- 1Department of Experimental Psychology, University of Oxford, Oxford, United Kingdom
- 2Chronobiology Unit, Groningen Institute for Evolutionary Life Sciences, University of Groningen, Groningen, Netherlands
The human pupillary light response is driven by all classes of photoreceptors in the human eye—the three classes of cones, the rods, and the intrinsically photosensitive retinal ganglion cells (ipRGCs) expressing the photopigment melanopsin. These photoreceptor classes have distinct but overlapping spectral tuning, and even a monochromatic light with a wavelength matched to the peak spectral sensitivity of a given photoreceptor will stimulate all photoreceptors. The method of silent substitution uses pairs of lights (“metamers”) to selectively stimulate a given class of photoreceptors while keeping the activation of all others constant. In this primer, we describe the method of silent substitution and provide an overview of studies that have used it to examine inputs to the human pupillary light response.
Introduction
At the input level, the size of the pupil is controlled by the activity of the different photoreceptors in the human eye (1). These different photoreceptors differ in many respects: their wavelength tuning (spectral sensitivity), their temporal properties, their operating range and their distribution across the retina. The goal of this primer is to describe the method of silent substitution for examining photoreceptor-specific pupil responses. We start with the fundamentals underlying the method of silent substitution, provide an overview of studies that have used this method, provide a practical guide and R code to implement silent substitution and highlight a few challenges to the method of silent substitution.
Fundamentals
Overlapping Spectral Sensitivities of the Human Photoreceptors
Photoreception in the human retina is based on the signals produced by the three types of cones—the long[L]-wavelength-sensitive cones, the medium[M]-wavelength-sensitive cones, and the short[S]-wavelength-sensitive cones—, the rods, and the intrinsically photosensitive retinal ganglion cells (ipRGCs), which contain the photopigment melanopsin (2–6). ipRGCs receive synaptic input from cones and rods but, in the absence of those inputs, these cells themselves are photosensitive due to the expression of the melanopsin photopigment in the cell membrane. The peak spectral sensitivities (λmax) of the human photoreceptors are distinct. The photopigments (cone opsins) in the L, M, and S cones peak around 420, 530, and 558 nm, respectively; rhodopsin, the pigment in rods, has a peak at around 495 nm. Finally, the melanopsin photopigment has a peak spectral sensitivity at around 480 nm. Even though these peaks are spectrally distinct and distant, the spectral sensitivities overlap quite extensively due to the relative broadband tuning of photopigments (Figure 1A). One challenge in targeting the operation of a single class of photoreceptor is that the spectral sensitivities of the photoreceptors in vivo does not necessarily correspond to the spectral sensitivity of a pigment. All light that reaches the retina is filtered by the lens and ocular media (7), thereby shifting the effective spectral sensitivity. Typically, this pre-receptoral filtering is accounted for in the spectral sensitivities for cones, rods, and melanopsin-containing ipRGCs.
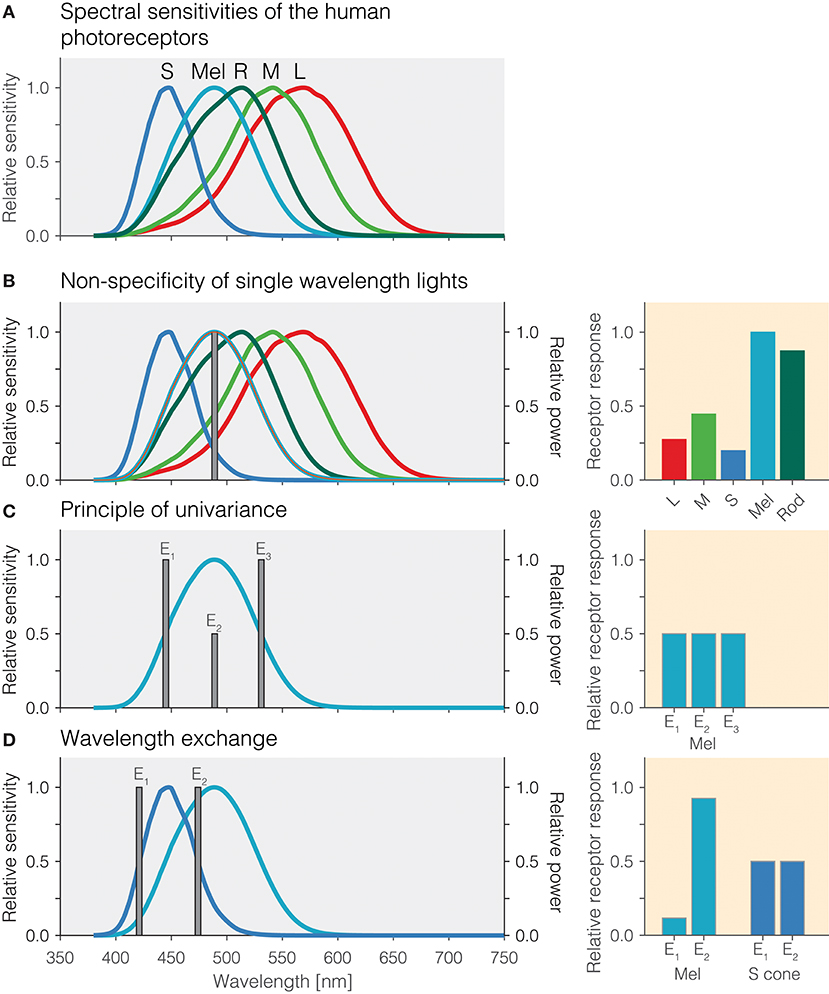
Figure 1. (A) Overlapping spectral sensitivities of the human photoreceptors. (B) Non-specificity of single-wavelength lights. Right panel: Pattern of photoreceptor responses to the single-wavelength light at 490 nm. (C) Principle of univariance. Right panel: Pattern of photoreceptor responses to the single-wavelength lights E1, E2, and E3 designed to elicit the same response in melanopsin. (D) Wavelength exchange between two short-wavelength lights E1 and E2 which stimulate S cones at the same level but yield different photoreceptor responses for melanopsin. Right panel: Pattern of excitations for lights E1 and E2.
Non-specificity of Single-Wavelength Lights
An important desideratum for examining how the different photoreceptors contribute to the human pupillary light response is that stimuli produce responses specific to a given photoreceptor class. One consequence of the extensive spectral overlap of the photoreceptors is that most light sources activate all photoreceptors, and therefore, the responses elicited are largely nonspecific. For example, monochromatic light with a peak spectral output of 490 nm will activate melanopsin maximally relative to the other photoreceptors, but it will also lead to substantial activation of rods and the cones (Figure 1B). The relative amounts by which a monochromatic light of a given wavelength activates all photoreceptors is directly predicted from the relative spectral sensitivity of the photoreceptors at that wavelength. Monochromatic lights have been of great use in determining the spectral sensitivity of the sustained pupil constrictions that match that of melanopsin (8–11). This specific type of measurement is called the “post-illumination pupil response,” abbreviated PIPR, in which the pupil response is typically measured in response to a non-specific short-wavelength light flash and a non-specific long-wavelength light flash against a dim or no background.
Principle of Univariance
One property of photoreceptors is the principle of univariance (12), which states that a given photoreceptor has only scalar output, namely its photocurrent: It cannot distinguish between changes in intensity and changes in wavelength. This is shown in Figure 1C using theoretical lights containing power at only a single wavelength (monochromatic light): Lights E1, E2, and E3 all nominally elicit the same photoreceptor excitation. Lights E1 and E3 have their peak emission at the 50% point of spectral sensitivity on either side of the peak; light E2 is scaled to be 50% of the emission of lights E1 and E3. To the photoreceptor (in this case melanopsin), which weights the input light by its spectral sensitivity, the lights are equally effective. The key insight is that photoreceptors integrate light of different wavelengths, weighting the input spectrum by their spectral sensitivity and summing it up. A consequence of the principle of univariance is that single photoreceptors are color-blind: Whether two lights differ in wavelength or intensity cannot be determined from the photoreceptor output alone.
Wavelength Exchange
Because photoreceptors weight input light by their spectral sensitivity function, in the case of two photoreceptors, it is possible to find two lights and scale them such that the excitation of one of the photoreceptors remains constant in this wavelength exchange, while the other one “sees” a difference. This is shown in Figure 1D: The peak emissions of lights E1 and E2 have been chosen to match the two 50% points of the S-cone spectral sensitivity, thereby eliciting the same responses. This is called silencing the S cones. Because the spectral sensitivity of melanopsin is different from that of the S cones, our two lights E1 and E2 necessarily produce a different response, and in this case we call melanopsin the stimulated photoreceptor. Wavelength exchange for two photopigments is the most simple case of silent substitution. But, with the exception of certain classes of color-blindness such as rod monochromacy, the human retina contains five photoreceptors. Fortunately, the same principle can be extended to more than two photoreceptors.
The Method of Silent Substitution
In the method of silent substitution, pairs of light are found that have the property that they stimulate the targeted photoreceptor class (or classes) whilst not changing the excitation of the other photoreceptors, the silenced ones. The method has a long history for determining the properties of the mechanisms of human color vision (13, 14).
Fundamentals
To introduce the method of silent substitution we begin with an example from human color vision. Human color vision is trichromatic under daylight conditions, i.e., when rods do not participate: A color-normal observer can match the color appearance of any light using a combination of three primary lights (15). Under these conditions, it is assumed that only the three classes of cones participate in the color match; it follows that because three photoreceptors participate, three independent primary lights need to be used. It is impossible to match the activation of three photoreceptors in one condition using just two primary lights.
In general, to stimulate one class of photoreceptor classes out of NR photoreceptor classes while leaving the activation of the other NR−1 unchanged, at least NR primary lights (Np) are necessary. When NR = NP (i. e. there are as many primary lights as photoreceptor classes under consideration), there is only one algebraic solution to match the activation of the NR−1 photoreceptors under one set of settings for the NP lights to another other setting that will only stimulate the remaining photoreceptor class.
For the case of four photoreceptor classes in the human retina (three classes of cones and melanopsin), four lights are necessary to match the activation of cones and stimulate melanopsin. When including the rods, five lights are necessary to match the activation of cones and rods and stimulate melanopsin.
It is possible to have more primary lights than photoreceptors under consideration, i.e., NR < NP. This would for example be the case when there were, e.g., eight independent primaries and the retina to be studied was a human one. In that case, there are infinitely many solutions to match the activation of the NR−1 photoreceptors under one set of settings for the NP lights to any other setting that will only stimulate the remaining photoreceptor class. In practice, this is typically solved by implementing a numerical optimization which maximizes the contrast seen by the stimulated photoreceptor while setting a constraint to have no contrast on the unstimulated ones, and enforcing additional constraints on the optimisation.
Contrast
The term contrast refers to a specific quantity, which is the fractional difference of activation of a photopigment around a background:
Intuitively, when the light-adapted background activates a given photoreceptor by some amount, e.g., 100 (arbitrary units), and the modulation activates it by a higher amount, e.g., 120 (arbitrary units), the contrast in that case would be 0.2 or 20%. Contrast can be specified either as fractions or as percentages.
An Intuitive Example
We now describe an example case for the method of silent substitution corresponding to the stimuli used in Spitschan, Jain, Brainard and Aguirre (16). These authors used a calibrated spectrally tuneable light source that modified the output of a broadband Xenon arc lamp using a digital micromirror device (DMD) to produce, effectively, arbitrary spectral power distributions. While this is a special case of light sources, most experimenters have used a set of discrete lights, the intensities of which are controlled to produce silent substitution stimuli. The goal is to produce two lights with spectral power distributions that do not differ in the amount they activate the cones, and only yield a change in the amount they activate melanopsin. Such pairs of stimuli are also called metamers–they are indistinguishable to the cones, despite having different spectral power distributions. In this example, we ignore the rods.
1. Background spectrum: In the first instance, we begin with a background spectrum of known spectral power distribution (Figure 2A). We call this the background spectrum because the observer is typically light-adapted to this spectrum, and the silent-substitution stimuli are shown to the observer “around” this background in the form of pulses or temporal modulations. This background spectrum elicits a pattern of photoreceptors responses (Figure 2B, right panel). The activation of photoreceptors is calculated by weighting the spectrum by the spectral sensitivities and summing it up for each photoreceptor class.
2. Increasing melanopsin activation: Pragmatically, we can increase the amount of light seen by melanopsin by simply increasing the amount of light emitted near the melanopsin peak. This is shown in Figure 2B. However, this is only partly successful: Because of the overlapping peak spectral sensitivities of the human photoreceptors, such an increase in emitted light leads to an increase in activation of all photoreceptors (Figure 2B, middle panel). Rather than considering the absolute amount of activation of the photoreceptors (which is also dependent on the exact light level), it is customary to speak of contrast (Figure 2B, right panel). Contrast here refers to the percentage difference in activation of photoreceptors between the modulation spectrum (red line in Figure 2B) and the background spectrum (Figure 2A and dashed line in Figures 2B–F). As can be seen in the right panel in Figure 2B, the increase in light near the melanopsin peak leads to an increase in contrast to all photoreceptors. To reiterate, the desideratum here is to have no contrast seen by L, M and S cones, and positive contrast seen by melanopsin.
3. Silencing S cones: To zero, or silence, the S cones, we decrease the amount of short-wavelength light, to which the S cones are most sensitive (Figure 2C). This indeed leads to a silencing of S cones (Figure 2C, middle panel). There is no difference in the absolute activation of S cones, and consequently, the S cone contrast is zero–they are silent.
4. Silencing L and M cones: To silence the L and M cones, a similar trick is applied: Light near the peak spectral sensitivity of L and M cones is decreased to reduce the overall absolute activation of L and M cones (Figure 2D). However, we note that there has been an “overshoot” in the decrease in L and M cones activation (Figure 2D, middle panel): The modulation spectrum is now producing less activation in the L and M cones than the background spectrum. This translates into a small amount of negative contrast seen by the L and M cones (Figure 2D, right panel). This can be overcome by again increasing the amount of long-wavelength light in the modulation spectrum (Figure 2E), thereby equalizing the activation of L and M cones relative to the background spectrum (Figure 2D, middle panel). The L, M and S cones are now silent (Figure 2E, right panel), and melanopsin is stimulated at 50%. Because no attempt was taken to silence the rods, they are also stimulated by this spectral exchange.
5. Inverting the melanopsin activation: The modulation spectrum shown in Figure 2E (red line) produces a significant increase in melanopsin excitation. By “mirroring” the modulation spectrum around the background spectrum (i.e., an increase in emitted light in the positive modulation spectrum becomes a decrease by the same amount in emitted light in the negative modulation spectrum), we can also generate a negative (rather than a positive) melanopsin stimulus (Figure 2F, blue line), thereby producing negative, or decremental, contrast on melanopsin (Figure 2F, right panel). In practice, negative and positive modulation spectra are alternated to yield the highest differential activation possible.
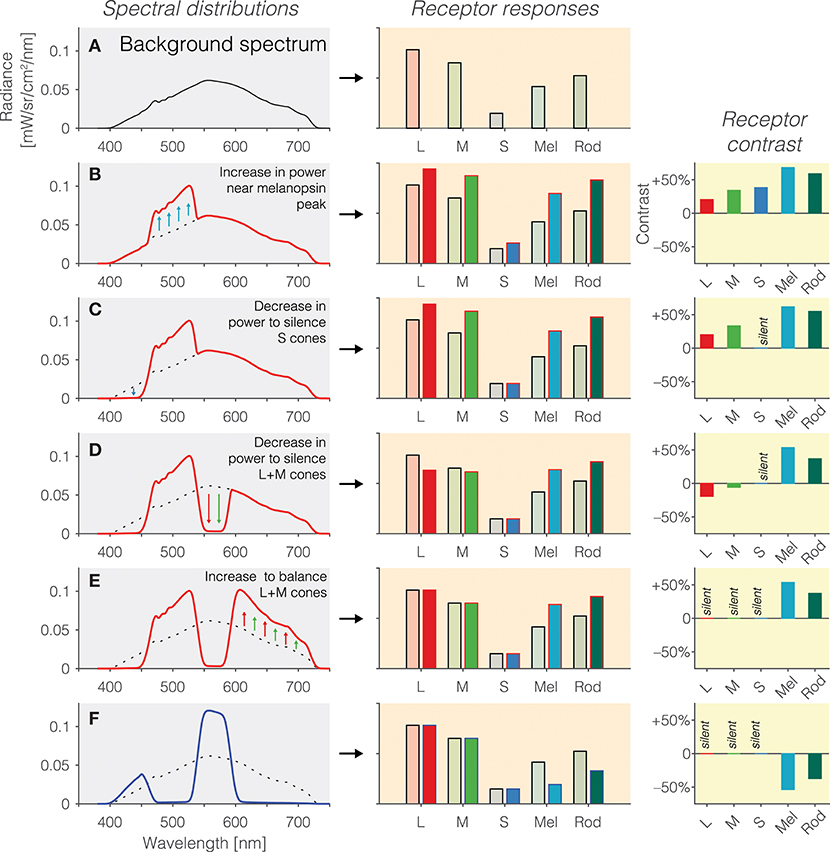
Figure 2. (A) Background spectrum (left panel) to which the observer is light-adapted, eliciting a pattern of responses in the photoreceptors (right panel). (B) Increase in emitted light near the melanopsin peak relative to the background spectrum (left panel; dashed line = background spectrum, red line = modulation spectrum) leads to an increase in the excitation of all photoreceptors (middle panel), or equivalently, positive contrast on the photoreceptors (right panel). (C) To balance the excitation of the S cones, a decrease in emitted short-wavelength light (left panel) leads to silencing of the S cones (middle panel), or equivalently, zero contrast on the S cones (right panel). (D) To balance the excitation of the L and M cones, a decrease in emitted medium-wavelength light (left panel) leads to a reduction in L and M cone activity (middle panel) but not yet zero contrast on the L and M cones (right panel); indeed, the contrast seen by the L and M cones is now negative. (E) To silence the excitation of the L and M cones, a decrease in emitted long-wavelength light (left panel) leads to balancing of the L and M cones (middle panel), or equivalently, zero contrast on the L and M cones (right panel). The contrast seen by melanopsin is 50%. (F) The modulation spectrum shown in (E) yields positive contrast relative to the background spectrum but the spectrum can also be “mirrored” around the background spectrum, thereby leading to a negative modulation of melanopsin (and rods).
A Quantitative Example
We provide a quantitative example along with code in Appendices A1, A2, respectively. We use the stimuli from Woelders et al. (17) for this example.
History of Silent Substitution
The method of silent substitution has enjoyed use in empirical work well before the discovery of melanopsin and the ipRGCs. We point the reader to Estévez and Spekreijse (13) for an exposé of the early history of the method, which indeed dates back to experiments involving wavelength exchanges performed in 1906 (18) (see above section “Wavelength exchange”). From the insight that metameric lights such as those obtained in color matching experiments are silent substitution stimuli (i.e., matching the activation of the three cone types), the extension to experimentally control more photoreceptors is conceptually straightforward. In the 1990s, methods to manipulate four photoreceptors independently (three cone classes and rods) using mixtures of four primary lights were developed (18, 19). These methods were then expanded to examining melanopsin function either by assuming rod saturation at high light levels (20), or using five primaries (21).
Overview of Silent Substitution Studies Concerning the Pupil
We provide an overview of extant studies examining specifically melanopsin photoreception using the method of silent substitution in Table 1 and hope that it serves to the reader as an orientation to the literature. This overview includes literature available in early September 2018. We note that both authors of this article have published papers using the method of silent substitution which are included in the table [M.S.: (16, 28), T.W.: (17)]. The table shows that there is a set of experimental parameters that are subject to the experimenters' discretion. We summarise these here.
Number of Primaries
As described above (The method of silent substitution–Fundamentals), when stimulating melanopsin, at least four (for matching the cones) or five (for matching both cones and rods) independent primary lights are necessary. Most silent substitution studies that have examined pupil responses to photoreceptor-specific modulations have employed a finite set of LEDs (four or five), though using spectrally tuneable light sources, more effective primaries are possible.
Peak Wavelength and Width of the Primary Lights
In the case where the primary lights are discrete (such as LEDs), the peak emission wavelengths are subject to design considerations when building the apparatus. Both the choice of peak wavelengths and primary widths affects the contrast available for the silent substitution modulations. The contrast available is also called the gamut. In principle, choosing broader primaries will reduce also the amount of susceptibility to individual differences in the cone spectral sensitivities (29). In practice, unless a spectrally tuneable light source is used allowing to create arbitrary spectral power distributions, the choices of primary wavelengths and widths is limited by what is commercially available. When building a system, we recommend first estimating the gamut for a given configuration of peak wavelengths and widths.
Viewing Geometry
Typical viewing geometries include Ganzfeld viewing conditions (in which the stimulus is a homogenous field in an integrating sphere) or Maxwellian view (in which an image is focused on the entrance pupil of the observer). These again depend on the type of design used when building the stimulation system.
Field Size
As can be seen in the table, the field sizes used in the field vary somewhat, and will again depend on constraints set by the optical apparatus used to deliver the stimuli, as well as theoretical considerations such as the distribution of the photoreceptor types across the retina.
Modulations and Contrast
Depending on the spectra of the primary lights, different amounts of contrast are available to stimulate melanopsin. Typically, the highest contrast can be achieved when LEDs are chosen of which the distribution of peak wavelengths is as broad as possible.
Background Light
The choice of background light level is again somewhat arbitrary in many situations, though experimenters typically strive to be well in photopic conditions, where rods are assumed to be saturated, and can therefore be ignored (but see Rod intrusion below).
Spectral Sensitivities Assumed
The extent to which a given melanopsin-stimulating modulation silences the cones depends on the spectral sensitivities assumed. Various spectral sensitivities are available (30). Choosing the wrong spectral sensitivities can lead to artefactual results, unless care is taken to correct the modulations. We recommend the use of the CIE 2006 “physiologically relevant” cone fundamentals (31) as it allows for flexible extensions to simulate individual differences parametrically (32). For melanopsin, there is currently no standard(ised) spectral sensitivity, though by using a template (also called nomogram) centered at 480 nm and assuming a low peak optical density, such a spectral sensitivity can easily be derived (33, 34).
Challenges to Silent Substitution
There are various sources of uncertainty when using silent substitution stimuli. We highlight a few of these here.
Retinal Inhomogenities
The human retina is inhomogeneous. One obvious feature of the retina making it inhomogeneous is the spatial location of the macular pigment around the fovea, with a drop-off toward the periphery. A consequence of macular pigment is that all light seen by the fovea is filtered through the pigment, thereby shifting the effective peak spectral sensitivity of the foveal cones vs. the peripheral cones. In addition, there are also differences in how much photopigment is expressed in foveal vs. peripheral cones—the optical densities are different. Another source of retinal inhomogeneity is that cones that are in the partial shadow of retinal blood vessels—penumbral cones—have a different spectral sensitivity than the open-field cones (35). Effectively, for the method of silent substitution, this means that that there are three additional photoreceptor classes that need to be silenced, and therefore, more primaries are necessary. Practically, penumbral cones can be desensitized using a white-noise stimulus (26), or silenced, though with a significant drop in contrast (36).
Individual Differences in the Cone Spectral Sensitivities
There are individual differences in the spectral sensitivities of the cones and this biological variability will affect the degree to which the cones are truly silenced in a melanopsin-directed modulation. Inter-observer differences have been a concern in the accurate specification of cone signals well before the discovery of melanopsin (37–39). Biological variability arises from inter-observer variability in lens density, macular pigment density, taxial density of the pigment (32, 37–39); and the peak spectral sensitivity due to polymorphisms in the opsin genes (40–43). A given set of cone fundamentals only describes the average spectral sensitivities within a population and ignoring biological variability will introduce error. In the field of melanopsin-mediated pupillometry, some experimenters correct the stimuli by having the observers perform a color matching procedure (25, 26), while others (16, 17, 28) simulate the variability of the stimuli using simulations based on estimates of the biological variability of parameters of the cones (32).
Melanopsin Bistability and Tristability
The method of silent substitution assumes that melanopsin the spectral sensitivity of melanopsin can be described by a single function. There is ample evidence that melanopsin is a bistable (44–49) or tristable photopigment (50). While the cone and rod photopigment is regenerated in the retinal pigment epithelium (RPE), melanopsin, being expressed in ipRGCs in the inner retina, and therefore removed from the RPE is thought to rely on a different mechanism for pigment regeneration. A bistable (or tristable) photopigment relies on light itself to regenerate the pigment, and that this regeneration process again is wavelength-dependent and therefore has a separate spectral sensitivity. It is controversial whether the multistable photochemistry of melanopsin has physiological consequences (51–53). Under conditions of adaptating to a constant background light as employed in silent substitution, melanopsin will be in photoequlibrium, i.e., the different states of the pigment will exist in fixed (though possibly unknown) proportions.
Rod Intrusion
Under daylight conditions, rods are typically thought to be saturated (54, 55), though the range of light levels in which both rods and cones are known to be active is substantial (56, 57). Using a five-primary stimulator [e.g., (25, 26)], it is possible to generate melanopsin-directed stimuli which not only silence the cones but also silence rods. Typically, when rods are silenced, the contrast available to melanopsin is typically only around 1/3 relative to a stimulus in which rods are ignored, though this will depend on the choice of background.
Scatter
The human eye is an imperfect optical system. In cases where the stimulus is a spatially extended light source and there is light outside the primary stimulation area (both centrally, if the macular region is blocked, and in the far periphery), there will be undesired stimulation of potentially unadapted photoreceptors (such as the rods). This can be addressed by adding a light outside the primary stimulation area that light-adapts the photoreceptors outside of the primary stimulation area.
Device Uncertainty
The light source used may not be stable over time and change spectral output between operations, or throughout the sessions. These drifts in device output need to be either calibrated, or at least characterized.
Exploiting Properties Other Than Spectral Sensitivity
We have noted in the introduction that the photoreceptors contributing to pupillary control differ not only in their spectral sensitivity (as is exploited in the method of silent substitution) but also in their temporal properties, their operating range and their distribution across the retina. These properties might also be exploited to selectively stimulate melanopsin. For example, the retinal location corresponding to the blind spot does not contain rods and cones, but light might stimulate melanopsin in the axons of ipRGCs. Delivering a stimulus only in the blind spot would therefore ensure that only melanopsin would be activated (58–60), but there could be scatter on rod and cone photoreceptors near the blind spot, and accidental displacement of a small circumscribed stimulus field would need to be controlled for. In the temporal domain, melanopsin photoreception is much slower than cone- and rod-mediated photoreception, and thus, the temporal properties of a stimulus can be optimized to bias the measured response toward melanopsin-mediated properties, e.g., the steady-state pupil size under continuous light (61).
Conclusion
The method of silent substitution is a powerful technique to stimulate a specific photoreceptor class or specific photoreceptor classes in the living human retina while leaving other classes un-stimulated. The method has been used successfully to examine the photoreceptor contributions to the human pupillary light responses. The method is not failsafe as several factors need to be considered (retinal inhomogeneities, individual differences, rod intrusion, scatter, and device uncertainty), but these can be addressed experimentally or in simulation. We hope that the method of silent substitution will gain traction to tease apart the contributions of different photoreceptors to human vision and to elucidate their role in the non-invasive assessment of the human visual system using pupillometry.
Author Contributions
All authors listed have made a substantial, direct and intellectual contribution to the work, and approved it for publication.
Funding
MS is supported by a Sir Henry Wellcome Trust Fellowship (Wellcome Trust 204686/Z/16/Z).
Conflict of Interest Statement
The authors declare that the research was conducted in the absence of any commercial or financial relationships that could be construed as a potential conflict of interest.
Acknowledgments
We thank Prof. Hannah Smithson for comments on the manuscript.
Supplementary Material
The Supplementary Material for this article can be found online at: https://www.frontiersin.org/articles/10.3389/fneur.2018.00941/full#supplementary-material
References
1. McDougal DH, Gamlin PD. Autonomic control of the eye. Compr Physiol. (2015) 5:439–73. doi: 10.1002/cphy.c140014
2. Provencio I, Rodriguez IR, Jiang G, Hayes WP, Moreira EF, Rollag MD. A novel human opsin in the inner retina. J Neurosci. (2000) 20:600–5. doi: 10.1523/JNEUROSCI.20-02-00600.2000
3. Hattar S, Liao HW, Takao M, Berson DM, Yau KW. Melanopsin-containing retinal ganglion cells: architecture, projections, and intrinsic photosensitivity. Science (2002) 295:1065–70. doi: 10.1126/science.1069609
4. Berson DM, Dunn FA, Takao M. Phototransduction by retinal ganglion cells that set the circadian clock. Science (2002) 295:1070–3. doi: 10.1126/science.1067262
5. Dacey DM, Liao HW, Peterson BB, Robinson FR, Smith VC, Pokorny J, et al. Melanopsin-expressing ganglion cells in primate retina signal colour and irradiance and project to the LGN. Nature (2005) 433:749–54. doi: 10.1038/nature03387
6. Lucas RJ, Hattar S, Takao M, Berson DM, Foster RG, Yau KW. Diminished pupillary light reflex at high irradiances in melanopsin-knockout mice. Science (2003) 299:245–7. doi: 10.1126/science.1077293
7. Norren DV, Vos JJ. Spectral transmission of the human ocular media. Vision Res. (1974) 14:1237–44. doi: 10.1016/0042-6989(74)90222-3
8. Gamlin PD, McDougal DH, Pokorny J, Smith VC, Yau KW, Dacey DM. Human and macaque pupil responses driven by melanopsin-containing retinal ganglion cells. Vision Res. (2007) 47:946–54. doi: 10.1016/j.visres.2006.12.015
9. McDougal DH, Gamlin PD. The influence of intrinsically-photosensitive retinal ganglion cells on the spectral sensitivity and response dynamics of the human pupillary light reflex. Vision Res. (2010) 50:72–87. doi: 10.1016/j.visres.2009.10.012
10. Adhikari P, Zele AJ, Feigl B. The post-illumination pupil response (PIPR). Invest Ophthalmol Vis Sci. (2015) 56:3838–49. doi: 10.1167/iovs.14-16233
11. Kankipati L, Girkin CA, Gamlin PD. Post-illumination pupil response in subjects without ocular disease. Invest Ophthalmol Vis Sci. (2010) 51:2764–9. doi: 10.1167/iovs.09-4717
13. Estévez O, Spekreijse H. The “silent substitution” method in visual research. Vision Res. (1982) 22:681–91. doi: 10.1016/0042-6989(82)90104-3
14. Estévez O, Spekrijse H. A spectral compensation method for determining the flicker characteristics of the human colour mechanisms. Vision Res. (1974) 14:823–30. doi: 10.1016/0042-6989(74)90147-3
15. Brainard DH, Stockman A. Colorimetry. In: Bass M editor. OSA Handbook of Optics, 3rd Edn. New York, NY: McGraw-Hill (2010). pp. 10.11–10.56.
16. Spitschan M, Jain S, Brainard DH, Aguirre GK. Opponent melanopsin and S-cone signals in the human pupillary light response. Proc Natl Acad Sci USA. (2014) 111:15568–72. doi: 10.1073/pnas.1400942111
17. Woelders T, Leenheers T, Gordijn MCM, Hut RA, Beersma DGM, Wams EJ. Melanopsin- and L-cone-induced pupil constriction is inhibited by S- and M-cones in humans. Proc Natl Acad Sci USA. (2018) 115:792–7. doi: 10.1073/pnas.1716281115
18. Shapiro AG, Pokorny J, Smith VC. Cone-rod receptor spaces with illustrations that use CRT phosphor and light-emitting-diode spectra. J Opt Soc Am A Opt Image Sci Vis. (1996) 13:2319–28. doi: 10.1364/JOSAA.13.002319
19. Pokorny J, Smithson H, Quinlan J. Photostimulator allowing independent control of rods and the three cone types. Vis Neurosci. (2004) 21:263–7. doi: 10.1017/S0952523804213207
20. Tsujimura S, Ukai K, Ohama D, Nuruki A, Yunokuchi K. Contribution of human melanopsin retinal ganglion cells to steady-state pupil responses. Proc Biol Sci. (2010) 277:2485–92. doi: 10.1098/rspb.2010.0330
21. Viénot F, Bailacq S, Rohellec JL. The effect of controlled photopigment excitations on pupil aperture. Ophthalmic Physiol Opt. (2010) 30:484–91. doi: 10.1111/j.1475-1313.2010.00754.x
22. Tsujimura S, Tokuda Y. Delayed response of human melanopsin retinal ganglion cells on the pupillary light reflex. Ophthalmic Physiol Opt. (2011) 31:469–79. doi: 10.1111/j.1475-1313.2011.00846.x
23. Barrionuevo PA, Nicandro N, McAnany JJ, Zele AJ, Gamlin P, Cao D. Assessing rod, cone, and melanopsin contributions to human pupil flicker responses. Invest Ophthalmol Vis Sci. (2014) 55:719–27. doi: 10.1167/iovs.13-13252
24. Barrionuevo PA, Cao D. Luminance and chromatic signals interact differently with melanopsin activation to control the pupil light response. J Vis. (2016) 16:29. doi: 10.1167/16.11.29
25. Cao D, Nicandro N, Barrionuevo PA. A five-primary photostimulator suitable for studying intrinsically photosensitive retinal ganglion cell functions in humans. J Vis. (2015) 15:15.1.27. doi: 10.1167/15.1.27
26. Zele AJ, Feigl B, Adhikari P, Maynard ML, Cao D. Melanopsin photoreception contributes to human visual detection, temporal and colour processing. Sci Rep. (2018) 8:3842. doi: 10.1038/s41598-018-22197-w
27. Murray IJ, Kremers J, McKeefry D, Parry NRA. Paradoxical pupil responses to isolated M-cone increments. J Opt Soc Am A Opt Image Sci Vis. (2018) 35:B66–B71. doi: 10.1364/JOSAA.35.000B66
28. Spitschan M, Bock AS, Ryan J, Frazzetta G, Brainard DH, Aguirre GK. The human visual cortex response to melanopsin-directed stimulation is accompanied by a distinct perceptual experience. Proc Natl Acad Sci USA. (2017) 114:12291–6. doi: 10.1073/pnas.1711522114
29. Ramanath R. Minimizing observer metamerism in display systems. Color Res Appl. (2009) 34:391–8. doi: 10.1002/col.20523
30. Stockman A, Brainard DH. Color vision mechanisms. In: Bass M editor. OSA Handbook of Optics, 3rd Edn. New York, NY: McGraw-Hill (2010). pp. 11.11–11.104.
31. CIE. Fundamental Chromaticity Diagram With Physiological Axes–Part 1. Technical Report 170-1, Central Bureau of the Commission Internationale de l' Éclairage, (Vienna) (2006).
32. Asano Y, Fairchild MD, Blondé L. Individual colorimetric observer model. PLoS ONE (2016) 11:e0145671. doi: 10.1371/journal.pone.0145671
33. Enezi JA, Revell V, Brown T, Wynne J, Schlangen L, Lucas R. A “melanopic” spectral efficiency function predicts the sensitivity of melanopsin photoreceptors to polychromatic lights. J Biol Rhythms (2011) 26:314–23. doi: 10.1177/0748730411409719
34. Lucas RJ, Peirson SN, Berson DM, Brown TM, Cooper HM, Czeisler CA, et al. Measuring and using light in the melanopsin age. Trends Neurosci. (2014) 37:1–9. doi: 10.1016/j.tins.2013.10.004
35. Spitschan M, Aguirre GK, Brainard DH. Selective stimulation of penumbral cones reveals perception in the shadow of retinal blood vessels. PLoS ONE (2015) 10:e0124328. doi: 10.1371/journal.pone.0124328
36. Spitschan M, Datta R, Stern AM, Brainard DH, Aguirre GK. Human visual cortex responses to rapid cone and melanopsin-directed flicker. J Neurosci. (2016) 36:1471–82. doi: 10.1523/JNEUROSCI.1932-15.2016
37. Smith VC, Pokorny J. Chromatic-discrimination axes, CRT phosphor spectra, and individual variation in color vision. J Opt Soc Am A Opt Image Sci Vis. (1995) 12:27–35. doi: 10.1364/JOSAA.12.000027
38. Golz J, MacLeod DI. Colorimetry for CRT displays. J Opt Soc Am A Opt Image Sci Vis. (2003) 20:769–81. doi: 10.1364/JOSAA.20.000769
39. Webster MA, MacLeod DI. Factors underlying individual differences in the color matches of normal observers. J Opt Soc Am A (1988) 5:1722–35. doi: 10.1364/JOSAA.5.001722
40. Neitz J, Jacobs GH. Polymorphism of the long-wavelength cone in normal human colour vision. Nature (1986) 323:623–5. doi: 10.1038/323623a0
41. Neitz J, Jacobs GH. Polymorphism in normal human color vision and its mechanism. Vision Res. (1990) 30:621–36. doi: 10.1016/0042-6989(90)90073-T
42. Merbs SL, Nathans J. Absorption spectra of the hybrid pigments responsible for anomalous color vision. Science (1992) 258:464–6. doi: 10.1126/science.1411542
43. Sanocki E, Lindsey DT, Winderickx J, Teller DY, Deeb SS, Motulsky AG. Serine/alanine amino acid polymorphism of the L and M cone pigments: effects on Rayleigh matches among deuteranopes, protanopes and color normal observers. Vision Res. (1993) 33:2139–52. doi: 10.1016/0042-6989(93)90012-L
44. Sexton TJ, Golczak M, Palczewski K, Van Gelder RN. Melanopsin is highly resistant to light and chemical bleaching in vivo. J Biol Chem. (2012) 287:20888–97. doi: 10.1074/jbc.M111.325969
45. Matsuyama T, Yamashita T, Imamoto Y, Shichida Y. Photochemical properties of mammalian melanopsin. Biochemistry (2012) 51:5454–62. doi: 10.1021/bi3004999
46. Mure LS, Cornut PL, Rieux C, Drouyer E, Denis P, Gronfier C, et al. Melanopsin bistability: a fly's eye technology in the human retina. PLoS ONE (2009) 4:e5991. doi: 10.1371/journal.pone.0005991
47. Mure LS, Rieux C, Hattar S, Cooper HM. Melanopsin-dependent nonvisual responses: evidence for photopigment bistability in vivo. J Biol Rhythms (2007) 22:411–24. doi: 10.1177/0748730407306043
48. Melyan Z, Tarttelin EE, Bellingham J, Lucas RJ, Hankins MW. Addition of human melanopsin renders mammalian cells photoresponsive. Nature (2005) 433:741–5. doi: 10.1038/nature03344
49. Panda S, Nayak SK, Campo B, Walker JR, Hogenesch JB, Jegla T. Illumination of the melanopsin signaling pathway. Science (2005) 307:600–4. doi: 10.1126/science.1105121
50. Emanuel AJ, Do MT. Melanopsin tristability for sustained and broadband phototransduction. Neuron (2015) 85:1043–55. doi: 10.1016/j.neuron.2015.02.011
51. Mawad K, Van Gelder RN. Absence of long-wavelength photic potentiation of murine intrinsically photosensitive retinal ganglion cell firing in vitro. J Biol Rhythms (2008) 23:387–91. doi: 10.1177/0748730408323063
52. Rollag MD. Does melanopsin bistability have physiological consequences? J Biol Rhythms (2008) 23:396–9. doi: 10.1177/0748730408323067
53. Brown TM, Allen AE, al-Enezi J, Wynne J, Schlangen L, Hommes V, et al. The melanopic sensitivity function accounts for melanopsin-driven responses in mice under diverse lighting conditions. PLoS ONE (2013) 8:e53583. doi: 10.1371/journal.pone.0053583
54. Aguilar M, Stiles WS. Saturation of the rod mechanism of the retina at high levels of stimulation. Opt Acta (1954) 1:59–65. doi: 10.1080/713818657
55. Adelson EH. Saturation and adaptation in the rod system. Vision Res. (1982) 22:1299–312. doi: 10.1016/0042-6989(82)90143-2
56. Zele AJ, Cao D. Vision under mesopic and scotopic illumination. Front Psychol. (2014) 5:1594. doi: 10.3389/fpsyg.2014.01594
57. Shapiro AG. Cone-specific mediation of rod sensitivity in trichromatic observers. Invest Ophthalmol Vis Sci. (2002) 43:898–905.
58. Alpern M, Campbell FW. The spectral sensitivity of the consensual light reflex. J Physiol. (1962) 164:478–507. doi: 10.1113/jphysiol.1962.sp007033
59. Saito M, Miyamoto K, Uchiyama Y, Murakami I. Invisible light inside the natural blind spot alters brightness at a remote location. Sci Rep. (2018) 8:7540. doi: 10.1038/s41598-018-25920-9
60. Miyamoto K, Murakami I. Pupillary light reflex to light inside the natural blind spot. Sci Rep. (2015) 5:11862. doi: 10.1038/srep11862
Keywords: pupil, melanopsin, silent substitution, color vision, pupillometry, ipRGC (intrinsically photosensitive retinal ganglion cell), metamers
Citation: Spitschan M and Woelders T (2018) The Method of Silent Substitution for Examining Melanopsin Contributions to Pupil Control. Front. Neurol. 9:941. doi: 10.3389/fneur.2018.00941
Received: 04 September 2018; Accepted: 17 October 2018;
Published: 27 November 2018.
Edited by:
Andrew J. Zele, Queensland University of Technology, AustraliaReviewed by:
Sei-ichi Tsujimura, Kagoshima University, JapanDingcai Cao, University of Illinois at Chicago, United States
Copyright © 2018 Spitschan and Woelders. This is an open-access article distributed under the terms of the Creative Commons Attribution License (CC BY). The use, distribution or reproduction in other forums is permitted, provided the original author(s) and the copyright owner(s) are credited and that the original publication in this journal is cited, in accordance with accepted academic practice. No use, distribution or reproduction is permitted which does not comply with these terms.
*Correspondence: Manuel Spitschan, bWFudWVsLnNwaXRzY2hhbkBwc3kub3guYWMudWs=