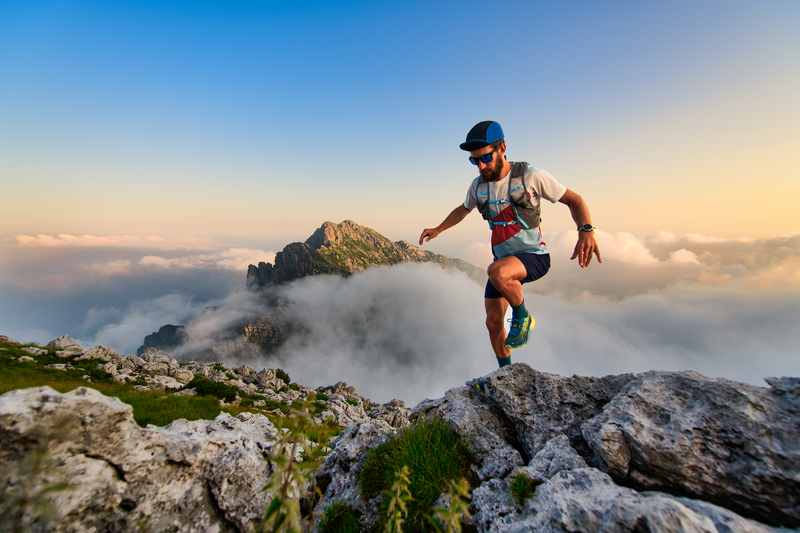
94% of researchers rate our articles as excellent or good
Learn more about the work of our research integrity team to safeguard the quality of each article we publish.
Find out more
REVIEW article
Front. Neurol. , 01 February 2018
Sec. Headache and Neurogenic Pain
Volume 9 - 2018 | https://doi.org/10.3389/fneur.2018.00019
Spreading depression (SD) is a neurophysiological phenomenon characterized by abrupt changes in intracellular ion gradients and sustained depolarization of neurons. It leads to loss of electrical activity, changes in the synaptic architecture, and an altered vascular response. Although SD is often described as a unique phenomenon with homogeneous characteristics, it may be strongly affected by the particular triggering event and by genetic background. Furthermore, SD may contribute differently to the pathogenesis of widely heterogeneous clinical conditions. Indeed, clinical disorders related to SD vary in their presentation and severity, ranging from benign headache conditions (migraine syndromes) to severely disabling events, such as cerebral ischemia, or even death in people with epilepsy. Although the characteristics and mechanisms of SD have been dissected using a variety of approaches, ranging from cells to human models, this phenomenon remains only partially understood because of its complexity and the difficulty of obtaining direct experimental data. Currently, clinical monitoring of SD is limited to patients who require neurosurgical interventions and the placement of subdural electrode strips. Significantly, SD events recorded in humans display electrophysiological features that are essentially the same as those observed in animal models. Further research using existing and new experimental models of SD may allow a better understanding of its core mechanisms, and of their differences in different clinical conditions, fostering opportunities to identify and develop targeted therapies for SD-related disorders and their worst consequences.
Transient brain dysfunctions characterize several neurological disorders, leading to episodic manifestations, e.g., headache or epileptic seizures. In most transient neurological disorders, the complex pathophysiological processes underlying the occurrence of neuronal and/or glial breakdown and subsequent functional recovery are largely unknown; however, the spreading depression (SD) phenomenon is undoubtedly one of the main ones and seems to play a crucial role in several conditions, primarily ischemia, seizures, migraine, and migraine variants.
The term “spreading depression” indicates slowly propagating changes in neuronal electrical potentials, coinciding with or leading to a silencing of brain electrical activity. The phenomenon self-propagates as a wave in the gray matter by means of contiguity, regardless of functional divisions or arterial territories (1). The first description of SD appeared in 1944 in a study showing a spreading decrease of excitability in experimental epilepsy (2). Numerous studies have further investigated this phenomenon in normal and pathological conditions, with the aim of reaching a better understanding of its involvement in clinical conditions like migraine, ischemic and traumatic brain injury (TBI), transient global amnesia, and epilepsy. However, it remains incompletely understood due to the complexity of synaptic physiology and the difficulty in obtaining direct experimental data. Moreover, it is still debated whether the spreading phenomenon should more properly be considered to refer to the depression or to the depolarization, even though the two propagates in the tissue together (3–5). Some authors use “spreading depolarization” as a generic term indicating the biophysical mechanism underlying the progressive, self-propagating, and ultimately near-complete neuronal depolarization (6), and “spreading depression” to refer to the silencing of brain electrical activity, considered a consequence or epiphenomenon of the spreading depolarization. Others, instead, use the terms “spreading depression” and “spreading depolarization” according to the presence, respectively, of normal or impaired local metabolic conditions when the phenomenon occurs (7, 8). Differences in terminology arise from the evidence that there exist different types of propagating depolarization characterized by heterogeneous molecular signatures. Indeed, pharmacological inhibition of the presumed molecular pathways gives different results according to the initial metabolic condition (8). The purpose of this review is to explain why these phenomena may be regarded as the pathophysiological correlates of different disorders, and from this perspective we consider the first definition less confusing. Therefore, in the present review, the term SD is taken to refer to both spreading phenomena: the propagating depolarization, understood as the initial phenomenon, and the SD proper, understood as its ultimate consequence or epiphenomenon.
Neurons use electrochemical energy to drive signaling in the brain. They store this energy in the form of ion gradients across the cell membrane, and the main ions involved in neuronal excitability are sodium, potassium, and chloride. An electrical signal in a neuron requires only a small amount of this energy (9). During an action potential, the flux of very few ions through specific membrane channels causes the membrane potential to rise and fall within the space in a millisecond (10). The activity of the Na/K-ATPase pump is crucial in restoring ion homeostasis. The brain consumes about 20% of the body’s basal energy (11) and Na/K pumps use about half of this 20% share in maintaining ionic gradients.
Glial cells also play a critical role, serving to buffer K+ ions in the extracellular space and absorb the glutamate released from excitatory synapses, thereby avoiding excitotoxicity reactions. However, when the concentration of these molecules exceeds a certain threshold, neuronal and glial transporters can no longer cope with the efflux. This results in massive extracellular accumulation of potassium and glutamate and in large cell depolarization accompanied by loss of membrane resistance and large shifts in the intra- and extracellular ion concentrations, preventing the generation of action potentials (12).
At the onset of an action potential, the membrane potential rises rapidly because of the opening of sodium channels, which temporarily generates an inward current that is not balanced by outward currents. By contrast, the slow temporal evolution of the depolarization typical of SD is due to a more gradual modification of the resting membrane potential, mediated by changes in intracellular and extracellular ion concentrations. Extracellular K+ concentrations, in particular, seem to be decisive in triggering and propagating the SD phenomenon. In physiological conditions, extracellular concentrations of K+ are relatively low, and the extracellular space is relatively small. K+ concentrations can increase rapidly by means of transmembrane fluxes (6, 7). Since the electroneutrality between the two sides of the cell membrane needs to be preserved and the membrane has a limited capacitance, changes in ion concentrations and sustained depolarization cannot result from a single ionic current but are mediated by sets of opposing currents. It is necessary to bear this concept in mind when seeking to interpret data from experimental studies aimed at blocking specific currents and investigating those involved in the SD phenomenon. SD is a process resting on breakdown of ion homeostasis that occurs when passive cation influx across cell membranes exceeds ATP-dependent Na+ and Ca2+ pump capacity (13). The massive discharge of the membrane potential seen in SD creates a signature shift of the extracellular potential that can be as great as −30 mV. This potential shift has a slow onset (many seconds), and it is difficult to detect with traditional high-pass AC amplifiers. To observe it, an unfiltered direct-coupled (DC) amplification is required; for this reason, it has classically been referred to as the DC shift (14) (Figure 1).
Figure 1. Full-band (DC-coupled) recordings of spreading depression. Two events propagate across the full electrode strip from electrodes 6 to 1, as shown by negative DC shifts and 0.5–50 Hz depressions of spontaneous activity. Analysis focused on electrode 3 in monopolar mode (electrode 3 versus reference, gold). Adapted from Ref. (14).
The propagating depolarization phenomenon results in a reduction of electrical activity that shows up as a marked negative slow potential change (4, 15, 16). This change may result from longitudinal gradients of depolarization along neurons (6). In fact, neurons do not seem to be inactivated along their entire anatomy during the massive cell depolarization; instead they maintain their integrity and electrical function, except in the dendritic zone where ion channel opening allows large sustained influxes of small cations, such as sodium and calcium (6). Intracellular potential gradients, from zero to rest, can be supported by a combination of shunted membranes and ion redistribution along discrete cell subregions.
The suppression of electrical activity in SD rests on reduction of synaptic currents that, in turn, reduces neuronal energy requirements. The cause of this reduction seems to be presynaptic. Synaptic failure is induced by high extracellular levels of adenosine, a breakdown product of ATP, which prevents the vesicular release of glutamate. Adenosine levels may rise as a result of increased ATP consumption (17, 18). Electrical activity remains suppressed for several minutes after repolarization, and neurons do not generate action potentials upon application of glutamate during this period (18). Recent experiments demonstrated that the large reduction in action potential firing observed after SD could be due to a shift in the excitation/inhibition ratio toward inhibition. They showed that reduced action potential firing, like post-synaptic potential amplitude changes, lasted at least an hour after the depolarizing event (19).
Neurons have been found to show a morphological change during SD. Authors describe neuronal swelling and a transitory change in dendritic structures (“beading”) with an apparent loss of many dendritic spines (20). Extracellular volume decreases from 20 to ~5% because of the water influx due to ion changes, leading to intracellular hyperosmolality (21–24). The change in morphology of most dendrites is reversible and neuronal cell bodies were found to regain their previous volumes within 8–10 min after SD (20, 25–27). Moreover neurotransmitters, such as acetylcholine, γ-aminobutyric acid (GABA), and glutamate, are released in large amounts (28). Among these, GABA is particularly interesting as it can play two roles; indeed, GABAA receptors contribute to limit the propagation rate (29), but may also facilitate cell swelling through chloride entry (30, 31). Indeed, blockade of Cl-coupled transporters leads to a significant reduction in dendritic beading without interfering with SD (32).
The depolarization propagates through the gray matter like a wave, typically spreading at a rate of 2–6 mm/min (15). Rather in the manner of an action potential, the depolarization wave, once triggered, propagates in an all-or-none fashion, regardless of the stimulus type or intensity. The exact propagation mechanism is not yet completely understood, even though some hypotheses have been advanced (33). Non-mutually exclusive mechanisms involved in the propagation may rely on the diffusion of extracellular potassium or glutamate, or alternatively on the opening of neuronal or glial gap junctions (12). However, the hypothesis of propagation based on regenerative glutamate release via N-methyl-d-aspartate receptor activation (28) runs into the problem that indirect calcium release from mitochondria seems insufficient to drive this process—depolarization in naive tissues has been found to be blocked by removal of extracellular calcium or inhibition of voltage-gated calcium channels (34–36)—while a transcellular pathway for diffusion via neuronal gap junctions (37) also seems highly unlikely, given that pyramidal cells display gap junctions only in early brain development (38), while in adult animals these junctions are present only between interneurons (39).
Under normal oxygen conditions, electrical activity returns to its normal state within a few minutes after tissue depolarization, and then ion homeostasis is restored. Several mechanisms are involved in this tissue recovery: the Na/K-pump is activated as a result of increased intracellular sodium and extracellular potassium levels (40–42); repolarization of the neuronal membrane potential closes the voltage-gated channels, decreasing the potassium efflux and thereby allowing the pump to restore physiological ion concentrations. The repolarization is also promoted by the glial buffering of extracellular potassium in the extracellular space (43, 44). Under ischemic conditions, the compensatory action of astrocytes is hindered because astrocytic Na/K-ATPases lack ATP. Consequently, intra-astrocytic sodium rises (45), and potassium is spilled out instead of taken up (46). Loss of astrocytic function may severely limit neuronal survival under ischemia (47). SD is due, primarily, to disruption of neurons, while astrocytes remain functional and support neuronal recovery. In this process, changes in intracellular calcium, which rises first in neurons, then in astrocytes, suggest that neurons lead and astrocytes follow (48). Moreover, SD and the associated neuronal calcium wave remain unaffected when the astrocytic calcium wave is blocked by the depletion of internal calcium stores (35). However, SD involves a complex interplay between the activities of neurons, glia, and blood vessels. Interestingly, one study found that inhibition of astrocyte signaling did not abolish SD, although the changes in vascular caliber normally associated with SD were absent, suggesting that astrocytes play a key role in mediating the vascular response to the SD phenomenon, but not necessarily its propagation (48). Moreover, astrocytes swell markedly under ischemic conditions (26). Recent optogenetic studies have shown that SD can be elicited by specific stimulation of astrocytes. The finding that light stimulation of mice expressing a light-activated channel specific for astrocytes resulted in sustained depolarization of astrocytes and eventually elicited SD further adds to the evidence that astrocytes could have a primary role in SD initiation (49).
In healthy tissues, increased electrical activity is coupled with the release of vasodilator factors, such as nitric oxide (NO) and arachidonic acid metabolites, which increase local blood flow to meet increased energy expenditure (50). This system, known as “neurovascular coupling,” involves neurons, astrocytes, and arterioles (48, 51–53). In damaged tissues, on the other hand, the restorative vascular response is absent, and indeed there is a vasoconstrictor response (1, 13).
In the presence of extensive disruptions, such as SD, the vessel response is strongly nonlinear. While moderate increases in extracellular potassium cause vasodilation, stronger increases induce vasoconstriction (53). The neurovascular response to SD typically shows a triphasic pattern (constriction, dilation, and then prolonged slight constriction), but it differs greatly between species and conditions, ranging from pure constriction to pure dilation (54).
Experimentally, SD can be induced by various stimuli, including intense electrical stimulation, mechanical damage (needle prick), and administration of K+ or glutamate (55). Ultimately, these stimuli lead to glutamate-induced toxicity (21). Glutamate activates calcium and sodium channels, particularly the NMDA receptor channel, in a pathologic manner, causing the membrane potential to change from approximately −70 to −10 mV (13). Triggers of SD fall into two categories: those that depolarize neurons through sodium and/or calcium channel activation, and those that depolarize neurons indirectly via Na/K-ATPase activity reduction (7). Stimuli belonging to the first group include ictal epileptic events, administration of glutamate or potassium, and administration of neurotoxins, e.g., veratridine. The second group includes conditions associated with ATP depletion, such as ischemia, hypoxia and hypoglycemia, drugs such as ouabain or palytoxin, and brain topical superfusion of the vasoconstrictor endothelin-1 (56).
Spreading depression induced using transcranial stimulation of channelrhodopsin-2 ion channels was recently found to be similar to the SD changes evoked with KCl (57). Since different mechanisms of induction lead to different pharmacological effects, it is important to understand which experimental methods of SD induction are most relevant to specific human conditions (8).
Gene mutations can decrease the threshold for the SD, by leading to spontaneous excessive localized increases in K+ or glutamate in some individuals. A genetic contribution to this phenomenon has emerged through study of, for example, mutations in the hemiplegic migraine-associated genes (58–62). Different genetically modified mice, characterized by astrocyte-directed inactivation of Cx43, showed a similar increased propensity to SD (63), since functional gap junctions are involved in spatial potassium buffering. Studies indicate that the propensity to SD in rodent models could also be related to gender (64), with female mice showing a lower threshold for induction of SD compared with their male counterparts (59, 65). The influence of sex hormones in this process is reported in different studies: SD susceptibility was found to increase in the presence of estrogen, whereas exposure to testosterone had the opposite effect (59, 66). This important sex-related effect might be due to the sexual dimorfism of chloride regulation as it has been shown that the main cotransporter responsible for chloride extrusion, KCC2, is downregulated by sex hormones in certain brain areas leading to less effective inhibition (67, 68).
A few studies have recently investigated the occurrence of SD as a characteristic of disease states in humans. The depolarizing phenomenon in SD is not substantially assessable by scalp EEG due to the filtering effect of the scalp, dura, and bone. Scalp EEG allows the detection of low amplitude and high frequency changes, like those seen during seizures, but not the slower, although higher amplitude, changes characteristic of SD (13). However, an attempt to find the scalp correlate of SD has been made through scalp EEG performed simultaneously with cortical surface recording using electrocorticography strips (69). Clinical monitoring of SD is currently limited to patients who need neurosurgical interventions necessitating the placement of subdural electrode strips (14, 69). SD events, recorded in humans, have electrophysiological features that are essentially the same as those observed in animal models. Furthermore, measurement of regional cerebral blood flow in migraine patients has revealed spreading oligemia whose scale and timing are similar to what is observed in SD (70).
Migraine is a common disorder characterized by recurrent attacks of severe, usually unilateral, headache accompanied by other symptoms (nausea and/or vomiting, photophobia and/or phonophobia). It is one of the most prevalent neurological disorders, affecting on average more than 15–20% of the population and showing a female:male ratio of 3:1. There are several subtypes of migraine; the two major ones are migraine with aura and migraine without aura (71). The migraine aura is a complex of neurological signs and symptoms that typically precedes headache onset by 20 min, and lasts 5–60 min before disappearing. More rarely, however, aura symptoms can accompany the headache for hours or even days. Auras consist mainly of transient visual, sensory, or language disturbances. Visual symptoms are the most common, and typically consist of unformed flashes of light moving across the visual field. Progression of the aura in migraine shows the typical spatial and temporal features of experimentally induced cortical SD in animals (56, 72). Moreover, waves of alterations in cortical activity and blood flow have been captured through functional imaging studies and found to show SD-like features both in migraineurs with and in those without aura (73–75). However, the link between SD and migraine without aura is still poorly understood, and it is not yet clear whether the SD runs over silent areas of the cortex, for example, subcortical regions (e.g., the hippocampus) (63), or whether, instead, it fails to reach the clinical threshold (76). Hemodynamic data are also conflicting, since some studies have shown no changes in cerebral blood flow during migraine attacks without aura, by contrast with the post-SD oligemia observed during auras in other research (77).
Cortical SD is now widely assumed to be the electrophysiological mechanism of migraine aura, and a large body of evidence supports this assumption. SD is the first endogenous event identified upstream to trigeminovascular activation leading to pain and blood flow changes. Although speculative, SD would emerge as a common pathophysiological mechanism causing pain and local autonomic changes, as well as hemodynamic patterns similar to migraine headaches in humans (78). A link between headache localization and the neurobiology of aura is provided by SD-induced activation and sensitization of neuropeptide-containing trigeminal peripheral nociceptors innervating ipsilateral cranial tissues (8). A drastic depolarization, as those occurring during cortical SD, will release noxious molecules such as different ions, i.e., H+ and K+, or NO, in the neocortical extracellular space (76). The rise in extracellular K+ during SD is thought to depolarize and excite the nociceptive fibers in the ophthalmic division of the trigeminal nerve enveloping the pial arteries, resulting in release, from the primary meningeal afferents, of pro-inflammatory peptides, i.e., calcitonin gene-related peptide and substance P (CGRP), that induce vasodilation and plasma protein extravasation, thus triggering the headache (77, 79, 80).
This theory has been confirmed by the increased pro-inflammatory peptide levels seen in patients with migraine attacks (81) as well as in electrically stimulated rats (82). Repetitive episodes of SD, moreover, have been found to induce inflammatory cascades that resulted in the production of cyclooxygenase 2 and inducible NO synthase, and in the activation of microglia (82, 83). The clearance of extracellular macromolecules is thought to be mediated mainly by the glymphatic pathway, a system consisting of astroglial cells forming series of perivascular compartments that clear waste products from the brain (84). Significantly (from the perspective of the migraine pathomechanism), it has recently been demonstrated that SD impairs glymphatic flow, thus interfering with the clearance of excitatory and inflammatory chemicals and might foster localized cortical hyperexcitability and structural thickening (85). SD, therefore, by supporting inflammatory responses and the consequent activation and sensitization of meningeal trigeminal afferents, seems to play a strong role in headache pathophysiology. A mutually causative link between inflammation and SD is suggested by the recent observation that maternal inflammation interferes with chloride regulation of the off-springs, delaying the switching from depolarizing (high intracellular Cl) to hyperpolarizing GABA (86) (low intracellular Cl). If the connection between inflammation and chloride control was established also in adults, SD and inflammation might constitute a self-supporting system that may lead to a gradual worsening of the condition.
The exact mechanisms of brain dysfunction leading to the onset of a migraine attack, as well as the determinants affecting individual susceptibility to SD in the human brain, remain unknown. Genetic factors predisposing to migraine with aura also enhance susceptibility to SD (87). Most studies exploring these aspects involve transgenic mice expressing mutations associated with the different forms of familial hemiplegic migraine (FHM), or with cerebral autosomal dominant arteriopathy with subcortical infarcts and leukoencephalopathy (88, 89). Migraine is multifactorial and its rare monogenic forms (i.e., FHMs) phenocopy most or all the clinical features of the classic form of the disease, although they typically show a longer duration of auras as well as the presence of motor features (90). Three main FHM genes have been identified: CACNA1A (FHM1) encoding the alpha subunit of the neuronal voltage-gated Ca2+ channel (Cav2.1) (91), the ATP1A2 gene (FHM2) encoding the alpha-2 subunit of a Na+/K+ pump (61), and the SCN1A gene (FHM3) whose mutations result in defects of the alpha-1 pore-forming subunit of the neuronal voltage-gated Na+ channel (Nav1.1) (92).
Acetazolamide, used to treat headaches associated with FHM, has been shown to decrease the frequency of attacks, and its effectiveness has been suggested to be due to improvements in ion channel function (93, 94). In the central nervous system, acetazolamide penetrates the blood–brain barrier slowly and causes carbonic acidosis through carbonic anhydrase inhibition (95); this acidosis may also serve to selectively reduce the buffering of rapid pH changes occurring in SD. Indeed, at the onset of SD, there is a brief extracellular alkaline shift (96) that transiently promotes neuronal injury by eliminating the proton block of NMDA receptors (97). Transgenic mice expressing either FHM1 or FHM2 mutations show both enhanced susceptibility to SD and altered synaptic transmission, thus highlighting possible pathophysiological mechanisms underlying FHM (98). However, whether these mutations also predispose to typical migraine remains poorly understood (99). Finally, there is evidence to suggest that sex hormones may modulate SD susceptibility in humans (100), as well as in FHM animal models (66). Sex hormones may enhance susceptibility to SD in FHM1 female mice, and this increased susceptibility may recover after oophorectomy or estradiol treatment (59, 66). These findings may, in part, explain the increased prevalence of migraine in females and its variability across the individual life span.
Different classes of prophylactic drugs for migraine, such as antiepileptic drugs (topiramate, valproate, and lamotrigine), as well as propranolol, amitriptyline and methysergide, are able to suppress SD, through different mechanisms of action (101), and they are equally efficacious both in forms with and without aura (102). Lamotrigine, in particular, targeting Ca2+ and Na2+ channels and glutamatergic or GABAergic transmission (mechanisms common to most antiepileptic drugs), has been shown to be effective in the prevention of migraine aura possibly through an inhibitory effect on SD and a consequent suppressive effect on the aura phenomenon (103, 104). Furthermore tonabersat, a relatively novel benzopyran derivative known to inhibit gap-junction communication between neurons and satellite glial cells in the trigeminal ganglion (105) and to markedly reduce SD-associated events in preclinical studies (106) has been proposed in migraine prophylaxis and to relieve headache in migraineurs (107). However, results of randomized, double-blind, placebo-controlled studies showed benefit on attacks of migraine aura but no effects in reducing migraine pain days when chronically administered to patients (108). Results in acute studies are conflicting.
Administration of the serotonin precursor 5-hydroxytryptophan in animal models has also been shown to decrease the frequency of KCl-induced SD in female rats, suggesting a possible role for serotonergic agents as therapeutic tools in migraine with aura in females (109). Blocking the SD mechanism, therefore, seems to be a rational approach to explore in molecular research aimed at improving the prevention and treatment of migraine with aura. However, the evidence that different symptoms of migraine may already be present at the time the visual aura occurs, and the fact that aura can be painless in some migraineurs, confirm that the real role of SD in migraine is still to be entirely clarified.
New approaches to migraine treatment were recently developed adopting fremanezumab, a humanized monoclonal antibody (CGRP-mAb) able to reduce the availability of CGRP. Fremanezumab pre-treatments prevents headache by selectively inhibiting the responsiveness of Aδ neurons (peripherally) and high threshold neurons (centrally), but not C-fiber neurons, as reflected in a decreased percentage of neurons showing activation by SD. The selective inhibition of fremanezumab on meningeal nociceptors might explain why a CGRP-mAb may not be effective in all migraineurs (110). Another CGRP receptor antagonist, MK-8825, dose dependently seems to attenuate SD-induced trigeminal nerve mediated pain response but without altering SD waves (111).
It is worth noting that the International Classification of Headache Disorders, third version (71) lists features for other less frequent migraine disorders, such as status migrainosus, persistent aura without infarction, and migrainous infarction (71). The aforementioned SD mechanisms may play a significant role in these conditions, as demonstrated specifically in migrainous infarction, a condition that may lie on a continuum with cerebral ischemia (see below) (112–114). It has been shown that FHM1 mutations may share genetic determinants of migraine with aura and stroke; indeed, these mutations, typically associated with migraine with aura, increase stroke vulnerability and also accelerate stroke evolution (115) by enhancing the susceptibility to ischemic depolarizations, a process akin to SD. In addition, in a subset of migraineurs, the presence of small microemboli could trigger SD and thus possibly be a stimulating mechanism for aura even in the absence of tissue injury (116).
The term ischemia (or stroke) refers to a decrease in blood supply to a tissue or organ. In focal cerebral ischemia, in particular, cerebral blood flow reduction is confined to a single anatomic area of the brain (117, 118), and has a number of effects on the surrounding nervous tissue, including excitotoxicity, oxidative stress, and apoptosis (119). In the early phase of ischemia, tissue damage is primarily related to direct metabolic failure caused by the decreased blood flow (120); this leads to impairment of ionic pumps (7), which in turn results in ionic imbalance and accumulation of cytosolic sodium and calcium ions and extracellular potassium (121), causing water influx into cells, tissue swelling, and permanent depolarization of cell membranes. Immediately after the acute phase, the damage spreads, over several hours, to the adjacent areas, or ischemic penumbra (122), i.e., to a wider area of tissue that also becomes severely compromised by the blood flow reduction (123). This process is driven by a plethora of electrochemical events, so-called peri-infarct depolarizations (PIDs) that produce multiple waves of cortical SD-like activity and are fundamental mechanisms in the continuous elicitation of the biochemical cascade of cell injury. While SD is not directly linked to cell death or damage in a normally perfused brain, recurrent PIDs in an ischemic brain are associated with enlargement of the infarcted volume (13, 124, 125).
The features PIDs are similar to those of SD, with extracellular DC shifts of 20 mV, a propagation velocity of 3–5 mm/min, and disruption of the cellular ion balance. In all animal studies of focal ischemia, the vascular responses of ischemic tissue and adjacent areas were seen to vary considerably, depending on distance from the ischemic focus (126–128). In more severely ischemic areas PIDs mainly cause monophasic hypoperfusion; instead, where ischemia is milder, the response becomes biphasic, with an initial hypoperfusion followed by peak hyperemia. In non-ischemic adjacent areas, PIDs cause only hyperemia, and there is no hypoperfusion (129, 130). NO may play a role (129, 131), since the reduction in O2 availability, due to the ischemic condition, may in fact reduce NO synthase. This would result in a diminished NO-dependent vasodilation. Another factor is the extracellular concentration of K+ that, in the injured brain, is usually higher than normal. High concentrations of K+ are linked to a stronger vasoconstriction (132).
Spreading depression has also been observed in the brains of patients affected by cerebral ischemia, and in these subject PIDs, similarly to those measured in animals and associated with different hemodynamic responses, such as hyperemia, biphasic response, or hypoperfusion, have been linked to increase of the infarcted volume (133, 134).
Taken together, animal models and human studies show that SD-like depolarizations, i.e., PIDs, may represent a core mechanism for stroke pathophysiology, clearly related to the expansion of the infarcted volume and the spread of ischemia (83).
About 10–20% of all cases of stroke, however, result from subarachnoid hemorrhage, usually originating from the rupture of an aneurysm (135). After the occurrence of a subarachnoid hemorrhage, delayed ischemia affects the injured brain and can cause fatal damage or permanent injury (136). SD may play a pivotal role in this delayed cerebral ischemia and contribute to its diffusion (137). Although SD has been observed in animal models of brain hemorrhage, its precise role has never been demonstrated in a clear and reproducible manner, and therefore it remains debated (138, 139). For example, local superfusion of hemoglobin in rat brains, together with elevated concentrations of extracellular K+, induces SD with a monophasic vasoconstriction response. Hemoglobin is indeed thought to reduce NO concentration, by acting as a scavenger (140). Moreover, the superfusion of hemoglobin or NO synthase inhibitors, with the addition of either endothelin-1 or a small amount of glucose, is sufficient to produce SD and the spreading of ischemia (141).
Spreading depression after subarachnoid hemorrhage has also been observed in humans, accompanied by an increase in the infarcted tissue volume and delayed ischemia (16). In subarachnoid hemorrhage, as in ischemia, SD and PIDs may be worsening factors, given their detrimental impact on already damaged brain tissue. They have a dual mechanism of action: increasing metabolic activity and reducing blood supply.
In recent clinical studies, SD has emerged as a potent pathomechanism of the progression of secondary injury in TBI (142–144). The incidence of SD among TBI patients is reported to be around 50–70%, and SD often occurs in repetitive patterns over a period of at least 7 days post-trauma (143, 144). Although the probability of SD is theoretically increased as a result of lower levels of cerebral perfusion and high systemic temperature, which lead to an increased mismatch between energy supply and demand (145), the vast majority of SD in TBI occurs when the systemic variables are in the normal range (142). In post-TBI patients, who underwent craniectomy, the EEG was able to track 40% of depolarizations, recorded simultaneously with cortical electrodes (144). Following TBI, increased glutamate levels trigger SD (146–148). In addition, astrocyte activity increases after TBI (149) while the expression of GLT-1, a glutamate transporter, is reduced (146). It remains unknown how an acute cortical lesion might, in relation to the site of the injury, affect the site of initiation and subsequent propagation of SD. The cortical injury site is not capable of initiating SD, and the depolarization takes place in the surrounding peri-injury zone. Instead, the injury zone has been found to act as an attractor, having the ability to support SD initiated in nearby, less compromised networks (150).
In addition to its possible roles in migraine and cerebral ischemia, subarachnoid hemorrhage, and TBI, SD has been also related to epileptiform activity (13, 56, 151–153). SD, as we have seen, is a disruption of electrical activity caused by a strong ion concentration change in the extracellular space. The impaired ion homeostasis associated with SD triggers a cascade of cellular events leading to toxic release of glutamate (21). From this perspective, excitotoxic states emerge as a mechanism common to both SD and seizures, in the former caused by neuronal activity depression, and in the latter by neuronal hyperactivation.
Because of this shared background, there exists a complex and as yet poorly understood interrelation between epileptic activity and SD. Several studies have shown that seizures can either potentiate or limit SD waves, depending on whether they occur before, during or after the SD event. Some authors, examining brain slices from individuals with epilepsy, showed that their tissue was highly susceptible to generating SD waves (154). Similarly, studies of animal models of seizures, induced pharmacologically, have shown an increase in SD wave susceptibility after seizure events (155, 156). The depression of neuronal electrical activity caused by depolarizing waves may be the cause of the post-ictal depression state typically seen in epilepsy. Multiple SD waves have been shown to occur during post-ictal periods, providing a possible explanation for the observed slow post-seizure cognitive recovery (155). Looking at the question from the opposite perspective, SD may create an environment conducive to the onset of epileptiform activity. Both mouse and human experiments have shown states of hyperexcitability following a cortical depression period, akin to that seen prior to epileptic seizures (157–159). An explanation for this may be related to the neuronal swelling that occurs following SD waves, which may increase neuronal excitability through activation of NMDA receptor-dependent inward currents (160). Furthermore, a change in the pattern of neurotransmitter receptor distribution has been noticed after cortical SD events, and this may in turn imply a lowering of the seizure threshold (161). Contrary to this potentiation effect, SD is also capable of interrupting a seizure, even leading to refractory periods of seizure silencing in rats (155, 162, 163). Conversely, stimulation-induced recurrent epileptiform discharges have been shown to block cortical and subcortical SD in rats (164). These findings do not allow a single interpretation of the interplay between SD and epilepsy, but they strongly suggest that there is a common mechanism in which a pivotal role may be played by NMDA receptors (165).
It has also been suggested that the process of SD could underlie failure of the brain to recover following a seizure (166). Seizures are typically accompanied by transient alterations in cardiac and respiratory rates, but these events are generally reversible as soon as the seizure ends. However, SUDEP, a condition where death is caused by seizure-induced cardiorespiratory collapse, is among the leading causes of mortality associated with epilepsy-related disorders. Although it accounts for about 17% of epilepsy-related deaths (167) (~50% among cases of refractory epilepsy) (168, 169), there is still no consistent and strong body of literature on the topic, containing comparable data linking SUDEP with its risk factors. This knowledge gap is probably attributable largely to the great heterogeneity of research approaches, the multifactorial nature of SUDEP, and also the impracticality of following a large number of individuals with epilepsy from diagnosis to death. Furthermore, investigation of SUDEP is also complicated by the fact that the exact mechanism underlying this phenomenon remains to be fully elucidated.
Studies both in humans and in mice (170–172) have suggested that a genetic misregulation of brain centers controlling autonomic functions, resulting in alterations in membrane excitability or synaptic activity in pathways that control heart rate or agonal respiration, is the basis of increased risk of SUDEP (173, 174). Moreover, several mutations in genes linked to increased SUDEP susceptibility have been demonstrated to be directly linked to a lower threshold for SD in mice (170, 171). Against this background, Aiba and Noebels (166) have proposed that the SD phenomenon is the causative process leading to cardiac and respiratory shutdown in susceptible individuals (175). If triggered in the brainstem, the depolarizing electrical wave may diffuse across cardiorespiratory centers in the medulla, leading to complete arrest of pace-making neuronal centers, hindering recovery of heartbeat and normal respiration after a seizure and leading to death (166). In the same study, cortical seizures, obtained by cortical application of 4-aminopyridine, were found to be capable of causing a slowly spreading negative DC potential which also can diffuse down to the dorsal medulla.
Two relatively recent genetic mouse models, Kv1.1 potassium channel and Scn1a sodium channel KO animals, which replicate many aspects of human SUDEP, have provided strong indications that SD acts as an intermediary between a severe seizure event and consequent cardiorespiratory failure leading to sudden death. Functional alterations of these genes impair neuronal excitability through different membrane and synaptic mechanisms (166, 174, 176). Ablation of the Kv1.1 gene has a strong effect on both excitatory and inhibitory neurons, whereas lack of the Scn1a channel reduces forebrain interneuron excitability (177–180). In both cases, the result is disruption of the excitatory/inhibitory equilibrium and a dramatic lowering of the threshold for triggering SD waves. As a consequence, whereas these animals recover normally if seizures remain below this threshold, severe seizures can trigger an SD event, leading to death within minutes (166, 176). This mechanistic interpretation of SUDEP may explain why epileptic individuals with seizure-induced cardiorespiratory arrest may fail to be revived using conventional cardiopulmonary resuscitation procedures, and suggests a possible therapeutic target for this fatal complication of epilepsy.
In the past few years, the main hindrance to the study of SD was the difficulty in detecting depolarization wave non-invasively in the human brain (181). On the other side evidence associates SD to a plethora of phenomena, from relatively benign migraine aura attacks to severe brain injuries, such as ischemic strokes, subarachnoid or intracerebral hemorrhage, and SUDEP. This heterogeneous presentation increases the interest on SD as a pathological mechanism of great importance for clinical neuroscience. The “dark side” of this process is that it is hard to explain the heterogeneity of SD and to predict ab initio its severity. As already speculated by others, the mechanisms underlying the different clinical outcomes of SD can depend on several factors. One of these is the specific brain “status” based on the patient’s vascular and metabolic conditions, an as yet vague genetic predisposition, and eventually preexistent pathological conditions (13, 50). For instance SD is a relatively benign phenomenon in migraineurs because it occurs in normal human brains with preserved perfusion and energy metabolism. Conversely, in injured brain with compromised energy metabolism and perfusion, one could hypothesize that SD might contribute to further brain damage, such as expansions of brain infarcts, and could impair clinical recovery, or trigger new deficits. Yet, other factors need to be determined in the frequency, properties, and impact of SD, including the role of chronic medications and variations in plasma osmolality, and serum levels of electrolytes and glucose (147). In sight of these speculations, we need further understanding on how perturbed tissue will affect the expression and the consequences of SD.
The ambiguous interplay between SD and the various related clinical phenotypes precludes univocal identification of its precise role in brain disease. Even though it is often considered a single phenomenon with homogeneous characteristics—its signature features being slowly propagating changes in neuronal electrical potentials and silencing of brain electrical activity, together with neuronal swelling and distortion of dendritic spines (147)—SD shows some heterogeneity in its cellular and molecular mechanisms (8), and appears to play different roles in different clinical disorders (as an event-triggering process, secondary effect, or simple epiphenomenon) (Figure 2). Further advances in our knowledge of this phenomenon are necessary in order to improve our understanding of these different conditions, since better disentangling of the underlying processes may also foster the development of targeted interventions able to prevent their most dramatic consequences.
Figure 2. Schematic diagram summarizing the complex mechanisms underlying the spreading depression (SD) phenomenon at the tripartite synapse. High extracellular K+ concentration causes an increase of neuronal excitability and a high level of extracellular Glu. Increased electrical activity leads to release of vasodilator factors with a consequent increase in blood flow. ATPase activity is insufficient to maintain ion homeostasis and leads to high level of adenosine which, in turn, prevents vesicular release of Glu at presynaptic level. A change in dendritic structures and the loss of dendritic spines occur («beading»). The astrocyte-mediated buffering of K+ and Glu is inadequate to quickly restore the electrochemical homeostasis, and thus sustains the SD mechanism.
However, as well as studying the specific mechanisms characterizing the different disorders, it is still necessary and may well be useful to focus, also, on the common features of SD in the various conditions. A number of models of genetically determined migraines (like FHMs) or epilepsy, such as Kv1.1 potassium channel and Scn1a−/− animals (177–180), are already widely available and may serve as useful, paradigmatic tools able to disclose the core mechanisms of the SD phenomenon, common to the different clinical disorders. This might help to identify new treatment targets, possibly making it possible to overcome the traditional, only partially efficacious, therapeutic approaches, i.e., with classic antimigraine or antiepileptic drugs, and open new perspectives for treating these disorders or preventing their worst consequences.
Despite the development of several animal models for the study of SD, many questions remain open as a result of its complexity and the difficulty of obtaining direct experimental data in humans. In addition to more detailed characterization of existing or new models of spontaneous SD, which may be useful for dissecting and clarifying the pathomechanisms in animals, we need to continue developing non-invasive technologies (182) that might allow us to gather additional information in humans, since, for the moment, subdural strips continue to be the main means of monitoring SD in this setting.
All the authors have contributed substantially to the writing and revising of the manuscript. OC, MM, FT, FS, and FMS participated in the conception and design of the work, collected the literature, prepared the figures, and wrote the manuscript. EP, GR, and MB reviewed and edited the manuscript, and approved the final version.
The authors declare that the research was conducted in the absence of any commercial or financial relationships that could be construed as a potential conflict of interest.
We also thank Catherine J. Wrenn for providing expert editing.
Research in our laboratories is partially supported by grants from the Italian Ministry of Health (Ricerca Corrente to FMS) and Telethon-Italy (http://www.telethon.it/en; Grant no. GGP11188 to FS).
1. Offenhauser N, Windmüller O, Strong AJ, Fuhr S, Dreier JP. The gamut of blood flow responses coupled to spreading depolarization in rat and human brain: from hyperemia to prolonged ischemia. Acta Neurochir Suppl (2011) 110:119–24. doi:10.1007/978-3-7091-0353-1_21
2. Leão AAP. Spreading depression of activity in the cerebral cortex. J Neurophysiol (1944) 7. Available from: http://jn.physiology.org/content/7/6/359
3. Kager H, Wadman WJ, Somjen GG. Conditions for the triggering of spreading depression studied with computer simulations. J Neurophysiol (2002) 88:2700–12. doi:10.1152/jn.00237.2002
4. Hossmann K-A. Viability thresholds and the penumbra of focal ischemia. Ann Neurol (1994) 36:557–65. doi:10.1002/ana.410360404
5. Dreier JP, Ebert N, Priller J, Megow D, Lindauer U, Klee R, et al. Products of hemolysis in the subarachnoid space inducing spreading ischemia in the cortex and focal necrosis in rats: a model for delayed ischemic neurological deficits after subarachnoid hemorrhage? J Neurosurg (2000) 93:658–66. doi:10.3171/jns.2000.93.4.0658
6. Canals S, Makarova I, López-Aguado L, Largo C, Ibarz JM, Herreras O. Longitudinal depolarization gradients along the somatodendritic axis of CA1 pyramidal cells: a novel feature of spreading depression. J Neurophysiol (2005) 94:943–51. doi:10.1152/jn.01145.2004
7. Somjen GG. Mechanisms of spreading depression and hypoxic spreading depression-like depolarization. Physiol Rev (2001) 81:1065–96. doi:10.1152/physrev.2001.81.3.1065
8. Pietrobon D, Moskowitz MA. Chaos and commotion in the wake of cortical spreading depression and spreading depolarizations. Nat Rev Neurosci (2014) 15:379–93. doi:10.1038/nrn3770
9. Dreier JP, Isele T, Reiffurth C, Offenhauser N, Kirov SA, Dahlem MA, et al. Is spreading depolarization characterized by an abrupt, massive release of Gibbs free energy from the human brain cortex? Neuroscientist (2013) 19:25–42. doi:10.1177/1073858412453340
10. Alberts B, Johnson A, Lewis J, Raff M, Roberts K, Walter P. Molecular Biology of the Cell. New York: Garland Science (2002).
11. Rolfe DF, Brown GC. Cellular energy utilization and molecular origin of standard metabolic rate in mammals. Physiol Rev (1997) 77:731–58. doi:10.1152/physrev.1997.77.3.731
12. Zandt B-J, Stigen T, Ten Haken B, Netoff T, van Putten MJAM. Single neuron dynamics during experimentally induced anoxic depolarization. J Neurophysiol (2013) 110:1469–75. doi:10.1152/jn.00250.2013
13. Dreier JP. The role of spreading depression, spreading depolarization and spreading ischemia in neurological disease. Nat Med (2011) 17:439–47. doi:10.1038/nm.2333
14. Hartings JA, Li C, Hinzman JM, Shuttleworth CW, Ernst GL, Dreier JP, et al. Direct current electrocorticography for clinical neuromonitoring of spreading depolarizations. J Cereb Blood Flow Metab (2017) 37:1857–70. doi:10.1177/0271678X16653135
15. Leão AAP. Further observations on the spreading depression of activity in the cerebral cortex. J Neurophysiol (1947) 10. Available from: http://jn.physiology.org/content/10/6/409.1.long
16. Dreier JP, Woitzik J, Fabricius M, Bhatia R, Major S, Drenckhahn C, et al. Delayed ischaemic neurological deficits after subarachnoid haemorrhage are associated with clusters of spreading depolarizations. Brain (2006) 129:3224–37. doi:10.1093/brain/awl297
17. Schock SC, Munyao N, Yakubchyk Y, Sabourin LA, Hakim AM, Ventureyra ECG, et al. Cortical spreading depression releases ATP into the extracellular space and purinergic receptor activation contributes to the induction of ischemic tolerance. Brain Res (2007) 1168:129–38. doi:10.1016/j.brainres.2007.06.070
18. Lindquist BE, Shuttleworth CW. Adenosine receptor activation is responsible for prolonged depression of synaptic transmission after spreading depolarization in brain slices. Neuroscience (2012) 223:365–76. doi:10.1016/j.neuroscience.2012.07.053
19. Sawant-Pokam PM, Suryavanshi P, Mendez JM, Dudek FE, Brennan KC. Mechanisms of neuronal silencing after cortical spreading depression. Cereb Cortex (2017) 81:bhv328. doi:10.1093/cercor/bhv328
20. Takano T, Tian G-F, Peng W, Lou N, Lovatt D, Hansen AJ, et al. Cortical spreading depression causes and coincides with tissue hypoxia. Nat Neurosci (2007) 10:754–62. doi:10.1038/nn1902
21. Kraig RP, Nicholson C. Extracellular ionic variations during spreading depression. Neuroscience (1978) 3:1045–59. doi:10.1016/0306-4522(78)90122-7
22. Vorísek I, Syková E. Ischemia-induced changes in the extracellular space diffusion parameters, K+, and pH in the developing rat cortex and corpus callosum. J Cereb Blood Flow Metab (1997) 17:191–203. doi:10.1097/00004647-199702000-00009
23. Mazel T, Richter F, Vargová L, Syková E. Changes in extracellular space volume and geometry induced by cortical spreading depression in immature and adult rats. Physiol Res (2002) 51(Suppl 1):S85–93.
24. Windmuller O, Lindauer U, Foddis M, Einhäupl KM, Dirnagl U, Heinemann U, et al. Ion changes in spreading ischaemia induce rat middle cerebral artery constriction in the absence of NO. Brain (2005) 128:2042–51. doi:10.1093/brain/awh545
25. Murphy TH, Li P, Betts K, Liu R. Two-photon imaging of stroke onset in vivo reveals that NMDA-receptor independent ischemic depolarization is the major cause of rapid reversible damage to dendrites and spines. J Neurosci (2008) 28:1756–72. doi:10.1523/JNEUROSCI.5128-07.2008
26. Risher WC, Andrew RD, Kirov SA. Real-time passive volume responses of astrocytes to acute osmotic and ischemic stress in cortical slices and in vivo revealed by two-photon microscopy. Glia (2009) 57:207–21. doi:10.1002/glia.20747
27. Risher WC, Ard D, Yuan J, Kirov SA. Recurrent spontaneous spreading depolarizations facilitate acute dendritic injury in the ischemic penumbra. J Neurosci (2010) 30:9859–68. doi:10.1523/JNEUROSCI.1917-10.2010
28. Zhou N, Rungta RL, Malik A, Han H, Wu DC, MacVicar BA. Regenerative glutamate release by presynaptic NMDA receptors contributes to spreading depression. J Cereb Blood Flow Metab (2013) 33:1582–94. doi:10.1038/jcbfm.2013.113
29. Aiba I, Carlson AP, Sheline CT, Shuttleworth CW. Synaptic release and extracellular actions of Zn2+ limit propagation of spreading depression and related events in vitro and in vivo. J Neurophysiol (2012) 107:1032–41. doi:10.1152/jn.00453.2011
30. Allen NJ, Rossi DJ, Attwell D. Sequential release of GABA by exocytosis and reversed uptake leads to neuronal swelling in simulated ischemia of hippocampal slices. J Neurosci (2004) 24:3837–49. doi:10.1523/JNEUROSCI.5539-03.2004
31. Rungta RL, Choi HB, Tyson JR, Malik A, Dissing-Olesen L, Lin PJC, et al. The cellular mechanisms of neuronal swelling underlying cytotoxic edema. Cell (2015) 161:610–21. doi:10.1016/j.cell.2015.03.029
32. Steffensen AB, Sword J, Croom D, Kirov SA, MacAulay N. Chloride cotransporters as a molecular mechanism underlying spreading depolarization-induced dendritic beading. J Neurosci (2015) 35:12172–87. doi:10.1523/JNEUROSCI.0400-15.2015
33. Zandt B-J, ten Haken B, van Putten MJAM, Dahlem MA. How does spreading depression spread? Physiology and modeling. Rev Neurosci (2015) 26:183–98. doi:10.1515/revneuro-2014-0069
34. Jing J, Aitken PG, Somjen GG. Role of calcium channels in spreading depression in rat hippocampal slices. Brain Res (1993) 604:251–9. doi:10.1016/0006-8993(93)90376-X
35. Peters O, Schipke CG, Hashimoto Y, Kettenmann H. Different mechanisms promote astrocyte Ca2+ waves and spreading depression in the mouse neocortex. J Neurosci (2003) 23:9888–96.
36. Dietz RM, Weiss JH, Shuttleworth CW. Zn2+ influx is critical for some forms of spreading depression in brain slices. J Neurosci (2008) 28:8014–24. doi:10.1523/JNEUROSCI.0765-08.2008
37. Herreras O, Largo C, Ibarz JM, Somjen GG, Martín del Río R. Role of neuronal synchronizing mechanisms in the propagation of spreading depression in the in vivo hippocampus. J Neurosci (1994) 14:7087–98.
38. Sutor B, Luhmann HJ. Development of excitatory and inhibitory postsynaptic potentials in the rat neocortex. Perspect Dev Neurobiol (1995) 2:409–19.
39. Fukuda T, Kosaka T, Singer W, Galuske RAW. Gap junctions among dendrites of cortical GABAergic neurons establish a dense and widespread intercolumnar network. J Neurosci (2006) 26:3434–43. doi:10.1523/JNEUROSCI.4076-05.2006
40. Skou JC. The (Na++K+) activated enzyme system and its relationship to transport of sodium and potassium. Q Rev Biophys (1974) 7:401–34. doi:10.1017/S0033583500001475
41. Glitsch HG. Electrophysiology of the sodium-potassium-ATPase in cardiac cells. Physiol Rev (2001) 81:1791–826. doi:10.1152/physrev.2001.81.4.1791
42. Somjen GG. Ions in the Brain: Normal Function, Seizures, and Stroke. Oxford University Press (2004). Available from: https://global.oup.com/academic/product/ions-in-the-brain-9780195151718?cc=it&lang=en&#
43. Kager H, Wadman WJ, Somjen GG. Simulated seizures and spreading depression in a neuron model incorporating interstitial space and ion concentrations. J Neurophysiol (2000) 84:495–512. doi:10.1152/jn.2000.84.1.495
44. Hübel N, Dahlem MA. Dynamics from seconds to hours in Hodgkin-Huxley model with time-dependent ion concentrations and buffer reservoirs. PLoS Comput Biol (2014) 10:e1003941. doi:10.1371/journal.pcbi.1003941
45. Rose CR, Waxman SG, Ransom BR. Effects of glucose deprivation, chemical hypoxia, and simulated ischemia on Na+ homeostasis in rat spinal cord astrocytes. J Neurosci (1998) 18:3554–62.
46. Largo C, Cuevas P, Herreras O. Is glia disfunction the initial cause of neuronal death in ischemic penumbra? Neurol Res (1996) 18:445–8. doi:10.1080/01616412.1996.11740449
47. Largo C, Cuevas P, Somjen GG, Martín del Río R, Herreras O. The effect of depressing glial function in rat brain in situ on ion homeostasis, synaptic transmission, and neuron survival. J Neurosci (1996) 16:1219–29.
48. Chuquet J, Hollender L, Nimchinsky EA. High-resolution in vivo imaging of the neurovascular unit during spreading depression. J Neurosci (2007) 27:4036–44. doi:10.1523/JNEUROSCI.0721-07.2007
49. Charles AC, Baca SM. Cortical spreading depression and migraine. Nat Rev Neurol (2013) 9:637–44. doi:10.1038/nrneurol.2013.192
50. Attwell D, Laughlin SB. An energy budget for signaling in the grey matter of the brain. J Cereb Blood Flow Metab (2001) 21:1133–45. doi:10.1097/00004647-200110000-00001
51. Anderson CM, Nedergaard M. Astrocyte-mediated control of cerebral microcirculation. Trends Neurosci (2003) 26:340–4; author reply 344–5. doi:10.1016/S0166-2236(03)00141-3
52. Zonta M, Angulo MC, Gobbo S, Rosengarten B, Hossmann K-A, Pozzan T, et al. Neuron-to-astrocyte signaling is central to the dynamic control of brain microcirculation. Nat Neurosci (2002) 6:43–50. doi:10.1038/nn980
53. Farr H, David T. Models of neurovascular coupling via potassium and EET signalling. J Theor Biol (2011) 286:13–23. doi:10.1016/j.jtbi.2011.07.006
54. Ayata C. Spreading depression and neurovascular coupling. Stroke (2013) 44:S87–9. doi:10.1161/STROKEAHA.112.680264
55. Ayata C. Pearls and pitfalls in experimental models of spreading depression. Cephalalgia (2013) 33:604–13. doi:10.1177/0333102412470216
56. Dreier JP, Kleeberg J, Petzold G, Priller J, Windmüller O, Orzechowski H-D, et al. Endothelin-1 potently induces Leão’s cortical spreading depression in vivo in the rat: a model for an endothelial trigger of migrainous aura? Brain (2002) 125:102–12. doi:10.1093/brain/awf007
57. Houben T, Loonen IC, Baca SM, Schenke M, Meijer JH, Ferrari MD, et al. Optogenetic induction of cortical spreading depression in anesthetized and freely behaving mice. J Cereb Blood Flow Metab (2017) 37:1641–55. doi:10.1177/0271678X16645113
58. van den Maagdenberg AMJM, Pietrobon D, Pizzorusso T, Kaja S, Broos LAM, Cesetti T, et al. A Cacna1a knockin migraine mouse model with increased susceptibility to cortical spreading depression. Neuron (2004) 41:701–10. doi:10.1016/S0896-6273(04)00085-6
59. Eikermann-Haerter K, Dileköz E, Kudo C, Savitz SI, Waeber C, Baum MJ, et al. Genetic and hormonal factors modulate spreading depression and transient hemiparesis in mouse models of familial hemiplegic migraine type 1. J Clin Invest (2009) 119:99–109. doi:10.1172/JCI36059
60. Eising E, Shyti R, ’t Hoen PAC, Vijfhuizen LS, Huisman SMH, Broos LAM, et al. Cortical spreading depression causes unique dysregulation of inflammatory pathways in a transgenic mouse model of migraine. Mol Neurobiol (2017) 54:2986–96. doi:10.1007/s12035-015-9681-5
61. De Fusco M, Marconi R, Silvestri L, Atorino L, Rampoldi L, Morgante L, et al. Haploinsufficiency of ATP1A2 encoding the Na+/K+ pump α2 subunit associated with familial hemiplegic migraine type 2. Nat Genet (2003) 33:192–6. doi:10.1038/ng1081
62. Costa C, Tozzi A, Rainero I, Cupini LM, Calabresi P, Ayata C, et al. Cortical spreading depression as a target for anti-migraine agents. J Headache Pain (2013) 14:62. doi:10.1186/1129-2377-14-62
63. Theis M, Jauch R, Zhuo L, Speidel D, Wallraff A, Döring B, et al. Accelerated hippocampal spreading depression and enhanced locomotory activity in mice with astrocyte-directed inactivation of Connexin43. J Neurosci (2003) 23:766–76.
64. Pavlovic JM, Akcali D, Bolay H, Bernstein C, Maleki N. Sex-related influences in migraine. J Neurosci Res (2017) 95:587–93. doi:10.1002/jnr.23903
65. Brennan KC, Romero-Reyes M, López Valdés HE, Arnold AP, Charles AC. Reduced threshold for cortical spreading depression in female mice. Ann Neurol (2007) 61:603–6. doi:10.1002/ana.21138
66. Eikermann-Haerter K, Baum MJ, Ferrari MD, van den Maagdenberg AMJM, Moskowitz MA, Ayata C. Androgenic suppression of spreading depression in familial hemiplegic migraine type 1 mutant mice. Ann Neurol (2009) 66:564–8. doi:10.1002/ana.21779
67. Galanopoulou AS. Sexually dimorphic expression of KCC2 and GABA function. Epilepsy Res (2008) 80:99–113. doi:10.1016/j.eplepsyres.2008.04.013
68. Perrot-Sinal TS, Sinal CJ, Reader JC, Speert DB, McCarthy MM. Sex differences in the chloride cotransporters, NKCC1 and KCC2, in the developing hypothalamus. J Neuroendocrinol (2007) 19:302–8. doi:10.1111/j.1365-2826.2007.01530.x
69. Drenckhahn C, Winkler MKL, Major S, Scheel M, Kang E-J, Pinczolits A, et al. Correlates of spreading depolarization in human scalp electroencephalography. Brain (2012) 135:853–68. doi:10.1093/brain/aws010
70. Olesen J, Larsen B, Lauritzen M. Focal hyperemia followed by spreading oligemia and impaired activation of rCBF in classic migraine. Ann Neurol (1981) 9:344–52. doi:10.1002/ana.410090406
71. Headache Classification Committee of the International Headache Society (IHS). The International Classification of Headache Disorders, 3rd edition (beta version). Cephalalgia (2013) 33:629–808. doi:10.1177/0333102413485658
72. Shimazawa M, Hara H. An experimental model of migraine with aura: cortical hypoperfusion following spreading depression in the awake and freely moving rat. Clin Exp Pharmacol Physiol (1996) 23:890–2. doi:10.1111/j.1440-1681.1996.tb01139.x
73. Olesen J, Friberg L, Olsen TS, Iversen HK, Lassen NA, Andersen AR, et al. Timing and topography of cerebral blood flow, aura, and headache during migraine attacks. Ann Neurol (1990) 28:791–8. doi:10.1002/ana.410280610
74. Ostergaard L, Dreier JP, Hadjikhani N, Jespersen SN, Dirnagl U, Dalkara T. Neurovascular coupling during cortical spreading depolarization and -depression. Stroke (2015) 46:1392–401. doi:10.1161/STROKEAHA.114.008077
75. Woods RP, Iacoboni M, Mazziotta JC. Bilateral spreading cerebral hypoperfusion during spontaneous migraine headache. N Engl J Med (1994) 331:1689–92. doi:10.1056/NEJM199412223312505
76. Pietrobon D, Striessnig J. Neurological diseases: neurobiology of migraine. Nat Rev Neurosci (2003) 4:386–98. doi:10.1038/nrn1102
77. Moskowitz MA. The neurobiology of vascular head pain. Ann Neurol (1984) 16:157–68. doi:10.1002/ana.410160202
78. Moskowitz MA. Genes, proteases, cortical spreading depression and migraine: impact on pathophysiology and treatment. Funct Neurol (2007) 22:133–6.
79. Markowitz S, Saito K, Moskowitz MA. Neurogenically mediated leakage of plasma protein occurs from blood vessels in dura mater but not brain. J Neurosci (1987) 7:4129–36.
80. Buzzi MG, Carter WB, Shimizu T, Heath H, Moskowitz MA. Dihydroergotamine and sumatriptan attenuate levels of CGRP in plasma in rat superior sagittal sinus during electrical stimulation of the trigeminal ganglion. Neuropharmacology (1991) 30:1193–200. doi:10.1016/0028-3908(91)90165-8
81. Goadsby PJ, Edvinsson L, Ekman R. Vasoactive peptide release in the extracerebral circulation of humans during migraine headache. Ann Neurol (1990) 28:183–7. doi:10.1002/ana.410280213
82. Silverman WR, de Rivero Vaccari JP, Locovei S, Qiu F, Carlsson SK, Scemes E, et al. The pannexin 1 channel activates the inflammasome in neurons and astrocytes. J Biol Chem (2009) 284:18143–51. doi:10.1074/jbc.M109.004804
83. Gehrmann J, Mies G, Bonnekoh P, Banati R, Iijima T, Kreutzberg GW, et al. Microglial reaction in the rat cerebral cortex induced by cortical spreading depression. Brain Pathol (1993) 3:11–7. doi:10.1111/j.1750-3639.1993.tb00720.x
84. Jessen NA, Finmann Munk AS, Lundgaard I, Nedergaard M. The glymphatic system – a beginner’s guide Nadia. Neurochem Res (2015) 40:2583–99. doi:10.1007/s11064-015-1581-6.The
85. Schain AJ, Melo-Carrillo A, Strassman AM, Burstein R. Cortical spreading depression closes paravascular space and impairs glymphatic flow: implications for migraine headache. J Neurosci (2017) 37:2904–15. doi:10.1523/JNEUROSCI.3390-16.2017
86. Corradini I, Focchi E, Rasile M, Morini R, Desiato G, Tomasoni R, et al. Maternal immune activation delays excitatory-to-inhibitory gamma-aminobutyric acid switch in offspring. Biol Psychiatry (2017). doi:10.1016/j.biopsych.2017.09.030
87. Ayata C. Cortical spreading depression triggers migraine attack: pro. Headache (2010) 50:725–30. doi:10.1111/j.1526-4610.2010.01647.x
88. Guey S, Mawet J, Hervé D, Duering M, Godin O, Jouvent E, et al. Prevalence and characteristics of migraine in CADASIL. Cephalalgia (2016) 36:1038–47. doi:10.1177/0333102415620909
89. Tan RYY, Markus HS. CADASIL: migraine, encephalopathy, stroke and their inter-relationships. PLoS One (2016) 11:e0157613. doi:10.1371/journal.pone.0157613
90. Kazemi H, Speckmann E-J, Gorji A. Familial hemiplegic migraine and spreading depression. Iran J child Neurol (2014) 8:6–11.
91. Ophoff RA, Terwindt GM, Vergouwe MN, van Eijk R, Oefner PJ, Hoffman SM, et al. Familial hemiplegic migraine and episodic ataxia type-2 are caused by mutations in the Ca2+ channel gene CACNL1A4. Cell (1996) 87:543–52. doi:10.1016/S0092-8674(00)81373-2
92. Dichgans M, Freilinger T, Eckstein G, Babini E, Lorenz-Depiereux B, Biskup S, et al. Mutation in the neuronal voltage-gated sodium channel SCN1A in familial hemiplegic migraine. Lancet (2005) 366:371–7. doi:10.1016/S0140-6736(05)66786-4
93. Battistini S, Stenirri S, Piatti M, Gelfi C, Righetti PG, Rocchi R, et al. A new CACNA1A gene mutation in acetazolamide-responsive familial hemiplegic migraine and ataxia. Neurology (1999) 53:38–43. doi:10.1212/WNL.53.1.38
94. García-Baró-Huarte M, Iglesias-Mohedano AM, Slöcker-Barrio M, Vázquez-López M, García-Morín M, Miranda-Herrero MC, et al. Phenotypic variability in a four generation family with a p.Thr666Met CACNA1A gene mutation. Pediatr Neurol (2014) 51:557–9. doi:10.1016/j.pediatrneurol.2014.07.008
95. Donnini I, Nannucci S, Valenti R, Pescini F, Bianchi S, Inzitari D, et al. Acetazolamide for the prophylaxis of migraine in CADASIL: a preliminary experience. J Headache Pain (2012) 13:299–302. doi:10.1007/s10194-012-0426-9
96. Kraig RP, Ferreira-Filho CR, Nicholson C. Alkaline and acid transients in cerebellar microenvironment. J Neurophysiol (1983) 49:831–50. doi:10.1152/jn.1983.49.3.831
97. Tong CK, Chesler M. Endogenous pH shifts facilitate spreading depression by effect on NMDA receptors. J Neurophysiol (1999) 81:1988–91. doi:10.1152/jn.1999.81.4.1988
98. Eikermann-Haerter K, Negro A, Ayata C. Spreading depression and the clinical correlates of migraine. Rev Neurosci (2013) 24:353–63. doi:10.1515/revneuro-2013-0005
99. Hansen JM, Thomsen LL, Olesen J, Ashina M. Coexisting typical migraine in familial hemiplegic migraine. Neurology (2010) 74:594–600. doi:10.1212/WNL.0b013e3181cff79d
100. Maggioni F, Alessi C, Maggino T, Zanchin G. Headache during pregnancy. Cephalalgia (1997) 17:765–9. doi:10.1046/j.1468-2982.1997.1707765.x
101. Ayata C, Jin H, Kudo C, Dalkara T, Moskowitz MA. Suppression of cortical spreading depression in migraine prophylaxis. Ann Neurol (2006) 59:652–61. doi:10.1002/ana.20778
102. Chen SP, Ayata C. Spreading depression in primary and secondary headache disorders. Curr Pain Headache Rep (2016) 20:44. doi:10.1007/s11916-016-0574-8
103. Lampl C, Katsarava Z, Diener H-C, Limmroth V. Lamotrigine reduces migraine aura and migraine attacks in patients with migraine with aura. J Neurol Neurosurg Psychiatry (2005) 76:1730–2. doi:10.1136/jnnp.2005.063750
104. D’Andrea G, Granella F, Cadaldini M, Manzoni GC. Effectiveness of lamotrigine in the prophylaxis of migraine with aura: an open pilot study. Cephalalgia (1999) 19:64–6. doi:10.1111/j.1468-2982.1999.1901064.x
105. Sarrouilhe D, Dejean C, Mesnil M. Involvement of gap junction channels in the pathophysiology of migraine with aura. Front Physiol (2014) 5:78. doi:10.3389/fphys.2014.00078
106. Durham P, Garrett F. Neurological mechanisms of migraine: potential of the gap-junction modulator tonabersat in prevention of migraine. Cephalalgia (2009) 29:1–6. doi:10.1111/j.1468-2982.2009.01976.x
107. Silberstein S, Schoenen J, Göbel H, Diener H, Elkind A, Klapper J, et al. Tonabersat, a gap-junction modulator: efficacy and safety in two randomized, placebo-controlled, dose-ranging studies of acute migraineceph. Cephalalgia (2009) 29:17–27. doi:10.1111/j.1468-2982.2009.01974.x
108. Hauge AW, Asghar MS, Schytz HW, Christensen K, Olesen J. Effects of tonabersat on migraine with aura: a randomised, double-blind, placebo-controlled crossover study. Lancet Neurol (2009) 8:718–23. doi:10.1016/S1474-4422(09)70135-8
109. Chauvel V, Multon S, Schoenen J. Estrogen-dependent effects of 5-hydroxytryptophan on cortical spreading depression in rat: modelling the serotonin-ovarian hormone interaction in migraine aura. Cephalalgia (2017) 1:333102417690891. doi:10.1177/0333102417690891
110. Melo-Carrillo A, Strassman AM, Nir R-R, Schain A, Noseda R, Stratton J, et al. Fremanezumab – a humanized monoclonal anti-CGRP antibody—inhibits thinly myelinated (Aδ) but not unmyelinated (C) meningeal nociceptors. J Neurosci (2017) 37:2211–7. doi:10.1523/JNEUROSCI.2211-17.2017
111. Filiz A, Tepe N, Eftekhari S, Boran HE, Dilekoz E, Edvinsson L, et al. CGRP receptor antagonist MK-8825 attenuates cortical spreading depression induced pain behavior. Cephalalgia (2017) 1:333102417735845. doi:10.1177/0333102417735845
112. Thissen S, Koehler PJ. Persistent aura with small occipital cortical infarction: implications for migraine pathophysiology. Case Rep Neurol (2014) 6:217–21. doi:10.1159/000366409
113. Santos E, Sánchez-Porras R, Dohmen C, Hertle D, Unterberg AW, Sakowitz OW. Spreading depolarizations in a case of migraine-related stroke. Cephalalgia (2012) 32:433–6. doi:10.1177/0333102412441414
114. Dreier JP, Reiffurth C. The stroke-migraine depolarization continuum. Neuron (2015) 86:902–22. doi:10.1016/j.neuron.2015.04.004
115. Eikermann-Haerter K, Lee JH, Yuzawa I, Liu CH, Zhou Z, Shin HK, et al. Migraine mutations increase stroke vulnerability by facilitating ischemic depolarizations. Circulation (2012) 125:335–45. doi:10.1161/CIRCULATIONAHA.111.045096
116. Nozari A, Dilekoz E, Sukhotinsky I, Stein T, Eikermann-Haerter K, Liu C, et al. Microemboli may link spreading depression, migraine aura, and patent foramen ovale. Ann Neurol (2010) 67:221–9. doi:10.1002/ana.21871
117. Hossmann K-A. Pathophysiology and therapy of experimental stroke. Cell Mol Neurobiol (2006) 26:1055–81. doi:10.1007/s10571-006-9008-1
118. Siesjö BK. Pathophysiology and treatment of focal cerebral ischemia. J Neurosurg (1992) 77:169–84. doi:10.3171/jns.1992.77.2.0169
119. Rama R, García JC. In: Schaller B, editor. Excitotoxicity and Oxidative Stress in Acute Stroke, Ischemic Stroke – Updates. London: InTech. (2016)
120. Heiss W-D. The ischemic penumbra: how does tissue injury evolve? Ann N Y Acad Sci (2012) 1268:26–34. doi:10.1111/j.1749-6632.2012.06668.x
121. Besancon E, Guo S, Lok J, Tymianski M, Lo EH. Beyond NMDA and AMPA glutamate receptors: emerging mechanisms for ionic imbalance and cell death in stroke. Trends Pharmacol Sci (2008) 29:268–75. doi:10.1016/j.tips.2008.02.003
122. Astrup J, Siesjö BK, Symon L. Thresholds in cerebral ischemia – the ischemic penumbra. Stroke (1981) 12:723–5. doi:10.1161/01.STR.12.6.723
123. Farkas E, Bari F. Spreading depolarization in the ischemic brain: does aging have an impact? J Gerontol A Biol Sci Med Sci (2014) 69:1363–70. doi:10.1093/gerona/glu066
124. Nakamura H, Strong AJ, Dohmen C, Sakowitz OW, Vollmar S, Sué M, et al. Spreading depolarizations cycle around and enlarge focal ischaemic brain lesions. Brain (2010) 133:1994–2006. doi:10.1093/brain/awq117
125. Strong AJ, Anderson PJ, Watts HR, Virley DJ, Lloyd A, Irving EA, et al. Peri-infarct depolarizations lead to loss of perfusion in ischaemic gyrencephalic cerebral cortex. Brain (2007) 130:995–1008. doi:10.1093/brain/awl392
126. Bere Z, Obrenovitch TP, Kozák G, Bari F, Farkas E. Imaging reveals the focal area of spreading depolarizations and a variety of hemodynamic responses in a rat microembolic stroke model. J Cereb Blood Flow Metab (2014) 34:1695–705. doi:10.1038/jcbfm.2014.136
127. Clark D, Institoris A, Kozák G, Bere Z, Tuor U, Farkas E, et al. Impact of aging on spreading depolarizations induced by focal brain ischemia in rats. Neurobiol Aging (2014) 35:2803–11. doi:10.1016/j.neurobiolaging.2014.06.013
128. Kumagai T, Walberer M, Nakamura H, Endepols H, Sué M, Vollmar S, et al. Distinct spatiotemporal patterns of spreading depolarizations during early infarct evolution: evidence from real-time imaging. J Cereb Blood Flow Metab (2011) 31:580–92. doi:10.1038/jcbfm.2010.128
129. Ayata C, Lauritzen M. Spreading depression, spreading depolarizations, and the cerebral vasculature. Physiol Rev (2015) 95:953–93. doi:10.1152/physrev.00027.2014
130. Feuerstein D, Takagaki M, Gramer M, Manning A, Endepols H, Vollmar S, et al. Detecting tissue deterioration after brain injury: regional blood flow level versus capacity to raise blood flow. J Cereb Blood Flow Metab (2014) 34:1117–27. doi:10.1038/jcbfm.2014.53
131. Fabricius M, Akgoren N, Lauritzen M. Arginine-nitric oxide pathway and cerebrovascular regulation in cortical spreading depression. Am J Physiol (1995) 269:H23–9.
132. McCulloch J, Edvinsson L, Watt P. Comparison of the effects of potassium and pH on the calibre of cerebral veins and arteries. Pflugers Arch (1982) 393:95–8. doi:10.1007/BF00582399
134. Dohmen C, Sakowitz OW, Fabricius M, Bosche B, Reithmeier T, Ernestus R-I, et al. Spreading depolarizations occur in human ischemic stroke with high incidence. Ann Neurol (2008) 63:720–8. doi:10.1002/ana.21390
135. Chen S, Zeng L, Hu Z. Progressing haemorrhagic stroke: categories, causes, mechanisms and managements. J Neurol (2014) 261:2061–78. doi:10.1007/s00415-014-7291-1
136. Rowland MJ, Hadjipavlou G, Kelly M, Westbrook J, Pattinson KTS. Delayed cerebral ischaemia after subarachnoid haemorrhage: looking beyond vasospasm. Br J Anaesth (2012) 109:315–29. doi:10.1093/bja/aes264
137. Hamming AM, Wermer MJ, Umesh Rudrapatna S, Lanier C, van Os HJ, van den Bergh WM, et al. Spreading depolarizations increase delayed brain injury in a rat model of subarachnoid hemorrhage. J Cereb Blood Flow Metab (2016) 36:1224–31. doi:10.1177/0271678X15619189
138. Sánchez-Porras R, Zheng Z, Santos E, Schöll M, Unterberg AW, Sakowitz OW. The role of spreading depolarization in subarachnoid hemorrhage. Eur J Neurol (2013) 20:1121–7. doi:10.1111/ene.12139
139. Hubschmann OR, Kornhauser D. Cortical cellular response in acute subarachnoid hemorrhage. J Neurosurg (1980) 52:456–62. doi:10.3171/jns.1980.52.4.0456
140. Dreier JP, Körner K, Ebert N, Görner A, Rubin I, Back T, et al. Nitric oxide scavenging by hemoglobin or nitric oxide synthase inhibition by N-nitro-L-arginine induces cortical spreading ischemia when K+ is increased in the subarachnoid space. J Cereb Blood Flow Metab (1998) 18:978–90. doi:10.1097/00004647-199809000-00007
141. Petzold GC, Einhäupl KM, Dirnagl U, Dreier JP. Ischemia triggered by spreading neuronal activation is induced by endothelin-1 and hemoglobin in the subarachnoid space. Ann Neurol (2003) 54:591–8. doi:10.1002/ana.10723
142. Hartings JA, Strong AJ, Fabricius M, Manning A, Bhatia R, Dreier JP, et al. Spreading depolarizations and late secondary insults after traumatic brain injury. J Neurotrauma (2009) 26:1857–66. doi:10.1089/neu.2009.0961
143. Hartings JA, Bullock MR, Okonkwo DO, Murray LS, Murray GD, Fabricius M, et al. Spreading depolarisations and outcome after traumatic brain injury: a prospective observational study. Lancet Neurol (2011) 10:1058–64. doi:10.1016/S1474-4422(11)70243-5
144. Hinzman JM, Andaluz N, Shutter LA, Okonkwo DO, Pahl C, Strong AJ, et al. Inverse neurovascular coupling to cortical spreading depolarizations in severe brain trauma. Brain (2014) 137:2960–72. doi:10.1093/brain/awu241
145. von Bornstädt D, Houben T, Seidel JL, Zheng Y, Dilekoz E, Qin T, et al. Supply-demand mismatch transients in susceptible peri-infarct hot zones explain the origins of spreading injury depolarizations. Neuron (2015) 85:1117–31. doi:10.1016/j.neuron.2015.02.007
146. Raghavendra Rao VL, Başkaya MK, Doğan A, Rothstein JD, Dempsey RJ. Traumatic brain injury down-regulates glial glutamate transporter (GLT-1 and GLAST) proteins in rat brain. J Neurochem (2002) 70:2020–7. doi:10.1046/j.1471-4159.1998.70052020.x
147. Lauritzen M, Dreier JP, Fabricius M, Hartings JA, Graf R, Strong AJ. Clinical relevance of cortical spreading depression in neurological disorders: migraine, malignant stroke, subarachnoid and intracranial hemorrhage, and traumatic brain injury. J Cereb Blood Flow Metab (2011) 31:17–35. doi:10.1038/jcbfm.2010.191
148. Goodrich GS, Kabakov AY, Hameed MQ, Dhamne SC, Rosenberg PA, Rotenberg A. Ceftriaxone treatment after traumatic brain injury restores expression of the glutamate transporter, GLT-1, reduces regional gliosis, and reduces post-traumatic seizures in the rat. J Neurotrauma (2013) 30:1434–41. doi:10.1089/neu.2012.2712
149. Myer DJ, Gurkoff GG, Lee SM, Hovda DA, Sofroniew MV. Essential protective roles of reactive astrocytes in traumatic brain injury. Brain (2006) 129:2761–72. doi:10.1093/brain/awl165
150. Hosseini-Zare MS, Gu F, Abdulla A, Powell S, Žiburkus J. Effects of experimental traumatic brain injury and impaired glutamate transport on cortical spreading depression. Exp Neurol (2017) 295:155–61. doi:10.1016/j.expneurol.2017.05.002
151. Somjen GG. Aristides Leao’s discovery of cortical spreading depression. J Neurophysiol (2005) 94:2–4. doi:10.1152/classicessays.00031.2005
152. Teive HAG, Kowacs PA, Filho PM, Piovesan EJ, Werneck LC. Leão’s cortical spreading depression. Neurology (2005) 65:1455–9. doi:10.1212/01.wnl.0000183281.12779.cd
153. Petzold GC, Haack S, von Bohlen und Halbach O, Priller J, Lehmann T-N, Heinemann U, et al. Nitric oxide modulates spreading depolarization threshold in the human and rodent cortex. Stroke (2008) 39:1292–9. doi:10.1161/STROKEAHA.107.500710
154. Gorji A, Scheller D, Straub H, Tegtmeier F, Köhling R, Höhling JM, et al. Spreading depression in human neocortical slices. Brain Res (2001) 906:74–83. doi:10.1016/S0006-8993(01)02557-4
155. Koroleva VI, Bures J. Cortical penicillin focus as a generator of repetitive spike-triggered waves of spreading depression in rats. Exp Brain Res (1983) 51:291–7. doi:10.1007/BF00237205
156. Koroleva VI, Vinogradova LV, Bures J. Reduced incidence of cortical spreading depression in the course of pentylenetetrazol kindling in rats. Brain Res (1993) 608:107–14. doi:10.1016/0006-8993(93)90780-Q
157. Eickhoff M, Kovac S, Shahabi P, Khaleghi Ghadiri M, Dreier JP, Stummer W, et al. Spreading depression triggers ictaform activity in partially disinhibited neuronal tissues. Exp Neurol (2014) 253:1–15. doi:10.1016/j.expneurol.2013.12.008
158. Berger M, Speckmann E-J, Pape H, Gorji A. Spreading depression enhances human neocortical excitability in vitro. Cephalalgia (2008) 28:558–62. doi:10.1111/j.1468-2982.2008.01556.x
159. Ghadiri MK, Kozian M, Ghaffarian N, Stummer W, Kazemi H, Speckmann E-J, et al. Sequential changes in neuronal activity in single neocortical neurons after spreading depression. Cephalalgia (2012) 32:116–24. doi:10.1177/0333102411431308
160. Lauderdale K, Murphy T, Tung T, Davila D, Binder DK, Fiacco TA. Osmotic edema rapidly increases neuronal excitability through activation of NMDA receptor-dependent slow inward currents in juvenile and adult hippocampus. ASN Neuro (2015) 7:175909141560511. doi:10.1177/1759091415605115
161. Haghir H, Kovac S, Speckmann E-J, Zilles K, Gorji A. Patterns of neurotransmitter receptor distributions following cortical spreading depression. Neuroscience (2009) 163:1340–52. doi:10.1016/j.neuroscience.2009.07.067
162. Vinogradova L, Kuznetsova G, Coenen A. Audiogenic seizures associated with a cortical spreading depression wave suppress spike-wave discharges in rats. Physiol Behav (2005) 86:554–8. doi:10.1016/j.physbeh.2005.08.017
163. Samotaeva IS, Tillmanns N, van Luijtelaar G, Vinogradova LV. Intracortical microinjections may cause spreading depression and suppress absence seizures. Neuroscience (2013) 230:50–5. doi:10.1016/j.neuroscience.2012.11.013
164. Koroleva VI, Bures J. Stimulation induced recurrent epileptiform discharges block cortical and subcortical spreading depression in rats. Physiol Bohemoslov (1982) 31:385–400.
165. Kramer DR, Fujii T, Ohiorhenuan I, Liu CY. Interplay between cortical spreading depolarization and seizures. Stereotact Funct Neurosurg (2017) 95:1–5. doi:10.1159/000452841
166. Aiba I, Noebels JL. Spreading depolarization in the brainstem mediates sudden cardiorespiratory arrest in mouse SUDEP models. Sci Transl Med (2015) 7:282ra46. doi:10.1126/scitranslmed.aaa4050
167. Terra VC, Cysneiros R, Cavalheiro EA, Scorza FA. Sudden unexpected death in epilepsy: from the lab to the clinic setting. Epilepsy Behav (2013) 26:415–20. doi:10.1016/j.yebeh.2012.12.018
168. Devinsky O. Sudden, unexpected death in epilepsy. N Engl J Med (2011) 365:1801–11. doi:10.1056/NEJMra1010481
169. Tolstykh GP, Cavazos JE. Potential mechanisms of sudden unexpected death in epilepsy. Epilepsy Behav (2013) 26:410–4. doi:10.1016/j.yebeh.2012.09.017
170. Klassen T, Davis C, Goldman A, Burgess D, Chen T, Wheeler D, et al. Exome sequencing of ion channel genes reveals complex profiles confounding personal risk assessment in epilepsy. Cell (2011) 145:1036–48. doi:10.1016/j.cell.2011.05.025
171. Sowers LP, Massey CA, Gehlbach BK, Granner MA, Richerson GB. Sudden unexpected death in epilepsy: fatal post-ictal respiratory and arousal mechanisms. Respir Physiol Neurobiol (2013) 189:315–23. doi:10.1016/j.resp.2013.05.010
172. Kalume F, Westenbroek RE, Cheah CS, Yu FH, Oakley JC, Scheuer T, et al. Sudden unexpected death in a mouse model of Dravet syndrome. J Clin Invest (2013) 123:1798–808. doi:10.1172/JCI66220
173. Nei M, Mintzer S, Skidmore C, Sperling MR, Ho RT. Heart rate and blood pressure in sudden unexpected death in epilepsy (SUDEP). Epilepsy Res (2016) 122:44–6. doi:10.1016/j.eplepsyres.2016.02.008
174. Noebels J. Pathway-driven discovery of epilepsy genes. Nat Neurosci (2015) 18:344–50. doi:10.1038/nn.3933
175. Stecker EC, Reinier K, Uy-Evanado A, Teodorescu C, Chugh H, Gunson K, et al. Relationship between seizure episode and sudden cardiac arrest in patients with epilepsy: a community-based study. Circ Arrhythm Electrophysiol (2013) 6:912–6. doi:10.1161/CIRCEP.113.000544
176. Glasscock E, Yoo JW, Chen TT, Klassen TL, Noebels JL. Kv1.1 potassium channel deficiency reveals brain-driven cardiac dysfunction as a candidate mechanism for sudden unexplained death in epilepsy. J Neurosci (2010) 30:5167–75. doi:10.1523/JNEUROSCI.5591-09.2010
177. Smart SL, Lopantsev V, Zhang CL, Robbins CA, Wang H, Chiu SY, et al. Deletion of the K(V)1.1 potassium channel causes epilepsy in mice. Neuron (1998) 20:809–19. doi:10.1016/S0896-6273(00)81018-1
178. Goldberg EM, Clark BD, Zagha E, Nahmani M, Erisir A, Rudy B. K+ channels at the axon initial segment dampen near-threshold excitability of neocortical fast-spiking GABAergic interneurons. Neuron (2008) 58:387–400. doi:10.1016/j.neuron.2008.03.003
179. Ogiwara I, Miyamoto H, Morita N, Atapour N, Mazaki E, Inoue I, et al. Nav1.1 localizes to axons of parvalbumin-positive inhibitory interneurons: a circuit basis for epileptic seizures in mice carrying an Scn1a gene mutation. J Neurosci (2007) 27:5903–14. doi:10.1523/JNEUROSCI.5270-06.2007
180. Yu FH, Mantegazza M, Westenbroek RE, Robbins CA, Kalume F, Burton KA, et al. Reduced sodium current in GABAergic interneurons in a mouse model of severe myoclonic epilepsy in infancy. Nat Neurosci (2006) 9:1142–9. doi:10.1038/nn1754
181. Lauritzen M. Cortical spreading depression in migraine. Cephalalgia (2016) 21:757–60. doi:10.1111/J.1468-2982.2001.00244.x
Keywords: spreading depression, spreading depolarization, migraine, ischemia, subarachnoid hemorrhage, epilepsy, sudden unexpected death in epilepsy
Citation: Cozzolino O, Marchese M, Trovato F, Pracucci E, Ratto GM, Buzzi MG, Sicca F and Santorelli FM (2018) Understanding Spreading Depression from Headache to Sudden Unexpected Death. Front. Neurol. 9:19. doi: 10.3389/fneur.2018.00019
Received: 23 November 2017; Accepted: 11 January 2018;
Published: 01 February 2018
Edited by:
Vincenzo Guidetti, Sapienza Università di Roma, ItalyReviewed by:
Gianluca Coppola, Fondazione G.B. Bietti (IRCCS), ItalyCopyright: © 2018 Cozzolino, Marchese, Trovato, Pracucci, Ratto, Buzzi, Sicca and Santorelli. This is an open-access article distributed under the terms of the Creative Commons Attribution License (CC BY). The use, distribution or reproduction in other forums is permitted, provided the original author(s) and the copyright owner are credited and that the original publication in this journal is cited, in accordance with accepted academic practice. No use, distribution or reproduction is permitted which does not comply with these terms.
*Correspondence: Filippo M. Santorelli, ZmlsaXBwbzMzNjRAZ21haWwuY29t
†The authors wish it to be known that, in their opinion, the first two authors should be regarded as joint First Authors.
Disclaimer: All claims expressed in this article are solely those of the authors and do not necessarily represent those of their affiliated organizations, or those of the publisher, the editors and the reviewers. Any product that may be evaluated in this article or claim that may be made by its manufacturer is not guaranteed or endorsed by the publisher.
Research integrity at Frontiers
Learn more about the work of our research integrity team to safeguard the quality of each article we publish.