- Clinical Neuroscience Research Laboratory, Health Research Institute of Santiago de Compostela (IDIS), Santiago de Compostela, Spain
Stroke is the main cause of disability and death in the world within neurological diseases. Despite such a huge impact, enzymatic, and mechanical recanalization are the only treatments available so far for ischemic stroke, but only <20% of patients can benefit from them. The use of stem cells as a possible cell therapy in stroke has been tested for years. The results obtained from these studies, although conflicting or controversial in some aspects, are promising. In the last few years, the recent development of the induced pluripotent stem cells has opened new possibilities to find new cell therapies against stroke. In this review, we will provide an overview of the state of the art of cell therapy in stroke. We will describe the current situation of the most employed stem cells and the use of induced pluripotent stem cells in stroke pathology. We will also present a summary of the different clinical trials that are being carried out or that already have results on the use of stem cells as a potential therapeutic intervention for stroke.
Introduction
From the moment that the capacity of differentiation and self-renewal of stem cells became known, their use as cell therapy for a wide range of diseases has been considered. The international community has focused on this idea, starting a revolution in the study of stem cells (1). This revolution led to several important discoveries that, step by step, paved the way to convert cell therapy into reality. But the greatest discovery was made in 2006 when Yamanaka and Takahashi were able, for the first time, to generate induced pluripotent stem cells (iPSCs) from adult somatic cells by inducing the artificial expression of four transcriptional factors: OCT4, SOX2, c-MYC, and KLF4 (2). This new approach provided a considerable resource of human pluripotent stem cells that could be propagated during long-term culture and yet be differentiated to a variety of lineages representatives of the three embryonic germ layers, solving the ethical limitations caused by the use of human embryonic stem cells.
In addition, the generation of human iPSCs from different somatic cells of patients and the subsequent differentiation to the affected cell lineage has allowed the recapitulation of features of genetic pathologies through in vitro disease modeling and the discovery of new treatments directly tested on these human cells. Recently, the combination of iPSCs with the advances in genome editing techniques, such as the clustered regularly interspaced short palindromic repeat (CRISPR) system, has also provided a promising way to repair putative causative alleles in patient lines into a healthy cell line for future autologous cell therapy (3, 4) (Figure 1).
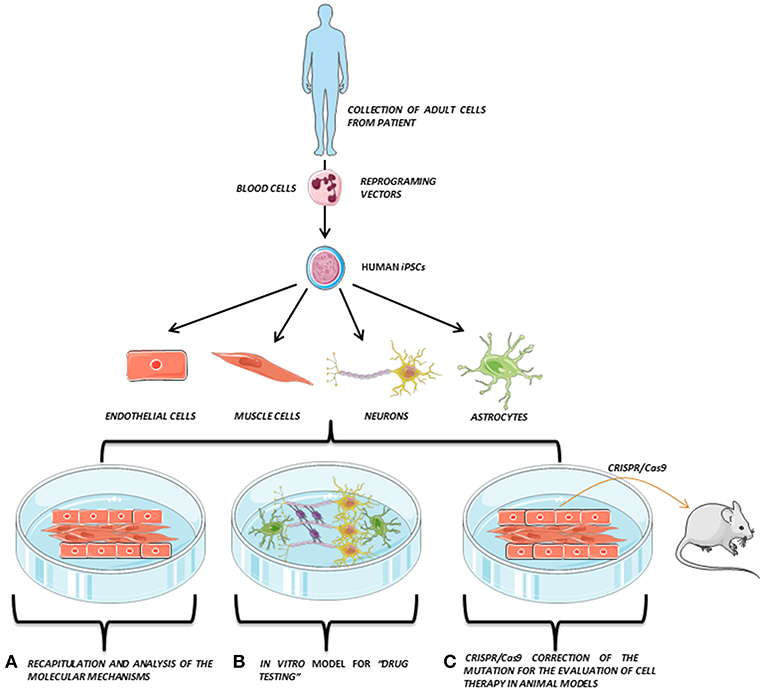
Figure 1. iPSCs modeling scheme. Adult somatic cells (e.g., blood cells) are collected from the patient, reprogrammed and derived to the affected cell types (e.g., endothelial cells, muscle cells, neurons, or astrocytes), which are co-cultured in vitro, opening the possibility to perform several studies directly on the patient's own cells. Adapted from Servier Medical Art by Servier is licensed under a Creative Commons Attribution 3.0 Unported License (https://smart.servier.com/).
The development of human iPSCs has also opened a new opportunity for those neurological diseases where the affected neuronal type is well-known or the genetic cause of the pathology is well-described such as (i) Alzheimer's (5, 6), (ii) Parkinson's (7, 8), (iii) amyotrophic lateral sclerosis (9), or (iv) Huntington disease (10). In these pathologies, iPSCs have been used to generate neuronal cell lines to recapitulate and study the mechanics of the pathology in in vitro models or to evaluate their neurorecovery capability.
In the field of stroke, like other stem cells, iPSCs have been used as a neuroprotective cell therapy (mainly based on their immunomodulatory capacity) or as a neuroreparative therapy (by inducing neurogenesis, angiogenesis, synaptogenesis, modulation of the immune response, or transdifferentiation) (Figure 2). Besides its neuroprotective or neuroreparative application, the use of iPSCs for stroke modeling has been poorly exploited mainly because this is a neurological pathology with multiple affected cells types and reduced genetic component, compared to other neurological diseases such as Alzheimer's or Parkinson's. However, the use of iPSCs has been recently explored to model neurovascular pathologies associated with risk of stroke (11, 12), opening a promising approach in the study of these neurovascular diseases.
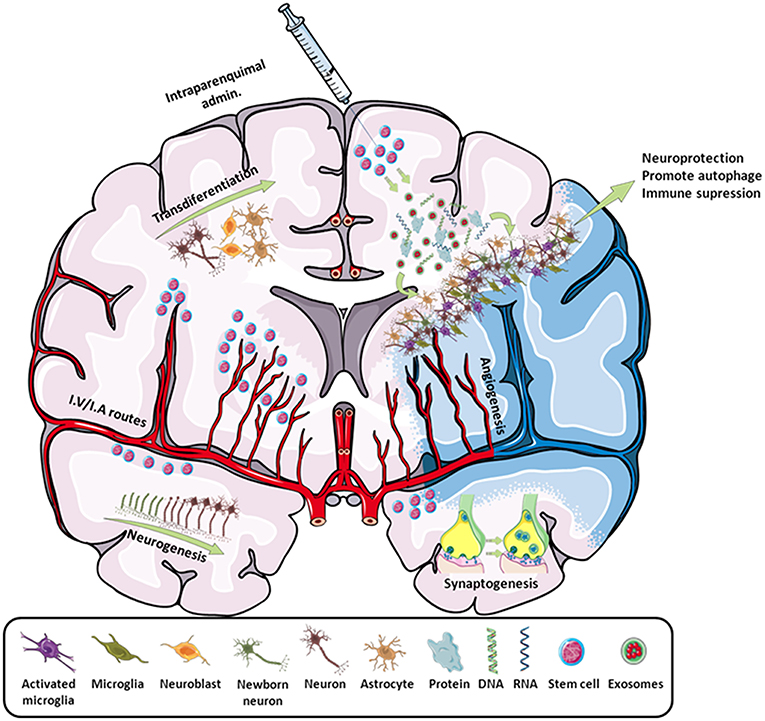
Figure 2. Scheme of all the main effects promoted by stem cells in stroke. By intraparenchymal injection or i.v./i.a. routes, stem cells induce neurogenesis, transdifferentiation, angiogenesis, synaptogenesis, and immune modulation by attracting or releasing trophic substances to the infarcted area. Adapted from Servier Medical Art by Servier is licensed under a Creative Commons Attribution 3.0 Unported License (https://smart.servier.com/).
In this review, we offer a general overview of the use of adult stem cells and iPSCs in stroke, addressing the main problems and the main clinical trials that already present results.
Adult Stem Cell Therapy in Stroke
Stroke, resulting from the interruption of blood supply to the brain, is the leading cause of disability and death in the world within neurological diseases despite a decrease in its mortality rate (13). Pharmacological or mechanical reperfusion therapies are the most effective treatments during the acute phase of ischemic stroke and it is associated with good outcome in 50–70% of cases. However, these treatments are only applicable to <20% of patients because of the short therapeutic window and side effects (14).
Stem-cell-based therapies have emerged as a promising tool for the treatment of both acute and delayed phases of stroke owing to their multipotentiality, ability to release growth factors, and immunomodulatory capacities. Thus, this transdifferentiation is able to produce cells with a neural lineage; induce neurogenesis, angiogenesis, and synaptogenesis; and activate endogenous restorative processes through the production of cytokines and trophic factors. Moreover, the regulation of cerebral blood flow (CBF), the blood–brain barrier (BBB), and other neuroprotective mechanisms, such as the reduction of apoptosis, inflammation, and demyelination or the increase of astrocyte survival, have also been described as beneficial after stroke (15).
While the technology of the iPSCs is quite new and deeper studies are being carried out to know its real translationality, studies with adult stem cells have been performed for much longer, and there is more information about their use in cell therapy for stroke. Furthermore, there are already clinical trials going on and even closed with adult stem cells. Focusing on stroke, the most frequently used stem cells are the mesenchymal stem cells (MSCs), due to their great trophic capabilities, and the neural stem cells (NSCs), because of their neurorecovery activity (15).
Methodology in Stem Cell Administration for Stroke
Despite the special attention on stem cells as a promising therapeutic candidate for stroke, parameters such as administration route or cell dosage are still under discussion.
There are relatively few studies that have compared the different possible routes of administration of stem cells. The first studies that used stem cells for cerebrovascular diseases were looking for a neuronal replacement, so they chose an intraparenchymal injection as the most direct route for cell engraftment. These studies showed that the stem cells not only survived but they migrated to the affected zone (16, 17). However, this choice is not the most suitable due to the need of opening a cranial window and also because it damages the brain parenchyma, which is not convenient for stroke patients.
The main alternatives to this route of administration are the vascular routes, either intra-arterial (i.a.) or intravenous (i.v.), which are currently the most used for cell delivery. Intravenous injections are minimally invasive, but cell tracking studies following this route have shown that most administered cells remain trapped mainly in the lungs (18, 19), liver (20), and spleen (21), indicating that a reduced number of cells reach the brain. On the other hand, i.a. administration is a promising strategy to direct the majority of injected cells to the brain, but this is a risky administration route and the fate of injected cells following this route remains unknown due to high variation in the reported results (22).
Whether one route is more efficient than the other is not clear and depends on the cell type used. Thus, in some studies, it was found that the injection of neural progenitor cells (NPCs) by i.a. through the carotid artery presented a higher migration rate in brain and a wider distribution pattern than i.v. administration. Nevertheless, the mortality rate for this i.a. delivery was significantly higher (41%) than in i.v. injection (8%) (23). However, in other studies with bone marrow stem cells (BMSCs) and bone marrow mononuclear cells, there was no greater mortality or greater recovery of infarct volume of one route with respect to the other (24, 25).
The size of stem cells is also a critical parameter when passing through the lungs and should be taken into consideration to decide the best route of administration. As an example, when using MSCs, the majority of them get trapped in the lungs, while the NSCs have a pass-through rate twofold higher (18).
Aimed to clarify the discrepancies about the best route for cell administration in stroke, we have recently reported an experimental study to investigate whether MSCs were able to reach the brain following i.a. or i.v. administration after transient cerebral ischemia in rats and to evaluate the therapeutic effects of both routes (22). Based on our findings, we could conclude that MSCs were found in the brain following i.a. but not i.v. administration in ischemic rats. However, the i.a. route increased the risk of cerebral lesions (microstrokes) and did not improve functional recovery, while the i.v. delivery produced functional recovery and was safe but MCSs did not reach the brain tissue. This fact implies that treatment benefits are not attributable to brain MCS engrafting after stroke (22).
Cell dose is another issue to take into account for both i.a. and i.v. administration that has not been very well-elucidated yet. In line with other studies, we have estimated that doses higher than 1 × 105-0.25 × 106 cells/mL administered i.a. as bolus infusion increase significantly the risk of arterial occlusion (22, 26), while other studies have estimated that doses to 3 × 107 cells are safe (24, 25).
Stem Cell Fate and Engraftment
It has been widely accepted that there is not engraftment of MSCs in the brain after i.v. administration and that the repair and recovery effects observed in some ischemic animals models are mediated by trophic factors secreted by MSCs (22, 24, 27, 28). However, other studies mainly focused on the intraparenchymal cell injection in brain have found successful engraftment results of cells in the ischemic tissue. The survival and the engraftment rate of the stem cells in brain are critical parameters that determine the successful rate of the repairing and healing effects after cerebral ischemia (29, 30).
Despite the low level of cell brain engraftment, it has been shown that intraparenchymal cells promote neovascularization and functional recovery (31). One of the most relevant studies about this issue was performed by Kokaia's team who carried out a 4-month follow-up of iPSC-derived NSCs transplanted into brain striatum and cortex of rats and mice subjected to stroke. They found that that engrafted cells survive in the brain for up to 4 months without forming tumors, observing a sensorimotor recovery even 1 week after cell administration. In this study, most of the cells had differentiated to neurons able to form axonal prolongations. In addition, the proliferative capacity of these engrafted cells diminished from 40% at 2 weeks to 8 and 0.5% at 2 and 4 months respectively, probably due to this NSC differentiation to neurons (29). The same findings have also been observed when BMSCs were intraparenchymally administered, observing an engraftment of the cells at the ischemic penumbra and at the subventricular zone (32).
Nowadays, cell targeting with contrast agents for the in vivo tracking by magnetic resonance imaging (MRI) or positron emission tomography (PET) are being established as powerful technology tools to determine fate and survival after cell treatment, after i.v., i.a., and intraparenchymal cell injection (33).
Indeed, studies using MRI have allowed follow-up of cells for up to 1 year after transplantation (34). One of the most important aspects of the in vivo cell tracking is that the cell tracers cannot interfere with the properties and cell viability. In this regard, contrast agents such as gadolinium, superparamagnetic iron oxide agents, or fluorine 19 (35, 36) have been widely used for MRI cell tracking as they do not interfere with cell viability or the migration capacity (33, 37).
Gadolinium (Gd3+) has been used to label and track different types of stem cells, such as hematopoietic progenitor cells, monocytic cells, endothelial progenitor cells, and MSCs in small cell transplantation studies. Because they are not nanoparticles, the cellular uptake of Gd3+ chelates occurs by pinocytosis (a non-specific form of endocytosis in which small particles present in the extracellular fluid are internalized into cells) or via electroporation. However, overall, the low sensitivity of these contrast agents and low uptake by cells are the main limitations to cell labeling with Gd3+ (37).
Superparamagnetic iron oxide agents, also known as SPIO nanoparticles, have a much stronger MR sensitivity compared to other paramagnetic agents. Thousands to millions of SPIOs can be internalized in the cells, which makes them generate an MRI signal that is strong enough to visualize a small number of transplanted cells under in vivo conditions. The use of SPIOs is mainly restricted to assessing the acute retention of labeled cells and their short-term distribution in the body as these nanoparticles may occasionally diffuse to other tissues or can be scavenged by macrophages, which can then generate a false signal on MRI (37).
Fluorine 19 as a contrast agent for MRI has recently gained more attraction because of several advantages. Fluorine 19 provides a more accurate, unambiguous detection of labeled cells (given the lack of background signal). Moreover, the relationship between the concentration of fluorine 19 and signal intensity is directly proportional and linear over a wide range of concentrations, and the signal can be quantified directly from the acquired images. In addition, the lack of a detectable background in biological tissues leads to higher visibility of the target cells, much like “hot spots” emerging from an empty background (37, 38).
The quantity threshold needed to detect labeled cells is clearly different for SPIOs and fluorine 19. A comparative analysis, using different types of iron-oxide-based contrast agents for MRI labeling of embryonic stem cells, NPCs, and dendritic cells, showed that there is a strong dependence of the iron oxide uptake and label stability on the type of iron oxide particles and cell lines used. Although sufficient uptake was achieved to allow for single-cell detection, in this study, it was estimated that the amount of iron necessary for single-cell imaging was 1–3 pg/cell (39). However, in other in vivo studies with fluorine 19 for MRI cell tracking, the minimal amount of tracer estimated for single-cell imaging was 0.1 pg/cell (40), which reflects the different sensitivity between both label types.
Nuclear medicine imaging techniques, such as single photon-emission computed tomography (SPECT) or PET, represent another promising modality to track stem cells in vivo for stroke treatment. Nuclear medicine imaging involves the use of radiotracers that can bind to different ligands. The main advantage of PET and SPECT is the extreme sensitivity, detecting molecules at the nanomolar level; however, due to the short half-life of the tracers, it is not possible to follow the cells longer than 3 or 4 days, in the best of cases (33).
Despite the high sensitivity and resolution obtained with MRI and PER probes for in vivo cell tracking, sometimes the image is not clear enough to visualize the graft or tracking of the targeted cells. The ambiguity of cell label detection and cell assignment that usually happens with MRI or PET can be successfully circumvented with gene reporter imaging, mainly used with bioluminescence and fluorescence reporters, a strategy commonly adopted in several studies (34, 38).
MSCs for Stroke Treatment
Cell therapy based on the use of autologous MSCs represents one of the most promising treatments to restore function after stroke. MSCs are pluripotent stem cells that are found, in small proportion, inside the bone marrow. These cells have the ability to differentiate into different cell-type precursors (chondrocytes, osteocytes, myocytes, etc.) but their use in cell therapy is mainly based on their ability to release a wide range of bioactive molecules, with immunoregulatory and regenerative properties (41). However, one of the main causes that has limited the advance of MSCs in stroke and other neurological pathologies are the arduous protocols that are sometimes required to obtain, expand, and characterize human MSCs for clinical use (42).
Among the MSCs, due to their great regenerative potential and tissue engineering, the BMSCs are the most promising ones (41, 43). It has been shown that the i.v. administration of BMSC 24 h after stroke induces angiogenesis within the surrounding lesion area in rats. This angiogenesis is mainly due to an increase of VEGF secretion by the BMSCs, and VEGFR2 expression in cerebral endothelial cells (44). Also, these BMSCs are able to induce the proliferation of endogenous NSCs (45). BMSCs also have a neuroplasticity function, induced by the release of trophic factors within the affected region, enhancing restructuration processes (46). Although multiple repairing mechanisms seem to be involved in the healing effects of the BMSCs (and MSCs in general), it is well-known that their ability of releasing high amounts of extracellular vesicles is a relevant pathway by which they exert these effects (47–49).
Despite being an interesting tool for the treatment of stroke, the use of BMSCs requires bone surgical interventions that sometimes limit their use. Comparing with BMSCs, the adipose tissue-derived mesenchymal stem cells (ADMSCs) come from a more accessible source and are more abundant. Also, they have already proved their effectiveness in stroke preclinical experiments, specifically as a promising treatment for stroke-related comorbidities (15).
Although the use of ADMSCs is less studied, there are works that show their positive effects. In a report from 2013, the effect of BMSCs vs. ADMSCs was compared. Neither BMSCs nor ADMSCs have shown a reduction in infarct size or any cellular migration or engraftment, but in both cases, a reduction in cell death and an increase in the proliferation rate with an increment in the levels of VEGF, oligodendrocytes, synaptophysin, and neurofilaments at day 14, which was associated with good functional recovery, were found (50). These results show that ADMSCs present the same regenerative abilities as BMSCs, but since the source of acquisition of the ADMSCs is better, the use of these stem cells could be more suitable. Regarding the way ADMSCs exert their regenerative properties, it seems that it is mediated by the extracellular vesicles release. In a previous study, it was proved that an intravenous injection of isolated extracellular vesicles from ADMSCs produced a greater beneficial effect in rats subjected to 50 min of middle cerebral artery occlusion than the injection of just ADMSCs (51). Also, the safety of the ADMSCs has already been tested. A study in 2011 proved that an intravenous injection of ADMSCs in immunodeficient mice didn't produce any toxicity effect all along 13 weeks, even at the highest cell dose (2.5 × 108 cells/kg body weight). In the same way, they tested the possibility of tumor formation along 26 weeks, but they also didn't find any evidence of tumorigenesis not even at the highest dose (2 × 108 cells/kg). In a 3-month follow-up of patients with spinal cord injury to whom a single dose of hADMSCs (4 × 108cells) was administered, again no evidence of any adverse effect was found (52).
The therapeutic use of MSCs has been also extended to other pathologies as hemorrhagic stroke where it has seen that administration of MSCs induce neuroprotective effects during the acute phase of the lesion (53) and functional recovery, even when cells are administered at long term (54). Moreover, MSCs have been tested in combination with recombinant tissue plasminogen activator to prevent the risk of hemorrhagic events thanks to the ability of the cells to inhibit endothelial dysfunction (55).
MSCs are also prone to cell engineering; for instance, excitatory amino acid transporter 2 (EAAT2) plays a pivotal role in glutamate clearance in the adult brain, thereby preventing excitotoxic effects after cerebral ischemic damage. Considering the high efficacy of EAAT2 for glutamate uptake, we have recently induced the expression of this transporter in MSCs for systemic administration, combining the intrinsic properties of these cells with excitotoxic protection (56).
NSCs for Stroke Treatment
NSCs are adult stem cells present at the central nervous system that are capable of self-renewal and give birth to new neurons and glial cells, thus contributing to the plasticity and brain repair (57). These processes are enhanced within ischemic brain as a neurorepairing mechanism (58, 59). However, these processes do not occur at a rate high enough to be effective (60). Because of that, several approaches in pre-clinical studies have been performed in order to compensate or supplement these endogenous mechanisms. The most used approach consists of the administration of exogenous NSCs (61, 62). This approach has shown that i.a. administration of NSCs 24 h after stroke, in a mouse model, promotes a reduction on the inflammatory process and also reduces the BBB damage (63). This reduction of the inflammatory process was also seen in a pig stroke model, also by intraparenchymal injection, but 5 days after stroke (64). Nevertheless, the total percentage of NSCs that successfully migrate and engraft within the ischemic area is quite low. To this respect, genetic modifications that may make the cells more resistant to ischemic conditions (65, 66), such as the use of NSCs submitted to pre-conditioning conditions before administration (67, 68) or their release with various biomaterials as vehicles (69, 70), would increase this percentage. NSCs derived from human iPSCs have been also tested in rodent stroke models. The cells did not reduce stroke volume or improve behavioral recovery during the month following transplantation, but no tumor formation was observed (71). Regarding intracerebral hemorrhages, there are fewer studies, but they report improvements in functional performance after 2–8 weeks, independently of the administration route (72, 73).
iPSCs in Stroke
On the first years after Yamanaka and Takhashi iPSC research, the experiments and preclinical studies on stroke assayed the effect of a direct injection of iPSCs into the affected region. Several studies reported improvements both in infarct volume reduction and in functional recovery (74–76). Also, improvements in the neurological function and survival rate in hemorrhagic stroke were reported (77). Most of these improvements attributed to the iPSCs were due to a differentiation of these iPSCs into different adult stem cells in the affected region (78, 79).
Although some studies have reported that iPSC-derived NSCs transplanted in the brain of mice subjected to stroke have no tumorigenicity risk (29) or iPSCs in intracerebral hemorrhage stroke (77), one of the main limitations of iPSCs in order to achieve a future translationality to the clinical is the formation of teratomas or tumorigenicity in the following weeks after cell administration (80). This is due to the environmental effects of the niche where the iPSCs are implanted. Formation of teratomas can also occur by transformation of residual iPSCs that remain on the implanted area and can become benign teratomas after some time (81, 82). To this respect, there are some studies that tried to solve this issue. For example, a study published by Chen et al. compared the formation of teratomas after the injection of iPSCs with and without fibrin glue as vehicular agent into the subdural region instead of its injection right into the cortex. In the injection of iPSCs by themselves, there was always formation of teratomas after 4 weeks, whereas in the ones injected with fibrin glue, there was not. The authors pointed out that this was not only due to the fibrin glue but also because the subdural region was not a niche appropriate enough to induce an uncontrolled growth of the iPSCs (74). Besides, another study showed the possibility of inhibiting the formation of tumors due to this residual iPSCs by treatments with inhibitors of specific pro-apoptotic routes of stem cells, inducing their apoptosis and erasing them; meanwhile, the derived differentiated cells survived and maintained their functionality (82).
In conclusion, although the therapy with iPSCs is yet on preclinical experimentation, the use and the evaluation of this cell therapy is increasing significantly every year, since this new approach is a faster way to generate human stem cells (83–85). However, because this is a relatively recent discovery, the use of iPSCs for cell therapy still presents some issues that need to be explored such as the optimal time window, optimal cell/dose, or tumorigenicity (86). In case of stroke, it is also not clear if the therapeutic effect observed in ischemic animal models treated with iPSCs is mediated by cell renewal and/or by replacement of the damaged tissue (87) as the transplanted cells disappear few weeks after administration (30).
Currently, the proper differentiation iPSCs to the cell line of interest (e.g., neuronal, epithelial, etc.) with reduced division capacity seems to be the most convenient way to address these limitations.
iPSCs and CADASIL
Within the field of stroke, 25–30% of events are caused by cerebral small vessel diseases (SVDs). Although stroke is a well-studied disease and its mechanisms and underlying processes are well-known, there are some pathologies that can be the cause of cerebral infarcts like SVDs, which present a lack of approaches and understanding (88). Despite the impact that these SVDs have on the brain, nowadays there are no specific treatments for them. Furthermore, there are limited therapeutic options for secondary prevention compared with those for other common causes of stroke. As a result, even though it is the cause of the onset of the stroke in many cases, currently there are no solutions or treatments available. The few studies that have been carried out on these diseases point to the study of monogenic variants of SVDs, in order to provide valuable insights into the molecular mechanisms underpinning idiopathic SVDs.
One of these variants is the cerebral autosomal dominant arteriopathy with subcortical infarcts and leukoencephalopathy, commonly known as CADASIL. CADASIL patients develop leukoencephalopathy, migraines with aura, recurrent ischemic strokes, motor disability, and dementia as main symptoms. Currently, there is no treatment for this disease. These symptoms are caused by progressive weakness of the small brain vessels, which coincide spatially with granular osmiophilic material (GOM). This vessel weakness is due to a continuous aberrant accumulation of the extracellular component of the Notch3 protein membrane in the GOM (89). In CADASIL, the NOTCH3 gene presents a mutation in one of its exons that results in a loss or a gain of a cysteine residue. This mutation leads to an incorrect process of the metabolic pathway when the Notch3 signal is triggered, which ends with the accumulation of the extracellular domain of the protein in the GOM (89, 90).
Although there are several mouse models of CADASIL (knockouts for several and different mutations), it has been impossible to find any solution to the progressive accumulation of the extracellular domain of Notch3. In this line, iPSCs may shed a light over this disease. The main cell types affected in CADASIL are the vascular smooth muscle cells (VSMCs), which express the mutation, and the vascular endothelial cells (VECs), which interact with the VSMCs. With cell modeling, it would be possible to generate these cells from reprogrammed iPSCs from adult somatic cells of a CADASIL patient and perform mono and co-cultures that would allow one to study and better understand the molecular mechanisms of this disease and clarify the confusing and sometimes contradictory information that the mouse models provide (90). Also, over these cultures, a drug screening may be performed to see if any drug is able to slow down or even stop the accumulation of the extracellular domain of Notch3. Lastly, it would also be possible, by genetic editing, to repair the mutation within the iPSCs and, once differentiated into VSMCs, administer them in Notch3 knockouts mice to see if the healthy VSMCs replace the damaged endogenous ones.
At the moment, there are just two recent studies where, for the first time, the reprogramming of adult somatic cells from a patient with CADASIL to iPSCs was made, proving that NOTCH3 mutation is not a limitation to the reprogramming (11, 12). The generation of this iPSC line offers an unprecedented opportunity for studying and modeling both CADASIL and other pathologies related to the vascular risk of stroke.
Clinical Trials
Currently, there are no clinical trials for stroke with iPSCs. This is due to the fact that the iPSCs are yet in a relatively early stage of study, and that they present several problems yet to solve and previously described regarding their safety.
However, in the last decade, different clinical trials have been carried out or are being carried out all over the world, mainly with MSCs (Table 1). These trials are shedding light about quantities, routes of administration, and efficacy at different times with stem cells, as well as their safety.
Some of the already finished trials, despite their limitations, show results that seem to point into the right direction. For example, the InVeST trial designed to evaluate the effect of intravenous BMSCs injection did not show a beneficial effect but proved the safeness of BMSCs use in humans (92). In 2016, another clinical trial with BMSCs, apart from corroborating the safeness of BMSCs administration, found improvements in clinical outcome (NIHSS, ESS, and Fugl–Meyer scores) with stroke patients. However, it was a non-randomized small trial (n = 18), so its results should be taken with caution (99). On the other hand, the PISCES study (98) tried to prove the beneficial effect of human NSCs in patients who have had a first ischemic stroke in the last 6–60 months. After a follow-up of 2 years, they found improvements in neurological function and no adverse effects in cell dosage up to 20 million cells. Nonetheless, it was also a small trial (n = 13, split into four different groups depending on cell dosage), and in two patients, a slight increase in hyperintensity in pre-existing peri-infarct white matter hyperintensity was found, although there were no clinical changes associated to this MRI changes.
As it can be deduced from the trials above, in general, the ongoing and finished clinical trials present in their majority some limitations that must be solved in the subsequent trials. While all trials provide the number of transplanted cells and their route of administration, some of them omit the cell dosage. On the other hand, some of the clinical trials in which no benefit is found point to a late injection time. To this regard, choosing the first days after stroke as the moment for injection may be a critical point in order to obtain benefits from the injection, but it also involves the risk that the action of the cytokines that happens during the first days after stroke may mask the real effect of the cell dosage. Also, there is the risk that the patient could be subjected to unforeseen surgeries, like hemicraniectomy, that may alter the cell dosage effect. Besides, and in the same way as happens in preclinical experiments, the choice of the administration route is also a key factor, and while new trials base their choice in previous trials, there is still work to do to establish secure criteria for route of administration and cell dosage based on the cell type.
Finally, current clinical trials are, in general, small trials waiting for their expansion or the creation of new and bigger ones with a higher recruitment capacity that may corroborate the results obtained by these pioneering trials.
Discussion
In recent years, a multitude of studies about stem cells and iPSCs are being carried out within the field of cerebrovascular diseases. These studies have been proving the efficiency to induce both neuroreparative and neuroprotective effect, but they also have permitted to refine some important issues like the number of cells and the most appropriated route of administration depending on the cell type, which has allowed various centers all over the world to start different clinical trials, getting closer to the final goal, a consolidated cell therapy for stroke. However, the ongoing trials are yet too small to be really conclusive, and bigger ones with a wider number of patients would have to be carried out in order to achieve more conclusive results.
There are still several important limitations to consider in case cell therapy on stroke becomes a reality in clinical practice. Stroke is a cerebrovascular pathology and, as such, it is not a chronic disease that may be treated over time. In stroke, the therapeutic window for recanalization or neuroprotective treatments comprehends the first hours after stroke, and that is why the treatment should be ready at the arrival of the patient to the hospital. In this case, the availability of stem cells would not reach this period of time, since their use would implicate their previous extraction from the patient for a neuroreparative treatment of these cells; it would also be hardly achievable to have the cells on time, since the reprogramming and/or differentiation can take up to 6 months easily. To solve this problem, researchers addressed the possibility of establishing universal stem cell banks, derived from an universal pull of iPSCs coming from a population set that would cover all the combinations of the human leucocyte antigen alleles (HLA), avoiding any chance of immune rejection (100, 101).
Another problem to take into consideration would be the future commercialization of the cells. The pharmaceutical industry tends to be interested in drugs of easy distribution and maintenance, while the cells, being living organisms, would require a special treatment on this regard. This would not make them especially attractive for the industry, and even if the maintenance cost could be handled by the hospitals, the distribution cost would still exist.
In the last few years, a new field of research has been developed, focused on the extracellular vesicles released by adult stem cells, since it is through them that they exert their main benefits. The use of these vesicles will replace the need to use adult stem cells, obviating their associated problems. However, this technology comes with other problems that need to be solved, like the vesicle dosage (which is unsettled), time and mode of application, or their biodistribution (102).
Future Perspectives and Alternatives
Cell therapy (especially MSCs and NSCs) in stroke based on the use of stem cells has advanced considerably but, to become a reality, while wider and deeper clinical trials are carried out, limitations such as immediate availability and distribution of stem cells for clinical use must be overcome. Extracellular vesicles released by MSC are emerging as a novel alternative to cell therapy as they could have similar beneficial effects to MSC but with lower risk effect (in terms of vessel occlusion); they are easier to handle with respect to cells and can also be used as biomarkers to evaluate stroke recovery after cell therapy.
The potential risk of tumorigenicity associated with the direct use of iPSCs as cell therapy for human treatment represents the main limitation for immediate clinical use. The improvement in genome editing techniques, such as CRISPR/Cas9, has expanded the use of iPSCs derived from patients to generate healthy cells that can provide a useful channel for precision therapy with potentially lower tumorigenic risk. The use of the iPSC-based disease model in stroke-related pathologies as CADASIL is expected to provide potential therapeutic strategies for cerebral SVDs.
Author Contributions
Drafting of the article was done by HF-S, AB-C, and FC. Manuscript revision was done by JC and FC.
Funding
This study has been partially supported by grants from Instituto de Salud Carlos III (PI17/0054), Spanish Research Network on Cerebrovascular Diseases RETICS-INVICTUS (RD12/0014), Fundación Mutua Madrileña, the Ministry of Economy and Competitiveness of Spain (SAF2017-84267-R), the European Union program FEDER, and the European Regional Development Fund—ERDF, MADIA project No. 732678 to FC. Furthermore, FC (CP14/00154) is a recipient of a research contract from the Miguel Servet Program of Instituto de Salud Carlos III.
Conflict of Interest Statement
The authors declare that the research was conducted in the absence of any commercial or financial relationships that could be construed as a potential conflict of interest.
References
1. Watt FM, Driskell RR. The therapeutic potential of stem cells. Philos Trans R Soc Lond B Biol Sci. (2009) 365:155–63. doi: 10.1098/rstb.2009.0149
2. Takahashi K, Yamanaka S. Induction of pluripotent stem cells from mouse embryonic and adult fibroblast cultures by defined factors. Cell. (2006) 126:663–76. doi: 10.1016/j.cell.2006.07.024
3. Bilic J, Belmonte JCI. Concise review: induced pluripotent stem cells versus embryonic stem cells: close enough or yet too far apart? Stem Cells. (2012) 30:33–41. doi: 10.1002/stem.700
4. Halevy T, Urbach A. Comparing ESC and iPSC-based models for human genetic disorders. J Clin Med. (2014) 3:1146–62. doi: 10.3390/jcm3041146
5. Israel MA, Yuan SH, Bardy C, Reyna SM, Mu Y, Herrera C, et al. Probing sporadic and familial Alzheimer's disease using induced pluripotent stem cells. Nature. (2012) 482:216–20. doi: 10.1038/nature10821
6. Kondo T, Asai M, Tsukita K, Kutoku Y, Ohsawa Y, Sunada Y, et al. Modeling Alzheimer's disease with iPSCs reveals stress phenotypes associated with intracellular Aβ and differential drug responsiveness. Cell Stem Cell. (2013) 12:487–96. doi: 10.1016/j.stem.2013.01.009
7. Swistowski A, Peng J, Liu Q, Mali P, Rao MS, Cheng L, et al. Efficient generation of functional dopaminergic neurons from human induced pluripotent stem cells under defined conditions. Stem Cells. (2010) 28:1893–904. doi: 10.1002/stem.499
8. Kikuchi T, Morizane A, Doi D, Magotani H, Onoe H, Hayashi T, et al. Human iPS cell-derived dopaminergic neurons function in a primate Parkinson's disease model. Nature. (2017) 548:592–6. doi: 10.1038/nature23664
9. Lee S, Huang EJ. Modeling ALS and FTD with iPSC-derived neurons. Brain Res. (2017) 1656:88–97. doi: 10.1016/j.brainres.2015.10.003
10. Szlachcic WJ, Wiatr K, Trzeciak M, Figlerowicz M, Figiel M. The generation of mouse and human huntington disease iPS cells suitable for in vitro studies on Huntingtin function. Front Mol Neurosci. (2017) 10:253. doi: 10.3389/fnmol.2017.00253
11. Fernández-Susavila H, Mora C, Aramburu-Núñez M, Quintas-Rey R, Arias S, Collado M, et al. Generation and characterization of the human iPSC line IDISi001-A isolated from blood cells of a CADASIL patient carrying a NOTCH3 mutation. Stem Cell Res. (2018) 28:16–20. doi: 10.1016/j.scr.2018.01.023
12. Ling C, Liu Z, Song M, Zhang W, Wang S, Liu X, et al. Modeling CADASIL vascular pathologies with patient-derived induced pluripotent stem cells. Protein Cell. (2019) 10:249–71. doi: 10.1007/s13238-019-0608-1
13. Feigin VL, Norrving B, Mensah GA. Global burden of stroke. Circ Res. (2017) 120:439–48. doi: 10.1161/CIRCRESAHA.116.308413
14. Thiebaut AM, Gauberti M, Ali C, Martinez De Lizarrondo S, Vivien D, Yepes M, et al. The role of plasminogen activators in stroke treatment: fibrinolysis and beyond. Lancet Neurol. (2018) 17:1121–32. doi: 10.1016/S1474-4422(18)30323-5
15. Laso-García F, Diekhorst L, Gómez-De Frutos MC, Otero-Ortega L, Fuentes B, Ruiz-Ares G, et al. Cell-based therapies for stroke: promising solution or dead end? Mesenchymal stem cells and comorbidities in preclinical stroke research. Front Neurol. (2019) 10:332. doi: 10.3389/fneur.2019.00332
16. Kelly S, Bliss TM, Shah AK, Sun GH, Ma M, Foo WC, et al. Transplanted human fetal neural stem cells survive, migrate, and differentiate in ischemic rat cerebral cortex. Proc Natl Acad Sci USA. (2004) 101:11839–44. doi: 10.1073/pnas.0404474101
17. Darsalia V, Kallur T, Kokaia Z. Survival, migration and neuronal differentiation of human fetal striatal and cortical neural stem cells grafted in stroke-damaged rat striatum. Eur J Neurosci. (2007) 26:605–14. doi: 10.1111/j.1460-9568.2007.05702.x
18. Fischer UM, Harting MT, Jimenez F, Monzon-Posadas WO, Xue H, Savitz SI, et al. Pulmonary passage is a major obstacle for intravenous stem cell delivery: the pulmonary first-pass effect. Stem Cells Dev. (2009) 18:683–92. doi: 10.1089/scd.2008.0253
19. Eggenhofer E, Benseler V, Kroemer A, Popp FC, Geissler EK, Schlitt HJ, et al. Mesenchymal stem cells are short-lived and do not migrate beyond the lungs after intravenous infusion. Front Immunol. (2012) 3:297. doi: 10.3389/fimmu.2012.00297
20. Ezquer F, Morales P, Quintanilla ME, Santapau D, Lespay-Rebolledo C, Ezquer M, et al. Intravenous administration of anti-inflammatory mesenchymal stem cell spheroids reduces chronic alcohol intake and abolishes binge-drinking. Sci Rep. (2018) 8:4325. doi: 10.1038/s41598-018-22750-7
21. Acosta SA, Tajiri N, Hoover J, Kaneko Y, Borlongan CV. Intravenous bone marrow stem cell grafts preferentially migrate to spleen and abrogate chronic inflammation in stroke. Stroke. (2015) 46:2616–27. doi: 10.1161/STROKEAHA.115.009854
22. Argibay B, Trekker J, Himmelreich U, Beiras A, Topete A, Taboada P, et al. Intraarterial route increases the risk of cerebral lesions after mesenchymal cell administration in animal model of ischemia. Sci Rep. (2017) 7:40758. doi: 10.1038/srep40758
23. Li L, Jiang Q, Ding G, Zhang L, Zhang ZG, Li Q, et al. Effects of administration route on migration and distribution of neural progenitor cells transplanted into rats with focal cerebral ischemia, an MRI study. J Cereb Blood Flow Metab. (2009) 30:653–62. doi: 10.1038/jcbfm.2009.238
24. Vasconcelos-Dos-Santos A, Rosado-De-Castro PH, Lopes De Souza SA, Da Costa Silva J, Ramos AB, Rodriguez De Freitas G, et al. Intravenous and intra-arterial administration of bone marrow mononuclear cells after focal cerebral ischemia: is there a difference in biodistribution and efficacy? Stem Cell Res. (2012) 9:1–8. doi: 10.1016/j.scr.2012.02.002
25. Yang B, Migliati E, Parsha K, Schaar K, Xi X, Aronowski J, et al. Intra-arterial delivery is not superior to intravenous delivery of autologous bone marrow mononuclear cells in acute ischemic stroke. Stroke. (2013) 44:3463–72. doi: 10.1161/STROKEAHA.111.000821
26. Hosoda T, Yavagal DR, Lin B, Raval AP, Garza PS, Dong C, et al. Efficacy and dose-dependent safety of intra-arterial delivery of mesenchymal stem cells in a rodent stroke model. PLoS ONE. (2014) 9:e93735. doi: 10.1371/journal.pone.0093735
27. Zhang L, Li Y, Zhang C, Chopp M, Gosiewska A, Hong K. Delayed administration of human umbilical tissue-derived cells improved neurological functional recovery in a rodent model of focal ischemia. Stroke. (2011) 42:1437–44. doi: 10.1161/STROKEAHA.110.593129
28. Vu Q, Xie K, Eckert M, Zhao W, Cramer SC. Meta-analysis of preclinical studies of mesenchymal stromal cells for ischemic stroke. Neurology. (2014) 82:1277–86. doi: 10.1212/WNL.0000000000000278
29. Oki K, Tatarishvili J, Wood J, Koch P, Wattananit S, Mine Y, et al. Human-induced pluripotent stem cells form functional neurons and improve recovery after grafting in stroke-damaged brain. Stem Cells. (2012) 30:1120–33. doi: 10.1002/stem.1104
30. Rosenblum S, Wang N, Smith TN, Pendharkar AV, Chua JY, Birk H, et al. Timing of intra-arterial neural stem cell transplantation after hypoxia–ischemia influences cell engraftment, survival, and differentiation. Stroke. (2012) 43:1624–31. doi: 10.1161/STROKEAHA.111.637884
31. Vawda R, Badner A, Hong J, Mikhail M, Lakhani A, Dragas R, et al. Early intravenous infusion of mesenchymal stromal cells exerts a tissue source age-dependent beneficial effect on neurovascular integrity and neurobehavioral recovery after traumatic cervical spinal cord injury. Stem Cells Transl Med. (2019) 8:639–49. doi: 10.1002/sctm.18-0192
32. Park JW, Ku SH, Moon H-H, Lee M, Choi D, Yang J, et al. Cross-linked iron oxide nanoparticles for therapeutic engineering and in vivo monitoring of mesenchymal stem cells in cerebral ischemia model. Macromol Biosci. (2014) 14:380–9. doi: 10.1002/mabi.201300340
33. Chao F, Shen Y, Zhang H, Tian M. Multimodality molecular imaging of stem cells therapy for stroke. Biomed Res Int. (2013) 2013:849819. doi: 10.1155/2013/849819
34. Modo M, Beech JS, Meade TJ, Williams SCR, Price J. A chronic 1 year assessment of MRI contrast agent-labelled neural stem cell transplants in stroke. Neuroimage. (2009) 47:T133–42. doi: 10.1016/j.neuroimage.2008.06.017
35. Bible E, Dell'acqua F, Solanky B, Balducci A, Crapo PM, Badylak SF, et al. Non-invasive imaging of transplanted human neural stem cells and ECM scaffold remodeling in the stroke-damaged rat brain by 19F- and diffusion-MRI. Biomaterials. (2012) 33:2858–71. doi: 10.1016/j.biomaterials.2011.12.033
36. Nicholls FJ, Rotz MW, Ghuman H, Macrenaris KW, Meade TJ, Modo M. DNA–gadolinium–gold nanoparticles for in vivo T1 MR imaging of transplanted human neural stem cells. Biomaterials. (2016) 77:291–306. doi: 10.1016/j.biomaterials.2015.11.021
37. Srivastava AK, Kadayakkara DK, Bar-Shir A, Gilad AA, Mcmahon MT, Bulte JW. Advances in using MRI probes and sensors for in vivo cell tracking as applied to regenerative medicine. Dis Model Mech. (2015) 8:323–36. doi: 10.1242/dmm.018499
38. Boehm-Sturm P, Aswendt M, Minassian A, Michalk S, Mengler L, Adamczak J, et al. A multi-modality platform to image stem cell graft survival in the naive and stroke-damaged mouse brain. Biomaterials. (2014) 35:2218–26. doi: 10.1016/j.biomaterials.2013.11.085
39. Kustermann E, Himmelreich U, Kandal K, Geelen T, Ketkar A, Wiedermann D, et al. Efficient stem cell labeling for MRI studies. Contrast Media Mol Imaging. (2008) 3:27–37. doi: 10.1002/cmmi.229
40. Srinivas M, Boehm-Sturm P, Aswendt M, Pracht ED, Figdor CG, De Vries IJ, et al. In vivo 19F MRI for cell tracking. J Vis Exp. (2013) 81:e50802. doi: 10.3791/50802
41. Caplan AI. Adult mesenchymal stem cells for tissue engineering versus regenerative medicine. J Cell Physiol. (2007) 213:341–7. doi: 10.1002/jcp.21200
42. Ullah I, Subbarao RB, Rho GJ. Human mesenchymal stem cells—Current trends and future prospective. Biosci Rep. (2015) 35:1–18. doi: 10.1042/BSR20150025
43. Sobrino T, Arias S, Pérez-Mato M, Agulla J, Brea D, Rodríguez-Yáñez M, et al. Cd34+ progenitor cells likely are involved in the good functional recovery after intracerebral hemorrhage in humans. J Neurosci Res. (2011) 89:979–85. doi: 10.1002/jnr.22627
44. Chen J, Zhang ZG, Li Y, Wang L, Xu YX, Gautam SC, et al. Intravenous administration of human bone marrow stromal cells induces angiogenesis in the ischemic boundary zone after stroke in rats. Circ Res. (2003) 92:692–9. doi: 10.1161/01.RES.0000063425.51108.8D
45. Seung-Wan Y, Sung-Soo K, Soo-Yeol L, Hey-Sun L, Hyun-Soo K, Young-Don L, et al. Mesenchimal stem cells promote proliferation of endogenous neural stem cells and survival of newborn cells in a rat stroke model. Exp Mol Med. (2008) 40:387–97. doi: 10.3858/emm.2008.40.4.387
46. Andrews EM, Tsai SY, Johnson SC, Farrer JR, Wagner JP, Kopen GC, et al. Human adult bone marrow-derived somatic cell therapy results in functional recovery and axonal plasticity following stroke in the rat. Exp Neurol. (2008) 211:588–92. doi: 10.1016/j.expneurol.2008.02.027
47. Xin H, Li Y, Cui Y, Yang JJ, Zhang ZG, Chopp M. Systemic administration of exosomes released from mesenchymal stromal cells promote functional recovery and neurovascular plasticity after stroke in rats. J Cereb Blood Flow Metab. (2013) 33:1711–5. doi: 10.1038/jcbfm.2013.152
48. Zhang B, Yin Y, Lai RC, Tan SS, Choo ABH, Lim SK. Mesenchymal stem cells secrete immunologically active exosomes. Stem Cells Dev. (2014) 23:1233–44. doi: 10.1089/scd.2013.0479
49. Zhang Y, Chopp M, Meng Y, Katakowski M, Xin H, Mahmood A, et al. Effect of exosomes derived from multipluripotent mesenchymal stromal cells on functional recovery and neurovascular plasticity in rats after traumatic brain injury. J Neurosurg. (2015) 122:856–67. doi: 10.3171/2014.11.JNS14770
50. Gutiérrez-Fernández M, Rodríguez-Frutos B, Ramos-Cejudo J, Teresa Vallejo-Cremades M, Fuentes B, Cerdán S, et al. Effects of intravenous administration of allogenic bone marrow- and adipose tissue-derived mesenchymal stem cells on functional recovery and brain repair markers in experimental ischemic stroke. Stem Cell ResTher. (2013) 4:11. doi: 10.1186/scrt159
51. Chen K, Chen C, Wallace C, Yuen C, Kao G, Chen Y, et al. Intravenous administration of xenogenic adipose-derived mesenchymal stem cells (ADMSC) and ADMSC-derived exosomes markedly reduced brain infarct volume and preserved neurological function in rat after acute ischemic stroke. Oncotarget. (2016) 7:74537–56. doi: 10.18632/oncotarget.12902
52. Ra JC, Shin IS, Kim SH, Kang SK, Kang BC, Lee HY, et al. Safety of intravenous infusion of human adipose tissue-derived mesenchymal stem cells in animals and humans. Stem Cells Dev. (2011) 20:1297–308. doi: 10.1089/scd.2010.0466
53. Wang S-P, Wang Z-H, Peng D-Y, Li S-M, Wang H, Wang X-H. Therapeutic effect of mesenchymal stem cells in rats with intracerebral hemorrhage: reduced apoptosis and enhanced neuroprotection. Mol Med Rep. (2012) 6:848–54. doi: 10.3892/mmr.2012.997
54. Vaquero J, Otero L, Bonilla C, Aguayo C, Rico MA, Rodriguez A, et al. Cell therapy with bone marrow stromal cells after intracerebral hemorrhage: impact of platelet-rich plasma scaffolds. Cytotherapy. (2013) 15:33–43. doi: 10.1016/j.jcyt.2012.10.005
55. Nakazaki M, Sasaki M, Kataoka-Sasaki Y, Oka S, Namioka T, Namioka A, et al. Intravenous infusion of mesenchymal stem cells inhibits intracranial hemorrhage after recombinant tissue plasminogen activator therapy for transient middle cerebral artery occlusion in rats. J Neurosurg. (2017) 127:917–26. doi: 10.3171/2016.8.JNS16240
56. Pérez-Mato M, Iglesias-Rey R, Vieites-Prado A, Dopico-López A, Argibay B, Fernández-Susavila H, et al. Blood glutamate EAAT2-cell grabbing therapy in cerebral ischemia. EBio Med. (2018). doi: 10.1016/j.ebiom.2018.11.024
57. Bond AM, Ming G-L, Song H. Adult mammalian neural stem cells and neurogenesis: five decades later. Cell Stem Cell. (2015) 17:385–95. doi: 10.1016/j.stem.2015.09.003
58. Jin K, Wang X, Xie L, Mao XO, Zhu W, Wang Y, et al. Evidence for stroke-induced neurogenesis in the human brain. Proc Natl Acad Sci USA. (2006) 103:13198–202. doi: 10.1073/pnas.0603512103
59. Thored P, Arvidsson A, Cacci E, Ahlenius H, Kallur T, Darsalia V, et al. Persistent production of neurons from adult brain stem cells during recovery after stroke. Stem Cells. (2006) 24:739–47. doi: 10.1634/stemcells.2005-0281
60. Schaapsmeerders P, Maaijwee NAM, Van Dijk EJ, Rutten-Jacobs LCA, Arntz RM, Schoonderwaldt HC, et al. Long-term cognitive impairment after first-ever ischemic stroke in young adults. Stroke. (2013) 44:1621–8. doi: 10.1161/STROKEAHA.111.000792
61. Bacigaluppi M, Pluchino S, Jametti LP, Kilic E, Kilic Ü., Salani G, et al. Delayed post-ischaemic neuroprotection following systemic neural stem cell transplantation involves multiple mechanisms. Brain. (2009) 132:2239–51. doi: 10.1093/brain/awp174
62. Bacigaluppi M, Russo GL, Peruzzotti-Jametti L, Rossi S, Sandrone S, Butti E, et al. Neural stem cell transplantation induces stroke recovery by upregulating glutamate transporter GLT-1 in astrocytes. J Neurosci. (2016) 36:10529–44. doi: 10.1523/JNEUROSCI.1643-16.2016
63. Eckert A, Huang L, Gonzalez R, Kim H-S, Hamblin MH, Lee J-P. Bystander effect fuels human induced pluripotent stem cell-derived neural stem cells to quickly attenuate early stage neurological deficits after stroke. Stem Cells Transl Med. (2015) 4:841–51. doi: 10.5966/sctm.2014-0184
64. Baker EW, Platt SR, Lau VW, Grace HE, Holmes SP, Wang L, et al. Induced pluripotent stem cell-derived neural stem cell therapy enhances recovery in an ischemic stroke pig model. Sci Rep. (2017) 7:10075. doi: 10.1038/s41598-017-10406-x
65. Sakata H, Niizuma K, Wakai T, Narasimhan P, Maier CM, Chan PH. Neural stem cells genetically modified to overexpress Cu/Zn-superoxide dismutase enhance amelioration of ischemic stroke in mice. Stroke. (2012) 43:2423–9. doi: 10.1161/STROKEAHA.112.656900
66. Wakai T, Sakata H, Narasimhan P, Yoshioka H, Kinouchi H, Chan PH. Transplantation of neural stem cells that overexpress sod1 enhances amelioration of intracerebral hemorrhage in mice. J Cereb Blood Flow Metab. (2013) 34:441–9. doi: 10.1038/jcbfm.2013.215
67. Theus MH, Wei L, Cui L, Francis K, Hu X, Keogh C, et al. In vitro hypoxic preconditioning of embryonic stem cells as a strategy of promoting cell survival and functional benefits after transplantation into the ischemic rat brain. Exp Neurol. (2008) 210:656–70. doi: 10.1016/j.expneurol.2007.12.020
68. Sakata H, Niizuma K, Yoshioka H, Kim GS, Jung JE, Katsu M, et al. Minocycline-preconditioned neural stem cells enhance neuroprotection after ischemic stroke in rats. J Neurosci. (2012) 32:3462–73. doi: 10.1523/JNEUROSCI.5686-11.2012
69. Zhong J, Chan A, Morad L, Kornblum HI, Guoping F, Carmichael ST. Hydrogel matrix to support stem cell survival after brain transplantation in stroke. Neurorehabil Neural Repair. (2010) 24:636–44. doi: 10.1177/1545968310361958
70. Chan HH, Wathen CA, Ni M, Zhuo S. Stem cell therapies for ischemic stroke: current animal models, clinical trials and biomaterials. RSC Adv. (2017) 7:18668–80. doi: 10.1039/C7RA00336F
71. Jensen MB, Yan H, Krishnaney-Davison R, Al Sawaf A, Zhang S-C. Survival and differentiation of transplanted neural stem cells derived from human induced pluripotent stem cells in a rat stroke model. J Stroke Cerebrovasc Dis. (2013) 22:304–8. doi: 10.1016/j.jstrokecerebrovasdis.2011.09.008
72. Jeong S-W, Chu K, Jung K-H, Kim SU, Kim M, Roh J-K. Human Neural Stem Cell Transplantation promotes functional recovery in rats with experimental intracerebral hemorrhage. Stroke. (2003) 34:2258–63. doi: 10.1161/01.STR.0000083698.20199.1F
73. Lee HJ, Kim KS, Kim EJ, Choi HB, Lee KH, Park IH, et al. Brain transplantation of immortalized human neural stem cells promotes functional recovery in mouse intracerebral hemorrhage stroke model. Stem Cells. (2007) 25:1204–12. doi: 10.1634/stemcells.2006-0409
74. Chen S-J, Chang C-M, Tsai S-K, Chang Y-L, Chou S-J, Huang S-S, et al. Functional improvement of focal cerebral ischemia injury by subdural transplantation of induced pluripotent stem cells with fibrin glue. Stem Cells Dev. (2010) 19:1757–67. doi: 10.1089/scd.2009.0452
75. Jiang M, Lv L, Ji H, Yang X, Zhu W, Cai L, et al. Induction of pluripotent stem cells transplantation therapy for ischemic stroke. Mol Cell Biochem. (2011) 354:67–75. doi: 10.1007/s11010-011-0806-5
76. Tornero D, Wattananit S, Grønning Madsen M, Koch P, Wood J, Tatarishvili J, et al. Human induced pluripotent stem cell-derived cortical neurons integrate in stroke-injured cortex and improve functional recovery. Brain. (2013) 136:3561–77. doi: 10.1093/brain/awt278
77. Qin J, Ma X, Qi H, Song B, Wang Y, Wen X, et al. Transplantation of induced pluripotent stem cells alleviates cerebral inflammation and neural damage in hemorrhagic stroke. PLoS ONE. (2015) 10:e0129881. doi: 10.1371/journal.pone.0129881
78. Takagi Y, Nishimura M, Morizane A, Takahashi J, Nozaki K, Hayashi J. Survival and differentiation of neural progenitor cells derived from embryonic stem cells and transplanted into ischemic brain. J Neurosurg. (2005) 103:304–10. doi: 10.3171/jns.2005.103.2.0304
79. Buhnemann C, Scholz A, Bernreuther C, Malik CY, Braun H, Schachner M, et al. Neuronal differentiation of transplanted embryonic stem cell-derived precursors in stroke lesions of adult rats. Brain. (2006) 129:3238–48. doi: 10.1093/brain/awl261
80. Marei HE, Hasan A, Rizzi R, Althani A, Afifi N, Cenciarelli C, et al. Potential of stem cell-based therapy for ischemic stroke. Front Neurol. (2018) 9:34. doi: 10.3389/fneur.2018.00034
81. Lee AS, Tang C, Rao MS, Weissman IL, Wu JC. Tumorigenicity as a clinical hurdle for pluripotent stem cell therapies. Nat Med. (2013) 19:998–1004. doi: 10.1038/nm.3267
82. Lee MO, Moon SH, Jeong HC, Yi JY, Lee TH, Shim SH, et al. Inhibition of pluripotent stem cell-derived teratoma formation by small molecules. Proc Natl Acad Sci USA. (2013) 110:E3281–90. doi: 10.1073/pnas.1303669110
83. Chambers SM, Fasano CA, Papapetrou EP, Tomishima M, Sadelain M, Studer L. Highly efficient neural conversion of human ES and iPS cells by dual inhibition of SMAD signaling. Nat Biotechnol. (2009) 27:275–80. doi: 10.1038/nbt.1529
84. Dash BC, Jiang Z, Suh C, Qyang Y. Induced pluripotent stem cell-derived vascular smooth muscle cells: methods and application. Biochem J. (2015) 465:185–94. doi: 10.1042/BJ20141078
85. Harding A, Cortez-Toledo E, Magner NL, Beegle JR, Coleal-Bergum DP, Hao D, et al. Highly efficient differentiation of endothelial cells from pluripotent stem cells requires the MAPK and the PI3K pathways. Stem Cells. (2017) 35:909–19. doi: 10.1002/stem.2577
86. Bang OY, Kim EH, Cha JM, Moon GJ. Adult stem cell therapy for stroke: challenges and progress. J Stroke. (2016) 18:256–66. doi: 10.5853/jos.2016.01263
87. Dihné M, Hartung H-P, Seitz RJ. Restoring neuronal function after stroke by cell replacement. Stroke. (2011) 42:2342–50. doi: 10.1161/STROKEAHA.111.613422
88. Joutel A, Faraci FM. Cerebral small vessel disease (SVD): insights and opportunities from mouse models of collagen IV-related SVD and CADASIL. Stroke. (2014) 45:1215–21. doi: 10.1161/STROKEAHA.113.002878
89. Ayata C. CADASIL: experimental insights from animal models. Stroke. (2010) 41:S129–34. doi: 10.1161/STROKEAHA.110.595207
90. Coupland K, Lendahl U, Karlström H. Role of NOTCH3 mutations in the cerebral small vessel disease cerebral autosomal dominant arteriopathy with subcortical infarcts and leukoencephalopathy. Stroke. (2018) 49:2793–800. doi: 10.1161/STROKEAHA.118.021560
91. Moniche F, Gonzalez A, Gonzalez-Marcos J-R, Carmona M, Piñero P, Espigado I, et al. Intra-arterial bone marrow mononuclear cells in ischemic stroke. Stroke. (2012) 43:2242–4. doi: 10.1161/STROKEAHA.112.659409
92. Prasad K, Sharma A, Garg A, Mohanty S, Bhatnagar S, Johri S, et al. Intravenous autologous bone marrow mononuclear stem cell therapy for ischemic stroke. Stroke. (2014) 45:3618–24. doi: 10.1161/STROKEAHA.114.007028
93. Díez-Tejedor E, Gutiérrez-Fernández M, Martínez-Sánchez P, Rodríguez-Frutos B, Ruiz-Ares G, Lara ML, et al. Reparative therapy for acute ischemic stroke with allogeneic mesenchymal stem cells from adipose tissue: a safety assessment. J Stroke Cerebrovasc Dis. (2014) 23:2694–700. doi: 10.1016/j.jstrokecerebrovasdis.2014.06.011
94. Savitz SI, Misra V, Kasam M, Juneja H, Cox CS, Alderman S, et al. Intravenous autologous bone marrow mononuclear cells for ischemic stroke. Ann Neurol. (2011) 70:59–69. doi: 10.1002/ana.22458
95. Kim S, Moon G, Chang W, Kim Y, Bang O, Collaborators S.-S.C.a.R.a.T.I.N. Intravenous transplantation of mesenchymal stem cells preconditioned with early phase stroke serum: current evidence and study protocol for a randomized trial. TRIALS. (2013) 14:317. doi: 10.1186/1745-6215-14-317
96. Hess DC, Wechsler LR, Clark WM, Savitz SI, Ford GA, Chiu D, et al. Safety and efficacy of multipotent adult progenitor cells in acute ischaemic stroke (MASTERS): a randomised, double-blind, placebo-controlled, phase 2 trial. Lancet Neurol. (2017) 16:360–8. doi: 10.1016/S1474-4422(17)30046-7
97. Banerjee S, Bentley P, Hamady M, Marley S, Davis J, Shlebak A, et al. Intra-arterial immunoselected CD34+ stem cells for acute ischemic stroke. Stem Cells Transl Med. (2014) 3:1322–30. doi: 10.5966/sctm.2013-0178
98. Kalladka D, Sinden J, Pollock K, Haig C, Mclean J, Smith W, et al. Human neural stem cells in patients with chronic ischaemic stroke (PISCES): a phase 1, first-in-man study. Lancet. (2016) 388:787–96. doi: 10.1016/S0140-6736(16)30513-X
99. Steinberg GK, Kondziolka D, Wechsler LR, Lunsford LD, Coburn ML, Billigen JB, et al. Clinical outcomes of transplanted modified bone marrow–derived mesenchymal stem cells in stroke. Stroke. (2016) 47:1817–24. doi: 10.1161/STROKEAHA.116.012995
100. Taylor CJ, Peacock S, Chaudhry AN, Bradley JA, Bolton EM. Generating an iPSC bank for HLA-matched tissue transplantation based on known donor and recipient HLA types. Cell Stem Cell. (2012) 11:147–52. doi: 10.1016/j.stem.2012.07.014
101. Solomon S, Pitossi F, Rao MS. Banking on iPSC—Is it doable and is it worthwhile. Stem Cell Rev Rep. (2014) 11:1–10. doi: 10.1007/s12015-014-9574-4
Keywords: adult stem cell, cell therapy, clinical trial, induced pluripotent stem cell, stroke
Citation: Fernández-Susavila H, Bugallo-Casal A, Castillo J and Campos F (2019) Adult Stem Cells and Induced Pluripotent Stem Cells for Stroke Treatment. Front. Neurol. 10:908. doi: 10.3389/fneur.2019.00908
Received: 19 May 2019; Accepted: 05 August 2019;
Published: 28 August 2019.
Edited by:
Isabelle Loubinoux, INSERM U1214 Centre d'Imagerie Neuro Toulouse (ToNIC), FranceReviewed by:
María Gutiérrez Fernández, University Hospital La Paz, SpainMathias Hoehn, Max-Planck-Gesellschaft (MPG), Germany
Copyright © 2019 Fernández-Susavila, Bugallo-Casal, Castillo and Campos. This is an open-access article distributed under the terms of the Creative Commons Attribution License (CC BY). The use, distribution or reproduction in other forums is permitted, provided the original author(s) and the copyright owner(s) are credited and that the original publication in this journal is cited, in accordance with accepted academic practice. No use, distribution or reproduction is permitted which does not comply with these terms.
*Correspondence: Francisco Campos, ZnJhbmNpc28uY2FtcG9zLnBlcmV6JiN4MDAwNDA7c2VyZ2FzLmVz