- 1Department of Neurology and Neuroscience Center, The First Hospital of Jilin University, Changchun, China
- 2Department of Plastic and Reconstructive Surgery, The First Hospital of Jilin University, Changchun, China
- 3Department of Cardiology, The First Hospital of Jilin University, Changchun, China
Ischemic stroke is a multi-factorial cerebrovascular disease with high worldwide morbidity and mortality. In the past few years, multiple studies have revealed the underlying mechanism of ischemia/reperfusion injury, including calcium overload, amino acid toxicity, oxidative stress, and inflammation. Connexin 43 (Cx43), the predominant connexin protein in astrocytes, has been recently proven to display non-substitutable roles in the pathology of ischemic stroke development and progression through forming gap junctions and hemichannels. Under normal conditions, astrocytic Cx43 could be found in hemichannels or in the coupling with other hemichannels on astrocytes, neurons, or oligodendrocytes to form the neuro–glial syncytium, which is involved in metabolites exchange between communicated cells, thus maintaining the homeostasis of the CNS environment. In ischemic stroke, the phosphorylation of Cx43 might cause the degradation of gap junctions and the opening of hemichannels, contributing to the release of inflammatory mediators. However, the remaining gap junctions could facilitate the exchange of protective and harmful metabolites between healthy and injured cells, protecting the injured cells to some extent or damaging the healthy cells depending on the balance of the exchange of protective and harmful metabolites. In this study, we review the changes in astrocytic Cx43 expression and distribution as well as the influence of these changes on the function of astrocytes and other cells in the CNS, providing new insight into the pathology of ischemic stroke injury; we also discuss the potential of astrocytic Cx43 as a target for the treatment of ischemic stroke.
Introduction
Ischemic stroke is caused by the stenosis or occlusion of the cerebral blood supply. It is the most common cerebral vascular disease (contributing to ~80% of strokes) with high morbidity and mortality (1, 2). It was recently reported that ischemic stroke, cardiovascular diseases, and malignant tumors constitute the three major causes of human death (3, 4). Although research into the mechanisms of ischemic stroke injury has made advanced progress in the last few years, effective strategies for ischemic stroke treatment to protect residual neurons by restoring brain perfusion as soon as possible via intravenous thrombolysis and mechanical thrombectomy (5, 6) remain limited.
Among the glial cells in the brain parenchyma, astrocytes are the most abundant and critical (7, 8) and may modulate the homeostasis of the central nervous system (CNS) environment and support the survival of neurons (8, 9). The roles of astrocytes in the pathology of ischemic stroke are double-edged, while they can help maintain the homeostasis of the CNS micro-environment to protect neurons by maintaining ion and pH balance, transporting metabolic substrates, and clearing neuronal waste. Conversely, the inflammatory mediators and excitatory amino acids produced and released by astrocytes might promote the death of neurons (10). Connexin 43 (Cx43), one of the most abundant Cxs in the brain tissue, is essential for astrocytes to exert their various physiological functions by forming gap junctions and hemichannels (11, 12) and its role in the development and progression of ischemic stroke have received increasing attention in recent years (13, 14). However, the currently available data show that the change in astrocytic Cx43 after ischemic stroke and the roles it plays are controversial (15–17). Therefore, in this study, we reviewed the syncytium structures that astrocytes form with other cells (astrocytes, neurons, and oligodendrocytes), and the change in astrocytic Cx43 expression and distribution after stroke as well as how these changes influence the functions of astrocytes and the neuro–glial syncytium, subsequently regulating ischemic injury. The delineation of the roles of astrocytic Cx43 in ischemic stroke could help elucidate the initiation and spread of inflammation and neuronal damage after ischemic stroke, which might provide some new targets for the treatment of ischemic stroke.
The Structure, Distribution, and Physiological Functions of Cx43
Cxs in the CNS are important membrane proteins of a family that consist of 21 members that can form gap junctions and hemichannels. Eleven of these Cxs are expressed in the adult mammalian brain and are distributed differently on glial cells and neurons in the CNS (Table 1) (31–33). Among these Cxs, astrocytic Cx43 is the most widely expressed and studied in the CNS, playing essential roles in the communication between astrocytes and other cells or with the extracellular milieu, as they form gap junction channels or hemichannels (34).
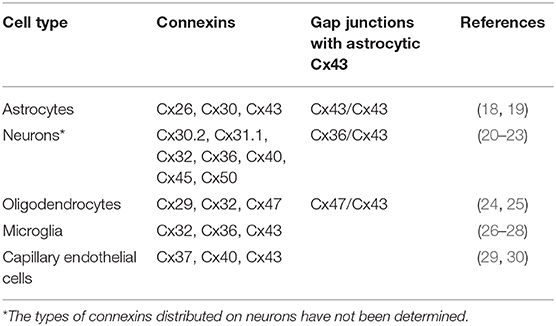
Table 1. Cellular distribution of connexins expressed by glia and neurons in the adult mammalian central nervous system.
Structure and Distribution of Cx43
Cx43 in the adult mammalian brain, named after its molecular weight of ~43 kDa, belongs to the α-Cx family and consists of 382 amino acids (31, 35, 36). The same as other Cxs, Cx43 contains four transmembrane regions, the intracellular N-terminal and C-terminal and two extracellular loops. The two extracellular loops and the N-terminal are relatively conserved, while the intracellular loop and C-terminus determine the different biological characteristics in different species (37, 38).
Cx43 is the dominant Cx protein in astrocytes and the main component of astrocytic gap junctions and hemichannels (1). Individual Cx assembles into hexamers around a central pore to form transmembrane channels named connexons, also known as hemichannels (39). They can exist as free, no-junctional channels on the astrocytic membrane and form gap junction channels with other hemichannels on the membrane of adjacent astrocytes or other cells (18). Further studies have shown that hemichannels may be homomeric or heteromeric, depending on the Cx composition. Similarly, gap junctions are homotypic if the paired hemichannels contain the same Cxs, and heterotypic if they contain different Cxs (40).
Hemichannels
Hemichannels are not closed under resting conditions; their opening probability is very low but not zero (41). However, under certain physiological and pathological stimulation conditions (such as the presence pro-inflammatory cytokines, increase in intracellular calcium concentration, and metabolic inhibition), hemichannels might increase their opening probability (42–47). Consequently, activated astrocytic Cx43 hemichannels are critical ion diffusion (including of Ca2+, K+, Na+) from astrocytes to the extracellular milieu as well as for the release of adenosine triphosphate (ATP) and gliotransmitters [including of glutamate (Glu), adenosine, and glutathione], thus forming chemical coupling between cells and the micro-environment through autocrine and paracrine approaches (48–50). The activated hemichannels might play dual roles in the CNS. Recent reports have shown that the opening of hemichannels during resting conditions is involved in basal synaptic transmission and long-term potentiation (51, 52). Other studies have reported that the opening of hemichannels can facilitate the release of D-serine, further enhancing excitatory synaptic transmission in the hippocampus or olfactory bulb (53). However, in pathological situations, the changes in hemichannels may activate inflammatory signaling, damaging the survival of glial cells and producing excitotoxic molecules (54). After ischemic stroke, inflammation followed by ischemia/reperfusion (I/R) injury could activate the astrocytic hemichannels by increasing extracellular Ca2+ and inflammatory cytokine release (15). Then, the opened hemichannels could promote the differentiation of microglia to the M1 phenotype, which could produce pro-inflammatory cytokines including tumor necrosis factor-α (TNF-α) and interleukin-1β (IL-1β), consequently triggering the opening of astrocytic hemichannels (39, 55). The vicious cycle caused the uncontrolled release of ATP, Glu, and Ca2+ overload could induce increase in the number of abnormally opened hemichannels, which in turn could lead to tissue excitotoxicity, inflammation amplification, and finally irreversible brain damage (56).
Gap Junction
Astrocytic gap junctions are axially aligned hexamers of connexins, which can connect adjacent cells (57). Thousands of gap junctions clustered into discoid gap junction plaques could further combine with specific protein binding subunits of paired connexons on the membrane of adjacent cells to form the supramolecular gap junction nexus (58). Gap junctions are the major structures of electrical transmission and metabolic and ionic coupling between adjacent cells (41). In contrast to hemichannels, gap junctions are always open under physiological conditions, allowing intercellular communication (59). They allow small molecules, below 1,200 Daltons, to diffuse, including ATP, inositol trisphosphate, and ions (such as K+, Na+, and Ca2+), cyclic nucleotides, and oligonucleotides and small peptides, facilitating metabolites exchange and information communication among astrocytes, neurons, and oligodendrocytes, maintaining the intracellular and extracellular homeostasis (60–62). More importantly, astrocytic gap junction channels also transmit chemical signals and metabolites (glucose and lactate) between glial cells, facilitating the function of neuronal, glial, and vascular tissues (63).
The Neuron–Glial Syncytium Structure in the Central Nervous System
As mentioned above, astrocytic Cx43 can both form hemichannels on the cell membrane of astrocytes and form gap junctions with Cx43 or other Cxs on the adjacent astrocytes (18). Considering the hemichannels and gap junctions, astrocytes play a central role in the formation of neuro–glial syncytium structures, where neurons, microglia, oligodendrocytes, and even capillaries could combine to perform various physiological functions (Figure 1) (64).
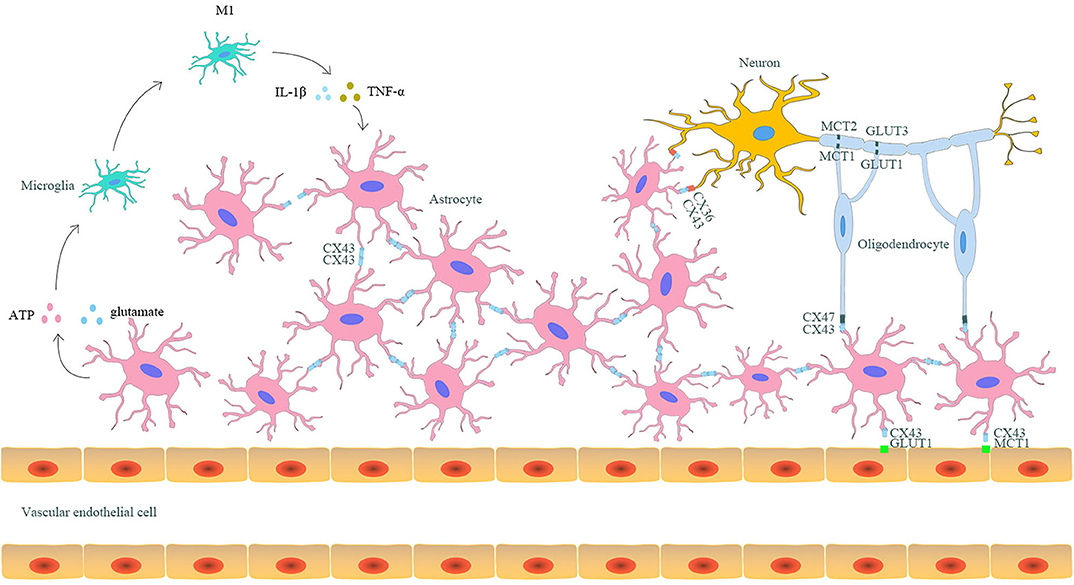
Figure 1. The interaction between astrocytic Cx43 and other parenchymal cells in the CNS. Astrocytes and other parenchyma cells in the CNS form neuro–glial syncytium structures via Cx43/Cx43 gap junctions between astrocytes, Cx43/Cx36 gap junctions between astrocytes and neurons, and Cx43/Cx47 gap junctions between astrocytes and oligodendrocytes. Such structures allow the exchange of metabolites and rapid signal communication, resulting in synergistic response to stimuli. Astrocytic Cx43 is also involved in the capillary-astrocyte-oligodendrocyte-neuron axis and participates in the lactate and glucose transport between capillaries and neurons. Moreover, astrocytes can also indirectly promote the differentiation of microglia to the M1 phenotype through releasing pro-inflammatory mediators via hemichannels. In turn, the differentiated M1 microglia could aggravate the destruction of gap junctions, enhancing injury after ischemic stroke.
First, astrocytes can be coupled by Cx43 gap junctions to form syncytium structures, which allows groups of cells to synchronously respond to stimuli (61). The coupled astrocytes can differentiate together during the developmental process (65). They also participate in various physiological processes, including clearing K+ from the extracellular space, synthesizing neurotransmitters, propagating calcium waves, balancing Glu and γ-aminobutyric acid, and the immune response (59, 66, 67). However, in ischemic stroke, gap junctions might also act as channels for the transmission of cytotoxic molecules (including of Ca2+, excessive ATP, and Glu) from dying astrocytes to their coupled cells, amplifying ischemia-induced brain injury (68).
Apart from astrocyte–astrocyte coupling, astrocytes can also couple with neurons and oligodendrocytes through Cx43 (11, 69). Various studies have confirmed that neurons mainly express Cx36, Cx45, and Cx32, all of which can serve as hemichannels (20, 21). Among these Cxs, Cx36 on neurons can couple with astrocytic Cx43, thus forming Cx43/Cx36 heterotypic gap junctions, which facilitate direct metabolic and electrical communication between astrocytes and neurons (20, 70). Additionally, the existence of Cx43/Cx36 gap junctions can combine neurons into the syncytium network. Under physiological conditions, astrocytes can provide energetic substrates (glucose, lactate, citrate, and glutamine) for neurons. Furthermore, Cx32, Cx47, and Cx29 are primarily expressed on oligodendrocytes (24, 25). Among these Cxs on oligodendrocytes, Cx47 participates in the formation of gap junctions with astrocytic Cx43 (19). Recently, studies have revealed that astrocyte–oligodendrocyte gap junctions are essential for CNS myelination and homeostasis (71). Some studies have found that astrocytes can deliver glucose and lactate to oligodendrocytes through gap junctions, which is essential for the survival of neuronal axons (72, 73). Additionally, heterotypic Cx43/Cx47 gap junctions have been shown to mediate the spatial buffering of K+ and the bi-directional transmission of Ca2+ between astrocytes and oligodendrocytes (74). The loss of Cx43/Cx47 gap junctions might disrupt the spatial buffering of K+, subsequently leading to myelin swelling and axonal degeneration (75, 76).
Although Cxs expressed on capillary endothelial cells (mainly Cx 37, Cx40, and Cx43) can also form hemichannels and gap junctions (29, 30), there is no evidence that astrocytes establish direct contact with endothelial cells, which might account for the obstruction of the basal lamina between these two cell types (77). Astrocytes can attach to capillaries through their end-feet (78, 79). Under normal conditions, capillary endothelial cells can take up blood-borne glucose and lactate by glucose transporter 1 and monocarboxylate transporter (MCT) 1, respectively, which then diffuse through gap junction channels between adjacent endothelial cells. Both glucose and lactate are eventually taken up by the astrocytic end-feet via MCT4 and Cx43 hemichannels, respectively, or released to the extracellular space (80, 81). Thus, glucose and lactate can be transported through astrocytes and their gap junctions with neighboring astrocytes to reach relatively distant areas (82). Furthermore, astrocytes can transfer lactate and glucose to oligodendrocytes through the heterotypic Cx43/Cx47 gap junction channels between them (80). Finally, the lactate in oligodendrocytes can be transported to neuronal axons, inducing axonal degeneration, while the glucose can supply energy for neurons (72, 73).
Taken together, astrocytic Cx43 places astrocytes to a central position in neuron–glial syncytium structures to enroll neurons, oligodendrocytes, microglia, and even capillaries in the CNS into this network, facilitating these cells and structures to respond to changes in the CNS micro-environment, thus maintaining the stability of the CNS milieu and regulating the development, differentiation, and function of neurons.
Change in Astrocytic Cx43 in Ischemic Stroke
After ischemic stroke, various types of cells in the CNS, including neurons, glial cells, and vascular endothelial cells, sustain different degrees of damage. Astrocytes could be activated and proliferate after ischemic stroke, which is known as reactive astrogliosis (81, 83). Reactive astrogliosis is a type of multistage and pathology-specific reaction, which represents a series of alterations that occur in astrocytes in response to any insult to the CNS (84). At the earlier stage of ischemic stroke, reactive astrocytes can seal the damaged area (85), maintain the balance of the micro-environment, provide nutrients for the neurons, reduce the excitatory toxicity of amino acids, and activate local immune reactions (86). These processes can promote the remodeling of surrounding structural tissues, avoiding secondary damage to neurons (87). However, at the later stage of ischemic stroke, excessive proliferation of reactive astrocytes can change the axon regeneration micro-environment to restrain axonal growth (88), form glial scars, and inhibit the information communication of neurons (89), which can suppress the recovery of nerve function.
Cx43 on astrocytes is an important mediator of CNS ischemic injury, and change in Cx43 expression and distribution has been associated with the outcome of ischemic injury (59). The change in Cx43 expression in the CNS after ischemic stroke remains controversial and depends on ischemia severity, regions, and phase. Cx43 immunoreactivity (Cx43-ir) in the hippocampus and striatum could increase under mild to moderate ischemic conditions (90). However, further studies have indicated that there is an area of reduced Cx43-ir surrounded by a zone of increased Cx43-ir following severe ischemia (66). Another study found that there was a transient downregulation of Cx43 mRNA on day 1 and then upregulation on day 7 after ischemic stroke (91). However, some studies have revealed that there is no significant change in the total amount of Cx43 in in vitro and in vivo hypoxia models of astrocytes (92). Apart from change in expression, change in Cx43 distribution has also been reported in ischemic stroke. A recent study showed that Cx43 decreased on the astrocytic plasma membrane, whereas it increased in the cytoplasm after ischemic stroke (93). In addition, the reorganization Cx43 gap junctions was also confirmed by immuno-electron microscopy (94).
The C-terminal of astrocytic Cx43 has critical roles in regulating astrocytic functions. Various studies have indicated that the phosphorylation status of the astrocytic Cx43 C-terminal is an important mediator modulating the gap junction channels and hemichannels after ischemic stroke, thus influencing the functions of astrocytes and neurons (Figure 2) (95). It has been reported that the C-terminal of astrocytic Cx43 could be phosphorylated after ischemic stroke via several protein kinases including protein kinase C (96), mitogen-activated protein kinase (MAPK) (97), pp60Src kinase (98), and casein kinase 1δ (99), inducing Cx43 internalization, further contributing to the uncoupling process of astrocytes (100, 101). Interestingly, other studies have found that in vitro hypoxia may lead to the dephosphorylation of the C-terminal of astrocytic Cx43, accompanied by the uncoupling of astrocytes (101, 102). This controversy arises because the phosphorylation and dephosphorylation of Cx43 as well as astrocytic uncoupling all occur within a short period after ischemia. Further studies have revealed that astrocytic coupling was significantly reduced by 77% after 15 min of hypoxia, 92% after 30 min of hypoxia, and 97% after 1 h of hypoxia, while subsequent substantial Cx43 dephosphorylation was observed at 30 min or 60 min after hypoxia. In addition, a greater quantity of dephosphorylated Cx43 was observed at 60 min than at 30 min. Moreover, dephosphorylated Cx43 became predominant after 60 min. Subsequent studies have also found that phosphorylated Cx43 remained preponderant from 1 min until 30 min after hypoxia, and the level of preserved astrocytic coupling was 34% after 30 min of hypoxia with addition of phosphatase inhibitors to hypoxic astrocytes (101, 103). These studies indicated that the gap junction uncoupling process of astrocytes lies between the phosphorylation and dephosphorylation of Cx43 and might be the result of the phosphorylation of Cx43 and the cause of Cx43 dephosphorylation on hemichannels. However, the precise phosphorylation and dephosphorylation sites of the astrocytic Cx43 C-terminal remain undetermined. Márquez-Rosado et al. revealed that phosphorylation and dephosphorylation occurred at the serine 325/328/330/365/368 (104) site, while Freitas-Andrade et al. demonstrated that phosphorylation and dephosphorylation of serine 255/262/279/282 (105) also occurred. It is unknown whether the phosphorylation and dephosphorylation of astrocytic Cx43 after stroke occurred at the same site and whether the phosphorylation and dephosphorylation of different Cx43 sites might trigger different degrees of Cx43 degradation. Additionally, Cx43 cysteine residues of S-Nitrosylation have been observed in in vitro ischemic models induced by nitric oxide (NO) (106). Other in vitro studies have found that astrocytic Cx43 was S-Nitrosylated in cultured astrocytes treated with NO for 50 min and that S-Nitrosylated Cx43 could increase the numbers and opening probability of hemichannels (107).
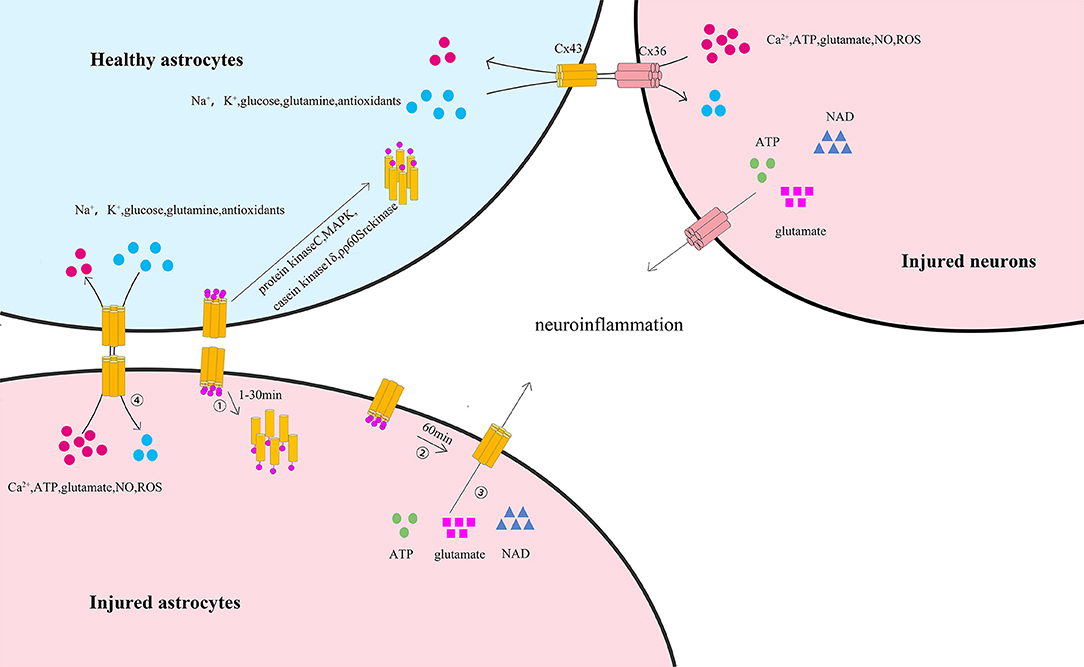
Figure 2. The phosphorylation of astrocytic Cx43 C-terminal and its influence on astrocytes and neurons after ischemic stroke. ① At the early stage of ischemic stroke, astrocytic membrane Cx43, which is involved in the formation of gap junction channels and hemichannels, is phosphorylated at the C-terminal (predominantly from 1 to 30 min) via several protein kinases including protein kinase C, MAPK, pp60Src kinase, and casein kinase 1δ. This process leads to the internalization of membrane Cx43 to the cytoplasm and the degradation of gap junctions. ② At the later stage of ischemic stroke, the remaining astrocytic membrane Cx43 is dephosphorylated (predominantly after 60 min), increasing the permeability of hemichannels. ③ Thus, resulting in the release of ATP, glutamate, and nicotinamide adenine dinucleotide from the cytoplasm of injured astrocytes or injured neurons to the extracellular space, initiating or enhancing neuroinflammation through the remaining gap junctions. ④ In coupled astrocytes, healthy astrocytes could transfer essential ions and metabolites (including Na+, K+, glutamine, antioxidants, and glucose) to injured astrocytes by Cx43/Cx43 gap junction channels or injured neurons by Cx43/Cx36 gap junction channels and protect the injured astrocytes. In turn, harmful ions and metabolites (including Ca2+, ATP, glutamate, NO, and ROS) might also be transferred from injured astrocytes or injured neurons to healthy astrocytes, thus spreading the death wave.
Meanwhile, in vitro studies have confirmed that astrocytic Cx43 C-terminal dephosphorylation may increase the opening of hemichannels (108, 109). Previous studies have used astrocytes cultured in in vitro ischemia to study the activity of astrocytic Cx43 gap junctions and hemichannels. Among such studies, it has been found that Cx43 gap junctions between dying astrocytes remained functional under ischemic conditions, although Cx43 gap junction coupling decreased (101, 110), signifying that intercellular communication can still occur via astrocytic Cx43 gap junctions under ischemic conditions. Although the opening hemichannels may disrupt the electrochemical and metabolic gradients across the plasma membrane, studies have proven that the remaining gap junctions can protect dying astrocytes to some extent by transferring ions and essential metabolites from healthy astrocytes to dying ones with open hemichannels (107). In contrast, dying astrocytes can also transfer harmful ions and metabolites to neighboring healthy ones with open hemichannels via the remaining gap junctions, which can again cause the death of their neighbors (111). This wave-like spread of the death process is much similar to the extension of infarct regions under ischemic conditions (108, 112, 113).
Effects of Astrocytic Cx43 in Ischemic Stroke
Functions of Astrocyte–Astrocyte Coupling
Coupled astrocytes in the astrocytic network share the same fate in ischemic stroke, while the uncoupled ones do not (61, 114). Gap junction communication could positively regulate astrocytic activation and proliferation (115, 116). In permanent right middle cerebral artery occlusion (MCAO) models of Cx43+/− and Cx43+/+ mice, Cx43+/− mice showed a significantly larger infarct size but in a smaller area of astrogliosis than did Cx43+/+ mice (63), indicating that astrocytic Cx43 gap junctions indeed display a vital role in astrocyte activation and cytotoxic-molecule removal, thus facilitating neuronal survival. Moreover, the astrocytic network formed by gap junctions could also provide energy substrates (glucose and lactate) to neurons (63). Besides, studies with oxygen glucose deprivation (OGD) and MCAO models have found that astrocytic Cx43 is important for astrocytic integrity and stability by activating either astrocytic Cx43 gap junctions or hemichannels (117, 118). Astrocytic Cx43 gap junctions can protect astrocytes by permitting the exit of toxic molecules [including Ca2+, excessive ATP, Glu, NO, and radical oxygen species (ROS)] out of the injured astrocyte and the entrance of neuroprotective metabolites (including Na+, K+, glutamine, antioxidants, glucose) into the astrocytes under ischemic conditions (119, 120). However, if too many toxic molecules are transferred into healthy astrocytes, beyond their bearing load, astrocytic injury might spread to the adjacent astrocytes. Metabolites released by Cx43 hemichannels can also act on the neighboring astrocytes (121). In ischemic stroke, the internalization of astrocytic Cx43 induced by the phosphorylation of the C-terminal might contribute to the uncoupling of astrocytes, thus reducing the mutual support of astrocytes and aggravating their injury (99). In addition, the opening of hemichannels induced by the dephosphorylation of astrocytic Cx43 could promote the release of inflammatory mediators, increasing neuroinflammation after ischemic stroke (108, 109).
Functions of Astrocyte–Neuronal Coupling
Recent studies have found that astrocytes could protect neurons by producing glutathione to exert anti-oxidant effects, reducing inflammatory media, enlarging the gap junctions, regulating energy metabolism, inhibiting apoptosis, up-taking excitatory amino acids, inducing cerebral ischemic tolerance in response to ischemic preconditioning, and other processes (122, 123). Accumulating evidence indicates that the amount of Cx43/Cx36 gap junctions decreases due to Cx43 internalization after ischemic stroke, which reduces the transportation of neurotransmitters and metabolites between astrocytes and neurons (100). However, aerobic metabolism and ATP production declined while toxic ions and molecules, such as Ca2+, glutamate, ROS, and NO, accumulated in damaged neurons after ischemic stroke (124). These toxic metabolites may be released by hemichannels and transmitted from damaged neurons to healthy astrocytes via Cx43/Cx36 gap junctions, thus reducing the load of neurons and activating astrocytes (125). The activated astrocytes could release pro-inflammatory cytokines and chemokines (80), which could in turn lead to changes in the neuronal functions that affect behavior, mood, and cognitive abilities (126). Furthermore, pro-inflammatory cytokines decreased the gap junctions between astrocytes and increased the opening of neuronal Cx36 and astrocytic Cx43 hemichannels, finally causing an increase in the release of ATP, Glu, prostaglandins, and NO (127–129). These molecules are toxic to adjacent cells, which may amplify the inflammation and cause secondary damage to distant cells, leading to tissue excitotoxicity and irreversible brain damage (56, 130).
Functions of Astrocyte–Oligodendrocyte Coupling
Studies have reported that the existence of astrocytic Cx43 is necessary for the functions of oligodendrocytes (71, 131). The loss of astrocytic Cx43, which forms hemichannels and gap junction channels on the astrocytic membrane, could disrupt the Cx43/Cx47 gap junctions, which are harmful for the transmission of ions and nutrients between astrocytes and oligodendrocytes (132). Studies have found that Cx43 displays multiple metabolic and signaling roles in astrocytes, which can affect oligodendrocytes independently of gap junctions (133). Studies with astrocytic Cx43-deficient mice have revealed that Cx47 was not stabilized and its amount was strongly decreased because of internalization and degradation (131), which lead to the diffusion of Cx47 away from the oligodendrocytic cell membrane, aggravating the post-ischemic inflammatory response and myelin loss of oligodendrocytes (134, 135).
Interaction of Astrocytes and Microglia
Although microglia express Cx36, Cx32, and Cx43 (26, 136), gap junctions between microglia and astrocytes have not been observed. In addition, extracellular ATP released from astrocytes accounting for the opening of hemichannels after ischemic stroke could activate the purine ionotropic receptors on microglia (including P2X4R and P2Y12R), thereby promoting the differentiation of microglia to the M1 subtype (56, 137). Activated microglia could secret TNF-α and IL-1β, which could further aggravate the inflammation (138). Activated microglia could inhibit gap junction communication and downregulate Cx43 expression in astrocytes through the release of TNF-α and IL-1β by mix culturing of astrocytes and activated microglia induced by lipopolysaccharides. Interestingly, Meme et al. found in subsequent experiments that activated microglia treated with lipopolysaccharides were four times more efficient than untreated microglia in inhibiting gap junction communication and Cx43 expression (139). We assume that the reason for this diversity might be that TNF-α and IL-1β are mainly secreted by activated, rather by untreated, microglia. More importantly, TNF-α and IL-1β released by activated microglia could increase astrocytic-hemichannel activity. The increased hemichannels can continuously release ATP, further activating the microglia (38, 128). Thus, abnormally opened astrocytic hemichannels, secondary ATP release, and activated microglia-mediated neuroinflammation may complement each other, leading to a vicious cycle of continuously aggravating post-ischemic tissue damage.
However, the interactions of astrocytes and microglia exert neuroprotective effects under some pathological conditions such as traumatic brain injury (TBI). Microglia in the injury core could be activated after TBI and then release ATP and inflammatory cytokines, and ensuing downregulation of the P2Y1 receptor could then transform the astrocytes to a neuroprotective phenotype (140). Then, reactive astrogliosis would occur in the peri-injured region and accelerate neuroprotective astrocytic scar formation, thus relieving inflammation (141).
Interaction of Astrocytes and Capillaries
The interaction between astrocytes and capillaries is quite meaningful for the energy supply of neurons by astrocytic MCT4 and capillary GLUT. In addition, the lactate in capillaries could also be transported to astrocytes by astrocytic Cx43 hemichannels and capillary MCT1, and further delivered to neuronal axons, inducing axonal degeneration (80, 82). Recent studies have revealed that the opening of astrocytic Cx43 hemichannels could increase after ischemic stroke, contributing to substantial lactate diffusion to astrocytes and neuronal axons (93, 142). Studies have also found that the overexpression of astrocytic MCT4 under hypoxia provides more rapid transmission of glucose, facilitating the energy supply of neurons (82). Furthermore, astrocytes were considered to produce vasoactive factors in response to neuronal activity by their hemichannels, causing rapid and localized changes in cerebral blood flow after ischemic stroke (143, 144). Thus, astrocytes may nourish neurons by controlling the glucose and lactate availability through the regulation of blood flow (81).
Therapies and Applications
Nowadays, an increasing number of studies focus on the significance of Cx43 for irreversible injury after ischemic stroke. The influence of the expression and distribution of Cx43 and the block of hemichannels might affect the outcomes of ischemic stroke. Several astrocytic Cx43 targeted reagents or drugs have been considered to be potential therapeutic in cerebral I/R injury (145).
Leptin is a multifunctional hormone secreted by adipocytes and could regulate food intake and energy metabolism (146). Deng et al. found that leptin could also suppress the elevation of Cx43 expression via the ERK/MAPK signaling pathway in MCAO mice in vivo, further alleviating cerebral I/R injury. In the same study, the authors also demonstrated that leptin could inhibit Cx43 elevation in SY5Y and U87 cells, thus reducing Glu release by inhibiting the function of Cx43 hemichannels and decreasing cell death in in vitro OGD models (147). Carbenoxolone (CBX) is another widely used hemichannel blocker in diverse pathological processes in the brain. It has been proven that CBX can inhibit the release of ATP, further inhibiting or reversing the activation of microglia (148). The latest studies have revealed that CBX can switch the differentiation of activated microglia from M1 to M2, thus providing effective neuroprotection after ischemic stroke (93, 149). Apart from directly blocking hemichannels, a recent study revealed that CBX may influence Cx43 hemichannels and gap junctions by indirect mechanisms such as phosphorylation or internalization of Cx43 subunits (150).
Cx43 mimetic peptides, including Gap 19, Gap 26, Gap 27, peptide 5, and L2 peptide, can also serve as Cx43 hemichannel blockers, further reducing I/R injury (151, 152). In these Cx43 mimetic peptides, Gap26 and Gap27 were found to not only inhibit the opening of hemichannels after ischemic stroke in neonatal rats, but also to modulate gap junction communication due to their poor specificity to Cx43 hemichannels at high concentrations and/or following prolonged exposure (153, 154). Indeed, Gap26 has also been confirmed to protect the heart of rats against myocardial ischemic injury induced by ligation of the left anterior descendent (LAD) by selective inhibition of hemichannels at low concentrations of Gap26 (0.5 μM) (155). Gap27 could also reduce the myocardial infarct size in rat LAD models (156). Interestingly, the function of peptide 5 was shown to be concentration dependent; it could block hemichannels at 5 μM, while blocking gap junctions at 500 μM (157). The L2 sequence is located on the cytoplasmic loop of Cx43, and the Gap19 sequence is a nine amino acid stretch within the L2 domain (158). The L2 peptide can stabilize the open state of gap junctions while blocking hemichannels (158). Slightly different from the L2 peptide, Gap19 blocks hemichannels while not influencing gap junctions on short exposure (30 min) but slightly inhibits them on longer exposures (24–48 h) (158). In addition, studies with Gap19-treated mice after ischemic stroke induced by MCAO found that Gap19 could attenuate the white matter infarct volume by suppressing the expression of Cx43 and of inflammatory cytokines (TNF-α and IL-1β) as well as inhibiting Toll-like receptor 4 pathway activation; experiments with in vitro OGD ischemic stroke models have also confirmed these results (55). Furthermore, treating mouse MCAO models with Gap19 also reduced the myocardial infarct size by blocking Cx43 hemichannels (159). More importantly, Gap19 appeared to be more effective than Gap26/27 in reducing the myocardial infarct volume after heart ischemia (160), possibly because Gap26/27 is less selective to Cx43 gap junctions and hemichannels and it can inhibit channels composed of Cxs other than Cx43 (161).
Conclusion
Astrocytes are the central cells in the neuron–glial syncytium, which can combine parenchyma cells in the CNS into a whole to rapidly and synchronously respond to stimuli by forming Cx43/Cx43 gap junctions with other astrocytes, Cx43/Cx36 gap junctions with neurons, Cx43/Cx47 gap junctions with oligodendrocytes, and indirect interactions with microglia. This markedly large network ensures better between-cell communication and increases tolerance to ischemia. The gap junctions between astrocytes and other cells have an important role in substance and metabolite exchange and cell communication. In ischemic stroke, the phosphorylation of astrocytic Cx43 might lead to the uncoupling of gap junctions between astrocytes and other parenchymal cells, reducing the direct communication between these cells. The subsequent dephosphorylation of Cx43 on hemichannels activates the opening of hemichannels, promoting the release of various pro-inflammatory mediators and toxic molecules, such as ATP and Glu. Meanwhile, astrocytic Cx43 could also become S-Nitrosylated after ischemic stroke, increasing the numbers and opening probability of hemichannels. However, the change in astrocytic Cx43 expression and distribution remains controversial and might depend on ischemia duration, region, and severity. Besides, how ischemia induces change in astrocytic Cx43 expression and distribution and the underlying mechanism also need to be delineated.
The destruction of the neuro-glial syncytium structure resulting from the uncoupling of corresponding gap junctions might weaken the mutual support between astrocytes and other cells, and the increase in hemichannel numbers caused by the uncoupling of gap junctions and permeability caused by the dephosphorylation of Cx43 might enhance and spread neuroinflammation and aggravate injury after ischemic stroke. Based on these theories, astrocytic Cx43 might be a potential target for ischemic stroke treatment. However, Cx43 participates both in the formation of gap junctions and of hemichannels. The former is more likely to play a beneficial role, whereas the latter is more likely to be deleterious in ischemic stroke; accordingly, agents that simply target Cx43 might not have the expected therapeutic effect. Therefore, it might be more meaningful to explore agents that can specifically block hemichannels or promote the maintenance of gap junctions. What is more important is that the currently available research that has focused on Cx43-associated agents was conducted using animal and cell models; whether these agents act protectively in patients with ischemia and their safety in the clinic require further exploration.
Author Contributions
ZL contributed to the design of the study and wrote the first draft of the manuscript. YH contributed to the design of the study. LQ, YL, and YZ drew the pictures. XW, DM, and JF contributed to the editing of the manuscript. All authors contributed to the article and approved the submitted version.
Funding
The work of the authors was supported by the National Science Foundation of China, Grant Nos. 81771257 and 81701158; The science and technology planning project of Jilin Province, Grant No. 20180101161JC and No. 20180520134JH; and National Key Program of China, grant 2017YFC1308401 and 2017YFC0110304.
Conflict of Interest
The authors declare that the research was conducted in the absence of any commercial or financial relationships that could be construed as a potential conflict of interest.
Acknowledgments
We would like to thank Editage (www.editage.cn) for English language editing.
Abbreviations
ATP, Adenosine triphosphate; CBX, carbenoxolone; CNS, central nervous system; Cx, connexin; Glu, glutamate; GLUT, glucose transporters; IL-1β, interleukin-1β; LAD, left anterior descendent; MAPK, mitogen-activated protein kinase; MCAO, middle cerebral artery occlusion; MCT, monocarboxylate transporter; NO, nitric oxide; OGD, oxygen glucose deprivation; ROS, radical oxygen species; TNF-α, tumor necrosis factor-α.
References
1. Carmichael ST. Rodent models of focal stroke: size, mechanism, and purpose. NeuroRx. (2005) 2:396–409. doi: 10.1602/neurorx.2.3.396
2. Lo EH, Dalkara T, Moskowitz MA. Mechanisms, challenges and opportunities in stroke. Nat Rev Neurosci. (2003) 4:399–415. doi: 10.1038/nrn1106
3. Lerario MP, Segal AZ. Arterial ischemic stroke prevention and risk factor management. Prim Care Rep. (2016) 22. Available online at: https://search.proquest.com/docview/1991845173/abstract/DE6C9BF5333B4807PQ/1?accountid=11718
4. Guzik A, Bushnell C. Stroke epidemiology and risk factor management. Continuum. (2017) 23:15–39. doi: 10.1212/CON.0000000000000416
5. Zini A. [Reperfusion therapies in acute ischemic stroke]. G Ital Cardiol. (2019) 20:279–88. doi: 10.1186/s12883-017-1007-y
6. Sallustio F, Koch G, Alemseged F, Konda D, Fabiano S, Pampana E, et al. Effect of mechanical thrombectomy alone or in combination with intravenous thrombolysis for acute ischemic stroke. J Neurol. (2018) 265:2875–80. doi: 10.1007/s00415-018-9073-7
7. Barres BA. The mystery and magic of glia: a perspective on their roles in health and disease. Neuron. (2008) 60:430–40. doi: 10.1016/j.neuron.2008.10.013
8. Xing L, Yang T, Cui S, Chen G. Connexin hemichannels in astrocytes: role in CNS disorders. Front Mol Neurosci. (2019) 12:23. doi: 10.3389/fnmol.2019.00023
9. Hirayama Y, Koizumi S. Astrocytes and ischemic tolerance. Neurosci Res. (2018) 126:53–9. doi: 10.1016/j.neures.2017.11.013
10. Nedergaard M, Ransom B, Goldman SA. New roles for astrocytes: redefining the functional architecture of the brain. Trends Neurosci. (2003) 26:523–30. doi: 10.1016/j.tins.2003.08.008
11. Rash JE, Yasumura T, Dudek FE, Nagy JI. Cell-specific expression of connexins and evidence of restricted gap junctional coupling between glial cells and between neurons. J Neurosci. (2001) 21:1983–2000. doi: 10.1523/JNEUROSCI.21-06-01983.2001
12. Ma D, Feng L, Cheng Y, Xin M, You J, Yin X, et al. Astrocytic gap junction inhibition by carbenoxolone enhances the protective effects of ischemic preconditioning following cerebral ischemia. J Neuroinflammation. (2018) 15:198. doi: 10.1186/s12974-018-1230-5
13. Davidson JO, Green CR, Nicholson LF, O'Carroll SJ, Fraser M, Bennet L, et al. Connexin hemichannel blockade improves outcomes in a model of fetal ischemia. Ann Neurol. (2012) 71:121–32. doi: 10.1002/ana.22654
14. Davidson JO, Green CR, Nicholson LF, Bennet L, Gunn AJ. Deleterious effects of high dose connexin 43 mimetic peptide infusion after cerebral ischaemia in near-term fetal sheep. Int J Mol Sci. (2012) 13:6303–19. doi: 10.3390/ijms13056303
15. Davidson JO, Green CR, Bennet L, Nicholson LF, Danesh-Meyer H, O'Carroll SJ, et al. A key role for connexin hemichannels in spreading ischemic brain injury. Curr Drug Targets. (2013) 14:36–46. doi: 10.2174/138945013804806479
16. Davidson JO, Drury PP, Green CR, Nicholson LF, Bennet L, Gunn AJ. Connexin hemichannel blockade is neuroprotective after asphyxia in preterm fetal sheep. PLoS ONE. (2014) 9:e0096558.doi: 10.1371/journal.pone.0096558
17. Unwin PN, Zampighi G. Structure of the junction between communicating cells. Nature. (1980) 283:545–9. doi: 10.1038/283545a0
18. Dermietzel R, Traub O, Hwang TK, Beyer E, Bennett MV, Spray DC, et al. Differential expression of three gap junction proteins in developing and mature brain tissues. Proc Natl Acad Sci USA. (1989) 86:10148–52. doi: 10.1073/pnas.86.24.10148
19. Nagy JI, Ionescu AV, Lynn BD, Rash JE. Coupling of astrocyte connexins Cx26, Cx30, Cx43 to oligodendrocyte Cx29, Cx32, Cx47: Implications from normal and connexin32 knockout mice. Glia. (2003) 44:205–18. doi: 10.1002/glia.10278
20. Condorelli DF, Belluardo N, Trovato-Salinaro A, Mudo G. Expression of Cx36 in mammalian neurons. Brain Res Brain Res Rev. (2000) 32:72–85. doi: 10.1016/S0165-0173(99)00068-5
21. Chapman RJ, Lall VK, Maxeiner S, Willecke K, Deuchars J, King AE. Localization of neurones expressing the gap junction protein connexin45 within the adult spinal dorsal horn: a study using Cx45-eGFP reporter mice. Brain Struct Func. (2013) 218:751–65. doi: 10.1007/s00429-012-0426-1
22. Bruzzone R, Hormuzdi SG, Barbe MT, Herb A, Monyer H. Pannexins, a family of gap junction proteins expressed in brain. Proc Natl Acad Sci USA. (2003) 100:13644–9. doi: 10.1073/pnas.2233464100
23. Dere E, Zheng-Fischhofer Q, Viggiano D, Gironi Carnevale UA, Ruocco LA, Zlomuzica A, et al. Connexin31.1 deficiency in the mouse impairs object memory and modulates open-field exploration, acetylcholine esterase levels in the striatum, and cAMP response element-binding protein levels in the striatum and piriform cortex. Neuroscience. (2008) 153:396–405. doi: 10.1016/j.neuroscience.2008.01.077
24. Scherer SS, Deschenes SM, Xu YT, Grinspan JB, Fischbeck KH, Paul DL. Connexin32 is a myelin-related protein in the PNS and CNS. J Neurosci. (1995) 15:8281–94. doi: 10.1523/JNEUROSCI.15-12-08281.1995
25. Odermatt B, Wellershaus K, Wallraff A, Seifert G, Degen J, Euwens C, et al. Connexin 47 (Cx47)-deficient mice with enhanced green fluorescent protein reporter gene reveal predominant oligodendrocytic expression of Cx47 and display vacuolized myelin in the CNS. J Neurosci. (2003) 23:4549–59. doi: 10.1523/JNEUROSCI.23-11-04549.2003
26. Eugenin EA, Eckardt D, Theis M, Willecke K, Bennett MV, Saez JC. Microglia at brain stab wounds express connexin 43 and in vitro form functional gap junctions after treatment with interferon-gamma and tumor necrosis factor-alpha. Proc Natl Acad Sci USA. (2001) 98:4190–5. doi: 10.1073/pnas.051634298
27. Orellana JA, Moraga-Amaro R, Diaz-Galarce R, Rojas S, Maturana CJ, Stehberg J, et al. Restraint stress increases hemichannel activity in hippocampal glial cells and neurons. Front Cell Neurosci. (2015) 9:102. doi: 10.3389/fncel.2015.00102
28. Dobrenis K, Chang HY, Pina-Benabou MH, Woodroffe A, Lee SC, Rozental R, et al. Human and mouse microglia express connexin36, and functional gap junctions are formed between rodent microglia and neurons. J Neurosci Res. (2005) 82:306–15. doi: 10.1002/jnr.20650
29. Errede M, Benagiano V, Girolamo F, Flace P, Bertossi M, Roncali L, et al. Differential expression of connexin43 in foetal, adult and tumour-associated human brain endothelial cells. Histochem J. (2002) 34:265–71. doi: 10.1023/a:1023344106815
30. Little TL, Beyer EC, Duling BR. Connexin 43 and connexin 40 gap junctional proteins are present in arteriolar smooth muscle and endothelium in vivo. Am J Physiol. (1995) 268:H729–39. doi: 10.1152/ajpheart.1995.268.2.H729
31. Goodenough DA, Paul DL. Gap junctions. Cold Spring Harbor Perspec Biol. (2009) 1:a002576. doi: 10.1101/cshperspect.a002576
32. Rash JE, Yasumura T, Davidson KG, Furman CS, Dudek FE, Nagy JI. Identification of cells expressing Cx43, Cx30, Cx26, Cx32 and Cx36 in gap junctions of rat brain and spinal cord. Cell Commun Adhes. (2001) 8:315–20. doi: 10.3109/15419060109080745
33. Theis M, Giaume C. Connexin-based intercellular communication and astrocyte heterogeneity. Brain Res. (2012) 1487:88–98. doi: 10.1016/j.brainres.2012.06.045
34. Trosko JE. The gap junction as a “biological rosetta stone”: implications of evolution, stem cells to homeostatic regulation of health and disease in the barker hypothesis. J Cell Commun Signal. (2011) 5:53–66. doi: 10.1007/s12079-010-0108-9
35. Ek Vitorin JF, Pontifex TK, Burt JM. Determinants of Cx43 channel gating and permeation: the amino terminus. Biophys J. (2016) 110:127–40. doi: 10.1016/j.bpj.2015.10.054
36. Beyer EC, Paul DL, Goodenough DA. Connexin family of gap junction proteins. J Membr Biol. (1990) 116:187–94. doi: 10.1007/BF01868459
37. Leithe E, Mesnil M, Aasen T. The connexin 43 C-terminus: a tail of many tales. Biochim Biophys Acta Biomembr. (2018) 1860:48–64. doi: 10.1016/j.bbamem.2017.05.008
38. Faustmann PM, Haase CG, Romberg S, Hinkerohe D, Szlachta D, Smikalla D, et al. Microglia activation influences dye coupling and Cx43 expression of the astrocytic network. Glia. (2003) 42:101–8. doi: 10.1002/glia.10141
39. Giaume C, Leybaert L, Naus CC, Saez JC. Connexin and pannexin hemichannels in brain glial cells: properties, pharmacology, and roles. Front Pharmacol. (2013) 4:88. doi: 10.3389/fphar.2013.00088
40. Orthmann-Murphy JL, Freidin M, Fischer E, Scherer SS, Abrams CK. Two distinct heterotypic channels mediate gap junction coupling between astrocyte and oligodendrocyte connexins. J Neurosci. (2007) 27:13949–57. doi: 10.1523/JNEUROSCI.3395-07.2007
41. Contreras JE, Saez JC, Bukauskas FF, Bennett MV. Gating and regulation of connexin 43 (Cx43) hemichannels. Proc Natl Acad Sci USA. (2003) 100:11388–93. doi: 10.1073/pnas.1434298100
42. Li H, Liu TF, Lazrak A, Peracchia C, Goldberg GS, Lampe PD, et al. Properties and regulation of gap junctional hemichannels in the plasma membranes of cultured cells. J Cell Biol. (1996) 134:1019–30. doi: 10.1083/jcb.134.4.1019
43. Ebihara L, Liu X, Pal JD. Effect of external magnesium and calcium on human connexin46 hemichannels. Biophys J. (2003) 84:277–86. doi: 10.1016/S0006-3495(03)74848-6
44. Valiunas V. Biophysical properties of connexin-45 gap junction hemichannels studied in vertebrate cells. J Gen Physiol. (2002) 119:147–64. doi: 10.1085/jgp.119.2.147
45. John SA, Kondo R, Wang SY, Goldhaber JI, Weiss JN. Connexin-43 hemichannels opened by metabolic inhibition. J Biol Chem. (1999) 274:236–40. doi: 10.1074/jbc.274.1.236
46. Chever O, Lee CY, Rouach N. Astroglial connexin43 hemichannels tune basal excitatory synaptic transmission. J Neurosci. (2014) 34:11228–32. doi: 10.1523/JNEUROSCI.0015-14.2014
47. Ambrosi C, Gassmann O, Pranskevich JN, Boassa D, Smock A, Wang JJ, et al. Pannexin1 and pannexin2 channels show quaternary similarities to connexons and different oligomerization numbers from each other. J Biol Chem. (2010) 285:24420–31. doi: 10.1074/jbc.M110.115444
48. Spray DC, Ye ZC, Ransom BR. Functional connexin “hemichannels”: a critical appraisal. GLIA. (2006) 54:758–73. doi: 10.1002/glia.20429
49. Saez JC, Berthoud VM, Branes MC, Martinez AD, Beyer EC. Plasma membrane channels formed by connexins: their regulation and functions. Physiol Rev. (2003) 83:1359–400. doi: 10.1152/physrev.00007.2003
50. Montero TD, Orellana JA. Hemichannels: new pathways for gliotransmitter release. Neuroscience. (2015) 286:45–59. doi: 10.1016/j.neuroscience.2014.11.048
51. Meunier C, Wang N, Yi C, Dallerac G, Ezan P, Koulakoff A, et al. Contribution of astroglial Cx43 hemichannels to the modulation of glutamatergic currents by D-serine in the mouse prefrontal cortex. J Neurosci. (2017) 37:9064–75. doi: 10.1523/JNEUROSCI.2204-16.2017
52. Stehberg J, Moraga-Amaro R, Salazar C, Becerra A, Echeverria C, Orellana JA, et al. Release of gliotransmitters through astroglial connexin 43 hemichannels is necessary for fear memory consolidation in the basolateral amygdala. FASEB J. (2012) 26:3649–57. doi: 10.1096/fj.11-198416
53. Abudara V, Retamal MA, Del Rio R, Orellana JA. Synaptic functions of hemichannels and pannexons: a double-edged sword. Front Mol Neurosci. (2018) 11:435. doi: 10.3389/fnmol.2018.00435
54. Beyer EC, Berthoud VM. Gap junction gene and protein families: connexins, innexins, and pannexins. Biochim Biophys Acta Biomembr. (2018) 1860:5–8. doi: 10.1016/j.bbamem.2017.05.016
55. Chen Y, Wang L, Zhang L, Chen B, Yang L, Li X, et al. Inhibition of connexin 43 hemichannels alleviates cerebral ischemia/reperfusion injury via the TLR4 signaling pathway. Front Cell Neurosci. (2018) 12:372. doi: 10.3389/fncel.2018.00372
56. Kim Y, Davidson JO, Green CR, Nicholson LFB, O'Carroll SJ, Zhang J. Connexins and pannexins in cerebral ischemia. Biochim Biophys Acta Biomembr. (2018) 1860:224–36. doi: 10.1016/j.bbamem.2017.03.018
57. Scott CA, Tattersall D, O'Toole EA, Kelsell DP. Connexins in epidermal homeostasis and skin disease. Biochim Biophys Acta. (2012) 1818:1952–61. doi: 10.1016/j.bbamem.2011.09.004
58. Duffy HS, Delmar M, Spray DC. Formation of the gap junction nexus: binding partners for connexins. J Physiol. (2002) 96:243–9. doi: 10.1016/S0928-4257(02)00012-8
59. Meier C, Rosenkranz K. Cx43 expression and function in the nervous system-implications for stem cell mediated regeneration. Front Physiol. (2014) 5:106. doi: 10.3389/fphys.2014.00106
60. Lapato AS, Tiwari-Woodruff SK. Connexins and pannexins: at the junction of neuro-glial homeostasis & disease. J Neurosci Res. (2018) 96:31–44. doi: 10.1002/jnr.24088
61. Cronin M, Anderson PN, Cook JE, Green CR, Becker DL. Blocking connexin43 expression reduces inflammation and improves functional recovery after spinal cord injury. Mol Cell Neurosci. (2008) 39:152–60. doi: 10.1016/j.mcn.2008.06.005
62. Wallraff A, Kohling R, Heinemann U, Theis M, Willecke K, Steinhauser C. The impact of astrocytic gap junctional coupling on potassium buffering in the hippocampus. J Neurosci. (2006) 26:5438–47. doi: 10.1523/JNEUROSCI.0037-06.2006
63. Pereda AE. Electrical synapses and their functional interactions with chemical synapses. Nat Rev Neurosci. (2014) 15:250–63. doi: 10.1038/nrn3708
64. Altevogt BM, Paul DL. Four classes of intercellular channels between glial cells in the CNS. J Neurosci. (2004) 24:4313–23. doi: 10.1523/JNEUROSCI.3303-03.2004
65. Allen NJ, Eroglu C. Cell biology of astrocyte-synapse interactions. Neuron. (2017) 96:697–708. doi: 10.1016/j.neuron.2017.09.056
66. Chew SS, Johnson CS, Green CR, Danesh-Meyer HV. Role of connexin43 in central nervous system injury. Exp Neurol. (2010) 225:250–61. doi: 10.1016/j.expneurol.2010.07.014
67. Sohl G, Maxeiner S, Willecke K. Expression and functions of neuronal gap junctions. Nat Rev Neurosci. (2005) 6:191–200. doi: 10.1038/nrn1627
68. Spray DC, Hanstein R, Lopez-Quintero SV, Stout RF Jr, Suadicani SO, et al. Gap junctions and bystander effects: good samaritans and executioners. Wiley interdisciplinary reviews. Membrane Transport Signal. (2013) 2:1–15. doi: 10.1002/wmts.72
69. Massa PT, Mugnaini E. Cell junctions and intramembrane particles of astrocytes and oligodendrocytes: a freeze-fracture study. Neuroscience. (1982) 7:523–38. doi: 10.1016/0306-4522(82)90285-8
70. Kay CW, Ursu D, Sher E, King AE. The role of Cx36 and Cx43 in 4-aminopyridine-induced rhythmic activity in the spinal nociceptive dorsal horn: an electrophysiological study in vitro. Physiol Rep. (2016) 4:e12852.doi: 10.14814/phy2.12852
71. Papaneophytou C, Georgiou E, Kleopa KA. The role of oligodendrocyte gap junctions in neuroinflammation. Channels. (2019) 13:247–63. doi: 10.1080/19336950.2019.1631107
72. Rinholm JE, Vervaeke K, Tadross MR, Tkachuk AN, Kopek BG, Brown TA, et al. Movement and structure of mitochondria in oligodendrocytes and their myelin sheaths. Glia. (2016) 64:810–25. doi: 10.1002/glia.22965
73. Rinholm JE, Hamilton NB, Kessaris N, Richardson WD, Bergersen LH, Attwell D. Regulation of oligodendrocyte development and myelination by glucose and lactate. J Neurosci. (2011) 31:538–48. doi: 10.1523/JNEUROSCI.3516-10.2011
74. Parys B, Cote A, Gallo V, De Koninck P, Sik A. Intercellular calcium signaling between astrocytes and oligodendrocytes via gap junctions in culture. Neuroscience. (2010) 167:1032–43. doi: 10.1016/j.neuroscience.2010.03.004
75. Zahs KR. Heterotypic coupling between glial cells of the mammalian central nervous system. Glia. (1998) 24:85–96. doi: 10.1002/(SICI)1098-1136(199809)24:1<85::AID-GLIA9>3.0.CO;2-#
76. Menichella DM, Majdan M, Awatramani R, Goodenough DA, Sirkowski E, Scherer SS, et al. Genetic and physiological evidence that oligodendrocyte gap junctions contribute to spatial buffering of potassium released during neuronal activity. J Neurosci. (2006) 26:10984–91. doi: 10.1523/JNEUROSCI.0304-06.2006
77. Braet K, Paemeleire K, D'Herde K, Sanderson MJ, Leybaert L. Astrocyte-endothelial cell calcium signals conveyed by two signalling pathways. Eur J Neurosci. (2001) 13:79–91. doi: 10.1046/j.1460-9568.2001.01372.x
78. Newman EA. High potassium conductance in astrocyte endfeet. Science. (1986) 233:453–4. doi: 10.1126/science.3726539
79. Miyata M, Mandai K, Maruo T, Sato J, Shiotani H, Kaito A, et al. Localization of nectin-2delta at perivascular astrocytic endfoot processes and degeneration of astrocytes and neurons in nectin-2 knockout mouse brain. Brain Res. (2016) 1649:90–101. doi: 10.1016/j.brainres.2016.08.023
80. Orellana JA, Saez PJ, Shoji KF, Schalper KA, Palacios-Prado N, Velarde V, et al. Modulation of brain hemichannels and gap junction channels by pro-inflammatory agents and their possible role in neurodegeneration. Antioxid Redox Sign. (2009) 11:369–99. doi: 10.1089/ars.2008.2130
81. Pellerin L, Bouzier-Sore AK, Aubert A, Serres S, Merle M, Costalat R, et al. Activity-dependent regulation of energy metabolism by astrocytes: an update. Glia. (2007) 55:1251–62. doi: 10.1002/glia.20528
82. Abe T, Takahashi S, Suzuki N. Oxidative metabolism in cultured rat astroglia: effects of reducing the glucose concentration in the culture medium and of D-aspartate or potassium stimulation. J Cereb Blood Flow Metab. (2006) 26:153–60. doi: 10.1038/sj.jcbfm.9600175
83. Seifert G, Schilling K, Steinhauser C. Astrocyte dysfunction in neurological disorders: a molecular perspective. Nat Rev Neurosci. (2006) 7:194–206. doi: 10.1038/nrn1870
84. Jha MK, Lee WH, Suk K. Functional polarization of neuroglia: implications in neuroinflammation and neurological disorders. Biochem Pharmacol. (2016) 103:1–16. doi: 10.1016/j.bcp.2015.11.003
85. Pekny M, Nilsson M. Astrocyte activation and reactive gliosis. Glia. (2005) 50:427–34. doi: 10.1002/glia.20207
86. Sofroniew MV. Reactive astrocytes in neural repair and protection. Neuroscientist. (2005) 11:400–7. doi: 10.1177/1073858405278321
87. Bush TG, Puvanachandra N, Horner CH, Polito A, Ostenfeld T, Svendsen CN, et al. Leukocyte infiltration, neuronal degeneration, and neurite outgrowth after ablation of scar-forming, reactive astrocytes in adult transgenic mice. Neuron. (1999) 23:297–308. doi: 10.1016/S0896-6273(00)80781-3
88. Faulkner JR, Woo MJ, Sislak MD, Safroniew MV. Genetically targeted astrocyte scar ablation results in modest local growth of axons after spinal cord injury. J Neurotraum. (2004) 21:1340. Available online at: http://apps.webofknowledge.com/Search.do?product=UA&SID=7DVluIHdjOnkbar3NOy&search_mode=GeneralSearch&prID=08c6d970-23a1-4778-b550-a8380bec950c
89. Ikeda H, Murase K. Glial nitric oxide-mediated long-term presynaptic facilitation revealed by optical imaging in rat spinal dorsal horn. J Neurosci. (2004) 24:9888–96. doi: 10.1523/JNEUROSCI.2608-04.2004
90. Rami A, Volkmann T, Winckler J. Effective reduction of neuronal death by inhibiting gap junctional intercellular communication in a rodent model of global transient cerebral ischemia. Exp Neurol. (2001) 170:297–304. doi: 10.1006/exnr.2001.7712
91. Haupt C, Witte OW, Frahm C. Temporal profile of connexin 43 expression after photothrombotic lesion in rat brain. Neuroscience. (2007) 144:562–70. doi: 10.1016/j.neuroscience.2006.09.054
92. Haupt C, Witte OW, Frahm C. Up-regulation of connexin43 in the glial scar following photothrombotic ischemic injury. Mol Cell Neurosci. (2007) 35:89–99. doi: 10.1016/j.mcn.2007.02.005
93. Yin X, Feng L, Ma D, Yin P, Wang X, Hou S, et al. Roles of astrocytic connexin-43, hemichannels, and gap junctions in oxygen-glucose deprivation/reperfusion injury induced neuroinflammation and the possible regulatory mechanisms of salvianolic acid B and carbenoxolone. J Neuroinflam. (2018) 15:97. doi: 10.1186/s12974-018-1127-3
94. Hossain MZ, Peeling J, Sutherland GR, Hertzberg EL, Nagy JI. Ischemia-induced cellular redistribution of the astrocytic gap junctional protein connexin43 in rat brain. Brain Res. (1994) 652:311–22. doi: 10.1016/0006-8993(94)90242-9
95. Sosinsky GE, Nicholson BJ. Structural organization of gap junction channels. Biochim Biophys Acta. (2005) 1711:99–125. doi: 10.1016/j.bbamem.2005.04.001
96. Lampe PD, TenBroek EM, Burt JM, Kurata WE, Johnson RG, Lau AF. Phosphorylation of connexin43 on serine368 by protein kinase C regulates gap junctional communication. J Cell Biol. (2000) 149:1503–12. doi: 10.1083/jcb.149.7.1503
97. Warn-Cramer BJ, Lampe PD, Kurata WE, Kanemitsu MY, Loo LW, Eckhart W, et al. Characterization of the mitogen-activated protein kinase phosphorylation sites on the connexin-43 gap junction protein. J Biol Chem. (1996) 271:3779–86. doi: 10.1074/jbc.271.7.3779
98. Loo LW, Berestecky JM, Kanemitsu MY, Lau AF. pp60src-mediated phosphorylation of connexin 43, a gap junction protein. J Biol Chem. (1995) 270:12751–61. doi: 10.1074/jbc.270.21.12751
99. Cooper CD, Lampe PD. Casein kinase 1 regulates connexin-43 gap junction assembly. J Biol Chem. (2002) 277:44962–8. doi: 10.1074/jbc.M209427200
100. Solan JL, Lampe PD. Connexin43 phosphorylation: structural changes and biological effects. Biochem J. (2009) 419:261–72. doi: 10.1042/BJ20082319
101. Li WE, Nagy JI. Connexin43 phosphorylation state and intercellular communication in cultured astrocytes following hypoxia and protein phosphatase inhibition. Eur J Neurosci. (2000) 12:2644–50. doi: 10.1046/j.1460-9568.2000.00162.x
102. Beckmann A, Grissmer A, Wolf S, Recktenwald J, Meier C. Oxygen-glucose deprivation in mouse astrocytes is associated with ultrastructural changes in connexin 43 gap junctions. Neuroscience. (2019) 397:67–79. doi: 10.1016/j.neuroscience.2018.11.043
103. Moreno AP, Saez JC, Fishman GI, Spray DC. Human connexin43 gap junction channels. Regulation of unitary conductances by phosphorylation. Circ Res. (1994) 74:1050–7. doi: 10.1161/01.RES.74.6.1050
104. Marquez-Rosado L, Solan JL, Dunn CA, Norris RP, Lampe PD. Connexin43 phosphorylation in brain, cardiac, endothelial and epithelial tissues. Biochim Biophys Acta. (2012) 1818:1985–92. doi: 10.1016/j.bbamem.2011.07.028
105. Freitas-Andrade M, Wang N, Bechberger JF, De Bock M, Lampe PD, Leybaert L, et al. Targeting MAPK phosphorylation of connexin43 provides neuroprotection in stroke. J Exp Med. (2019) 216:916–35. doi: 10.1084/jem.20171452
106. Narne P, Pandey V, Phanithi PB. Role of nitric oxide and hydrogen sulfide in ischemic stroke and the emergent epigenetic underpinnings. Mol Neurobiol. (2019) 56:1749. doi: 10.1007/s12035-018-1141-6
107. Retamal MA, Cortes CJ, Reuss L, Bennett MVL, Saez JC. S-nitrosylation and permeation through connexin 43 hemichannels in astrocytes: induction by oxidant stress and reversal by reducing agents. P Natl Acad Sci USA. (2006) 103:4475–80. doi: 10.1073/pnas.0511118103
108. Contreras JE, Sanchez HA, Eugenin EA, Speidel D, Theis M, Willecke K, et al. Metabolic inhibition induces opening of unapposed connexin 43 gap junction hemichannels and reduces gap junctional communication in cortical astrocytes in culture. Proc Natl Acad Sci USA. (2002) 99:495–500. doi: 10.1073/pnas.012589799
109. Ek-Vitorin JF, Pontifex TK, Burt JM. Cx43 channel gating and permeation: multiple phosphorylation-dependent roles of the carboxyl terminus. Int J Mol Sci. (2018) 19:1659. doi: 10.3390/ijms19061659
110. Cotrina ML, Kang J, Lin JH, Bueno E, Hansen TW, He L, et al. Astrocytic gap junctions remain open during ischemic conditions. J Neurosci. (1998) 18:2520–37. doi: 10.1523/JNEUROSCI.18-07-02520.1998
111. Contreras JE, Sanchez HA, Veliz LP, Bukauskas FF, Bennett MV, Saez JC. Role of connexin-based gap junction channels and hemichannels in ischemia-induced cell death in nervous tissue. Brain Res Brain Res Rev. (2004) 47:290–303. doi: 10.1016/j.brainresrev.2004.08.002
112. Rawanduzy A, Hansen A, Hansen TW, Nedergaard M. Effective reduction of infarct volume by gap junction blockade in a rodent model of stroke. J Neurosurg. (1997) 87:916–20. doi: 10.3171/jns.1997.87.6.0916
113. Nodin C, Nilsson M, Blomstrand F. Gap junction blockage limits intercellular spreading of astrocytic apoptosis induced by metabolic depression. J Neurochem. (2005) 94:1111–23. doi: 10.1111/j.1471-4159.2005.03241.x
114. Hansson E, Muyderman H, Leonova J, Allansson L, Sinclair J, Blomstrand F, et al. Astroglia and glutamate in physiology and pathology: aspects on glutamate transport, glutamate-induced cell swelling and gap-junction communication. Neurochem Int. (2000) 37:317–29. doi: 10.1016/S0197-0186(00)00033-4
115. Asklund T, Appelskog IB, Ammerpohl O, Langmoen IA, Dilber MS, Aints A, et al. Gap junction-mediated bystander effect in primary cultures of human malignant gliomas with recombinant expression of the HSVtk gene. Exp Cell Res. (2003) 284:185–95. doi: 10.1016/S0014-48270200052-6
116. Giaume C, Tabernero A, Medina JM. Metabolic trafficking through astrocytic gap junctions. Glia. (1997) 21:114–23. doi: 10.1002/SICI1098-113619970921:1114::AID-GLIA133.0.CO;2-V
117. Freitas-Andrade M, Naus CC. Astrocytes in neuroprotection and neurodegeneration: the role of connexin43 and pannexin1. Neuroscience. (2016) 323:207–21. doi: 10.1016/j.neuroscience.2015.04.035
118. Wu LY, Yu XL, Feng LY. Connexin 43 stabilizes astrocytes in a stroke-like milieu to facilitate neuronal recovery. Acta Pharmacol Sinica. (2015) 36:928–38. doi: 10.1038/aps.2015.39
119. Walz W, Hertz L. Functional interactions between neurons and astrocytes. II. Potassium homeostasis at the cellular level. Prog Neurobiol. (1983) 20:133–83. doi: 10.1016/0301-0082(83)90013-8
120. Goldberg GS, Lampe PD, Nicholson BJ. Selective transfer of endogenous metabolites through gap junctions composed of different connexins. Nat Cell Biol. (1999) 1:457–9. doi: 10.1038/15693
121. Kang J, Kang N, Lovatt D, Torres A, Zhao Z, Lin J, et al. Connexin 43 hemichannels are permeable to ATP. J Neurosci. (2008) 28:4702–11. doi: 10.1523/JNEUROSCI.5048-07.2008
122. Barber PA, Demchuk AM, Hirt L, Buchan AM. Biochemistry of ischemic stroke. Adv Neurol. (2003) 92:151–64.
123. Aschner M. Astrocytes as mediators of immune inflammatory responses in the CNS. Neurotoxicology. (1998) 19:269–81.
124. Alvarez-Maubecin V, Garcia-Hernandez F, Williams JT, Van Bockstaele EJ. Functional coupling between neurons and glia. J Neurosci. (2000) 20:4091–8. doi: 10.1523/JNEUROSCI.20-11-04091.2000
125. Takeuchi H, Suzumura A. Gap junctions and hemichannels composed of connexins: potential therapeutic targets for neurodegenerative diseases. Front Cell Neurosci. (2014) 8:189. doi: 10.3389/fncel.2014.00189
126. Sofroniew MV. Multiple roles for astrocytes as effectors of cytokines and inflammatory mediators. Neuroscientist. (2014) 20:160–72. doi: 10.1177/1073858413504466
127. Avila-Munoz E, Arias C. When astrocytes become harmful: functional and inflammatory responses that contribute to alzheimer's disease. Ageing Res Rev. (2014) 18:29–40. doi: 10.1016/j.arr.2014.07.004
128. Retamal MA, Froger N, Palacios-Prado N, Ezan P, Saez PJ, Saez JC, et al. Cx43 hemichannels and gap junction channels in astrocytes are regulated oppositely by proinflammatory cytokines released from activated microglia. J Neurosci. (2007) 27:13781–92. doi: 10.1523/JNEUROSCI.2042-07.2007
129. Orellana JA, Froger N, Ezan P, Jiang JX, Bennett MV, Naus CC, et al. ATP and glutamate released via astroglial connexin 43 hemichannels mediate neuronal death through activation of pannexin 1 hemichannels. J Neurochem. (2011) 118:826–40. doi: 10.1111/j.1471-4159.2011.07210.x
130. Castellano P, Nwagbo C, Martinez LR, Eugenin EA. Methamphetamine compromises gap junctional communication in astrocytes and neurons. J Neurochem. (2016) 137:561–75. doi: 10.1111/jnc.13603
131. May D, Tress O, Seifert G, Willecke K. Connexin47 protein phosphorylation and stability in oligodendrocytes depend on expression of Connexin43 protein in astrocytes. J Neurosci. (2013) 33:7985–96. doi: 10.1523/JNEUROSCI.5874-12.2013
132. Basu R, Bose A, Thomas D, Das Sarma J. Microtubule-assisted altered trafficking of astrocytic gap junction protein connexin 43 is associated with depletion of connexin 47 during mouse hepatitis virus infection. J Biol Chem. (2017) 292:14747–63. doi: 10.1074/jbc.M117.786491
133. Cotrina ML, Lin JH, Alves-Rodrigues A, Liu S, Li J, Azmi-Ghadimi H, et al. Connexins regulate calcium signaling by controlling ATP release. Proc Natl Acad Sci USA. (1998) 95:15735–40. doi: 10.1073/pnas.95.26.15735
134. Papaneophytou CP, Georgiou E, Karaiskos C, Sargiannidou I, Markoullis K, Freidin MM, et al. Regulatory role of oligodendrocyte gap junctions in inflammatory demyelination. Glia. (2018) 66:2589–603. doi: 10.1002/glia.23513
135. Giepmans BN. Gap junctions and connexin-interacting proteins. Cardiovasc Res. (2004) 62:233–45. doi: 10.1016/j.cardiores.2003.12.009
136. Kielian T. Glial connexins and gap junctions in CNS inflammation and disease. Journal of neurochemistry. (2008) 106:1000–16. doi: 10.1111/j.1471-4159.2008.05405.x
137. Wixey JA, Reinebrant HE, Carty ML, Buller KM. Delayed P2X4R expression after hypoxia-ischemia is associated with microglia in the immature rat brain. J Neuroimmunol. (2009) 212:35–43. doi: 10.1016/j.jneuroim.2009.04.016
138. Zhou JJ, Cheng C, Qiu Z, Zhou WH, Cheng GQ. Decreased connexin 43 in astrocytes inhibits the neuroinflammatory reaction in an acute mouse model of neonatal sepsis. Neurosci Bull. (2015) 31:763–8. doi: 10.1007/s12264-015-1561-5
139. Meme W, Calvo CF, Froger N, Ezan P, Amigou E, Koulakoff A, et al. Proinflammatory cytokines released from microglia inhibit gap junctions in astrocytes: potentiation by beta-amyloid. FASEB J. (2006) 20:494–6. doi: 10.1096/fj.05-4297fje
140. Shinozaki Y, Shibata K, Yoshida K, Shigetomi E, Gachet C, Ikenaka K, et al. Transformation of astrocytes to a neuroprotective phenotype by microglia via P2Y1 receptor downregulation. Cell Rep. (2017) 19:1151–64. doi: 10.1016/j.celrep.2017.04.047
141. Anderson MA, Burda JE, Ren Y, Ao Y, O'Shea TM, Kawaguchi R, et al. Astrocyte scar formation aids central nervous system axon regeneration. Nature. (2016) 532:195–200. doi: 10.1038/nature17623
142. Abe T, Takahashi S, Suzuki N. Metabolic properties of astrocytes differentiated from rat neurospheres. Brain Res. (2006) 1101:5–11. doi: 10.1016/j.brainres.2006.05.009
143. Metea MR, Newman EA. Glial cells dilate and constrict blood vessels: a mechanism of neurovascular coupling. J Neurosci. (2006) 26:2862–70. doi: 10.1523/JNEUROSCI.4048-05.2006
144. Zonta M, Angulo MC, Gobbo S, Rosengarten B, Hossmann KA, Pozzan T, et al. Neuron-to-astrocyte signaling is central to the dynamic control of brain microcirculation. Nat Neurosci. (2003) 6:43–50. doi: 10.1038/nn980
145. Manjarrez-Marmolejo J, Franco-Perez J. Gap junction blockers: an overview of their effects on induced seizures in animal models. Curr Neuropharmacol. (2016) 14:759–71. doi: 10.2174/1570159X14666160603115942
146. Zhang Y, Proenca R, Maffei M, Barone M, Leopold L, Friedman JM. Positional cloning of the mouse obese gene and its human homologue. Nature. (1994) 372:425–32. doi: 10.1038/372425a0
147. Deng ZH, Liao J, Zhang JY, Liang C, Song CH, Han M, et al. Inhibition of the connexin 43 elevation may be involved in the neuroprotective activity of leptin against brain ischemic injury. Cell Mol Neurobiol. (2014) 34:871–9. doi: 10.1007/s10571-014-0066-5
148. Zhang L, Li YM, Jing YH, Wang SY, Song YF, Yin J. Protective effects of carbenoxolone are associated with attenuation of5 oxidative stress in ischemic brain injury. Neurosci Bull. (2013) 29:311–20. doi: 10.1007/s12264-013-1342-y
149. Tamura K, Alessandri B, Heimann A, Kempski O. The effect of a gap-junction blocker, carbenoxolone, on ischemic brain injury and cortical spreading depression. Neuroscience. (2011) 194:262–71. doi: 10.1016/j.neuroscience.2011.07.043
150. Goldberg GS, Moreno AP, Bechberger JF, Hearn SS, Shivers RR, MacPhee DJ, et al. Evidence that disruption of connexon particle arrangements in gap junction plaques is associated with inhibition of gap junctional communication by a glycyrrhetinic acid derivative. Exp Cell Res. (1996) 222:48–53. doi: 10.1006/excr.1996.0006
151. Evans WH, Leybaert L. Mimetic peptides as blockers of connexin channel-facilitated intercellular communication. Cell Commun Adhes. (2007) 14:265–73. doi: 10.1080/15419060801891034
152. Li X, Zhao H, Tan X, Kostrzewa RM, Du G, Chen Y, et al. Inhibition of connexin43 improves functional recovery after ischemic brain injury in neonatal rats. Glia. (2015) 63:1553–67. doi: 10.1002/glia.22826
153. Decrock E, De Vuyst E, Vinken M, Van Moorhem M, Vranckx K, Wang N, et al. Connexin 43 hemichannels contribute to the propagation of apoptotic cell death in a rat C6 glioma cell model. Cell Death Differ. (2009) 16:151–63. doi: 10.1038/cdd.2008.138
154. Evans WH, De Vuyst E, Leybaert L. The gap junction cellular internet: connexin hemichannels enter the signalling limelight. Biochem J. (2006) 397:1–14. doi: 10.1042/BJ20060175
155. Hawat G, Benderdour M, Rousseau G, Baroudi G. Connexin 43 mimetic peptide Gap26 confers protection to intact heart against myocardial ischemia injury. Pflugers Archiv. (2010) 460:583–92. doi: 10.1007/s00424-010-0849-6
156. Hawat G, Helie P, Baroudi G. Single intravenous low-dose injections of connexin 43 mimetic peptides protect ischemic heart in vivo against myocardial infarction. J Mol Cell Cardiol. (2012) 53:559–66. doi: 10.1016/j.yjmcc.2012.07.008
157. O'Carroll SJ, Alkadhi M, Nicholson LF, Green CR. Connexin 43 mimetic peptides reduce swelling, astrogliosis, and neuronal cell death after spinal cord injury. Cell Commun Adhes. (2008) 15:27–42. doi: 10.1080/15419060802014164
158. D'Hondt C, Iyyathurai J, Wang N, Gourdie RG, Himpens B, Leybaert L, et al. Negatively charged residues (Asp378 and Asp379) in the last ten amino acids of the C-terminal tail of Cx43 hemichannels are essential for loop/tail interactions. Biochem Biophys Res Commun. (2013) 432:707–12. doi: 10.1016/j.bbrc.2013.01.066
159. Wang N, De Vuyst E, Ponsaerts R, Boengler K, Palacios-Prado N, Wauman J, et al. Selective inhibition of Cx43 hemichannels by Gap19 and its impact on myocardial ischemia/reperfusion injury. Basic Res Cardiol. (2013) 108:309. doi: 10.1007/s00395-012-0309-x
160. Schulz R, Gorge PM, Gorbe A, Ferdinandy P, Lampe PD, Leybaert L. Connexin 43 is an emerging therapeutic target in ischemia/reperfusion injury, cardioprotection and neuroprotection. Pharmacol Ther. (2015) 153:90–106. doi: 10.1016/j.pharmthera.2015.06.005
Keywords: ischemic stroke, connexin 43, astrocyte, gap junction, hemichannel, syncytium
Citation: Liang Z, Wang X, Hao Y, Qiu L, Lou Y, Zhang Y, Ma D and Feng J (2020) The Multifaceted Role of Astrocyte Connexin 43 in Ischemic Stroke Through Forming Hemichannels and Gap Junctions. Front. Neurol. 11:703. doi: 10.3389/fneur.2020.00703
Received: 20 February 2020; Accepted: 09 June 2020;
Published: 31 July 2020.
Edited by:
Emmanuel Pinteaux, University of Manchester, United KingdomReviewed by:
Jong Eun Lee, Yonsei University, South KoreaMauricio Antonio Retamal, Universidad del Desarrollo, Chile
Eliseo A. Eugenin, University of Texas Medical Branch at Galveston, United States
Copyright © 2020 Liang, Wang, Hao, Qiu, Lou, Zhang, Ma and Feng. This is an open-access article distributed under the terms of the Creative Commons Attribution License (CC BY). The use, distribution or reproduction in other forums is permitted, provided the original author(s) and the copyright owner(s) are credited and that the original publication in this journal is cited, in accordance with accepted academic practice. No use, distribution or reproduction is permitted which does not comply with these terms.
*Correspondence: Di Ma, YXByaWw4MzE2JiN4MDAwNDA7aG90bWFpbC5jb20=; Jiachun Feng, ZmVuZ2pjZnJhbmsmI3gwMDA0MDsxMjYuY29t