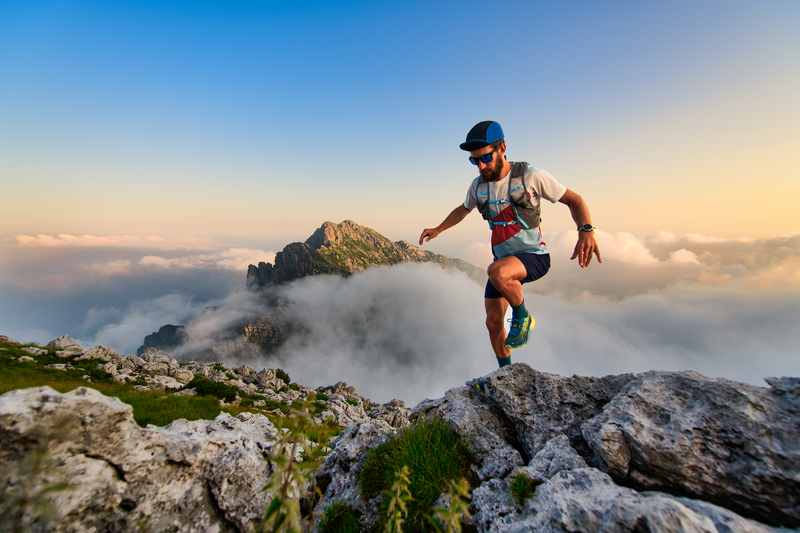
94% of researchers rate our articles as excellent or good
Learn more about the work of our research integrity team to safeguard the quality of each article we publish.
Find out more
ORIGINAL RESEARCH article
Front. Neurol. , 19 June 2020
Sec. Neurotrauma
Volume 11 - 2020 | https://doi.org/10.3389/fneur.2020.00511
This article is part of the Research Topic On the Basis of Sex: Impact on Traumatic Brain Injury View all 14 articles
Every year, millions of people in the US suffer brain damage from mild to severe traumatic brain injuries (TBI) that result from a sudden impact to the head. Despite TBI being a leading cause of death and disability worldwide, sex differences that contribute to varied outcomes post-injury are not extensively studied and therefore, poorly understood. In this study, we aimed to explore biological sex as a variable influencing response to TBI using Drosophila melanogaster as a model, since flies have been shown to exhibit symptoms commonly seen in other mammalian models of TBI. After inflicting TBI using the high-impact trauma device, we isolated w1118 fly brains and assessed gene transcription changes in male and female flies at control and 1, 2, and 4 hr after TBI. Our results suggest that overall, Drosophila females show more gene transcript changes than males. Females also exhibit upregulated expression changes in immune response and mitochondrial genes across all time-points. In addition, we looked at the impact of injury on mitochondrial health and motor function in both sexes before and after injury. Although both sexes report similar changes in mitochondrial oxidation and negative geotaxis, locomotor activity appears to be more impaired in males than females. These data suggest that sex-differences not only influence the response to TBI but also contribute to varied outcomes post-injury.
Traumatic brain injuries (TBI), sudden jolts to the head that cause brain damage (1), can result from sports, domestic violence, auto accidents, falls or explosive blasts (2, 3). TBI is an emerging health epidemic with ~2.5 million cases occurring annually that are severe enough to require hospitalization or cause death (4). Although there is growing evidence that TBI outcome is influenced by host genotype and sex (5), research has largely overlooked investigating sex of the patient as a contributing factor to varied outcomes between males and females. For instance, fewer women than men are recruited for clinical trials and male animal models are predominantly used in TBI research (6). In addition to the differences in how men and women may acquire injuries, there are also sex-specific hormones that affect outcome to TBI (7). Brain function, pharmacokinetics and cellular pathways are all influenced by biological sex (6), therefore, consideration of sex as a variable is crucial for development of successful treatments.
Research that does exist regarding sex differences in TBI outcomes suggests females may be more affected than males (8). Neurocognitive computerized testing in college athletes who sustained sports-related concussions, showed females were 1.7 times more frequently cognitively impaired than males following injury (9). A meta-analysis of 8 TBI studies (20 outcome variables) reported that women were worse than men for 85% of the measured variables (8). Although no differences were seen in neurodegeneration, blood-brain barrier alteration or microglia activation between male and female adult mice after a moderate controlled cortical impact showed that females exhibited more astrocytic hypertrophy at 1-day post-injury (10). Male and female rats given either a single mild TBI or repetitive mild TBI (rmTBI) using a lateral impact model exhibit a sex-dependent response to trauma. All rats that were given rmTBI showed balance, locomotion and motor coordination deficits, but only males had short-term working memory deficits and only females had increased depressive-like behavior in response to rmTBI (11). In an attempt to study the effects of hormone levels, controlled cortical impact was performed on adult rats and endogenous hormones measured by gas chromatography/mass spectrometry. Increased levels of corticosterone, indicative of acute stress was seen in both sexes whereas an increase in progesterone and its metabolites varied by sex, time and location of injury (12). In spite of growing evidence that points toward sex-dependent changes in response to TBI, inclusion of both sexes in all preclinical TBI research is not widely conducted.
Brain injury after TBI typically occurs in 2 stages resulting in primary and secondary damage (13). Primary damage which starts at the moment of impact, resulting from the brain crashing back and forth inside the skull causing bruising, bleeding or even skull fractures, can involve the entire brain or be isolated to a specific part (14, 15). Secondary damage which can continue over several weeks, months or even years (14) after the TBI event is characterized by disruption of cellular processes (16), membrane depolarization, excessive release of excitatory neurotransmitters, activation of NMDA and Ca2+ and Na+ channels (13). Secondary damage also results in activation of apoptotic and inflammatory pathways, mitochondrial dysfunction, over-production of reactive oxygen species and structural changes in biological membranes (13, 17). The most pronounced effect of TBI is axonal damage which when coupled with brain injury triggers a cascade of events increasing tau phosphorylation and neurofibrillary tangle formation (18–20). Intriguingly, the expression of hyperphosphorylated human tau (hTau) has also been shown to elongate mitochondria resulting in mitochondrial dysfunction and cell-death, suggesting a possible cause of mitochondrial abnormalities that have been implicated in neurodegenerative disorders (21). Accumulation of several neurodegeneration-related proteins like synuclein, amyloid-beta, tau, TAR DNA-binding protein 43, presenilin and ubiquitin is also seen post-TBI (22–24).
In this study, we are using Drosophila melanogaster as a model to study TBI. The complex brain and nervous system of flies make it a very powerful model for neuroscience research (25, 26). Consistent with mammalian and human TBI studies, flies subjected to rapid acceleration and impact exhibit TBI related secondary phase symptoms including innate immune response, neurodegeneration, disrupted sleep cycles and a decreased lifespan (27, 28). The few studies that looked at responses to TBI in Drosophila have reported data either from one sex only (29, 30), both sexes combined (31) or only studied epigenetic changes in offspring after injury in both sexes (32). Therefore, we sought to compare response to traumatic injury in both male and female fly brains.
w1118 male and female flies were inflicted with full body trauma using the high-impact trauma (HIT) device and brains were isolated for further analysis. We report changes in gene expression and motor function in both sexes 1, 2, and 4 hr after TBI and show that transcriptional changes in Drosophila females are more pronounced than males. In addition, both sexes show effects on motor function in response to TBI. To the best of our knowledge, this is the first study to report changes in gene transcription at immediate time-points post-injury and to do so in both sexes.
All fly stocks were stored at 25°C at constant humidity and fed with standard sugar/yeast/agar medium. w1118 and UAS-MitoTimer (#57323) were obtained from the Bloomington Drosophila Stock Center. elav-Gal4 (#458) was obtained from Dr. Sokol Todi (Wayne State University). UAS-MitoTimer and elav-Gal4 crosses were performed at the conditions described above. All assays were performed on adult mated flies (10–14 days old).
Full body trauma from a single strike of a modified high impact trauma (HIT) device with the impact arm constrained to a 45° angle was used to inflict male and female files with TBI (27, 33). No more than 50 flies were placed in a plastic vial before being confined to the bottom quarter of the vial by a stationary cotton ball. When the spring was deflected and released, the vial rapidly contacted the pad delivering trauma to the flies as they contact the vial wall.
Mitochondrial oxidation was assessed using a modified MitoTimer protocol (34). Brains were dissected from either control or TBI flies expressing MitoTimer. Three replicates of 10 brains per condition were placed in each well of a 96-well clear-bottom plate containing 50 μl 1XPBS. Red and green fluorescence were measured immediately after dissecting brains for each time-point at the excitation/emission wavelengths of 543/572 and 485/528, respectively, using the Synergy H1 microplate reader (Biotek). The ratio of red over green fluorescence was reported as an indicator of the level of mitochondrial oxidation. All data are represented as mean ± standard error of the mean (SEM). All statistical analyses were performed using GraphPad Prism. One-way ANOVA with Dunnett's multiple comparisons test was used to compute statistical significance (p < 0.05) between groups.
Total RNA was extracted from single fly brains using the QIAzol® lysis reagent and Direct-zol™ RNA MicroPrep kit (Zymo Research) following manufacturer's instructions.
Expression analysis was conducted in collaboration with the Wayne State University Genome Sciences Core. Three biological replicates were used for each condition.
QuantSeq 3′ mRNA-Seq Library Prep Kit FWD for Illumina (Lexogen) was used to generate libraries of sequences close to 3′ end of polyadenylated RNA from 15 ng of total RNA in 5 μl of nuclease-free water following low-input protocol. Library aliquots were assessed for overall quality using the ScreenTape for the Agilent 2200 TapeStation and quantified using Qubit™ 1X dsDNA HS Assay kit (Invitrogen). Barcoded libraries were normalized to 2 nM before sequencing at 300 pM on one lane of a NovaSeq 6000 SP flow cell. After de-multiplexing with Illumina's CASAVA 1.8.2 software, the 50 bp reads were aligned to the Drosophila genome (Build dm3) with STAR_2.4 (35) and tabulated for each gene region (36). Differential gene expression analysis was used to compare transcriptome changes between conditions using edgeR v.3.22.3 (37) and transcripts were defined as significantly differentially expressed at absolute log2 fold change (|log2FC|) > 1 with an FDR <0.05. Significant gene expression changes were submitted for gene ontology analyses using RDAVID (38) for the following categories: GOTERM_BP_ALL, GOTERM_MF_ALL, UP_KEYWORDS, GOTERM_BP_DIRECT, and GOTERM_MF_DIRECT.
Heatmaps were generated using Java Treeview (39). Counts representing the number of reads mapped to each gene were obtained using HTSeq (36) on STAR alignments (35) before normalization. To normalize, a scaling factor was determined by dividing the uniquely mapped reads for each sample by the sample with the highest uniquely mapped number of reads. Each gene count for all samples was multiplied with this scaling factor for normalization. Log2 of the normalized averaged counts for all 3 replicates is represented for each condition on the orange scale (0–10). The log2 fold change, represented on yellow-blue scale (0–6), for each gene is obtained from differential expression analysis across all 3 replicates (37). Genes significant (|log2 FC| > 1, p < 0.05) in at least 1 time point are indicated in black text.
qRT-PCR was performed on select genes to validate 3′ mRNA-Seq results. Total RNA was isolated from 10 fly brains for males and females at control and 2 hr after TBI as described in RNA isolation. To measure the expression levels of target genes, 2 ng RNA was mixed with TaqMan™ Gene Expression Primers (Thermo Fisher, Waltham, MA, USA) and TaqMan™ RNA-to-C 1-Step Kit (Thermo Fisher, Waltham, MA, USA). qRT-PCR reactions were run in a 384-well plate containing 2 biological and 3 technical replicates of each condition. Drs (Dm01822006_s1), DptB (Dm01821557_g1), Mtk (Dm01821460_s1), mRpL55 (Dm02142138_g1), and AttA (Dm02362218_s1) were quantified with QuantStudio 12K Flex run to 40 cycles, using 2−ΔΔCt (cycle threshold) methods and normalizing all transcripts to the reference gene, RpL32 (Dm02151827_g1). Significant change (p < 0.05) was computed using 2-tailed Student's t-test for unequal variance.
Negative geotaxis was measured using a modified climbing assay protocol (31, 40–42). Approximately 20 flies per condition were placed in plastic vials. Flies were gently tapped to the bottom of the vials and then the number of flies that climbed above a 7 cm mark within 15 sec were recorded. The assay was carried out in triplicate (60 flies total) for each of the following conditions: 10 min after flies were inflicted with trauma for immediate response with measurements repeated at 24, 48, and 72 hr. The average percent climbed across all 3 replicates is reported as mean ± SEM. Flies were maintained at 25°C for the duration of the assay. Mixed design ANOVA was used to compute significance (p < 0.05) with condition (Control or TBI) as a between-subjects factor, time (10 min, 24, 48, and 72 hr) as a within-subjects factor and vial as random factor with Tukey for post-hoc comparison. The mixed design ANOVA was performed in R Core Team (43) using the “emmeans” package.
To measure locomotor activity, individual flies (24 biological replicates/condition) for control and TBI condition were placed in tubes containing regular fly food in a Drosophila activity monitoring system which measures the number of times a given fly crosses an infrared beam (TriKinetics Inc., Waltham, MA) (44). The activity was assessed for 2 days. Flies were subjected to 12-hr light/dark cycles with activity summarized every 30 min producing 96 timepoints of data. The number of beam breaks occurring as a result of fly movement in 30-min time-bins before the specified time-point are plotted as locomotor activity for that time-point. Flies that did not live through the recording period were not used in the calculations. Repeated measures ANOVA with Fisher's Least Significant Difference (LSD) and Bonferroni for multiple comparisons test was used to compute statistical significance (p < 0.05) between control and TBI groups using SPSS.
Following brain injury, cellular cascades activate in response to the damage sustained by the primary and secondary effects of the insult (14). To identify genes involved in these pathways, we used 3′ mRNA-Seq to study genome wide gene expression changes between control and TBI flies in both sexes. The majority of TBI data identifying transcriptional changes has focused on investigating gene profiles several hours or days post-injury (29, 30, 33). In our study, we collected individual brains from control and 1-, 2-, and 4-hr post-injury (single strike) time points to capture gene expression changes within the immediate timeframe of TBI which may include primary and secondary effects. Differential gene expression analysis shows significant changes in both sexes after TBI (Figure 1). Gene expression changes in response to TBI were less pronounced in males (Figures 1A–C) as compared to females (Figures 1D–F). Females show more genes effected and more pronounced fold changes with the majority of the transcripts upregulated (|log FC| > 2; p < 0.05) across all time-points.
Figure 1. Gene expression changes after TBI in male and female flies. Volcano plots depicting log2 fold change and –log10(PV) of differentially expressed genes at 1, 2, and 4 hr after injury compared to control are indicated for males (A–C) and females (D–F). The number of significantly upregulated and downregulated gene changes are indicated in yellow in each plot (|log2FC| > 1; p < 0.05). Females show more upregulated gene expression in response to injury than males.
Significant genes identified from mRNA-Seq were classified for their biological functions using DAVID (38, 45). Several gene ontology (GO) categories were changed in both sexes in response to TBI (Tables 1, 2 and Table S1). In females, the highest number of significant categories (FDR < 0.05) were altered 2 hr after injury (141 GO terms) (Figure 2). In addition to the 11 GO terms that overlapped between all 3 time-points, a large overlap was observed between processes at 2 and 4 hr after TBI (49 shared GO terms) (Figure 2). We observed significant changes in GO terms for “mitochondrion,” “neurogenesis,” “humoral immune response,” “programmed cell death,” “nervous system development” and “cell communication” in females (Figure 2, Table 1). Several studies on TBI have reported changes consistent with our findings including mitochondrial dysfunction (17, 46), immune activation (47, 48), apoptosis (49, 50), and activation of axonal regeneration after injury (51). Interestingly, genes involved in “nucleotide binding,” “neurogenesis,” “immune response,” and “mitochondrial translation” were among the processes that were significantly changed after TBI at all time-points. These GO categories are also among the top 10 terms altered after injury (Tables 1, 2). The largest impact on outcome after injury comes from damage to axons and accumulation of proteins involved in maintaining the cytoskeleton (22). Several studies have also focused on determining the link between TBI and later development of neurodegenerative disease like Alzheimer's (52), Parkinson's (53), and Amyotrophic lateral sclerosis (54). Dysfunction or accumulation of proteins like tau (22, 55), and amyloid precursor protein (APP) (56) have been implicated in TBI-mediated neurodegeneration. Surprisingly, we did not see any change in transcription of tau or Appl in our data (GSE140663: Differential gene expression and counts data). It is possible that alteration to protein or post-transcriptional regulation mediates the effects of these genes in response to TBI. However, we did see significant enrichment of “cytoskeleton,” “microtubule organization” and “axon transport” GO terms in females after injury, indicative of neurodegeneration after trauma. For males, although there are significant changes observed in gene expression related to “nervous system development,” “immune effector process,” and “neurogenesis,” there was no overlap seen between processes across any of the 3 time-points after injury (Table 2).
Table 1. Gene ontology terms significantly (FDR < 0.05) changed in females in response to traumatic brain injury.
Table 2. Gene ontology terms significantly (FDR < 0.05) changed in males in response to traumatic brain injury.
Figure 2. Shared gene ontology terms in females across time-points after injury. The Venn diagram shows significantly changed GO terms for females at 1, 2, and 4 hr after injury as well as overlap between time-points. The number of GO terms differentially regulated at each time-point is indicated in parenthesis.
The pattern of changes between male and female flies indicates that females show more transcriptional changes in response to TBI that continues up to 4 hr of injury. In males, however, the transcriptional response is more subtle at these early time-points.
Multiple studies have explored the role of inflammatory processes and provided clues into the cell types and molecular pathways affected by TBI (30, 47, 48). TBI-related damage from secondary injuries due to activated immune response was shown previously in a similar fly model of head injury (57). In our analysis, we have also observed significant changes in transcript levels in several immune pathway genes in response to brain injury (Figure 3).
Figure 3. Immune gene expression changes in response to injury. Heatmaps depicting expression changes in immune function related genes for females (A) and males (B) at control, 1, 2, and 4 hr after injury. The orange scale represents average normalized counts for 3 replicates in each of the indicated groups. Yellow-blue scale shows fold change for each gene at 1, 2-, and 4-hr post-injury compared to control. 1/C, fold change at 1-hr compared to control; 2/C, fold change at 2-hr compared to control; and 4/C, fold change at 4-hr compared to control. All genes indicated in black font are significant (|log2FC| > 1, p < 0.05).
The Drosophila innate immune system is highly conserved with mammals and consists primarily of the Toll, Immunodeficiency (Imd) and Janus Kinase protein and the Signal Transducer and Activator of Transcription (JAK-STAT) pathways, which together combat fungal and bacterial infections (58, 59). Previous studies have explored activation of JAK-STAT (60) in response to injury but we did not see any change in gene expression associated with this pathway. We did, however, observe changes in transcript levels for genes involved in Toll, Imd pathway and JNK pathway as seen in other Drosophila TBI models (30, 57, 61). Although the genetic components for activation of Toll and Imd pathways are independent, induction of antimicrobial peptide genes like, Drosomycin (Drs), Defensin (Def), and Metchnikowin (Mtk) depends on the activation of both pathways (62). In females, we observed a phasic response in several antibacterial and antifungal effector proteins like Mtk, Drs, CecC, imd, Rel, Def, and CecB, all upregulated 2 hr after injury but not significantly changed at 1 and 4 hr post-TBI (Figure 3A). Mop, a positive regulator of Toll-NF-κB signaling, is significantly upregulated at all timepoints in response to TBI. Several immune response genes including AttA, AttC, CecA2, and Nup98-96 are induced in response to injury. Although significant at all time-points, a phasic change characterized by an increased upregulation of expression is observed in AttA, AttC, and CecA2 at 2 h.
Unlike females, males have no significant transcript level changes in the antibacterial and antifungal genes assessed (Figure 3B). Although we see no significant induction of immune response after injury in males, there is consistently high levels of transcripts seen for some genes including Rel and Drs. Rel, a transcription factor involved in the immune deficiency pathway is highly expressed in both sexes at control and TBI conditions. Similarly, Drs, an antifungal peptide, is expressed at all conditions in both sexes but significantly induced only in females after injury. We also observed a phasic change in transcription of repo, a transcription factor specifically expressed in glial cells, in both sexes after injury (Data available in GSE140663: Differential gene expression and counts data). Repo transcription is significantly upregulated in females at 1 hr (log2FC: 3.10; p < 0.05) and 4 hr (log2FC: 4.02; p < 0.05) after injury with no significant change at 2 hr whereas in males, repo is significantly downregulated only at 2 hr (log2FC: −2.142; p < 0.05) after injury. Drs, DptB, Mtk, and AttA expression was tested by qRT-PCR for both males and females (Table S2).
These data suggest that immune response varies in males and females post-TBI. Interestingly, females exhibit a phasic change in immune pathways with induction of some genes 2 hr after trauma but no significant change at 1 and 4 hr. Phasic activation of immune response genes has previously been observed in transcriptional studies from a mixture of male and female flies inflicted with TBI within 1–8 hr after injury (29). Thus, similar studies over longer time-points would be helpful to deduce if this pattern is repeated beyond the time-points assessed.
Mitochondria are subcellular organelles required for several metabolic processes and energy generation by oxidative phosphorylation (63). Mitochondria are present in all cell types and organ systems but differ in morphology and quantity suggesting tissue and system-specific roles (64). Injury to mitochondria leads to oxidative stress, subsequent apoptosis and decreased cellular energy (17). These changes impair neurologic functions, as commonly observed not only in TBI but also in other neurodegenerative diseases (46). We, therefore, looked at changes in expression of genes related to mitochondria and oxidative phosphorylation in Drosophila (Figure 4).
Figure 4. Mitochondrial gene expression changes after injury. Heatmaps depicting expression changes in mitochondria related genes for females (A) and males (B) at control, 1, 2, and 4 hr after injury. Orange scale represents the average of normalized counts for 3 replicates in each group indicated above. Yellow-blue scale shows fold change for each gene at 1, 2-, and 4-hr post-injury compared to control. 1/C, fold change at 1-hr compared to control; 2/C, fold change at 2-hr compared to control; and 4/C, fold change at 4-hr compared to control. All genes indicated in black font are significant (|log2FC| > 1, p < 0.05).
In female flies, a significant increase in transcripts was observed for Miro (vital for mitochondrial homeostasis and microtubule-based mitochondrial transport) and prel (which contributes to the integrity of mitochondrial structures and the activity of respiratory chain complex IV) (Figure 4A). Transcripts for vimar, a guanine nucleotide exchange factor for Miro, were significantly decreased post-TBI. Vimar has been previously shown to increase mitochondrial fission in Drosophila (65) so change in expression of vimar and miro post-TBI could be indicative of alteration in mitochondrial dynamics in response to injury. We also observed a significant increase in transcripts in the SLC25 family of mitochondrial transporters including colt and aralar1. SLC25 family of transport proteins shuttle metabolites, nucleotides and cofactors across the inner mitochondrial membrane and are essential for energy conversion and cell homeostasis (66). An increase in response to injury in these genes might be mediated through the increase in cellular energy demands for repair of damage sustained by TBI. Additionally, we have observed a significant increase in transcripts for genes involved in the Drosophila oxidative phosphorylation system including Surf1 (involved in the assembly of the mitochondrial respiratory chain Cytochrome Oxidase), SdhA and SdhB (encoding the iron-sulfur cluster-containing subunit of the succinate dehydrogenase complex, which oxidizes succinate to fumarate) after injury. The oxidative phosphorylation system drives the synthesis of ATP; therefore, it is not surprising to find an increase in gene expression related to this system post-injury to upregulate cell capacity and generate more ATP. In addition, upregulation of genes involved in mitochondrial biogenesis (mRpS21, mRpS25 and mRpL43) also occurs post-TBI. Mitochondrial biogenesis can be altered as part of a concerted cellular response to metabolic changes that demand more ATP output and increase in functional mitochondria (67). This is important to TBI pathology as mitochondrial dysfunction is associated with increased reactive oxygen species (ROS) production, an effect of mitochondrial dysfunction and contributes to toxicity (68).
Similar to the differences seen in immune response between sexes, males have few significant changes for mitochondria related genes (Figure 4B). Only two mitochondrial biogenesis genes mRpL55, increased transcripts at all time points, and mRpL43, decreased transcripts at 2 hr, show differential expression in males. In females mRpL55 is unchanged while mRpL43 is upregulated at all time points. mRpL55 expression was confirmed by qRT-PCR for both sexes (Table S2). This data demonstrates that TBI influences expression of genes involved in mitochondrial activity and oxidative phosphorylation significantly in females at early TBI timepoints with few changes seen in males. There is evidence of increased mitochondrial biogenesis 24 hr after TBI in male mice (69), so the disparity in transcription of mRpL55 and mRpL43 in male flies is surprising. For both sexes, it is possible that transcription of other biogenesis related genes may increase at later time-points.
Mitochondria play an important role in maintaining cellular energy homeostasis through oxidative phosphorylation system (70). Highly reactive oxygen species are byproducts typically generated during such respiration and metabolism processes (70). In healthy conditions, endogenous antioxidants like superoxide dismutase and glutathione molecules inhibit production of ROS (71). Under physiological stress conditions such as brain injury, ROS production increases dramatically causing significant cell damage (72). Impaired mitochondrial function as a result of excessive ROS is also seen in neurodegenerative diseases like Alzheimer's, Parkinson's, Huntington's, and tauopathies (73, 74). Mitochondrial dysfunction coupled with increased ROS and decreased ATP production is commonly seen in secondary damage to TBI (17). Thus, monitoring mitochondrial turnover is important considering its essential role in health and disease.
To assess mitochondrial health in vivo, we made use of the MitoTimer reporter gene (75, 76). MitoTimer encodes a DsRed mutant (DsRed-E5) that fluoresces green when mitochondria are newly synthesized and shifts irreversibly to red upon oxidation (75). The maturation from green to red is unaffected by pH, ionic strength or protein concentration, but is affected by light, temperature and oxygen exposure (77). In this study, we expressed UAS-MitoTimer using the pan-neural driver elav-Gal4 and measured fluorescence at control and TBI conditions. The ratio of red/green fluorescence intensity is a measure of mitochondrial stress (76). For both sexes, we observed no change in mitochondrial oxidation after 1 hr of injury (Figure 5, Figure S1). We saw a significant increase in red/green ratio after 2 and 4 hr of injury in females (Figure 5A, Figure S1A) whereas males show significant change only after 4 hr (Figure 5B, Figure S1B). Although this indicates increased mitochondrial turnover and oxidation in both sexes, it also suggests a delayed response in males. In a previous study, we have also shown significant increase in COX activity and decrease in ATP production 24 hr after TBI (33).
Figure 5. Mitochondrial stress increases in both sexes after injury. Measurement of mitochondrial oxidation in female (A) and male (B) flies at control and 1, 2, and 4-hr post-injury using the UAS-MitoTimer reporter shows increased oxidation after injury. In both sexes, mitochondrial oxidation is significantly (*p < 0.05; One-way ANOVA with Dunnett test) increased post-TBI. Overall, males show lower levels of red/green fluorescence intensity ratio than females at each time-point. For each condition, 3 replicates of 10 brains each were assessed and mean ± SEM were plotted.
Behavioral effects like loss of motor skills, coordination, and balance impairment are commonly observed post-TBI in experimental models and also in clinical patients (25). Mild TBI in mice is shown to alter diurnal locomotor activity and response to light (78). A comparison of mobility in people who suffered a moderate to severe TBI compared to controls suggests that even if TBI patients seem to have generally recovered their locomotor abilities, deficits can persist (79). Flies also exhibit ataxia and incapacitation not attributable to damaged legs and wings after injury (27). To assess the extent of injury in movement behavior after TBI, we analyzed climbing ability and locomotor activity at control and TBI conditions for both male and female flies (Figure 6). The climbing assay employs tapping of the vials to cluster flies at the bottom, thus subjecting them to a mechanical stimulus which has a rapid kinetic effect on flies. Locomotion behavior, however, assesses motor coordination in the absence of a stimulus and climbing-independent movement.
Figure 6. Motor function is affected after injury in both sexes. Plots show defects in climbing ability (top-panel) and locomotor activity (bottom-panel) in females (A,C) and males (B,D) after TBI. Climbing ability is indicated as percent climbed for an average of 3 replicates (20 flies per replicate) and assessed at control, 10 min, 24-, 48-, and 72- h after injury. Significant decrease in percent climbed is observed for both sexes at 10 min after injury (p < 0.05 from mixed design ANOVA with Tukey). Locomotor activity at control, 1-, 2-, 4-, and 24-hr post-injury is shown as an average of activity for at least 20 flies in each group. A significant decrease in locomotor activity is observed for both sexes after TBI (*p < 0.05 from LSD and #p < 0.05 from Bonferroni).
Normally, when stimulated by tapping to the bottom of the vial, flies rapidly climb to the top and stay there. This behavior in Drosophila is called negative geotactic response and has been studied in fly models of neurodegeneration to identify molecules involved in fine motor control (80). We used a climbing assay to assess defects in this response after injury by tapping them to the bottom of vial and recording the number of flies that cross 70% height of the vial in 15 sec. We observed a significant decrease (mixed design ANOVA with Tukey) in climbing ability only at 10 min after injury for both females (Figure 6A) and males (Figure 6B) implying similar recovery in climbing ability for both sexes (Figures 6A,B).
Locomotor activity was assessed in flies using the Drosophila activity monitoring system (TriKinetics Inc., Waltham, MA). Adult flies were placed in monitors immediately after being inflicted with brain injury and activity data was collected for 48 hr. We observed a significant decrease in activity in both sexes immediately after TBI at 0 hr (repeated measures ANOVA with LSD and Bonferroni) (Figure 6, Figure S2). Females show some recovery starting at 1 hr and continuing through 24 hr (Figure 6C, Figure S2A), but males have significantly decreased activity up to 24 hr (only with LSD comparison) (Figure 6D, Figure S2B). Overall, females have lower locomotor activity than males at all time-points including control flies. We saw no change after 24 hr (data not shown).
These data suggest that although both sexes exhibit motor defects in response to TBI, females show faster recovery than males.
Sex as a confounding biological variable to TBI outcome has not been widely considered in previous transcriptomic studies (81–85). In flies, genome-wide mRNA expression profiles were studied in whole male flies to compare age and diet related mechanisms that contribute to injuries after TBI (27, 29) and transcriptional changes at 1-, 3-, and 7-days post-injury in whole heads using Translating Ribosome Affinity Purification and Sequencing (TRAP-seq) (30). We have also previously shown selective intron retention in genes associated with tricarboxylic acid (TCA) cycle 24 hr post-TBI in whole heads from male and female flies pooled together after 1- or 2-strikes (27, 33). Since the Drosophila brain occupies only a very small part of the head, about 14% dry weight (86), the previous whole head studies may include transcript information derived from non-brain tissue (30, 33). In this present study, 3′ mRNA libraries were constructed from isolated adult male and female brains before and after TBI to examine sex dependent outcomes post-injury in the fly brain (27). Our results suggest that, overall, Drosophila females exhibit stronger gene expression changes in response to TBI than males. Although we see sex differences in gene transcription, the cause remains unclear. The presence of metabolic tissues or sex-specific gene expression could be potential factors for these differences and require further study. We also assessed motor function and mitochondrial health in both sexes after injury and observed that although both sexes show motor defects and increased mitochondrial oxidation, males exhibit subtle changes in both the number of altered transcripts and magnitude of differential gene expression post-injury than females.
Immune response to injury in the brain is a key mediator in recovery, and progressive impairments become apparent when compromised (20, 87). Many groups have reported activation of neuroinflammatory response after TBI, which is also recognized as a key player in recovery (88, 89) but very few studies have reported sex divergence of TBI-mediated neuroinflammation. In humans, gender-differences in immunity are well-established (90, 91) as seen from the fact that females produce more severe immune reactions and have a higher incidence of autoimmune diseases, which may result from the influence of sex hormones on the immune system (92). In Drosophila too, dichotomy between the sexes in the gene mRNA levels of the antifungal genes Drs and Mtk has previously been observed (90). In our study, we saw upregulation of several genes involved in Toll and Imd pathways in females with no significant changes observed in males (Figure 3). Several of the genes implicated in the Toll pathway have increased transcripts in females but no change in males. Transcripts of other immune response genes (Def, Attacins, Cecropins) that are seen to be increased in females in our model, were also seen to be higher in males from a Drosophila closed head injury model 1 and 3- days post-injury (30). Since we observed no transcriptional changes in males up to 4 hr after injury, one possible explanation which would require future study is that the immune response to injury in Drosophila males is not as immediate as in females. It is also important to consider that some of the immune effects could result from the sex-dependent response to full body trauma.
The neuroinflammatory reaction that follows TBI is a result of the interactions between several immune pathways (48). Here, we observed significantly increased transcripts only at 2 hr for Mtk, Drs, CecC, imd, and Rel in females (Figure 3). It is not known whether the expression profile of these genes continues to remain unchanged after 4 hr or another elevated response would be observed in additional timepoints. Considering that there are several immune genes changed only in females after injury, it is possible that the immune response in females is induced immediately after trauma and plateaus within a few hours. In males, however, it is likely that the response initiates after a few hours and continues to remain upregulated for a few days post-trauma, as seen in a male mouse model of controlled cortical impact where microglia decreased 1 day after injury and increased at 1 and 2 weeks post-injury (93). In general, immune response is intended to promote neuroprotection and recovery, but when dysregulation arises, it can become maladaptive (94). Therefore, it is important to better understand the timing and dynamic changes in immune response after TBI. Altogether, our results show that the immune response in female and male Drosophila are not identical and result from complex interplay of many factors including a phasic change in gene expression.
It is becoming increasingly apparent that mitochondrial metabolism is also sexually dimorphic (95). Sex specificities in mitochondrial biogenesis, ATP production, oxidative phosphorylation activities, oxygen consumption, ROS production and calcium uptake have been observed in different tissues from rodents and humans (96–99). In our analysis, we observed higher transcription of genes involved in oxidative phosphorylation, mitochondrial protein transporters, and mitochondrial translation in females than males after injury (Figure 4). At baseline, the expression of several genes is similar in both sexes, but transcription levels exhibit diverse changes in response to injury. These findings match reports in a pediatric TBI model of rats, where mitochondrial activity is higher in females after injury (100). However, we did observe significant upregulation in mitochondrial turnover in both sexes (Figure 5, Figure S1), indicating gender similarities in clearance of damaged mitochondria. In the incidence of stroke and neurodegenerative diseases, sex differences in mitochondrial protective effects of estrogen were also identified (101). Although, it has not been extensively studied in Drosophila, the possibility of sex-specific genes regulating hormones which in turn influence the response to brain injury cannot be ruled out. Emerging evidence also suggests that mitochondria provide a platform for signaling pathways involved in immune response mainly through transcriptional regulation of inflammatory chemokines/cytokines and their maturation of inflammasomes (102, 103). It is, therefore, not surprising that females show increased transcript levels of mitochondrial and immune genes in response to TBI in our study. It is, however, yet to be established, what sex-specific genes or hormones contribute to a possible delayed response in males but an immediate response in females.
Individuals with traumatic brain injury often experience lasting locomotor deficits and impaired motor coordination (79). Sexual dimorphism in locomotor activity of D. melanogaster has been widely studied (104–106). We also observed activity differences in our study where male flies exhibit reduced locomotion, but females do not show similar changes post-injury (Figure 6, Figure S2). This disparity in recovery after TBI suggests that impairments cannot be fully attributed to physical damages but may also involve sex-specific gene changes. Interestingly, we observed significant increase in Tip60 transcripts after injury in females. In a Drosophila model of Alzheimer's, increasing levels of Tip60 histone acetyltransferase rescued axonal transport and larval motor function defects (107). Therefore, it is possible that certain locomotor or movement associated gene changes in females are protective and contribute toward faster recovery than males.
In this study, we looked at changes in gene expression and motor function in response to TBI in w1118 male and female flies fed standard diet. In summary, we found an effect of biological sex on brain injury response and outcome. Throughout post-TBI assessment, we saw elevated immune genes with peak transcript levels occurring at 2 hr post-TBI in females. Our findings offer insights into the complexities of the different outcomes of brain injury that can be further explored in the development of treatment and management strategies to improve outcomes.
Gene expression data are available in the GEO database under accession number GSE140663. Table S1 contains gene ontology analyses for significant gene transcription changes.
DR and KG are senior authors and conceived the project. ES and KG conceptualized the content. ES performed the data analysis and wrote the manuscript. KG edited the manuscript and assisted with data analysis.
This work was funded by the National Institutes of Health [Grant Nos. 5R01ES012933 (DR), 5P30ES020957 (DR), and 1UG3OD023285 (DR)]. In addition, the Office of the Vice President for Research at Wayne State University provided pilot funds for DR.
The authors declare that the research was conducted in the absence of any commercial or financial relationships that could be construed as a potential conflict of interest.
We thank Dr. Sokol Todi for his insight and useful suggestions in this project as well as for his generous contribution of the elav-Gal4 fly line.
The Supplementary Material for this article can be found online at: https://www.frontiersin.org/articles/10.3389/fneur.2020.00511/full#supplementary-material
Figure S1. Individual data points for MitoTimer assay. Plots show individual data points for MitoTimer reporter assay for females (A) and males (B) at control and 1, 2, and 4 hr post-TBI. Three replicates of 10 brains each were assessed for every time-point and mean ± SEM were plotted.
Figure S2. Individual data points for locomotor activity. Plots show individual data points for locomotor activity for females (A) and males (B) at control and 1, 2, 4, and 24 hr post-TBI (n > 20). Each data point represents average activity of the fly in the 30-min bin before the specified time-point.
Table S1. Gene Ontology tables for males and females.
Table S2. qRT-PCR data for select genes.
1. Mayfield Brain & Spine. Traumatic Brain Injury (2018). Available online at: https://mayfieldclinic.com/pe-tbi.htm
2. Hyder AA, Wunderlich CA, Puvanachandra P, Gururaj G, Kobusingye OC. The impact of traumatic brain injuries: a global perspective. NeuroRehabilitation. (2007) 22:341–53. doi: 10.3233/NRE-2007-22502
3. DeKosky ST, Ikonomovic MD, Gandy S. Traumatic brain injury–football, warfare, and long-term effects. N Engl J Med. (2010) 363:1293–6. doi: 10.1056/NEJMp1007051
4. Taylor CA, Bell JM, Breiding MJ, Xu L. Traumatic brain injury–related emergency department visits, hospitalizations, and deaths — United States, 2007 and 2013. Surveill. Summ. (2017) 66:1–16. doi: 10.15585/mmwr.ss6609a1
5. McAllister TW. Genetic factors in traumatic brain injury. Handb Clin Neurol. (2015) 128:723–39. doi: 10.1016/B978-0-444-63521-1.00045-5
6. Gupte R, Brooks W, Vukas R, Pierce J, Harris J. Sex differences in traumatic brain injury: what we know and what we should know. J Neurotrauma. (2019) 36, 3063–91. doi: 10.1089/neu.2018.6171
7. Roof RL, Duvdevani R, Stein DG. Gender influences outcome of brain injury: progesterone plays a protective role. Brain Res. (1993) 607:333–6. doi: 10.1016/0006-8993(93)91526-X
8. Farace E, Alves WM. Do women fare worse: a metaanalysis of gender differences in traumatic brain injury outcome. J Neurosurg. (2000) 93:539–45. doi: 10.3171/jns.2000.93.4.0539
9. Broshek DK, Kaushik T, Freeman JR, Erlanger D, Webbe F, Barth JT. Sex differences in outcome following sports-related concussion. J Neurosurg. (2005) 102:856–63. doi: 10.3171/jns.2005.102.5.0856
10. Jullienne A, Salehi A, Affeldt B, Baghchechi M, Haddad E, Avitua A, et al. Male and female mice exhibit divergent responses of the cortical vasculature to traumatic brain injury. J Neurotrauma. (2018) 35:1646–58. doi: 10.1089/neu.2017.5547
11. Wright DK, O'Brien TJ, Shultz SR, Mychasiuk R. Sex matters: repetitive mild traumatic brain injury in adolescent rats. Ann Clin Transl Neurol. (2017) 4:640–54. doi: 10.1002/acn3.441
12. Meffre D, Pianos A, Liere P, Eychenne B, Cambourg A, Schumacher M, et al. Steroid profiling in brain and plasma of male and pseudopregnant female rats after traumatic brain injury: analysis by gas chromatography/mass spectrometry. Endocrinology. (2007) 148:2505–17. doi: 10.1210/en.2006-1678
13. Werner C, Engelhard K. Pathophysiology of traumatic brain injury. Br J Anaesth. (2007) 99:4–9. doi: 10.1093/bja/aem131
14. Ciuffreda KJ, Ludlam DP, Yadav NK, Thiagarajan P. Traumatic brain injury. Adv Ophthalmol Optom. (2016) 1:307–33. doi: 10.1016/j.yaoo.2016.03.013
15. Adams H, Mitchell DE, Graham DI, Doyle D. Diffuse brain damage of immediate impact type. Its relationship to 'primary brain-stem damage' in head injury. Brain. (1977) 100:489–502. doi: 10.1093/brain/100.3.489
16. Xiong Y, Mahmood A, Chopp M. Animal models of traumatic brain injury. Nat Rev Neurosci. (2013) 14:128–42. doi: 10.1038/nrn3407
17. Hiebert JB, Shen Q, Thimmesch AR, Pierce JD. Traumatic brain injury and mitochondrial dysfunction. Am J Med Sci. (2015) 350:132–8. doi: 10.1097/MAJ.0000000000000506
18. Rubenstein R, Chang B, Grinkina N, Drummond E, Davies P, Ruditzky M, et al. Tau phosphorylation induced by severe closed head traumatic brain injury is linked to the cellular prion protein. Acta Neuropathol Commun. (2017) 5:30. doi: 10.1186/s40478-017-0435-7
19. Blennow K, Hardy J, Zetterberg H. The neuropathology and neurobiology of traumatic brain injury. Neuron. (2012) 76:886–99. doi: 10.1016/j.neuron.2012.11.021
20. Kokiko-Cochran ON, Godbout JP. The inflammatory continuum of traumatic brain injury and Alzheimer's disease. Front Immunol. (2018) 9:672. doi: 10.3389/fimmu.2018.00672
21. DuBoff B, Gotz J, Feany MB. Tau promotes neurodegeneration via DRP1 mislocalization in vivo. Neuron. (2012) 75:618–32. doi: 10.1016/j.neuron.2012.06.026
22. Sivanandam TM, Thakur MK. Traumatic brain injury: a risk factor for Alzheimer's disease. Neurosci Biobehav Rev. (2012) 36:1376–81. doi: 10.1016/j.neubiorev.2012.02.013
23. Jain KK. Neuroprotection in traumatic brain injury. Handb Neuroprotection. (2019) 13:281–336. doi: 10.1007/978-1-4939-9465-6_4
24. Factor SA, Weiner WJ. Prior history of head trauma in Parkinson's disease. Mov Disord. (1991) 6:225–9. doi: 10.1002/mds.870060306
25. Shah EJ, Gurdziel K, Ruden DM. Mammalian models of traumatic brain injury and a place for Drosophila in TBI research. Front Neurosci. (2019) 13:409. doi: 10.3389/fnins.2019.00409
26. Pandey UB, Nichols CD. Human disease models in Drosophila melanogaster and the role of the fly in therapeutic drug discovery. Pharmacol Rev. (2011) 63:411–36. doi: 10.1124/pr.110.003293
27. Katzenberger RJ, Loewen CA, Wassarman DR, Petersen AJ, Ganetzky B, Wassarman DA. A Drosophila model of closed head traumatic brain injury. Proc Natl Acad Sci USA. (2013) 110:E4152–9. doi: 10.1073/pnas.1316895110
28. Barekat A, Gonzalez A, Mauntz RE, Kotzebue RW, Molina B, El-Mecharrafie N, et al. Using Drosophila as an integrated model to study mild repetitive traumatic brain injury. Sci Rep. (2016) 6:25252. doi: 10.1038/srep25252
29. Katzenberger RJ, Ganetzky B, Wassarman DA. Age and diet affect genetically separable secondary injuries that cause acute mortality following traumatic brain injury in Drosophila. G3 (Bethesda). (2016) 6:4151–66. doi: 10.1534/g3.116.036194
30. van Alphen B, Stewart S, Iwanaszko M, Xu F, Bang E, Rozenfeld S, et al. Glial immune-related pathways as mediators of closed head TBI effects on behavior in Drosophila. BioRxiv. (2018) 422535. doi: 10.1101/422535
31. Lateef S, Holman A, Carpenter J, James J. Can therapeutic hypothermia diminish the impact of traumatic brain injury in Drosophila melanogaster? J Exp Neurosci. (2019) 13:1179069518824852. doi: 10.1177/1179069518824852
32. Chauhan V, Chauhan A. Traumatic injury in female Drosophila melanogaster affects the development and induces behavioral abnormalities in the offspring. Behav Brain Funct. (2019) 15:11. doi: 10.1186/s12993-019-0163-1
33. Sen A, Gurdziel K, Liu J, Qu W, Nuga OO, Burl RB, et al. Smooth, an hnRNP-L homolog, might decrease mitochondrial metabolism by post-transcriptional regulation of isocitrate dehydrogenase (Idh) and other metabolic genes in the sub-acute phase of traumatic brain injury. Front Genet. (2017) 8:175. doi: 10.3389/fgene.2017.00175
34. Bardai FH, Ordonez DG, Bailey RM, Hamm M, Lewis J, Feany MB. Lrrk promotes tau neurotoxicity through dysregulation of actin and mitochondrial dynamics. PLoS Biol. (2018) 16:e2006265. doi: 10.1371/journal.pbio.2006265
35. Dobin A, Davis CA, Schlesinger F, Drenkow J, Zaleski C, Jha S, et al. STAR: ultrafast universal RNA-seq aligner. Bioinformatics. (2013) 29:15–21. doi: 10.1093/bioinformatics/bts635
36. Anders S, Pyl PT, Huber W. HTSeq–a python framework to work with high-throughput sequencing data. Bioinformatics. (2015) 31:166–9. doi: 10.1093/bioinformatics/btu638
37. Robinson MD, McCarthy DJ, Smyth GK. edgeR: A bioconductor package for differential expression analysis of digital gene expression data. Bioinformatics. (2010) 26:139–40. doi: 10.1093/bioinformatics/btp616
38. Fresno C, Fernandez EA. RDAVIDWebService: a versatile R interface to DAVID. Bioinformatics. (2013) 29:2810–1. doi: 10.1093/bioinformatics/btt487
39. Saldanha AJ. Java treeview–extensible visualization of microarray data. Bioinformatics. (2004) 20:3246–8. doi: 10.1093/bioinformatics/bth349
40. Sutton JR, Blount JR, Libohova K, Tsou WL, Joshi GS, Paulson HL, et al. Interaction of the polyglutamine protein ataxin-3 with Rad23 regulates toxicity in Drosophila models of spinocerebellar ataxia type 3. Hum Mol Genet. (2017) 26:1419–31. doi: 10.1093/hmg/ddx039
41. Ordonez DG, Lee MK, Feany MB. α-synuclein induces mitochondrial dysfunction through spectrin and the actin cytoskeleton. Neuron. (2018) 97:108–24.e6. doi: 10.1016/j.neuron.2017.11.036
42. Barone MC, Bohmann D. Assessing neurodegenerative phenotypes in Drosophila dopaminergic neurons by climbing assays and whole brain immunostaining. J Vis Exp. 2013:e50339. doi: 10.3791/50339
43. R Core Team. R: A Language and Environment for Statistical Computing. Vienna: R Foundation for Statistical Computing (2019). Available online at: https://www.R-project.org/ (accessed May 6, 2020).
44. Harbison ST, McCoy LJ, Mackay TF. Genome-wide association study of sleep in Drosophila melanogaster. BMC Genomics. (2013) 14:281. doi: 10.1186/1471-2164-14-281
45. Huang da W, Sherman BT, Lempicki RA. Bioinformatics enrichment tools: paths toward the comprehensive functional analysis of large gene lists. Nucleic Acids Res. (2009) 37:1–13. doi: 10.1093/nar/gkn923
46. Fischer TD, Hylin MJ, Zhao J, Moore AN, Waxham MN, Dash PK. Altered mitochondrial dynamics and TBI pathophysiology. Front Syst Neurosci. (2016) 10:29. doi: 10.3389/fnsys.2016.00029
47. Simon DW, McGeachy MJ, Bayir H, Clark RS, Loane DJ, Kochanek PM. The far-reaching scope of neuroinflammation after traumatic brain injury. Nat Rev Neurol. (2017) 13:171–91. doi: 10.1038/nrneurol.2017.13
48. Jassam YN, Izzy S, Whalen M, McGavern DB, El Khoury J. Neuroimmunology of traumatic brain injury: time for a paradigm shift. Neuron. (2017) 95:1246–65. doi: 10.1016/j.neuron.2017.07.010
49. Raghupathi R, Graham DI, McIntosh TK. Apoptosis after traumatic brain injury. J Neurotrauma. (2000) 17:927–38. doi: 10.1089/neu.2000.17.927
50. Keane RW, Kraydieh S, Lotocki G, Alonso OF, Aldana P, Dietrich WD. Apoptotic and antiapoptotic mechanisms after traumatic brain injury. J Cereb Blood Flow Metab. (2001) 21:1189–98. doi: 10.1097/00004647-200110000-00007
51. Shoichet MS, Tate CC, Baumann MD, LaPlaca MC. Strategies for regeneration and repair in the injured central nervous system. In: Reichert WM, editor. Indwelling Neural Implants: Strategies for Contending with the In Vivo Environment. Boca Raton, FL: CRC Press/Taylor & Francis (2008). doi: 10.1201/9781420009309.ch8
52. Ramos-Cejudo J, Wisniewski T, Marmar C, Zetterberg H, Blennow K, de Leon MJ, et al. Traumatic brain injury and Alzheimer's disease: the cerebrovascular link. EBioMedicine. (2018) 28:21–30. doi: 10.1016/j.ebiom.2018.01.021
53. Gardner RC, Byers AL, Barnes DE, Li Y, Boscardin J, Yaffe K. Mild TBI and risk of Parkinson disease: a chronic effects of neurotrauma consortium study. Neurology. (2018) 90:e1771–9. doi: 10.1212/WNL.0000000000005522
54. Anderson EN, Gochenaur L, Singh A, Grant R, Patel K, Watkins S, et al. Traumatic injury induces stress granule formation and enhances motor dysfunctions in ALS/FTD models. Hum Mol Genet. (2018) 27:1366–81. doi: 10.1093/hmg/ddy047
55. Yang WJ, Chen W, Chen L, Guo YJ, Zeng JS, Li GY, et al. Involvement of tau phosphorylation in traumatic brain injury patients. Acta Neurol Scand. (2017) 135:622–7. doi: 10.1111/ane.12644
56. Cartagena CM, Mountney A, Hwang H, Swiercz A, Rammelkamp Z, Boutte AM, et al. Subacute changes in cleavage processing of amyloid precursor protein and tau following penetrating traumatic brain injury. PLoS ONE. (2016) 11:e0158576. doi: 10.1371/journal.pone.0158576
57. Katzenberger RJ, Chtarbanova S, Rimkus SA, Fischer JA, Kaur G, Seppala JM, et al. Death following traumatic brain injury in Drosophila is associated with intestinal barrier dysfunction. Elife. (2015) 4:e04790. doi: 10.7554/eLife.04790
58. Lemaitre B, Hoffmann J. The host defense of Drosophila melanogaster. Annu Rev Immunol. (2007) 25:697–743. doi: 10.1146/annurev.immunol.25.022106.141615
59. Sudmeier LJ, Samudrala S-S, Howard SP, Ganetzky B. Persistent activation of the innate immune response in adult Drosophila following radiation exposure during larval development. G3 (Bethesda). (2015) 5:2299–306. doi: 10.1534/g3.115.021782
60. Zhao JB, Zhang Y, Li GZ, Su XF, Hang CH. Activation of JAK2/STAT pathway in cerebral cortex after experimental traumatic brain injury of rats. Neurosci Lett. (2011) 498:147–52. doi: 10.1016/j.neulet.2011.05.001
61. Ortolano F, Colombo A, Zanier ER, Sclip A, Longhi L, Perego C, et al. c-Jun N-terminal kinase pathway activation in human and experimental cerebral contusion. J Neuropathol Exp Neurol. (2009) 68:964–71. doi: 10.1097/NEN.0b013e3181b20670
62. Tanji T, Hu X, Weber AN, Ip YT. Toll and IMD pathways synergistically activate an innate immune response in Drosophila melanogaster. Mol Cell Biol. (2007) 27:4578–88. doi: 10.1128/MCB.01814-06
63. Walker MA, Volpi S, Sims KB, Walter JE, Traggiai E. Powering the immune system: mitochondria in immune function and deficiency. J Immunol Res. (2014) 2014:164309. doi: 10.1155/2014/164309
64. Rossignol R, Malgat M, Mazat JP, Letellier T. Threshold effect and tissue specificity. Implication for mitochondrial cytopathies. J Biol Chem. (1999) 274:33426–32. doi: 10.1074/jbc.274.47.33426
65. Ding L, Lei Y, Han Y, Li Y, Ji X, Liu L. Vimar is a novel regulator of mitochondrial fission through Miro. PLoS Genet. (2016) 12:e1006359. doi: 10.1371/journal.pgen.1006359
66. Ruprecht JJ, Kunji ERS. The SLC25 mitochondrial carrier family: structure and mechanism. Trends Biochem Sci. (2019) 45:244–58. doi: 10.1016/j.tibs.2019.11.001
67. Ploumi C, Daskalaki I, Tavernarakis N. Mitochondrial biogenesis and clearance: a balancing act. FEBS J. (2017) 284:183–95. doi: 10.1111/febs.13820
68. Sen N. Aberrant cell cycle induction is pivotal for mitochondrial biogenesis after traumatic brain injury. Neural Regen Res. (2019) 14:1215–6. doi: 10.4103/1673-5374.251305
69. Li X, Wang H, Gao Y, Li L, Tang C, Wen G, et al. Protective effects of quercetin on mitochondrial biogenesis in experimental traumatic brain injury via the Nrf2 signaling pathway. PLoS ONE. (2016) 11:e0164237. doi: 10.1371/journal.pone.0164237
70. Semple BD. Early preservation of mitochondrial bioenergetics supports both structural and functional recovery after neurotrauma. Exp Neurol. (2014) 261:291–7. doi: 10.1016/j.expneurol.2014.07.013
71. Schieber M, Chandel NS. ROS function in redox signaling and oxidative stress. Curr Biol. (2014) 24:R453–62. doi: 10.1016/j.cub.2014.03.034
72. Nathan C, Cunningham-Bussel A. Beyond oxidative stress: an immunologist's guide to reactive oxygen species. Nat Rev Immunol. (2013) 13:349–61. doi: 10.1038/nri3423
73. Matsuda N, Sato S, Shiba K, Okatsu K, Saisho K, Gautier CA, et al. PINK1 stabilized by mitochondrial depolarization recruits parkin to damaged mitochondria and activates latent parkin for mitophagy. J Cell Biol. (2010) 189:211–21. doi: 10.1083/jcb.200910140
74. Gogvadze V, Orrenius S, Zhivotovsky B. Mitochondria as targets for chemotherapy. Apoptosis. (2009) 14:624–40. doi: 10.1007/s10495-009-0323-0
75. Laker RC, Xu P, Ryall KA, Sujkowski A, Kenwood BM, Chain KH, et al. A novel MitoTimer reporter gene for mitochondrial content, structure, stress, and damage in vivo. J Biol Chem. (2014) 289:12005–15. doi: 10.1074/jbc.M113.530527
76. Hernandez G, Thornton C, Stotland A, Lui D, Sin J, Ramil J, et al. MitoTimer: a novel tool for monitoring mitochondrial turnover. Autophagy. (2013) 9:1852–61. doi: 10.4161/auto.26501
77. Terskikh A, Fradkov A, Ermakova G, Zaraisky A, Tan P, Kajava AV, et al. “Fluorescent timer”: protein that changes color with time. Science. (2000). 290:1585–8. doi: 10.1126/science.290.5496.1585
78. Wang Y-S, Hsieh W, Chung J-R, Lan T-H, Wang Y. Repetitive mild traumatic brain injury alters diurnal locomotor activity and response to the light change in mice. Sci Rep. (2019) 9:14067. doi: 10.1038/s41598-019-50513-5
79. McFadyen BJ, Cantin JF, Swaine B, Duchesneau G, Doyon J, Dumas D, et al. Modality-specific, multitask locomotor deficits persist despite good recovery after a traumatic brain injury. Arch Phys Med Rehabil. (2009) 90:1596–606. doi: 10.1016/j.apmr.2009.03.010
80. Aggarwal A, Reichert H, VijayRaghavan K. A locomotor assay reveals deficits in heterozygous Parkinson's disease model and proprioceptive mutants in adult Drosophila. Proc Natl Acad Sci USA. (2019) 116:24830–9. doi: 10.1073/pnas.1807456116
81. Arneson D, Zhang G, Ying Z, Zhuang Y, Byun HR, Ahn IS, et al. Single cell molecular alterations reveal target cells and pathways of concussive brain injury. Nat Commun. (2018) 9:3894. doi: 10.1038/s41467-018-06222-0
82. Zhong J, Jiang L, Cheng C, Huang Z, Zhang H, Liu H, et al. Altered expression of long non-coding RNA and mRNA in mouse cortex after traumatic brain injury. Brain Res. (2016) 1646:589–600. doi: 10.1016/j.brainres.2016.07.002
83. Yang LX, Yang LK, Zhu J, Chen JH, Wang YH, Xiong K. Expression signatures of long non-coding RNA and mRNA in human traumatic brain injury. Neural Regen Res. (2019) 14:632–41. doi: 10.4103/1673-5374.247467
84. Wang CF, Zhao CC, Weng WJ, Lei J, Lin Y, Mao Q, et al. Alteration in long non-coding RNA expression after traumatic brain injury in rats. J Neurotrauma. (2017) 34:2100–8. doi: 10.1089/neu.2016.4642
85. Lipponen A, Paananen J, Puhakka N, Pitkanen A. Analysis of post-traumatic brain injury gene expression signature reveals tubulins, Nfe2l2, Nfkb, Cd44, and S100a4 as treatment targets. Sci Rep. (2016) 6:31570. doi: 10.1038/srep31570
86. Posey KL, Jones LB, Cerda R, Bajaj M, Huynh T, Hardin PE, et al. Survey of transcripts in the adult Drosophila brain. Genome Biol. (2001) 2:RESEARCH0008. doi: 10.1186/gb-2001-2-3-research0008
87. Petersen AJ, Wassarman DA. Drosophila innate immune response pathways moonlight in neurodegeneration. Fly. (2012) 6:169–72. doi: 10.4161/fly.20999
88. Rubin TG, Lipton ML. Sex differences in animal models of traumatic brain injury. J Exp Neurosci. (2019) 13:1179069519844020. doi: 10.1177/1179069519844020
89. Villapol S, Loane DJ, Burns MP. Sexual dimorphism in the inflammatory response to traumatic brain injury. Glia. (2017) 65:1423–38. doi: 10.1002/glia.23171
90. Taylor K, Kimbrell DA. Host immune response and differential survival of the sexes in Drosophila. Fly. (2007) 1:197–204. doi: 10.4161/fly.5082
91. Marriott I, Huet-Hudson YM. Sexual dimorphism in innate immune responses to infectious organisms. Immunol Res. (2006) 34:177–92. doi: 10.1385/IR:34:3:177
92. Bouman A, Heineman MJ, Faas MM. Sex hormones and the immune response in humans. Hum Reprod Update. (2005) 11:411–23. doi: 10.1093/humupd/dmi008
93. Jin X, Ishii H, Bai Z, Itokazu T, Yamashita T. Temporal changes in cell marker expression and cellular infiltration in a controlled cortical impact model in adult male C57BL/6 mice. PLoS ONE. (2012) 7:e41892. doi: 10.1371/journal.pone.0041892
94. McKee CA, Lukens JR. Emerging roles for the immune system in traumatic brain injury. Front Immunol. (2016) 7:556. doi: 10.3389/fimmu.2016.00556
95. Demarest TG, McCarthy MM. Sex differences in mitochondrial (dys)function: implications for neuroprotection. J Bioenerg Biomembr. (2015) 47:173–88. doi: 10.1007/s10863-014-9583-7
96. Ventura-Clapier R, Moulin M, Piquereau J, Lemaire C, Mericskay M, Veksler V, et al. Mitochondria: a central target for sex differences in pathologies. Clin Sci. (2017) 131:803–22. doi: 10.1042/CS20160485
97. Harish G, Venkateshappa C, Mahadevan A, Pruthi N, Bharath MM, Shankar SK. Mitochondrial function in human brains is affected by pre- and post mortem factors. Neuropathol Appl Neurobiol. (2013) 39:298–315. doi: 10.1111/j.1365-2990.2012.01285.x
98. Escames G, Diaz-Casado ME, Doerrier C, Luna-Sanchez M, Lopez LC, Acuna-Castroviejo D. Early gender differences in the redox status of the brain mitochondria with age: effects of melatonin therapy. Horm Mol Biol Clin Investig. (2013) 16:91–100. doi: 10.1515/hmbci-2013-0026
99. Kim HJ, Magrane J, Starkov AA, Manfredi G. The mitochondrial calcium regulator cyclophilin D is an essential component of oestrogen-mediated neuroprotection in amyotrophic lateral sclerosis. Brain. (2012) 135(Pt 9):2865–74. doi: 10.1093/brain/aws208
100. Fraunberger EA, Shutt TE, Esser MJ. Sex-dependent and chronic alterations in behavior and mitochondrial function in a rat model of pediatric mild traumatic brain injury. Brain Inj. (2019) 33:534–42. doi: 10.1080/02699052.2019.1565898
101. Razmara A, Sunday L, Stirone C, Wang XB, Krause DN, Duckles SP, et al. Mitochondrial effects of estrogen are mediated by estrogen receptor alpha in brain endothelial cells. J Pharmacol Exp Ther. (2008) 325:782–90. doi: 10.1124/jpet.107.134072
102. Sandhir R, Halder A, Sunkaria A. Mitochondria as a centrally positioned hub in the innate immune response. Biochim Biophys Acta Mol Basis Dis. (2017) 1863:1090–7. doi: 10.1016/j.bbadis.2016.10.020
103. Monlun M, Hyernard C, Blanco P, Lartigue L, Faustin B. Mitochondria as molecular platforms integrating multiple innate immune signalings. J Mol Biol. (2017) 429:1–13. doi: 10.1016/j.jmb.2016.10.028
104. Tompkins L, Gross AC, Hall JC, Gailey DA, Siegel RW. The role of female movement in the sexual behavior of Drosophila melanogaster. Behav Genet. (1982) 12:295–307. doi: 10.1007/BF01067849
105. Cook RM. Courtship processing in Drosophila melanogaster. II. An adaptation to selection for receptivity to wingless males. Anim Behav. (1973) 21:349–58. doi: 10.1016/S0003-3472(73)80077-6
106. Cobb M, Connolly K, Burnet B. The relationship between locomotor activity and courtship in the melanogaster species sub-group of Drosophila. Anim Behav. (1987) 35:705–13. doi: 10.1016/S0003-3472(87)80106-9
Keywords: TBI, immune, mitochondria, sex-differences, bimodal recovery
Citation: Shah EJ, Gurdziel K and Ruden DM (2020) Drosophila Exhibit Divergent Sex-Based Responses in Transcription and Motor Function After Traumatic Brain Injury. Front. Neurol. 11:511. doi: 10.3389/fneur.2020.00511
Received: 06 December 2019; Accepted: 08 May 2020;
Published: 19 June 2020.
Edited by:
T. John Wu, Uniformed Services University of the Health Sciences, United StatesReviewed by:
David Andrew Wassarman, University of Wisconsin-Madison, United StatesCopyright © 2020 Shah, Gurdziel and Ruden. This is an open-access article distributed under the terms of the Creative Commons Attribution License (CC BY). The use, distribution or reproduction in other forums is permitted, provided the original author(s) and the copyright owner(s) are credited and that the original publication in this journal is cited, in accordance with accepted academic practice. No use, distribution or reproduction is permitted which does not comply with these terms.
*Correspondence: Katherine Gurdziel, Z3VyZHppZWxAd2F5bmUuZWR1; Douglas M. Ruden, ZG91Z2xhc3JAd2F5bmUuZWR1
†These authors share senior authorship
Disclaimer: All claims expressed in this article are solely those of the authors and do not necessarily represent those of their affiliated organizations, or those of the publisher, the editors and the reviewers. Any product that may be evaluated in this article or claim that may be made by its manufacturer is not guaranteed or endorsed by the publisher.
Research integrity at Frontiers
Learn more about the work of our research integrity team to safeguard the quality of each article we publish.