- 1Laboratory for Experimental Brain Research, Division of Neurosurgery, Department of Clinical Sciences, Lund University, Lund, Sweden
- 2CICS-UBI-Health Sciences Research Centre, Faculdade de Ciências da Saúde, Universidade da Beira Interior, Covilha, Portugal
- 3LUBIN Lab-Lunds Laboratorium för Neurokirurgisk Hjärnskadeforskning, Division of Neurosurgery, Department of Clinical Sciences, Lund University, Lund, Sweden
Thyroid hormones are of fundamental importance for brain development and essential factors to warrant brain functions throughout life. Their actions are mediated by binding to specific intracellular and membranous receptors regulating genomic and non-genomic mechanisms in neurons and populations of glial cells, respectively. Among others, mechanisms include the regulation of neuronal plasticity processes, stimulation of angiogenesis and neurogenesis as well modulating the dynamics of cytoskeletal elements and intracellular transport processes. These mechanisms overlap with those that have been identified to enhance recovery of lost neurological functions during the first weeks and months after ischemic stroke. Stimulation of thyroid hormone signaling in the postischemic brain might be a promising therapeutic strategy to foster endogenous mechanisms of repair. Several studies have pointed to a significant association between thyroid hormones and outcome after stroke. With this review, we will provide an overview on functions of thyroid hormones in the healthy brain and summarize their mechanisms of action in the developing and adult brain. Also, we compile the major thyroid-modulated molecular pathways in the pathophysiology of ischemic stroke that can enhance recovery, highlighting thyroid hormones as a potential target for therapeutic intervention.
Introduction
Thyroid hormones (TH), 3,5,3′,5′-tetraiodo-L-thyronine (T4) and 3,5,3′-triiodo-L-thyronine (T3), are important for brain development in mammals, during embryonic and fetal stages, regulating processes of neuronal proliferation, migration and differentiation, neurite outgrowth, synaptic plasticity, dendritic branching, and myelination (1–5). Also, after birth, TH are crucial for normal brain function throughout the entire life. Specifically for the central nervous system (CNS) the active form T3 is a key regulator for normal metabolism in humans and rodents (1, 6, 7).
Availability of T3 to the developing and adult brain is tightly controlled by mechanisms regulating TH secretion, free fraction unbound to thyroxine binding globulins (TBG), transmembrane transporters and the activity of iodothyronine deiodinases (DIO). The pattern of these regulatory processes may vary according to the developmental stage and in the adult brain. T3 plays an essential role for neurological functions, and minimal disturbances of these mechanisms may have consequences for normal brain development and function (1, 8).
Dependence of the CNS on T3 at all stages of development prompted us to review the actions of TH and the relevance of these mechanisms for processes of recovery after ischemic stroke. We will begin to provide an overview on TH signaling in the brain during development and throughout adult life. Thereafter, we will focus on molecules involved in TH signaling after stroke. TH actions at specific time points after the insult, that are dependent of carrier proteins, transmembrane transporters, DIO activity, thyroid hormone receptors (TR) and co-factors, may provide information on underlying molecular and cellular mechanisms that enhance functional recovery of lost neurological functions. Moreover, we will discuss which mechanisms of action of TH in the brain may contribute to enhance functional outcome in stroke patients.
Thyroid Hormone Transport and Availability to the Human and Rodent Brain
In the adult, TH originate in the thyroid gland that secretes ~93% as T4 and 7% as T3. Once secreted to plasma, TH binding proteins play an important role to maintain TH homeostasis and distribution into tissues, and <0.1% of TH circulate free in the blood (9, 10). In humans, 65% of TH bind to TBG, 20% to albumin and about 15% to transthyretin (TTR) (11, 12) while in rodents TTR is the main protein carrier in the blood circulation (13, 14). TH also bind to lipoproteins to a less extent (15, 16).
In contrary to processes during brain development, a different fraction of T3 in the adult brain is provided from free T3 available in the blood circulation and in the cerebrospinal fluid (CSF) (17, 18). Most of TH provided to the brain crosses the blood brain barrier (BBB) and around 20% the blood cerebrospinal fluid barrier (BCSFB) (19, 20). This passage is mediated by transmembrane transporter proteins with overlapping specificity that were identified in endothelial cells of brain microvessels that constitute the BBB and epithelial cells of the choroid plexus (CP) of humans and rodents. These transporters are also important for brain development, and include monocarboxylate transporter (MCT), organic anion transporting polypeptide (OATP), large neutral aminoacid transporter (LAT) and sodium/taurocholate co-transporting polypeptide (SLC10A1) families (17, 21–31). Among those, MCT8 is of particular importance for T3 (21, 26, 29), and in mice deficient for this transporter, T3 uptake is compromised (32). In humans, MCT10 also facilitates uptake and efflux of TH, in particular for T3 (33). The gene that encodes MCT8 (Slc16a2) is also present in membranes of neurons, astrocytes, tanycytes and oligodendrocyte precursor cells and OATP1C1 mRNA has been found in astrocytes (34), mediating intracellular TH transport. In addition, it has been proposed that either T4 or T3 or both are captured via gaps at the endfeet of astrocytes covering brain microvessels (35, 36).
In contrast to the rodent, MCT8 deficiency in humans results in low T3 levels in the brain and high levels in the serum due to TH transport deficiency. Thus, the development of the cerebral cortex is impaired accompanied with severe neurological impairment (37–40). The lack of alternative TH transporters such as OATP1C1 (28) and MCT10 (41) in the adult human brain to compensate the transport of T3, may contribute to the neurological deficits observed in humans with MCT8 mutations. The activity of DIO2 and DIO3 in the brain is important to balance neuronal intracellular T3 levels in the adult brain, according to the developmental stage and brain region (42–44). DIO enzymes catalyze and remove specific iodine atoms from iodothyronine molecules. In rodents, ~50% of T3 levels localized in the brain relies on local deiodination of T4 in astrocytes and tanycytes by DIO2 (45). T3 produced in glial cells is able to promote T3 driven transcriptional activity in neurons, demonstrated by in vitro experiments in co-cultures of H4 human glioma cells expressing DIO2 and neuroblastoma cells (46). Homeostasis of T3 in the CNS is also controlled by DIO3 activity in neurons, that converts T4 into 3,3′,5′ reverse triiodo-L-thyronine (rT3) and inactivates T3 into 3,3′-diiodo-L-thyronine (T2) (43, 47, 48).
Other compensatory mechanisms to maintain sufficient T3 levels in the rodent brain include the reduction of DIO3 activity and consequently T3 degradation, the increase of DIO2 activity in astrocytes (17, 32, 49) and the increase of Dio2 expression in interneurons in the cerebral cortex (50).
Thyroid Hormones in Brain Development
It has been shown that mechanisms of brain development might be re-activated in processes of brain reorganization following stroke (51) involving cascades regulated by TH. Therefore, knowledge of TH actions critical and specific for each step of brain development is instrumental to understand their functions following stroke. Epidemiological and clinical studies in humans clearly show that several conditions that compromise maternal TH availability to the fetus impair brain development and are associated with neurological disorders and structural defects, most of them irreversible. A detailed review about TH transport, metabolism and function in the developing brain was recently published (52).
Despite epidemiological and clinical studies have demonstrated the demand of TH during brain development, animal experimental models are of high relevance to identify molecular mechanisms and detailed morphological changes of their biological function during brain development (53). Processes of neurogenesis, proliferation, migration, and maturation show different temporal profiles between humans and rodents, however, basic mechanisms and pathways that regulate brain development are similar (54, 55) allowing the extrapolation of TH deficiency mediated effects in rodents to abnormal TH signaling in humans. Maternal TH are crucial for early cortical neurogenesis, neuronal migration and maturation, during the first trimester of gestation, when fetal brain development occurs (1, 56–58). Both T4 and T3 are detected in the human brain embryo even before fetal thyroid gland maturation (59) that occurs at 11–12th week of gestation and starts to secrete TH at week 16 (60).
Also in the rat, the embryo is exposed to maternal TH after embryonic day 11 (E11), before the start of thyroid gland development at E17 (61–64). Experimental hypothyroxinemia induced in rats during this period (before E18) causes abnormal neurogenesis in the cortex and hippocampus, leading to impairment of synaptic plasticity and cognitive deficits (65–67), processes of high relevance in mechanisms of recovery after stroke.
Most of TH dependent processes during brain development are due to the interaction of T3 with nuclear receptors and regulation of gene expression (68). Increasing levels of protein and mRNA encoding TR alpha and beta (TRα and TRβ) isoforms in the cerebrum and cerebellum start from the 8th to 10th week and increase over gestational time (69, 70) and in rodents there is expression of nuclear TR protein before thyroid gland functioning (71) suggesting transcriptional activity of TH. Several TH dependent genes expressed in the fetal rat brain and neuronal cultures, such as cytoskeletal proteins, are involved in mechanisms of neuronal migration and maturation, branching in neurons and astrocytes (1, 72–74). In both human and rodent species, mutations at the TRα and TRβ result in several neurological disorders (75–79).
The expression pattern of TR in the brain changes during CNS development. TRα1 is the predominant isoform with mRNA and protein expression in the entire brain, in rodents from E14 (80, 81) and in humans from 8th week of gestation (82, 83) onwards, importantly the expression decreases during brain development (84). TRβ1 is expressed at later stages of brain development and in contrary to TRα, TRβ1 mRNA levels do not decrease over gestational time (80, 84, 85). These studies indicate that gene transcription mediated by nuclear TR has spatiotemporal expression patterns and, therefore, TH actions are distinct in all stages of brain development.
In an in vitro model of differentiating mouse embryonic stem cell line (ES-E14TG2a) T3 treatment (1 nM) enhanced the number of nestin-positive neuronal progenitors, accelerated differentiation and increased survival of pyramidal neurons (86). T3 mediated differentiation was associated with the regulation of genes involved in corticogenesis namely nestin, empty spiracles homeobox 1 (Emx1), T-box brain gene 1 (Tbr1), Calmodulin kinase 4 (Camk4), and RC3/Neurogranin (Nrgn) (86). Regulation of gene expression during differentiation seems to be inversely correlated with levels of chicken ovalbumin upstream-transcription factor 1 (COUP-TF1) (86), that is crucial for adequate neuronal development (87).
Mechanisms of Thyroid Hormones Actions in the Adult Brain
Cellular actions of TH in the adult brain can be mediated by nuclear receptors and transcriptional activity, and also by non-genomic actions (85, 88, 89). Here we will elaborate in relevant TH actions described in the literature, and below we will delineate TH actions that might be involved in neurorepair processes.
Genomic Actions of TH
Actions of T3 in the brain are mainly dependent on transcription mediated by T3 binding to the nuclear receptors and formation of regulatory complexes (85, 88–91). In the presence of TH, TR are regulated by corepressors (CoR) and coactivators (CoA), proteins, that repress or activate transcription, respectively (36, 85, 88, 89, 92) (Figure 1).
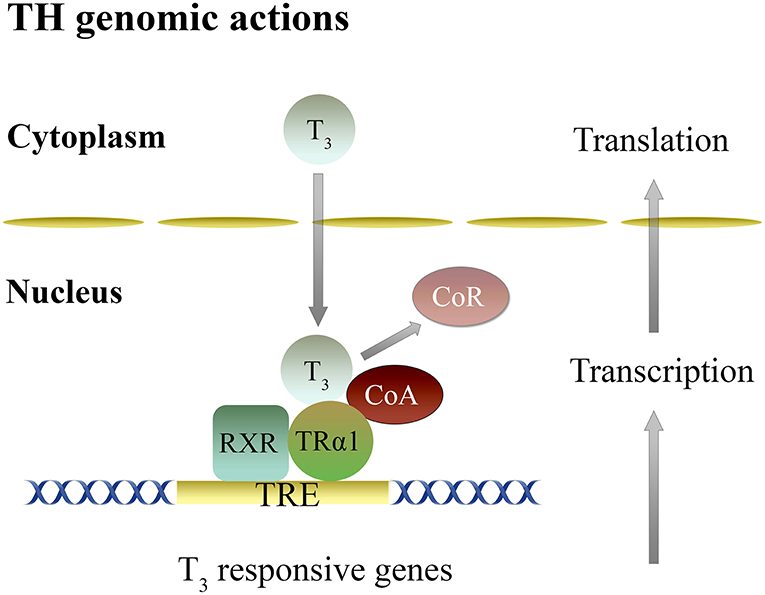
Figure 1. Genomic mechanisms of thyroid hormones action in the brain. Genomic actions of T3 are dependent on gene transcription mediated by its binding to nuclear TRα and TRβ, and the formation of heterodimer complex with RXR (RXR-TR) that binds to a TRE, located at the regulatory region of T3 target genes. This activity is regulated by an exchange of CoR for CoA. CoA, Co-activator; CoR, Co-repressor; RXR, Retinoid X receptor; TR, Thyroid hormone receptor; TRE, Thyroid response element; T3, 3,5,3′-triiodo-L-thyronine.
In mammals, there are four isoforms of TR (TRα1, TRα2, TRβ1, and TRβ2) encoded by genes alpha (Thra) and beta (Thrb), which expression and distribution is different to the developmental brain (93). These isoforms are differently distributed in the tissues, regulate the transcription of different genes and exert different biological actions (94).
TRα1 and TRβ1 are the predominant isoforms in the CNS. TRα1 mRNA and protein accounts to 70–80% of TR expression in the brain (36, 95, 96). Thus, genomic actions of T3 in the brain are mainly, but not exclusively, dependent of TRα1 signaling (97). The analysis of brains from TRα1—green fluorescent protein (GFP) mice revealed that this receptor is expressed in all NeuN positive neurons, especially in the nucleus (83). TRα1 is expressed in both excitatory glutamatergic and inhibitory GABAergic neurons in several brain regions including the striatum, cerebral cortex, hippocampus and dentate gyrus, hypothalamus and cerebellum (83, 98). This isoform of TR is also found in tanycytes lining the third ventricle and oligodendrocytes in the hypothalamus, but not in the striatum, somatosensory cortex or hippocampus (83, 99). Its presence in astrocytes is not completely clear (100), however may be dependent on the activation status. Although lower concentration of the receptor is found in cultured rat astrocytes (101), it is not expressed in glial fibrillary acidic protein (GFAP) positive astrocytes in the naïve rat and mouse brain (83, 99). TRα1 is absent in Purkinje cells in the cerebellum (83). Levels of TRα2, a non T3 binding isoform, is also detected in the adult brain in a similar pattern as TRα1 (98).
TRβ1 is expressed in the neocortex, and mainly expressed in the pyramidal cell layers of the hippocampus, granule cells of dentate gyrus and paraventricular hypothalamic nucleus (102). It is also expressed in myelin basic protein positive oligodendrocytes (99). In contrary to TRα1, this isoform is highly expressed in Purkinje neurons (94). The TRβ2 isoform is restricted to the anterior pituitary gland and hypothalamus (102–105). As for TRα isoforms, TRβ1 and TRβ2 were not observed in positive GFAP positive astrocytes in the rat brain (99).
Although TR are mainly localized in the cell nucleus and nuclear membrane, TRα1 and TRβ1 isoforms have also been found in the cytoplasm of neurons and astroglia, and this shuttle may increase the rate of T3 nuclear import (106). It has been suggested that T4 may also exert genomic actions in the brain through binding to TRα1, that is more susceptible to T4 than TRβ1 (36), however we did not find experimental studies supporting this hypothesis. So far, a total of 4,108 genes, of which 734 have been identified as being repeatedly regulated by T3 in the rodents' brain by microarray analysis (72). In this review, we provide an overview on T3-modulated genes that might be involved in brain repair mechanisms (Table 1). Hence, different T3–dependent transcriptional activities have been observed in different cell types and brain regions.
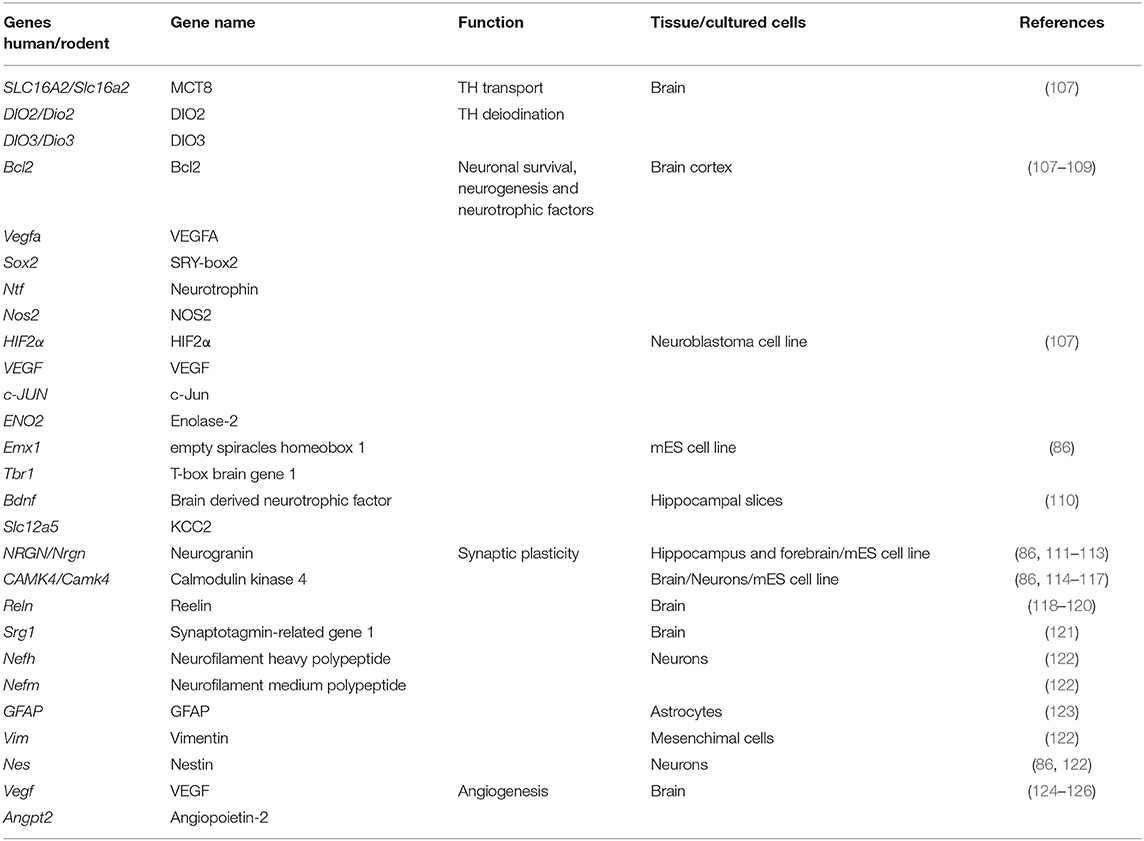
Table 1. List of genes regulated by thyroid hormones involved in their transport into the brain, mechanisms of tissue repair, and neuronal plasticity following ischemic stroke.
Non-genomic Actions of TH
TH non-genomic actions that do not require TH binding to nuclear receptors are well-described in the literature (127–130). Actions are immediate and include several interactions of TH with extranuclear receptors, including TRα and TRβ, located in the cytoplasm, cellular membrane, cytoskeleton and mitochondria, modulating several intracellular pathways.
The following points summarize relevant non-genomic actions of TH binding to membranous and cytoplasmic receptors (i–iii), cytoplasmic TH binding proteins affecting ion pumps activity (iv) and the action of TH on the cytoskeleton (v) (Figure 2). (i) T3 complexed to TRβ1 in the cytoplasm interacts with p85α subunit of phosphatidylinositol 3-kinase (PI3K), resulting in phosphorylation and activation of protein kinase (PK) B/Akt signal transduction pathway, rapamycin (mTOR) and phosphorylation of p70S6K (131–136). (ii) T3 is able to bind to integrin αvβ3 S1 domain in plasma membranes and activates PI3K via Src kinase. T4 and T3 interact with integrin αvβ3 S2 domain and activate mitogen-activated protein kinase 1/2 (MAPK 1/2) signaling cascade, through phospholipase C (PLC) and PKC (127, 133, 137–140). Subsequently, it results in an nuclear translocation of TRβ1 (141), estrogen receptor α (142), signal transducing and activator of transcription (STAT) 1α, interferon gamma (IFN-γ) (143) and CoA protein Trip230 (144). In addition, hormone activated MAPK 1/2 phosphorylates TRβ1 at Ser-142, leading to recruitment of CoA proteins (145). (iii) T4 non-gnomically activates MAPK 1/2 in HeLa and CV-1 cultured cells (146, 147) and phosphorylation of p53 (148) and STAT3 (147). (iv) T3 modulates Na+/H+ exchanger in myoblasts (149); Na-K-ATPase activity in alveolar epithelial cells (150–152), embryonal hepatocytes (153), and synaptosomes (154, 155) through either the PI3K or MAPK pathways (152, 156); the Ca-ATPase activity in erythrocytes (88), the sarcoplasmic reticulum in the heart (157) and in cerebrocortical synaptosomes (158). (v) T4 and rT3 stimulate polymerization of actin components of the cytoskeleton neuronal and astrocyte cell cultures, through TH binding to an extranuclear truncated form of TRα1 (TRΔα1) (159–162).
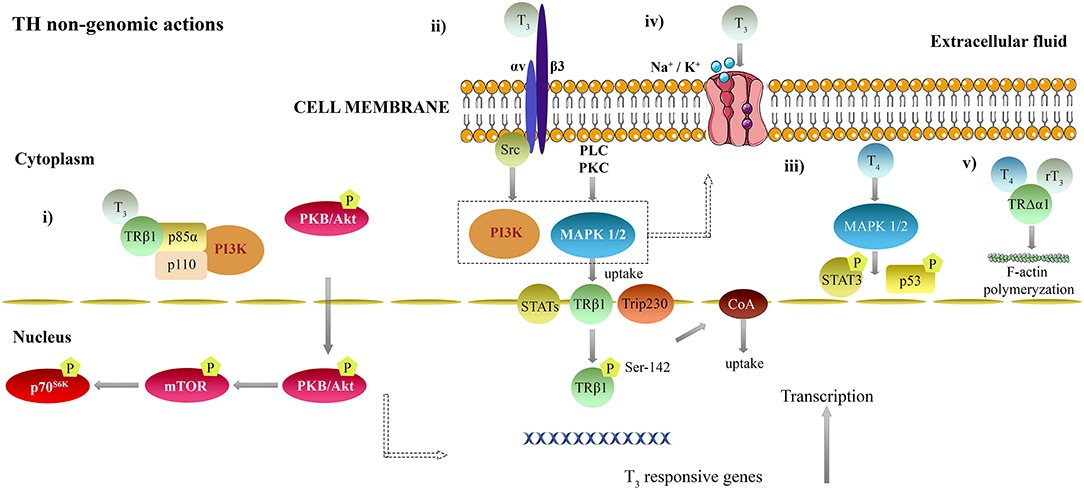
Figure 2. Non-genomic mechanisms of thyroid hormones action in the brain. T3 can also modulate other genes not containing TRE, by non-genomic actions. (i) T3 complexed to TRβ1 in the cytoplasm interacts with p85α subunit of PI3K, resulting in phosphorylation and activation of PKB/Akt signal transduction pathway, mTOR and phosphorylation of p70S6K (131–136). (ii) T3 is able to bind to integrin αvβ3 S1 domain in plasma membranes and activates PI3K via Src kinase. T4 and T3 interact with integrin αvβ3 S2 domain and activate MAPK 1/2 signaling cascade, PLC and PKC (127, 133, 137–140). Subsequently, it results in an nuclear translocation of TRβ1 (141), estrogen receptor α (142), STAT1α, IFN-γ (143) and CoA protein Trip230 (144). In addition, hormone activated MAPK 1/2 phosphorylates TRβ1 at Ser-142, leading to recruitment of CoA proteins (145). (iii) T4 non-gnomically activates MAPK 1/2 in HeLa and CV-1 cultured cells (146, 147) and phosphorylation of p53 (148) and STAT3 (147). (iv) T3 modulates Na+/H+ exchanger in myoblasts (149); Na-K-ATPase activity in alveolar epithelial cells (150–152), embryonal hepatocytes (153) and synaptosomes (154, 155) through either the PI3K or MAPK pathways (152, 156) the Ca-ATPase activity in erythrocytes (88), the sarcoplasmic reticulum in the heart (157) and in cerebrocortical synaptosomes (158). (v) T4 and rT3 stimulate polymerization of actin components of the cytoskeleton neuronal and astrocyte cell cultures, through TH binding to an extranuclear truncated form of TRα1 (TRΔα1) (159–162). IFN-γ, interferon gamma; MAPK 1/2, mitogen-activated protein kinase 1/2; PLC, phospholipase C; PI3K, phosphatidylinositol 3-kinase; PK - protein kinase; mTOR – rapamycin; STAT - signal transducing and activator of transcription; TH, Thyroid hormones; TR, Thyroid hormone receptor; TRE, Thyroid response element; T4, 3,5,3′,5′-tetraiodo-L-thyronine; T3, 3,5,3′-triiodo-L-thyronine.
Thyroid Hormones in the Aging Brain and Ischemic Stroke
The complex process of aging is associated with changes in TH metabolism and action in all tissues. During aging, the disruption of circadian rhythm leads to a reduction in thyroid stimulating hormone (TSH) secretion (163–165) and circulating TH levels, in particular T3, in humans (166, 167) and rodents (168). Nevertheless, TH signaling is well-preserved in the aging brain, as demonstrated in mouse models of aging (169). However, hypothyroidism and decreased TH availability to the brain has been considered a risk factor for the development of neurodegenerative diseases (170, 171) and acute ischemic stroke (172, 173). In addition, recent epidemiological studies have associated low levels of T3 with poor functional outcome after acute ischemic stroke (174–179). Interestingly, lower total T3 levels is not related with poor functional recovery after ischemic stroke in patients below 65 years of age, suggesting that the association between levels of T3 and stroke recovery may be clinically important in older patients (180). Non-thyroidal illness syndrome also impairs functional recovery after stroke (181). Besides, stroke patients with thyroid dysfunction (lower levels of TSH and higher levels of free T4) are associated with poorer clinical outcome (182). Together, studies point toward the need for a systemic assessment of thyroid dysfunction and stroke outcome.
Some reports suggest neuroprotective effects of hypothyroidism prior to brain ischemia in humans (183, 184), as well as experimental studies in rodents (185, 186). In a recent systematic review, stroke patients with subclinical hypothyroidism (higher levels of TSH and normal levels of free T4 within the reference range) were more prone to suffer a non-fatal stroke and minor adverse events (182). However, we lack mechanistic studies how systemic levels of TH exactly influence processes in the postischemic brain. It is likely that hypothyroid episodes prior to stroke only delayed neuronal death, due to decreased metabolic demand of neurons, decreased glutamate production and delayed oxidative stress (185, 187). There is no evidence from experimental studies that show beneficial effects in hypothyroid animals after stroke. A recent animal study suggested that daily intravenous administrations of rT3, an inactive form of T3, prevents ischemic-reperfusion injury in rats subjected to transient MCAO, however authors did not evaluate if rT3 induced an hypothyroid state (188). Similarly, in rats subjected to permanent middle cerebral artery occlusion (MCAO), TH serum levels are reduced 14 days after injury correlated with increased neurological impairment (189).
On the other hand, hyperthyroidism has been associated with an increased risk for ischemic stroke in humans (190–192). However, the population-based study was performed in patients aged 18–44 years. Hence, this study has not been adjusted for other risk factors such as hypertension and atrial fibrillation that may independently contribute for stroke. Larger infarct volumes also have been found in hyperthyroid rats after transient MCAO (193). Hence, hyperthyroid rats (oral administration of TH for 4 weeks) showed profound effects on the cardiovascular system including hypertension and tachyarrhythmia and treatment resulted in a catabolic metabolism (193).
Interestingly, increased mRNA expression of Dio2 has been found in astrocytes during the first 72 h after transient MCAO (194). Together with modulation of Thrb expression, that is reduced in the infarct core and increased in the peri-infarct area, it suggests a local action of T3 (189). Repeated daily administrations of T4, before and on days one, two and three after stroke, decrease neuronal damage in the cornu ammonis CA1 pyramidal cells in the hippocampus (195). In an animal model of MCAO, intraperitoneal injection of T4 (11 μg/kg, 1 h after ischemia and 6 h after reperfusion) reduced cortical and striatal infarct volume 24 h after stroke, with a reduction of GFAP, Iba-1, PKC, and MAPK 1/2 expression (196). Treatment with levothyroxine (25 μg/kg intraperitoneal) 1 h after traumatic brain injury stimulated mRNA expression of genes encoding MCT8, DIO2, and DIO3; genes related with neuronal survival and neurogenesis, namely Bcl2, vascular endothelial growth factor A (Vegfa), Sox2, and neurotrophin (Ntf ) in the cortex, and of inducible nitrite oxide synthase 2 (Nos2) (107).
Moreover, intraperitoneal administration of T3 at 12 μg/kg 1 h after traumatic brain injury reduced lesion size and inflammation (197). T3 treatment 25 μg/kg 30 min after transient MCAO also reduced volume of stroke damage in mice through stimulation of fatty acid oxidation by astrocytes (198). A combination therapy of bone marrow stromal cells, daily injections of T3 200 μg/kg and mild exercise was related to reduce ischemic damage 7 days after transient MCAO in rats (199). Likewise, intraperitoneal administration of thyroxine derivates, 3-iodothyronamine and thyronamine, 50 mg/kg 1 h after MCAO in mice, also reduced infarct volume (200). Neuroprotective action of 3-iodothyronamine administered 2 days before MCAO was associated with hypothermia (200). Although molecular mechanisms have not been evaluated, these studies suggest that non-genomic actions of TH contribute to neuroprotection in the acute phase following stroke. In addition, T3 treatment prior to brain ischemia (25 μg/kg intravenous) has prevented edema through suppression of aquaporin-4 (AQP4) water channel expression and thereby reducing infarct volume and improving neurological outcome after transient MCAO (109), effects that were enhanced when T3 was administrated in nanoparticles at equivalent doses (108). Recently, it has been demonstrated that T3 modulates AQP4 expression dependent on developmental stage of the CNS. Treatment of mice with T3 at 1 μg/g increased AQP4 expression in astrocytes in the cerebral cortex until the 60th postnatal day. In contrast, whereas in glioblastoma cell lines stimulation with T3 (50 nM) treatment was downregulating the expression of AQP4 (201).
Few experimental studies have been performed to identify the mechanisms of TH actions in neuroprotection. Treatment of mouse hippocampal slices lesioned between CA3 and CA1 with T4 increases levels K-Cl cotransporter (KCC2) mRNA in a brain derived neurotrophic factor (BDNF) dependent manner, that promoted survival and regeneration of damaged neurons in the CA1 region (110). Moreover, it has been demonstrated that treatment with T3 has a protective effect against glutamate toxicity in cultured astrocytes and neurons (202, 203). This action has been linked to non-genomic actions of TH on Na+/H+ exchange activity and glutamate transport (204). Also, T3 treatment stimulated the expression of HIF2α, VEGF, c-JUN, and Enolase-2 (ENO2) in the neuroblastoma in an in vitro hypoxia model (107). Although only a few scattered studies have been performed, they indicate an involvement of T3 in pathways that promote neuronal protection and recovery, through genomic or non-genomic actions.
The expression of TR in the human brain after ischemic stroke have not been studied. Also in experimental models, TR expression has not been investigated, and we found only one experimental study reporting TR expression after permanent MCAO (189). Interestingly, TRα1 expression is increased in microglial cells in the infarct core and in neurons in the peri-infarct area. Astrocytes mildly express nuclear TRα1 and expression of TRβ1 is strongly expressed in the astrocytic scar. If TRα1 and TRβ1 play a crucial role for recovery after brain stroke, in humans and rodents, it remains to be investigated. In fact, TRα1 have been demonstrated to play a crucial role for cardiomyocyte survival after myocardial infarction (205) Therefore, the idea that TRα1 could be a target to promote stroke recovery definitively needs to be further investigated.
Mechanisms of Thyroid Hormones that may Enhance Mechanisms of Recovery After Stroke
Beyond the acute phase after stroke, the brain shows the capacity of spontaneous recovery of lost neurological functions albeit to a limited extent. This process remains slow, however, the intrinsic mechanisms are present and patients may benefit from enhancing those. TH regulate several pathways that are involved in neurorepair, namely regulation of processes of neuronal plasticity, neurogenesis, angiogenesis, and glutamate toxicity. Adjuvant therapies that modulate those processes may improve recovery of function after stroke, in particular when applied in combination with physiotherapy in stroke patients or an enriched environment in rodent models of stroke (206, 207).
Neuronal Plasticity
Neuronal reorganization occurs during the recovery phase of stroke and is initiated by cellular responses to degeneration. Cell death in the infarct core results in synaptic degeneration, instigating regenerative responses among surviving neurons, as the formation of new synaptic connections (208). Neuronal plasticity includes all mechanisms involved in modulation of dendritic and axonal arborization, dendritic spine density and neuronal density that will determine the formation of new synaptic connections and neuronal networks (209, 210).
Neuroplasticity occurs spontaneously during stroke recovery and TH have been identified as a modulator of several genes that may stimulate endogenous neuroplasticity and therefore contributing to facilitate recovery (Table 1).
In rodents, T3 regulates neuron specific RC3/Neurogranin gene (Nrgn), that encodes a calmodulin binding protein (112) which binds to calmodulin in the absence of calcium distribution in spines and enhances synaptic plasticity (211). Nrgn is highly expressed in dendritic spines in the hippocampus and forebrain and deficiency of Nrgn in mice has been reported to induce deficits in spatial learning and anxiety-like tendencies (113). In the human, the homolog gene NRGN is also a direct TH target, during development and in the adult brain (111). TH also regulates Reelin (Reln) expression during brain development (118). Administration of T3 increases Reln expression in the hippocampus of adult rats (212). Reelin is involved in the migration of multipolar neurons in the developing neocortex (120) and in the adult brain interacts with apoliprotein E receptors and regulates synaptic plasticity and neurogenesis (119).
During brain development, T3 regulates genes related with the calcium/Calmodulin-activated kinase 4—cAMP responsive element-binding protein 1 (CaMK4/Creb1) signaling pathway, as demonstrated in cultured fetal neurons (213), a mouse embryonic stem cell line (86) and in vivo studies (114–117). The CaMK4/Creb1 pathway regulates calcium influx and dendritic growth during development (214), inhibits apoptosis through phosphorylation of Creb and increases anti-apoptotic gene expression. Synaptotagmin-related gene 1 (Srg1) is also a TH responsive gene during brain development, that has a putative role as a mediator of synaptic structure and activity (121).
Reorganization of spine cytoskeleton, principally microtubules and actin filaments, can be dynamically modulated and consequently change the pattern of synaptic activity (215). Several studies have shown that TH modulate tubulins (216, 217), microtubule associated proteins (218) and Tau protein (219) in the cytoskeleton during brain development and in the adult brain. TH has also been demonstrated to modulate transcription of genes of intermediate filaments, namely neurofilaments (Nefh and Nefm), GFAP in mature astrocytes, vimentin in mesenchymal cells and nestin (116, 122). Experiments in cerebral cortex slices suggest that TH activates phosphorylation of cytoskeletal proteins mediated by GABAergic signaling dependent on PKA and PKCaMII activity (220). Studies conducted in cultured glial cells also suggests that TH reorganize the cytoskeleton through GFAP phosphorylation mediated by RhoA signaling pathway (221).
Actions of TH in the cytoskeleton are particularly important during brain development, to guarantee proper cell migration and to foster neurite outgrowth (160). T3 also regulates transcription of genes involved in cytoskeleton formation in neurons and astrocytes, during fetal and postnatal brain development (74). Hypothyroidism leads to impaired actin cytoskeleton formation in neurons and astrocytes, affecting neuronal migration and neurite outgrowth. Both rT3 or T4 administration can restore polymerization of intracellular filaments F-actin (222, 223) and laminin (223, 224), but this effect was not observed by T3 administration. Also in neuronal and astrocyte cultures, T4 and rT3 stimulate polymerization of the actin cytoskeleton, as already mentioned above (Figure 2) (159, 160, 162). As during brain development, basic transcription element-binding protein is upregulated by TH and this protein may play a role in neuronal outgrowth, modulating changes in the cytoskeleton, and cell differentiation (225, 226).
Studies also demonstrate that TH signaling is critical to proper functioning of short term (227) and long term synaptic plasticity (228). Induced hypothyroidism has been related with disruption in synaptic plasticity (229, 230) and long term potentiation in the CA1 neonatal (231, 232) and adult (233) rat hippocampus. Hyperthyroidism has been also related to detrimental effects in dendritic spines. Intraperitoneal injection of T3 750 μg/kg during 5 consecutive days in adult rats significantly decreased dendritic spine density in CA1 pyramidal cells in the hippocampus (234) and thyroxine induced hyperthyroidism impairs special learning and synaptic plasticity in rats (235).
On the cellular level, TH genomic or non-genomic actions may modulate the activity of ion pumps that are important for normal excitable cell function. Particularly in brain tissues affected by ischemia, directly or indirectly, adapted function of ion pumps is required to avoid intracellular overload of H+ and Ca2+, preventing cell acidosis and excitotoxicity. T3 has been shown to decrease the activity of Na, K-ATPase (154, 155) and to stimulate the Na+/H+ exchanger (149) and Ca2+/Mg2+ ATPase pump activity (158) in cerebrocortical synaptosomes. T3 increases the transcription of SR Ca2+-ATPase gene (ATP2A2) in the sarcoplasmic reticulum (157). In addition, T3 has been demonstrated to be benefical in in vitro and in vivo experimental myocardial ischemia preventing excessive intracellular Ca2+ accumulation (236, 237).
Also, T3 contributes to glutamate uptake by astrocytes, protecting neurons from intracellular calcium toxicity and death (203). Neuroprotective effect was attributed to an increased expression of mRNA and protein levels of GLT-1 and GLAST in the astrocytes. It also has been demonstrated that T3 decreases N-methyl-d-aspartate (NMDA)-evoked currents and prevent glutamate-induced neuronal death in hippocampal neurons (202). Together, these actions might be beneficial to prevent cell dysfunction or death of principal neurons during the acute phase after ischemic stroke. Conversely, these mechanisms might be involved to reduce the activity of inhibitory neurons in critical periods of plasticity during the first weeks after stroke. Hence, these actions most likely will dependent on receptor expression profiles in different neuron populations.
The balance between excitation and inhibition is of particular importance for neuronal plasticity processes potentially relevant for recovery. During development, TH increases the level of γ-aminobutyric acid (GABA) in the brain, while the opposite effect is observed in the adult brain. In the developing brain, hypothyroidism impairs the generation of interneurons including reduced proliferation and delayed differentiation of precursor cells in the cerebellum and their migration to the cerebellar cortex (238). These effects could be antagonized by administration of T3 binding to the TRα1. Likewise, deletion of TRα1 reduced cerebellar GAT-1 expression and Pax-2 precursor cell proliferation (238). TH also affect the release and uptake of GABA from the neuron into the synapse. T3 stimulates depolarization and release of GABA in synaptosomes from rat cerebral cortex (239). In the adult brain, hypothyroidism is reported to increase glutamic acid decarboxylase (GAD) activity and GABA reuptake, from cerebral cortex homogenates (240, 241) while hyperthyroidism has no effect on GABA uptake (241). In addition, T3 administration inhibits GABA-induced Cl− currents, which may affect GABAA receptors in the cerebral cortex, by non-genomic mechanisms (242).
Adult Neurogenesis
TH signaling is crucial for proper neurogenesis during brain development (1). Several studies have demonstrated that neurogenic events in the adult brain are dependent on TH actions (243–250) and have been reviewed in detail (251, 252). Particularly T3 is involved in mechanisms of proliferation, survival, differentiation and maturation of neuronal precursors in the adult brain (246, 251). With potential contribution of TH NSPCs from the SVZ may proliferate, migrate and differentiate into neurons, astrocytes or oligodendrocytes in the damaged region and thereby contribute to brain plasticity after ischemic stroke or other brain injury (253, 254). Stem cell therapy and neurogenesis have been explored as a potential therapeutic strategy for neuronal repair after ischemic stroke (255).
Angiogenesis
Therapeutic angiogenesis has been used to enhance brain repair promoting the formation of new blood vessels and restoration of blood flow in the damaged area (256–258). Angiogenic effects of TH have been demonstrated in infarcted tissue of the myocardium (259). Moreover, an increased number of new blood vessels has been found in the brain of hypothyroid rats after administration of 3,5-diiothyroprionic acid (a thyroid hormone analog) or T4 (260). The proangiogenic effects of TH are apparently mediated by non-genomic actions, through binding to integrin αvβ3 resulting in activation of MAPK 1/2 and STAT3. TH binding to αvβ3 directs transcription of genes that promote angiogenesis, namely fibroblast factors, VEGF and angiopoietin-2 (124–126, 137, 147, 261, 262). To our knowledge, so far no experimental studies have been performed to investigate pro-angiogenic effects of TH after stroke.
Translation to Clinical Studies
Current epidemiological studies in humans and experimental evidence from rodents strongly suggest that TH signaling plays a crucial for stroke recovery. In particular T3, the active form in the brain, exerts genomic and non-genomic actions that may foster functional outcome after stroke.
Although several epidemiological studies have associated low levels of TH with poor outcome, no clinical trials have been performed to evaluate the recovery promoting effects of TH in stroke patients. At the current stage the first step of translational studies will be to understand exact mechanisms underlying beneficial action of TH after stroke in animal models, in particular T3. Based on knowledge about mechanisms of action, exact treatment regimens with specific targets can be developed and tested during critical windows of stroke recovery. In this context, the development of cell-specific approaches to target TH signaling in the postischemic brain may result in specific treatments in experimental stroke models, that later, might be translated into clinical studies.
Conclusions
Several mechanisms in the brain are tightly regulated by TH and T3 availability to the brain is dependent on factors including (i) maternal TH release before fetus thyroid gland development; (ii) TSH levels and TH release by thyroid gland; (iii) passage of TH through placenta in the fetus; (iv) control of free fraction of TH determined by TH binding proteins; (v) TH transmembrane transport into the cytoplasm; (vi) local activity of iodothyronine deiodinases; (vii) expression and distribution of TR; (viii) and translational activity and non-genomic actions of TH in the brain. Disruption in these mechanisms compromise TH availability and actions in the brain and may result in impairments of neurological functions. There is clinical and preclinical evidence that TH are involved in mechanisms of neuronal plasticity and function of glial cells after ischemic stroke. Further understanding and targeting those might be exploited in future therapies to enhance functional recovery in stroke patients.
Author Contributions
DT conceptualized and wrote the review, under supervision of KR. KR, CS, and IG revised the manuscript. All authors approved the final version of the manuscript for submission and publication.
Funding
This work was supported by funds from the Health Sciences Research Center (CICS-UBI) through National Funds by FCT—Foundation for Science and Technology (UID/Multi/00709/2019); by a doctoral fellowship (SFRH/BD/104679/2014) from Fundação para a Ciência e Tecnologia; partially supported by Programa Operacional do Centro, Centro 2020 through the funding of the ICON project (Interdisciplinary Challenges on Neurodegeneration; CENTRO-01-0145-FEDER-000013); the Swedish Brain Fund; the Crafoord Foundation, Sveriges Stroke Riksförbundet; the Hans-Gabriel and Alice Wachtmeister Foundation, Sparbanksstiftelsen Färs & Frosta and the Stiftelsen Sven-Olof Jansons livsverk.
Conflict of Interest
The authors declare that the research was conducted in the absence of any commercial or financial relationships that could be construed as a potential conflict of interest.
References
1. Bernal J. Thyroid hormones in brain development and function. In: Feingold KR, Anawalt B, Boyce A, editors. Endotext. South Dartmouth, MA: MDText.com, Inc. (2000) 1–136.
2. Gothié JD, Demeneix B, Remaud S. Comparative approaches to understanding thyroid hormone regulation of neurogenesis. Mol Cell Endocrinol. (2017) 459:104–15. doi: 10.1016/j.mce.2017.05.020
3. Hartley MD, Banerji T, Tagge IJ, Kirkemo LL, Chaudhary P, Calkins E, et al. Myelin repair stimulated by CNS-selective thyroid hormone action. JCI Insight. (2019) 4:e126329. doi: 10.1172/jci.insight.126329
4. Moog NK, Entringer S, Heim C, Wadhwa PD, Kathmann N, Buss C. Influence of maternal thyroid hormones during gestation on fetal brain development. Neuroscience. (2017) 342:68–100. doi: 10.1016/j.neuroscience.2015.09.070
5. Morreale de Escobar G, Obregon MJ, Escobar del Rey F. Role of thyroid hormone during early brain development. Eur J Endocrinol. (2004) 151:U25–37. doi: 10.1530/EJE.0.151U025
6. Bárez-López S, Guadaño-Ferraz A. Thyroid hormone availability and action during brain development in rodents. Front Cell Neurosci. (2017) 11:240. doi: 10.3389/fncel.2017.00240
7. Mullur R, Liu YY, Brent GA. Thyroid hormone regulation of metabolism. Physiol Rev. (2014) 94:355–82. doi: 10.1152/physrev.00030.2013
8. Ahmed OM, El-Gareib AW, El-bakry AM, Abd El-Tawab SM, Ahmed RG. Thyroid hormones states and brain development interactions. Int J Dev Neurosci. (2008) 26:147–209. doi: 10.1016/j.ijdevneu.2007.09.011
9. Mendel CM, Weisiger RA, Jones AL, Cavalieri RR. Thyroid hormone-binding proteins in plasma facilitate uniform distribution of thyroxine within tissues: a perfused rat liver study. Endocrinology. (1987) 120:1742–9. doi: 10.1210/endo-120-5-1742
10. Palha JA. Transthyretin as a thyroid hormone carrier: function revisited. Clin Chem Lab Med. (2002) 40:1292–300. doi: 10.1515/CCLM.2002.223
11. Refetoff S. Thyroid hormone serum transport proteins. In: Feingold KR, Anawalt B, Boyce A, editors. Endotext. South Dartmouth. MA: MDText.com, Inc. (2015). p. 1–13.
12. Schreiber G, Richardson SJ. The Evolution of Gene Expression, Structure and Function of Transthyretin. Comp Biochem Physiol Part B Biochem Mol Biol. (1997) 116:137–60. doi: 10.1016/S0305-0491(96)00212-X
13. Vranckx R, Rouaze M, Savu L, Nunez EA, Beaumont C, Flink IL. The hepatic biosynthesis of rat thyroxine binding globulin (TBG): demonstration, ontogenesis, and UP-regulation in experimental hypothyroidism. Biochem Biophys Res Commun. (1990) 167:317–22. doi: 10.1016/0006-291X(90)91767-M
14. Vranckx R, Savu L, Maya M, Nunez EA. Characterization of a major development-regulated serum thyroxine-binding globulin in the euthyroid mouse. Biochem J. (1990) 271:373–9. doi: 10.1042/bj2710373
15. Benvenga S, Cahnmann HJ, Robbins J. Localization of the thyroxine binding sites in apolipoprotein B-100 of human low density lipoproteins. Endocrinology. (1990) 127:2241–6. doi: 10.1210/endo-127-5-2241
16. Benvenga S, Lapa D, Trimarchi F. Re-evaluation of the thyroxine binding to human plasma lipoproteins using three techniques. J Endocrinol Invest. (2001) 24:RC16–8. doi: 10.1007/BF03343863
17. Bernal J, Guadaño-Ferraz A, Morte B. Thyroid hormone transporters—functions and clinical implications. Nat Rev Endocrinol. (2015) 11:1–12. doi: 10.1038/nrendo.2015.66
18. Morte B, Bernal J. Thyroid hormone action: astrocyte-neuron communication. Front Endocrinol. (2014) 5:82. doi: 10.3389/fendo.2014.00082
19. Chanoine JP, Alex S, Fang SL, Stone S, Leonard JL, Korhle J, et al. Role of transthyretin in the transport of thyroxine from the blood to the choroid plexus, the cerebrospinal fluid, and the brain. Endocrinology. (1992) 130:933–8. doi: 10.1210/endo.130.2.1733735
20. Richardson SJ, Wijayagunaratne RC, D'Souza DG, Darras VM, Van Herck SLJ. Transport of thyroid hormones via the choroid plexus into the brain: the roles of transthyretin and thyroid hormone transmembrane transporters. Front. Neurosci. (2015) 9:66. doi: 10.3389/fnins.2015.00066
21. Friesema EC, Ganguly S, Abdalla A, Manning Fox JE, Halestrap AP, Visser TJ. Identification of monocarboxylate transporter 8 as a specific thyroid hormone transporter. J Biol Chem. (2003) 278:40128–35. doi: 10.1074/jbc.M300909200
22. Friesema EC, Jansen J, Milici C, Visser TJ. Thyroid hormone transporters. Vitam Horm. (2005) 70:137–67. doi: 10.1016/S0083-6729(05)70005-4
23. Hagenbuch B. Cellular entry of thyroid hormones by organic anion transporting polypeptides. Best Pract Res Clin Endocrinol Metab. (2007) 21:209–21. doi: 10.1016/j.beem.2007.03.004
24. Halestrap AP. The monocarboxylate transporter family-Structure and functional characterization. IUBMB Life. (2012) 64:1–9. doi: 10.1002/iub.573
25. Halestrap AP, Wilson MC. The monocarboxylate transporter family-Role and regulation. IUBMB Life. (2012) 64:109–19. doi: 10.1002/iub.572
26. Jansen J, Friesema EC, Milici C, Visser TJ. Thyroid Hormone Transporters in Health and Disease. Thyroid. (2005) 15:757–68. doi: 10.1089/thy.2005.15.757
27. Kinne A, Schülein R, Krause G. Primary and secondary thyroid hormone transporters. Thyroid Res. (2011) 4:S7. doi: 10.1186/1756-6614-4-S1-S7
28. Roberts LM, Woodford K, Zhou M, Black DS, Haggerty JE, Tate EH, et al. Expression of the thyroid hormone transporters monocarboxylate transporter-8 (SLC16A2) and organic ion transporter-14 (SLCO1C1) at the blood-brain barrier. Endocrinology. (2008) 149:6251–61. doi: 10.1210/en.2008-0378
29. Van Der Deure WM, Peeters RP, Visser TJ. Molecular aspects of thyroid hormone transporters, including MCT8, MCT10, and OATPs, and the effects of genetic variation in these transporters. J Mol Endocrinol. (2010) 44:1–11. doi: 10.1677/JME-09-0042
30. Visser WE, Friesema EC, Jansen J, Visser TJ. Thyroid hormone transport by monocarboxylate transporters. Best Pract Res Clin Endocrinol Metab. (2007) 21:223–36. doi: 10.1016/j.beem.2007.03.008
31. Visser WE, Friesema EC, Visser TJ. Minireview: thyroid hormone transporters: the knowns and the unknowns. Mol Endocrinol. (2011) 25:1–14. doi: 10.1210/me.2010-0095
32. Trajkovic M, Visser TJ, Mittag J, Horn S, Lukas J, Darras VM, et al. Abnormal thyroid hormone metabolism in mice lacking the monocarboxylate transporter 8. J Clin Invest. (2007) 117:627–35. doi: 10.1172/JCI28253
33. Friesema EC, Jansen J, Jachtenberg J, Visser WE, Kester MHA, Visser TJ. Effective cellular uptake and efflux of thyroid hormone by human monocarboxylate transporter 10. Mol Endocrinol. (2008) 22:1357–69. doi: 10.1210/me.2007-0112
34. Zhang Y, Chen K, Sloan SA, Bennett ML, Scholze AR, O'Keeffe S, et al. An RNA-sequencing transcriptome and splicing database of glia, neurons, and vascular cells of the cerebral cortex. J Neurosci. (2014) 34:11929–47. doi: 10.1523/JNEUROSCI.1860-14.2014
35. Mathiisen TM, Lehre KP, Danbolt NC, Ottersen OP. The perivascular astroglial sheath provides a complete covering of the brain microvessels: an electron microscopic 3D reconstruction. Glia. (2010) 58:1094–103. doi: 10.1002/glia.20990
36. Schroeder AC, Privalsky ML. Thyroid hormones, T3 and T4, in the brain. Front Endocrinol. (2014) 5:40. doi: 10.3389/fendo.2014.00040
37. Dumitrescu AM, Liao XH, Best TB, Brockmann K, Refetoff S. A novel syndrome combining thyroid and neurological abnormalities is associated with mutations in a monocarboxylate transporter gene. Am J Hum Genet. (2004) 74:168–75. doi: 10.1086/380999
38. Friesema EC, Grueters A, Biebermann H, Krude H, Von Moers A, Reeser M, et al. Association between mutations in a thyroid hormone transporter and severe X-linked psychomotor retardation. Lancet. (2004) 364:1435–7. doi: 10.1016/S0140-6736(04)17226-7
39. López-Espíndola D, Morales-Bastos C, Grijota-Martínez C, Liao XH, Lev D, Sugo E, et al. Mutations of the thyroid hormone transporter MCT8 cause prenatal brain damage and persistent hypomyelination. J Clin Endocrinol Metab. (2014) 99:E2799–E2804. doi: 10.1210/jc.2014-2162
40. Schwartz CE, May MM, Carpenter NJ, Rogers RC, Martin J, Bialer MG, et al. Allan-Herndon-Dudley syndrome and the monocarboxylate transporter 8 (MCT8) gene. Am J Hum Genet. (2005) 77:41–53. doi: 10.1086/431313
41. Kim DK, Kanai Y, Chairoungdua A, Matsuo H, Cha SH, Endou H. Expression cloning of a Na+-independent aromatic amino acid transporter with structural similarity to H+/monocarboxylate transporters. J Biol Chem. (2001) 276:17221–8. doi: 10.1074/jbc.M009462200
42. Bianco AC. Minireview: cracking the metabolic code for thyroid hormone signaling. Endocrinology. (2011) 152:3306–11. doi: 10.1210/en.2011-1104
43. Bianco AC, Kim BW. Deiodinases: implications of the local control of thyroid hormone action. J Clin Invest. (2006) 116:2571–9. doi: 10.1172/JCI29812
44. Dentice M, Marsili A, Zavacki A, Larsen PR, Salvatore D. The deiodinases and the control of intracellular thyroid hormone signaling during cellular differentiation. Biochim Biophys Acta Gen Subj. (2013) 1830:3937–45. doi: 10.1016/j.bbagen.2012.05.007
45. Tu HM, Kim SW, Salvatore D, Bartha T, Legradi G, Larsen PR, et al. Regional distribution of type 2 thyroxine deiodinase messenger ribonucleic acid in rat hypothalamus and pituitary and its regulation by thyroid hormone. Endocrinology. (1997) 138:3359–68. doi: 10.1210/endo.138.8.5318
46. Freitas BC, Gereben B, Castillo M, Kalló I, Zeöld A, Egri P, et al. Paracrine signaling by glial cell-derived triiodothyronine activates neuronal gene expression in the rodent brain and human cells. J Clin Invest. (2010) 120:2206–17. doi: 10.1172/JCI41977
47. Gereben B, Zavacki AM, Ribich S, Kim BW, Huang SA, Simonides WS, et al. Cellular and molecular basis of deiodinase-regulated thyroid hormone signaling. Endocr Rev. (2008) 29:898–938. doi: 10.1210/er.2008-0019
48. Tu HM, Legradi G, Bartha T, Salvatore D, Lechan RM, Larsen PR. Regional expression of the type 3 iodothyronine deiodinase messenger ribonucleic acid in the rat central nervous system and its regulation by thyroid hormone. Endocrinology. (1999) 140:784–90. doi: 10.1210/endo.140.2.6486
49. Dumitrescu AM, Liao XH, Weiss RE, Millen K, Refetoff S. Tissue-specific thyroid hormone deprivation and excess in monocarboxylate transporter (Mct) 8-deficient mice. Endocrinology. (2006) 147:4036–43. doi: 10.1210/en.2006-0390
50. Guadaño-Ferraz A, Obregón MJ, St Germain DL, Bernal J, Obregon MJ, StGermain DL. The type 2 iodothyronine deiodinase is expressed primarily in glial cells in the neonatal rat brain. Proc Natl Acad Sci USA. (1997) 94:10391–6. doi: 10.1073/pnas.94.19.10391
51. Cramer SC, Chopp M. Recovery recapitulates ontogeny. Trends Neurosci. (2000) 23:265–71. doi: 10.1016/S0166-2236(00)01562-9
52. Stepien BK, Huttner WB. Transport, metabolism, and function of thyroid hormones in the developing mammalian brain. Front Endocrinol. (2019) 10:209. doi: 10.3389/fendo.2019.00209
53. Anderson GW, Schoonover CM, Jones SA. Control of thyroid hormone action in the developing rat brain. Thyroid. (2003) 13:1039–56. doi: 10.1089/105072503770867219
54. Clancy B, Finlay BL, Darlington RB, Anand KJ. Extrapolating brain development from experimental species to humans. Neurotoxicology. (2007) 28:931–7. doi: 10.1016/j.neuro.2007.01.014
55. Semple BD, Blomgren K, Gimlin K, Ferriero DM, Noble-Haeusslein LJ. Brain development in rodents and humans: identifying benchmarks of maturation and vulnerability to injury across species. Prog Neurobiol. (2013) 106–107:1–16. doi: 10.1016/j.pneurobio.2013.04.001
56. Berbel P, Mestre JL, Santamaría A, Palazón I, Franco A, Graells M, et al. Delayed neurobehavioral development in children born to pregnant women with mild hypothyroxinemia during the first month of gestation: the importance of early iodine supplementation. Thyroid. (2009) 19:511–9. doi: 10.1089/thy.2008.0341
57. Carreón-Rodríguez A, Perez-Martínez L. Clinical implications of thyroid hormones effects on nervous system development. Pediatr Endocrinol Rev. (2012) 9:644–9.
58. Downing S, Halpern L, Carswell J, Brown RS. Severe maternal hypothyroidism corrected prior to the third trimester is associated with normal cognitive outcome in the offspring. Thyroid. (2012) 22:625–30. doi: 10.1089/thy.2011.0257
59. Contempré B, Jauniaux E, Calvo R, Jurkovic D, Campbell S, de Escobar GM. Detection of thyroid hormones in human embryonic cavities during the first trimester of pregnancy. J Clin Endocrinol Metab. (1993) 77:1719–22. doi: 10.1210/jcem.77.6.8263162
60. Obregon M, Calvo R, Escobar Del Rey F, Morreale De Escobar G. Ontogenesis of thyroid function and interactions with maternal function. Endocr Dev. (2007) 10:86–98. doi: 10.1159/000106821
61. Obregon MJ, Mallol J, Pastor R, Morreale de Escobar G, Escobar Del Rey F. L-thyroxine and 3,5,3'-triiodo-L-thyronine in rat embryos before onset of fetal thyroid function. Endocrinology. (1984) 114:305–7. doi: 10.1210/endo-114-1-305
62. Porterfield SP, Hendrich CE. Tissue iodothyronine levels in fetuses of control and hypothyroid rats at 13 and 16 days gestation. Endocrinology. (1992) 131:195–200. doi: 10.1210/endo.131.1.1611997
63. Woods RJ, Sinha AK, Ekins RP. Uptake and metabolism of thyroid hormones by the rat foetus in early pregnancy. Clin Sci. (1984) 67:359–63.
64. Morreale De Escobar GMD, Pastor R, Obregon MJ, Del Rey FE. Effects of maternal hypothyroidism on the weight and thyroid hormone content of rat embryonic tissues, before and after onset of fetal thyroid function. Endocrinology. (1985) 117:1890–900. doi: 10.1210/endo-117-5-1890
65. Ausó E, Lavado-Autric R, Cuevas E, Escobar Del Rey F, Morreale De Escobar G, Berbel P. A moderate and transient deficiency of maternal thyroid function at the beginning of fetal neocorticogenesis alters neuronal migration. Endocrinology. (2004) 145:4037–47. doi: 10.1210/en.2004-0274
66. Gilbert ME, Goodman JH, Gomez J, Johnstone AFM, Ramos RL. Adult hippocampal neurogenesis is impaired by transient and moderate developmental thyroid hormone disruption. Neurotoxicology. (2017) 59:9–21. doi: 10.1016/j.neuro.2016.12.009
67. Mohan V, Sinha RA, Pathak A, Rastogi L, Kumar P, Pal A, et al. Maternal thyroid hormone deficiency affects the fetal neocorticogenesis by reducing the proliferating pool, rate of neurogenesis and indirect neurogenesis. Exp Neurol. (2012) 237:477–88. doi: 10.1016/j.expneurol.2012.07.019
68. Bernal J. Thyroid hormone receptors in brain development and function. Nat Clin Pract Endocrinol Metab. (2007) 3:249–59. doi: 10.1038/ncpendmet0424
69. Bernal J, Pekonen F. Ontogenesis of the nuclear 3,5,3'-triiodothyronine receptor in the human fetal brain. Endocrinology. (1984) 114:677–9. doi: 10.1210/endo-114-2-677
70. Kilby MD, Gittoes N, McCabe C, Verhaeg J, Franklyn JA. Expression of thyroid receptor isoforms in the human fetal central nervous system and the effects of intrauterine growth restriction. Clin Endocrinol. (2000) 53:469–77. doi: 10.1046/j.1365-2265.2000.01074.x
71. Perez-Castillo A, Bernal J, Ferreiro B, Pans T. The early ontogenesis of thyroid hormone receptor in the rat fetus. Endocrinology. (1985) 117:2457–61. doi: 10.1210/endo-117-6-2457
72. Chatonnet F, Flamant F, Morte B. A temporary compendium of thyroid hormone target genes in brain. Biochim Biophys Acta Gene Regul Mech. (2015) 1849:122–9. doi: 10.1016/j.bbagrm.2014.05.023
73. Horn S, Heuer H. Thyroid hormone action during brain development: more questions than answers. Mol Cell Endocrinol. (2010) 315:19–26. doi: 10.1016/j.mce.2009.09.008
74. Sampson D, Pickard MR, Sinha AK, Evans IM, Leonard AJ, Ekins RP. Maternal thyroid status regulates the expression of neuronal and astrocytic cytoskeletal proteins in the fetal brain. J Endocrinol. (2000) 167:439–45. doi: 10.1677/joe.0.1670439
75. Bochukova E, Schoenmakers N, Agostini M, Schoenmakers E, Rajanayagam O, Keogh JM, et al. A mutation in the thyroid hormone receptor alpha gene. N Engl J Med. (2012) 366:243–9. doi: 10.1056/NEJMoa1110296
76. Forrest D, Golarai G, Connor J, Curran T. Genetic analysis of thyroid hormone receptors in development and disease. Recent Prog Horm Res. (1996) 51:1–22.
77. Jones I, Srinivas M, Ng L, Forrest D. The thyroid hormone receptor beta gene: structure and functions in the brain and sensory systems. Thyroid. (2003) 13:1057–68. doi: 10.1089/105072503770867228
78. Refetoff S, Dumitrescu AM. Syndromes of reduced sensitivity to thyroid hormone: genetic defects in hormone receptors, cell transporters and deiodination. Best Pract Res Clin Endocrinol Metab. (2007) 21:277–305. doi: 10.1016/j.beem.2007.03.005
79. van Mullem AA, Visser TJ, Peeters RP. Clinical consequences of mutations in thyroid hormone receptor-alpha1. Eur Thyroid J. (2014) 3:17–24. doi: 10.1159/000360637
80. Bradley DJ, Towle HC, Young WS. Spatial and temporal expression of alpha- and beta-thyroid hormone receptor mRNAs, including the beta 2-subtype, in the developing mammalian nervous system. J Neurosci. (1992) 12:2288–302.
81. Forrest D, Hallböök F, Persson H, Vennström B. Distinct functions for thyroid hormone receptors alpha and beta in brain development indicated by differential expression of receptor genes. EMBO J. (1991) 10:269–75.
82. Iskaros J, Pickard M, Evans I, Sinha A, Hardiman P, Ekins R. Thyroid hormone receptor gene expression in first trimester human fetal brain. J Clin Endocrinol Metab. (2000) 85:2620–3. doi: 10.1210/jcem.85.7.6766
83. Wallis K, Dudazy S, van Hogerlinden M, Nordström K, Mittag J, Vennström B. The thyroid hormone receptor α1 protein is expressed in embryonic postmitotic neurons and persists in most adult neurons. Mol Endocrinol. (2010) 24:1904–16. doi: 10.1210/me.2010-0175
84. Hodin RA, Lazar MA, Chin WW. Differential and tissue-specific regulation of the multiple rat c-erbA messenger RNA species by thyroid hormone. J Clin Invest. (1990) 85:101–5. doi: 10.1172/JCI114398
85. Sinha R, Yen PM. Cellular action of thyroid hormone. In: Feingold KR, Anawalt B, Boyce A, editors. Endotext. South Dartmouth, MA: MDTextcom, Inc. (2013). p. 1–27. doi: 10.1089/105072502760143827
86. Teng X, Lin YY, Teng W, Brent GA. COUP-TF1 modulates thyroid hormone action in an embryonic stem-cell model of cortical pyramidal neuronal differentiation. Thyroid. (2018) 28:667–78. doi: 10.1089/thy.2017.0256
87. Faedo A, Tomassy GS, Ruan Y, Teichmann H, Krauss S, Pleasure SJ, et al. COUP-TFI coordinates cortical patterning, neurogenesis, and laminar fate and modulates MAPK/ERK, AKT, and beta-catenin signaling. Cereb Cortex. (2008) 18:2117–31. doi: 10.1093/cercor/bhm238
88. Cheng SY, Leonard JL, Davis PJ. Molecular aspects of thyroid hormone actions. Endocr Rev. (2010) 31:139–70. doi: 10.1210/er.2009-0007
89. Yen PM. Physiological and molecular basis of thyroid hormone action. Physiol Rev. (2001) 81:1097–142. doi: 10.1152/physrev.2001.81.3.1097
90. Bernal J. Action of thyroid hormone in brain. J Endocrinol Invest. (2002) 25:268–88. doi: 10.1007/BF03344003
91. Harvey CB, Williams GR. Mechanism of thyroid hormone action. Thyroid. (2002) 12:441–6. doi: 10.1089/105072502760143791
92. McKenna NJ, Lanz RB, O'Malley BW. Nuclear receptor coregulators: cellular and molecular biology. Endocr Rev. (1999) 20:321–44. doi: 10.1210/edrv.20.3.0366
93. Brent GA. Mechanisms of thyroid hormone action. J Clin Invest. (2012) 122:3035–43. doi: 10.1172/JCI60047
94. Flamant F, Gauthier K. Thyroid hormone receptors: the challenge of elucidating isotype-specific functions and cell-specific response. Biochim Biophys Acta Gen Subj. (2013) 1830:3900–7. doi: 10.1016/j.bbagen.2012.06.003
95. Chan IH, Privalsky ML. Isoform-specific transcriptional activity of overlapping target genes that respond to thyroid hormone receptors alpha1 and beta1. Mol Endocrinol. (2009) 23:1758–75. doi: 10.1210/me.2009-0025
96. Schwartz HL, Strait KA, Ling NC, Oppenheimer JH. Quantitation of rat tissue thyroid hormone binding receptor isoforms by immunoprecipitation of nuclear triiodothyronine binding capacity. J Biol Chem. (1992) 267:11794–9.
97. Gil-Ibañez P, Morte B, Bernal J. Role of thyroid hormone receptor subtypes α and β on gene expression in the cerebral cortex and striatum of postnatal mice. Endocrinology. (2013) 154:1940–7. doi: 10.1210/en.2012-2189
98. Constantinou C, Margarity M, Valcana T. Region-specific effects of hypothyroidism on the relative expression of thyroid hormone receptors in adult rat brain. Mol Cell Biochem. (2005) 278:93–100. doi: 10.1007/s11010-005-6934-z
99. Carlson DJ, Strait KA, Schwartz HL, Oppenheimer JH. Immunofluorescent localization of thyroid hormone receptor isoforms in glial cells of rat brain. Endocrinology. (1994) 135:1831–6. doi: 10.1210/endo.135.5.7525253
100. Mohácsik P, Zeöld A, Bianco AC, Gereben B. Thyroid hormone and the neuroglia: Both source and target. J Thyroid Res. (2011) 2011:16. doi: 10.4061/2011/215718
101. Yusta B, Besnard F, Ortiz-Caro J, Pascual A, Aranda A, Sarlieve L. Evidence for the presence of nuclear 3,5,3'- triiodothyronine receptors in secondary cultures of pure rat oligodendrocytes. Endocrinology. (1988) 122:2278–84. doi: 10.1210/endo-122-5-2278
102. Bradley DJ, Young WS, Weinberger C. Differential expression of alpha and beta thyroid hormone receptor genes in rat brain and pituitary. Proc Natl Acad Sci USA. (1989) 86:7250–4. doi: 10.1073/pnas.86.18.7250
103. Cook CB, Kakucska I, Lechan RM, Koenig RJ. Expression of thyroid hormone receptor beta 2 in rat hypothalamus. Endocrinology. (1992) 130:1077–9. doi: 10.1210/endo.130.2.1733708
104. Lechan RM, Qi Y, Berrodin TJ, Davis KD, Schwartz HL, Strait KA, et al. Immunocytochemical delineation of thyroid hormone receptor beta 2-like immunoreactivity in the rat central nervous system. Endocrinology. (1993) 132:2461–9. doi: 10.1210/endo.132.6.7684976
105. Williams GR. Cloning and characterization of two novel thyroid hormone receptor beta isoforms. Mol Cell Biol. (2000) 20:8329–42. doi: 10.1128/MCB.20.22.8329-8342.2000
106. Anyetei-Anum CS, Roggero VR, Allison LA. Thyroid hormone receptor localization in target tissues. J Endocrinol. (2018) 237:R19–34. doi: 10.1530/JOE-17-0708
107. Li J, Donangelo I, Abe K, Scremin O, Ke S, Li F, et al. Thyroid hormone treatment activates protective pathways in both in vivo and in vitro models of neuronal injury. Mol Cell Endocrinol. (2017) 452:120–30. doi: 10.1016/j.mce.2017.05.023
108. Mdzinarishvili A, Sutariya V, Talasila PK, Geldenhuys WJ, Sadana P. Engineering triiodothyronine (T3) nanoparticle for use in ischemic brain stroke. Drug Deliv Transl Res. (2013) 3:309–17. doi: 10.1007/s13346-012-0117-8
109. Sadana P, Coughlin L, Burke J, Woods R, Mdzinarishvili A. Anti-edema action of thyroid hormone in MCAO model of ischemic brain stroke: possible association with AQP4 modulation. J Neurol Sci. (2015) 354:37–45. doi: 10.1016/j.jns.2015.04.042
110. Shulga A, Blaesse A, Kysenius K, Huttunen HJ, Tanhuanpää K, Saarma M, et al. Thyroxin regulates BDNF expression to promote survival of injured neurons. Mol Cell Neurosci. (2009) 42:408–18. doi: 10.1016/j.mcn.2009.09.002
111. De Arrieta CM, Morte B, Coloma A, Bernal J. The human RC3 gene homolog, NRGN contains a thyroid hormone-responsive element located in the first intron. Endocrinology. (1999) 140:335–43. doi: 10.1210/en.140.1.335
112. Iniguez MA, De Lecea L, Guadano-Ferraz A, Morte B, Gerendasy D, Sutcliffe JG, et al. Cell-specific effects of thyroid hormone on RC3/neurogranin expression in rat brain. Endocrinology. (1996) 137:1032–41. doi: 10.1210/endo.137.3.8603571
113. Miyakawa T, Yared E, Pak JH, Huang FL, Huang KP, Crawley JN. Neurogranin null mutant mice display performance deficits on spatial learning tasks with anxiety related components. Hippocampus. (2001) 11:763–75. doi: 10.1002/hipo.1092
114. Krebs J, Honegger P. Calmodulin kinase IV: Expression and function during rat brain development. Biochim Biophys Acta Mol Cell Res. (1996) 1313:217–22. doi: 10.1016/0167-4889(96)00092-4
115. Liu YY, Brent GA. Thyroid hormone-dependent gene expression in differentiated embryonic stem cells and embryonal carcinoma cells: identification of novel thyroid hormone target genes by deoxyribonucleic acid microarray analysis. Endocrinology. (2005) 146:776–83. doi: 10.1210/en.2004-1177
116. Morte B, Díez D, Ausó E, Belinchón MM, Gil-Ibáñez P, Grijota-Martínez C, et al. Thyroid hormone regulation of gene expression in the developing rat fetal cerebral cortex: prominent role of the Ca2+/calmodulin-dependent protein kinase IV pathway. Endocrinology. (2010) 151:810–20. doi: 10.1210/en.2009-0958
117. Navarro D, Alvarado M, Morte B, Berbel D, Sesma J, Pacheco P, et al. Late maternal hypothyroidism alters the expression of CAMK4 in neocortical subplate neurons: a comparison with nurr1 labeling. Cereb Cortex. (2014) 24:2694–706. doi: 10.1093/cercor/bht129
118. Alvarez-Dolado M, Ruiz M, Del Río JA, Alcántara S, Burgaya F, Sheldon M, et al. Thyroid hormone regulates reelin and dab1 expression during brain development. J. Neurosci. (1999) 19:6979–93.
119. Herz J, Chen Y. Reelin, lipoprotein receptors and synaptic plasticity. Nat Rev Neurosci. (2006) 7:850–9. doi: 10.1038/nrn2009
120. Jossin Y, Cooper JA. Reelin, Rap1 and N-cadherin orient the migration of multipolar neurons in the developing neocortex. Nat Neurosci. (2011) 14:697–703. doi: 10.1038/nn.2816
121. Thompson CC, Potter GB. Thyroid hormone action in neural development. Cereb Cortex. (2000) 10:939–45. doi: 10.1093/cercor/10.10.939
122. Morte B, Ceballos A, Diez D, Grijota-Martínez C, Dumitrescu AM, Di Cosmo C, et al. Thyroid hormone-regulated mouse cerebral cortex genes are differentially dependent on the source of the hormone: a study in monocarboxylate transporter-8- and deiodinase-2-deficient mice. Endocrinology. (2010) 151:2381–7. doi: 10.1210/en.2009-0944
123. Morte B, Gil-Ibáñez P, Bernal J. Regulation of gene expression by thyroid hormone in primary astrocytes: factors influencing the genomic response. Endocrinology. (2018) 159:2083–92. doi: 10.1210/en.2017-03084
124. Davis FB, Mousa SA, O'Connor L, Mohamed S, Lin HY, Cao HJ, et al. Proangiogenic action of thyroid hormone is fibroblast growth factor-dependent and is initiated at the cell surface. Circ Res. (2004) 94:1500–6. doi: 10.1161/01.RES.0000130784.90237.4a
125. Liu X, Zheng N, Shi YN, Yuan J, Li L. Thyroid hormone induced angiogenesis through the integrin αvβ3/protein kinase D/histone deacetylase 5 signaling pathway. J Mol Endocrinol. (2014) 52:245–54. doi: 10.1530/JME-13-0252
126. Luidens MK, Mousa SA, Davis FB, Lin HY, Davis PJ. Thyroid hormone and angiogenesis. Vascul Pharmacol. (2010) 52:142–5. doi: 10.1016/j.vph.2009.10.007
127. Davis PJ, Leonard JL, Davis FB. Mechanisms of nongenomic actions of thyroid hormone. Front Neuroendocrinol. (2008) 29:211–8. doi: 10.1016/j.yfrne.2007.09.003
128. Davis PJ, Goglia F, Leonard JL. Nongenomic actions of thyroid hormone. Nat Rev Endocrinol. (2016) 12:111–21. doi: 10.1038/nrendo.2015.205
129. Hiroi Y, Kim HH, Ying H, Furuya F, Huang Z, Simoncini T, et al. Rapid nongenomic actions of thyroid hormone. Proc Natl Acad Sci USA. (2006) 103:14104–9. doi: 10.1073/pnas.0601600103
130. Osmak-Tizon L, Poussier M, Cottin Y, Rochette L. Non-genomic actions of thyroid hormones: molecular aspects. Arch Cardiovasc Dis. (2014) 107:207–11. doi: 10.1016/j.acvd.2014.02.001
131. Cao X, Kambe F, Moeller LC, Refetoff S, Seo H. Thyroid hormone induces rapid activation of Akt/protein kinase B-mammalian target of rapamycin-p70S6K cascade through phosphatidylinositol 3-kinase in human fibroblasts. Mol Endocrinol. (2005) 19:102–12. doi: 10.1210/me.2004-0093
132. Furuya F, Guigon CJ, Zhao L, Lu C, Hanover JA, Cheng S. Nuclear receptor corepressor is a novel regulator of phosphatidylinositol 3-kinase signaling. Mol Cell Biol. (2007) 27:6116–26. doi: 10.1128/MCB.00900-07
133. Lin HY, Sun M, Tang HY, Lin C, Luidens MK, Mousa SA, et al. L-Thyroxine vs. 3,5,3'-triiodo-L-thyronine and cell proliferation: activation of mitogen-activated protein kinase and phosphatidylinositol 3-kinase. Am J Physiol Cell Physiol. (2009) 296:C980–91. doi: 10.1152/ajpcell.00305.2008
134. Martin NP, Marron De Velasco E, Mizuno F, Scappini EL, Gloss B, Erxleben C, et al. A rapid cytoplasmic mechanism for PI3 kinase regulation by the nuclear thyroid hormone receptor, TRβ, and genetic evidence for its role in the maturation of mouse hippocampal synapses in vivo. Endocrinology. (2014) 155:3713–24. doi: 10.1210/en.2013-2058
135. Moeller LC, Cao X, Dumitrescu AM, Seo H, Refetoff S. Thyroid hormone mediated changes in gene expression can be initiated by cytosolic action of the thyroid hormone receptor beta through the phosphatidylinositol 3-kinase pathway. Nucl Recept Signal. (2006) 4:e020. doi: 10.1621/nrs.04020
136. Storey NM, Gentile S, Ullah H, Russo A, Muessel M, Erxleben C, et al. Rapid signaling at the plasma membrane by a nuclear receptor for thyroid hormone. Proc Natl Acad Sci USA. (2006) 103:5197–201. doi: 10.1073/pnas.0600089103
137. Bergh JJ, Lin HY, Lansing L, Mohamed SN, Davis FB, Mousa S, et al. Integrin alphaVbeta3 contains a cell surface receptor site for thyroid hormone that is linked to activation of mitogen-activated protein kinase and induction of angiogenesis. Endocrinology. (2005) 146:2864–71. doi: 10.1210/en.2005-0102
138. Davis PJ, Davis FB, Cody V. Membrane receptors mediating thyroid hormone action. Trends Endocrinol Metab. (2005) 16:429–35. doi: 10.1016/j.tem.2005.09.007
139. Davis PJ, Davis FB, Mousa SA, Luidens MK, Lin HY. Membrane receptor for thyroid hormone: physiologic and pharmacologic implications. Annu Rev Pharmacol Toxicol. (2011) 51:99–115. doi: 10.1146/annurev-pharmtox-010510-100512
140. Kalyanaraman H, Schwappacher R, Joshua J, Zhuang S, Scott BT, Klos M, et al. Nongenomic thyroid hormone signaling occurs through a plasma membrane-localized receptor. Sci Signal. (2014) 7:ra48. doi: 10.1126/scisignal.2004911
141. Cao HJ, Lin HY, Luidens MK, Davis FB, Davis PJ. Cytoplasm-to-nucleus shuttling of thyroid hormone receptor-β1 (TRβ1) is directed from a plasma membrane integrin receptor by thyroid hormone. Endocr Res. (2009) 34:31–42. doi: 10.1080/07435800902911810
142. Lin HY, Tang HY, Shih A, Keating T, Cao G, Davis PJ, et al. Thyroid hormone is a MAPK-dependent growth factor for thyroid cancer cells and is anti-apoptotic. Steroids. (2007) 72:180–7. doi: 10.1016/j.steroids.2006.11.014
143. Lin HY, Martino LJ, Wilcox BD, Davis FB, Gordinier JK, Davis PJ. Potentiation by thyroid hormone of human IFN-gamma-induced HLA-DR expression. J Immunol. (1998) 161:843–9.
144. Chen Y, Chen PL, Chen CF, Sharp ZD, Lee WH. Thyroid hormone, T3-dependent phosphorylation and translocation of Trip230 from the Golgi complex to the nucleus. Proc Natl Acad Sci USA. (1999) 96:4443–8. doi: 10.1073/pnas.96.8.4443
145. Davis PJ, Shih A, Lin HY, Martino LJ, Davis FB. Thyroxine promotes association of mitogen-activated protein kinase and nuclear thyroid hormone receptor (TR) and causes serine phosphorylation of TR. J Biol Chem. (2000) 275:38032–9. doi: 10.1074/jbc.M002560200
146. Lin HY, Davis FB, Gordinier JK, Martino LJ, Davis PJ. Thyroid hormone induces activation of mitogen-activated protein kinase in cultured cells. Am J Physiol Physiol. (1999) 276:C1014–24. doi: 10.1152/ajpcell.1999.276.5.C1014
147. Lin HY, Shih A, Davis FB, Davis PJ. Thyroid hormone promotes the phosphorylation of STAT3 and potentiates the action of epidermal growth factor in cultured cells. Biochem J. (1999) 338(Pt 2):427–32. doi: 10.1042/0264-6021:3380427
148. Shih A, Lin HY, Davis FB, Davis PJ. Thyroid hormone promotes serine phosphorylation of p53 by mitogen-activated protein kinase. Biochemistry. (2001) 40:2870–8. doi: 10.1021/bi001978b
149. Incerpi S, Luly P, De Vito P, Farias RN. Short-term effects of thyroid hormones on the Na/H antiport in L-6 myoblasts: high molecular specificity for 3,3',5-triiodo-L-thyronine. Endocrinology. (1999) 140:683–9. doi: 10.1210/endo.140.2.6535
150. Bhargava M, Lei J, Mariash CN, Ingbar DH. Thyroid hormone rapidly stimulates alveolar Na,K-ATPase by activation of phosphatidylinositol 3-kinase. Curr Opin Endocrinol Diabetes Obes. (2007) 14:416–20. doi: 10.1097/MED.0b013e3282f02ae8
151. Lei J, Mariash CN, Ingbar DH. 3,3',5-Triiodo-L-thyronine up-regulation of Na,K-ATPase activity and cell surface expression in alveolar epithelial cells is Src kinase- and phosphoinositide 3-kinase-dependent. J Biol Chem. (2004) 279:47589–600. doi: 10.1074/jbc.M405497200
152. Lei J, Mariash CN, Bhargava M, Wattenberg EV, Ingbar DH. T3 increases Na-K-ATPase activity via a MAPK/ERK1/2-dependent pathway in rat adult alveolar epithelial cells. Am J Physiol Lung Cell Mol Physiol. (2008) 294:L749–54. doi: 10.1152/ajplung.00335.2007
153. Scapin S, Leoni S, Spagnuolo S, Fiore AM, Incerpi S. Short-term effects of thyroid hormones on Na+-K+-ATPase activity of chick embryo hepatocytes during development: focus on signal transduction. Am J Physiol Cell Physiol. (2009) 296:C4–12. doi: 10.1152/ajpcell.90604.2007
154. Sarkar PK, Biswas A, Ray AK, Martin JV. Mechanisms of L-triiodothyronine-induced inhibition of synaptosomal na(+)-k(+)-ATPase activity in young adult rat brain cerebral cortex. J Thyroid Res. (2013) 2013:457953. doi: 10.1155/2013/457953
155. Sarkar PK, Ray AK. Specific binding of L-triiodothyronine modulates Na+-K+-ATPase activity in adult rat cerebrocortical synaptosomes. Neuroreport. (1998) 9:1149–52.
156. Davis PJ, Zhou M, Davis FB, Lansing L, Mousa SA, Lin HY. Mini-review: cell surface receptor for thyroid hormone and nongenomic regulation of ion fluxes in excitable cells. Physiol Behav. (2010) 99:237–9. doi: 10.1016/j.physbeh.2009.02.015
157. Kahaly GJ, Dillmann WH. Thyroid hormone action in the heart. Endocr Rev. (2005) 26:704–28. doi: 10.1210/er.2003-0033
158. Chakrabarti N, Ray AK. Stimulation of Ca2+/Mg2+-ATPase activity in adult rat cerebrocortical synaptosomes by 3-5-3′-L-triiodothyronine. Neurosci Res Commun. (2002) 31:193–201. doi: 10.1002/nrc.10052
159. Farwell AP, Lynch RM, Okulicz WC, Comi AM, Leonard JL. The actin cytoskeleton mediates the hormonally regulated translocation of type II iodothyronine 5'-deiodinase in astrocytes. J Biol Chem. (1990) 265:18546–553.
160. Farwell AP, Dubord-tomasetti SA, Pietrzykowski AZ, Stachelek SJ, Leonard JL. Regulation of cerebellar neuronal migration and neurite outgrowth by thyroxine and 3,3′,5′-triiodothyronine. Dev Brain Res. (2005) 154:121–35. doi: 10.1016/j.devbrainres.2004.07.016
161. Leonard JL, Farwell AP. Thyroid. hormone-regulated actin polymerization in brain. Thyroid. (1997) 7:147–51. doi: 10.1089/thy.1997.7.147
162. Siegrist-Kaiser CA, Juge-Aubry C, Tranter MP, Ekenbarger DM, Leonard JL. Thyroxine-dependent modulation of actin polymerization in cultured astrocytes. A novel, extranuclear action of thyroid hormone. J Biol Chem. (1990) 265:5296–302.
163. Campos Costa I, Nogueira Carvalho H, Fernandes L. Aging, circadian rhythms and depressive disorders: a review. Am J Neurodegener Dis. (2013) 2:228–46.
164. Froy O. Circadian rhythms, aging, and life span in mammals. Physiology. (2011) 26:225–35. doi: 10.1152/physiol.00012.2011
165. Morris CJ, Aeschbach D, Scheer FA. Circadian system, sleep and endocrinology. Mol Cell Endocrinol. (2012) 349:91–104. doi: 10.1016/j.mce.2011.09.003
166. Bensenor IM, Olmos RD, Lotufo PA. Hypothyroidism in the elderly: diagnosis and management. Clin Interv Aging. (2012) 7:97–111. doi: 10.2147/CIA.S23966
167. Chakraborti S, Chakraborti T, Mandal M, Das S, Batabyal SK. Hypothalamic-pituitary-thyroid axis status of humans during development of ageing process. Clin Chim Acta. (1999) 288:137–45. doi: 10.1016/S0009-8981(99)00061-3
168. Cao L, Jiang W, Wang F, Yang QG, Wang C, Chen YP, et al. The reduced serum free triiodothyronine and increased dorsal hippocampal SNAP-25 and Munc18-1 had existed in middle-aged CD-1 mice with mild spatial cognitive impairment. Brain Res. (2013) 1540:9–20. doi: 10.1016/j.brainres.2013.09.034
169. Visser WE, Bombardieri CR, Zevenbergen C, Barnhoorn S, Ottaviani A, Van Der Pluijm I, et al. Tissue-specific suppression of thyroid hormone signaling in various mouse models of aging. PLoS ONE. (2016) 11:1–25. doi: 10.1371/journal.pone.0149941
170. Fu AL, Zhou CY, Chen X. Thyroid hormone prevents cognitive deficit in a mouse model of Alzheimer's disease. Neuropharmacology. (2010) 58:722–9. doi: 10.1016/j.neuropharm.2009.12.020
171. Villanueva I, Alva-Sánchez C, Pacheco-Rosado J. The role of thyroid hormones as inductors of oxidative stress and neurodegeneration. Oxid Med Cell Longev. (2013) 2013:218145. doi: 10.1155/2013/218145
172. Qureshi AI, Suri FK, Nasar A, Kirmani JF, Divani AA, Giles WH. Free thyroxine index and risk of stroke: results from the National Health and Nutrition Examination Survey Follow-up Study. Med Sci Monit. (2006) 12:CR501–6.
173. Remmel KS, Wanahita A, Moore K, Gruenthal M. Acute ischemic stroke and hypothyroidism. J Ky Med Assoc. (2006) 104:191–3.
174. Dhital R, Poudel DR, Tachamo N, Gyawali B, Basnet S, Shrestha P, et al. Ischemic stroke and impact of thyroid profile at presentation: a systematic review and meta-analysis of observational studies. J Stroke Cerebrovasc Dis. (2017) 26:2926–34. doi: 10.1016/j.jstrokecerebrovasdis.2017.07.015
175. Jiang X, Xing H, Wu J, Du R, Liu H, Chen J, et al. Prognostic value of thyroid hormones in acute ischemic stroke – a meta analysis. Sci Rep. (2017) 7:16256. doi: 10.1038/s41598-017-16564-2
176. Lamba N, Liu C, Zaidi H, Broekman MLD, Simjian T, Shi C, et al. A prognostic role for Low tri-iodothyronine syndrome in acute stroke patients: a systematic review and meta-analysis. Clin Neurol Neurosurg. (2018) 169:55–63. doi: 10.1016/j.clineuro.2018.03.025
177. Liu J, Wang D, Xiong Y, Yuan R, Tao W, Liu M. Low free triiodothyronine levels are related to symptomatic intracranial hemorrhage and poor functional outcomes after intravenous thrombolysis in acute ischemic stroke patients. Neurol Res. (2016) 38:429–33. doi: 10.1080/01616412.2016.1178480
178. O'Keefe LM, Conway SE, Czap A, Malchoff CD, Benashski S, Fortunato G, et al. Thyroid hormones and functional outcomes after ischemic stroke. Thyroid Res. (2015) 8:9. doi: 10.1186/s13044-015-0021-7
179. Suda S, Muraga K, Kanamaru T, Okubo S, Abe A, Aoki J, et al. Low free triiodothyronine predicts poor functional outcome after acute ischemic stroke. J Neurol Sci. (2016) 368:89–93. doi: 10.1016/j.jns.2016.06.063
180. Li LQ, Xu XY, Li WY, Hu XY, Lv W. The prognostic value of total T3 after acute cerebral infarction is age-dependent: a retrospective study on 768 patients. BMC Neurol. (2019) 19:54. doi: 10.1186/s12883-019-1264-z
181. Hama S, Kitaoka T, Shigenobu M, Watanabe A, Imura I, Seno H, et al. Malnutrition and nonthyroidal illness syndrome after stroke. Metabolism. (2005) 54:699–704. doi: 10.1016/j.metabol.2004.11.016
182. Chaker L, Baumgartner C, Den Elzen WPJ, Collet TH, Ikram MA, Blum MR, et al. Thyroid function within the reference range and the risk of stroke: an individual participant data analysis. J Clin Endocrinol Metab. (2016) 101:4270–82. doi: 10.1210/jc.2016-2255
183. Akhoundi FH, Ghorbani A, Soltani A, Meysamie A. Favorable functional outcomes in acute ischemic stroke patients with subclinical hypothyroidism. Neurology. (2011) 77:349–54. doi: 10.1212/WNL.0b013e3182267ba0
184. Alevizaki M, Synetou M, Xynos K, Alevizaki CC, Vemmos KN. Hypothyroidism as a protective factor in acute stroke patients. Clin Endocrinol. (2006) 65:369–72. doi: 10.1111/j.1365-2265.2006.02606.x
185. Rastogi L, Godbole MM, Ray M, Rathore P, Rathore P, Pradhan S, et al. Reduction in oxidative stress and cell death explains hypothyroidism induced neuroprotection subsequent to ischemia/reperfusion insult. Exp Neurol. (2006) 200:290–300. doi: 10.1016/j.expneurol.2006.02.013
186. Shuaib A, Ijaz S, Mazagri R, Kalra J, Hemmings S, Senthilsvlan A, et al. Hypothyroidism protects the brain during transient forebrain ischemia in gerbils. Exp Neurol. (1994) 127:119–25. doi: 10.1006/exnr.1994.1085
187. Lee CH, Yoo KY, Hwang IK, Choi JH, Park OK, Li H, et al. Hypothyroid state does not protect but delays neuronal death in the hippocampal CA1 region following transient cerebral ischemia: focus on oxidative stress and gliosis. J Neurosci Res. (2010) 88:2661–8. doi: 10.1002/jnr.22436
188. Rastogi L, Godbole MM, Sinha RA, Pradhan S. Reverse triiodothyronine (rT3) attenuates ischemia-reperfusion injury. Biochem Biophys Res Commun. (2018) 506:597–603. doi: 10.1016/j.bbrc.2018.10.031
189. Lourbopoulos A, Mourouzis I, Karapanayiotides T, Nousiopoulou E, Chatzigeorgiou S, Mavridis T, et al. Changes in thyroid hormone receptors after permanent cerebral ischemia in male rats. J Mol Neurosci. (2014) 54:78–91. doi: 10.1007/s12031-014-0253-3
190. Bengtsson D, Brudin L, Wanby P, Carlsson M. Previously unknown thyroid dysfunction in patients with acute ischemic stroke. Acta Neurol Scand. (2012) 126:98–102. doi: 10.1111/j.1600-0404.2011.01604.x
191. Sheu JJ, Kang JH, Lin HC, Lin HC. Hyperthyroidism and risk of ischemic stroke in young adults: a 5-year follow-up study. Stroke. (2010) 41:961–6. doi: 10.1161/STROKEAHA.109.577742
192. Wollenweber FA, Zietemann V, Gschwendtner A, Opherk C, Dichgans M. Subclinical hyperthyroidism is a risk factor for poor functional outcome after ischemic stroke. Stroke. (2013) 44:1446–8. doi: 10.1161/STROKEAHA.113.000833
193. Keshavarz S, Dehghani GA. Cerebral ischemia/reperfusion injury in the hyperthyroid rat. Iran J Med Sci. (2017) 42, 48–56.
194. Margaill I, Royer J, Lerouet D, Ramaugé M, Le Goascogne C, Li WW, et al. Induction of type 2 iodothyronine deiodinase in astrocytes after transient focal cerebral ischemia in the rat. J Cereb Blood Flow Metab. (2005) 25:468–76. doi: 10.1038/sj.jcbfm.9600041
195. Rami A, Krieglstein J. Thyroxine attenuates hippocampal neuronal damage caused by ischemia in the rat. Life Sci. (1992) 50:645–50. doi: 10.1016/0024-3205(92)90251-J
196. Genovese T, Impellizzeri D, Ahmad A, Cornelius C, Campolo M, Cuzzocrea S, et al. Post-ischaemic thyroid hormone treatment in a rat model of acute stroke. Brain Res. (2013) 1513:92–102. doi: 10.1016/j.brainres.2013.03.001
197. Crupi R, Paterniti I, Campolo M, Di Paola R, Cuzzocrea S, Esposito E. Exogenous T3 administration provides neuroprotection in a murine model of traumatic brain injury. Pharmacol Res. (2013) 70:80–9. doi: 10.1016/j.phrs.2012.12.009
198. Sayre NL, Sifuentes M, Holstein D, Cheng SY, Zhu X, Lechleiter JD. Stimulation of astrocyte fatty acid oxidation by thyroid hormone is protective against ischemic stroke-induced damage. J Cereb Blood Flow Metab. (2017) 37:514–27. doi: 10.1177/0271678X16629153
199. Akhoundzadeh K, Vakili A, Sameni HR, Vafaei AA, Rashidy-Pour A, Safari M, et al. Effects of the combined treatment of bone marrow stromal cells with mild exercise and thyroid hormone on brain damage and apoptosis in a mouse focal cerebral ischemia model. Metab Brain Dis. (2017) 32:1267–77. doi: 10.1007/s11011-017-0034-0
200. Doyle KP, Suchland KL, Ciesielski TMP, Lessov NS, Grandy DK, Scanlan TS, et al. Novel thyroxine derivatives, thyronamine and 3-iodothyronamine, induce transient hypothermia and marked neuroprotection against stroke injury. Stroke. (2007) 38:2569–76. doi: 10.1161/STROKEAHA.106.480277
201. Costa LES, Clementino-Neto J, Mendes CB, Franzon NH, De Oliveira Costa E, Moura-Neto V, et al. Evidence of aquaporin 4 regulation by thyroid hormone during mouse brain development and in cultured human glioblastoma multiforme cells. Front Neurosci. (2019) 13:317. doi: 10.3389/fnins.2019.00317
202. Losi G, Garzon G, Puia G. Nongenomic regulation of glutamatergic neurotransmission in hippocampus by thyroid hormones. Neuroscience. (2008) 151:155–63. doi: 10.1016/j.neuroscience.2007.09.064
203. Mendes-de-Aguiar CBN, Alchini R, Decker H, Alvarez-Silva M, Tasca CI, Trentin AG. Thyroid hormone increases astrocytic glutamate uptake and protects astrocytes and neurons against glutamate toxicity. J Neurosci Res. (2008) 86:3117–25. doi: 10.1002/jnr.21755
204. Guo ZH, Li F, Wang WZ. The mechanisms of brain ischemic insult and potential protective interventions. Neurosci Bull. (2009) 25:139–52. doi: 10.1007/s12264-009-0104-3
205. Pantos C, Mourouzis I. Thyroid hormone receptor α1 as a novel therapeutic target for tissue repair. Ann Transl Med. (2018) 6:254. doi: 10.21037/atm.2018.06.12
206. Madinier A, Quattromani MJ, Sjölund C, Ruscher K, Wieloch T. Enriched housing enhances recovery of limb placement ability and reduces aggrecan-containing perineuronal nets in the rat somatosensory cortex after experimental stroke. PLoS ONE. (2014) 9:e93121. doi: 10.1371/journal.pone.0093121
207. Quattromani MJ, Cordeau P, Ruscher K, Kriz J, Wieloch T. Enriched housing down-regulates the Toll-like receptor 2 response in the mouse brain after experimental stroke. Neurobiol Dis. (2014) 66:66–73. doi: 10.1016/j.nbd.2014.02.010
208. Wieloch T, Nikolich K. Mechanisms of neural plasticity following brain injury. Curr Opin Neurobiol. (2006) 16:258–64. doi: 10.1016/j.conb.2006.05.011
209. Pekna M, Pekny M, Nilsson M. Modulation of neural plasticity as a basis for stroke rehabilitation. Stroke. (2012) 43:2819–28. doi: 10.1161/STROKEAHA.112.654228
210. Sharma N, Classen J, Cohen LG. Neural plasticity and its contribution to functional recovery. Handb Clin Neurol. (2013) 110:3–12. doi: 10.1016/B978-0-444-52901-5.00001-0
211. Zhong L, Gerges NZ. Neurogranin targets calmodulin and lowers the threshold for the induction of long-term potentiation. PLoS ONE. (2012) 7:e41275. doi: 10.1371/journal.pone.0041275
212. Sui L, Ren WW, Li BM. Administration of thyroid hormone increases reelin and brain-derived neurotrophic factor expression in rat hippocampus in vivo. Brain Res. (2010) 1313:9–24. doi: 10.1016/j.brainres.2009.12.010
213. Krebs J, Means RL, Honegger P. Induction of calmodulin kinase IV by the thyroid hormone during the development of rat brain. J Biol Chem. (1996) 271:11055–8. doi: 10.1074/jbc.271.19.11055
214. Redmond L, Kashani AH, Ghosh A. Calcium regulation of dendritic growth via CaM kinase IV and CREB-mediated transcription. Neuron. (2002) 34:999–1010. doi: 10.1016/S0896-6273(02)00737-7
215. Gordon-Weeks PR, Fournier AE. Neuronal cytoskeleton in synaptic plasticity and regeneration. J Neurochem. (2014) 129:206–12. doi: 10.1111/jnc.12502
216. Aniello F, Couchie D, Gripois D, Nunez J. Regulation of five tubulin isotypes by thyroid hormone during brain development. J Neurochem. (1991) 57:1781–6. doi: 10.1111/j.1471-4159.1991.tb06381.x
217. Lorenzo PI, Ménard C, Miller FD, Bernal J. Thyroid hormone-dependent regulation of Talpha1 alpha-tubulin during brain development. Mol Cell Neurosci. (2002) 19:333–43. doi: 10.1006/mcne.2001.1087
218. Silva JE, Rudas P. Effects of congenital hypothyroidism on microtubule- associated protein-2 expression in the cerebellum of the rat. Endocrinology. (1990) 126:1276–82. doi: 10.1210/endo-126-2-1276
219. Aniello F, Couchie D, Bridoux AM, Gripois D, Nunez J. Splicing of juvenile and adult tau mRNA variants is regulated by thyroid hormone. Proc Natl Acad Sci USA. (1991) 88:4035–9. doi: 10.1073/pnas.88.9.4035
220. Zamoner A, Funchal C, Heimfarth L, Silva FR, Pessoa-Pureur R. Short-term effects of thyroid hormones on cytoskeletal proteins are mediated by GABAergic mechanisms in slices of cerebral cortex from young rats. Cell Mol Neurobiol. (2006) 26:209–24. doi: 10.1007/s10571-006-9027-y
221. Zamoner A, Funchal C, Jacques-Silva MC, Gottfried C, Barreto Silva FR, Pessoa-Pureur R. Thyroid hormones reorganize the cytoskeleton of glial cells through Gfap phosphorylation and Rhoa-dependent mechanisms. Cell Mol Neurobiol. (2007) 27:845–65. doi: 10.1007/s10571-006-9084-2
222. Faivre-Sarrailh C, Rabié A. Developmental study of factors controlling microtubule in vitro cold-stability in rat cerebrum. Dev Brain Res. (1988) 42:199–204. doi: 10.1016/0165-3806(88)90238-6
223. Farwell AP, Dubord-Tomasetti SA, Pietrzykowski AZ, Leonard JL. Dynamic nongenomic actions of thyroid hormone in the developing rat brain. Endocrinology. (2006) 147:2567–74. doi: 10.1210/en.2005-1272
224. Farwell AP, Dubord-Tomasetti SA. Thyroid hormone regulates the expression of laminin in the developing rat cerebellum. Endocrinology. (1999) 140:4221–7. doi: 10.1210/endo.140.9.7007
225. Cayrou C, Denver RJ, Puymirat J. Suppression of the basic transcription element-binding protein in brain neuronal cultures inhibits thyroid hormone-induced neurite branching. Endocrinology. (2002) 143:2242–9. doi: 10.1210/en.143.6.2242
226. Martel J, Cayrou C, Puymirat J. Identification of new thyroid hormone-regulated genes in rat brain neuronal cultures. Neuroreporte. (2002) 13:1849–51. doi: 10.1097/00001756-200210280-00003
227. Vara H, Martínez B, Santos A, Colino A. Thyroid hormone regulates neurotransmitter release in neonatal rat hippocampus. Neuroscience. (2002) 110:19–28. doi: 10.1016/S0306-4522(01)00541-3
228. Liu W, Dong J, Wang Y, Xi Q, Chen J. Developmental iodine deficiency and hypothyroidism impaired in vivo synaptic plasticity and altered PKC activity and GAP-43 expression in rat hippocampus. Nutr Neurosci. (2010) 11:50. doi: 10.1179/147683010x12611460764525
229. Dong J, Yin H, Liu W, Wang P, Jiang Y, Chen J. Congenital iodine deficiency and hypothyroidism impair LTP and decrease c-fos and c-jun expression in rat hippocampus. Neurotoxicology. (2005) 26:417–26. doi: 10.1016/j.neuro.2005.03.003
230. Sui L, Gilbert ME. Pre- and postnatal propylthiouracil-induced hypothyroidism impairs synaptic transmission and plasticity in area CA1 of the neonatal rat hippocampus. Endocrinology. (2003) 144:4195–203. doi: 10.1210/en.2003-0395
231. Gerges NZ, Alkadhi KA. Hypothyroidism impairs late LTP in CA1 region but not in dentate gyrus of the intact rat hippocampus: MAPK involvement. Hippocampus. (2004) 14:40–5. doi: 10.1002/hipo.10165
232. Niemi WD, Slivinski K, Audi J, Rej R, Carpenter DO. Propylthiouracil treatment reduces long-term potentiation in area CA1 of neonatal rat hippocampus. Neurosci Lett. (1996) 210:127–9. doi: 10.1016/0304-3940(96)12676-8
233. Gilbert ME. Alterations in synaptic transmission and plasticity in area CA1 of adult hippocampus following developmental hypothyroidism. Dev Brain Res. (2004) 148:11–8. doi: 10.1016/j.devbrainres.2003.09.018
234. Gould E, Allan MD, McEwen BS. Dendritic spine density of adult hippocampal pyramidal cells is sensitive to thyroid hormone. Brain Res. (1990). doi: 10.1016/0006-8993(90)90884-E
235. Taşkin E, Artis AS, Bitiktas S, Dolu N, Liman N, Süer C. Experimentally induced hyperthyroidism disrupts hippocampal long-term potentiation in adult rats. Neuroendocrinology. (2011) 94:218–27. doi: 10.1159/000328513
236. Forini F, Kusmic C, Nicolini G, Mariani L, Zucchi R, Matteucci M, et al. Triiodothyronine prevents cardiac ischemia/reperfusion mitochondrial impairment and cell loss by regulating miR30a/p53 axis. Endocrinology. (2014) 155:4581–90. doi: 10.1210/en.2014-1106
237. Zinman T, Shneyvays V, Tribulova N, Manoach M, Shainberg A. Acute, nongenomic effect of thyroid hormones in preventing calcium overload in newborn rat cardiocytes. J Cell Physiol. (2006) 207:220–31. doi: 10.1002/jcp.20562
238. Manzano J, Cuadrado M, Morte B, Bernal J. Influence of thyroid hormone and thyroid hormone receptors in the generation of cerebellar γ-aminobutyric acid-ergic interneurons from precursor cells. Endocrinology. (2007) 148:5746–51. doi: 10.1210/en.2007-0567
239. Hashimoto H, Walker CH, Prange AJJ, Mason GA. The effects of thyroid hormones on potassium-stimulated release of 3H-GABA by synaptosomes of rat cerebral cortex. Neuropsychopharmacology. (1991) 5:49–54.
240. Kalaria RN, Prince AK. Effects of thyroid deficiency on the development of cholinergic, GABA, dopaminergic and glutamate neuron markers and DNA concentrations in the rat corpus striatum. Int J Dev Neurosci. (1985) 3:655–66. doi: 10.1016/0736-5748(85)90056-5
241. Mason GA, Walker CH, Prange AJ, Bondy SC. GABA uptake is inhibited by thyroid hormones: implications for depression. Psychoneuroendocrinology. (1987) 12:53–9. doi: 10.1016/0306-4530(87)90022-9
242. Martin JV, Williams DB, Fitzgerald RM, Im HK, Vonvoigtlander PF. Thyroid hormonal modulation of the binding and activity of the GABA(A) receptor complex of brain. Neuroscience. (1996) 73:705–13. doi: 10.1016/0306-4522(96)00052-8
243. Ambrogini P, Cuppini R, Ferri P, Mancini C, Ciaroni S, Voci A, et al. Thyroid hormones affect neurogenesis in the dentate gyrus of adult rat. Neuroendocrinology. (2005) 81:244–53. doi: 10.1159/000087648
244. Desouza LA, Ladiwala U, Daniel SM, Agashe S, Vaidya RA, Vaidya VA. Thyroid hormone regulates hippocampal neurogenesis in the adult rat brain. Mol Cell Neurosci. (2005) 29:414–26. doi: 10.1016/j.mcn.2005.03.010
245. Fanibunda SE, Desouza LA, Kapoor R, Vaidya RA, Vaidya VA. Thyroid hormone regulation of adult neurogenesis. Vitam Horm. (2017) 106:211–51. doi: 10.1016/bs.vh.2017.04.006
246. Kapoor R, Desouza LA, Nanavaty IN, Kernie SG, Vaidya VA. Thyroid hormone accelerates the differentiation of adult hippocampal progenitors. J Neuroendocrinol. (2012) 24:1259–71. doi: 10.1111/j.1365-2826.2012.02329.x
247. Lemkine GF, Raj A, Alfama G, Turque N, Hassani Z, Alegria-Prévot O, et al. Adult neural stem cell cycling in vivo requires thyroid hormone and its alpha receptor. FASEB J. (2005) 19:863–5. doi: 10.1096/fj.04-2916fje
248. López-Juárez A, Remaud S, Hassani Z, Jolivet P, Pierre Simons J, Sontag T, et al. Thyroid hormone signaling acts as a neurogenic switch by repressing Sox2 in the adult neural stem cell niche. Cell Stem Cell. (2012) 10:531–43. doi: 10.1016/j.stem.2012.04.008
249. Montero-Pedrazuela A, Venero C, Lavado-Autric R, Fernández-Lamo I, García-Verdugo JM, Bernal J, et al. Modulation of adult hippocampal neurogenesis by thyroid hormones: implications in depressive-like behavior. Mol Psychiatry. (2006) 11:361–71. doi: 10.1038/sj.mp.4001802
250. Zhang L, Blomgren K, Kuhn HG, Cooper-Kuhn CM. Effects of postnatal thyroid hormone deficiency on neurogenesis in the juvenile and adult rat. Neurobiol Dis. (2009) 34:366–74. doi: 10.1016/j.nbd.2009.02.006
251. Kapoor R, Fanibunda SE, Desouza LA, Guha SK, Vaidya VA. Perspectives on thyroid hormone action in adult neurogenesis. J Neurochem. (2015) 133:599–616. doi: 10.1111/jnc.13093
252. Remaud S, Gothié JD, Morvan-Dubois G, Demeneix BA. Thyroid hormone signaling and adult neurogenesis in mammals. Front Endocrinol. (2014) 5:62. doi: 10.3389/fendo.2014.00062
253. Christie KJ, Turnley AM. Regulation of endogenous neural stem/progenitor cells for neural repair—factors that promote neurogenesis and gliogenesis in the normal and damaged brain. Front Cell Neurosci. (2013) 6:70. doi: 10.3389/fncel.2012.00070
254. Ming GL, Song H. Adult neurogenesis in the mammalian brain: significant answers and significant questions. Neuron. (2011) 70:687–702. doi: 10.1016/j.neuron.2011.05.001
255. Lu J, Manaenko A, Hu Q. Targeting adult neurogenesis for poststroke therapy. Stem Cells Int. (2017) 2017:5868632. doi: 10.1155/2017/5868632
256. Angels Font M, Arboix A, Krupinski J. Angiogenesis, neurogenesis and neuroplasticity in ischemic stroke. Curr Cardiol Rev. (2010) 6:238–44. doi: 10.2174/157340310791658802
257. Arai K, Jin G, Navaratna D, Lo EH. Brain angiogenesis in developmental and pathological processes: neurovascular injury and angiogenic recovery after stroke. FEBS J. (2009) 276:4644–52. doi: 10.1111/j.1742-4658.2009.07176.x
258. Arenillas JF, Sobrino T, Castillo J, Dávalos A. The role of angiogenesis in damage and recovery from ischemic stroke. Curr Treat Options Cardiovasc Med. (2007) 9:205–12. doi: 10.1007/s11936-007-0014-5
259. Tomanek RJ, Zimmerman MB, Suvarna PR, Morkin E, Pennock GD, Goldman S. A thyroid hormone analog stimulates angiogenesis in the post-infarcted rat heart. J Mol Cell Cardiol. (1998) 30:923–32. doi: 10.1006/jmcc.1998.0671
260. Schlenker EH, Hora M, Liu Y, Redetzke RA, Morkin E, Gerdes AM. Effects of thyroidectomy, T4, and DITPA replacement on brain blood vessel density in adult rats. AJP Regul Integr Comp Physiol. (2008) 294:R1504–9. doi: 10.1152/ajpregu.00027.2008
261. Davis PJ, Sudha T, Lin HY, Mousa SA. Thyroid hormone, hormone analogs, and angiogenesis. Compr Physiol. (2016) 6:353–62. doi: 10.1002/cphy.c150011
Keywords: brain, recovery, stroke, thyroid hormones, 3,5,3′,5′-tetraiodo-L-thyronine (T4), 3,5,3′-triiodo-L-thyronine (T3)
Citation: Talhada D, Santos CRA, Gonçalves I and Ruscher K (2019) Thyroid Hormones in the Brain and Their Impact in Recovery Mechanisms After Stroke. Front. Neurol. 10:1103. doi: 10.3389/fneur.2019.01103
Received: 29 May 2019; Accepted: 02 October 2019;
Published: 18 October 2019.
Edited by:
Stephan Schilling, Fraunhofer Society (FHG), GermanyReviewed by:
Steffen Rossner, Leipzig University, GermanyTobias Bobinger, University Hospital Erlangen, Germany
Copyright © 2019 Talhada, Santos, Gonçalves and Ruscher. This is an open-access article distributed under the terms of the Creative Commons Attribution License (CC BY). The use, distribution or reproduction in other forums is permitted, provided the original author(s) and the copyright owner(s) are credited and that the original publication in this journal is cited, in accordance with accepted academic practice. No use, distribution or reproduction is permitted which does not comply with these terms.
*Correspondence: Karsten Ruscher, a2Fyc3Rlbi5ydXNjaGVyJiN4MDAwNDA7bWVkLmx1LnNl