations between duration of illicit drug use and health conditions: results from the 2005-2007 national surveys on drug use and health. Ann Epidemiol. (2010) 20:289–97. doi: 10.1016/j.annepidem.2010.01.003
184. Qian ZJ, Alyono JC. An association between marijuana use and tinnitus. Am J Otolaryngol. (2020) 41:2314. doi: 10.1016/j.amjoto.2019.102314
185. Spaderna M, Addy PH, D'Souza DC. Spicing things up: synthetic cannabinoids. Psychopharmacology. (2013) 228:525–40. doi: 10.1007/s00213-013-3188-4
186. Sutlović D, Prkačin I, Vaiano F, Bertol E, Bratinčević MV, Definis-Gojanović M. A case of synthetic cannabinoid poisoning in Croatia. Arh Hig Rada Toksikol. (2018) 69:186–90. doi: 10.2478/aiht-2018-69-3100
187. Gertsch J, Pertwee RG, Di Marzo V. Phytocannabinoids beyond the Cannabis plant- do they exist? Br J Pharmacol. (2010) 160:523–9. doi: 10.1111/j.1476-5381.2010.00745.x
188. Woelkart K, Salo-Ahen OM, Bauer R. CB receptor ligands from plants. Curr Top Med Chem. (2008) 8:173–86. doi: 10.2174/156802608783498023
189. ElSohly MA, Radwan MM, Gul W, Chandra S, Galal A. Phytochemistry of Cannabis sativa, L. Prog Chem Org Nat Prod. (2017) 103:1–36. doi: 10.1007/978-3-319-45541-9_1
190. Pisanti S, Malfitano AM, Ciaglia E, Lamberti A, Ranieri R, Cuomo G, et al. Cannabidiol: state of the art and new challenges for therapeutic applications. Pharmacol Ther. (2017) 175:133–50. doi: 10.1016/j.pharmthera.2017.02.041
191. Edery H, Grunfeld Y, Porath G, Ben-Zvi Z, Shani A, Mechoulam R. Structure-activity relationships in the tetrahydrocannabinol series. Modifications on the aromatic ring and it the side-chain. Arzneimittelforschung. (1972) 22:1995–2003.
192. Mechoulam R, Braun P, Gaoni Y. Syntheses of 1-tetrahydrocannabinol and related cannabinoids. J Am Chem Soc. (1972) 94:6159–65. doi: 10.1021/ja00772a038
193. ElSohly MA, Gul W, Wanas AS, Radwan MM. Synthetic cannabinoids: analysis and metabolites. Life Sci. (2014) 97:78–90. doi: 10.1016/j.lfs.2013.12.212
194. Shevyrin V, Melkozerov V, Endres GW, Safran Y, Morzherin Y. On a new cannabinoid classification system: a sight on the illegal market of novel psychoactive substances. Cannabis Cannabinoid Res. (2016) 1:186–94. doi: 10.1089/can.2016.0004
195. Pertwee RG. Targeting the endocannabinoid system with cannabinoid receptor agonists: pharmacological strategies and therapeutic possibilities. Philos Trans R Soc Lond B Biol Sci. (2012) 367:3353–63. doi: 10.1098/rstb.2011.0381
196. Kohnz RA, Nomura DK. Chemical approaches to therapeutically target the metabolism and signaling of the endocannabinoid 2-AG and eicosanoids. Chem Soc Rev. (2014) 43:6859–69. doi: 10.1039/C4CS00047A
197. Le Boisselier R, Alexandre J, Lelong-Boulouard V, Debruyne D. Focus on cannabinoids and synthetic cannabinoids. Clin Pharmacol Ther. (2017) 101:220–9. doi: 10.1002/cpt.563
198. De Luca MA, Fattore L. Therapeutic use of synthetic cannabinoids: still an open issue? Clin Ther. (2018) 40:1457–66. doi: 10.1016/j.clinthera.2018.08.002
199. Tai S, Fantegrossi WE. Synthetic cannabinoids: pharmacology, behavioral effects, and abuse potential. Curr Addict Rep. (2014) 1:129–36. doi: 10.1007/s40429-014-0014-y
200. Swortwood MJ, Newmeyer MN, Andersson M, Abulseoud OA, Scheidweiler KB, Huestis MA. Cannabinoid disposition in oral fluid after controlled smoked, vaporized, and oral cannabis administration. Drug Test Anal. (2017) 9:905–15. doi: 10.1002/dta.2092
201. Lucas CJ, Galettis P, Schneider J. The pharmacokinetics and the pharmacodynamics of cannabinoids. Br J Clin Pharmacol. (2018) 84:2477–82. doi: 10.1111/bcp.13710
202. Grotenhermen F. Clinical pharmacokinetics of cannabinoids. J Cannabis Ther. (2003) 3:3–51. doi: 10.1300/J175v03n01_02
203. Toennes SW, Ramaekers JG, Theunissen EL, Moeller MR, Kauert GF. Comparison of cannabinoid pharmacokinetic properties in occasional and heavy users smoking a marijuana or placebo joint. J Anal Toxicol. (2008) 32:470–7. doi: 10.1093/jat/32.7.470
204. Huestis MA. Human cannabinoid pharmacokinetics. Chem Biodivers. (2007) 4:1770–804. doi: 10.1002/cbdv.200790152
205. Badowski ME. A review of oral cannabinoids and medical marijuana for the treatment of chemotherapy-induced nausea and vomiting: a focus on pharmacokinetic variability and pharmacodynamics. Cancer Chemother Pharmacol. (2017) 80:441–9. doi: 10.1007/s00280-017-3387-5
206. Siemens AJ, Walczak D, Buckley FE. Characterization of blood disappearance and tissue distribution of [3H]cannabidiol. Biochem Pharmacol. (1980) 29:462–4. doi: 10.1016/0006-2952(80)90532-8
207. Hazekamp A, Ware MA, Muller-Vahl KR, Abrams D, Grotenhermen F. The medicinal use of cannabis and cannabinoids-an international cross-sectional survey on administration forms. J Psychoactive Drugs. (2013) 45:199–210. doi: 10.1080/02791072.2013.805976
208. Baguley D, McFerran D, Hall D. Tinnitus. Lancet. (2013) 382:1600–7. doi: 10.1016/S0140-6736(13)60142-7
209. McFerran DJ, Stockdale D, Holme R, Large CH, Baguley DM. Why is there no cure for tinnitus? Front Neurosci. (2019) 13:802. doi: 10.3389/fnins.2019.00802
210. Shore SE, Roberts LE, Langguth B. Maladaptive plasticity in tinnitus–triggers, mechanisms and treatment. Nat Rev Neurol. (2016) 12:150–60. doi: 10.1038/nrneurol.2016.12
211. Brotherton H, Turtle C, Plack CJ, Munro KJ, Schaette R. Earplug-induced changes in acoustic reflex thresholds suggest that increased subcortical neural gain may be necessary but not sufficient for the occurrence of tinnitus. Neuroscience. (2019) 407:192–9. doi: 10.1016/j.neuroscience.2019.03.017
212. Guest H, Munro KJ, Prendergast G, Howe S, Plack CJ. Tinnitus with a normal audiogram: Relation to noise exposure but no evidence for cochlear synaptopathy. Hear Res. (2017) 344:265–74. doi: 10.1016/j.heares.2016.12.002
213. Hullfish J, Sedley W, Vanneste S. Prediction and perception: Insights for (and from) tinnitus. Neurosci Biobehav Rev. (2019) 102:1–12. doi: 10.1016/j.neubiorev.2019.04.008
214. Möhrle D, Hofmeier B, Amend M, Wolpert S, Ni K, Bing D, et al. Enhanced central neural gain compensates acoustic trauma-induced cochlear impairment, but unlikely correlates with tinnitus and hyperacusis. Neuroscience. (2019) 407:146–69. doi: 10.1016/j.neuroscience.2018.12.038
215. Sedley W. Tinnitus: does gain explain? Neuroscience. (2019) 407:213–28. doi: 10.1016/j.neuroscience.2019.01.027
216. Rauschecker JP, May ES, Maudoux A, Ploner M. Frontostriatal gating of tinnitus and chronic pain. Trends Cogn Sci. (2015) 19:567–78. doi: 10.1016/j.tics.2015.08.002
217. Sedley W, Friston KJ, Gander PE, Kumar S, Griffiths TD. An integrative tinnitus model based on sensory precision. Trends Neurosci. (2016) 39:799–812. doi: 10.1016/j.tins.2016.10.004
218. Brozoski TJ, Bauer CA. Animal models of tinnitus. Hear Res. (2016) 338:88–97. doi: 10.1016/j.heares.2015.10.011
219. Wallhäusser-Franke E, D'Amelio R, Glauner A, Delb W, Servais JJ, Hörmann K, et al. Transition from acute to chronic tinnitus: predictors for the development of chronic distressing tinnitus. Front Neurol. (2017) 8:605. doi: 10.3389/fneur.2017.00605
220. Husain FT. Neural networks of tinnitus in humans: Elucidating severity and habituation. Hear Res. (2016) 334:37–48. doi: 10.1016/j.heares.2015.09.010
221. Elgoyhen AB, Langguth B, De Ridder D, Vanneste S. Tinnitus: perspectives from human neuroimaging. Nat Rev Neurosci. (2015) 16:632–42. doi: 10.1038/nrn4003
222. Baizer JS, Manohar S, Paolone NA, Weinstock N, Salvi RJ. Understanding tinnitus: the dorsal cochlear nucleus, organization and plasticity. Brain Res. (2012) 1485:40–53. doi: 10.1016/j.brainres.2012.03.044
223. Zhao Y, Rubio M, Tzounopoulos T. Mechanisms underlying input-specific expression of endocannabinoid-mediated synaptic plasticity in the dorsal cochlear nucleus. Hear Res. (2011) 279:67–73. doi: 10.1016/j.heares.2011.03.007
224. Ghosh S, Sheth S, Sheehan K, Mukherjea D, Dhukhwa A, Borse V, et al. The endocannabinoid/cannabinoid receptor 2 system protects against cisplatin-induced hearing loss. Front Cell Neurosci. (2018) 12:271. doi: 10.3389/fncel.2018.00271
225. Bhatta P, Dhukhwa A, Sheehan K, Al Aameri RFH, Borse V, Ghosh S, et al. Capsaicin protects against cisplatin ototoxicity by changing the STAT3/STAT1 ratio and activating cannabinoid (CB2) receptors in the cochlea. Sci Rep. (2019) 9:4131. doi: 10.1038/s41598-019-40425-9
226. Chen YC, Li X, Liu L, Wang J, Lu CQ, Yang M, et al. Tinnitus and hyperacusis involve hyperactivity and enhanced connectivity in auditory-limbic-arousal-cerebellar network. Elife. (2015) 4:e06576. doi: 10.7554/eLife.06576.012
227. He W, Yu J, Sun Y, Kong W. Macrophages in noise-exposed cochlea: changes, regulation and the potential role. Aging Dis. (2020) 11:191–9. doi: 10.14336/AD.2019.0723
228. Miyao M, Firestein GS, Keithley EM. Acoustic trauma augments the cochlear immune response to antigen. Laryngoscope. (2008) 118:1801–8. doi: 10.1097/MLG.0b013e31817e2c27
229. Baizer JS, Wong KM, Manohar S, Hayes SH, Ding D, Dingman R, et al. Effects of acoustic trauma on the auditory system of the rat: the role of microglia. Neuroscience. (2015) 303:299–311. doi: 10.1016/j.neuroscience.2015.07.004
230. Hu SS, Mei L, Chen JY, Huang ZW, Wu H. Effects of salicylate on the inflammatory genes expression and synaptic ultrastructure in the cochlear nucleus of rats. Inflammation. (2014) 37:365–73. doi: 10.1007/s10753-013-9748-2
231. Manohar S, Dahar K, Adler HJ, Dalian D, Salvi R. Noise-induced hearing loss: neuropathic pain via Ntrk1 signaling. Mol Cell Neurosci. (2016) 75:101–12. doi: 10.1016/j.mcn.2016.07.005
232. Fuentes-Santamaría V, Alvarado JC, Melgar-Rojas P, Gabaldón-Ull MC, Miller JM, Juiz JM. The role of glia in the peripheral and central auditory system following noise overexposure: contribution of TNF-α and IL-1β to the pathogenesis of hearing loss. Front Neuroanat. (2017) 11:9. doi: 10.3389/fnana.2017.00009
233. Zheng Y, Baek JH, Smith PF, Darlington CL. Cannabinoid receptor down-regulation in the ventral cochlear nucleus in a salicylate model of tinnitus. Hear Res. (2007) 228:105–11. doi: 10.1016/j.heares.2007.01.028
234. Curry RJ, Peng K, Lu Y. Neurotransmitter- and release-mode-specific modulation of inhibitory transmission by group I metabotropic glutamate receptors in central auditory neurons of the mouse. J Neurosci. (2018) 38:8187–99. doi: 10.1523/JNEUROSCI.0603-18.2018
235. Valdés-Baizabal C, Parras GG, Ayala YA, Malmierca MS. Endocannabinoid modulation of stimulus-specific adaptation in inferior colliculus neurons of the rat. Sci Rep. (2017). 7:6997. doi: 10.1038/s41598-017-07460-w
236. Medeiros P, de Freitas RL, Silva MO, Coimbra NC, Melo-Thomas L. CB1 cannabinoid receptor-mediated anandamide signaling mechanisms of the inferior colliculus modulate the haloperidol-induced catalepsy. Neuroscience. (2016) 337:17–26. doi: 10.1016/j.neuroscience.2016.08.047
237. Trettel J, Levine ES. Cannabinoids depress inhibitory synaptic inputs received by layer 2/3 pyramidal neurons of the neocortex. J Neurophysiol. (2002) 88:534–9. doi: 10.1152/jn.2002.88.1.534
238. Toal KL, Radziwon KE, Holfoth DP, Xu-Friedman MA, Dent ML. Audiograms, gap detection thresholds, and frequency difference limens in cannabinoid receptor 1 knockout mice. Hear Res. (2016) 332:217–22. doi: 10.1016/j.heares.2015.09.013
239. Leishman E, Mackie K, Bradshaw HB. Elevated levels of arachidonic acid-derived lipids including prostaglandins and endocannabinoids are present throughout ABHD12 knockout brains: novel insights into the neurodegenerative phenotype. Front Mol Neurosci. (2019) 12:142. doi: 10.3389/fnmol.2019.00142
240. Kedzior KK, Martin-Iverson MT. Chronic cannabis use is associated with attention-modulated reduction in prepulse inhibition of the startle reflex in healthy humans. J Psychopharmacol. (2006) 20:471–84. doi: 10.1177/0269881105057516
241. Lisboa SF, Gomes FV, Terzian AL, Aguiar DC, Moreira FA, Resstel LB, et al. The endocannabinoid system and anxiety. Vitam Horm. (2017) 103:193–279. doi: 10.1016/bs.vh.2016.09.006
242. Fiskerstrand T, H'mida-Ben Brahim D, Johansson S, et al. Mutations in ABHD12 cause the neurodegenerative disease PHARC: An inborn error of endocannabinoid metabolism. Am J Hum Genet. (2010). 87:410–7. doi: 10.1016/j.ajhg.2010.08.002
243. Blankman JL, Long JZ, Trauger SA, Siuzdak G, Cravatt BF. ABHD12 controls brain lysophosphatidylserine pathways that are deregulated in a murine model of the neurodegenerative disease PHARC. Proc Natl Acad Sci USA. (2013). 110:1500–5. doi: 10.1073/pnas.1217121110
244. Kamat SS, Camara K, Parsons WH, et al. Immunomodulatory lysophosphatidylserines are regulated by ABHD16A and ABHD12 interplay. Nat Chem Biol. (2015). 11:164–71. doi: 10.1038/nchembio.1721
245. Ogasawara D, Ichu TA, Vartabedian VF, Benthuysen J, Jing H, Reed A, et al. Selective blockade of the lyso-PS lipase ABHD12 stimulates immune responses in vivo. Nat Chem Biol. (2018) 14:1099–108. doi: 10.1038/s41589-018-0155-8
246. Tschöp J, Kasten KR, Nogueiras R, Goetzman HS, Cave CM, England LG, et al. The cannabinoid receptor 2 is critical for the host response to sepsis. J Immunol. (2009) 183:499–505. doi: 10.4049/jimmunol.0900203
247. Li Y, Kim J. CB2 cannabinoid receptor knockout in mice impairs contextual long-term memory and enhances spatial working memory. Neural Plast. (2016). 2016:9817089. doi: 10.1155/2016/9817089
248. Hwang JH, Chan YC. Expression of dopamine receptor 1A and cannabinoid receptor 1 genes in the cochlea and brain after salicylate-induced tinnitus. ORL J Otorhinolaryngol Relat Spec. (2016) 78:268–75. doi: 10.1159/000449170
249. Sheth S, Mukherjea D, Rybak LP, Ramkumar V. Mechanisms of cisplatin-induced ototoxicity and otoprotection. Front Cell Neurosci. (2017) 11:338. doi: 10.3389/fncel.2017.00338
250. Jiang M, Li H, Johnson A, Karasawa T, Zhang Y, Meier WB, et al. Inflammation up-regulates cochlear expression of TRPV1 to potentiate drug-induced hearing loss. Sci Adv. (2019) 5:eaaw1836. doi: 10.1126/sciadv.aaw1836
251. Blair HA. Capsaicin 8% dermal patch: a review in peripheral neuropathic pain. Drugs. (2018) 78:1489–500. doi: 10.1007/s40265-018-0982-7
252. Ashton JC, Appleton I, Darlington CL, Smith PF. Cannabinoid CB1 receptor protein expression in the rat choroid plexus: a possible involvement of cannabinoids in the regulation of cerebrospinal fluid. Neurosci Lett. (2004) 364:40–2. doi: 10.1016/j.neulet.2004.04.016
253. Baek JH, Zheng Y, Darlington CL, Smith PF. Cannabinoid CB2 receptor expression in the rat brainstem cochlear and vestibular nuclei. Acta Otolaryngol. (2008) 128:961–7. doi: 10.1080/00016480701796944
254. Molina-Holgado F, Rubio-Araiz A, García-Ovejero D, Williams RJ, Moore JD, Arévalo-Martín A, et al. CB2 cannabinoid receptors promote mouse neural stem cell proliferation. Eur J Neurosci. (2007) 25:629–34. doi: 10.1111/j.1460-9568.2007.05322.x
255. Tighilet B, Dutheil S, Siponen MI, Noreña AJ. Reactive neurogenesis and down-regulation of the potassium-chloride cotransporter KCC2 in the cochlear nuclei after cochlear deafferentation. Front Pharmacol. (2016) 7:281. doi: 10.3389/fphar.2016.00281
256. Terr LI, Edgerton BJ. Physical effects of the choroid plexus on the cochlear nuclei in man. Acta Otolaryngol. (1985) 100:210–7. doi: 10.3109/00016488509104783
257. Perin P, Voigt FF, Bethge P, Helmchen F, Pizzala R. iDISCO+ for the study of neuroimmune architecture of the rat auditory brainstem. Front Neuroanat. (2019) 13:15. doi: 10.3389/fnana.2019.00015
258. Marques F, Sousa JC, Brito MA, Pahnke J, Santos C, Correia-Neves M, et al. The choroid plexus in health and in disease: dialogues into and out of the brain. Neurobiol Dis. (2017). 107:32–40. doi: 10.1016/j.nbd.2016.08.011
259. Bhattacharyya S, Falkenberg I, Martin-Santos R, Atakan Z, Crippa JA, Giampietro V, et al. Cannabinoid modulation of functional connectivity within regions processing attentional salience. Neuropsychopharmacology. (2015) 40:1343–52. doi: 10.1038/npp.2014.258
260. Bonn-Miller MO, Zvolensky MJ, Bernstein A. Marijuana use motives: concurrent relations to frequency of past 30-day use and anxiety sensitivity among young adult marijuana smokers. Addict Behav. (2007) 32:49–62. doi: 10.1016/j.addbeh.2006.03.018
261. Hall W, Solowij N. Adverse effects of cannabis. Lancet. (1998) 352:1611–6. doi: 10.1016/S0140-6736(98)05021-1
262. Viveros MP, Llorente R, Moreno E, Marco EM. Behavioural and neuroendocrine effects of cannabinoids in critical developmental periods. Behav Pharmacol. (2005) 16:353–62. doi: 10.1097/00008877-200509000-00007
263. Fusar-Poli P, Crippa JA, Bhattacharyya S, Borgwardt SJ, Allen P, Martin-Santos R, et al. Distinct effects of {delta}9-tetrahydrocannabinol and cannabidiol on neural activation during emotional processing. Arch Gen Psychiatry. (2009) 66:95–105. doi: 10.1001/archgenpsychiatry.2008.519
264. Papagianni EP, Stevenson CW. Cannabinoid regulation of fear and anxiety: an update. Curr Psychiatry Rep. (2019) 21:38. doi: 10.1007/s11920-019-1026-z
265. White CM. A Review of human studies assessing Cannabidiol's (CBD) therapeutic actions and potential. J Clin Pharmacol. (2019) 47:10. doi: 10.1002/jcph.1387
266. Elms L, Shannon S, Hughes S, Lewis N. Cannabidiol in the treatment of post-traumatic stress disorder: a case series. J Altern Complement Med. (2019) 25:392–7. doi: 10.1089/acm.2018.0437
267. Zheng Y, Reid P, Smith PF. Cannabinoid CB1 receptor agonists do not decrease, but may increase acoustic trauma-induced tinnitus in rats. Front Neurol. (2015) 6:60. doi: 10.3389/fneur.2015.00060
268. Zheng Y, Stiles L, Hamilton E, Smith PF, Darlington CL. The effects of the synthetic cannabinoid receptor agonists, WIN55,212-2 and CP55,940, on salicylate-induced tinnitus in rats. Hear Res. (2010) 268:145–50. doi: 10.1016/j.heares.2010.05.015
269. Berger JI, Coomber B, Hill S, Alexander SPH, Owen W, Palmer AR, et al. Effects of the cannabinoid CB1 agonist ACEA on salicylate ototoxicity, hyperacusis and tinnitus in guinea pigs. Hear Res. (2017) 356:51–62. doi: 10.1016/j.heares.2017.10.012
270. Boggs DL, Nguyen JD, Morgenson D, Taffe MA, Ranganathan M. Clinical and preclinical evidence for functional interactions of cannabidiol and Δ9-tetrahydrocannabinol. Neuropsychopharmacology. (2018) 43:142–54. doi: 10.1038/npp.2017.209
271. Bow EW, Rimoldi JM. The structure-function relationships of classical cannabinoids: CB1/CB2 modulation. Perspect Medicin Chem. (2016) 8:17–39. doi: 10.4137/PMC.S32171
272. Morgan CJ, Schafer G, Freeman TP, Curran HV. Impact of cannabidiol on the acute memory and psychotomimetic effects of smoked cannabis: naturalistic study: naturalistic study [corrected]. Br J Psychiatry. (2010) 197:285–90. doi: 10.1192/bjp.bp.110.077503
273. Ibeas Bih C, Chen T, Nunn AV, Bazelot M, Dallas M, Whalley BJ. Molecular targets of cannabidiol in neurological disorders. Neurotherapeutics. (2015) 12:699–730. doi: 10.1007/s13311-015-0377-3
274. Elsaid S, Kloiber S, Le Foll B. Effects of cannabidiol (CBD) in neuropsychiatric disorders: a review of pre-clinical and clinical findings. Prog Mol Biol Transl Sci. (2019) 167:25–75. doi: 10.1016/bs.pmbts.2019.06.005
275. Thomas A, Baillie GL, Phillips AM, Razdan RK, Ross RA, Pertwee RG. Cannabidiol displays unexpectedly high potency as an antagonist of CB1 and CB2 receptor agonists in vitro. Br J Pharmacol. (2007) 150:613–23. doi: 10.1038/sj.bjp.0707133
276. Laprairie RB, Bagher AM, Kelly MEM, Denovan-Wright EM. Cannabidiol is a negative allosteric modulator of the cannabinoid CB1 receptor. Br J Pharmacol. (2015) 172:4790–805. doi: 10.1111/bph.13250
277. Bisogno T, Sepe N, Melck D, Maurelli S, De Petrocellis L, Di Marzo V. Biosynthesis, release and degradation of the novel endogenous cannabimimetic metabolite 2-arachidonoylglycerol in mouse neuroblastoma cells. Biochem J. (1997) 322 (Pt 2)(Pt 2):671–7. doi: 10.1042/bj3220671
278. Massi P, Valenti M, Vaccani A, Gasperi V, Perletti G, Marras E, et al. 5-Lipoxygenase and anandamide hydrolase (FAAH) mediate the antitumor activity of cannabidiol, a non-psychoactive cannabinoid. J Neurochem. (2008) 104:1091–100. doi: 10.1111/j.1471-4159.2007.05073.x
279. Pazos MR, Mohammed N, Lafuente H, Santos M, Martínez-Pinilla E, Moreno E, et al. Mechanisms of cannabidiol neuroprotection in hypoxic-ischemic newborn pigs: role of 5HT(1A) and CB2 receptors. Neuropharmacology. (2013) 71:282–91. doi: 10.1016/j.neuropharm.2013.03.027
280. Laun AS, Song Z-H. GPR3 and GPR6, novel molecular targets for cannabidiol. Biochem Biophys Res Commun. (2017) 490:17–21. doi: 10.1016/j.bbrc.2017.05.165
281. Russo EB, Burnett A, Hall B, Parker KK. Agonistic properties of cannabidiol at 5-HT1a receptors. Neurochem Res. (2005) 30:1037–43. doi: 10.1007/s11064-005-6978-1
282. Austgen JR, Kline DD. Endocannabinoids blunt the augmentation of synaptic transmission by serotonin 2A receptors in the nucleus tractus solitarii (nTS). Brain Res. (2013) 1537:27–36. doi: 10.1016/j.brainres.2013.09.006
283. Yang KH, Galadari S, Isaev D, Petroianu G, Shippenberg TS, Oz M. The nonpsychoactive cannabinoid cannabidiol inhibits 5-hydroxytryptamine3A receptor-mediated currents in Xenopus laevis oocytes. J Pharmacol Exp Ther. (2010) 333:547–54. doi: 10.1124/jpet.109.162594
284. Hind WH, England TJ, O'Sullivan SE. Cannabidiol protects an in vitro model of the blood-brain barrier from oxygen-glucose deprivation via PPARγ and 5-HT1A receptors. Br J Pharmacol. (2016) 173:815–25. doi: 10.1111/bph.13368
285. de Mello Schier RA, de Oliveira Ribeiro PN, Coutinho SD, Machado S, Arias-Carrión O, Crippa AJ, et al. Antidepressant-like and anxiolytic-like effects of cannabidiol: a chemical compound of cannabis sativa. CNS Neurol Disord Drug Targets. (2014) 13:953–60. doi: 10.2174/1871527313666140612114838
286. Gonca E, Darici F. The effect of cannabidiol on ischemia/reperfusion-induced ventricular arrhythmias: the role of adenosine A1 receptors. J Cardiovasc Pharmacol Ther. (2015) 20:76–83. doi: 10.1177/1074248414532013
287. Castillo A, Tolón MR, Fernández-Ruiz J, Romero J, Martinez-Orgado J. The neuroprotective effect of cannabidiol in an in vitro model of newborn hypoxic-ischemic brain damage in mice is mediated by CB(2) and adenosine receptors. Neurobiol Dis. (2010) 37:434–40. doi: 10.1016/j.nbd.2009.10.023
288. Ahrens J, Demir R, Leuwer M, De La Roche J, Krampfl K, Foadi N, et al. The nonpsychotropic cannabinoid cannabidiol modulates and directly activates alpha-1 and alpha-1-beta glycine receptor function. Pharmacology. (2009) 83:217. doi: 10.1159/000201556
289. Xiong W, Cui T, Cheng K, Yang F, Chen SR, Willenbring D, et al. Cannabinoids suppress inflammatory and neuropathic pain by targeting alpha3 glycine receptors. J Exp Med. (2012) 209:1121. doi: 10.1084/jem.20120242
290. Mahgoub M, Keun-Hang SY, Sydorenko V, Ashoor A, Kabbani N, Al Kury L, et al. Effects of cannabidiol on the function of α7-nicotinic acetylcholine receptors. Eur J Pharmacol. (2013) 720:310–9. doi: 10.1016/j.ejphar.2013.10.011
291. Kathmann M, Flau K, Redmer A, Tränkle C, Schlicker E. Cannabidiol is an allosteric modulator at mu- and delta-opioid receptors. Naunyn Schmiedebergs Arch Pharmacol. (2006) 372:354–61. doi: 10.1007/s00210-006-0033-x
292. Deiana S, Watanabe A, Yamasaki Y, Amada N, Arthur M, Fleming S, et al. Plasma and brain pharmacokinetic profile of cannabidiol (CBD), cannabidivarine (CBDV), Δ9-tetrahydrocannabivarin (THCV) and cannabigerol (CBG) in rats and mice following oral and intraperitoneal administration and CBD action on obsessive-compulsive behaviour. Psychopharmacology. (2012). 219:859–73. doi: 10.1007/s00213-011-2415-0
293. Papke RL, Jon M. Lindstrom, Nicotinic acetylcholine receptors: conventional and unconventional ligands and signaling, Neuropharmacology. (2020) 168:8021. doi: 10.1016/j.neuropharm.2020.108021
294. Egea JI, Buendia E, Parada E, Navarro R, Leon MG. Lopez Anti-inflammatory role of microglial alpha, nAChRs and its role in neuroprotection. Biochem Pharmacol. (2015) 97:463–72. doi: 10.1016/j.bcp.2015.07.032
295. de Jonge WJ, van der Zanden EP, The FO, Bijlsma MF, van Westerloo DJ, Bennink RJ, et al. Stimulation of the vagus nerve attenuates macrophage activation by activating the Jak2-STAT3 signaling pathway. Nat Immunol. (2005) 6:844–51. doi: 10.1038/ni1229
296. Chatterjee PK, Al-Abed YB, Sherry CN. Metz Cholinergic agonists regulate JAK2/STAT3 signaling to suppress endothelial cell activation. Am J Physiol Cell Physiol. (2009) 297:C1294–306. doi: 10.1152/ajpcell.00160.2009
297. Marrero MB, Bencherif M. Convergence of alpha 7 nicotinic acetylcholine receptor-activated pathways for anti-apoptosis and anti-inflammation: central role for JAK2 activation of STAT3 and NF-kappaB. Brain Res. (2009) 1256:1–7. doi: 10.1016/j.brainres.2008.11.053
298. Zhang Q, Lu Y, Bian H, Guo L, Zhu H. Activation of the alpha7 nicotinic receptor promotes lipopolysaccharide-induced conversion of M1 microglia to M2. Am J Transl Res. (2017) 9:971–85.
299. Watkins AR. Cannabinoid interactions with ion channels and receptors. Channels. (2019) 13:162–7. doi: 10.1080/19336950.2019.1615824
300. Ross HR, Napier I, Connor M. Inhibition of recombinant human T-type calcium channels by Delta9-tetrahydrocannabinol and cannabidiol. J Biol Chem. (2008) 283:16124–34. doi: 10.1074/jbc.M707104200
301. De Petrocellis L, Ligresti A, Moriello AS, Allarà M, Bisogno T, Petrosino S, et al. Effects of cannabinoids and cannabinoid-enriched Cannabis extracts on TRP channels and endocannabinoid metabolic enzymes. Br J Pharmacol. (2011) 163:1479–94. doi: 10.1111/j.1476-5381.2010.01166.x
302. Bisogno TL, Hanus L, De Petrocellis S, Tchilibon DE, Ponde I, et al. Molecular targets for cannabidiol and its synthetic analogues: effect on vanilloid VR1 receptors and on the cellular uptake and enzymatic hydrolysis of anandamide. Br J Pharmacol. (2001) 134:845–52. doi: 10.1038/sj.bjp.0704327
Keywords: cannabinoids, tinnitus, auditory, neuroimmune, hearing
Citation: Perin P, Mabou Tagne A, Enrico P, Marino F, Cosentino M, Pizzala R and Boselli C (2020) Cannabinoids, Inner Ear, Hearing, and Tinnitus: A Neuroimmunological Perspective. Front. Neurol. 11:505995. doi: 10.3389/fneur.2020.505995
Received: 18 October 2019; Accepted: 18 August 2020;
Published: 23 November 2020.
Edited by:
Jose Antonio Lopez-Escamez, Andalusian Autonomous Government of Genomics and Oncological Research (GENYO), SpainReviewed by:
Paula Morales, Instituto de Química Médica (IQM), SpainZheng-Xiong Xi, National Institute on Drug Abuse (NIDA), United States
Copyright © 2020 Perin, Mabou Tagne, Enrico, Marino, Cosentino, Pizzala and Boselli. This is an open-access article distributed under the terms of the Creative Commons Attribution License (CC BY). The use, distribution or reproduction in other forums is permitted, provided the original author(s) and the copyright owner(s) are credited and that the original publication in this journal is cited, in accordance with accepted academic practice. No use, distribution or reproduction is permitted which does not comply with these terms.
*Correspondence: Paola Perin, cHBlcmluJiN4MDAwNDA7dW5pcHYuaXQ=