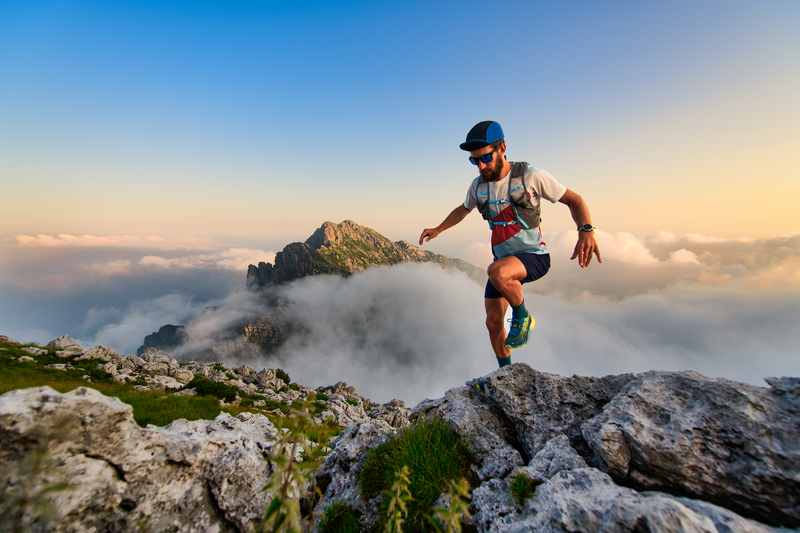
95% of researchers rate our articles as excellent or good
Learn more about the work of our research integrity team to safeguard the quality of each article we publish.
Find out more
CLINICAL TRIAL article
Front. Neurol. , 27 August 2020
Sec. Neurorehabilitation
Volume 11 - 2020 | https://doi.org/10.3389/fneur.2020.00948
This article is part of the Research Topic Neuropsychological and Cognitive-Behavioral Assessment of Neurodegenerative Disease and Rehabilitation Using New Technologies and Virtual Reality. View all 14 articles
A correction has been applied to this article in:
Corrigendum: Using Transcranial Direct Current Stimulation to Augment the Effect of Motor Imagery-Assisted Brain-Computer Interface Training in Chronic Stroke Patients—Cortical Reorganization Considerations
Introduction: Transcranial direct current stimulation (tDCS) has been shown to modulate cortical plasticity, enhance motor learning and post-stroke upper extremity motor recovery. It has also been demonstrated to facilitate activation of brain-computer interface (BCI) in stroke patients. We had previously demonstrated that BCI-assisted motor imagery (MI-BCI) can improve upper extremity impairment in chronic stroke participants. This study was carried out to investigate the effects of priming with tDCS prior to MI-BCI training in chronic stroke patients with moderate to severe upper extremity paresis and to investigate the cortical activity changes associated with training.
Methods: This is a double-blinded randomized clinical trial. Participants were randomized to receive 10 sessions of 20-min 1 mA tDCS or sham-tDCS before MI-BCI, with the anode applied to the ipsilesional, and the cathode to the contralesional primary motor cortex (M1). Upper extremity sub-scale of the Fugl-Meyer Assessment (UE-FM) and corticospinal excitability measured by transcranial magnetic stimulation (TMS) were assessed before, after and 4 weeks after intervention.
Results: Ten participants received real tDCS and nine received sham tDCS. UE-FM improved significantly in both groups after intervention. Of those with unrecordable motor evoked potential (MEP-) to the ipsilesional M1, significant improvement in UE-FM was found in the real-tDCS group, but not in the sham group. Resting motor threshold (RMT) of ipsilesional M1 decreased significantly after intervention in the real-tDCS group. Short intra-cortical inhibition (SICI) in the contralesional M1 was reduced significantly following intervention in the sham group. Correlation was found between baseline UE-FM score and changes in the contralesional SICI for all, as well as between changes in UE-FM and changes in contralesional RMT in the MEP- group.
Conclusion: MI-BCI improved the motor function of the stroke-affected arm in chronic stroke patients with moderate to severe impairment. tDCS did not confer overall additional benefit although there was a trend toward greater benefit. Cortical activity changes in the contralesional M1 associated with functional improvement suggests a possible role for the contralesional M1 in stroke recovery in more severely affected patients. This has important implications in designing neuromodulatory interventions for future studies and tailoring treatment.
Clinical Trial Registration: The study was registered at https://clinicaltrials.gov (NCT01897025).
Post-stroke recovery of upper extremity (UE) function remains a challenge. Less than 15% of stroke survivors with severe impairment experience complete motor recovery (1, 2). Intensive and repetitive practice is effective for motor recovery (3), but is labor-intensive and costly. More effective rehabilitation strategies that will deliver better functional outcomes without increasing cost of care are needed.
Motor imagery (MI), or mental practice is a mental rehearsal process of a specific movement without physical performance to enhance post-stroke upper extremity motor recovery (4–10). It has been demonstrated to be a safe, self-paced method to improve motor performance in athletes (6) and is effective in augmenting the effects of motor practice in stroke patients (7–9).
MI shares similar neural substrates with motor execution (11, 12). Functional neural changes induced by MI is similar to that of short-term motor learning (5) with corresponding changes in corticospinal excitability and reorganization of motor representation have been demonstrated with MI (4, 13).
Robot-assisted training is typically applied to deliver intensive, task-specific training in rehabilitation of motor function, but has also been used to provide appropriate sensorimotor integration through guidance of movement along a trajectory (14–18). The coupling of MI and robot-assisted arm movement through brain computer interface (MI-BCI) has been postulated to enhance sensorimotor integration by bridging the motor intent and providing appropriate somatosensory feedback through passive manipulation of the paretic arm, thereby guiding activity-dependent cortical plasticity through feedback on brain activity (19). Our previous studies of MI-BCI in chronic stroke demonstrated better improvement in motor function with fewer repetitions in the same time of training (20, 21). Others have found similar benefit using BCI-driven orthoses for rehabilitation of severe UE paresis (22).
Transcranial direct current stimulation (tDCS) is a non-invasive method of modulating corticospinal excitability by changing the firing threshold of neuronal membrane and modifying spontaneous activity according to the direction of current, such that cathodal tDCS decreases cortical excitability while anodal tDCS increases it (23–25). Good functional recovery has frequently been associated with a rebalancing of interhemispheric inhibition (17, 26). Based on this, cathodal tDCS is applied to the contralesional primary motor cortex (M1) and anodal tDCS to the ipsilesional M1 to enhance corticospinal excitability. This is the paradigm most frequently studied to enhance motor recovery after stroke (27–31), and has thus far yielded mixed results (32).
Additionally, tDCS has also been explored as a priming tool to improve the accuracy of BCI, both in healthy subjects (33, 34) and in stroke patients with mixed results (35, 36). We had previously reported the preliminary results of the first ever study to investigate the effect of a course of training with BCI-assisted motor imagery (MI-BCI) with tDCS priming (simultaneous anodal stimulation to the ipsilesional M1 and cathodal stimulation to the contralesional M1) prior to each session, compared to MI-BCI with sham tDCS, on recovery of chronic stroke patients with moderate to severe impairment (37). This population was chosen as they have the most difficulty engaging in active motor task training. The stimulation protocol was selected based on the intent to rebalance transcallosal inhibition, as suggested by previous studies (28, 30, 38). Clinical improvement was observed post-training, with online BCI accuracies being significantly better in the tDCS group, compared to the sham group.
The neurobiological principles that govern post-stroke recovery of motor function are incompletely understood. While task-specific training, and MI as an extension, is applied based on principles of activity-dependent cortical plasticity, and non-invasive brain stimulation is applied based on rebalancing of interhemispheric inhibitions, a more detailed understanding of the cortical reorganization associated with the combination of therapeutic modalities, and indeed of the recovery process itself, is required in order to tailor therapeutic approaches. TMS may be used to probe these changes in cortical excitability. Here we report the changes in cortical activity associated with this training protocol, which will inform the design of future studies.
A total of 42 participants were screened for eligibility for the study. All screening and study procedures were performed at the National University Hospital, Singapore. All partcipants provided voluntary, written informed consent in accordance with the Declaration of Helsinki. The study was approved by the National Healthcare Group Domain-Specific Review Board and was registered at https://clinicaltrials.gov (NCT01897025).
Patients 21–80 years old with a history of unilateral, single, hemorrhagic, or ischemic supratentorial stroke more than 9 months prior to enrolment, with upper extremity component of the Fugl-Meyer Assessment (UE-FM) (39) between 11 and 45 (moderate to severe motor impairment of arm) were eligible for inclusion. Participants were excluded based on the following: (1) inability to operate the MI-BCI system; (2) contraindications to TMS/tDCS including previous cranial surgeries, ferromagnetic implants, and seizures; (3) other factors affecting UE movement: severe pain in the affected UE that may be exacerbated by the use of the robotic device, major depression; (4) other neurological disorders.
Sample size calculation was based on the minimal clinically important difference (MCID) score of the UE-FMA score, which is estimated to be 10 in a population of stroke patients with severe UE paresis (standard deviation of 10.73) (40). Based on a two-sided level of significance of 5% and a statistical power of 80%, the number of participants required is estimated to be 40 for a two-armed parallel-design study.
This was a prospective, double-blinded, randomized controlled trial. Participants were randomized into real- or sham-tDCS intervention groups using a computer-generated stratified randomization approach. The randomization number generated was kept in a sealed envelope and was issued to the study coordinator before the start of intervention for each participant. Both the participants and assessors were blinded to the intervention that participants received.
Participants were initially screened for eligibility and ability to effectively activate the BCI system. Those who passed screening were randomly allocated to either real-tDCS or sham-tDCS group. Each received 10 sessions of real- or sham-tDCS, followed immediately by MI-BCI assisted robotic arm training. The intervention was conducted daily over 2 consecutive weeks.
Direct current was delivered by a stimulator (NeuroConn, Germany) through rubber electrodes embedded in saline-soaked 50 ×70 mm2 sponge bags at an intensity of 1 mA. The anodal electrode was placed over the ipsilesional M1 and the cathodal electrode was placed over the contralesional M1. Stimulation intensity was ramped up to 1 mA over 30 s and maintained for 20 min, before ramping down. Sham-tDCS was delivered by similarly ramping up to 1 mA but maintained for only 20 s to give participants the same scalp sensation, before ramping down (29). tDCS intervention lasted for 20 min for both groups so that participants were blinded to their group allocation.
The MI-BCI protocol has been detailed in previous publication (37). In short, 27-channel electroencephalogram (EEG) signals were recorded by NuAmp EEG amplifier (Compumedic, Germany). The Inmotion2 MIT-Manus robot (Interactive Motion Technologies, MA, USA) was used to provide unrestricted unilateral passive and active shoulder and elbow movements in the horizontal plane (41). Visual feedback from the screen indicated the success or failure of MI detection for each MI task. Once motor intention was successfully detected, the robot-assisted motion would be triggered according to the clock exercise therapy of the MIT-Manus robot (42).
All outcome measures were performed within 1 week prior to commencement of the intervention (PRE), within 1 week after the intervention was completed (POST1), and again at 4 weeks post-intervention (POST2).
The UE-FM (39) was the primary outcome measure in this study. UE-FM assessment was performed by a single senior occupational therapist who was blinded to the group allocation.
TMS measurement of corticospinal excitability was performed by a single research assistant. Resting motor threshold (RMT), short intra-cortical inhibition (SICI) and short intra-cortical facilitation (SICF) were measured using transcranial magnetic stimulation of the primary motor cortex, with participant sitting upright in a chair with back supported, looking forward, with both forearms resting comfortably on pillows and elbows supported at 90°. Motor-evoked potentials (MEPs) were recorded from the abductor pollicus brevis (APB) via surface electrodes in a belly-tendon arrangement, by Medelec Synergy Electromyography (EMG) system (VIASYS Healthcare, UK). Single- and paired-pulse TMS were delivered through a 70 mm figure-of-eight coil using Bistim 2002 (Magstim Co., UK). The coil was placed on the scalp with the handle pointing posteriorly at a 45° between the coronal and sagittal planes. The optimal scalp position for activating APB was determined from initial exploration over a 1-cm grid marked on a swimming cap worn over the head.
RMT was defined by the lowest intensity eliciting peak-to-peak MEP amplitude of 50 μV, in at least five out of 10 trials from single-pulse TMS stimulation (43). SICI and SICF were measured using paired-pulse stimulation with a conditioning stimulus of 80% of RMT followed by a test stimulus of 120% of RMT. MEPs were recorded with different inter-stimulus intervals (ISIs). ISI of 2 ms typically induces SICI while ISIs of 10 and 15 ms reflect SICF (44, 45).
Adverse effects were monitored using a questionnaire documenting pain and discomfort at the stimulation site. The Beck Depression Inventory, the Fatigue Severity Scale, and the forward and backward digit span tests were administered for possible psychological and cognitive changes which may be potential confounders.
All statistical analysis was carried out using the IBM SPSS version 23 software. Linear mixed model with an unstructured covariance matrix and Bonferroni adjustment was used to compare differences between the two intervention groups (real-tDCS vs. sham tDCS) and among three time points (PRE, POST1, and POST2). Differences in the baseline UE-FM between two groups were analyzed by the student t-test. Chi-Square test was used to compare differences between categorical data. Correlation of non-parametric data was analyzed using Spearman Correlation. P <0.05 was set as the level for statistical significance.
We further analyzed the data from participants whose MEP of the ipsilesional M1 were unrecordable even at maximal TMS stimulator output (MEP-). Absence of MEP is associated with poorer functional outcomes in stroke patients (46).
We were not be able to reach the planned recruitment target. Of 42 chronic stroke patients who underwent screening, 19 were recruited, 10 to the real-tDCS group and nine to the sham-tDCS group. All completed the intervention and the follow-up evaluations (Figure 1). Six patients in the real-tDCS group and five patients in the sham-tDCS group were MEP- at the baseline. There was no statistical difference between groups in baseline demographic and stroke characteristics (Table 1), as has been reported previously (37).
Figure 1. CONSORT flow diagram. Forty-two participants were screened. Nineteen participants completed the intervention and follow-up evaluation and were included in the final analysis−10 in the real-tDCS group, nine in the sham-tDCS group.
Both the real- and sham-tDCS groups improved significantly in the UE-FM after intervention [from 35.3 ± 7.8 (PRE) to 36.2 ± 8.8 (POST1) and 40.3 ± 7.8 (POST2), F = 7.64; p = 0.01 for real-tDCS group; from 32.6 ± 8.1 (PRE) to 35.3 ± 9.6 (POST1) and 37.8 ± 11.4 (POST2), F = 4.85; p = 0.04 for sham group], with no statistically significant difference between groups (F = 0.23, p = 0.64). The analysis on ΔUE-FM (UE-FM compared to pre-intervention) was previously reported (37). ΔUE-FM was significantly higher at POST2, compared to POST1 in real-tDCS group [from 0.9 ± 3.0 (POST1) to 5.0 ± 4.4 (POST2), F = 13.64; p = 0.005], but not in the sham group [from 2.8 ± 4.0 (POST1) to 6.1 ± 5.7 (POST2), F = 4.45; p = 0.07] (Figure 2).
Figure 2. UE-FM score (A) and ΔUE-FM (B) in both groups. (A) Both groups improved significantly in UE-FM at POST2 after intervention (n = 10 for real-tDCS group, n = 9 for sham-tDCS group). Between group difference was not statistically significant. (B) ΔUE-FM (changes in UE-FM score compared to PRE) was significantly higher at POST2, compared to POST1 in the real-tDCS group, not in the sham group. Data shows mean ± SEM.
When MEP- participants were considered alone, significant improvement in UE-FM was found only in the real-tDCS group [from 36.0 ± 5.5 (PRE) to 38.0 ± 6.4 (POST1) and 41.3 ± 7.1 (POST2), F = 9.71, p = 0.02], but not in the sham-tDCS group [from 31.2 ± 6.8 (PRE) to 32.6 ± 8.3 (POST1) and 32.5 ± 6.5 (POST2), F = 0.88, p = 0.50] (Figure 3).
Figure 3. UE-FM (A) and ΔUE-FM (B) in both groups in MEP- participants. (A) Significant improvement in UE-FM in the real-tDCS group (n = 6), but not the sham-tDCS group (n = 5). (B) No significant difference in ΔUE-FM was shown between groups, or over time. Data shows mean ± SEM.
There was significant difference in RMT of the ipsilesional M1 over time in real-tDCS group [from 0.80 ± 0.04 (PRE) to 0.72 ± 0.07 (POST1) and 0.67 ± 0.06 (POST2), F = 12.67; p = 0.00], but not in the sham-tDCS group [from 0.83 ± 0.17 (PRE) to 0.87 ± 0.08 (POST1) and 0.82 ± 0.12 (POST2), F = 3.00; p = 0.19]. Post-hoc Bonferroni test showed that RMT of the real-tDCS group was significantly lower at POST1 and POST2, compared to PRE (p = 0.0001 and 0.01, respectively). The overall difference between real and sham groups was statistically significant (F = 15.12; p = 0.01). No significant within- and between-group differences in the RMT were found in the contralesional M1 (Figure 4).
Figure 4. RMT in the ipsilesional M1 (A) and contralesional M1 (B). (A) Significant reduction in RMT in ipsilesional M1 in real-tDCS (n = 6) group at POST1 and POST2, compared to PRE, but not in sham-tDCS group (n = 5). The overall difference between groups was statistically significant. (B) No significant difference in RMT in contralesional side between two groups (n = 10 for real-tDCS group, n = 9 for sham-tDCS group), or over time. Data shows mean ± SEM.
The interventions reduced SICI in the contralesional M1 significantly, as measured at ISI of 2 ms (SICI2ms), in the contralesional M1 (F = 9.34, p = 0.00), when both groups were combined. The sham-tDCS group had significantly reduced SICI2ms following intervention [from −52.2 ± 11.6 (PRE) to −36.3 ± 8.3 (POST1) and −35.9 ± 8.7 (POST2), F = 27.15, p = 0.00]. The difference in the real-tDCS group was not significant, as was the difference between groups (Figure 5). There was no significant difference in SICF between groups, or over time.
Figure 5. SICI (ISI 2ms) in contralesional M1 in both groups. The sham-tDCS group (n = 9) had significantly reduced SICI2ms at POST1 and POST2, compared PRE. No difference over time was observed in real-tDCS group (n = 10). Between-group difference was not significant.
Spearman's correlation was used to investigate the relationships between clinical outcome measures (UE-FM) and contralesional corticospinal excitability. Correlation was found between the UE-FM score and the contralesional RMT such that a higher UE-FM score was associated with lower contralesional RMT (r = −0.315, p = 0.019). A lower UE-FM score was also associated with greater changes in contralesional SICI2ms (ΔSICI2ms) (r = −0.420, p = 0.012) (Table 2). When MEP- participants were considered alone, a greater reduction in contralesional RMT (ΔRMT, difference from PRE) was associated with greater improvement in UE-FM score (ΔUE-FM, difference from PRE) (r = −0.463; p = 0.034) (Table 2).
No complications of tDCS or MI-BCI were reported by participants during and after intervention. There was no within- and between-group difference in the forward and backward digit-span, the Beck Depression Inventory and the Fatigue Severity Scale.
In this preliminary study, both the real- and sham-tDCS groups improved significantly in UE function with MI-BCI training. The intervention of MI-BCI with tDCS prior to it was safe and well-tolerated by our patients. MI-BCI training was again demonstrated to improve motor function despite initial moderate to severe motor impairment, with gains continuing up to 4 weeks post-intervention, which were greater in extent in the real-tDCS group.
Previous evidence suggests that modulation of cortical excitability with tDCS prior to task training may result in greater improvements in motor outcomes (27, 29–31, 47). A recent systematic review suggested that response to contralesional inhibitory neuromodulation may be affected by timing—while smaller studies demonstrated a definite effect in UE stroke recovery in the post-acute stage, one large study in the chronic stage did not demonstrate improved UE function (48). Whether this was because of the heterogeneity of the participants or the decreased response to modulation in the chronic phase is debatable. The lack of clear, additional clinical benefit in adding tDCS to MI-BCI training in our study may be attributed to the small sample size which had not reach our planned recruitment target, and the relatively short training period in the context of chronic stroke. Indeed, there was a trend toward significant difference between the tDCS and sham groups, in favor of the tDCS group. With the inclusion of more patients and a longer training duration, we may see a significant effect between groups.
Patient selection and the stimulation protocol selected, may have also contributed to the lack of observable difference. Recent literature suggests that recovery of motor function post-stroke follows a relatively predictable “proportional recovery rule” (49–51), which describes the potential for recovery ~70% of the maximum possible. Integrity of the corticospinal tract is an important factor determining adherence to this rule (“fitters”) (49, 51). Those without intact corticospinal tracts tend to be “non-fitters” to the rule, have more severe impairments and show poorer recovery.
Our population of patients were mostly those with undetectable MEPs at the time of recruitment. It has been suggested that such “non-fitters” may adopt different neural mechanisms to achieve recovery compared to those with greater integrity of the corticospinal tracts. Di Pino et al. proposed a bimodal balance-recovery model in which those with high structural reserves would achieve best recovery through rebalancing of interhemispheric inhibition, while those with low structural reserves (i.e., Larger area of damage and more severe impairment) may achieve better outcomes through promotion of vicarious activity in the unaffected hemisphere (52). We based our choice of tDCS protocol on the intent to rebalance interhemispheric inhibition, based on previous literature, without considering the integrity of the corticospinal tract. This may have contributed to the lack of observed efficacy. Indeed, more recent studies have applied a stratified approach using clinical and functional imaging cut-offs to facilitate selection of a tailored stimulation protocol (facilitation vs. inhibition of the contralesional hemisphere) (53).
Notwithstanding, we were able to observe a clinical improvement in both groups. We found a correlation between the degree of impairment in the stroke-affected arm and the degree of change in intracortical inhibition on the non-lesioned hemisphere. Furthermore, in the MEP- group, improvement in function was associated with increased corticospinal excitability on the contralesional motor cortex. These findings suggest a role of the contralesional hemisphere in the recovery of motor function post-stroke.
Cortical reorganization, with an increase of excitability of the contralesional hemisphere has been observed repeatedly following stroke (54–56). But the significance of this in motor recovery remains uncertain (57). Inhibition of the contralesional hemisphere has been shown to lead to worsening of function in the stroke-affected limb in both animals and humans (58, 59). Whether bilateral activation during task performance reflects poorer outcomes is debated. Some have found that bilateral activation portends poor outcome (60), while others have found it persists in well-recovered stroke patients (61, 62). Of note, the extent of contralesional activation relates to the degree of motor skill challenge (63) and would be an important consideration in relation to the extent of motor impairment. In terms of exploring alternative approaches to non-invasive brain stimulation, the contralesional premotor cortex has been identified as a promising target in preliminary studies to augment recovery for the more severely affected stroke patients, while little effect has been demonstrated with facilitation or perturbation of the contralesional M1 (53, 59).
The mechanism by which the contralesional motor cortex may facilitates motor recovery is a matter of active investigation. Indeed, the interhemispheric inhibition in stroke recovery has been questioned in recent studies. Premovement interhemispheric inhibition in a group of mild to moderately impaired stroke patients was found to be preserved early post-stroke and only became abnormal in the chronic phase, with no cross-sectional correlation with functional recovery (64). Studies using TMS have demonstrated that the increased contralesional M1 excitability is not causally related to the decreased transcallosal inhibition from the ipsilesional M1 (56). But rather, a decrease in intracortical inhibition as measured by SICI, which reflects the activity of GABAAergic interneurons (65), may mediate the contralesional M1 reorganization. The relatively suppressed inhibitory effect of the conditioning stimulus at higher intensity suggests a shift in the balance of excitatory and inhibitory activity toward an increase in contralesional excitatory activity (55). Such a reduction in SICI in the subacute period post-stroke is associated with significant functional improvement and may reflect the unmasking of latent networks critical for cortical reorganization (55, 66). Our finding that clinical improvement correlated with a reduction in contralesional SICI suggests that such a decrease in GABAA-mediated inhibition may also play a role in contralesional reorganization associated with functional improvement, even in the chronic phase of stroke. Further investigation is required to ascertain this.
Finally, with regard to how tDCS may augment MI-BCI training, we had previously demonstrated an increase in MI detection accuracy with real-tDCS compared to sham-tDCS (21). A higher accuracy for classifying MI was observed in stroke participants following bi-hemispheric tDCS (67). Others have demonstrated a modulation of event-related desynchrony during MI with tDCS, which may enhance BCI accuracy and contribute to more effective training (35, 68, 69).
Anodal tDCS may also exert influence on training efficacy through enhancing implicit motor learning (24), or by improving attention (70). The greater delayed improvement demonstrated by the tDCS group may also reflect NMDA-dependent long-term changes in synaptic efficacy, an important mechanism underlying learning, and memory processes (23, 71).
In conclusion, MI-BCI resulted in significant UE improvement in chronic stroke patients with moderate to severe impairment. A trend toward better outcomes in the real-tDCS group was observed with significant benefit seen in the MEP- group. Future studies with more participants should focus on elucidating the specific neural mechanisms underlying motor recovery and the interaction of individual and stroke factors, tailoring neuromodulatory interventions using a stratified approach, and determining the optimal approach to combining MI-BCI with non-invasive brain stimulation to enhance motor recovery.
All datasets generated for this study are included in the article/supplementary material.
The studies involving human participants were reviewed and approved by National Healthcare Group Domain-Specific Review Board. The patients/participants provided their written informed consent to participate in this study.
EC, YN, and CG designed and directed the study. CG and KA developed the MI-BCI EEG analysis system. KP and LZ carried out the clinical trial procedures including MI-BCI training and trial coordination. EC, W-PT, and NT contributed to the data analysis and to the writing of the manuscript. All authors contributed to the article and approved the submitted version.
This study was supported by grants from the National Medical Research Council, Singapore (NIG09may022).
The authors declare that the research was conducted in the absence of any commercial or financial relationships that could be construed as a potential conflict of interest.
The authors thank Senior Occupattional Therapist Mr. Changwu Chen for administration of upper extremity motor function evaluation.
APB, abductor pollicus brevis; BCI, brain-computer interface; EEG, electroencephalogram; ISI, inter-stimulus interval; MEP, motor evoked potential; MI, motor imagery; MI-BCI, motor imagery-assisted brain-computer interface; POST1, within 1 week after the intervention; POST2, 4 weeks post-intervention; PRE, 1 week prior to commencement of the intervention; RMT, Resting motor threshold; SICF, intracortical facilitation; SICI, Short intra-cortical inhibition; tDCS, Transcranial direct current stimulation; TMS, transcranial magnetic stimulation; UE, upper extremity; UE-FM, upper extremity component of the Fugl-Meyer Assessment.
1. Nakayama H, Jorgensen HS, Raaschou HO, Olsen TS. The influence of age on stroke outcome. Copenhagen Stroke Study Stroke. (1994) 25:808–13. doi: 10.1161/01.STR.25.4.808
2. Kwakkel G, Kollen BJ, Van Der Grond J, Prevo AJ. Probability of regaining dexterity in the flaccid upper limb: impact of severity of paresis and time since onset in acute stroke. Stroke. (2003) 34:2181–6. doi: 10.1161/01.STR.0000087172.16305.CD
3. Lo AC, Guarino PD, Richards LG, Haselkorn JK, Wittenberg GF, Federman DG, et al. Robot-assisted therapy for long-term upper-limb impairment after stroke. N Engl J Med. (2010) 362:1772–83. doi: 10.1056/NEJMoa0911341
4. Facchini S, Muellbacher W, Battaglia F, Boroojerdi B, Hallett M. Focal enhancement of motor cortex excitability during motor imagery: a transcranial magnetic stimulation study. Acta Neurologica Scandinavica. (2002) 105:146–51. doi: 10.1034/j.1600-0404.2002.1o004.x
5. Lafleur MF, Jackson PL, Malouin F, Richards CL, Evans AC, Doyon J. Motor learning produces parallel dynamic functional changes during the execution and imagination of sequential foot movements. NeuroImage. (2002) 16:142–57. doi: 10.1006/nimg.2001.1048
6. Dunsky A, Dickstein R, Ariav C, Deutsch J, Marcovitz E. Motor imagery practice in gait rehabilitation of chronic post-stroke hemiparesis: four case studies. Int J Rehabil Res. (2006) 29:351–6. doi: 10.1097/MRR.0b013e328010f559
7. Dickstein R, Deutsch JE. Motor imagery in physical therapist practice. Phys Ther. (2007) 87:942–53. doi: 10.2522/ptj.20060331
8. Page SJ, Levine P, Leonard A. Mental practice in chronic stroke: results of a randomized, placebo-controlled trial. Stroke. (2007) 38:1293–7. doi: 10.1161/01.STR.0000260205.67348.2b
9. Dunsky A, Dickstein R, Marcovitz E, Levy S, Deutsch JE. Home-based motor imagery training for gait rehabilitation of people with chronic poststroke hemiparesis. Arch Phys Med Rehabil. (2008) 89:1580–8. doi: 10.1016/j.apmr.2007.12.039
10. Min BK, Marzelli MJ, Yoo SS. Neuroimaging-based approaches in the brain-computer interface. Trends Biotechnol. (2010) 28:552–60. doi: 10.1016/j.tibtech.2010.08.002
11. Daly JJ, Cheng R, Rogers J, Litinas K, Hrovat K, Dohring M. Feasibility of a new application of noninvasive Brain Computer Interface (BCI): a case study of training for recovery of volitional motor control after stroke. J Neurol Phys Ther. (2009) 33:203–11. doi: 10.1097/NPT.0b013e3181c1fc0b
12. La Fougere C, Zwergal A, Rominger A, Forster S, Fesl G, Dieterich M, et al. Real versus imagined locomotion: a [18F]-FDG PET-fMRI comparison. Neuroimage. (2010) 50:1589–98. doi: 10.1016/j.neuroimage.2009.12.060
13. Cicinelli P, Marconi B, Zaccagnini M, Pasqualetti P, Filippi MM, Rossini PM. Imagery-induced cortical excitability changes in stroke: a transcranial magnetic stimulation study. Cereb Cortex. (2006) 16:247–53. doi: 10.1093/cercor/bhi103
14. Bi S, Ji L, Wang Z. Robot-aided sensorimotor arm training methods based on neurological rehabilitation principles in stroke and brain injury patients. Conf Proc IEEE Eng Med Biol Soc. (2005) 5:5025–7. doi: 10.1109/IEMBS.2005.1615604
15. Coyle SM, Ward NS, Markham CM. Brain-computer interface using a simplified functional near-infrared spectroscopy system. J Neural Eng. (2007) 4:219–26. doi: 10.1088/1741-2560/4/3/007
16. Mellinger J, Schalk G, Braun C, Preissl H, Rosenstiel W, Birbaumer N, et al. An MEG-based brain-computer interface (BCI). Neuroimage. (2007) 36:581–93. doi: 10.1016/j.neuroimage.2007.03.019
17. Calabro RS, Accorinti M, Porcari B, Carioti L, Ciatto L, Billeri L, et al. Does hand robotic rehabilitation improve motor function by rebalancing interhemispheric connectivity after chronic stroke? Encouraging data from a randomised-clinical-trial. Clin Neurophysiol. (2019) 130:767–80. doi: 10.1016/j.clinph.2019.02.013
18. Carrillo-De-La-Peña MT, Galdo-Alvarez S, Lastra-Barreira C. Equivalent is not equal: primary motor cortex (MI) activation during motor imagery and execution of sequential movements. Brain Res. (2008) 21:134–43. doi: 10.1016/j.brainres.2008.05.089
19. Daly JJ, Wolpaw JR. Brain-computer interfaces in neurological rehabilitation. Lancet Neurol. (2008) 7:1032–43. doi: 10.1016/S1474-4422(08)70223-0
20. Ang KK, Guan C, Chua KS, Ang BT, Kuah C, Wang C, et al. A clinical study of motor imagery-based brain-computer interface for upper limb robotic rehabilitation. Conf Proc IEEE Eng Med Biol Soc. (2009) 2009:5981–4. doi: 10.1109/IEMBS.2009.5335381
21. Ang KK, Guan C, Chua KS, Ang BT, Kuah CW, Wang C, et al. A large clinical study on the ability of stroke patients to use an EEG-based motor imagery brain-computer interface. Clin EEG Neurosci. (2011) 42:253–8. doi: 10.1177/155005941104200411
22. Ramos-Murguialday A, Broetz D, Rea M, Laer L, Yilmaz O, Brasil FL, et al. Brain-machine interface in chronic stroke rehabilitation: a controlled study. Ann Neurol. (2013) 74:100–8. doi: 10.1002/ana.23879
23. Nitsche MA, Paulus W. Excitability changes induced in the human motor cortex by weak transcranial direct current stimulation. J Physiol. (2000) 527:633–9. doi: 10.1111/j.1469-7793.2000.t01-1-00633.x
24. Nitsche MA, Schauenburg A, Lang N, Liebetanz D, Exner C, Paulus W, et al. Facilitation of implicit motor learning by weak transcranial direct current stimulation of the primary motor cortex in the human. J Cogn Neurosci. (2003) 15:619–26. doi: 10.1162/089892903321662994
25. Paulus W. Transcranial direct current stimulation (tDCS). Suppl Clin Neurophysiol. (2003) 56:249–54. doi: 10.1016/S1567-424X(09)70229-6
26. Nowak DA, Grefkes C, Ameli M, Fink GR. Interhemispheric competition after stroke: brain stimulation to enhance recovery of function of the affected hand. Neurorehabil Neural Repair. (2009) 23:641–56. doi: 10.1177/1545968309336661
27. Fregni F, Boggio PS, Mansur CG, Wagner T, Ferreira MJ, Lima MC, et al. Transcranial direct current stimulation of the unaffected hemisphere in stroke patients. Neuroreport. (2005) 16:1551–5. doi: 10.1097/01.wnr.0000177010.44602.5e
28. Hummel F, Celnik P, Giraux P, Floel A, Wu WH, Gerloff C, et al. Effects of non-invasive cortical stimulation on skilled motor function in chronic stroke. Brain. (2005) 128:490–9. doi: 10.1093/brain/awh369
29. Gandiga PC, Hummel FC, Cohen LG. Transcranial DC stimulation (tDCS): a tool for double-blind sham-controlled clinical studies in brain stimulation. Clin Neurophysiol. (2006) 117:845–50. doi: 10.1016/j.clinph.2005.12.003
30. Boggio PS, Nunes A, Rigonatti SP, Nitsche MA, Pascual-Leone A, Fregni F. Repeated sessions of noninvasive brain DC stimulation is associated with motor function improvement in stroke patients. Restor Neurol Neurosci. (2007) 25:123–9.
31. Hesse S, Waldner A, Mehrholz J, Tomelleri C, Pohl M, Werner C. Combined transcranial direct current stimulation and robot-assisted arm training in subacute stroke patients: an exploratory, randomized multicenter trial. Neurorehabil Neural Repair. (2011) 25:838–46. doi: 10.1177/1545968311413906
32. Elsner B, Kugler J, Pohl M, Mehrholz J. Transcranial direct current stimulation (tDCS) for improving activities of daily living, and physical and cognitive functioning, in people after stroke. Cochrane Database Syst Rev. (2016) 3:CD009645. doi: 10.1002/14651858.CD009645.pub3
33. He W, Wei P, Zhou Y, Wang L. Modulation effect of transcranial direct current stimulation on phase synchronization in motor imagery brain-computer interface. Conf Proc IEEE Eng Med Biol Soc. (2014) 2014:1270–3.
34. Soekadar SR, Witkowski M, Cossio EG, Birbaumer N, Cohen LG. Learned EEG-based brain self-regulation of motor-related oscillations during application of transcranial electric brain stimulation: feasibility and limitations. Front Behav Neurosci. (2014) 8:93. doi: 10.3389/fnbeh.2014.00093
35. Kasashima Y, Fujiwara T, Matsushika Y, Tsuji T, Hase K, Ushiyama J, et al. Modulation of event-related desynchronization during motor imagery with transcranial direct current stimulation (tDCS) in patients with chronic hemiparetic stroke. Exp Brain Res. (2012) 221:263–8. doi: 10.1007/s00221-012-3166-9
36. Angulo-Sherman IN, Rodriguez-Ugarte M, Ianez E, Azorin JM. Low intensity focused tDCS over the motor cortex shows inefficacy to improve motor imagery performance. Front Neurosci. (2017) 11:391. doi: 10.3389/fnins.2017.00391
37. Ang KK, Guan C, Phua KS, Wang C, Zhao L, Teo WP, et al. Facilitating effects of transcranial direct current stimulation on motor imagery brain-computer interface with robotic feedback for stroke rehabilitation. Arch Phys Med Rehabil. (2015) 96:S79–87. doi: 10.1016/j.apmr.2014.08.008
38. Bastani A, Jaberzadeh S. Does anodal transcranial direct current stimulation enhance excitability of the motor cortex and motor function in healthy individuals and subjects with stroke: a systematic review and meta-analysis. Clin Neurophysiol. (2012) 123:644–57. doi: 10.1016/j.clinph.2011.08.029
39. Fugl-Meyer AR, Jaasko L, Leyman I, Olsson S, Steglind S. The post-stroke hemiplegic patient. 1. a method for evaluation of physical performance. Scand J Rehabil Med. (1975) 7:13–31.
40. Arya KN, Verma R, Garg RK. Estimating the minimal clinically important difference of an upper extremity recovery measure in subacute stroke patients. Top Stroke Rehabil. (2011) 18(Suppl.1):599–610. doi: 10.1310/tsr18s01-599
41. Kranczioch C, Mathews S, Dean PJ, Sterr A. On the equivalence of executed and imagined movements: evidence from lateralized motor and nonmotor potentials. Hum Brain Mapp. (2009) 30:3275–86. doi: 10.1002/hbm.20748
42. Pfurtscheller G, Neuper C. Event-related synchronization of mu rhythm in the EEG over the cortical hand area in man. Neurosci Lett. (1994) 174:93–6. doi: 10.1016/0304-3940(94)90127-9
43. Rossini PM, Barker AT, Berardelli A, Caramia MD, Caruso G, Cracco RQ, et al. Non-invasive electrical and magnetic stimulation of the brain, spinal cord and roots: basic principles and procedures for routine clinical application. Report of an IFCN committee. Electroencephalogr Clin Neurophysiol. (1994) 91:79–92. doi: 10.1016/0013-4694(94)90029-9
44. Kujirai T, Caramia MD, Rothwell JC, Day BL, Thompson PD, Ferbert A, et al. Corticocortical inhibition in human motor cortex. J Physiol. (1993) 471:501–19. doi: 10.1113/jphysiol.1993.sp019912
45. Ziemann U, Rothwell JC, Ridding MC. Interaction between intracortical inhibition and facilitation in human motor cortex. J Physiol. (1996) 496:873–81. doi: 10.1113/jphysiol.1996.sp021734
46. Stinear CM, Barber PA, Smale PR, Coxon JP, Fleming MK, Byblow WD. Functional potential in chronic stroke patients depends on corticospinal tract integrity. Brain. (2007) 130:170–80. doi: 10.1093/brain/awl333
47. Brown JA, Lutsep HL, Weinand M, Cramer SC. Motor cortex stimulation for the enhancement of recovery from stroke: a prospective, multicenter safety study. Neurosurgery. (2006) 58:464–73. doi: 10.1227/01.NEU.0000197100.63931.04
48. Lefaucheur JP, Aleman A, Baeken C, Benninger DH, Brunelin J, Di Lazzaro V, et al. Evidence-based guidelines on the therapeutic use of repetitive transcranial magnetic stimulation (rTMS): an update (2014–2018). Clin Neurophysiol. (2020) 131:474–528. doi: 10.1016/j.clinph.2019.11.002
49. Byblow WD, Stinear CM, Barber PA, Petoe MA, Ackerley SJ. Proportional recovery after stroke depends on corticomotor integrity. Ann Neurol. (2015) 78:848–59. doi: 10.1002/ana.24472
50. Winters C, Van Wegen EE, Daffertshofer A, Kwakkel G. Generalizability of the proportional recovery model for the upper extremity after an ischemic stroke. Neurorehabil Neural Repair. (2015) 29:614–22. doi: 10.1177/1545968314562115
51. Stinear CM, Byblow WD, Ackerley SJ, Smith MC, Borges VM, Barber PA. Proportional motor recovery after stroke: implications for trial design. Stroke. (2017) 48:795–8. doi: 10.1161/STROKEAHA.116.016020
52. Di Pino G, Pellegrino G, Assenza G, Capone F, Ferreri F, Formica D, et al. Modulation of brain plasticity in stroke: a novel model for neurorehabilitation. Nat Rev Neurol. (2014) 10:597–608. doi: 10.1038/nrneurol.2014.162
53. Sankarasubramanian V, Machado AG, Conforto AB, Potter-Baker KA, Cunningham DA, Varnerin NM, et al. Inhibition versus facilitation of contralesional motor cortices in stroke: deriving a model to tailor brain stimulation. Clin Neurophysiol. (2017) 128:892–902. doi: 10.1016/j.clinph.2017.03.030
54. Shimizu T, Hosaki A, Hino T, Sato M, Komori T, Hirai S, et al. Motor cortical disinhibition in the unaffected hemisphere after unilateral cortical stroke. Brain. (2002) 125:1896–907. doi: 10.1093/brain/awf183
55. Butefisch CM, Netz J, Wessling M, Seitz RJ, Homberg V. Remote changes in cortical excitability after stroke. Brain. (2003) 126:470–81. doi: 10.1093/brain/awg044
56. Butefisch CM, Wessling M, Netz J, Seitz RJ, Homberg V. Relationship between interhemispheric inhibition and motor cortex excitability in subacute stroke patients. Neurorehabil Neural Repair. (2008) 22:4–21. doi: 10.1177/1545968307301769
57. Buetefisch CM. Role of the contralesional hemisphere in post-stroke recovery of upper extremity motor function. Front Neurol. (2015) 6:214. doi: 10.3389/fneur.2015.00214
58. Biernaskie J, Szymanska A, Windle V, Corbett D. Bi-hemispheric contribution to functional motor recovery of the affected forelimb following focal ischemic brain injury in rats. Eur J Neurosci. (2005) 21:989–99. doi: 10.1111/j.1460-9568.2005.03899.x
59. Harrington RM, Chan E, Rounds AK, Wutzke CJ, Dromerick AW, Turkeltaub PE, et al. Roles of lesioned and nonlesioned hemispheres in reaching performance poststroke. Neurorehabil Neural Repair. (2020) 34:61–71. doi: 10.1177/1545968319876253
60. Ward NS, Brown MM, Thompson AJ, Frackowiak RS. Neural correlates of outcome after stroke: a cross-sectional fMRI study. Brain. (2003) 126:1430–48. doi: 10.1093/brain/awg145
61. Luft AR, Mccombe-Waller S, Whitall J, Forrester LW, Macko R, Sorkin JD, et al. Repetitive bilateral arm training and motor cortex activation in chronic stroke: a randomized controlled trial. JAMA. (2004) 292:1853–61. doi: 10.1001/jama.292.15.1853
62. Han KJ, Kim JY. The effects of bilateral movement training on upper limb function in chronic stroke patients. J Phys Ther Sci. (2016) 28:2299–302. doi: 10.1589/jpts.28.2299
63. Schaechter JD, Perdue KL. Enhanced cortical activation in the contralesional hemisphere of chronic stroke patients in response to motor skill challenge. Cereb Cortex. (2008) 18:638–47. doi: 10.1093/cercor/bhm096
64. Xu J, Branscheidt M, Schambra H, Steiner L, Widmer M, Diedrichsen J, et al. Rethinking interhemispheric imbalance as a target for stroke neurorehabilitation. Ann Neurol. (2019) 85:502–13. doi: 10.1002/ana.25452
65. Ziemann U, Lonnecker S, Steinhoff BJ, Paulus W. The effect of lorazepam on the motor cortical excitability in man. Exp Brain Res. (1996) 109:127–35. doi: 10.1007/BF00228633
66. Huynh W, Vucic S, Krishnan AV, Lin CS-Y, Hornberger M, Kiernan MC. Longitudinal plasticity across the neural axis in acute stroke. Neurorehabilitation Neural Repair. (2013) 27:219–29. doi: 10.1177/1545968312462071
67. Ang KK, Guan C, Phua KS, Wang C, Teh I, Chen CW, et al. Transcranial direct current stimulation and EEG-based motor imagery BCI for upper limb stroke rehabilitation. Conf Proc IEEE Eng Med Biol Soc. (2012) 2012:4128–31. doi: 10.1109/EMBC.2012.6346875
68. Matsumoto J, Fujiwara T, Takahashi O, Liu M, Kimura A, Ushiba J. Modulation of mu rhythm desynchronization during motor imagery by transcranial direct current stimulation. J Neuroeng Rehabil. (2010) 7:27. doi: 10.1186/1743-0003-7-27
69. Tohyama T, Fujiwara T, Matsumoto J, Honaga K, Ushiba J, Tsuji T, et al. Modulation of event-related desynchronization during motor imagery with transcranial direct current stimulation in a patient with severe hemiparetic stroke: a case report. Keio J Med. (2011) 60:114–8. doi: 10.2302/kjm.60.114
70. Kang EK, Baek MJ, Kim S, Paik NJ. Non-invasive cortical stimulation improves post-stroke attention decline. Restor Neurol Neurosci. (2009) 27:645–50. doi: 10.3233/RNN-2009-0514
Keywords: stroke, motor recovery, transcranial direct current stimulation, brain-computer interface, motor imagery
Citation: Chew E, Teo W-P, Tang N, Ang KK, Ng YS, Zhou JH, Teh I, Phua KS, Zhao L and Guan C (2020) Using Transcranial Direct Current Stimulation to Augment the Effect of Motor Imagery-Assisted Brain-Computer Interface Training in Chronic Stroke Patients—Cortical Reorganization Considerations. Front. Neurol. 11:948. doi: 10.3389/fneur.2020.00948
Received: 23 March 2020; Accepted: 22 July 2020;
Published: 27 August 2020.
Edited by:
Alessandro Oronzo Caffò, University of Bari Aldo Moro, ItalyReviewed by:
Antonino Naro, Centro Neurolesi Bonino Pulejo (IRCCS), ItalyCopyright © 2020 Chew, Teo, Tang, Ang, Ng, Zhou, Teh, Phua, Zhao and Guan. This is an open-access article distributed under the terms of the Creative Commons Attribution License (CC BY). The use, distribution or reproduction in other forums is permitted, provided the original author(s) and the copyright owner(s) are credited and that the original publication in this journal is cited, in accordance with accepted academic practice. No use, distribution or reproduction is permitted which does not comply with these terms.
*Correspondence: Effie Chew, ZWZmaWVfY2hld0BudWhzLmVkdS5zZw==
Disclaimer: All claims expressed in this article are solely those of the authors and do not necessarily represent those of their affiliated organizations, or those of the publisher, the editors and the reviewers. Any product that may be evaluated in this article or claim that may be made by its manufacturer is not guaranteed or endorsed by the publisher.
Research integrity at Frontiers
Learn more about the work of our research integrity team to safeguard the quality of each article we publish.