- 1Inselspital, Bern University Hospital, University of Bern, Bern, Switzerland
- 2Department of Neurology, Center for Experimental Neurology, Inselspital, Bern University Hospital, University of Bern, Bern, Switzerland
- 3Department of Biomedical Research, Inselspital, Bern University Hospital, University of Bern, Bern, Switzerland
- 4Ohio Sleep Medicine Institute, Dublin, OH, United States
The hypocretin (Hcrt) system has been implicated in a wide range of physiological functions from sleep-wake regulation to cardiovascular, behavioral, metabolic, and thermoregulagtory control. These wide-ranging physiological effects have challenged the identification of a parsimonious function for Hcrt. A compelling hypothesis suggests that Hcrt plays a role in the integration of sleep-wake neurophysiology with energy metabolism. For example, Hcrt neurons promote waking and feeding, but are also sensors of energy balance. Loss of Hcrt function leads to an increase in REM sleep propensity, but a potential role for Hcrt linking energy balance with REM sleep expression has not been addressed. Here we examine a potential role for Hcrt and the lateral hypothalamus (LH) in state-dependent resource allocation as a means of optimizing resource utilization and, as a result, energy conservation. We review the energy allocation hypothesis of sleep and how state-dependent metabolic partitioning may contribute toward energy conservation, but with additional examination of how the loss of thermoregulatory function during REM sleep may impact resource optimization. Optimization of energy expenditures at the whole organism level necessitates a top-down network responsible for coordinating metabolic operations in a state-dependent manner across organ systems. In this context, we then specifically examine the potential role of the LH in regulating this output control, including the contribution from both Hcrt and melanin concentrating hormone (MCH) neurons among a diverse LH cell population. We propose that this hypothalamic integration system is responsible for global shifts in state-dependent resource allocations, ultimately promoting resource optimization and an energy conservation function of sleep-wake cycling.
Introduction
In the 20 years since the discovery of the hypocretin/orexin (Hcrt) system, a growing diversity of physiological and neurobehavioral responses under Hcrt control has challenged identification of a unifying function. A compelling hypothesis suggests that Hcrt plays a role in the integration of sleep-wake neurophysiology with energy metabolism (1–3). Hcrt neurons, for example, are sensors of energy balance through their receptivity to leptin, ghrelin, and glucose (1, 4–6). It has been proposed that the Hcrt system may coordinate the waking response during periods of negative energy balance to promote foraging behavior. However, many questions still remain. Although the loss of Hcrt leads to an increase in REM sleep propensity associated with narcolepsy (7–9), a parsimonious role for Hcrt in both REM sleep regulation and energy metabolism has remained unknown.
Hypocretins 1 and 2 (Hcrt1 and Hcrt2), also called orexins A and B, are excitatory hypothalamic neuropeptides that are derived from a single precursor molecule by proteolytic processing and are largely co-localized in the same neurons (10). They were independently described by two different groups in 1998 (11, 12). While early studies emphasized the role of the Hcrt system in feeding and energy balance, subsequent research focused on sleep-wake regulation based on the discovery that Hcrt dysfunction underlies the sleep disorder narcolepsy.
Hcrt neurons are found exclusively in the posterior lateral hypothalamus (LH) (11–13), numbering between 4,000–5,000 in the rat (13–15) and 50,000–80,000 in humans (16). The anatomy and the projections of the Hcrt system (see below) strongly suggest involvement in diverse physiological functions including sleep-wake control, feeding, thermoregulation, blood pressure, motivation/reward, and neuroendocrine regulation. Long before its discovery, the region of the LH containing Hcrt cells had been implicated in arousal state control (17, 18). It is now well-understood that Hcrt signaling within the LH promotes wakefulness. Indeed, Hcrt neurons are wake-active as measured by Fos expression (19), electrophysiology (20–22), or brain/CSF peptide content (23–25). Hcrt increases arousal when infused into the brain (26–32), and optogenetic stimulation or inhibition of Hcrt signaling increases or decreases wakefulness, respectively (33–36).
Here, on the 20th anniversary of its discovery, we examine a potential role for Hcrt in optimizing efficiencies in energy utilization and, thus, energy conservation. Our recent energy allocation hypothesis of sleep proposes that state-dependent metabolic partitioning at the whole organism level provides greater daily energy conservation through resource optimization than the measured metabolic rate reduction observed during sleep (37, 38). A basic tenet of this hypothesis from an evolutionary perspective is that “optimization of rates of return on energy investments is the singular design principle for the organization of sleep and wakefulness” (p. 126) (37). We propose that optimization of energy expenditures at the whole organism level necessitates a top-down network responsible for coordinating biological operations in a state-dependent manner across organ systems. We will also examine the hypothalamic control of REM sleep vs. wakefulness in the setting of their competing thermoregulatory demands and how loss of thermoregulatory control during REM sleep may enhance resource utilization when REM sleep expression can be modulated as a function of ambient temperature. Finally, we propose that the Hcrt system is part of a dynamic hypothalamic integration system responsible for optimizing global shifts in state-dependent resource allocations central to an energy conservation function of sleep-wake cycling.
Sleep-Wake Cycling, Resource Optimization, and Energy Conservation
Sleep has long been considered an energy conservation strategy, potentially explaining its universal presence across the animal kingdom (39–41). However, the mechanism by which sleep conserves energy historically has been studied only in terms of the degree to which metabolic rate is reduced during sleep compared to quiet wakefulness, similar in concept to torpor or hibernation. For example, mammals typically decrease metabolic rate by 15–30% during sleep (42–44). For an organism with an 8 h sleep quota per day, this 8 h metabolic rate reduction equates to a calculated energy savings of 5–9% per 24 h. Although significant, this only modest daily energy savings has been considered insufficient to explain the universal presence of sleep, particularly when considering the costs of a sleep strategy, including increased predation risks and lost mating, parental care, and foraging opportunities secondary to the decreased behavioral responsiveness associated with sleep (43, 45, 46).
Moreover, metabolic rate reduction during sleep, analogous to torpor as the principal mechanism of energy conservation, assumes that all biological processes are equally reduced during sleep compared to wake. However, sleep is a highly active metabolic state during which many specific biological operations are upregulated, contradicting the notion that sleep, like hibernation, involves the global downregulation of metabolic processes. These functions specifically upregulated during sleep compared to wake include protein biosynthesis, intracellular transport and membrane repair (47, 48), immune function (49), elimination of biological waste or restorative processes (50–52), and neural network reorganization for memory processing (53–57), to name a few.
Our recent energy allocation hypothesis views sleep-wake cycling as a behavioral strategy promoting energy conservation through dynamic state-dependent metabolic partitioning and resource optimization (37, 38). A basic premise of this theory is that the partitioning of metabolic functions by behavioral state occurs at the whole organism level and is not restricted to a single organ or structure. Thus, sleep-wake cycling increases total energy savings through resource optimization beyond what a single organ system could otherwise achieve. Indeed, a great diversity of gene expression is specifically coupled with either sleep or wakefulness in both central and peripheral tissues (47, 48, 58), consistent with state-dependent metabolic partitioning at the whole organism level. As shown in Figure 1A, specific mechanisms are known to synchronize or coordinate brain with periphery according to behavioral state, including state-specific hormonal release (anabolic in sleep, catabolic in wake), synchronization of central and peripheral circadian clocks, and direct autonomic innervation of peripheral tissues (37).
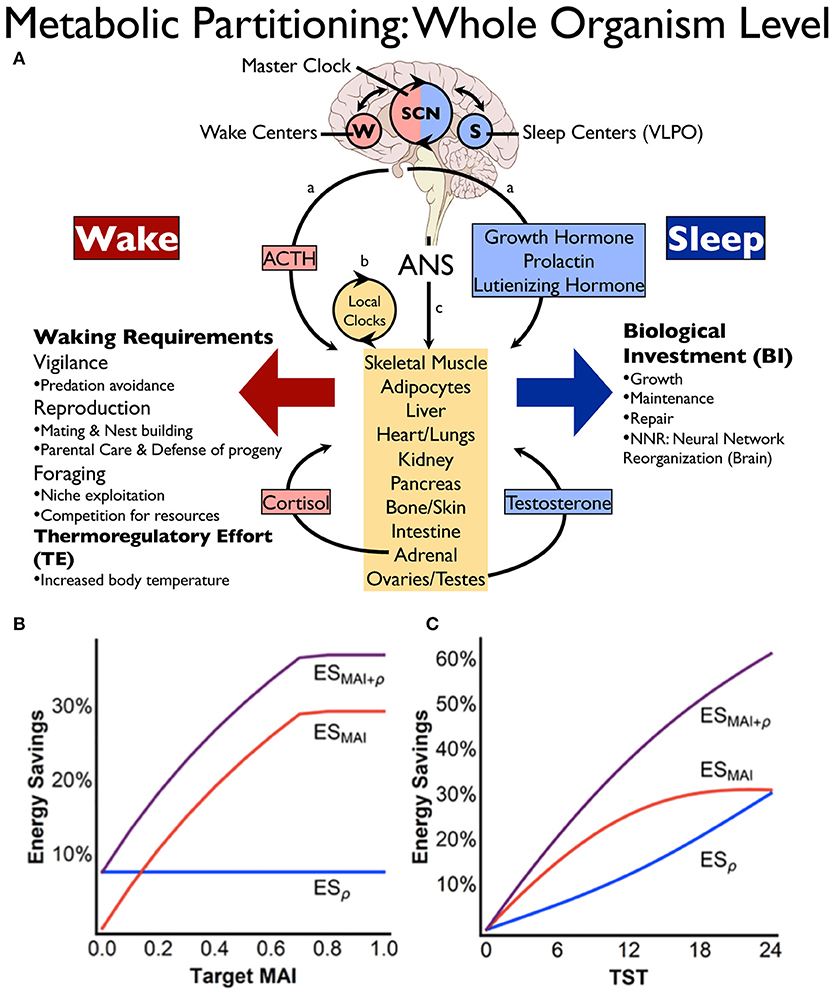
Figure 1. Energy conservation through state-dependent (sleep-wake) metabolic partitioning. (A) Control of resource allocations involves both brain and periphery. Within the brain, sleep or wakefulness is expressed based on the interplay between sleep (S) and wake (W) promoting centers, the timing of which is modulated by the suprachiasmatic nucleus (SCN). Wake predominant (red) or sleep predominant (blue) processes in the periphery are synchronized with behavioral state (brain) through: (a), hormonal control, (b) local autonomous circadian clocks in peripheral tissues, and (c) direct descending autonomic nervous system (ANS) innervation from brain to periphery [reprinted with permission from Schmidt (37)]. (B,C) Energy savings calculations based on mathematical modeling showing the potential impact of metabolic partitioning (B) or sleep quota (C) on energy savings derived from sleep-wake cycling. In (B), metabolic partitioning, represented by the metabolic allocation index (MAI), can be varied in the model from 0 to 1 (0 indicating all biological processes are equally performed in both states, whereas MAI = 1 signifies that all functions performed during wake are different from sleep). Target MAI is varied in the figure while holding metabolic rate reduction during sleep (ρ) and total sleep time (TST) constant. Note in the figure that an 8 h sleep quota and a metabolic rate reduction during sleep of 30% (ρ = 0.3) constrain maximum MAI to ~0.7. In (C), TST is varied while holding target MAI = 0.4 and ρ = 0.3. Blue line is energy savings from metabolic rate reduction (ESρ), red line is saving from MAI (ESMAI), and purple line is overall energy savings (ESMAI+ρ). Reprinted from Schmidt et al. (38).
The energy allocation hypothesis of sleep proposes that state-dependent metabolic partitioning provides greater daily energy conservation than the measured metabolic rate reduction observed during sleep (37, 38). Based on relative rates of energy deployment for biological processes upregulated during either wake or sleep, the potential energy savings as derived from state-dependent metabolic partitioning has been calculated using mathematical modeling (38). This modeling suggests that whole organism energy savings is amplified to over 35% per 24 h for an 8 h sleep quota when unique biological processes are partitioned by behavioral state (see Figures 1B,C).
Several important principles emerge from this mathematical modeling, including why sleep may be a better behavioral strategy than quiet wakefulness with respect to resource optimization and energy savings. This modeling suggests that metabolic partitioning by state is constrained if energy deployment toward waking-related processes is maintained during the rest phase, whereas energy savings are amplified if waking-related allocations during the rest phase are eliminated (38). This mechanism of energy conservation requires a system capable of not only monitoring the energy status of the organism, but also integrating many key physiological variables including core and ambient temperature to provide a coordinated output to a wide range of central and peripheral tissues.
Hypocretin and Its Role in Diverse Functions
We will now review the role of Hcrt on modulating a wide range of physiological responses. Understanding these diverse physiological effects will allow us to explore a potential role for Hcrt in a broader perspective as a network control system promoting resource optimization.
Hcrt in Arousal and Sleep-Wake Transitions
The Hcrt system was first linked to sleep function through the discovery that a significant loss of Hcrt neurons leads to narcolepsy, a condition characterized by excessive daytime sleepiness, cataplexy, sleep paralysis and hypnagogic hallucinations (16, 59). These latter symptoms of narcolepsy, including loss of muscle tone and hallucinations, are thought to be components of REM sleep that inappropriately intrude into wakefulness, consistent with a dysregulation of boundary state control in narcolepsy. In canine narcolepsy, a mutation of the Hcrt 2 receptor leads to these manifestations (60). In rats, Hcrt 1 administration (i.c.v.) increases wakefulness in a dose-dependent manner (30), whereas pharmacological antagonism of the Hcrt receptors results in increased NREM and REM sleep and reduces wakefulness in both animals and humans (61). In squirrel monkeys who show a similar diurnal wake pattern to that of humans, Hcrt levels in the cerebro-spinal fluid peak toward the end of the light (waking) period (25).
Optogenetic activation of Hcrt neurons increases the probability of sleep to wake transitions from either NREM or REM sleep (34) throughout both the light and dark phases but with decreased effectiveness following sleep-deprivation and marked increases in sleep pressure (35). On the other hand, optogenetic inhibition of Hcrt neurons during waking in the rest (light) phase increases NREM sleep, while its inhibition during the active (dark) phase has produced mixed effects (33). In support of its role as a waking system, Hcrt has been shown to interact with several neuronal groups that are known to be wake-promoting, such as histaminergic neurons of the tuberomammilary nucleus and noradrenergic neurons of the locus coeruleus (26, 27, 62). In summary, stimulating Hcrt neurons increases the likelihood of transitions into wakefulness, whereas its inhibition increases the likelihood of transitions into sleep.
The narcoleptic phenotype not only leads to excessive daytime sleepiness, but also to fragmented sleep with many brief arousals (63–65). Such transitions between states have also been demonstrated in Hcrt-knockout (KO) mice, a phenotype showing poor maintenance of wakefulness and fragmented NREM sleep even after 8 h of sleep deprivation (66). These transitions result from behavioral state instability, as a loss of Hcrt may to lead to a breakdown of neural control processes that lower the thresholds to transition between behavioral states, producing the fragmented wakefulness and sleep typical of the narcolepsy condition (66). Saper et al. (67) hypothesize that Hcrt acts as a stabilizer on a “flip-flop switch,” avoiding transitional states by not only “flipping” the organism between wake and sleep but also providing stability between two opposing behavioral states (67). In support of this perspective, narcoleptics easily fall asleep during the day but wake up more often at night while leaving the net time asleep unchanged (68, 69).
Hcrt and Thermoregulation
Thermoregulation is tightly integrated with the sleep-wake cycle in mammals and is an important function of Hcrt. In healthy subjects during sleep, decreased heat production from reduced muscle activity and lower basal metabolism contribute to a body temperature that is regulated at a lower level (70, 71). Additionally, peripheral vasodilatation helps decrease the core body temperature during sleep initiation (72). Sleep-promoting neurons in the preoptic area also activate heat loss mechanisms (71, 73, 74), and a modest fall in body temperature is common to mammalian sleep.
Compared to healthy controls, narcoleptics show lower core body temperatures while awake (75) and higher than normal body temperature during sleep (76, 77). Hcrt deficient mice also show similar body temperature abnormalities (78). Hcrt-KO mice show significantly smaller deviations from peak to trough core body temperatures than wild type mice, demonstrating more frequent small fluctuations and fewer large alterations of core body temperature and energy expenditure (78, 79). It appears that narcolepsy is a condition that does not allow the body temperature and basal metabolic rate to decrease properly during sleep or to rise appropriately during active periods. However, it remains to be determined if these frequent, yet small changes in body temperature amplitude directly result from metabolic alterations secondary to Hcrt cell loss or simply indirectly reflect the instability of sleep/wake states in narcoleptics.
An altered pattern of skin-temperature regulation is also reported in patients suffering from narcolepsy (80). In healthy controls, distal skin temperature shows a rhythm that is the inverse to the core body temperature rhythm (81). During daytime in humans, core body temperature is high and distal skin temperature is relatively low, a combination that correlates with optimal vigilance (82–87). In contrast, core body temperature decreases at night, while distal skin temperature is relatively high, signifying optimal sleep (82–87). Indeed, sleep onset is characterized by a rise in temperature of the distal skin compared to the temperature of the proximal skin (known as the distal-to-proximal gradient) (72).
Narcoleptic subjects during the waking period show higher distal skin temperatures with lower proximal skin temperatures, which may either reflect or contribute to the sleepiness of narcolepsy (80) as this combination is associated with sleep onset in healthy subjects (72). It has also been demonstrated that narcoleptic patients are better able to maintain vigilance when their core temperature is artificially raised or when distal skin temperature is experimentally lowered, thereby simulating the conditions known to be correlated with optimal vigilance (75). Taken together, these data demonstrate an inability to appropriately modulate thermoregulatory control according to behavioral state in narcolepsy. The extent to which this breakdown in thermoregulatory responses may also lead to inefficiencies in resource allocations contributing to the narcolepsy phenotype has yet to be explored.
The Hcrt system also plays an important role in increasing core body temperature by activating brown adipose tissue (BAT) (88, 89), suggesting an important role of Hcrt in responding to a cooling of the ambient temperature by activating both waking and heat production (90). BAT plays an important role in regulating body temperature in mammals. It expresses the protein UCP1 which uncouples ATP production from respiration to liberate energy in the form of heat (90). This UCP1-driven heat dissipation is termed “non-shivering thermogenesis” and allows mammals to generate heat without needing to resort to the higher energy demands of shivering to keep warm in cold conditions (91). Non-shivering thermogenesis occurring in BAT is largely under sympathetic control and can be activated by catecholamines (92, 93). Hcrt modulates sympathetic activity and, through its projections to the raphe pallidus, can increase BAT thermogenesis during arousal (88, 90). By increasing body temperature during periods of wakefulness, the Hcrt system may act to optimize metabolism during periods requiring enhanced performance such as food seeking or reproduction (90). In contrast to waking, evidence suggests that BAT thermogenesis may be state-specifically inhibited or reduced during REM-sleep (94, 95). Although narcoleptic patients appear capable of activating BAT during cold ambient temperature exposure (96), more work is needed to determine if narcoleptics show deficiencies with respect to dynamic BAT modulation and coordination with peripheral vasomotor responses.
Role of Hcrt in Food Seeking and Reward
Hypocretin is also called orexin because of its role in feeding, as orexin means “appetite” in Greek. In mammals, arousal is reduced after feeding and increased during fasting (1, 97–99), and Hcrt neurons are inhibited following food-intake and are activated during periods of fasting (100). In mice with a genetic ablation of Hcrt neurons, normal responses to fasting, such as increased wakefulness and foraging behaviors, are not observed (1, 4, 101). Finally, Hcrt may play a role in mediating shifts in circadian rhythms in response to changes in the timing of food availability or nutritional status. For example, genetic elimination of Hcrt results in reduced food anticipatory activity and the expected temporal expression of numerous clock genes (101, 102).
Food seeking behavior caries an essential survival function and is a potent reward stimulus. Multiple studies show that Hcrt plays an active role in reward processing for both food and drug-seeking behaviors. Human narcoleptics rarely show stimulant abuse or drug seeking behavior even though they are treated for years with stimulants (103). The first observation of a possible role for hypocretins in addiction showed that these neurons play a role in opiate withdrawal (104). Harris et al. (105) demonstrated a potential implication of Hcrt neurons in reward related phenomena showing the activation of Hcrt neurons after acquiring reward and that C-Fos activation in Hcrt neurons is strongly associated with the expression of conditioned place preference (CPP) for drug or natural food rewards. Additionally, Hcrt-KO mice completely lack CPP for morphine (106). Finally, although its etiology is likely multifactorial in origin, narcoleptic patients have a higher risk of depression (107–109). Taken together, these findings indicate that Hcrt neurons play a role in motivation-driven behavior and are part of a circuitry involved in reward processing (110, 111).
Hcrt and Cardiovascular Control
Finally, Hcrt also mediates autonomic and cardiovascular effects associated with sleep and waking. For example, there are several well-known sleep-associated cardiovascular changes. One such change is the decrease in blood pressure that occurs during sleep in a variety of species, a phenomenon generally referred to as “dipping” (112–115). Dipping is thought to be influenced by a change in the activity of the autonomic nervous system, since non-dippers have an increase in sympathetic tone at sleep onset as opposed to the normal reduction in sympathetic tone and concomitant increase in parasympathetic tone that typically occurs during sleep (116, 117). Hcrt plays an important role in regulating blood pressure across behavioral states. For example, the lack of Hcrt in transgenic animals is associated with lower systemic blood pressure and a blunting of the decrease in blood pressure during sleep compared to wake (118, 119). Finally, Hcrt increases sympathetic outflow and has effects on the hypothalamic-pituitary-adrenal (HPA) axis, resulting in an increase in catecholamine release (118, 120, 121). Indeed, optogenetic approaches demonstrate that Hcrt neuronal activity regulates corticosterone release through the HPA axis and resulting in behavioral correlates of stress responses (121). These findings highlight the ability of Hcrt to potentially affect wide ranging peripheral tissues through both autonomic and neuroendocrine effects.
In summary, these data demonstrate that Hcrt plays a role in feeding and sleep-wake modulation, as well as numerous other physiological processes such as thermoregulation, motivation and reward, autonomic and neuroendocrine modulation. We will next review how the Hcrt system, together with other LH cell populations, may integrate these physiological responses with respect to the energy status of the organism. Finally, we will propose a unifying role for Hcrt that includes NREM and REM sleep regulation, particularly NREM-REM cycling, with respect to resource optimization and energy conservation.
The LH in Energy Balance, Behavioral State Transitions and Resource Optimization
In this section, we will describe how, during fasting when energy resources are low, Hcrt promotes wakefulness and food seeking behavior. In contrast, in the presence of a positive energy balance after feeding, other cell types within the LH, such as melanin concentrating hormone (MCH) neurons, promote sleep. We will also examine how the LH, through reciprocal interactions between heterogeneous neuronal populations, may coordinate global shifts in resource utilization through sleep-wake state transitions. Importantly, these reciprocal interactions within hypothalamic circuits suggest that Hcrt not only mobilizes or promotes a waking response, but actively inhibits sleep-related processes and thus contributes to a state-dependent partitioning of biological operations, a mechanism that we propose is central to an energy conservation function of sleep-wake cycling.
Anterior vs. Posterior Hypothalamus and Behavioral State Modulation
As was originally hypothesized in the 1930's, behavioral state control is, in part, anatomically segregated within the hypothalamus (18, 122). Historically, the anterior hypothalamus has been viewed as a “sleep center” since lesions in this area are known to cause long-lasting insomnia, whereas the posterior hypothalamus has been viewed as a “waking center” since its lesioning causes excessive sleepiness [see Saper et al. (67)]. Although this anterior-posterior classification of “sleep” vs. “waking” centers may be an oversimplification given the role other brain circuits (123–125), many of these historical insights still hold true today.
Anteriorly, several structures are particularly noteworthy. The ventrolateral preoptic nucleus (VLPO) within the preoptic area plays an important role in initiating sleep and driving NREM sleep (67, 126, 127). An extensive literature demonstrates that many sleep active neurons within the preoptic area, regions encompassing both the VLPO and the median preoptic nucleus (MnPO), are also warm sensitive and thought to promote sleep when the animal is in a warm, thermoneutral environment (128–132). Although unclear if arising from the same neuronal population, the MnPO also contains temperature sensitive neurons that receive either warm or cold ambient temperature input from the periphery (skin) via relay connections through the brainstem parabrachial nucleus (128, 133) (see Figure 2). Thermoregulatory information from the MnPO and Medial Preoptic nucleus (MPO) are then relayed to the raphe pallidus (RPa), either directly or via the dorsomedial hypothalamus (DMH), for output effector modulation of vasomotor responses and BAT (133–138) (Figure 2). Numerous structures involved in REM sleep regulation, such as the LH and periaqueductal gray (PAG), also control thermoregulatory responses (Figure 2), demonstrating a close anatomical relationship between sleep and thermoregulatory systems.
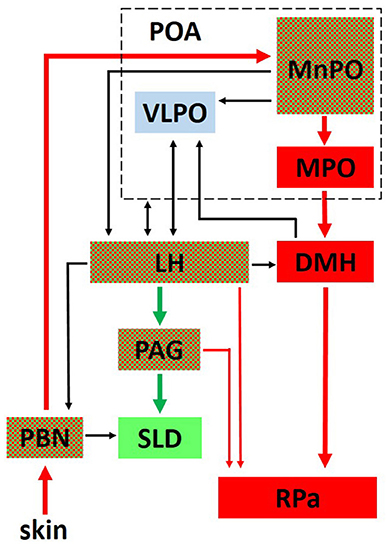
Figure 2. The interplay between critical networks controlling REM sleep (green), thermoregulation (red) or both (checkered). Ambient temperature information from thermosensors in the skin is carried through the dorsal columns of the spinal cord to the parabrachial nucleus (PBN) where it is then relayed to the median preoptic nucleus (MnPO) in the preoptic area (POA). Both warm and cold sensitive neurons in the MnPO relay information to the medial preoptic nucleus (MPO) and to the dorsomedial hypothalamic nucleus (DMH). From there, a major thermoregulatory pathway involves the connection to the raphe pallidus (RPa) which is a major output pathway for the control of brown adipose tissue (BAT). Not shown in the figure is a direct pathway from the MnPO and MPO to the RPa for the output control of vasomotor thermoregulatory responses (137). The LH receives either direct or indirect input from the POA related to sleep and thermoregulatory centers and plays an important role in REM sleep control through its descending connections to the ventrolateral periaqueductal gray (PAG) which projects to the sublaterodorsal tegmental nucleus (SLD) in the pons. Hcrt neurons in the LH send projections to the RPa and play an important role in modulating thermoregulatory control. Reprinted with permission from Cerri et al. (134).
Initially considered as only a waking center, the posterior LH has been shown to regulate behavioral state transitions from NREM sleep to either REM sleep or wakefulness (1, 139). Although we will focus on the Hcrt and MCH systems, it is important to note that the LH also contains many other cell types involved in sleep, wakefulness and metabolism (3). These include several subtypes of non-MCH GABAergic neurons, including those that trigger wakefulness through inhibition of the thalamic reticular nucleus (140), and others that may drive REM sleep (141, 142) or feeding behavior (143, 144). Finally, the LH receives widespread inputs, and appears to integrate this diverse information for output behavioral state modulation. For example, a prevailing view suggests that information flow from the VLPO and MnPO related to sleep pressure and temperature may either directly or indirectly modulate the LH (134, 136) (see Figure 2).
Hcrt and MCH in State Control and Energy Balance
In contrast to Hcrt and its predominant role in wakefulness, it is now well-established that the MCH neuronal system modulates REM sleep expression (145–147), at least in part through a GABAergic inhibitory system (148–150). C-Fos studies have shown that MCH neurons are most active after REM sleep hypersomnia (151, 152), consistent with the observation that MCH neurons fire maximally during REM sleep (153). Measurement of MCH levels within the amygdala across the sleep-wake cycle in humans also show that the onset of sleep is associated with an increase in MCH, consistent with a hypothesis that MCH may also play a role in generating NREM sleep (154, 155). Concordantly, administration of an MCH antagonist decreases the amount of REM and NREM sleep and can lengthen the NREM-REM cycle (156). Optogenetic activation of MCH neurons during NREM sleep increases the number of NREM to REM sleep transitions, whereas state-specific activation of MCH neurons during REM sleep prolongs REM sleep bout durations (148).
The Hcrt and MCH neuronal systems are intermingled within the LH and generally fire reciprocally (153, 157). For example, in a head-restrained rat preparation, MCH neuronal activity is maximal during REM sleep when Hcrt neurons are virtually silent. During waking, in contrast, Hcrt neurons are maximally active, while MCH neuronal activity is absent. It should be noted, however, that calcium imaging of MCH neurons shows an increase in activity during some waking behaviors specifically related to novel object presentation (158). Although the relationship between MCH and Hcrt appears complex, evidence suggests either a direct or indirect reciprocal inhibition (159). Optogenetic stimulation of Hcrt neurons, for example, promotes inhibition of some MCH neurons by increasing presumably GABAergic interneuronal input onto MCH cells, whereas other MCH neurons are directly excited by Hcrt (159). The colocalization of endogenous opioids in Hcrt neurons, in contrast, appear to hyperpolarize MCH cells (160–162). Whatever the mechanism, the interconnection between Hcrt and MCH generally favors a reciprocal firing pattern of these two neuronal systems.
Hcrt and MCH neurons also generally respond with opposite activity patterns to the nutritional state of the organism, and thus energy balance, through their receptivity to numerous biomarkers related to energy status. To illustrate, Hcrt neurons are inhibited by biomarkers which are released in response to food intake, such as leptin (satiety hormone produced by adipose tissues), glucose, and neuropeptide Y (1, 4, 5, 163–165), whereas ghrelin, which is an appetite-stimulating hormone produced in the gut, increases Hcrt activity (6). The inhibitory response of Hcrt neurons mediated by leptin appears secondary to GABAergic interneurons within the LH colocalizing neurotensin and expressing the leptin (LepRb) receptor (166–169). Finally, Hcrt neurons express the adenosine A1 receptor and are inhibited by extracellular adenosine (170–172), suggesting that adenosine can potentially promote sleep by decreasing Hcrt activity.
MCH neurons are responsive to insulin and glucose, but they demonstrate an opposite response pattern compared to Hcrt neurons (173, 174). For example, approximately 70% of MCH neurons are excited by physiological elevations in glucose (5 mM) and dose-dependently hyperpolarize as glucose levels are lowered, whereas Hcrt neuronal activity shows opposite effects to these same glucose concentrations (174, 175). This coordinated response from glucose involving inhibition of Hcrt with activation of MCH appears to promote sleep, thus activating sleep-dependent processes when body energy resources are high after feeding (1, 174). Finally, MCH neurons also release Nesfatin-1, a satiety hormone, and disruption of Nesfatin-1 signaling decreases REM sleep expression (176).
Taken together, these data demonstrate multiple complimentary and redundant mechanisms by which the LH may tightly monitor energy status of the organism. Moreover, these data are consistent with the hypothesis that the posterior LH integrates energy status and sleep-wake modulation, at least in part through the reciprocal activation of the MCH and Hcrt systems.
MCH and Feeding Behavior
Although MCH promotes sleep and is responsive to energy status, a considerable body of literature indicates that MCH, like Hcrt, also regulates feeding behavior. Interestingly, the MCH system may promote feeding behavior while also decreasing both locomotor activity and BAT thermogenesis, thereby contributing to a positive energy balance and potentially conserving energy for homeostatic purposes (177–187). Diniz and Bittencourt (188) suggest that MCH can drive periodic feeding, even when energy balance is positive, given the potential danger in waiting for energy levels to deplete. Indeed, when energy balance is positive and food is available, MCH may promote feeding, thereby maintaining a positive energy-balance. If food is not available, but energy balance is still favorable, MCH may promote a change of state, favoring sleep with a concomitant decrease in both locomotor activity and thermoregulatory defenses (188). Diminishment of energy reserves eventually leads to decreased glucose levels, causing decreased excitability of MCH cells and, together with increased Ghrelin, an excitation of Hcrt activity (174). Hcrt activity, therefore, may predominate when the animal reaches a negative energy balance, leading to increased wakefulness and thermogenesis, both necessary conditions for active food seeking.
Hcrt and MCH as Network Control Systems
Consistent with their roles in behavioral state modulation and global shifts in peripheral responses, both Hcrt and MCH neuronal populations show wide-spread projections throughout the brain to similar brain areas as shown in Figure 3 (13, 189–191). Peyron et al. (13) observed that Hcrt neurons project throughout the hypothalamus and to extrahypothalamic areas, the densest of which involving the locus coeruleus. Fibers are also observed in the septal nuclei, bed nucleus of the stria terminalis, paraventricular and reuniens nuclei of the thalamus, zona incerta, subthalamic nucleus, central gray, substantia nigra, raphe nuclei, parabrachial area, medullary reticular formation, and the nucleus of the solitary tract (13). For melanin-concentrating hormone, similar projections are observed (190, 192), suggesting that MCH acts on the same brain structures, but with potentially opposing effects. These widespread projections to diverse brain structures, together with the known impact of the Hcrt and MCH systems on many physiological functions such as food intake, regulation of blood pressure, the neuroendocrine system, body temperature and the sleep-wake cycle, places both Hcrt and MCH in key positions. They can be considered top-down network control systems in behavioral state modification while also controlling physiological responses appropriate for the behavioral state. Moreover, they are in a position within the hypothalamus to receive diverse information related to temperature, energy status and homeostatic sleep pressure, suggesting that these opposing neuronal systems are part of a complex integration system to regulate output control for behavioral state and its physiological correlates.
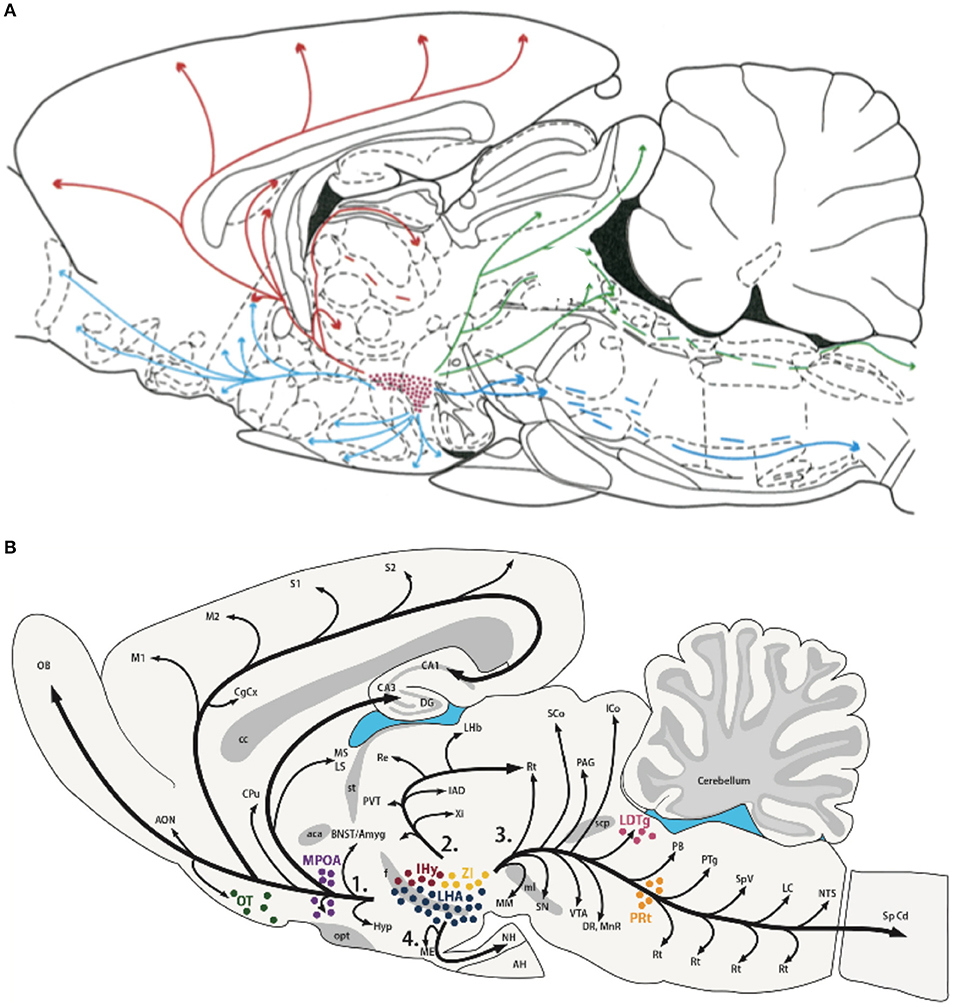
Figure 3. Efferent projections of the hypocretin/orexin (Hcrt) (A) and melanin concentrating hormone (MCH) (B) systems. The extensive efferent projections from both the Hcrt and MCH neuronal populations originating from within the LH generally overlap and include similar target structures. The diversity of their projections, together with their reciprocal activity patterns and known opposing effects on target systems, is consistent with their hypothesized role in modulating global shifts in network activity and coordinating behavioral state transitions. Figures reprinted with permission (A) Peyron et al. (13), and (B) Diniz and Bittencourt (188). For abbreviations in (B), see Diniz and Bittencourt (188).
To REM or to Wake? Optimizing Resource Allocations Within Sleep
After entering NREM sleep, an organism must make a decision as to when it should transition back to wake or, instead, enter REM sleep. One of the most consistent findings in mammalian sleep is the highly structured cycling between NREM and REM sleep. Although the purpose of this cycling is not understood, the duration from one REM period to the next is markedly consistent within any given species of mammal even when considering inter-individual variation. Indeed, one of the strongest phylogenetic correlations across species with respect to sleep parameters thus far examined is between REM-REM cycle length and body mass (193–196). That is, species with a smaller body mass, such as a mouse, cycle much faster from one REM period to another and exhibit much shorter REM sleep bout durations compared to species with a larger body mass such as in man or the elephant. However, the slope of this positive correlation between body mass and cycle length is considerably lower than what one would expect if this correlation were simply based on metabolic rate [see (37, 195)].
We recently proposed that REM sleep cycles with NREM sleep based on thermoregulatory constraints as a means of optimizing REM sleep expression and resource allocations (37). During REM sleep, thermoregulatory control is suspended (197, 198). Although mammals will pant, shiver or sweat during NREM sleep if thermally challenged, these thermoregulatory defenses cease during REM sleep, even when sleeping in ambient temperatures well outside of the thermoneutral zone (TNZ). Thermoregulation and the ability to maintain a constant body temperature is the most energetically expensive biological function encountered by endotherms, increasing energy requirements approximately 10-fold over a similar sized ectotherm (199–201). A constraint of REM sleep is that the longer any single bout of this sleep state, the more likely core body temperature may deviate toward the ambient temperature (202, 203). As a result, REM sleep bout durations are constrained in large part by the animal's surface area to volume ratio (its ability to retain heat) if the animal is to avoid spending excess energy to defend the core temperature because of REM sleep (37).
The energy allocation hypothesis postulates that REM sleep is a behavioral strategy that reallocates resources away from costly thermoregulatory defenses into REM sleep-specific biological functions to improve reproductive success or evolutionary fitness (37). Indeed, although REM sleep is a time of generalized skeletal muscle atonia associated with decreases in BAT, core and liver temperatures (95), brain temperature and brain metabolic rate markedly increase during REM sleep (95, 204, 205). Even though brain metabolic rate significantly increases during REM sleep, whole organism metabolic rate remains unchanged when comparing NREM to REM sleep (42, 79, 206–209). This finding suggests a major internal reallocation of resources during transitions from NREM to REM sleep while maintaining sleep as a net energy neutral state with respect to whole organism energy consumption. The central nervous system and the reproductive system (as seen by penile erections during REM sleep) appear to be major beneficiaries of this energy allocation strategy of REM sleep (37). The cost of this strategy is that the animal is more susceptible to thermal challenges. However, if the organism can cycle NREM and REM sleep in a manner that optimizes total REM sleep quantity while minimizing the need for thermoregulatory defense, such a strategy would by highly advantageous if it achieves greater CNS benefit at a lower, whole organism, energy cost (37).
It is well-established that ambient temperature warming toward the high end of the TNZ increases REM sleep expression across mammalian species, whereas any deviation away from thermoneutrality preferentially decreases REM sleep over NREM sleep (202, 210–215). Moreover, increases in ambient temperature, even within the narrow TNZ, increase NREM to REM sleep transitions, increase REM sleep bout durations, and shorten REM-to-REM sleep cycle lengths (210, 212, 216).
The LH may play an important role in integrating inputs related to ambient temperature, energy balance and sleep pressure for the output control of transitions from NREM sleep to either wakefulness or REM sleep (see Figure 4). As shown in Figures 2 and 4, the LH receives either direct or indirect inputs regarding ambient temperature and sleep drive from the preoptic area (134). Moreover, the Hcrt and MCH systems are both receptive to the energy status of the organism as described above. The reciprocal firing patterns of the Hcrt and MCH systems, together with their opposing effects on diverse peripheral tissues and behavioral state (REM vs. wake), implicate these two neuronal groups within the LH as critical for its output control. In this view, the MCH system will be favored during warm thermoneutral ambient temperatures, particularly when energy balance is positive or during high sleep pressure, thus increasing the probability of transitions from NREM to REM sleep, decreasing the REM-to-REM sleep cycle length, or increasing REM sleep bout durations. We predict that disruption to either the Hcrt or MCH systems will adversely affect normal output control of NREM sleep transitions with respect to the appropriate integration of such sensory inputs.
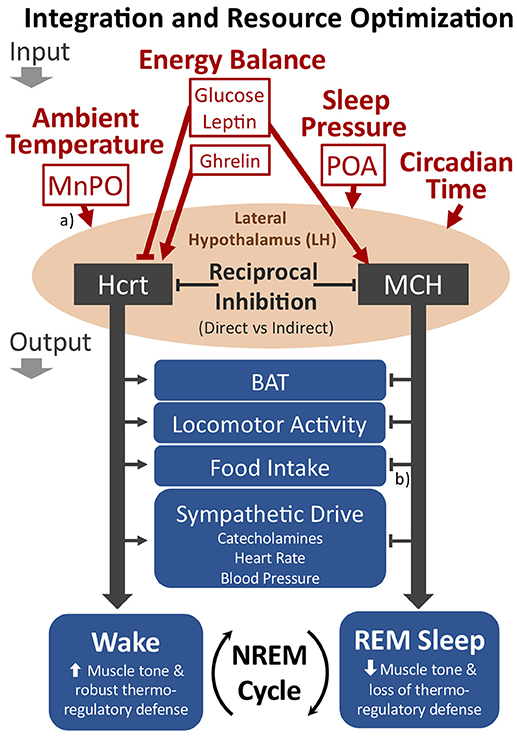
Figure 4. The proposed role of the LH as an integrator of key inputs related to ambient temperature, energy balance, and sleep pressure for the cycling of NREM sleep to either wakefulness or REM sleep. The Hcrt and MCH neuronal systems within the LH show reciprocal firing patterns, suggesting either direct or indirect reciprocal inhibition. If Hcrt activity predominates, the behavioral state of wakefulness is favored, including the promotion of brown adipose tissue (BAT) activation, locomotor activity, food intake, and sympathetic drive. With MCH activity predominating, however, REM sleep is favored. The LH may optimize resource allocations within sleep by modulating the cycling of NREM sleep to either REM sleep or wakefulness (see text). Additional relevant inputs to the LH not depicted in the figure include information pertaining to positive or negative emotional content. (a) The MnPO and POA contain warm sensitive sleep active neurons that may directly innervate the LH. Additionally, the MnPO contains thermosensitive neurons receiving input from the periphery that may also indirectly convey thermoregulatory information to the LH. (b) Although the MCH system appears to have opposing output effects with respect to Hcrt, MCH may either increase or decrease food intake depending on food availability and energy status (see text). Straight arrowheads or bars represent activation or inhibition, respectively. Not shown in the figure are additional cell types within the LH that are involved in behavioral state transitions, such as GABAergic neurons that project to the thalamic reticular nucleus for activation of wakefulness (140). MnPO, median preoptic nucleus; POA, preoptic area.
Consistent with the energy allocation hypothesis, we propose that the cycling of NREM and REM sleep optimizes resource allocations within sleep by integrating the competing benefits from either thermoregulation vs. activation of REM sleep-coupled functions, including memory consolidation (217, 218), sensory-motor integration (219), visual system development and maintenance (220–222), and reproductive function (37, 223). Organisms that optimize such competing investments at lower, whole organism, energy costs would carry a selective advantage, particularly if able to opportunistically increase REM sleep in warm, thermoneutral, ambient temperatures, or selectively reduce REM sleep if ambient temperatures deviate from thermoneutrality. The LH and the Hcrt system appear to play a major role in optimizing behavioral state transitions. Indeed, loss of Hcrt, as seen in narcolepsy, leads to the abnormally frequent brief awakenings during sleep with behavioral state instability and the inappropriate intrusion of REM sleep or its associated components into wakefulness.
Summary
In the 20 years since the discovery of Hcrt, our field has come to appreciate the great diversity of physiological processes under its control, including sleep-wake modulation, feeding behavior, thermoregulation, reward processing, sympathetic output, and cardiovascular control. A compelling hypothesis has been that the Hcrt system integrates the sleep-wake cycle with energy status of the organism given its receptivity to signals of energy balance. Although Hcrt may promote wakefulness and feeding behavior during periods of hunger, a parsimonious role for Hcrt in REM sleep regulation with respect to energy or energy balance has yet to be addressed.
We propose that Hcrt and MCH, through their complex interactions with heterogeneous neuronal populations within the LH, dynamically optimize energy utilization through global shifts in resource allocations. These global shifts are achieved through sleep-wake transitions and the coupling of specific biological processes with behavioral state (37, 38). Mathematical modeling suggests that energy savings from sleep-wake cycling are amplified as either waking-related allocations are eliminated during sleep or sleep-dependent functions are decreased during wakefulness (38). Moreover, this modeling shows that metabolic partitioning, or resource optimization, involving both central and peripheral tissues has a greater theoretical impact on total daily energy conservation than what a single organ or structure could otherwise achieve. Optimization of resource allocations at the whole organism level requires a top-down network control system capable of integrating key input variables such as energy status, thermoregulatory demands, and homeostatic sleep need. The Hcrt and MCH systems through their diverse hypothalamic inputs and their extensive efferent projections are ideal candidate structures for this role.
We further postulate that optimization of resource allocations not only occurs between wake and sleep, but also within sleep through NREM-REM sleep cycling. Through an integration of thermoregulatory input, Hcrt and other hypothalamic circuits such as the MCH system appear to modulate the probability of behavioral state transitions from NREM sleep to either REM sleep or wakefulness. If the circumstances are not ideal for REM sleep, such as when ambient temperatures deviate from thermoneutrality and the organism must resort to thermoregulatory defenses such as shivering or BAT thermogenesis, Hcrt will inhibit REM sleep by promoting arousal and heat production. However, if the ambient temperature is within thermoneutrality, the organism may forego thermoregulatory defense and, instead, opportunistically invest into REM sleep coupled biological processes.
Future research is also needed to better understand mechanisms, including costs and benefits, of dynamic resource optimization. For example, although total (24-h) energy expenditure is decreased in Hcrt-KO mice compared to WT controls (79), the loss of Hcrt carries significant costs with respect to decreased locomotor activity, feeding behavior, or reward processing, as well as disruption of thermoregulatory control, and an inability to sustain prolonged waking periods. Organisms must expend energy not only to obtain energy, but also to maximize reproductive success and evolutionary fitness. We advance the hypothesis that the LH promotes optimization of such expenditures. This optimization brings efficiencies that ultimately allow organisms to essentially achieve more with less. This theoretical construct is consistent with the energy allocation hypothesis of sleep function (37, 38), providing a parsimonious perspective on the role of the LH in the cycling of NREM sleep, REM sleep and wakefulness as a means of dynamically optimizing resource allocations and the promotion of energy conservation.
Author Contributions
BL has written the majority of the text. AA and CB supported this article by revising it critically. MHS is responsible for the concepts and design of the review, as well as writing significant passages and text editing.
Conflict of Interest Statement
The authors declare that the research was conducted in the absence of any commercial or financial relationships that could be construed as a potential conflict of interest.
Acknowledgments
This work is supported by the Center for Experimental Neurology and the Department of Neurology at the University of Bern, Bern University Hospital, Inselspital, as well as the Insel Grant 84801184, and the Ohio Sleep Medicine Institute. AA was supported by the Human Frontier Science Program (RGY0076/2012), Inselspital University Hospital Bern, Swiss National Science Foundation (31003A_156156), European Research Council (725850), Sinergia (CRSII3_160803), and the University of Bern.
References
1. Yamanaka A, Beuckmann CT, Willie JT, Hara J, Tsujino N, Mieda M. Hypothalamic orexin neurons regulate arousal according to energy balance in mice. Neuron (2003) 38:701–13. doi: 10.1016/S0896-6273(03)00331-3
2. Adamantidis A, de Lecea L. The hypocretins as sensors for metabolism and arousal. J Physiol. (2009) 587:33–40. doi: 10.1113/jphysiol.2008.164400
3. Herrera CG, Ponomarenko A, Korotkova T, Burdakov D, Adamantidis A. Sleep & metabolism: The multitasking ability of lateral hypothalamic inhibitory circuitries. Front Neuroendocrinol. (2017) 44:27–34. doi: 10.1016/j.yfrne.2016.11.002
4. Mieda M, Williams SC, Sinton CM, Richardson JA, Sakurai T, Yanagisawa M. Orexin neurons function in an efferent pathway of a food-entrainable circadian oscillator in eliciting food-anticipatory activity and wakefulness. J Neurosci. (2004) 24:10493–501. doi: 10.1523/JNEUROSCI.3171-04.2004
5. Rao Y, Lu M, Ge F, Marsh DJ, Qian S, Wang AH. Regulation of synaptic efficacy in hypocretin/orexin-containing neurons by melanin concentrating hormone in the lateral hypothalamus. J Neurosci. (2008) 28:9101–10. doi: 10.1523/JNEUROSCI.1766-08.2008
6. Abizaid A, Horvath TL. Brain circuits regulating energy homeostasis. Regul Pept. (2008) 149:3–10. doi: 10.1016/j.regpep.2007.10.006
7. Roman A, Meftah S, Arthaud S, Luppi PH, Peyron C. The inappropriate occurrence of REM sleep in narcolepsy is not due to a defect in homeostatic regulation of REM sleep. Sleep (2018) 41:1–14. doi: 10.1093/sleep/zsy046
8. Bassetti CL. Spectrum of narcolepsy. In: Baumann CR, Scammell TE, Bassetti CL, editors. Narcolepsy: Pathophysiology, Diagnosis and Treatment. New York, NY: Springer (2011). p. 309–20.
9. Sturzenegger C, Bassetti CL. The clinical spectrum of narcolepsy with cataplexy: a reappraisal. J Sleep Res. (2004) 13:395–406. doi: 10.1111/j.1365-2869.2004.00422.x
10. Date Y, Ueta Y, Yamashita H, Yamaguchi H, Matsukura S, Kangawa K. Orexins, orexigenic hypothalamic peptides, interact with autonomic, neuroendocrine and neuroregulatory systems. Proc Natl Acad Sci USA. (1999) 96:748–53. doi: 10.1073/pnas.96.2.748
11. de Lecea L, Kilduff TS, Peyron C, Gao X, Foye PE, Danielson PE. The hypocretins: hypothalamus-specific peptides with neuroexcitatory activity. Proc Natl Acad Sci USA. (1998) 95:322–7. doi: 10.1073/pnas.95.1.322
12. Sakurai T, Amemiya A, Ishii M, Matsuzaki I, Chemelli RM, Tanaka H, et al. Orexins and orexin receptors: a family of hypothalamic neuropeptides and G protein-coupled receptors that regulate feeding behavior. Cell (1998) 92:573–85. doi: 10.1016/S0092-8674(00)80949-6
13. Peyron C, Tighe DK, van den Pol AN, de Lecea L, Heller HC, Sutcliffe JG. Neurons containing hypocretin (orexin) project to multiple neuronal systems. J Neurosci. (1998) 18:9996–10015. doi: 10.1523/JNEUROSCI.18-23-09996.1998
14. Harrison TA, Chen CT, Dun NJ, Chang JK. Hypothalamic orexin A-immunoreactive neurons project to the rat dorsal medulla. Neurosci Lett. (1999) 273:17–20. doi: 10.1016/S0304-3940(99)00611-4
15. Kilduff TS, Peyron C. The hypocretin/orexin ligand-receptor system: implications for sleep and sleep disorders. Trends Neurosci. (2000) 23:359–65. doi: 10.1016/S0166-2236(00)01594-0
16. Thannickal TC, Moore RY, Nienhuis R, Ramanathan L, Gulyani S, Aldrich M. Reduced number of hypocretin neurons in human narcolepsy. Neuron (2000) 27:469–74. doi: 10.1016/S0896-6273(00)00058-1
17. Nauta WJH. Hypothalamic regulation of sleep in rats; an experimental study. J Neurophysiol. (1946) 9:285–316. doi: 10.1152/jn.1946.9.4.285
18. von Economo C. Sleep as a problem of localization. J Nerv Ment Dis. (1930) 71:249–59. doi: 10.1097/00005053-193003000-00001
19. Estabrooke IV, McCarthy MT, Ko E, Chou TC, Chemelli RM, Yanagisawa M. Fos expression in orexin neurons varies with behavioral state. J Neurosci. (2001) 21:1656–62. doi: 10.1523/JNEUROSCI.21-05-01656.2001
20. Lee MG, Hassani OK, Jones BE. Discharge of identified orexin/hypocretin neurons across the sleep-waking cycle. J Neurosci. (2005) 25:6716–20. doi: 10.1523/JNEUROSCI.1887-05.2005
21. Mileykovskiy BY, Kiyashchenko LI, Siegel JM. Behavioral correlates of activity in identified hypocretin/orexin neurons. Neuron (2005) 46:787–98. doi: 10.1016/j.neuron.2005.04.035
22. Takahashi K, Lin J-S, Sakai K. Neuronal activity of orexin and non-orexin waking-active neurons during wake-sleep states in the mouse. Neuroscience (2008) 153:860–70. doi: 10.1016/j.neuroscience.2008.02.058
23. Kiyashchenko LI, Mileykovskiy BY, Maidment N, Lam HA, Wu MF, John J. Release of hypocretin (orexin) during waking and sleep states. J Neurosci. (2002) 22:5282–6. doi: 10.1523/JNEUROSCI.22-13-05282.2002
24. Salomon RM, Ripley B, Kennedy JS, Johnson B, Schmidt D, Zeitzer JM. Diurnal variation of cerebrospinal fluid hypocretin-1 (Orexin-A) levels in control and depressed subjects. Biol Psychiatry (2003) 54:96–104. doi: 10.1016/S0006-3223(02)01740-7
25. Zeitzer JM, Buckmaster CL, Parker KJ, Hauck CM, Lyons DM, Mignot E. Circadian and homeostatic regulation of hypocretin in a primate model: implications for the consolidation of wakefulness. J Neurosci. (2003) 23:3555–60. doi: 10.1523/JNEUROSCI.23-08-03555.2003
26. Hagan JJ, Leslie RA, Patel S, Evans ML, Wattam TA, Holmes S. Orexin A activates locus coeruleus cell firing and increases arousal in the rat. Proc Natl Acad Sci USA. (1999) 96:10911–6. doi: 10.1073/pnas.96.19.10911
27. Bourgin P, Huitrón-Résendiz S, Spier AD, Fabre V, Morte B, Criado JR. Hypocretin-1 modulates rapid eye movement sleep through activation of locus coeruleus neurons. J Neurosci. (2000) 20:7760–5. doi: 10.1523/JNEUROSCI.20-20-07760.2000
28. España RA, Baldo BA, Kelley AE, Berridge CW. Wake-promoting and sleep-suppressing actions of hypocretin (orexin): basal forebrain sites of action. Neuroscience (2001) 106:699–715. doi: 10.1016/S0306-4522(01)00319-0
29. Methippara MM, Alam MN, Szymusiak R, McGinty D. Effects of lateral preoptic area application of orexin-A on sleep-wakefulness. Neuroreport (2000) 11:3423–6. doi: 10.1097/00001756-200011090-00004
30. Piper DC, Upton N, Smith MI, Hunter AJ. The novel brain neuropeptide, orexin-A, modulates the sleep-wake cycle of rats. Eur J Neurosci. (2000) 12:726–30. doi: 10.1046/j.1460-9568.2000.00919.x
31. Xi MC, Morales FR, Chase MH. Effects on sleep and wakefulness of the injection of hypocretin-1 (orexin-A) into the laterodorsal tegmental nucleus of the cat. Brain Res. (2001) 901:259–64. doi: 10.1016/S0006-8993(01)02317-4
32. Morairty SR, Wisor J, Silveira K, Sinko W, Kilduff TS. The wake-promoting effects of hypocretin-1 are attenuated in old rats. Neurobiol Aging (2011) 32:1514–27. doi: 10.1016/j.neurobiolaging.2009.07.017
33. Tsunematsu T, Kilduff TS, Boyden ES, Takahashi S, Tominaga M, Yamanaka A. Acute optogenetic silencing of orexin/hypocretin neurons induces slow-wave sleep in mice. J Neurosci. (2011) 31:10529–39. doi: 10.1523/JNEUROSCI.0784-11.2011
34. Adamantidis AR, Zhang F, Aravanis AM, Deisseroth K, de Lecea L. Neural substrates of awakening probed with optogenetic control of hypocretin neurons. Nature (2007) 450:420–4. doi: 10.1038/nature06310
35. Carter ME, Adamantidis A, Ohtsu H, Deisseroth K, de Lecea L. Sleep homeostasis modulates hypocretin-mediated sleep-to-wake transitions. J Neurosci. (2009) 29:10939–49. doi: 10.1523/JNEUROSCI.1205-09.2009
36. Tsunematsu T, Tabuchi S, Tanaka KF, Boyden ES, Tominaga M, Yamanaka A. Long-lasting silencing of orexin/hypocretin neurons using archaerhodopsin induces slow-wave sleep in mice. Behav Brain Res. (2013) 255:64–74. doi: 10.1016/j.bbr.2013.05.021
37. Schmidt MH. The energy allocation function of sleep: a unifying theory of sleep, torpor, and continuous wakefulness. Neurosci Biobehav Rev. (2014) 47:122–53. doi: 10.1016/j.neubiorev.2014.08.001
38. Schmidt MH, Swang TW, Hamilton IM, Best JA. State-dependent metabolic partitioning and energy conservation: a theoretical framework for understanding the function of sleep. PLoS ONE (2017) 12:e0185746. doi: 10.1371/journal.pone.0185746
39. Allison T, Van Twyver H. Sleep in the moles, Scalopus aquaticus and Condylura cristata. Exp Neurol. (1970) 27:564–78. doi: 10.1016/0014-4886(70)90117-2
40. Berger RJ, Phillips NH. Energy conservation and sleep. Behav Brain Res. (1995) 69:65–73. doi: 10.1016/0166-4328(95)00002-B
41. Siegel JM, Siegel JM. Clues to the functions of mammalian sleep. Nature (2005) 437:1264–71. doi: 10.1038/nature04285
42. Jung CM, Melanson EL, Frydendall EJ, Perreault L, Eckel RH, Wright KP. Energy expenditure during sleep, sleep deprivation and sleep following sleep deprivation in adult humans. J Physiol. (2011) 589(Pt 1):235–44. doi: 10.1113/jphysiol.2010.197517
43. Rechtschaffen A. Current perspectives on the function of sleep. Perspect Biol Med. (1998) 41:359–90. doi: 10.1353/pbm.1998.0051
44. Zepelin H. Mammalian sleep. In: Kryger M, Roth T, Dement W, editorS. Mammalian Sleep. Philadelphia, PA: W.B. Saunders Co. (1989).
45. Zepelin H, Rechtschaffen A. Mammalian sleep, longevity, and energy metabolism. Brain Behav Evol. (1974) 10:425–70. doi: 10.1159/000124330
46. Horne J. Why We Sleep: The Functions of Sleep in Humans and Other Mammals. New York, NY: Oxford University Press (1988).
47. Mackiewicz M, Zimmerman JE, Shockley KR, Churchill GA, Pack AI. What are microarrays teaching us about sleep? Trends Mol Med. (2009) 15:79–87. doi: 10.1016/j.molmed.2008.12.002
48. Cirelli C. The genetic and molecular regulation of sleep: from fruit flies to humans. Nat Rev Neurosci. (2009) 10:549–60. doi: 10.1038/nrn2683
49. Imeri L, Opp MR. How (and why) the immune system makes us sleep. Nat Rev Neurosci. (2009) 10:199–210. doi: 10.1038/nrn2576
50. Xie L, Kang H, Xu Q, Chen MJ, Liao Y, Thiyagarajan M. Sleep drives metabolite clearance from the adult brain. Science (2013) 342:373–7. doi: 10.1126/science.1241224
51. Mignot E. Why we sleep: the temporal organization of recovery. PLoS Biol. (2008) 6:e106. doi: 10.1371/journal.pbio.0060106
52. Everson CA, Henchen CJ, Szabo A, Hogg N. Cell injury and repair resulting from sleep loss and sleep recovery in laboratory rats. Sleep (2014) 37:1929–40. doi: 10.5665/sleep.4244
53. Aton SJ, Seibt J, Dumoulin M, Jha SK, Steinmetz N, Coleman T. Mechanisms of sleep-dependent consolidation of cortical plasticity. Neuron (2009) 61:454–66. doi: 10.1016/j.neuron.2009.01.007
54. Krueger JM, Obál F, Fang J. Why we sleep: a theoretical view of sleep function. Sleep Med Rev. (1999) 3:119–29. doi: 10.1016/S1087-0792(99)90019-9
55. Stickgold R. Sleep-dependent memory consolidation. Nature (2005) 437:1272–8. doi: 10.1038/nature04286
56. Tononi G, Cirelli C. Sleep and the price of plasticity: from synaptic and cellular homeostasis to memory consolidation and integration. Neuron (2014) 81:12–34. doi: 10.1016/j.neuron.2013.12.025
57. Krueger JM, Frank MG, Wisor JP, Roy S. Sleep function: Toward elucidating an enigma. Sleep Med Rev. (2016) 28:46–54. doi: 10.1016/j.smrv.2015.08.005
58. Maret S, Dorsaz S, Gurcel L, Pradervand S, Petit B, Pfister C. Homer1a is a core brain molecular correlate of sleep loss. Proc Natl Acad Sci USA. (2007) 104:20090–5. doi: 10.1073/pnas.0710131104
59. Peyron C, Faraco J, Rogers W, Ripley B, Overeem S, Charnay Y. A mutation in a case of early onset narcolepsy and a generalized absence of hypocretin peptides in human narcoleptic brains. Nat Med. (2000) 6:991–7. doi: 10.1038/79690
60. Lin L, Faraco J, Li R, Kadotani H, Rogers W, Lin X. The sleep disorder canine narcolepsy is caused by a mutation in the hypocretin (orexin) receptor 2 gene. Cell (1999) 98:365–76. doi: 10.1016/S0092-8674(00)81965-0
61. Brisbare-Roch C, Dingemanse J, Koberstein R, Hoever P, Aissaoui H, Flores S, et al. Promotion of sleep by targeting the orexin system in rats, dogs and humans. Nat Med. (2007) 13:150–5. doi: 10.1038/nm1544
62. Eriksson KS, Sergeeva O, Brown RE, Haas HL. Orexin/hypocretin excites the histaminergic neurons of the tuberomammillary nucleus. J Neurosci. (2001) 21:9273–9. doi: 10.1523/JNEUROSCI.21-23-09273.2001
63. Kaitin KI, Kilduff TS, Dement WC. Sleep fragmentation in canine narcolepsy. Sleep (1986) 9(1 Pt 2):116–9. doi: 10.1093/sleep/9.1.116
64. Nishino S, Riehl J, Hong J, Kwan M, Reid M, Mignot E. Is narcolepsy a REM sleep disorder? Analysis of sleep abnormalities in narcoleptic Dobermans. Neurosci Res. (2000) 38:437–46. doi: 10.1016/S0168-0102(00)00195-4
65. Mukai J, Uchida S, Miyazaki S, Nishihara K, Honda Y. Spectral analysis of all-night human sleep EEG in narcoleptic patients and normal subjects. J Sleep Res. (2003) 12:63–71. doi: 10.1046/j.1365-2869.2003.00331.x
66. Mochizuki T, Crocker A, McCormack S, Yanagisawa M, Sakurai T, Scammell TE. Behavioral state instability in orexin knock-out mice. J Neurosci. (2004) 24:6291–300. doi: 10.1523/JNEUROSCI.0586-04.2004
67. Saper CB, Scammell TE, Lu J. Hypothalamic regulation of sleep and circadian rhythms. Nature (2005) 437:1257–63. doi: 10.1038/nature04284
68. Scammell TE. The neurobiology, diagnosis, and treatment of narcolepsy. Ann Neurol. (2003) 53:154–66. doi: 10.1002/ana.10444
69. Sakurai T, Nagata R, Yamanaka A, Kawamura H, Tsujino N, Muraki Y. Input of orexin/hypocretin neurons revealed by a genetically encoded tracer in mice. Neuron (2005) 46:297–308. doi: 10.1016/j.neuron.2005.03.010
70. Alföldi P, Rubicsek G, Cserni G, Obál F. Brain and core temperatures and peripheral vasomotion during sleep and wakefulness at various ambient temperatures in the rat. Pflugers Arch. (1990) 417:336–41. doi: 10.1007/BF00371001
71. McGinty D, Szymusiak R. Keeping cool: a hypothesis about the mechanisms and functions of slow-wave sleep. Trends Neurosci. (1990) 13:480–7. doi: 10.1016/0166-2236(90)90081-K
72. Kräuchi K, Cajochen C, Werth E, Wirz-Justice A. Warm feet promote the rapid onset of sleep. Nature (1999) 401:36–7. doi: 10.1038/43366
73. Gong H, Szymusiak R, King J, Steininger T, McGinty D. Sleep-related c-Fos protein expression in the preoptic hypothalamus: effects of ambient warming. Am J Physiol Regul Integr Comp Physiol. (2000) 279:R2079-2088. doi: 10.1152/ajpregu.2000.279.6.R2079
74. Yoshida K, Konishi M, Nagashima K, Saper CB, Kanosue K. Fos activation in hypothalamic neurons during cold or warm exposure: projections to periaqueductal gray matter. Neuroscience (2005) 133:1039–46. doi: 10.1016/j.neuroscience.2005.03.044
75. Fronczek R, Raymann RJ, Romeijn N, Overeem S, Fischer M, van Dijk JG. Manipulation of core body and skin temperature improves vigilance and maintenance of wakefulness in narcolepsy. Sleep (2008) 31:233–40. doi: 10.1093/sleep/31.2.233
76. Mosko SS, Holowach JB, Sassin JF, Mosko SS, Holowach JB, Sassin JF. The 24-hour rhythm of core temperature in narcolepsy. Sleep (1983) 6:137–46. doi: 10.1093/sleep/6.2.137
77. Pollak CP, Wagner DR. Core body temperature in narcoleptic and normal subjects living in temporal isolation. Pharmacol Biochem Behav. (1994) 47:65–71. doi: 10.1016/0091-3057(94)90112-0
78. Mochizuki T, Klerman EB, Sakurai T, Scammell TE. Elevated body temperature during sleep in orexin knockout mice. Am J Physiol Regul Integr Comp Physiol. (2006) 291:R533-540. doi: 10.1152/ajpregu.00887.2005
79. Zhang S, Zeitzer JM, Sakurai T, Nishino S, Mignot E. Sleep/wake fragmentation disrupts metabolism in a mouse model of narcolepsy. J Physiol. (2007) 581(Pt 2):649–63. doi: 10.1113/jphysiol.2007.129510
80. Someren EJWV. Sleep propensity is modulated by circadian and behavior-induced changes in cutaneous temperature. J Therm Biol. (2004) 7–8:437–44. doi: 10.1016/j.jtherbio.2004.08.003
81. Marotte H, Timbal J. Circadian rhythm of temperature in man. Comparative study with two experiment protocols. Chronobiologia (1981) 8:87–100.
82. Kleitman N, Jackson DP. Body temperature and performance under different routines. J Appl Physiol. (1950) 3:309–28. doi: 10.1152/jappl.1950.3.6.309
83. Hull JT, Wright KP, Czeisler CA. The influence of subjective alertness and motivation on human performance independent of circadian and homeostatic regulation. J Biol Rhythms (2003) 18:329–38. doi: 10.1177/0748730403253584
84. Wright KP, Hull JT, Czeisler CA. Relationship between alertness, performance, and body temperature in humans. Am J Physiol Regul Integr Comp Physiol. (2002) 283:R1370–1377. doi: 10.1152/ajpregu.00205.2002
85. Cajochen C, Khalsa SB, Wyatt JK, Czeisler CA, Dijk DJ. EEG and ocular correlates of circadian melatonin phase and human performance decrements during sleep loss. Am J Physiol. (1999) 277(3 Pt 2):R640–649.
86. Monk TH, Buysse DJ, Reynolds CF, Berga SL, Jarrett DB, Begley AE. Circadian rhythms in human performance and mood under constant conditions. J Sleep Res. (1997) 6:9–18. doi: 10.1046/j.1365-2869.1997.00023.x
87. Wyatt JK, Ritz-De Cecco A, Czeisler CA, Dijk DJ. Circadian temperature and melatonin rhythms, sleep, and neurobehavioral function in humans living on a 20-h day. Am J Physiol. (1999) 277(4 Pt 2):R1152–1163.
88. Tupone D, Madden CJ, Cano G, Morrison SF. An orexinergic projection from perifornical hypothalamus to raphe pallidus increases rat brown adipose tissue thermogenesis. J Neurosci. (2011) 31:15944–55. doi: 10.1523/JNEUROSCI.3909-11.2011
89. Sellayah D, Bharaj P, Sikder D. Orexin is required for brown adipose tissue development, differentiation, and function. Cell Metab. (2011) 14:478–90. doi: 10.1016/j.cmet.2011.08.010
90. Madden CJ, Tupone D, Morrison SF. Orexin modulates brown adipose tissue thermogenesis. Biomol Concepts (2012) 3:381–6. doi: 10.1515/bmc-2011-0066
91. Sellayah D, Sikder D. Orexin restores aging-related brown adipose tissue dysfunction in male mice. Endocrinology (2014) 155:485–501. doi: 10.1210/en.2013-1629
92. Yoneshiro T, Aita S, Matsushita M, Kameya T, Nakada K, Kawai Y. Brown adipose tissue, whole-body energy expenditure, and thermogenesis in healthy adult men. Obes Silver Spring Md (2011) 19:13–6. doi: 10.1038/oby.2010.105
93. Yoneshiro T, Aita S, Matsushita M, Okamatsu-Ogura Y, Kameya T, Kawai Y. Age-related decrease in cold-activated brown adipose tissue and accumulation of body fat in healthy humans. Obes Silver Spring Md (2011) 19:1755–60. doi: 10.1038/oby.2011.125
94. Calasso M, Zantedeschi E, Parmeggiani PL. Cold-defense function of brown adipose tissue during sleep. Am J Physiol. (1993) 265(5 Pt 2):R1060-1064.
95. Dewasmes G, Loos N, Delanaud S, Ramadan W, Dewasmes D. Liver temperature during sleep. Sleep (2003) 26:948–50. doi: 10.1093/sleep/26.8.948
96. Enevoldsen LH, Tindborg M, Hovmand NL, Christoffersen C, Ellingsgaard H, Suetta C. Functional brown adipose tissue and sympathetic activity after cold exposure in humans with type 1 narcolepsy. Sleep (2018) 41:1–9. doi: 10.1093/sleep/zsy092
97. Danguir J, Nicolaidis S. Dependence of sleep on nutrients' availability. Physiol Behav. (1979) 22:735–40. doi: 10.1016/0031-9384(79)90240-3
98. Dewasmes G, Duchamp C, Minaire Y. Sleep changes in fasting rats. Physiol Behav. (1989) 46:179–84. doi: 10.1016/0031-9384(89)90252-7
99. Harnish MJ, Greenleaf SR, Orr WC. A comparison of feeding to cephalic stimulation on postprandial sleepiness. Physiol Behav. (1998) 64:93–6. doi: 10.1016/S0031-9384(98)00025-0
100. Diano S, Horvath B, Urbanski HF, Sotonyi P, Horvath TL. Fasting activates the nonhuman primate hypocretin (orexin) system and its postsynaptic targets. Endocrinology (2003) 144:3774–8. doi: 10.1210/en.2003-0274
101. Akiyama M, Yuasa T, Hayasaka N, Horikawa K, Sakurai T, Shibata S. Reduced food anticipatory activity in genetically orexin (hypocretin) neuron-ablated mice. Eur J Neurosci. (2004) 20:3054–62. doi: 10.1111/j.1460-9568.2004.03749.x
102. Tyree SM, de Lecea L. Optogenetic investigation of arousal circuits. Int J Mol Sci. (2017) 18:E1773. doi: 10.3390/ijms18081773
103. Sakurai T. The neural circuit of orexin (hypocretin): maintaining sleep and wakefulness. Nat Rev Neurosci. (2007) 8:171–81. doi: 10.1038/nrn2092
104. Georgescu D, Zachariou V, Barrot M, Mieda M, Willie JT, Eisch AJ. Involvement of the lateral hypothalamic peptide orexin in morphine dependence and withdrawal. J Neurosci. (2003) 23:3106–11. doi: 10.1523/JNEUROSCI.23-08-03106.2003
105. Harris GC, Wimmer M, Aston-Jones G. A role for lateral hypothalamic orexin neurons in reward seeking. Nature (2005) 437:556–9. doi: 10.1038/nature04071
106. Narita M, Nagumo Y, Hashimoto S, Narita M, Khotib J, Miyatake M. Direct involvement of orexinergic systems in the activation of the mesolimbic dopamine pathway and related behaviors induced by morphine. J Neurosci. (2006) 26:398–405. doi: 10.1523/JNEUROSCI.2761-05.2006
107. Lee MJ, Lee SY, Yuan SS, Yang CJ, Yang KC, Lee TL. Comorbidity of narcolepsy and depressive disorders: a nationwide population-based study in Taiwan. Sleep Med. (2017) 39:95–100. doi: 10.1016/j.sleep.2017.07.022
108. Black J, Reaven NL, Funk SE, McGaughey K, Ohayon MM, Guilleminault C. Medical comorbidity in narcolepsy: findings from the Burden of Narcolepsy Disease (BOND) study. Sleep Med. (2017) 33:13–8. doi: 10.1016/j.sleep.2016.04.004
109. Morse AM, Sanjeev K. Narcolepsy and psychiatric disorders: comorbidities or shared pathophysiology? Med Sci Basel Switz. (2018) 6:E16. doi: 10.3390/medsci6010016.
110. Aston-Jones G, Smith RJ, Sartor GC, Moorman DE, Massi L, Tahsili-Fahadan P. Lateral hypothalamic orexin/hypocretin neurons: a role in reward-seeking and addiction. Brain Res. (2010) 1314:74–90. doi: 10.1016/j.brainres.2009.09.106
111. Matzeu A, Zamora-Martinez ER, Martin-Fardon R. The paraventricular nucleus of the thalamus is recruited by both natural rewards and drugs of abuse: recent evidence of a pivotal role for orexin/hypocretin signaling in this thalamic nucleus in drug-seeking behavior. Front Behav Neurosci. (2014) 8:117. doi: 10.3389/fnbeh.2014.00117
112. Berger RJ, Phillips NH. Comparative aspects of energy metabolism, body temperature and sleep. Acta Physiol Scand Suppl. (1988) 574:21–7.
113. Charloux A, Piquard F, Geny B, Ehrhart J, Brandenberger G. Circulating endothelin parallels arterial blood pressure during sleep in healthy subjects. Regul Pept. (2004) 119:133–8. doi: 10.1016/j.regpep.2004.01.006
114. Somers VK, Dyken ME, Mark AL, Abboud FM. Sympathetic-nerve activity during sleep in normal subjects. N Engl J Med. (1993) 328:303–7. doi: 10.1056/NEJM199302043280502
115. Van de Borne P, Nguyen H, Biston P, Linkowski P, Degaute JP. Effects of wake and sleep stages on the 24-h autonomic control of blood pressure and heart rate in recumbent men. Am J Physiol. (1994) 266(2 Pt 2):H548–554.
116. Kodama Y, Iwase S, Mano T, Cui J, Kitazawa H, Okada H. Attenuation of regional differentiation of sympathetic nerve activity during sleep in humans. J Auton Nerv Syst. (1998) 74:126–33. doi: 10.1016/S0165-1838(98)00150-7
117. Ziegler MG. Sleep disorders and the failure to lower nocturnal blood pressure. Curr Opin Nephrol Hypertens. (2003) 12:97–102. doi: 10.1097/00041552-200301000-00016
118. Schwimmer H, Stauss HM, Abboud F, Nishino S, Mignot E, Zeitzer JM. Effects of sleep on the cardiovascular and thermoregulatory systems: a possible role for hypocretins. J Appl Physiol Bethesda Md. (2010) 109:1053–63. doi: 10.1152/japplphysiol.00516.2010
119. Kayaba Y, Nakamura A, Kasuya Y, Ohuchi T, Yanagisawa M, Komuro I. Attenuated defense response and low basal blood pressure in orexin knockout mice. Am J Physiol Regul Integr Comp Physiol. (2003) 285:R581–93. doi: 10.1152/ajpregu.00671.2002
120. Shirasaka T, Nakazato M, Matsukura S, Takasaki M, Kannan H. Sympathetic and cardiovascular actions of orexins in conscious rats. Am J Physiol. (1999) 277(6 Pt 2):R1780–5.
121. Bonnavion P, Jackson AC, Carter ME, de Lecea L. Antagonistic interplay between hypocretin and leptin in the lateral hypothalamus regulates stress responses. Nat Commun. (2015) 6:6266. doi: 10.1038/ncomms7266
122. Hess WR. The autonomic nervous system. Lancet (1932) 220:1259–61. doi: 10.1016/S0140-6736(00)97332-X
123. Gent TC, Bandarabadi M, Herrera CG, Adamantidis AR. Thalamic dual control of sleep and wakefulness. Nat Neurosci. (2018) 21:974–84. doi: 10.1038/s41593-018-0164-7
124. Peever J, Fuller PM. The biology of REM sleep. Curr Biol. (2017) 27:R1237–48. doi: 10.1016/j.cub.2017.10.026
125. Luppi P-H, Fort P. Neuroanatomical and neurochemical bases of vigilance states. Handb Exp Pharmacol. (2018) 1–24. doi: 10.1007/164_2017_84
126. Gaus SE, Strecker RE, Tate BA, Parker RA, Saper CB. Ventrolateral preoptic nucleus contains sleep-active, galaninergic neurons in multiple mammalian species. Neuroscience (2002) 115:285–94. doi: 10.1016/S0306-4522(02)00308-1
127. Lu J, Greco MA, Shiromani P, Saper CB. Effect of lesions of the ventrolateral preoptic nucleus on NREM and REM sleep. J Neurosci. (2000) 20:3830–42. doi: 10.1523/JNEUROSCI.20-10-03830.2000
128. Tan CL, Cooke EK, Leib DE, Lin YC, Daly GE, Zimmerman CA. Warm-sensitive neurons that control body temperature. Cell (2016) 167:47–59.e15. doi: 10.1016/j.cell.2016.08.028
129. Alam MN, McGinty D, Szymusiak R. Preoptic/anterior hypothalamic neurons: thermosensitivity in wakefulness and non rapid eye movement sleep. Brain Res. (1996) 718:76–82. doi: 10.1016/0006-8993(96)00035-2
130. Alam MN, McGinty D, Szymusiak R. Neuronal discharge of preoptic/anterior hypothalamic thermosensitive neurons: relation to NREM sleep. Am J Physiol. (1995) 269(5 Pt 2):R1240–9.
131. Szymusiak R, Gvilia I, McGinty D. Hypothalamic control of sleep. Sleep Med. (2007) 8:291–301. doi: 10.1016/j.sleep.2007.03.013
132. Harding EC, Yu X, Miao A, Andrews N, Ma Y, Ye Z. A neuronal hub binding sleep initiation and body cooling in response to a warm external stimulus. Curr Biol. (2018) 28:2263–73.e4. doi: 10.1016/j.cub.2018.05.054
133. Morrison SF. Central neural control of thermoregulation and brown adipose tissue. Auton Neurosci Basic Clin. (2016) 196:14–24. doi: 10.1016/j.autneu.2016.02.010
134. Cerri M, Luppi M, Tupone D, Zamboni G, Amici R. REM Sleep and endothermy: potential sites and mechanism of a reciprocal interference. Front Physiol. (2017) 8:624. doi: 10.3389/fphys.2017.00624
135. Yoshida K, Li X, Cano G, Lazarus M, Saper CB. Parallel preoptic pathways for thermoregulation. J Neurosci. (2009) 29:11954–64. doi: 10.1523/JNEUROSCI.2643-09.2009
136. McKinley MJ, Yao ST, Uschakov A, McAllen RM, Rundgren M, Martelli D. The median preoptic nucleus: front and centre for the regulation of body fluid, sodium, temperature, sleep and cardiovascular homeostasis. Acta Physiol. (2015) 214:8–32. doi: 10.1111/apha.12487
137. Morrison SF. Central control of body temperature. F1000Res. (2016) 5:1–10. doi: 10.12688/f1000research.7958.1
138. Machado NLS, Abbott SBG, Resch JM, Zhu L, Arrigoni E, Lowell BB, et al. A Glutamatergic hypothalamomedullary circuit mediates thermogenesis, but not heat conservation, during stress-induced hyperthermia. Curr Biol. (2018) 28:2291–301.e5. doi: 10.1016/j.cub.2018.05.064
139. Yamashita T, Yamanaka A. Lateral hypothalamic circuits for sleep-wake control. Curr Opin Neurobiol. (2017) 44:94–100. doi: 10.1016/j.conb.2017.03.020
140. Herrera CG, Cadavieco MC, Jego S, Ponomarenko A, Korotkova T, Adamantidis A. Hypothalamic feedforward inhibition of thalamocortical network controls arousal and consciousness. Nat Neurosci. (2016) 19:290–8. doi: 10.1038/nn.4209
141. Sapin E, Bérod A, Léger L, Herman PA, Luppi P-H, Peyron C. A very large number of GABAergic neurons are activated in the tuberal hypothalamus during paradoxical (REM) sleep hypersomnia. PLoS ONE (2010) 5:e11766. doi: 10.1371/journal.pone.0011766
142. Hassani OK, Henny P, Lee MG, Jones BE. GABAergic neurons intermingled with orexin and MCH neurons in the lateral hypothalamus discharge maximally during sleep. Eur J Neurosci. (2010) 32:448–57. doi: 10.1111/j.1460-9568.2010.07295.x
143. Jennings JH, Rizzi G, Stamatakis AM, Ung RL, Stuber GD. The inhibitory circuit architecture of the lateral hypothalamus orchestrates feeding. Science (2013) 341:1517–21. doi: 10.1126/science.1241812
144. Jennings JH, Ung RL, Resendez SL, et al. Visualizing hypothalamic network dynamics for appetitive and consummatory behaviors. Cell (2015) 160:516–27. doi: 10.1016/j.cell.2014.12.026
145. Peyron C, Sapin E, Leger L, Luppi P-H, Fort P. Role of the melanin-concentrating hormone neuropeptide in sleep regulation. Peptides (2009) 30:2052–9. doi: 10.1016/j.peptides.2009.07.022
146. Varin C, Luppi P-H, Fort P. Melanin-Concentrating Hormone-expressing neurons adjust slow-wave sleep dynamics to catalyze paradoxical (REM) sleep. Sleep (2018) 41:1–12. doi: 10.1093/sleep/zsy068
147. Vetrivelan R, Kong D, Ferrari LL, Arrigoni E, Madara JC, Bandaru SS. Melanin-concentrating hormone neurons specifically promote rapid eye movement sleep in mice. Neuroscience (2016) 336:102–13. doi: 10.1016/j.neuroscience.2016.08.046
148. Jego S, Glasgow SD, Herrera CG, Ekstrand M, Reed SJ, Boyce R. Optogenetic identification of a rapid eye movement sleep modulatory circuit in the hypothalamus. Nat Neurosci. (2013) 16:1637–43. doi: 10.1038/nn.3522
149. Luppi P-H, Clément O, Fort P. Paradoxical (REM) sleep genesis by the brainstem is under hypothalamic control. Curr Opin Neurobiol. (2013) 23:786–92. doi: 10.1016/j.conb.2013.02.006
150. Chemelli RM, Willie JT, Sinton CM, Elmquist JK, Scammell T, Lee C. Narcolepsy in orexin knockout mice: molecular genetics of sleep regulation. Cell (1999) 98:437–51. doi: 10.1016/S0092-8674(00)81973-X
151. Hanriot L, Camargo N, Courau AC, Leger L, Luppi PH, Peyron C. Characterization of the melanin-concentrating hormone neurons activated during paradoxical sleep hypersomnia in rats. J Comp Neurol. (2007) 505:147–57. doi: 10.1002/cne.21482
152. Verret L, Goutagny R, Fort P, Cagnon L, Salvert D, Léger L. A role of melanin-concentrating hormone producing neurons in the central regulation of paradoxical sleep. BMC Neurosci. (2003) 4:19. doi: 10.1186/1471-2202-4-19
153. Hassani OK, Lee MG, Jones BE. Melanin-concentrating hormone neurons discharge in a reciprocal manner to orexin neurons across the sleep-wake cycle. Proc Natl Acad Sci USA. (2009) 106:2418–22. doi: 10.1073/pnas.0811400106
154. Blouin AM, Fried I, Wilson CL, Staba RJ, Behnke EJ, Lam HA. Human hypocretin and melanin-concentrating hormone levels are linked to emotion and social interaction. Nat Commun. (2013) 4:1547. doi: 10.1038/ncomms2461
155. Blanco-Centurion C, Liu M, Konadhode RP, Zhang X, Pelluru D, van den Pol AN. Optogenetic activation of melanin-concentrating hormone neurons increases non-rapid eye movement and rapid eye movement sleep during the night in rats. Eur J Neurosci. (2016) 44:2846–57. doi: 10.1111/ejn.13410
156. Ahnaou A, Drinkenburg WHIM, Bouwknecht JA, Alcazar J, Steckler T, Dautzenberg FM. Blocking melanin-concentrating hormone MCH1 receptor affects rat sleep-wake architecture. Eur J Pharmacol. (2008) 579:177–88. doi: 10.1016/j.ejphar.2007.10.017
157. Konadhode RR, Pelluru D, Shiromani PJ. Neurons containing orexin or melanin concentrating hormone reciprocally regulate wake and sleep. Front Syst Neurosci. (2014) 8:244. doi: 10.3389/fnsys.2014.00244
158. González JA, Iordanidou P, Strom M, Adamantidis A, Burdakov D. Awake dynamics and brain-wide direct inputs of hypothalamic MCH and orexin networks. Nat Commun. (2016) 7:11395. doi: 10.1038/ncomms11395
159. Apergis-Schoute J, Iordanidou P, Faure C, Jego S, Schöne C, Aitta-Aho T. Optogenetic evidence for inhibitory signaling from orexin to MCH neurons via local microcircuits. J Neurosci. (2015) 35:5435–41. doi: 10.1523/JNEUROSCI.5269-14.2015
160. Maolood N, Meister B. Nociceptin/orphanin FQ peptide in hypothalamic neurones associated with the control of feeding behaviour. J Neuroendocrinol. (2010) 22:75–82. doi: 10.1111/j.1365-2826.2009.01946.x
161. Li Y, van den Pol AN. Differential target-dependent actions of coexpressed inhibitory dynorphin and excitatory hypocretin/orexin neuropeptides. J Neurosci. (2006) 26:13037–47. doi: 10.1523/JNEUROSCI.3380-06.2006
162. Parsons MP, Hirasawa M. GIRK channel-mediated inhibition of melanin-concentrating hormone neurons by nociceptin/orphanin FQ. J Neurophysiol. (2011) 105:1179–84. doi: 10.1152/jn.00791.2010
163. Fu L-Y, Acuna-Goycolea C, van den Pol AN. Neuropeptide Y inhibits hypocretin/orexin neurons by multiple presynaptic and postsynaptic mechanisms: tonic depression of the hypothalamic arousal system. J Neurosci. (2004) 24:8741–51. doi: 10.1523/JNEUROSCI.2268-04.2004
164. Acuna-Goycolea C, van den Pol A. Glucagon-like peptide 1 excites hypocretin/orexin neurons by direct and indirect mechanisms: implications for viscera-mediated arousal. J Neurosci. (2004) 24:8141–52. doi: 10.1523/JNEUROSCI.1607-04.2004
165. Burdakov D, Jensen LT, Alexopoulos H, Williams RH, Fearon IM, O'Kelly I. Tandem-pore K+ channels mediate inhibition of orexin neurons by glucose. Neuron (2006) 50:711–22. doi: 10.1016/j.neuron.2006.04.032
166. Louis GW, Leinninger GM, Rhodes CJ, Myers MG. Direct innervation and modulation of orexin neurons by lateral hypothalamic LepRb neurons. J Neurosci. (2010) 30:11278–87. doi: 10.1523/JNEUROSCI.1340-10.2010
167. Leinninger GM, Opland DM, Jo YH, Faouzi M, Christensen L, Cappellucci LA. Leptin action via neurotensin neurons controls orexin, the mesolimbic dopamine system and energy balance. Cell Metab. (2011) 14:313–23. doi: 10.1016/j.cmet.2011.06.016
168. Goforth PB, Leinninger GM, Patterson CM, Satin LS, Myers MG. Leptin acts via lateral hypothalamic area neurotensin neurons to inhibit orexin neurons by multiple GABA-independent mechanisms. J Neurosci. (2014) 34:11405–15. doi: 10.1523/JNEUROSCI.5167-13.2014
169. Brown J, Sagante A, Mayer T, Wright A, Bugescu R, Fuller PM. Lateral hypothalamic area neurotensin neurons are required for control of orexin neurons and energy balance. Endocrinology (2018) doi: 10.1210/en.2018-00311
170. Thakkar MM, Winston S, McCarley RW. Orexin neurons of the hypothalamus express adenosine A1 receptors. Brain Res. (2002) 944:190–4. doi: 10.1016/S0006-8993(02)02873-1
171. Thakkar MM, Engemann SC, Walsh KM, Sahota PK. Adenosine and the homeostatic control of sleep: effects of A1 receptor blockade in the perifornical lateral hypothalamus on sleep-wakefulness. Neuroscience (2008) 153:875–80. doi: 10.1016/j.neuroscience.2008.01.017
172. Liu Z-W, Gao X-B. Adenosine inhibits activity of hypocretin/orexin neurons by the A1 receptor in the lateral hypothalamus: a possible sleep-promoting effect. J Neurophysiol. (2007) 97:837–48. doi: 10.1152/jn.00873.2006
173. Hausen AC, Ruud J, Jiang H, Hess S, Varbanov H, Kloppenburg P. Insulin-dependent activation of MCH neurons impairs locomotor activity and insulin sensitivity in obesity. Cell Rep. (2016) 17:2512–21. doi: 10.1016/j.celrep.2016.11.030
174. Burdakov D, Gerasimenko O, Verkhratsky A. Physiological changes in glucose differentially modulate the excitability of hypothalamic melanin-concentrating hormone and orexin neurons in situ. J Neurosci. (2005) 25:2429–33. doi: 10.1523/JNEUROSCI.4925-04.2005
175. Kong D, Vong L, Parton LE, Ye C, Tong Q, Hu X. Glucose stimulation of hypothalamic MCH neurons involves K(ATP) channels, is modulated by UCP2, and regulates peripheral glucose homeostasis. Cell Metab. (2010) 12:545–52. doi: 10.1016/j.cmet.2010.09.013
176. Jego S, Salvert D, Renouard L, Mori M, Goutagny R, Luppi PH. Tuberal hypothalamic neurons secreting the satiety molecule Nesfatin-1 are critically involved in paradoxical (REM) sleep homeostasis. PLoS ONE (2012) 7:e52525. doi: 10.1371/journal.pone.0052525
177. Ludwig DS, Tritos NA, Mastaitis JW, Kulkarni R, Kokkotou E, Elmquist J. Melanin-concentrating hormone overexpression in transgenic mice leads to obesity and insulin resistance. J Clin Invest. (2001) 107:379–86. doi: 10.1172/JCI10660
178. Qu D, Ludwig DS, Gammeltoft S, Piper M, Pelleymounter MA, Cullen MJ. A role for melanin-concentrating hormone in the central regulation of feeding behaviour. Nature (1996) 380:243–7. doi: 10.1038/380243a0
179. Rossi M, Choi SJ, O'Shea D, Miyoshi T, Ghatei MA, Bloom SR. Melanin-concentrating hormone acutely stimulates feeding, but chronic administration has no effect on body weight. Endocrinology (1997) 138:351–5. doi: 10.1210/endo.138.1.4887
180. Della-Zuana O, Presse F, Ortola C, Duhault J, Nahon JL, Levens N. Acute and chronic administration of melanin-concentrating hormone enhances food intake and body weight in Wistar and Sprague-Dawley rats. Int J Obes Relat Metab Disord J Int Assoc Study Obes. (2002) 26:1289–95. doi: 10.1038/sj.ijo.0802079
181. Gomori A, Ishihara A, Ito M, Mashiko S, Matsushita H, Yumoto M. Chronic intracerebroventricular infusion of MCH causes obesity in mice. Melanin-concentrating hormone. Am J Physiol Endocrinol Metab. (2003) 284:E583–588. doi: 10.1152/ajpendo.00350.2002
182. Whiddon BB, Palmiter RD. Ablation of neurons expressing melanin-concentrating hormone (MCH) in adult mice improves glucose tolerance independent of MCH signaling. J Neurosci. (2013) 33:2009–16. doi: 10.1523/JNEUROSCI.3921-12.2013
183. Pereira-da-Silva M, Torsoni MA, Nourani HV, Augusto VD, Souza CT, Gasparetti AL. Hypothalamic melanin-concentrating hormone is induced by cold exposure and participates in the control of energy expenditure in rats. Endocrinology (2003) 144:4831–40. doi: 10.1210/en.2003-0243
184. Oldfield BJ, Giles ME, Watson A, Anderson C, Colvill LM, McKinley MJ. The neurochemical characterisation of hypothalamic pathways projecting polysynaptically to brown adipose tissue in the rat. Neuroscience (2002) 110:515–26. doi: 10.1016/S0306-4522(01)00555-3
185. Guesdon B, Paradis E, Samson P, Richard D. Effects of intracerebroventricular and intra-accumbens melanin-concentrating hormone agonism on food intake and energy expenditure. Am J Physiol Regul Integr Comp Physiol. (2009) 296:R469-475. doi: 10.1152/ajpregu.90556.2008
186. Chung S, Wong T, Nagasaki H, Civelli O. Acute homeostatic responses to increased fat consumption in MCH1R knockout mice. J Mol Neurosci. (2010) 42:459–63. doi: 10.1007/s12031-010-9358-5
187. Verty ANA, Allen AM, Oldfield BJ. The endogenous actions of hypothalamic peptides on brown adipose tissue thermogenesis in the rat. Endocrinology (2010) 151:4236–46. doi: 10.1210/en.2009-1235
188. Diniz GB, Bittencourt JC. The melanin-concentrating hormone as an integrative peptide driving motivated behaviors. Front Syst Neurosci. (2017) 11:32. doi: 10.3389/fnsys.2017.00032
189. Peyron C, Kilduff TS. Mapping the hypocretin/orexin neuronal system: an unexpectedly productive journey. J Neurosci. (2017) 37:2268–72. doi: 10.1523/JNEUROSCI.1708-16.2016
190. Parks GS, Wang L, Wang Z, Civelli O. Identification of neuropeptide receptors expressed by melanin-concentrating hormone neurons. J Comp Neurol. (2014) 522:3817–33. doi: 10.1002/cne.23642
191. Ferreira JGP, Bittencourt JC, Adamantidis A. Melanin-concentrating hormone and sleep. Curr Opin Neurobiol. (2017) 44:152–8. doi: 10.1016/j.conb.2017.04.008
192. Bittencourt JC, Elias CF. Melanin-concentrating hormone and neuropeptide EI projections from the lateral hypothalamic area and zona incerta to the medial septal nucleus and spinal cord: a study using multiple neuronal tracers. Brain Res. (1998) 805:1–19. doi: 10.1016/S0006-8993(98)00598-8
193. Capellini I, Nunn CL, McNamara P, Preston BT, Barton RA. Energetic constraints, not predation, influence the evolution of sleep patterning in mammals. Funct Ecol. (2008) 22:847–53. doi: 10.1111/j.1365-2435.2008.01449.x
194. Elgar MA, Pagel MD, Harvey PH. Sleep in mammals. Anim Behav. (1988) 36:1407–19. doi: 10.1016/S0003-3472(88)80211-2
195. Savage VM, West GB. A quantitative, theoretical framework for understanding mammalian sleep. Proc Natl Acad Sci USA. (2007) 104:1051–6. doi: 10.1073/pnas.0610080104
196. Zepelin H, Siegel J, Tobler I. Mammalian sleep. In: Kryger MH, Roth T, Dement WC, editors. Principles and Practice of Sleep Medicine. Philadelphia, PA: Elsevier Saunders (2005). p. 91–100. doi: 10.1016/B0-72-160797-7/50015-X
197. Hendricks JC. Absence of shivering in the cat during paradoxical sleep without atonia. Exp Neurol. (1982) 75:700–10. doi: 10.1016/0014-4886(82)90036-X
198. Parmeggiani PL. Thermoregulation and sleep. Front Biosci J Virtual Libr. (2003) 8:s557–67. doi: 10.2741/1054
199. Else PL, Hulbert AJ. Comparison of the “mammal machine” and the “reptile machine”: energy production. Am J Physiol. (1981) 240:R3–9.
200. Hulbert AJ, Else PL. Evolution of mammalian endothermic metabolism: mitochondrial activity and cell composition. Am J Physiol. (1989) 256(1 Pt 2):R63–9.
201. Körtner G, Geiser F. The temporal organization of daily torpor and hibernation: circadian and circannual rhythms. Chronobiol Int. (2000) 17:103–28. doi: 10.1081/CBI-100101036
202. Sichieri R, Schmidek WR. Influence of ambient temperature on the sleep-wakefulness cycle in the golden hamster. Physiol Behav. (1984) 33:871–7. doi: 10.1016/0031-9384(84)90221-X
203. Walker JM, Walker LE, Harris DV, Berger RJ. Cessation of thermoregulation during REM sleep in the pocket mouse. Am J Physiol. (1983) 244:R114–8.
204. Wehr TA. A brain-warming function for REM sleep. Neurosci Biobehav Rev. (1992) 16:379–97. doi: 10.1016/S0149-7634(05)80208-8
205. Maquet P, Laureys S, Peigneux P, Fuchs S, Petiau C, Phillips C. Experience-dependent changes in cerebral activation during human REM sleep. Nat Neurosci. (2000) 3:831–6. doi: 10.1038/77744
206. Fontvieille AM, Rising R, Spraul M, Larson DE, Ravussin E. Relationship between sleep stages and metabolic rate in humans. Am J Physiol. (1994) 267(5 Pt 1):E732–7.
207. Haskell EH, Palca JW, Walker JM, Berger RJ, Heller HC. Metabolism and thermoregulation during stages of sleep in humans exposed to heat and cold. J Appl Physiol. (1981) 51:948–54. doi: 10.1152/jappl.1981.51.4.948
208. Katayose Y, Tasaki M, Ogata H, Nakata Y, Tokuyama K, Satoh M. Metabolic rate and fuel utilization during sleep assessed by whole-body indirect calorimetry. Metabolism (2009) 58:920–6. doi: 10.1016/j.metabol.2009.02.025
209. White DP, Weil JV, Zwillich CW. Metabolic rate and breathing during sleep. J Appl Physiol Bethesda Md (1985) 59:384–91.
210. Amici R, Cerri M, Ocampo-Garcés A, Baracchi F, Dentico D, Jones CA. Cold exposure and sleep in the rat: REM sleep homeostasis and body size. Sleep (2008) 31:708–15. doi: 10.1093/sleep/31.5.708
211. Muzet A, Ehrhart J, Candas V, Libert JP, Vogt JJ. REM sleep and ambient temperature in man. Int J Neurosci. (1983) 18:117–26. doi: 10.3109/00207458308985885
212. Szymusiak R, Satinoff E. Maximal REM sleep time defines a narrower thermoneutral zone than does minimal metabolic rate. Physiol Behav. (1981) 26:687–90. doi: 10.1016/0031-9384(81)90145-1
213. Valatx JL, Roussel B, Curé M. [Sleep and cerebral temperature in rat during chronic heat exposure]. Brain Res. (1973) 55:107–22. doi: 10.1016/0006-8993(73)90491-5
214. Haskell EH, Palca JW, Walker JM, Berger RJ, Heller HC. The effects of high and low ambient temperatures on human sleep stages. Electroencephalogr Clin Neurophysiol. (1981) 51:494–501. doi: 10.1016/0013-4694(81)90226-1
215. Heller HC. Temperature, thermoregulation, and sleep. In: Kryger MH, Roth T, Dement WC, editor. Principles and Practice of Sleep Medicine. Philadelphia, PA: Elsevier Saunders (2005). p. 292–304. doi: 10.1016/B0-72-160797-7/50031-8
216. Szymusiak R, Satinoff E, Schallert T, Whishaw IQ. Brief skin temperature changes towards thermoneutrality trigger REM sleep in rats. Physiol Behav. (1980) 25:305–11. doi: 10.1016/0031-9384(80)90221-8
217. Boyce R, Williams S, Adamantidis A. REM sleep and memory. Curr Opin Neurobiol. (2017) 44:167–77. doi: 10.1016/j.conb.2017.05.001
218. Boyce R, Glasgow SD, Williams S, Adamantidis A. Causal evidence for the role of REM sleep theta rhythm in contextual memory consolidation. Science (2016) 352:812–6. doi: 10.1126/science.aad5252
219. Blumberg MS. Beyond dreams: do sleep-related movements contribute to brain development? Front Neurol. (2010) 1:140. doi: 10.3389/fneur.2010.00140
220. Roffwarg HP, Muzio JN, Dement WC. Ontogenetic development of the human sleep-dream cycle. Science (1966) 152:604–19. doi: 10.1126/science.152.3722.604
221. Frank MG, Issa NP, Stryker MP. Sleep enhances plasticity in the developing visual cortex. Neuron (2001) 30:275–87. doi: 10.1016/S0896-6273(01)00279-3
222. Dumoulin Bridi MC, Aton SJ, Seibt J, Renouard L, Coleman T, Frank MG. Rapid eye movement sleep promotes cortical plasticity in the developing brain. Sci Adv. (2015) 1:e1500105. doi: 10.1126/sciadv.1500105
Keywords: hypocretin, orexin, melanin concentrating hormone (MCH), sleep function, energy conservation, resource optimization, rapid eye movement (REM) sleep, NREM sleep
Citation: Latifi B, Adamantidis A, Bassetti C and Schmidt MH (2018) Sleep-Wake Cycling and Energy Conservation: Role of Hypocretin and the Lateral Hypothalamus in Dynamic State-Dependent Resource Optimization. Front. Neurol. 9:790. doi: 10.3389/fneur.2018.00790
Received: 31 May 2018; Accepted: 31 August 2018;
Published: 05 October 2018.
Edited by:
Remi Martin-Fardon, The Scripps Research Institute, United StatesReviewed by:
Satvinder Kaur, Harvard Medical School, United StatesPablo Torterolo, Universidad de la República, Uruguay
Alexander C. Jackson, University of Connecticut, United States
Natalia Machado, Harvard Medical School, United States, contributed to the review of Satvinder Kaur
Copyright © 2018 Latifi, Adamantidis, Bassetti and Schmidt. This is an open-access article distributed under the terms of the Creative Commons Attribution License (CC BY). The use, distribution or reproduction in other forums is permitted, provided the original author(s) and the copyright owner(s) are credited and that the original publication in this journal is cited, in accordance with accepted academic practice. No use, distribution or reproduction is permitted which does not comply with these terms.
*Correspondence: Markus H. Schmidt, bWFya3VzLnNjaG1pZHRAaW5zZWwuY2g=