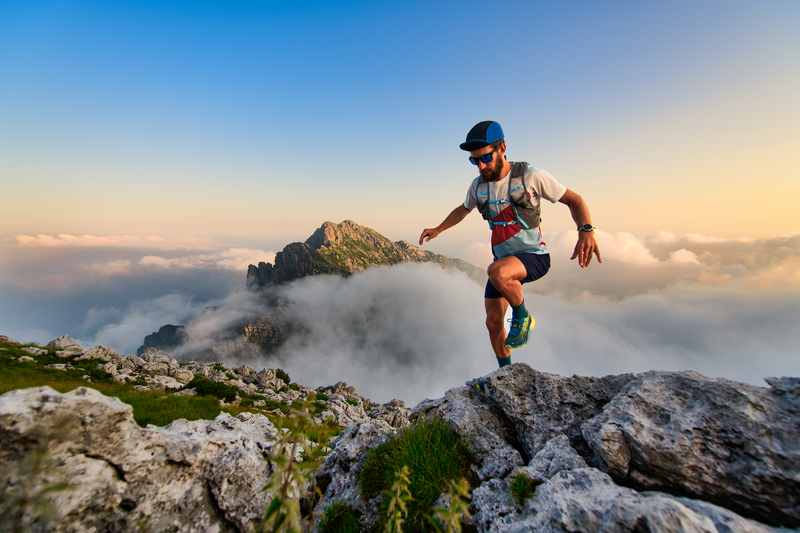
95% of researchers rate our articles as excellent or good
Learn more about the work of our research integrity team to safeguard the quality of each article we publish.
Find out more
MINI REVIEW article
Front. Neurol. , 04 October 2017
Sec. Movement Disorders
Volume 8 - 2017 | https://doi.org/10.3389/fneur.2017.00527
This article is part of the Research Topic Modeling Parkinson's Disease: Bridging the Translational Gap View all 11 articles
Neurons affected in Parkinson’s disease (PD) experience mitochondrial dysfunction and bioenergetic deficits that occur early and promote the disease-related α-synucleinopathy. Emerging findings suggest that the autophagy-lysosome pathway, which removes damaged mitochondria (mitophagy), is also compromised in PD and results in the accumulation of dysfunctional mitochondria. Studies using genetic-modulated or toxin-induced animal and cellular models as well as postmortem human tissue indicate that impaired mitophagy might be a critical factor in the pathogenesis of synaptic dysfunction and the aggregation of misfolded proteins, which in turn impairs mitochondrial homeostasis. Interventions that stimulate mitophagy to maintain mitochondrial health might, therefore, be used as an approach to delay the neurodegenerative processes in PD.
Parkinson’s disease (PD) is an incurable chronic progressive disease affecting nearly 2% of the “over 50” population with an approximately estimate of more than 6 million cases worldwide (1). The cause of PD is generally unknown, but it is believed to involve both genetic and environmental factors (2). Epidemiological studies have revealed that fewer than 10% of PD cases are inherited from family, whereas the majority of cases are sporadic (3). Discoveries of genes linked to rare familial forms of PD have confirmed the critical role of genes in the development of PD and made great contributions in understanding the molecular pathogenesis behind this common but complex illness. Autophagy is a conserved pathway that degrades damaged organelles and misfolded proteins (4). Here, we consider the roles of autophagy in neuronal health and the pathological mechanisms leading to disease progression to help us seek for potential targets for neuroprotective interventions, which may revolutionize the treatment of this incurable disease.
Parkinson’s disease is a neurodegenerative movement disorder characterized by the preferential loss of dopaminergic neurons in the substantia nigra, which results in progressive motor system malfunction (5). Primary motor signs that characterize PD include rigidity, bradykinesia, postural instability, and tremor (6). The pathology of PD remains unknown, but almost all cases show the presence of intraneuronal misfolded protein aggregates forming Lewy bodies, the primary component of which is α-synuclein (7). Protein homeostasis is crucial to sustain cellular health and viability in neurons (8). The process of α-synuclein accumulation resulting in the generation of highly diffusible small oligomers and fibrils, which abnormally aggregate and can be visualized as eosinophilic cytoplasmic inclusion in neurons (9). Evidence indicates that the accumulation of [α-synuclein, possibly oligomers, without insoluble aggregates, may lead to oxidative stress and give rise to deleterious effects in dopamine (DA) neurons (10–13)].
Recent evidence suggests that α-synuclein is a lipophilic protein, localized to mitochondria and connected to endoplasmic reticulum (ER) through mitochondrial-associated ER membrane (MAM) (14, 15). Overexpression of α-synuclein inhibits the normal function of inner-mitochondrial membrane-anchored respiratory chain complexes in whole brain of PD patients, but mostly in nigrostriatal neurons. Increased levels of reactive oxygen species (ROS) might be the cause of neuronal death (16). A study has also demonstrated that α-synuclein overexpression in mitochondria increases the number of fragmented mitochondria in vitro (17). In addition, intermediate α-synuclein accumulation (pre-fibrillar forms) reduces mitochondrial Ca2+ retention (18). Ca2+ is required by mitochondria for the generation of ATP via the tricarboxylic acid cycle (19). Perturbed neuronal Ca2+ levels caused by soluble pre-fibrillar α-synuclein lead to altered mitochondrial membrane potential and NADH oxidation, which indicate the dysfunction of complex I (20). The effect of complex I inhibitor 1-methyl-4-phenyl-1,2,3,6-tetrahydropyridine (MPTP) and its active metabolite 1-methyl-4-phenylpyridinium (MPP+) on dopaminergic cell death is inhibited in mouse models lacking α-synuclein, which is mainly due to the inactivation of nitric oxide synthase (NOS) (21). In addition, siRNA-mediated knockdown of α-synuclein also protects cells from NOS activation in cellular models, rescuing cells from MPP+-induced apoptosis (22).
Posttranslational modification of α-synuclein is also a crucial factor in the pathological mechanisms of PD. Many PD-associated mutations in α-synuclein also induce mitochondrial dysfunction. The H50Q mutation is proved to induce aggregation of α-synuclein oligomers in SH-SY5Y cells and increase the number of fragmented mitochondria in hippocampal neurons in vivo (23, 24). Ser129-induced α-synuclein aggregation is involved in the formation of Lewy bodies and plays a critical role in the neurodegenerative process (25). SH-SY5Y cells expressing A53T α-synuclein exhibit depolarized mitochondrial and increased ROS levels when exposed to rotenone (26). Studies in transgenic mice overexpressing the A53T-mutant human α-synuclein revealed that intracerebral inoculation of aggregated α-synuclein or preformed recombinant α-synuclein fibrils induces a progressive and ultimately lethal α-synucleinopathy in inoculated animals (27, 28).
Damaged cellular function and decreased ATP levels induced by α-synuclein are detrimental to dopaminergic neurons and provide implications for disease pathogenesis in PD. Impaired mitochondrial function may lead to a reduction in cellular energy levels and excessive ROS production in neurons, which in turn exacerbate mitochondrial damage (29). As a result, measures to enhance the degradation of abnormally aggregated proteins and the clearance of damaged mitochondria seem to be the most promising strategies in rescuing neurodegeneration in PD patients.
Autophagy is an evolutionarily conserved process in which cytoplasmic substrates are engulfed in autophagic vesicles and fused to lysosomes for degradation and recycling (30). The specific autophagic elimination of mitochondria is defined as mitophagy (31). Autophagy is classified into various subgroups based on the mechanism of substrate delivery to the lysosome, including macroautophagy, chaperone-mediated autophagy (CMA), and microautophagy (4). The process of mitophagy is directed mainly by macroautophagy. Genome-wide association studies implicate that PD-related genes and their products are responsible for mitochondrial homeostasis and mitophagy (32, 33).
PINK1 and Parkin are the most well-known proteins related to PD. PINK1, encoded by PARK6 gene, is a mitochondrial-targeted serine/threonine kinase, while Parkin, encoded by the PARK2 gene, is a 465-amino acid E3 ubiquitin ligase (34, 35). “Loss-of-function” mutations in either PINK1 or Parkin lead to autosomal recessive forms of PD (35, 36). PINK1-dependent activation of Parkin is recognized as a major pathway of mitophagy (37). When mitochondria become depolarized, PINK1 accumulates on the surface of the outer membrane of mitochondria, where it phosphorylates both ubiquitin and Parkin and activates Parkin’s ubiquitin E3-ligase activity. Moreover, it was recently shown that wild-type PINK1 recruits Parkin to damaged mitochondria during mitophagy rather than the PD-linked PINK1-mutant forms (38). The subsequent recruitment of ubiquitin-binding mitophagy receptors lead to the formation of LC3-positive phagophores, which sequester damaged mitochondria from the cytosol and eventually degrade by lysosomal hydrolases (39). PINK1 and Parkin are also important for sustaining mitochondrial homeostasis through the regulation of mitochondrial fission and fusion. A study has shown that the ubiquitination process of mitochondrial fusion protein mitofusin (Mfn) is mediated by both PINK1 and Parkin. Loss of PINK1 or Parkin causes damaged mitophagy process and elongated mitochondria in Drosophila (40). Genetic loss of Mfn1 and Mfn2 leads to the dissipation of membrane potential in a subset of mitochondria, preventing Parkin’s recruitment process through the translocase of the inner membrane complex (41). Parkin-mutant or PINK1-mutant Drosophila display a severe defect in flight muscle, leading to behavioral locomotive problems and greater susceptibility to oxidative stress (42, 43). Indirect flight muscles and DA neurons in this model are filled with swollen mitochondria (44, 45). Mutant-Parkin displays degeneration of a subset of DA neurons, exhibiting shrinkage in morphology and decreased DA level in Drosophila brains (42, 46, 47). PINK1 knockout fibroblasts and neurons exhibit reduced membrane potential, overloaded Ca2+ levels and increased ROS production in mitochondria (48, 49). Meanwhile, mitochondria isolated from the brain of PINK1 knockout mice show defects in Ca2+-buffering capacity and increased vulnerability of neurons in oxidative stress caused by inflammation (50). DA neuronal death is also observed in a conditional Parkin ablation mouse model after lentivirus delivers the Cre recombinase to the mouse brain, which suggests that Parkin plays an important role in neuronal survival (51).
Mutations in the PARK7 gene, which encode DJ-1, cause a rare autosomal recessive form of PD (52, 53). DJ-1, a transcriptional regulator, is often known as a redox sensor/reductase which influences mitochondrial homeostasis and mitophagy (54). It is long believed that DJ-1 is a neuroprotective factor (55). Mitochondria localized DJ-1 is a component of thioredoxin/apoptosis signal-regulating kinase 1 (Trx/Ask1) complex, which regulates the clearance of endogenous ROS through the modulation of scavenging systems (56). DJ-1 deficiency decreases brain mitochondria consumption of H2O2, leading to the increased level of oxidative stress, and eventually causes cell death in DA neurons (54, 57). In addition, DJ-1 directly interacts with α-synuclein. The mutant form of DJ-1 in PD causes misfolded α-synuclein aggregate in DA neurons, while the overexpression of DJ-1 reduces the dimerization of α-synuclein (55).
LRRK2 is a member of the leucine-rich repeat kinase family that is encoded by the PARK8 gene (58). Mutations in LRRK2 are associated with autosomal-dominant PD (33). Expression of mutant LRRK2 may have a variety of negative effects on mitochondrial and cellular health (59, 60). Endogenous LRRK2 directly interacts with the mitochondrial fission and fusion regulators dynamin-related protein 1, Mfn, and optic atrophy 1 (OPA1) to maintain the balance among mitochondrial biogenesis, intracellular material trafficking, metabolic demands, and mitochondrial morphology (61–63). G2019S mutant LRRK2 in sporadic PD patients showed decreased levels of OPA1, indicating that LRRK2 kinase activity is also an important factor in mitochondrial dynamics (64). The overexpression of G2019S mutant LRRK2 in mouse brains showed mitochondrial uncoupling accompanying with an increased basal oxygen consumption in both fibroblast and neuroblastoma cells, resulting in decreased ATP level and compromised cellular function (65). Fibroblasts with G2019S mutant LRRK2 from PD patients showed increased susceptibility to MPP+ induced cell death (66). Meanwhile, the depletion of LRRK2 or mutant LRRK2 impair the autophagy/lysosomal pathway, leading to the accumulation of autophagosomes (67, 68). The degradation of LRRK2 in lysosomes is mediated by CMA in nervous system, while the mutant forms of LRRK2 and also high concentrations of wild-type LRRK2 interfere with the CMA translocation complex, resulting in defective CMA (67, 69). Inhibition of CMA in neurons induces the accumulation of both soluble and insoluble α-synuclein, which in turn could compromise the degradation of α-synuclein and initiate protein aggregation in PD (70, 71).
Lysosomal defects in the clearance of cytosolic substrates also contribute to the progression of PD (72). PARK9 encoded lysosomal ATPase ATP13A2 is a P-type transport ATPase which protects against cellular dysfunction caused by α-synuclein (73). PD-linked mutations in ATP13A2 reduce the activity of proteolytic processing enzymes, disturbing the acid environment in lysosomes, resulting in the impaired degrading capacity of autophagosomes (74).
As we can see, these PD-related genes not only play a role in the maintenance of mitochondrial homeostasis but also are important for the clearance of aggregated proteins and damaged organelles through mitophagy. Mitochondrial deficiency is responsible for neurodegeneration in PD, but the specific mechanism between mitochondrial deficiency and α-synuclein aggregation remains to be discovered.
Intracellular misfolded proteins contribute to cellular dysfunction and neuronal death in PD patients. Moreover, compromised clearance pathways aggravate the pathological process of this neurodegenerative disease. Since autophagy plays an important role in selectively degrading misfolded proteins and damaged organelles, it could be an interesting target for the development of efficient treatment for PD. Nowadays, up-to-date researches also give us implications on PD-related genes and their influence on mitochondrial homeostasis. The obstacles between this promising therapeutic targets and mitochondrial dynamic are still a major challenge for us to overcome.
Methods identified to enhance autophagy in several preclinical PD models are proven to be effective. The serine/threonine protein kinase mTOR is a component of the mTOR complex 1 and suppresses autophagy under nutrient-rich conditions (75). The mTOR inhibitor rapamycin, which stabilizes the association of mTOR complex and inhibits the kinase activity, is the most widely used small molecule drug which is proved effective in enhancing autophagy activity in many disease models (76–81). Rapamycin selectively suppresses the activity of mTOR through the dephosphorylation of Akt kinase, which is crucial for neuronal survival in PD models (82, 83).
Beclin 1 is encoded by autophagy-related gene 6. This protein interacts with either BCL-2 or the class III phosphatidylinositol 3-kinase (PI3K) VPS34, playing a critical role in the localization of other autophagy-regulatory proteins to the preautophagosomal structure (84). Beclin 1 is negatively regulated by BCL-2 and BCL-XL at ER membranes (85). Mutations in BH3-related domain in Beclin 1 disrupt the formation of Beclin 1–BCL-2 complex, leading to enhanced autophagy (86). Chronic administration of trehalose results in a reduction of the frontal cortex p62/beclin 1 level, suggesting an elevated state of autophagy (87–90). Moreover, ER stress is responsible for the activation of autophagy through the unfolded-protein response (UPR) (91). Tunicamycin Induced mild ER stress shows a promising treatment potential in protecting dopaminergic neurons from death in PD models (92). Gene therapy approaches to handle the unfolded protein load via the activation of UPR are designed to manipulate autophagy in a more specific manner (93). Beclin 1 gene therapy mediated by lentivirus exhibits not only positive effects in the clearance of intraneuronal α-synuclein proteins but also a proved synaptic function in PD models (94). Gene therapy also exhibits great potential in the clearance of abnormally aggregated proteins in other neurodegenerative diseases through the activation of autophagy (95–97).
Although methods to activate autophagy are promising novel therapeutic approaches for PD, a complex scenario is emerging in which the alteration of distinct regulatory steps in autophagy may perturb the homeostasis of the cell, contributing to the disease progression as well (98). Therefore, the mere enhancement of autophagy may have detrimental consequences by provoking neurodegeneration and exacerbating disease progression. Thus, it is critical that this biological process should be precisely regulated and strictly monitored. Moreover, the specific mechanism behind each subtype of the disease that may link the defects of autophagy to PD still remains to be discovered. Considering the complex nature of PD, individualized interventional targets seem to be the most promising method for deciding the right timing and appropriate degree of activation of autophagy.
Significant progress has been made in understanding the causes of this neurodegenerative disorder. The accumulation of dysfunctional mitochondria and compromised mitophagy have emerged as common features of affected neurons in patients and animal models that may cause the accumulation of misfolded protein aggregates. In addition, aggregation of α-synuclein and deficiency in PD-related genes can impair neuronal mitophagy and mitochondrial homeostasis. It is crucial to find out the key factors and their roles involved in the pathogenesis of different form of PD. Further studies aiming at modulating the process of autophagy accurately and individually may provide novel therapeutic strategies for this widespread disease.
FG, JY, DW, CL, YF, and HW wrote the manuscript. WH and JZ edited the manuscript.
The authors declare that the research was conducted in the absence of any commercial or financial relationships that could be construed as a potential conflict of interest.
This work was supported by the National Natural Science Foundation of China (31471016 and 81602503), the CAMS Initiative for Innovative Medicine (2016-I2 M-1-008), and the National Key Research and Development Program of China (2016YFA0101001).
1. de Rijk MC, Breteler MM, Graveland GA, Ott A, Grobbee DE, van der Meché FG, et al. Prevalence of Parkinson’s disease in the elderly: the Rotterdam study. Neurology (1995) 45:2143–6. doi:10.1212/WNL.45.12.2143
2. Gan-Or Z, Dion PA, Rouleau GA. Genetic perspective on the role of the autophagy-lysosome pathway in Parkinson disease. Autophagy (2015) 11:1443–57. doi:10.1080/15548627.2015.1067364
3. Schapira AHV. Mitochondria in the aetiology and pathogenesis of Parkinson’s disease. Lancet Neurol (2008) 7:97–109. doi:10.1016/S1474-4422(07)70327-7
4. Galluzzi L, Baehrecke EH, Ballabio A, Boya P, Bravo-San Pedro JM, Cecconi F, et al. Molecular definitions of autophagy and related processes. EMBO J (2017) 36:1811–36. doi:10.15252/embj.201796697
5. Dauer W, Przedborski S. Parkinson’s disease. Neuron (2003) 39:889–909. doi:10.1016/S0896-6273(03)00568-3
6. Lang AE, Lozano AM. Parkinson’s disease. First of two parts. N Engl J Med (1998) 339:1044–53. doi:10.1056/NEJM199810083391506
7. Spillantini MG, Schmidt ML, Lee VM, Trojanowski JQ, Jakes R, Goedert M. Alpha-synuclein in Lewy bodies. Nature (1997) 388:839–40. doi:10.1038/42166
8. Taylor RC, Berendzen KM, Dillin A. Systemic stress signalling: understanding the cell non-autonomous control of proteostasis. Nat Rev Mol Cell Biol (2014) 15:211–7. doi:10.1038/nrm3752
9. Tsigelny IF, Bar-On P, Sharikov Y, Crews L, Hashimoto M, Miller MA, et al. Dynamics of alpha-synuclein aggregation and inhibition of pore-like oligomer development by beta-synuclein. FEBS J (2007) 274:1862–77. doi:10.1111/j.1742-4658.2007.05733.x
10. Decressac M, Mattsson B, Weikop P, Lundblad M, Jakobsson J, Björklund A. TFEB-mediated autophagy rescues midbrain dopamine neurons from α-synuclein toxicity. Proc Natl Acad Sci U S A (2013) 110:E1817–26. doi:10.1073/pnas.1305623110
11. Tokuda T, Qureshi MM, Ardah MT, Varghese S, Shehab SAS, Kasai T, et al. Detection of elevated levels of α-synuclein oligomers in CSF from patients with Parkinson disease. Neurology (2010) 75:1766–72. doi:10.1212/WNL.0b013e3181fd613b
12. Winner B, Jappelli R, Maji SK, Desplats PA, Boyer L, Aigner S, et al. In vivo demonstration that alpha-synuclein oligomers are toxic. Proc Natl Acad Sci U S A (2011) 108:4194–9. doi:10.1073/pnas.1100976108
13. Giehm L, Svergun DI, Otzen DE, Vestergaard B. Low-resolution structure of a vesicle disrupting alpha-synuclein oligomer that accumulates during fibrillation. Proc Natl Acad Sci U S A (2011) 108:3246–51. doi:10.1073/pnas.1013225108
14. Parihar MS, Parihar A, Fujita M, Hashimoto M, Ghafourifar P. Mitochondrial association of alpha-synuclein causes oxidative stress. Cell Mol Life Sci (2008) 65:1272–84. doi:10.1007/s00018-008-7589-1
15. Hayashi T, Rizzuto R, Hajnoczky G, Su T-P. MAM: more than just a housekeeper. Trends Cell Biol (2009) 19:81–8. doi:10.1016/j.tcb.2008.12.002
16. Subramaniam SR, Vergnes L, Franich NR, Reue K, Chesselet M-F. Region specific mitochondrial impairment in mice with widespread overexpression of alpha-synuclein. Neurobiol Dis (2014) 70:204–13. doi:10.1016/j.nbd.2014.06.017
17. Plotegher N, Gratton E, Bubacco L. Number and brightness analysis of alpha-synuclein oligomerization and the associated mitochondrial morphology alterations in live cells. Biochim Biophys Acta (2014) 1840:2014–24. doi:10.1016/j.bbagen.2014.02.013
18. Calì T, Ottolini D, Negro A, Brini M. α-Synuclein controls mitochondrial calcium homeostasis by enhancing endoplasmic reticulum-mitochondria interactions. J Biol Chem (2012) 287:17914–29. doi:10.1074/jbc.M111.302794
19. Rowland AA, Voeltz GK. Endoplasmic reticulum-mitochondria contacts: function of the junction. Nat Rev Mol Cell Biol (2012) 13:607–25. doi:10.1038/nrm3440
20. Luth ES, Stavrovskaya IG, Bartels T, Kristal BS, Selkoe DJ. Soluble, prefibrillar α-synuclein oligomers promote complex I-dependent, Ca2+-induced mitochondrial dysfunction. J Biol Chem (2014) 289:21490–507. doi:10.1074/jbc.M113.545749
21. Fountaine TM, Venda LL, Warrick N, Christian HC, Brundin P, Channon KM, et al. The effect of alpha-synuclein knockdown on MPP+ toxicity in models of human neurons. Eur J Neurosci (2008) 28:2459–73. doi:10.1111/j.1460-9568.2008.06527.x
22. Ryan BJ, Lourenço-Venda LL, Crabtree MJ, Hale AB, Channon KM, Wade-Martins R. α-Synuclein and mitochondrial bioenergetics regulate tetrahydrobiopterin levels in a human dopaminergic model of Parkinson disease. Free Radic Biol Med (2014) 67:58–68. doi:10.1016/j.freeradbiomed.2013.10.008
23. Appel-Cresswell S, Vilarino-Guell C, Encarnacion M, Sherman H, Yu I, Shah B, et al. Alpha-synuclein p.H50Q, a novel pathogenic mutation for Parkinson’s disease. Mov Disord (2013) 28:811–3. doi:10.1002/mds.25421
24. Khalaf O, Fauvet B, Oueslati A, Dikiy I, Mahul-Mellier A-L, Ruggeri FS, et al. The H50Q mutation enhances α-synuclein aggregation, secretion, and toxicity. J Biol Chem (2014) 289:21856–76. doi:10.1074/jbc.M114.553297
25. Fujiwara H, Hasegawa M, Dohmae N, Kawashima A, Masliah E, Goldberg MS, et al. alpha-Synuclein is phosphorylated in synucleinopathy lesions. Nat Cell Biol (2002) 4:160–4. doi:10.1038/ncb748
26. Perfeito R, Lázaro DF, Outeiro TF, Rego AC. Linking alpha-synuclein phosphorylation to reactive oxygen species formation and mitochondrial dysfunction in SH-SY5Y cells. Mol Cell Neurosci (2014) 62:51–9. doi:10.1016/j.mcn.2014.08.002
27. Mougenot A-L, Nicot S, Bencsik A, Morignat E, Verchère J, Lakhdar L, et al. Prion-like acceleration of a synucleinopathy in a transgenic mouse model. Neurobiol Aging (2012) 33:2225–8. doi:10.1016/j.neurobiolaging.2011.06.022
28. Luk KC, Kehm VM, Zhang B, O’Brien P, Trojanowski JQ, Lee VMY. Intracerebral inoculation of pathological α-synuclein initiates a rapidly progressive neurodegenerative α-synucleinopathy in mice. J Exp Med (2012) 209:975–86. doi:10.1084/jem.20112457
29. Mattson MP, Gleichmann M, Cheng A. Mitochondria in neuroplasticity and neurological disorders. Neuron (2008) 60:748–66. doi:10.1016/j.neuron.2008.10.010
31. Lemasters JJ. Selective mitochondrial autophagy, or mitophagy, as a targeted defense against oxidative stress, mitochondrial dysfunction, and aging. Rejuvenation Res (2005) 8:3–5. doi:10.1089/rej.2005.8.3
32. Ryan BJ, Hoek S, Fon EA, Wade-Martins R. Mitochondrial dysfunction and mitophagy in Parkinson’s: from familial to sporadic disease. Trends Biochem Sci (2015) 40:200–10. doi:10.1016/j.tibs.2015.02.003
33. International Parkinson Disease Genomics Consortium, Nalls MA, Plagnol V, Hernandez DG, Sharma M, Sheerin U-M, et al. Imputation of sequence variants for identification of genetic risks for Parkinson’s disease: a meta-analysis of genome-wide association studies. Lancet (2011) 377:641–9. doi:10.1016/S0140-6736(10)62345-8
34. Valente EM, Abou-Sleiman PM, Caputo V, Muqit MMK, Harvey K, Gispert S, et al. Hereditary early-onset Parkinson’s disease caused by mutations in PINK1. Science (2004) 304:1158–60. doi:10.1126/science.1096284
35. Moore DJ, West AB, Dawson VL, Dawson TM. Molecular pathophysiology of Parkinson’s disease. Annu Rev Neurosci (2005) 28:57–87. doi:10.1146/annurev.neuro.28.061604.135718
36. Zhang J, Ney PA. NIX induces mitochondrial autophagy in reticulocytes. Autophagy (2008) 4:354–6. doi:10.4161/auto.5552
37. Grenier K, McLelland G-L, Fon EA. Parkin- and PINK1-dependent mitophagy in neurons: will the real pathway please stand up? Front Neurol (2013) 4:100. doi:10.3389/fneur.2013.00100
38. Vives-Bauza C, Zhou C, Huang Y, Cui M, de Vries RLA, Kim J, et al. PINK1-dependent recruitment of Parkin to mitochondria in mitophagy. Proc Natl Acad Sci U S A (2010) 107:378–83. doi:10.1073/pnas.0911187107
39. Moore AS, Holzbaur ELF. Spatiotemporal dynamics of autophagy receptors in selective mitophagy. Autophagy (2016) 12:1956–7. doi:10.1080/15548627.2016.1212788
40. Ziviani E, Tao RN, Whitworth AJ. Drosophila Parkin requires PINK1 for mitochondrial translocation and ubiquitinates mitofusin. Proc Natl Acad Sci U S A (2010) 107:5018–23. doi:10.1073/pnas.0913485107
41. Pickrell AM, Youle RJ. The roles of PINK1, parkin, and mitochondrial fidelity in Parkinson’s disease. Neuron (2015) 85:257–73. doi:10.1016/j.neuron.2014.12.007
42. Greene JC, Whitworth AJ, Kuo I, Andrews LA, Feany MB, Pallanck LJ. Mitochondrial pathology and apoptotic muscle degeneration in Drosophila Parkin mutants. Proc Natl Acad Sci U S A (2003) 100:4078–83. doi:10.1073/pnas.0737556100
43. Pesah Y, Pham T, Burgess H, Middlebrooks B, Verstreken P, Zhou Y, et al. Drosophila Parkin mutants have decreased mass and cell size and increased sensitivity to oxygen radical stress. Development (2004) 131:2183–94. doi:10.1242/dev.01095
44. Clark IE, Dodson MW, Jiang C, Cao JH, Huh JR, Seol JH, et al. Drosophila pink1 is required for mitochondrial function and interacts genetically with Parkin. Nature (2006) 441:1162–6. doi:10.1038/nature04779
45. Park J, Lee SB, Lee S, Kim Y, Song S, Kim S, et al. Mitochondrial dysfunction in Drosophila PINK1 mutants is complemented by Parkin. Nature (2006) 441:1157–61. doi:10.1038/nature04788
46. Cha G-H, Kim S, Park J, Lee E, Kim M, Lee SB, et al. Parkin negatively regulates JNK pathway in the dopaminergic neurons of Drosophila. Proc Natl Acad Sci U S A (2005) 102:10345–50. doi:10.1073/pnas.0500346102
47. Whitworth AJ, Theodore DA, Greene JC, Benes H, Wes PD, Pallanck LJ. Increased glutathione S-transferase activity rescues dopaminergic neuron loss in a Drosophila model of Parkinson’s disease. Proc Natl Acad Sci U S A (2005) 102:8024–9. doi:10.1073/pnas.0501078102
48. Gandhi S, Wood-Kaczmar A, Yao Z, Plun-Favreau H, Deas E, Klupsch K, et al. PINK1-associated Parkinson’s disease is caused by neuronal vulnerability to calcium-induced cell death. Mol Cell (2009) 33:627–38. doi:10.1016/j.molcel.2009.02.013
49. Heeman B, Van den Haute C, Aelvoet S-A, Valsecchi F, Rodenburg RJ, Reumers V, et al. Depletion of PINK1 affects mitochondrial metabolism, calcium homeostasis and energy maintenance. J Cell Sci (2011) 124:1115–25. doi:10.1242/jcs.078303
50. Akundi RS, Huang Z, Eason J, Pandya JD, Zhi L, Cass WA, et al. Increased mitochondrial calcium sensitivity and abnormal expression of innate immunity genes precede dopaminergic defects in Pink1-deficient mice. PLoS One (2011) 6:e16038. doi:10.1371/journal.pone.0016038
51. Shin J-H, Ko HS, Kang H, Lee Y, Lee Y-I, Pletinkova O, et al. PARIS (ZNF746) repression of PGC-1α contributes to neurodegeneration in Parkinson’s disease. Cell (2011) 144:689–702. doi:10.1016/j.cell.2011.02.010
52. Bonifati V, Rizzu P, Squitieri F, Krieger E, Vanacore N, van Swieten JC, et al. DJ-1(PARK7), a novel gene for autosomal recessive, early onset parkinsonism. Neurol Sci (2003) 24:159–60. doi:10.1007/s10072-003-0108-0
53. Hague S, Rogaeva E, Hernandez D, Gulick C, Singleton A, Hanson M, et al. Early-onset Parkinson’s disease caused by a compound heterozygous DJ-1 mutation. Ann Neurol (2003) 54:271–4. doi:10.1002/ana.10663
54. Canet-Avilés RM, Wilson MA, Miller DW, Ahmad R, McLendon C, Bandyopadhyay S, et al. The Parkinson’s disease protein DJ-1 is neuroprotective due to cysteine-sulfinic acid-driven mitochondrial localization. Proc Natl Acad Sci U S A (2004) 101:9103–8. doi:10.1073/pnas.0402959101
55. Zondler L, Miller-Fleming L, Repici M, Gonçalves S, Tenreiro S, Rosado-Ramos R, et al. DJ-1 interactions with α-synuclein attenuate aggregation and cellular toxicity in models of Parkinson’s disease. Cell Death Dis (2014) 5:e1350. doi:10.1038/cddis.2014.307
56. Andres-Mateos E, Perier C, Zhang L, Blanchard-Fillion B, Greco TM, Thomas B, et al. DJ-1 gene deletion reveals that DJ-1 is an atypical peroxiredoxin-like peroxidase. Proc Natl Acad Sci U S A (2007) 104:14807–12. doi:10.1073/pnas.0703219104
57. Lopert P, Patel M. Brain mitochondria from DJ-1 knockout mice show increased respiration-dependent hydrogen peroxide consumption. Redox Biol (2014) 2:667–72. doi:10.1016/j.redox.2014.04.010
58. Paisán-Ruíz C, Jain S, Evans EW, Gilks WP, Simón J, van der Brug M, et al. Cloning of the gene containing mutations that cause PARK8-linked Parkinson’s disease. Neuron (2004) 44:595–600. doi:10.1016/j.neuron.2004.10.023
59. Zimprich A, Biskup S, Leitner P, Lichtner P, Farrer M, Lincoln S, et al. Mutations in LRRK2 cause autosomal-dominant parkinsonism with pleomorphic pathology. Neuron (2004) 44:601–7. doi:10.1016/j.neuron.2004.11.005
60. Manzoni C, Mamais A, Dihanich S, Abeti R, Soutar MPM, Plun-Favreau H, et al. Inhibition of LRRK2 kinase activity stimulates macroautophagy. Biochim Biophys Acta (2013) 1833:2900–10. doi:10.1016/j.bbamcr.2013.07.020
61. Stafa K, Tsika E, Moser R, Musso A, Glauser L, Jones A, et al. Functional interaction of Parkinson’s disease-associated LRRK2 with members of the dynamin GTPase superfamily. Hum Mol Genet (2014) 23:2055–77. doi:10.1093/hmg/ddt600
62. Wang X, Yan MH, Fujioka H, Liu J, Wilson-Delfosse A, Chen SG, et al. LRRK2 regulates mitochondrial dynamics and function through direct interaction with DLP1. Hum Mol Genet (2012) 21:1931–44. doi:10.1093/hmg/dds003
63. Niu J, Yu M, Wang C, Xu Z. Leucine-rich repeat kinase 2 disturbs mitochondrial dynamics via dynamin-like protein. J Neurochem (2012) 122:650–8. doi:10.1111/j.1471-4159.2012.07809.x
64. Su Y-C, Qi X. Inhibition of excessive mitochondrial fission reduced aberrant autophagy and neuronal damage caused by LRRK2 G2019S mutation. Hum Mol Genet (2013) 22:4545–61. doi:10.1093/hmg/ddt301
65. Papkovskaia TD, Chau K-Y, Inesta-Vaquera F, Papkovsky DB, Healy DG, Nishio K, et al. G2019S leucine-rich repeat kinase 2 causes uncoupling protein-mediated mitochondrial depolarization. Hum Mol Genet (2012) 21:4201–13. doi:10.1093/hmg/dds244
66. Yakhine-Diop SMS, Bravo-San Pedro JM, Gómez-Sánchez R, Pizarro-Estrella E, Rodríguez-Arribas M, Climent V, et al. G2019S LRRK2 mutant fibroblasts from Parkinson’s disease patients show increased sensitivity to neurotoxin 1-methyl-4-phenylpyridinium dependent of autophagy. Toxicology (2014) 324:1–9. doi:10.1016/j.tox.2014.07.001
67. Alegre-Abarrategui J, Christian H, Lufino MMP, Mutihac R, Venda LL, Ansorge O, et al. LRRK2 regulates autophagic activity and localizes to specific membrane microdomains in a novel human genomic reporter cellular model. Hum Mol Genet (2009) 18:4022–34. doi:10.1093/hmg/ddp346
68. Tong Y, Yamaguchi H, Giaime E, Boyle S, Kopan R, Kelleher RJ, et al. Loss of leucine-rich repeat kinase 2 causes impairment of protein degradation pathways, accumulation of alpha-synuclein, and apoptotic cell death in aged mice. Proc Natl Acad Sci U S A (2010) 107:9879–84. doi:10.1073/pnas.1004676107
69. Orenstein SJ, Kuo S-H, Tasset I, Arias E, Koga H, Fernandez-Carasa I, et al. Interplay of LRRK2 with chaperone-mediated autophagy. Nat Neurosci (2013) 16:394–406. doi:10.1038/nn.3350
70. Cuervo AM, Stefanis L, Fredenburg R, Lansbury PT, Sulzer D. Impaired degradation of mutant alpha-synuclein by chaperone-mediated autophagy. Science (2004) 305:1292–5. doi:10.1126/science.1101738
71. Martinez-Vicente M, Talloczy Z, Kaushik S, Massey AC, Mazzulli J, Mosharov EV, et al. Dopamine-modified alpha-synuclein blocks chaperone-mediated autophagy. J Clin Invest (2008) 118:777–88. doi:10.1172/JCI32806
72. Dehay B, Bové J, Rodríguez-Muela N, Perier C, Recasens A, Boya P, et al. Pathogenic lysosomal depletion in Parkinson’s disease. J Neurosci (2010) 30:12535–44. doi:10.1523/JNEUROSCI.1920-10.2010
73. Holemans T, Sørensen DM, van Veen S, Martin S, Hermans D, Kemmer GC, et al. A lipid switch unlocks Parkinson’s disease-associated ATP13A2. Proc Natl Acad Sci U S A (2015) 112:9040–5. doi:10.1073/pnas.1508220112
74. Dehay B, Ramirez A, Martinez-Vicente M, Perier C, Canron M-H, Doudnikoff E, et al. Loss of P-type ATPase ATP13A2/PARK9 function induces general lysosomal deficiency and leads to Parkinson disease neurodegeneration. Proc Natl Acad Sci U S A (2012) 109:9611–6. doi:10.1073/pnas.1112368109
75. Vidal RL, Matus S, Bargsted L, Hetz C. Targeting autophagy in neurodegenerative diseases. Trends Pharmacol Sci (2014) 35:583–91. doi:10.1016/j.tips.2014.09.002
76. Sarkar S, Ravikumar B, Floto RA, Rubinsztein DC. Rapamycin and mTOR-independent autophagy inducers ameliorate toxicity of polyglutamine-expanded huntingtin and related proteinopathies. Cell Death Differ (2009) 16:46–56. doi:10.1038/cdd.2008.110
77. Majumder S, Richardson A, Strong R, Oddo S. Inducing autophagy by rapamycin before, but not after, the formation of plaques and tangles ameliorates cognitive deficits. PLoS One (2011) 6:e25416. doi:10.1371/journal.pone.0025416
78. Miyara M, Kotake Y, Tokunaga W, Sanoh S, Ohta S. Mild MPP(+) exposure impairs autophagic degradation through a novel lysosomal acidity-independent mechanism. J Neurochem (2016) 139:294–308. doi:10.1111/jnc.13700
79. Liu K, Shi N, Sun Y, Zhang T, Sun X. Therapeutic effects of rapamycin on MPTP-induced parkinsonism in mice. Neurochem Res (2013) 38:201–7. doi:10.1007/s11064-012-0909-8
80. Cooper O, Seo H, Andrabi S, Guardia-Laguarta C, Graziotto J, Sundberg M, et al. Pharmacological rescue of mitochondrial deficits in iPSC-derived neural cells from patients with familial Parkinson’s disease. Sci Transl Med (2012) 4:141ra90. doi:10.1126/scitranslmed.3003985
81. Webb JL, Ravikumar B, Atkins J, Skepper JN, Rubinsztein DC. Alpha-synuclein is degraded by both autophagy and the proteasome. J Biol Chem (2003) 278:25009–13. doi:10.1074/jbc.M300227200
82. Heras-Sandoval D, Pérez-Rojas JM, Hernández-Damián J, Pedraza-Chaverri J. The role of PI3K/AKT/mTOR pathway in the modulation of autophagy and the clearance of protein aggregates in neurodegeneration. Cell Signal (2014) 26:2694–701. doi:10.1016/j.cellsig.2014.08.019
83. Malagelada C, Jin ZH, Jackson-Lewis V, Przedborski S, Greene LA. Rapamycin protects against neuron death in in vitro and in vivo models of Parkinson’s disease. J Neurosci (2010) 30:1166–75. doi:10.1523/JNEUROSCI.3944-09.2010
84. He C, Levine B. The Beclin 1 interactome. Curr Opin Cell Biol (2010) 22:140–9. doi:10.1016/j.ceb.2010.01.001
85. Ciechomska IA, Goemans GC, Skepper JN, Tolkovsky AM. Bcl-2 complexed with Beclin-1 maintains full anti-apoptotic function. Oncogene (2009) 28:2128–41. doi:10.1038/onc.2009.60
86. Maiuri MC, Le Toumelin G, Criollo A, Rain J-C, Gautier F, Juin P, et al. Functional and physical interaction between Bcl-X(L) and a BH3-like domain in Beclin-1. EMBO J (2007) 26:2527–39. doi:10.1038/sj.emboj.7601689
87. Sarkar S, Davies JE, Huang Z, Tunnacliffe A, Rubinsztein DC. Trehalose, a novel mTOR-independent autophagy enhancer, accelerates the clearance of mutant huntingtin and alpha-synuclein. J Biol Chem (2007) 282:5641–52. doi:10.1074/jbc.M609532200
88. He Q, Koprich JB, Wang Y, Yu W-B, Xiao B-G, Brotchie JM, et al. Treatment with trehalose prevents behavioral and neurochemical deficits produced in an AAV α-synuclein rat model of Parkinson’s disease. Mol Neurobiol (2016) 53:2258–68. doi:10.1007/s12035-015-9173-7
89. Perucho J, Casarejos MJ, Gomez A, Solano RM, de Yébenes JG, Mena MA. Trehalose protects from aggravation of amyloid pathology induced by isoflurane anesthesia in APP(swe) mutant mice. Curr Alzheimer Res (2012) 9:334–43. doi:10.2174/156720512800107573
90. Tanaka M, Machida Y, Niu S, Ikeda T, Jana NR, Doi H, et al. Trehalose alleviates polyglutamine-mediated pathology in a mouse model of Huntington disease. Nat Med (2004) 10:148–54. doi:10.1038/nm985
91. Høyer-Hansen M, Jäättelä M. Connecting endoplasmic reticulum stress to autophagy by unfolded protein response and calcium. Cell Death Differ (2007) 14:1576–82. doi:10.1038/sj.cdd.4402200
92. Fouillet A, Levet C, Virgone A, Robin M, Dourlen P, Rieusset J, et al. ER stress inhibits neuronal death by promoting autophagy. Autophagy (2012) 8:915–26. doi:10.4161/auto.19716
93. Hetz C, Chevet E, Harding HP. Targeting the unfolded protein response in disease. Nat Rev Drug Discov (2013) 12:703–19. doi:10.1038/nrd3976
94. Spencer B, Potkar R, Trejo M, Rockenstein E, Patrick C, Gindi R, et al. Beclin 1 gene transfer activates autophagy and ameliorates the neurodegenerative pathology in alpha-synuclein models of Parkinson’s and Lewy body diseases. J Neurosci (2009) 29:13578–88. doi:10.1523/JNEUROSCI.4390-09.2009
95. Pickford F, Masliah E, Britschgi M, Lucin K, Narasimhan R, Jaeger PA, et al. The autophagy-related protein beclin 1 shows reduced expression in early Alzheimer disease and regulates amyloid beta accumulation in mice. J Clin Invest (2008) 118:2190–9. doi:10.1172/JCI33585
96. Nascimento-Ferreira I, Nóbrega C, Vasconcelos-Ferreira A, Onofre I, Albuquerque D, Aveleira C, et al. Beclin 1 mitigates motor and neuropathological deficits in genetic mouse models of Machado-Joseph disease. Brain (2013) 136:2173–88. doi:10.1093/brain/awt144
97. Jaeger PA, Pickford F, Sun C-H, Lucin KM, Masliah E, Wyss-Coray T. Regulation of amyloid precursor protein processing by the Beclin 1 complex. PLoS One (2010) 5:e11102. doi:10.1371/journal.pone.0011102
Keywords: mitophagy, Parkinson disease, mitochondria, synuclein, autophagosome
Citation: Gao F, Yang J, Wang D, Li C, Fu Y, Wang H, He W and Zhang J (2017) Mitophagy in Parkinson’s Disease: Pathogenic and Therapeutic Implications. Front. Neurol. 8:527. doi: 10.3389/fneur.2017.00527
Received: 16 June 2017; Accepted: 21 September 2017;
Published: 04 October 2017
Edited by:
Graziella Madeo, National Institutes of Health (NIH), United StatesReviewed by:
Paola Bonsi, Fondazione Santa Lucia (IRCCS), ItalyCopyright: © 2017 Gao, Yang, Wang, Li, Fu, Wang, He and Zhang. This is an open-access article distributed under the terms of the Creative Commons Attribution License (CC BY). The use, distribution or reproduction in other forums is permitted, provided the original author(s) or licensor are credited and that the original publication in this journal is cited, in accordance with accepted academic practice. No use, distribution or reproduction is permitted which does not comply with these terms.
*Correspondence: Wei He, aGV3ZWluZ2RAMTI2LmNvbQ==;
Jianmin Zhang, anpoYW5nQGlibXMucHVtYy5lZHUuY24=
Disclaimer: All claims expressed in this article are solely those of the authors and do not necessarily represent those of their affiliated organizations, or those of the publisher, the editors and the reviewers. Any product that may be evaluated in this article or claim that may be made by its manufacturer is not guaranteed or endorsed by the publisher.
Research integrity at Frontiers
Learn more about the work of our research integrity team to safeguard the quality of each article we publish.