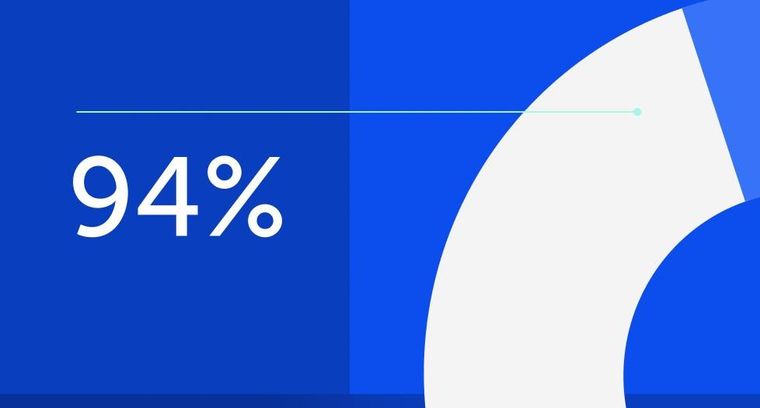
94% of researchers rate our articles as excellent or good
Learn more about the work of our research integrity team to safeguard the quality of each article we publish.
Find out more
REVIEW article
Front. Neurol., 13 March 2020
Sec. Multiple Sclerosis and Neuroimmunology
Volume 11 - 2020 | https://doi.org/10.3389/fneur.2020.00150
This article is part of the Research TopicUpdate on Translational Neuroimmunology - Research of ISNI 2018View all 21 articles
The human body has a large, diverse community of microorganisms which not only coexist with us, but also perform many important physiological functions, including metabolism of dietary compounds that we are unable to process ourselves. Furthermore, these bacterial derived/induced metabolites have the potential to interact and influence not only the local gut environment, but the periphery via interaction with and modulation of cells of the immune and nervous system. This relationship is being further appreciated every day as the gut microbiome is researched as a potential target for immunomodulation. A common feature among inflammatory diseases including relapsing-remitting multiple sclerosis (RRMS) is the presence of gut microbiota dysbiosis when compared to healthy controls. However, the specifics of these microbiota-neuro-immune system interactions remain unclear. Among all factors, diet has emerged as a strongest factor regulating structure and function of gut microbial community. Phytoestrogens are one class of dietary compounds emerging as potentially being of interest in this interaction as numerous studies have identified depletion of phytoestrogen-metabolizing bacteria such as Adlercreutzia, Parabacteroides and Prevotella in RRMS patients. Additionally, phytoestrogens or their metabolites have been reported to show protective effects when compounds are administered in the animal model of MS, Experimental Autoimmune Encephalomyelitis (EAE). In this review, we will illustrate the link between MS and phytoestrogen metabolizing bacteria, characterize the importance of gut bacteria and their mechanisms of action in the production of phytoestrogen metabolites, and discuss what is known about the interactions of specific compounds with cells immune and nervous system. A better understanding of gut bacteria-mediated phytoestrogen metabolism and mechanisms through which these metabolites facilitate their biological actions will help in development of novel therapeutic options for MS as well as other inflammatory diseases.
Relapsing-remitting multiple sclerosis (RRMS) is a chronic inflammatory disease of the central nervous system (CNS), affecting ~1 million people in the US and over 2.3 million worldwide (1). Collective evidence suggests that disease results from an aberrant T-cell mediated response to myelin-derived antigens in genetically susceptible individuals. Multiple genetic and environmental factors have been implicated in the predisposition to RRMS (2). Among a number of environmental risk factors linked with MS, the gut microbiota appear to be particularly important, as highlighted by a number of recent studies reporting gut dysbiosis in RRMS patients (3–8). Although the mechanism through which the gut microbiota influences RRMS pathogenesis is unknown, diet has emerged as the strongest factor influencing the gut microbiome.
The adult human gut is colonized by a large number of microorganisms (~1013 bacteria). The majority of which (~90%) belong to the Firmicutes and Bacteroidetes phyla. The remainder represent Actinobacteria, Proteobacteria, and few other phyla present at very low abundance (9). The fact that only a few bacterial phyla are present in the human gut suggests that they were actively selected during human evolution. As human evolution is nutrition centric, it is hypothesized that gut bacteria capable of efficiently extracting energy from ingested plants and animal meat would provide a survival advantage. Gut microbiota composition is heavily influenced by dietary habits, with unindustrialized rural communities showing higher abundance of bacteria enriched in enzymes capable of digesting plant-based complex polysaccharides. At the same time, individuals from industrialized nations and eating a western diet rich in animal protein, fats, and simple sugars are enriched in gut bacteria containing enzymes responsible for metabolism of simple sugars, amino acids and bile acids (10). Even within a population, gut microbiota composition can be altered due to seasonal change in the food source (11). A study of Hadza hunter-gatherers of Tanzania showed this seasonal change in gut microbiome based on dietary sources, as this population rely mostly on plant based foods during rainy season but shift to plant plus meat-based diet during dry season (11). These seasonal changes in gut microbiota confirm an important role of diet (plant and meat both) in influencing the bacterial community; however, it is unclear whether one diet has advantage over other. Specifically it is unknown whether proposed pathogenic effect of meat based and/or Western diet (12) is due to meat itself or due to factors associated with industrialization/modernization such as processing of foods, increased use of antibiotics etc. (13). However, evaluation of the microbiome-mediated benefits or drawbacks of plant- vs. meat-based diets, western diet, or any other dietary interventions of interest in the scientific community are beyond the scope of this review.
Breakdown of these foods might generate key metabolites necessary for various physiologic functions of the host including development and regulation of nervous and immune systems (14). It has been clearly established that change in diet changes the composition of the gut microbiota; however the mechanisms by which this affects host physiology are slowly being understood. Non-redundant bacterial metabolites which are dependent on ingestion of certain dietary components, such as the phytoestrogens and their metabolites discussed here, are of increasing interest. As the gut microbiome plays an important role in the energy harvesting for the host, therefore any changes in the composition of the gut microbiota could have widespread effects on physiologic homeostasis and overall human health (15–18).
Our recent summary of RRMS microbiome studies across different geographical regions (USA, Japan, UK and Italy) shows enrichment or depletion of specific bacterial genera when compared to healthy controls (HC) (19, 20). One observation was loss of Bacteroidetes species, especially those of the genera Prevotella and Parabacteroides suggesting a role of these bacteria in RRMS [Table 1; (3–8)]. Our group (19) and others (3–5) reported a lower abundance of Prevotella in fecal samples of RRMS patients compared to HC. Additionally, treatment with disease modifying therapies (DMT) led to a higher abundance of Prevotella in RRMS patients than in untreated patients (5, 20). Further, Cosorich et al. also reported lower level of Prevotella when analyzing duodenal biopsies from RRMS patients with active disease compared to HC (7). Another Bacteroidetes genus, Parabacteroides has been reported to be at lower abundance in adult RRMS patients when compared to HC (4, 8). We observed reduced abundance of Parabacteroides in RRMS patients vs. HC from the Midwestern United States (4). Similarly, Cekanaviciute et al. reported that Parabacteroides distasonis is at lower abundance in treatment naive RRMS patients from the US west coast than HC, suggesting that higher level of P. distasonis may protect against RRMS (8). Further, we also observed a lower abundance of the phytoestrogen metabolizing bacteria Adlercreutzia (equolifaciens) in RRMS patients compared to HC (4) and Adlercreutzia was also reported to be increased in germ-free (GF) mice transplanted with fecal matters from HC compared to mice receiving fecal transplant from RRMS patients (21).
Thus, these studies indicate that loss of Bacteroidetes genera Prevotella and Parabacteroides might play a role in the predisposition and/or exacerbation in RRMS. As MS is an inflammatory disease where balance between pro- and anti-inflammatory responses are shifted toward inflammatory responses, it is reasonable to hypothesize that bacteria depleted in MS were involved in induction/maintenance of anti-inflammatory responses. More discussion relating to the possible mechanism of this protective role through induction of immunoregulatory cells will be discussed in this review under Phytoestrogens and Immune Cells.
Conversely, Firmicutes such as Akkermansia, Dorea, and Archaea-Methanobrevibacter were more abundant in stool from RRMS patients (4, 5, 8), suggesting that these gut microbes might have pro-inflammatory effects. This increased abundance could reasonably contribute to the induction and/or maintenance of pro-inflammatory cells in the gut, thus influencing or contributing to a systemic inflammatory state consistent with RRMS. However, Akkermansia had been shown to have anti-inflammatory effects in obesity and diabetes due to their ability to produce short-chain fatty acids (SCFA) (22). Similarly, Dorea has been suggested to be anti-inflammatory based on the observation that patients with pouchitis and Crohn's disease-like have lower abundance of Dorea (23) Overall, the mechanisms through which these bacteria might induce inflammation and the factors which may influence this are not well-understood and beyond the scope of this review.
Phytoestrogens are compounds produced naturally in plant foods such as legumes, soybeans, beans, nuts, flax seeds, sesame seeds, hops, and other plants (Figure 1). They are known to have estrogenic/antiestrogenic, antioxidant, and anti-inflammatory effects, among others (24). It is important to highlight that the role of phytoestrogens in the cancer field has been studied extensively; however, their significance in inflammatory autoimmune diseases is less understood. Prevotella, Parabacteroides, and Adlercreutzia are known to metabolize phytoestrogens and produce secondary molecules such as equol, enterolactone, and secoisolariciresinol (Table 2). These bacteria can also metabolize fibers to produce SCFAs(as reviewed in Freedman et al. (62). Importance of the gut microbiota in MS has been studied extensively in its animal model experimental autoimmune encephalomyelitis (EAE). The suppression of EAE disease in GF mice on fecal transfer from HC and exacerbation of disease on fecal transfer from MS patients supports a critical role of gut microbiota in MS (8, 21). Gut bacteria have been shown to influence disease through modulation of multiple metabolic pathways such as short-chain fatty acid (63–65), tryptophan (62, 66), and phytoestrogen metabolism (66). As discussed before, we and others have reported the loss of bacteria involved in phytoestrogen metabolism (62). Through metabolism of phytoestrogens, these microbes may play an important role in the regulation of inflammation; thus, we hypothesize that reduced abundance of phytoestrogen metabolizing bacteria in the gut would influence the inflammation and demyelination in RRMS (4). Therefore, in this review we will focus on the importance of phytoestrogen metabolism by the gut microbiota, as well as on the effect of this phenomenon on the host physiology. We will discuss in detail: the mechanisms whereby gut bacteria metabolize phytoestrogens into structurally and functionally distinct metabolites; the ability of such metabolites to modulate various physiological processes, such as immune and neuronal/glial cell activity; and the ability of the metabolites to modulate disease in animal models of MS.
Figure 1. Phytoestrogens and their metabolites generated with the help of gut bacteria. Copyright © 2019 American Chemical Society. All Rights Reserved.
Phytoestrogens can be categorized, based on their structures, as flavonoids or nonflavonoids (Figure 1). Flavonoids are phenolic compounds with a basic structure consisting of 3 rings (denoted A, B, and C) comprised of 15 carbon atoms arranged in two aromatic rings connected by a 3-carbon bridge (67). These rings give them structural similarity to estradiol and the ability to mimic the function of estrogen. Further subclassification such as those discussed in this review (coumestans, prenylflavonoids, and isoflavones) are distinguished according to structural differences in the connection between the B and C rings, as well as the degrees of saturation, oxidation, and hydroxylation of the C ring [Figure 2; (67)]. Non-flavonoids consist of phenolic acids in either C6-C1 (benzoic acid) or C6-C3 (cinnamic acid) conformations, stilbenes, and lignans, the latter being the primary class implicated in microbiota-induced influence of human health (68).
Figure 2. Phytoestrogen structure and classification. Chemical Structures Copyright © 2019 American Chemical Society. All Rights Reserved.
The primary molecule studied in the coumestan family is coumestrol. In addition to the classical flavonoid structure, coumestrol has a furan ring in the junction between the C and B rings (69) and one hydroxyl group each at the C4 and C7 carbons, similar to the structure of estradiol (57). These moieties confer the ability to bind mammalian estrogen receptors (70) and provide free radical scavenging properties (69, 71), thus suggesting that coumestans may provide protection against breast, prostate, and ovarian cancers (72–74).
In vitro treatment of MCF7:WS8 (estrogen sensitive) and MCF7:5C (estrogen deprived) breast cancer cells with coumestrol had anti-proliferative and pro-apoptotic effects, respectively, which depended on estrogen receptor alpha (ERα) signaling (75). Similar observations were made in triple-negative breast cancer cells, but through the estrogen receptor (ER)-independent Bax/Bcl-2 pathway (76). Other coumestans have not been well-characterized in relation to their estrogenic activity. Furthermore, as coumestrol has an affinity for mammalian estrogen receptors reportedly only 10–20 times lower than 17β-estradiol (77), it is one of the more potent phytoestrogen compounds. Taken together, coumestrol and potentially novel coumestans or coumestan metabolites may prove to be an interesting target for study in neurological diseases such as MS.
Prenylflavonoids are characterized by prenylated side chains on the flavonoid backbone, generally at the 6, 8, 3, and/or 5 positions (78). Prenyl chains come in numerous forms, notably 3,3-dimethylallyl substituent, geranyl, 1,1-dimethylallyl, and is moieties (79). The most common prenylflavonoids in the human diet are xanthohumol and isoxanthohumol, both of which are found in hops, beer, and an increasing number of dietary supplements (80, 81). A systematic review of the prenylflavonoid literature in 2014 revealed an association of these molecules with a variety of biological effects (including cytotoxicity, particularly against tumor cells), as well as antibacterial effects (their primary function in the plant species in which they were originally identified) (79). Prenylflavonoids also modulate the functions of many enzymes; e.g., they inhibit the activities of cholinesterase and aldose reductase (AR), and they enhance those of alcohol- and aldehyde dehydrogenases (79). Like coumestans, they have also been shown to have anti-oxidant activity, including strong radical scavenging properties. Finally, xanthohumol metabolite 8-prenylnaringenin's (8PN) in vitro estrogenic activity was shown to be stronger than that of other phytoestrogens, including coumestrol, genistein, daidzein; as well as its precursors xanthohumol, isoxanthohumol, naringenin, and related compounds 6-prenylnaringenin, 6,8-diprenylnaringenin and 8-geranylnaringenin (82–85).
Numerous studies have described the processing of prenylflavonoid compounds, xanthohumol and isoxanthohumol, by human liver microsomes (81, 86–88). Nikolic et al. also characterized the processing of 8PN in human liver microsomes (80), which is thought to be more potent ligands of ER than original plant compounds. Recently, however, Eubacterium limosum was also shown to convert isoxanthohumol into 8PN and to demethylate some isoflavonoids (89). Furthermore, both the yeast species Pichia membranifaciens (ATCC 2254) and the fungal species Cunninghamella echinulata (NRRL 3655) were found to metabolize 8PN (90, 91). The additional metabolism by gut microbiota gives potential for more estrogenic compounds to be bioavailable. Thus, biological relevance of this metabolite may be greatly altered when considering the actions of commensal species, especially if prenylflavonoids follow similar patterns of absorption to other dietary polyphenols and 80–90% of consumed compound is potentially available to colonic bacteria (92). Furthermore, additional metabolites of 8PN produced with the help of gut bacteria, could exist and have various biological effects (80).
Although isoflavones are the best studied phytoestrogens, lignans are significantly more prominent in the Western diet (93–95). Dietary lignans are found at high levels in seeds such as flax and sesame seeds, and more broadly in cereals, fruits, and vegetables at moderate to high levels (58, 96). These non-flavonoid compounds are distinguished by a unique coupling of phenylpropanoid units at their 8' positions. The two major dietary lignans, secoisolariciresinol (or secoisolariciresinol-diglycoside) and matairesinol, give rise to the metabolites enterodiol and enterolactone. When first discovered, these metabolites were thought to originate from the ovaries, but further study in antibiotic-treated and germ-free rats indicated that intestinal bacteria are required for the production of both enterodiol and enterolactone (97). This was later confirmed in humans as well (98). The mechanism by which gut microbiota enzymes metabolize lignans is well-established (52, 99–101). From secoisolariciresinol-diglycoside, hydrolysis of the sugar moiety takes place first, followed by subsequent dehydroxylation, and demethylation to produce enterodiol (99, 102). Enterodiol can be further oxidized to enterolactone (99, 102). Similarly, matairesinol is dehydroxylated and demethylated to form enterolactone directly (102).
Both of these lignan metabolites have far greater biological effects than their precursors. The identification of both compounds during pregnancy and the cyclic pattern of excretion in females during menstrual cycle (established in both humans and monkeys) have physiological implications possibly related to interactions with ER, though whether these are estrogenic or antiestrogenic is unclear (103). Furthermore, both compounds are inhibitors of enzymes involved in steroid metabolism such as aromatase, 5 α-reductase, and 7β-hydroxysteroid dehydrogenase (103–106). Potential anticancer activity via antiestrogenic or antioxidant activity has been long proposed and well-studied (107–112). While parent compounds certainly have some physiological effects (113–117), the metabolism by microbiota serves to greatly alter and/or enhance the function of lignans.
Isoflavones are low molecular-weight compounds derived from plants with hydroxyl groups in the C4 and C7 positions, similar to coumestans discussed above, and estradiol (57). Isoflavones are among the most studied dietary phytoestrogens, and they are abundant in soybeans and soybean products, as well as in several other legumes (96, 118). Formononetin and biochanin A are processed, via either intestinal glucosidases (96) or enzymes in hepatic microsomes (118), to the more estrogenic compounds daidzein and genistein. Furthermore, daidzein, and genistein can be found directly in foods, generally in their glycoside forms bound to a sugar moiety. For gut absorption, isoflavone glycosides (e.g., daidzin and genistin), must be further processed into the aglycone form which lacks this sugar moiety (119, 120). Once hydrolyzed, they are readily absorbed and are detectable in plasma, urine, and feces (120). In rats, the aglycone forms of genistein and daidzein were detectable in plasma as soon as 3 min after an oral dose was administered (121). In a separate experiment in which glycoside forms of the same isoflavones were administered, detection was much slower because processing by intestinal β-glucosidases (expressed at highest levels in the duodenum) was required for absorption (121).
Isoflavones genistein, dihydrogenistein, and equol are proposed to bind ERβ with nearly the same, or slightly lower, affinity as 17β-estradiol, while affinity for ERα is generally weaker (122, 123). Isoflavones provide several benefits, including: antioxidant and antiangiogenic effects (57, 124–126); protection against breast cancer (127); and prevention of several menopause-related conditions (128–131). These effects are all thought to be mediated through the activation of ERα and/or ERβ, though in many cases the exact mechanisms are unknown.
The metabolism of daidzein to equol or O-desmethylangolensin (O-DMA) is entirely dependent on one or more bacterial strains in the gut, including but not limited to Adlercreutzia equolifaciens, Eggerthella sp., and Slackia isoflavoniconvertens (33, 132) isolated from humans, Asaccharobacter calatus and Enterorhabdus musicola identified in mice [Table 2; (133)]. These bacteria contain a specific set of enzymes, including daidzein reductase, dihydrodaidzein reductase, and tetrahydrodaidzein reductase required to metabolize daidzein into equol and/or O-desmethylangolensin (ODMA) (134). Biologically, it is S-equol (S-EQL) that is found in mammals and therefore has been the target of most research, whereas R-equol (R-EQL) could only be synthetically produced. However, more recently racemic mixture has been detected following synthesis by Lactococcus strain 20–92 and Eggerthella strain Julong 732 (135). The importance of O-DMA in human physiology is not well-understood and further research is needed to determine its significance to human health.
Gut microbes are thought to play an essential, non-redundant role in the metabolism of phytoestrogens in humans. This notion is supported by the fact that both GF mice on a soy-based diet and newborn infants up to 4 months of age (both of which lack diverse microbiota) lack equol (97, 136, 137). Additionally, culturing of human fecal matter from equol-producing individuals with soy or daidzein resulted in the formation of S-EQL (138, 139), and the inclusion of antibiotics in these cultures resulted in inhibition of equol production (139). Although the importance of intestinal bacteria in S-EQL production is well-established, the bacterial enzymes required and the microbes which contain them are slowly being characterized. Further research in this area and characterization of these bacteria in diseases vs. healthy states may provide important insight into mechanisms behind negative correlations observed with numerous diseases (e.g., obesity, breast cancer) in populations which consume high amounts of soy.
The majority of S-EQL is produced by conversion of daidzein, via enzymes derived from gut bacteria. However, daidzin, which is present in plant-based foods, must first be hydrolyzed into the bioactive aglycon form, daidzein. This hydrolysis step is catalyzed by β-glucosidase in the brush border membrane of the proximal intestine (140). As conjugated forms (glucosides) cannot cross intestinal epithelial cells, hydrolysis is a critical step in the formation of bioactive isoflavone metabolites. Three enzymes are required to metabolize daidzein into S-EQL and ODMA: daidzein reductase (DHNR), dihydrodaidzein reductase (DHDR), and tetrahydrodaidzein reductase (THDR) (46). Similar mechanisms might be involved in the digestion of other phytoestrogenic compounds and in the production of small metabolites.
The downstream effects of phytoestrogens are thought to be mediated, in part, through estrogen receptors, which are expressed widely, including in the cells of the immune and nervous systems (141, 142). Phytoestrogens and their metabolites can interact with the prototypic estrogen receptors, ERα and ERβ, effecting changes in cell physiology through modulation of transcription and gene expression (Table 3). Alternatively, phytoestrogen can also signal through the G-protein coupled estrogen receptor (GPER), which allows for more rapid and dynamic regulation of cell processes because the mechanisms are predominantly non-genomic (143–145). However, the majority of research in phytoestrogen signaling has focused on signaling through ER receptors and their ability to activate ER receptors compared to the natural ligand 17β- estradiol. Individual phytoestrogen metabolites have been proposed to have higher affinity for one ER over the other. For example, genistein and daidzein have significantly higher affinity for ERβ than for ERα (141). As ERα is the predominant estrogen receptor on the cells of the immune system (142) either the signaling pathways used by internal estrogens and phytoestrogens/phytoestrogen metabolites differ slightly; or ER-independent pathways such as GPER signaling might play a significant role in phytoestrogen-mediated modulation of immune cells. This may mean that greater shifts in phytoestrogen availability are needed to potentiate a change in signaling; however, additional research is needed to better understand the mechanisms of action of phytoestrogens and their metabolites in regard to receptor binding and the signaling pathways required for their biological activities, especially in the context of cells of immune and nervous systems.
After phytoestrogens are metabolized in the gut and transported to the liver, they may have systemic effects (52). With regard to the CNS, an organ system in which ERs are widely expressed, phytoestrogen metabolites have been found to have direct neuroprotective effects, based on both in vitro and in vivo studies (in animal models). Phytoestrogens can exert neuroprotective effects by attenuating toxic insults to neurons. For example, several studies showed that toxin-induced plasma-membrane damage was reduced in neurons treated in vitro with genistein and daidzein (146). In another study, such treatment resulted in neural-cell proliferation and improved cell viability (147). Furthermore, quercetin and kaempferol (phytoestrogen flavonoids) prevented neuronal cell death in the context of oxidative stress (148). Phytoestrogens can also exert neuroprotective effects by attenuating microglial mediated inflammatory responses; one study found that formononetin, daidzein, pratensein, calycosin, and irilone attenuated LPS-induced proinflammatory cytokine production by microglia (149).
Several in vivo studies have documented neuroprotective effects of phytoestrogens in the diet. The synaptic density was much greater in rats fed either a daidzein- plus genistein-based diet or a soy-enriched diet, compared to rats on either a standard chow or diet lacking soy (150). Furthermore, rats fed a diet containing soy-derived phytoestrogens exhibited improved learning and memory compared to rats on a control diet (151). Additionally, pretreatment with phytoestrogens protected mice from neurotoxicity in the CNS following 1-methyl-4-phenyl-1,2,3,6-tetrahydropyridine (MPTP)-induced Parkinson's Disease (PD) (152). These studies suggest that phytoestrogens might alter the structure and/or the function of both healthy and diseased neurons.
The mechanism underlying phytoestrogen-induced neuroprotective effects might be related to estrogen receptor agonistic activity. Estrogen replacement therapy (ERT) has been shown to improve CNS function, especially in Alzheimer's disease, by preventing oxidative stress and the formation of amyloid plaques (153). Indeed, genistein, daidzein, and zearalenone stimulate ERα- and ERβ-dependent transcription of genes that contain estrogen response elements (EREs) in their promoters (154). However, phytoestrogens have been shown to be 100–1,000 × less potent than 17β-estradiol, but they can also target GPR-30, a GPER on the plasma membrane of number of cells in a variety of tissues (155). However, further research is needed to determine the significance of the GPER receptor in phytoestrogen mediated signaling.
Phytoestrogens have varied effects on immune system function which have been summarized in Table 4. These effects are most often anti-inflammatory and protective in nature. In the case of the adaptive immune system, studies have shown that genistein and other isoflavones can suppress lymphocyte proliferation, allergic responses, and antigen-specific immune responses in both T- and B-cells (156, 158, 162–165). However, genistein has also been shown to enhance both the cytotoxic activity of CD8 T-cells, and the production of cytokines by T-cells more generally (156–161). Notably, this largely mirrors the effects of estrogens on these cells. One study by Kojima et al. showed that various phytoestrogens enhance gene expression mediated by retinoic-acid-receptor-related orphan receptor (ROR) γ and α in T-lymphoma cells, leading to increased expression of IL-17. Others have shown that when activated T-cells are treated with formononetin, daidzein, or equol, the levels of IL-4 expression increase (159). Collectively these studies suggest that phytoestrogens can interact with the T-cell compartment to induce various responses that may improve disease outcomes. Although the mechanisms leading to these actions are not well-understood, it has been suggested that either enhancement or inhibition of the NF-kB pathway could contribute, especially to cytokine responses.
Interactions of the B-cell compartment with phytoestrogens are less well-characterized, but limited studies have reported that phytoestrogens can induce an anti-inflammatory, anti-allergic phenotype that could be beneficial for the host. Specifically, multiple studies have shown that isoflavones and coumestrol can lower serum titers of immunoglobulin G2a (IgG2a) antibodies (162–165). One such study also showed that low-dose coumestrol can decrease the titers of antigen-specific IgG1 and IgG3 during experimental autoimmune thyroiditis (165). Further isoflavones can suppress the expression of IgE, possibly thereby contributing to the overall anti-allergic phenotype that has been reported in response to phytoestrogen treatment in several animal models, including but not limited to airway allergy and peanut-sensitization models (162).
Phytoestrogens have also been shown to modulate the innate immune system and the majority of studies suggest an anti-inflammatory role in this context. Combination of isoflavones genistein and daidzein alone, or these plus glycitein have been shown to inhibit the ability of dendritic cells (DCs) to induce the production of IFN-γ, TNF-α, IL-9, and IL-13 from CD4+ T-cells (162, 163). These phytoestrogens have also been shown to inhibit direct cytokine secretion from activated DCs (163). Phytoestrogens also suppress DC maturation and the expression of MHCI, but not MHCII, in an intra-nasal allergic response model. These data suggest that phytoestrogens might slow the inflammatory immune response by inhibiting the antigen-presentation and effector-cell priming functions of DCs (162, 163). Genistein and daidzein, in particular, can suppress allergic inflammation by significantly reducing (by 25–30%) mast cell degranulation (162, 164). However, treatment of activated DCs with genistein or daidzein led to increased NK-cell degranulation and cytotoxicity, outcomes that have not been studied in a disease (163). Phytoestrogens can also modulate NK cell activity by specifically reducing expression of IL-18 receptor α (IL-18Rα), and inhibiting IFN-γ production in response to IL-12 and IL-18 (167). These actions of phytoestrogens have not been found to reduce NK cell cytotoxicity (156, 157, 163, 166). Thus, it remains unclear why phytoestrogens might have anti-inflammatory functions in DC populations, but potentially mixed effects in NK cell populations.
In macrophages, phytoestrogens have been shown to induce overall anti-inflammatory responses. Dia et al. showed that genistein and daidzein can decrease the production of nitric oxide and the expression of iNOS (inducible nitric oxide synthase), as well as inducing the activities of super oxide dismutase and catalase (168). Another study found that genistein treatment can skew macrophage polarization toward an M2, anti-inflammatory phenotype, while also reducing systemic concentrations of inflammatory cytokines (161). The same study also found that macrophages induced to take on the M2 phenotype when treated with genistein express ARG-1 and IL-10 at higher levels than those induced to take on this phenotype by other agents (161). Thus, genistein appears to push macrophages toward an actively anti-inflammatory phenotype, i.e., its actions are not solely non-inflammatory. The collective activities of the phytoestrogens in regard to the innate immune compartment may explain some of the systemic anti-inflammatory effects of phytoestrogens that have been described in the literature (e.g., decreased allergic responses and decreased autoreactive immune responses).
EAE is a very well-studied model of MS in which myelin antigen in combination with pertussis toxin and complete Freud's adjuvant is used to induce an autoimmune response. It is characterized by spinal cord pathology, manifesting in ascending paralysis that can be scored on a standard 6 point scale (0–5) (170). Several groups have documented the therapeutic and disease-preventative potential of phytoestrogens using the murine EAE model of MS. These studies indicate that common phytoestrogens, especially isoflavones genistein and daidzein, have potential as therapeutics for autoimmunity affecting CNS components. One group showed that sub-cutaneous (s.c.) treatment with genistein post-EAE induction resulted in significantly ameliorated EAE (171). The genistein treatment group showed reduced production of pro-inflammatory cytokines (including TNFα, IFNγ, and IL-12p40) by splenocytes and/or CNS lymphocytes (171). Similarly, 7-O-tetradecanoyl (TDG), a lipophilic genistein analog, suppressed disease when administered s.c. 14-days post-EAE induction. In these studies, disease amelioration correlated with a decrease in the number of IL-17 producing CD4+ T cells, and an increase in the number of FoxP3+CD4+ T cells, in the brain (172). Another study reported that daily oral treatment with high-dose daidzein starting 10 days after EAE induction ameliorated disease and simultaneously reduced IFNγ levels in the brain and splenocytes compared to controls with induced disease (173). These studies suggest that phytoestrogenic compounds or their analogs might have therapeutic potential in an animal model of MS.
Besides isoflavones, other phytoestrogens have also been shown to exert a protective effect in EAE. Wei et al. showed that therapeutic oral administration of high-dose Icariin (ICA), a phytoestrogen from flowering plants of the Epimedium genus, ameliorated EAE (comparison was to vehicle control). The effectiveness of ICA was similar to that of estrogen, and high-dose ICA or estrogen treatment increased expression of both ERα and ERβ in the white matter of the CNS (174). In a separate study, these researchers reported that therapeutic oral administration of high-dose ICA in combination with methylprednisolone (MP), a corticosteroid used therapeutically in MS, had a greater disease-ameliorating effect than either treatment alone. This combination treatment (ICA+MP) also had a synergistic effect, enhancing both the reduction of serum IL-17 and apoptotic cell death (Annexin V+ cells) in the spinal cord (175). Taken together, these studies show the therapeutic potential of phytoestrogen compounds, both alone and in combination therapies, as a promising complementary and alternative therapy for further study.
Quercetin is a phytoestrogen flavonoid that is abundant in soybeans, vegetables, and fruits and has also been evaluated for its efficacy in the EAE model. Quercetin protects against EAE when injected intra-peritoneally (i.p.; comparison was to a vehicle control). Additionally, quercetin caused a dose dependent suppression of antigen specific proliferation and IL-12 in splenocytes in ex-vivo antigen-recall response (176). Thus, quercetin might be able to influence encephalitogenic T cells directly. Resveratrol, a phytoestrogen found in the skins of red grapes and berries, had been shown to ameliorate EAE by interfering with the miR-124/sphingosine kinase 1 (SK1) axis in encephalitogenic T cells, thereby resulting in cell-cycle arrest and apoptosis (177). These studies clearly indicate that phytoestrogen compounds protect against EAE and may have implications/therapeutic potential in MS as well.
However, several knowledge gaps remain to be addressed. For example, most of the studies described above introduced phytoestrogen compounds via a non-physiological route (s.c. or i.p.). Given that humans obtain phytoestrogens through diet, study of the effectiveness of oral delivery or consumption of these compounds would better reflect the mechanisms involved in a more physiological context. Furthermore, the studies using s.c. or i.p. routes of administration do not account for the importance of phytoestrogen-metabolizing gut bacteria, which humans rely on for proper breakdown of dietary phytoestrogens. This may explain why the studies that did provide oral phytoestrogens required a very high dose for protection.
Although the exact mechanism through which phytoestrogenic compounds suppress EAE is unknown, studies have suggested that their neuroprotective and immunomodulatory effects might play an important role in their ability to suppress disease as described above.
In RRMS patients, the presence of gut dysbiosis and the depletion of bacteria with the ability to metabolize phytoestrogens highlights the importance of these compounds in maintaining a disease free-state of the host. As stated above, various phytoestrogen metabolites play important roles in a number of biological processes including neuroprotection and regulation of the immune system. However, further research is certainly needed to better understand the pathways through which gut bacteria induced phytoestrogens metabolites regulate the balance between pro- and anti-inflammatory responses and provide neuroprotection. For example, future studies determining the relationship between levels of phytoestrogen metabolites and the severity of RRMS disease are expected to shed light on the extent to which phytoestrogen metabolism correlates with the etiopathogenesis of RRMS. Also, dissection of the role of phytoestrogen metabolism in the development and regulation of the immune system in germ-free mice is expected to reveal the significance of specific phytoestrogen metabolites in regulating the function of various immune subsets. In the meantime, however, the existing literature provides a solid rationale for the selection and testing of the therapeutic potential of various phytoestrogen metabolizing bacteria, including Prevotella, Parabacteroides, and Adlercreutzia in a preclinical model of MS. A successful outcome from these studies will help in development of bacteria as drug (BRUG) based treatment options for MS patients.
AM conceptualized the review, helped with overall structure of the manuscript, and gave final approval of the manuscript to be published. NC wrote the phytoestrogen metabolism section of the manuscript. SF wrote role of phytoestrogen in EAE and nervous system section. SP helped with writing the section on phytoestrogen receptors and effect of phytoestrogen on immune cells. All authors commented on the manuscript.
The authors acknowledge funding from the National Institute of Health/NIAID (1R01AI137075-01), The University of Iowa Environmental Health Sciences Research Center, NIEHS/NIH (P30 ES005605). SP and SF were supported on an institutional training grant (T32AI007485 to Dr. Gail Bishop).
AM is one of the inventor of a technology claiming the use of Prevotella histicola for the treatment of autoimmune diseases. The patent for the technology is owned by Mayo Clinic, who has given exclusive license to Evelo Biosciences. AM received royalties from Mayo Clinic (paid by Evelo Biosciences).
The remaining authors declare that the research was conducted in the absence of any commercial or financial relationships that could be construed as a potential conflict of interest.
1. Campbell JD, Ghushchyan V, Brett McQueen R, Cahoon-Metzger S, Livingston T, Vollmer T, et al. Burden of multiple sclerosis on direct, indirect costs and quality of life: national US estimates. Mult Scler Relat Disord. (2014) 3:227–36. doi: 10.1016/j.msard.2013.09.004
2. Oksenberg JR, Barcellos LF. The complex genetic aetiology of multiple sclerosis. J Neurovirol. (2000) 6(Suppl. 2):S10–4.
3. Miyake S, Kim S, Suda W, Oshima K, Nakamura M, Matsuoka T, et al. Dysbiosis in the gut microbiota of patients with multiple sclerosis, with a striking depletion of species belonging to clostridia XIVA and IV clusters. PLoS ONE. (2015) 10:e0137429. doi: 10.1371/journal.pone.0137429
4. Chen J, Chia N, Kalari KR, Yao JZ, Novotna M, Soldan MM, et al. Multiple sclerosis patients have a distinct gut microbiota compared to healthy controls. Sci Rep. (2016) 6:28484. doi: 10.1038/srep28484
5. Jangi S, Gandhi R, Cox LM, Li N, von Glehn F, Yan R, et al. Alterations of the human gut microbiome in multiple sclerosis. Nat Commun. (2016) 7:12015. doi: 10.1038/ncomms12015
6. Castillo Álvarez F, Pérez Matute P, Colina Lizuain S, Erdocia Goñi A, Iglesias Gutiérrez Cecchini C, Gómez Eguilaz M, et al. Intestinal Microbiota in Multiple Sclerosis: Influence of Treatment With Interferon β-1b. London: European Committee for Treatment and Research in Multiple Sclerosis (ECTRIMS), ECTRIMS Online Library (2016). p. 146290.
7. Cosorich I, Dalla-Costa G, Sorini C, Ferrarese R, Messina MJ, Dolpady J, et al. High frequency of intestinal TH17 cells correlates with microbiota alterations and disease activity in multiple sclerosis. Sci Adv. (2017) 3:e1700492. doi: 10.1126/sciadv.1700492
8. Cekanaviciute E, Yoo BB, Runia TF, Debelius JW, Singh S, Nelson CA, et al. Gut bacteria from multiple sclerosis patients modulate human T cells and exacerbate symptoms in mouse models. Proc Natl Acad Sci USA. (2017) 114:10713–8. doi: 10.1073/pnas.1711235114
9. Human Microbiome Project Consortium. Structure, function and diversity of the healthy human microbiome. Nature. (2012) 486:207–14. doi: 10.1038/nature11234
10. Yatsunenko T, Rey FE, Manary MJ, Trehan I, Dominguez-Bello MG, Contreras M, et al. Human gut microbiome viewed across age and geography. Nature. (2012) 486:222–7. doi: 10.1038/nature11053
11. Rampelli S, Schnorr SL, Consolandi C, Turroni S, Severgnini M, Peano C, et al. Metagenome sequencing of the hadza hunter-gatherer gut microbiota. Curr Biol. (2015) 25:1682–93. doi: 10.1016/j.cub.2015.04.055
12. Koeth RA, Lam-Galvez BR, Kirsop J, Wang Z, Levison BS, Gu X, et al. l-Carnitine in omnivorous diets induces an atherogenic gut microbial pathway in humans. J Clin Invest. (2019) 129:373–87. doi: 10.1172/JCI94601
13. Zinocker MK, Lindseth IA. The western diet-microbiome-host interaction and its role in metabolic disease. Nutrients. (2018) 10:365. doi: 10.20944/preprints201803.0064.v1
14. Sender R, Fuchs S, Milo R. Are we really vastly outnumbered? Revisiting the ratio of bacterial to host cells in humans. Cell. (2016) 164:337–40. doi: 10.1016/j.cell.2016.01.013
15. Colpitts SL, Kasper LH. Influence of the gut microbiome on autoimmunity in the central nervous system. J Immunol. (2017) 198:596–604. doi: 10.4049/jimmunol.1601438
16. Rothhammer V, Quintana FJ. Environmental control of autoimmune inflammation in the central nervous system. Curr Opin Immunol. (2016) 43:46–53. doi: 10.1016/j.coi.2016.09.002
17. Wu HJ, Wu E. The role of gut microbiota in immune homeostasis and autoimmunity. Gut Microb. (2012) 3:4–14. doi: 10.4161/gmic.19320
18. Chung H, Kasper DL. Microbiota-stimulated immune mechanisms to maintain gut homeostasis. Curr Opin Immunol. (2010) 22:455–60. doi: 10.1016/j.coi.2010.06.008
19. Shahi SK, Freedman SN, Mangalam AK. Gut microbiome in multiple sclerosis: the players involved and the roles they play. Gut Microb. (2017) 8:607–15. doi: 10.1080/19490976.2017.1349041
20. Castillo-Alvarez F, Perez-Matute P, Oteo JA, Marzo-Sola ME. The influence of interferon beta-1b on gut microbiota composition in patients with multiple sclerosis. Neurologia. (2018). 9:S0213–4853. doi: 10.1016/j.nrl.2018.04.006
21. Berer K, Gerdes LA, Cekanaviciute E, Jia X, Xiao L, Xia Z, et al. Gut microbiota from multiple sclerosis patients enables spontaneous autoimmune encephalomyelitis in mice. Proc Natl Acad Sci USA. (2017) 114:10719–24. doi: 10.1073/pnas.1711233114
22. Everard A, Belzer C, Geurts L, Ouwerkerk JP, Druart C, Bindels LB, et al. Cross-talk between Akkermansia muciniphila and intestinal epithelium controls diet-induced obesity. Proc Natl Acad Sci USA. (2013) 110:9066–71. doi: 10.1073/pnas.1219451110
23. Tyler AD, Knox N, Kabakchiev B, Milgrom R, Kirsch R, Cohen Z, et al. Characterization of the gut-associated microbiome in inflammatory pouch complications following ileal pouch-anal anastomosis. PLoS ONE. (2013) 8:e66934. doi: 10.1371/journal.pone.0066934
24. Peiroten A, Bravo D, Landete JM. Bacterial metabolism as responsible of beneficial effects of phytoestrogens on human health. Crit Rev Food Sci Nutr. (2019) 4:1–16. doi: 10.1080/10408398.2019.1622505
25. Hur H, Rafii F. Biotransformation of the isoflavonoids biochanin A, formononetin, glycitein by eubacterium limosum. FEMS Microbiol Lett. (2000) 192:21–5. doi: 10.1111/j.1574-6968.2000.tb09353.x
26. Hur HG, Lay JO Jr., Beger RD, Freeman JP, Rafii F. Isolation of human intestinal bacteria metabolizing the natural isoflavone glycosides daidzin and genistin. Arch Microbiol. (2000) 174:422–8. doi: 10.1007/s002030000222
27. Tsangalis D, Ashton JF, McGill AEJ, Shah NP. Enzymic transformation of isoflavone phytoestrogens in soymilk by β-glucosidase-producing bifidobacteria. J Food Sci. (2002) 67:3104–13. doi: 10.1111/j.1365-2621.2002.tb08866.x
28. Minamida K, Ota K, Nishimukai M, Tanaka M, Abe A, Sone T, et al. Asaccharobacter celatus gen. nov., sp. nov., isolated from rat caecum. Int J Syst Evolution Microbiol. (2008) 58:1238–40. doi: 10.1099/ijs.0.64894-0
29. Park HY, Kim M, Han J. Stereospecific microbial production of isoflavanones from isoflavones and isoflavone glucosides. Appl Microbiol Biotechnol. (2011) 91:1173–81. doi: 10.1007/s00253-011-3310-7
30. Shimada Y, Yasuda S, Takahashi M, Hayashi T, Miyazawa N, Sato I, et al. Cloning and expression of a novel NADP(H)-dependent daidzein reductase, an enzyme involved in the metabolism of daidzein, from equol-producing lactococcus strain 20–92. Appl Environ Microbiol. (2010) 76:5892–901. doi: 10.1128/AEM.01101-10
31. Hur HG, Beger RD, Heinze TM, Lay JO Jr., Freeman JP, Dore J, et al. Isolation of an anaerobic intestinal bacterium capable of cleaving the C-ring of the isoflavonoid daidzein. Arch Microbiol. (2002) 178:8–12. doi: 10.1007/s00203-002-0437-z
32. Tamura M, Tsushida T, Shinohara K. Isolation of an isoflavone-metabolizing, clostridium-like bacterium, strain TM-40, from human faeces. Anaerobe. (2007) 13:32–5. doi: 10.1016/j.anaerobe.2006.10.001
33. Maruo T, Sakamoto M, Ito C, Toda T, Benno Y. Adlercreutzia equolifaciens gen. nov., sp. nov., an equol-producing bacterium isolated from human faeces, emended description of the genus eggerthella. Int J Syst Evolution Microbiol. (2008) 58:1221–7. doi: 10.1099/ijs.0.65404-0
34. Minamida K, Tanaka M, Abe A, Sone T, Tomita F, Hara H, et al. Production of equol from daidzein by gram-positive rod-shaped bacterium isolated from rat intestine. J Biosci Bioeng. (2006) 102:247–50. doi: 10.1263/jbb.102.247
35. Tsuchihashi R, Sakamoto S, Kodera M, Nohara T, Kinjo J. Microbial metabolism of soy isoflavones by human intestinal bacterial strains. J Nat Med. (2008) 62:456–60. doi: 10.1007/s11418-008-0271-y
36. Ueno TUS. Identification of the specific intestinal bacteria capable of metabolising soy isoflavone to equol. Ann Nutr Metab. (2002) 114.
37. Raimondi S, Roncaglia L, De Lucia M, Amaretti A, Leonardi A, Pagnoni UM, et al. Bioconversion of soy isoflavones daidzin and daidzein by bifidobacterium strains. Appl Microbiol Biotechnol. (2009) 81:943–50. doi: 10.1007/s00253-008-1719-4
38. Matthies A, Clavel T, Gutschow M, Engst W, Haller D, Blaut M, et al. Conversion of daidzein and genistein by an anaerobic bacterium newly isolated from the mouse intestine. Appl Environ Microbiol. (2008) 74:4847–52. doi: 10.1128/AEM.00555-08
39. Yokoyama S, Suzuki T. Isolation and characterization of a novel equol-producing bacterium from human feces. Biosci Biotechnol Biochem. (2008) 72:2660–6. doi: 10.1271/bbb.80329
40. Decroos K, Vanhemmens S, Cattoir S, Boon N, Verstraete W. Isolation and characterisation of an equol-producing mixed microbial culture from a human faecal sample and its activity under gastrointestinal conditions. Arch Microbiol. (2005) 183:45–55. doi: 10.1007/s00203-004-0747-4
41. Wang XL, Hur HG, Lee JH, Kim KT, Kim SI. Enantioselective synthesis of S-equol from dihydrodaidzein by a newly isolated anaerobic human intestinal bacterium. Appl Environ Microbiol. (2005) 71:214–9. doi: 10.1128/AEM.71.1.214-219.2005
42. Wang XL, Kim HJ, Kang SI, Kim SI, Hur HG. Production of phytoestrogen S-equol from daidzein in mixed culture of two anaerobic bacteria. Arch Microbiol. (2007) 187:155–60. doi: 10.1007/s00203-006-0183-8
43. Tamura M, Hori S, Nakagawa H. Lactobacillus rhamnosus JCM 2771: impact on metabolism of isoflavonoids in the fecal flora from a male equol producer. Curr Microbiol. (2011) 62:1632–7. doi: 10.1007/s00284-011-9904-6
44. Blair RM, Appt SE, Franke AA, Clarkson TB. Treatment with antibiotics reduces plasma equol concentration in cynomolgus monkeys (Macaca fascicularis). J Nutr. (2003) 133:2262–7. doi: 10.1093/jn/133.7.2262
45. Jin JS, Nishihata T, Kakiuchi N, Hattori M. Biotransformation of C-glucosylisoflavone puerarin to estrogenic. (3S)-equol in co-culture of two human intestinal bacteria. Biol Pharm Bull. (2008) 31:1621–5. doi: 10.1248/bpb.31.1621
46. Matthies A, Blaut M, Braune A. Isolation of a human intestinal bacterium capable of daidzein and genistein conversion. Appl Environ Microbiol. (2009) 75:1740–4. doi: 10.1128/AEM.01795-08
47. Tsuji H, Moriyama K, Nomoto K, Miyanaga N, Akaza H. Isolation and characterization of the equol-producing bacterium Slackia sp. strain NATTS. Arch Microbiol. (2010) 192:279–87. doi: 10.1007/s00203-010-0546-z
48. Schoefer L, Mohan R, Braune A, Birringer M, Blaut M. Anaerobic C-ring cleavage of genistein and daidzein by Eubacterium ramulus. FEMS Microbiol Lett. (2002) 208:197–202. doi: 10.1111/j.1574-6968.2002.tb11081.x
49. Clavel T, Henderson G, Engst W, Dore J, Blaut M. Phylogeny of human intestinal bacteria that activate the dietary lignan secoisolariciresinol diglucoside. FEMS Microbiol Ecol. (2006) 55:471–8. doi: 10.1111/j.1574-6941.2005.00057.x
50. Roncaglia L, Amaretti A, Raimondi S, Leonardi A, Rossi M. Role of bifidobacteria in the activation of the lignan secoisolariciresinol diglucoside. Appl Microbiol Biotechnol. (2011) 92:159–68. doi: 10.1007/s00253-011-3338-8
51. Schogor AL, Huws SA, Santos GT, Scollan ND, Hauck BD, Winters AL, et al. Ruminal Prevotella spp. may play an important role in the conversion of plant lignans into human health beneficial antioxidants. PLoS ONE. (2014) 9:e87949. doi: 10.1371/journal.pone.0087949
52. Clavel T, Borrmann D, Braune A, Dore J, Blaut M. Occurrence and activity of human intestinal bacteria involved in the conversion of dietary lignans. Anaerobe. (2006) 12:140–7. doi: 10.1016/j.anaerobe.2005.11.002
53. Jin JS, Hattori M. Human intestinal bacterium, strain END-2 is responsible for demethylation as well as lactonization during plant lignan metabolism. Biol Pharm Bull. (2010) 33:1443–7. doi: 10.1248/bpb.33.1443
54. Jin JS, Zhao YF, Nakamura N, Akao T, Kakiuchi N, Min BS, Hattori M. Enantioselective dehydroxylation of enterodiol and enterolactone precursors by human intestinal bacteria. Biol Pharm Bull. (2007) 30:2113–9. doi: 10.1248/bpb.30.2113
55. Xie LH, Akao T, Hamasaki K, Deyama T, Hattori M. Biotransformation of pinoresinol diglucoside to mammalian lignans by human intestinal microflora, isolation of Enterococcus faecalis strain PDG-1 responsible for the transformation of (+)-pinoresinol to. (+)-lariciresinol. Chem Pharm Bull. (2003) 51:508–15. doi: 10.1248/cpb.51.508
56. Possemiers S, Heyerick A, Robbens V, De Keukeleire D, Verstraete W. Activation of proestrogens from hops (Humulus lupulus L.) by Intestinal microbiota; conversion of isoxanthohumol into 8-prenylnaringenin. J Agri Food Chem. (2005) 53:6281–8. doi: 10.1021/jf0509714
57. Lopes D, Dias de Queiros L, Ávila A, Monteiro N, Macedo G. The importance of microbial and enzymatic bioconversions of isoflavones in bioactive compounds. In: Grumezescu AM, Holban AM, editors. Food Bioconversion. London: Academic Press (2017). p. 55–93.
58. Yoder SC, Lancaster SM, Hullar MAJ, Lampe JW. Gut microbial metabolism of plant lignans: influence on human health. In: Tuohy K, Del Rio D, editors. Diet-Microbe Interactions in the Gut, San Diego, CA: Academic Press (2015). p. 103–117.
59. Sánchez-Calvo JM, Rodríguez-Iglesias MA, Molinillo JMG, Macías FA. Soy isoflavones and their relationship with microflora: beneficial effects on human health in equol producers. Phytochem Rev. (2013) 12:979–1000. doi: 10.1007/s11101-013-9329-x
60. Setchell KDR, Clerici C. Equol: history, chemistry, formation. J Nutr. (2010) 140:1355S−62. doi: 10.3945/jn.109.119776
61. Rafii F. The role of colonic bacteria in the metabolism of the natural isoflavone daidzin to equol. Metabolites. (2015) 5:56–73. doi: 10.3390/metabo5010056
62. Freedman SN, Shahi SK, Mangalam AK. The gut feeling: breaking down the role of gut microbiome in multiple sclerosis. Neurotherapeutics. (2018) 15:109–25. doi: 10.1007/s13311-017-0588-x
63. Fan Y, Zhang J. Dietary modulation of intestinal microbiota: future opportunities in experimental autoimmune encephalomyelitis and multiple sclerosis. Front Microbiol. (2019) 10:740. doi: 10.3389/fmicb.2019.00740
64. Haghikia A, Jorg S, Duscha A, Berg J, Manzel A, Waschbisch A, et al. Dietary fatty acids directly impact central nervous system autoimmunity via the small intestine. Immunity. (2015) 43:817–29. doi: 10.1016/j.immuni.2015.09.007
65. Melbye P, Olsson A, Hansen TH, Sondergaard HB, Bang Oturai A. Short-chain fatty acids and gut microbiota in multiple sclerosis. Acta Neurol Scand. (2019) 139:208–19. doi: 10.1111/ane.13045
66. Rothhammer V, Mascanfroni ID, Bunse L, Takenaka MC, Kenison JE, Mayo L, et al. Type I interferons and microbial metabolites of tryptophan modulate astrocyte activity and central nervous system inflammation via the aryl hydrocarbon receptor. Nat Med. (2016) 22:586–97. doi: 10.1038/nm.4106
67. Crozier A, Jaganath IB, Clifford MN. Dietary phenolics: chemistry, bioavailability and effects on health. Nat Prod Rep. (2009) 26:1001–43. doi: 10.1039/b802662a
68. Moreno J, Peinado R. Polyphenols. In: Moreno J, Peinado R, editors. Enological Chemistry, San Diego, CA: Academic Press (2012). p. 53–76.
69. Chandsawangbhuwana C, Baker ME. 3D models of human ERα and ERβ complexed with coumestrol. Steroids. (2014) 80:37–43. doi: 10.1016/j.steroids.2013.11.019
70. Montero G, Arriagada F, Günther G, Bollo S, Mura F, Berríos E, et al. Phytoestrogen coumestrol: antioxidant capacity and its loading in albumin nanoparticles. Int J Pharm. (2019) 562:86–95. doi: 10.1016/j.ijpharm.2019.03.029
71. Xi G-L, Liu Z-Q. Coumarin-fused coumarin: antioxidant story from N,N-dimethylamino and hydroxyl groups. J Agri Food Chem. (2015) 63:3516–23. doi: 10.1021/acs.jafc.5b00399
72. Dixon-Shanies D, Shaikh N. Growth inhibition of human breast cancer cells by herbs and phytoestrogens. Oncol Rep. (1999) 6:1383–7. doi: 10.3892/or.6.6.1383
73. Lim W, Jeong W, Song G. Coumestrol suppresses proliferation of ES2 human epithelial ovarian cancer cells. J Endocrinol. (2016) 228:149–60. doi: 10.1530/JOE-15-0418
74. Markaverich BM, Webb B, Densmore CL, Gregory RR. Effects of coumestrol on estrogen receptor function and uterine growth in ovariectomized rats. Environ Health Perspect. (1995) 103:574–81. doi: 10.1289/ehp.95103574
75. Obiorah IE, Fan P, Jordan VC. Breast cancer cell apoptosis with phytoestrogens is dependent on an estrogen-deprived state. Cancer Prevent Res. (2014) 7:939–49. doi: 10.1158/1940-6207.CAPR-14-0061
76. Zafar A, Singh S, Satija YK, Saluja D, Naseem I. Deciphering the molecular mechanism underlying anticancer activity of coumestrol in triple-negative breast cancer cells. Toxicol In Vitro. (2018) 46:19–28. doi: 10.1016/j.tiv.2017.10.007
77. Verdeal K, Ryan DS. Naturally-occurring estrogens in plant foodstuffs - a review. J Food Protect. (1979) 42:577–83. doi: 10.4315/0362-028X-42.7.577
78. Ibrahim R. A forty-year journey in plant research: original contributions to flavonoid biochemistry. Can J Bot. (2005) 83:433–50. doi: 10.1139/b05-030
79. Chen X, Mukwaya E, Wong M-S, Zhang Y. A systematic review on biological activities of prenylated flavonoids. Pharm Biol. (2014) 52:655–60. doi: 10.3109/13880209.2013.853809
80. Nikolic D, Li Y, Chadwick LR, Grubjesic S, Schwab P, Metz P, et al. Metabolism of 8-prenylnaringenin, a potent phytoestrogen from hops (Humulus lupulus), by human liver microsomes. Drug Metab Dispos. (2004) 32:272–9. doi: 10.1124/dmd.32.2.272
81. Nikolic D, Li Y, Chadwick LR, Pauli GF, van Breemen RB. Metabolism of xanthohumol and isoxanthohumol, prenylated flavonoids from hops (Humulus lupulus L.), by human liver microsomes. J Mass Spectrom. (2005) 40:289–99. doi: 10.1002/jms.753
82. Milligan SR, Kalita JC, Heyerick A, Rong H, De Cooman L, De Keukeleire D. Identification of a potent phytoestrogen in hops (Humulus lupulus L.) and beer. J Clin Endocrinol Metab. (1999) 84:2249–52. doi: 10.1210/jcem.84.6.5887
83. Milligan SR, Kalita JC, Pocock V, Van De Kauter V, Stevens JF, Deinzer ML, et al. The endocrine activities of 8-prenylnaringenin and related hop (Humulus lupulus L.) flavonoids. J Clin Endocrinol Metab. (2000) 85:4912–5. doi: 10.1210/jcem.85.12.7168
84. Milligan S, Kalita J, Pocock V, Heyerick A, De Cooman L, Rong H, et al. Oestrogenic activity of the hop phyto-oestrogen, 8-prenylnaringenin. Reproduction. (2002) 123:235–42. doi: 10.1530/rep.0.1230235
85. Zierau O, Gester S, Schwab P, Metz P, Kolba S, Wulf M, et al. Estrogenic activity of the phytoestrogens naringenin, 6-(1,1-dimethylallyl)naringenin and 8-prenylnaringenin. Planta Med. (2002) 68:449–51. doi: 10.1055/s-2002-32089
86. Yilmazer M, Stevens JF, Deinzer ML, Buhler DR. In vitro biotransformation of xanthohumol, a flavonoid from hops (Humulus lupulus), by rat liver microsomes. Drug Metab Dispos. (2001) 29:223–31.
87. Yilmazer M, Stevens JF, Buhler DR. In vitro glucuronidation of xanthohumol, a flavonoid in hop and beer, by rat and human liver microsomes. FEBS Lett. (2001) 491:252–6. doi: 10.1016/S0014-5793(01)02210-4
88. Stevens JF, Taylor AW, Nickerson GB, Ivancic M, Henning J, Haunold A, et al. Prenylflavonoid variation in Humulus lupulus: distribution and taxonomic significance of xanthogalenol and 4′-O-methylxanthohumol. Phytochemistry. (2000) 53:759–75. doi: 10.1016/S0031-9422(00)00005-4
89. Possemiers S, Bolca S, Grootaert C, Heyerick A, Decroos K, Dhooge W, et al. The prenylflavonoid isoxanthohumol from hops (Humulus lupulus L.) is activated into the potent phytoestrogen 8-prenylnaringenin in vitro and in the human intestine. J Nutr. (2006) 136:1862–7. doi: 10.1093/jn/136.7.1862
90. Herath W, Ferreira D, Khan SI, Khan IA. Identification and biological activity of microbial metabolites of xanthohumol. Chem Pharm Bull. (2003) 51:1237–40. doi: 10.1248/cpb.51.1237
91. Herath WHMW, Ferreira D, Khan IA. Microbial transformation of xanthohumol. Phytochemistry. (2003) 62:673–7. doi: 10.1016/S0031-9422(02)00615-5
92. Kuhnle G, Spencer JP, Chowrimootoo G, Schroeter H, Debnam ES, Srai SK, et al. Resveratrol is absorbed in the small intestine as resveratrol glucuronide. Biochem Biophys Res Commun. (2000) 272:212–7. doi: 10.1006/bbrc.2000.2750
93. Boker LK, Van der Schouw YT, De Kleijn MJ, Jacques PF, Grobbee DE, Peeters PH. Intake of dietary phytoestrogens by Dutch women. J Nutr. (2002) 132:1319–28. doi: 10.1093/jn/132.6.1319
94. de Kleijn MJ, van der Schouw YT, Wilson PW, Grobbee DE, Jacques PF. Dietary intake of phytoestrogens is associated with a favorable metabolic cardiovascular risk profile in postmenopausal U.S.women: the framingham study. J Nutr. (2002) 132:276–82. doi: 10.1093/jn/132.2.276
95. Valsta LM, Kilkkinen A, Mazur W, Nurmi T, Lampi AM, Ovaskainen ML, et al. Phyto-oestrogen database of foods and average intake in Finland. Br J Nutr. (2003) 89(Suppl. 1):S31–8. doi: 10.1079/BJN2002794
96. Murkies AL, Wilcox G, Davis SR. Phytoestrogens1. J Clin Endocrinol Metab. (1998) 83:297–303. doi: 10.1210/jcem.83.2.4577
97. Axelson M, Setchell KD. The excretion of lignans in rats – evidence for an intestinal bacterial source for this new group of compounds. FEBS Lett. (1981) 123:337–42. doi: 10.1016/0014-5793(81)80322-5
98. Setchell KDR, Borriello SP, Gordon H, Lawson AM, Harkness R, Morgan DM, et al. Lignan formation in man–microbial involvement and possible roles in relation to cancer. Lancet. (1981) 318:4–7. doi: 10.1016/S0140-6736(81)90250-6
99. Axelson M, Sjövall J, Gustafsson BE, Setchell KDR. Origin of lignans in mammals and identification of a precursor from plants. Nature. (1982) 298:659–60. doi: 10.1038/298659a0
100. Borriello SP, Setchell KD, Axelson M, Lawson AM. Production and metabolism of lignans by the human faecal flora. J Appl Bacteriol. (1985) 58:37–43. doi: 10.1111/j.1365-2672.1985.tb01427.x
101. Setchell KD, Brown NM, Zimmer-Nechemias L, Wolfe B, Jha P, Heubi JE. Metabolism of secoisolariciresinol-diglycoside the dietary precursor to the intestinally derived lignan enterolactone in humans. Food Funct. (2014) 5:491–501. doi: 10.1039/C3FO60402K
102. Kurzer MS, Xu X. Dietary Phytoestrogens. Annu Rev Nutr. (1997) 17:353–81. doi: 10.1146/annurev.nutr.17.1.353
103. Wang L-Q, Mammalian phytoestrogens: enterodiol and enterolactone. J Chromatogr B. (2002) 777:289–309. doi: 10.1016/S1570-0232(02)00281-7
104. Adlercreutz H, Bannwart C, Wahala K, Makela T, Brunow G, Hase T, et al. Inhibition of human aromatase by mammalian lignans and isoflavonoid phytoestrogens. J Steroid Biochem Mol Biol. (1993) 44:147–53. doi: 10.1016/0960-0760(93)90022-O
105. Wang C, Makela T, Hase T, Adlercreutz H, Kurzer MS. Lignans and flavonoids inhibit aromatase enzyme in human preadipocytes. J Steroid Biochem Mol Biol. (1994) 50:205–12. doi: 10.1016/0960-0760(94)90030-2
106. Evans BA, Griffiths K, Morton MS. Inhibition of 5 alpha-reductase in genital skin fibroblasts and prostate tissue by dietary lignans and isoflavonoids. J Endocrinol. (1995) 147:295–302. doi: 10.1677/joe.0.1470295
107. Adlercreutz H. Phytoestrogens: epidemiology and a possible role in cancer protection. Environ Health Perspect. (1995) 103(Suppl. 7):103–12. doi: 10.1289/ehp.95103s7103
108. Thompson LU. Experimental studies on lignans and cancer. Baillière's Clin Endocrinol Metab. (1998) 12:691–705. doi: 10.1016/S0950-351X(98)80011-6
109. Webb AL, McCullough ML. Dietary lignans: potential role in cancer prevention. Nutr Cancer. (2005) 51:117–31. doi: 10.1207/s15327914nc5102_1
110. Zálešák F, Bon DJ-YD, Pospíšil J. Lignans and neolignans: plant secondary metabolites as a reservoir of biologically active substances. Pharmacol Res. (2019) 146:104284. doi: 10.1016/j.phrs.2019.104284
111. Shin M-K, Jeon Y-D, Jin J-S. Apoptotic effect of enterodiol, the final metabolite of edible lignans, in colorectal cancer cells. J Sci Food Agri. (2019) 99:2411–9. doi: 10.1002/jsfa.9448
112. De Silva FS. Enterolactone suppress prostate cancer. (PC) cells linking cellular metabolism and TGFβ. FASEB J. (2019) 33:471.3–471.3.
113. Prasad K. Antioxidant activity of secoisolariciresinol diglucoside-derived metabolites, secoisolariciresinol, enterodiol, enterolactone. Int J Angiol. (2000) 9:220–5. doi: 10.1007/BF01623898
114. Prasad K. Antihypertensive activity of secoisolariciresinol diglucoside (SDG) isolated from flaxseed: role of guanylate cyclase. Int J Angiol. (2004) 13:7–14. doi: 10.1007/s00547-004-1060-4
115. Yamauchi S, Sugahara T, Nakashima Y, Okada A, Akiyama K, Kishida T, et al. Radical and superoxide scavenging activities of matairesinol and oxidized matairesinol. Biosci Biotechnol Biochem. (2006) 70:1934–40. doi: 10.1271/bbb.60096
116. Penumathsa SV, Koneru S, Thirunavukkarasu M, Zhan L, Prasad K, Maulik N. Secoisolariciresinol diglucoside: relevance to angiogenesis and cardioprotection against ischemia-reperfusion injury. J Pharmacol Exp Therapeut. (2007) 320:951. doi: 10.1124/jpet.106.114165
117. Aehle E, Müller U, Eklund PC, Willför SM, Sippl W, Dräger B. Lignans as food constituents with estrogen and antiestrogen activity. Phytochemistry. (2011) 72:2396–405. doi: 10.1016/j.phytochem.2011.08.013
118. Tolleson WH, Doerge DR, Churchwell MI, Marques MM, Roberts DW. Metabolism of biochanin A and formononetin by human liver microsomes in vitro. J Agri Food Chem. (2002) 50:4783–90. doi: 10.1021/jf025549r
119. Setchell KDR, Brown NM, Zimmer-Nechemias L, Brashear WT, Wolfe BE, Kirschner AS, et al. Evidence for lack of absorption of soy isoflavone glycosides in humans, supporting the crucial role of intestinal metabolism for bioavailability. Am J Clin Nutr. (2002) 76:447–53. doi: 10.1093/ajcn/76.2.447
120. Hendrich S. Bioavailability of isoflavones. J Chromatogr B. (2002) 777:203–10. doi: 10.1016/S1570-0232(02)00347-1
121. Piskula MK, Yamakoshi J, Iwai Y. Daidzein and genistein but not their glucosides are absorbed from the rat stomach. FEBS Lett. (1999) 447:287–91. doi: 10.1016/S0014-5793(99)00307-5
122. Morito K, Hirose T, Kinjo J, Hirakawa T, Okawa M, Nohara T, et al. Interaction of phytoestrogens with estrogen receptors alpha and beta. Biol Pharm Bull. (2001) 24:351–6. doi: 10.1248/bpb.24.351
123. Kuiper GG, Lemmen JG, Carlsson B, Corton JC, Safe SH, van der Saag PT, et al. Interaction of Estrogenic chemicals and phytoestrogens with estrogen receptor β. Endocrinology. (1998) 139:4252–63. doi: 10.1210/endo.139.10.6216
124. Krenn L, Paper DH. Inhibition of angiogenesis and inflammation by an extract of red clover (Trifolium pratense L.). Phytomedicine. (2009) 16:1083–8. doi: 10.1016/j.phymed.2009.05.017
125. Varinska L, Gal P, Mojzisova G, Mirossay L, Mojzis J. Soy and breast cancer: focus on angiogenesis. Int J Mol Sci. (2015) 16:11728–49. doi: 10.3390/ijms160511728
126. Yu X, Zhu J, Mi M, Chen W, Pan Q, Wei M. Anti-angiogenic genistein inhibits VEGF-induced endothelial cell activation by decreasing PTK activity and MAPK activation. Med Oncol. (2012) 29:349–57. doi: 10.1007/s12032-010-9770-2
127. Yamamoto S, Sobue T, Kobayashi M, Sasaki S, Tsugane S. Soy, isoflavones, breast cancer risk in Japan. J Natl Cancer Inst. (2003) 95:906–13. doi: 10.1093/jnci/95.12.906
128. Tousen Y, Matsumoto Y, Matsumoto C, Nishide Y, Nagahata Y, Kobayashi I, et al. The combined effects of soya isoflavones and resistant starch on equol production and trabecular bone loss in ovariectomised mice. Br J Nutr. (2016) 116:247–57. doi: 10.1017/S0007114516001537
129. Zheng X, Lee SK, Chun OK. Soy Isoflavones and osteoporotic bone loss: a review with an emphasis on modulation of bone remodeling. J Med Food. (2016) 19:1–14. doi: 10.1089/jmf.2015.0045
130. Hirose A, Terauchi M, Akiyoshi M, Owa Y, Kato K, Kubota T. Low-dose isoflavone aglycone alleviates psychological symptoms of menopause in Japanese women: a randomized, double-blind, placebo-controlled study. Arch Gynecol Obstet. (2016) 293:609–15. doi: 10.1007/s00404-015-3849-0
131. Malik S, Pannu D, Prateek S, Sinha R, Gaikwad H. Comparison of the symptomatic response in Indian menopausal women with different estrogen preparations for the treatment of menopausal symptoms: a randomized controlled trial. Arch Gynecol Obstet. (2016) 293:1325–33. doi: 10.1007/s00404-016-4034-9
132. Tamura M, Hori S, Nakagawa H, Yamauchi S, Sugahara T. Effects of an equol-producing bacterium isolated from human faeces on isoflavone and lignan metabolism in mice. J Sci Food Agri. (2016) 96:3126–32. doi: 10.1002/jsfa.7490
133. Kolatorova L, Lapcik O, Starka L. Phytoestrogens and the intestinal microbiome. Physiol Res. (2018) 67:S401–8. doi: 10.33549/physiolres.934022
134. Kawada Y, Yokoyama S, Yanase E, Niwa T, Suzuki T. The production of S-equol from daidzein is associated with a cluster of three genes in Eggerthella sp. YY7918. Biosci Microb Food Health. (2016) 35:113–21. doi: 10.12938/bmfh.2015-023
135. Shimada Y, Takahashi M, Miyazawa N, Abiru Y, Uchiyama S, Hishigaki H. Identification of a novel dihydrodaidzein racemase essential for biosynthesis of equol from daidzein in Lactococcus sp. strain 20–92. Appl Environ Microbiol. (2012) 78:4902–7. doi: 10.1128/AEM.00410-12
136. Rotimi VO, Duerden BI. The development of the bacterial flora in normal neonates. J Med Microbiol. (1981) 14:51–62. doi: 10.1099/00222615-14-1-51
137. Setchell KD, Zimmer-Nechemias L, Cai J, Heubi JE. Exposure of infants to phyto-oestrogens from soy-based infant formula. Lancet. (1997) 350:23–7. doi: 10.1016/S0140-6736(96)09480-9
138. Setchell KD, Borriello SP, Hulme P, Kirk DN, Axelson M. Nonsteroidal estrogens of dietary origin: possible roles in hormone-dependent disease. Am J Clin Nutr. (1984) 40:569–78. doi: 10.1093/ajcn/40.3.569
139. Atkinson C, Berman S, Humbert O, Lampe JW. In vitro incubation of human feces with daidzein and antibiotics suggests interindividual differences in the bacteria responsible for equol production. J Nutr. (2004) 134:596–9. doi: 10.1093/jn/134.3.596
140. Day AJ, DuPont MS, Ridley S, Rhodes M, Rhodes MJ, Morgan MR, et al. Deglycosylation of flavonoid and isoflavonoid glycosides by human small intestine and liver beta-glucosidase activity. FEBS Lett. (1998) 436:71–5. doi: 10.1016/S0014-5793(98)01101-6
141. Kuiper GG, Carlsson B, Grandien K, Enmark E, Haggblad J, Nilsson S, et al. Comparison of the ligand binding specificity and transcript tissue distribution of estrogen receptors alpha and beta. Endocrinology. (1997) 138:863–70. doi: 10.1210/endo.138.3.4979
142. Kovats S. Estrogen receptors regulate innate immune cells and signaling pathways. Cell Immunol. (2015) 294:63–9. doi: 10.1016/j.cellimm.2015.01.018
143. Prossnitz ER, Barton M. Estrogen biology: new insights into GPER function and clinical opportunities. Mol Cell Endocrinol. (2014) 389:71–83. doi: 10.1016/j.mce.2014.02.002
144. Farooq A. Structural and functional diversity of estrogen receptor ligands. Curr Topics Med Chem. (2015) 15:1372–84. doi: 10.2174/1568026615666150413154841
145. Fuentes N, Silveyra P. Estrogen receptor signaling mechanisms. Adv Protein Chem Struct Biol. (2019) 116:135–70. doi: 10.1016/bs.apcsb.2019.01.001
146. Zhao L, Chen Q, Diaz Brinton R. Neuroprotective and neurotrophic efficacy of phytoestrogens in cultured hippocampal neurons. Exp Biol Med. (2002) 227:509–19. doi: 10.1177/153537020222700716
147. Pan M, Han H, Zhong C, Geng Q. Effects of genistein and daidzein on hippocampus neuronal cell proliferation and BDNF expression in H19–7 neural cell line. J Nutr Health Aging. (2012) 16:389–94. doi: 10.1007/s12603-011-0140-3
148. Gagne B, Gelinas S, Bureau G, Lagace B, Ramassamy C, Chiasson K, et al. Effects of estradiol, phytoestrogens, ginkgo biloba extracts against 1-methyl-4-phenyl-pyridine-induced oxidative stress. Endocrine. (2003) 21:89–95. doi: 10.1385/ENDO:21:1:89
149. Chen HQ, Wang XJ, Jin ZY, Xu XM, Zhao JW, Xie ZJ. Protective effect of isoflavones from Trifolium pratense on dopaminergic neurons. Neurosci Res. (2008) 62:123–30. doi: 10.1016/j.neures.2008.07.001
150. MacLusky NJ, Thomas G, Leranth C. Low dietary soy isoflavonoids increase hippocampal spine synapse density in ovariectomized rats. Brain Res. (2017) 1657:361–7. doi: 10.1016/j.brainres.2017.01.002
151. Lee YB, Lee KH, Sohn HS, Lee SJ, Cho KH, Kang IJ, et al. Effects of soy phytoestrogens on reference memory and neuronal cholinergic enzymes in ovariectomized rats. J Med Food. (2009) 12:64–70. doi: 10.1089/jmf.2007.0633
152. Liu LX, Chen WF, Xie JX, Wong MS. Neuroprotective effects of genistein on dopaminergic neurons in the mice model of Parkinson's disease. Neurosci Res. (2008) 60:156–61. doi: 10.1016/j.neures.2007.10.005
153. Simpkins JW, Perez E, Wang X, Yang S, Wen Y, Singh M. The potential for estrogens in preventing Alzheimer's disease and vascular dementia. Ther Adv Neurol Disord. (2009) 2:31–49. doi: 10.1177/1756285608100427
154. Schreihofer DA. Transcriptional regulation by phytoestrogens in neuronal cell lines. Mol Cell Endocrinol. (2005) 231:13–22. doi: 10.1016/j.mce.2004.12.006
155. Prossnitz ER, Arterburn JB, Sklar LA. GPR30: a G protein-coupled receptor for estrogen. Mol Cell Endocrinol. (2007) 265–6:138–42. doi: 10.1016/j.mce.2006.12.010
156. Sirotkin AV, Harrath AH. Phytoestrogens and their effects. Eur J Pharmacol. (2014) 741:230–6. doi: 10.1016/j.ejphar.2014.07.057
157. Guo TL, McCay JA, Zhang LX, Brown RD, You L, Karrow NA. Genistein modulations immune responses and increases host resistance to B16F10 tumor in adult female B6cF1 mice. J Nutr. (2001) 3251–8. doi: 10.1093/jn/131.12.3251
158. Sakai T, Kogiso M. Soy isoflavones and immunity. J Med Investig. (2008) 55:167–73. doi: 10.2152/jmi.55.167
159. Park J, Kim SH, Cho D, Kim TS. Formononetin, a phyto-oestrogen, its metabolites up-regulate interleukin-4 production in activated T cells via increased AP-1 DNA binding activity. Immunology. (2005) 116:71–81. doi: 10.1111/j.1365-2567.2005.02199.x
160. Kojima H, Takeda Y, Muromoto R, Takahashi M, Hirao T, Takeuchi S, et al. Isoflavones enhance interleukin-17 gene expression via retinoic acid receptor-related orphan receptors alpha and gamma. Toxicology. (2015) 329:32–9. doi: 10.1016/j.tox.2015.01.007
161. Abron JD, Singh NP, Price RL, Nagarkatti M, Nagarkatti PS, Singh UP. Genistein induces macrophage polarization and systemic cytokine to ameliorate experimental colitis. PLoS ONE. (2018) 13:e0199631. doi: 10.1371/journal.pone.0199631
162. Masilamani M, Wei J, Bhatt S, Paul M, Yakir S, Sampson HA. Soybean isoflavones regulate dendritic cell function and suppress allergic sensitization to peanut. J Allergy Clin Immunol. (2011) 128:1242–50.e1. doi: 10.1016/j.jaci.2011.05.009
163. Wei J, Bhatt S, Chang LM, Sampson HA, Masilamani M. Isoflavones, genistein and daidzein, regulate mucosal immune response by suppressing dendritic cell function. PLoS ONE. (2012) 7:e47979. doi: 10.1371/journal.pone.0047979
164. Smith BN, Dilger RN. Immunomodulatory potential of dietary soybean-derived isoflavones and saponins in pigs. J Animal Sci. (2018) 96:1288–304. doi: 10.1093/jas/sky036
165. Jin X, Wang S, Zhao X, Jin Q, Fan C, Li J, et al. Coumestrol inhibits autoantibody production through modulating Th1 response in experimental autoimmune thyroiditis. Oncotarget. (2016) 7:52797–809. doi: 10.18632/oncotarget.10353
166. Guo TL, White KL Jr., Brown RD, Delclos KB, Newbold RR, Weis C, et al. Genistein modulates splenic natural killer cell activity, antibody-forming cell response, phenotypic marker expression in F(0) and F(1) generations of Sprague-Dawley rats. Toxicol Appl Pharmacol. (2002) 181:219–27. doi: 10.1006/taap.2002.9418
167. Mace TA, Ware MB, King SA, Loftus S, Farren MR, McMichael E, et al. Soy isoflavones and their metabolites modulate cytokine-induced natural killer cell function. Sci Rep. (2019) 9:5068. doi: 10.1038/s41598-019-41687-z
168. Dia VP, Berhow MA, Gonzalez De Mejia E. Bowman-Birk inhibitor and genistein among soy compounds that synergistically inhibit nitric oxide and prostaglandin E2 pathways in lipopolysaccharide-induced macrophages. J Agric Food Chem. (2008) 56:11707–17. doi: 10.1021/jf802475z
169. Choi C, Cho H, Park J, Cho C, Song Y. Suppressive effects of genistein on oxidative stress and NFkappaB activation in RAW 264.7 macrophages. Biosci Biotechnol Biochem. (2003) 67:1916–22. doi: 10.1271/bbb.67.1916
170. Denic A, Wootla B, Pirko I, Mangalam A. Pathophysiology of experimental autoimmune encephalomyelitis. In: Minagar A, editor. Multiple Sclerosis. San Diego, CA: Academic Press (2016). p. 249–80.
171. De Paula ML, Rodrigues DH, Teixeira HC, Barsante MM, Souza MA, Ferreira AP. Genistein down-modulates pro-inflammatory cytokines and reverses clinical signs of experimental autoimmune encephalomyelitis. Int Immunopharmacol. (2008) 8:1291–7. doi: 10.1016/j.intimp.2008.05.002
172. Castro SB, Junior CO, Alves CC, Dias AT, Alves LL, Mazzoccoli L, et al. Immunomodulatory effects and improved prognosis of experimental autoimmune encephalomyelitis after O-tetradecanoyl-genistein treatment. Int Immunopharmacol. (2012) 12:465–70. doi: 10.1016/j.intimp.2011.12.025
173. Razeghi Jahromi S, Arrefhosseini SR, Ghaemi A, Alizadeh A, Moradi Tabriz H, Togha M. Alleviation of experimental allergic encephalomyelitis in C57BL/6 mice by soy daidzein. Iran J Allergy Asthma Immunol. (2014) 13:256–64.
174. Wei Z, Wang M, Hong M, Diao S, Liu A, Huang Y, et al. Icariin exerts estrogen-like activity in ameliorating EAE via mediating estrogen receptor beta, modulating HPA function and glucocorticoid receptor expression. Am J Transl Res. (2016) 8:1910–8.
175. Wei Z, Deng X, Hong M, Su Q, Liu A, Huang Y, et al. Icariin has synergistic effects with methylprednisolone to ameliorate EAE via modulating HPA function, promoting anti-inflammatory and anti-apoptotic effects. Int J Clin Exp Med. (2015) 8:20188–97.
176. Muthian G, Bright JJ. Quercetin, a flavonoid phytoestrogen, ameliorates experimental allergic encephalomyelitis by blocking IL-12 signaling through JAK-STAT pathway in T lymphocyte. J Clin Immunol. (2004) 24:542–52. doi: 10.1023/B:JOCI.0000040925.55682.a5
177. Gandy KAO, Zhang J, Nagarkatti P, Nagarkatti M. Resveratrol. (3, 5, 4'-trihydroxy-trans-Stilbene) attenuates a mouse model of multiple sclerosis by altering the miR-124/sphingosine kinase 1 axis in encephalitogenic T cells in the brain. J Neuroimmune Pharmacol. (2019) 14:462–77. doi: 10.1007/s11481-019-09842-5
Keywords: multiple sclerosis and neuroimmunology, phytoestrogen, gut microbiome, immune system, nervous system, diet
Citation: Cady N, Peterson SR, Freedman SN and Mangalam AK (2020) Beyond Metabolism: The Complex Interplay Between Dietary Phytoestrogens, Gut Bacteria, and Cells of Nervous and Immune Systems. Front. Neurol. 11:150. doi: 10.3389/fneur.2020.00150
Received: 03 December 2019; Accepted: 14 February 2020;
Published: 13 March 2020.
Edited by:
Fabienne Brilot, University of Sydney, AustraliaReviewed by:
Javier Ochoa-Reparaz, Eastern Washington University, United StatesCopyright © 2020 Cady, Peterson, Freedman and Mangalam. This is an open-access article distributed under the terms of the Creative Commons Attribution License (CC BY). The use, distribution or reproduction in other forums is permitted, provided the original author(s) and the copyright owner(s) are credited and that the original publication in this journal is cited, in accordance with accepted academic practice. No use, distribution or reproduction is permitted which does not comply with these terms.
*Correspondence: Ashutosh K. Mangalam, YXNodXRvc2gtbWFuZ2FsYW1AdWlvd2EuZWR1
Disclaimer: All claims expressed in this article are solely those of the authors and do not necessarily represent those of their affiliated organizations, or those of the publisher, the editors and the reviewers. Any product that may be evaluated in this article or claim that may be made by its manufacturer is not guaranteed or endorsed by the publisher.
Research integrity at Frontiers
Learn more about the work of our research integrity team to safeguard the quality of each article we publish.