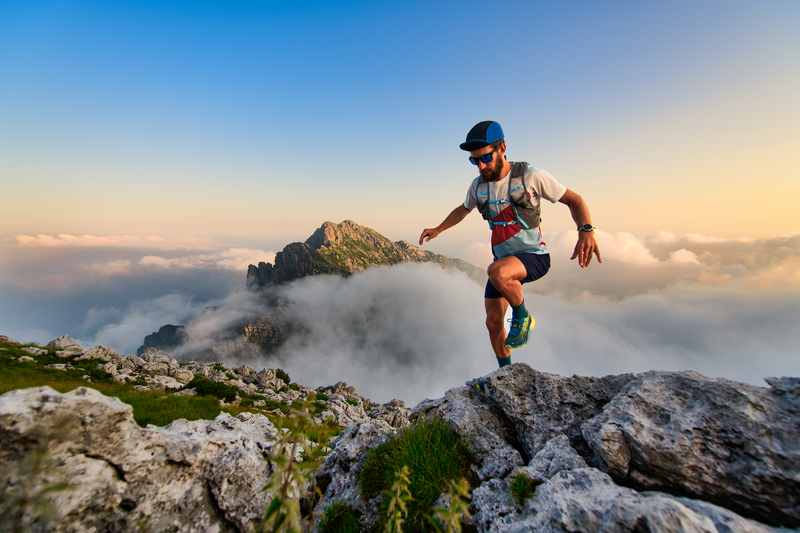
94% of researchers rate our articles as excellent or good
Learn more about the work of our research integrity team to safeguard the quality of each article we publish.
Find out more
MINI REVIEW article
Front. Neurol. , 09 June 2017
Sec. Sleep Disorders
Volume 8 - 2017 | https://doi.org/10.3389/fneur.2017.00264
This article is part of the Research Topic Intrinsic Clocks View all 12 articles
The small GTPase Ras is a universal eukaryotic cytoplasmic membrane-anchored protein, which regulates diverse downstream signal transduction pathways that play an important role in the proper functioning of neurons. Ras activity is a central regulator of structural and functional synaptic plasticity in the adult nervous system, where it channels neuronal responses to various extracellular cues allowing the organism to adapt to complex environmental stimuli. The suprachiasmatic nucleus (SCN) is the principle pacemaker of the circadian clock, and the circadian and photic regulation of Ras activity in the SCN is an important modulator of the clockwork. We have generated transgenic mouse expressing constitutively active V12-H-Ras selectively in neurons via a synapsin I promoter (synRas mice), which serves as a suitable model to study the role of neuronal Ras signaling. Modulation of Ras activity affects ERK1,2/CREB signaling and glycogen synthase kinase-3 beta expression in the SCN, which in turn modify the photoentrainment of the clock and the fine tuning the circadian period length. The main focus of this review is to offer an overview of the function of Ras signaling in the circadian rhythm and its potential role in learning and memory consolidation.
Most organisms living on earth exhibit circadian rhythm controlled by autonomous timekeeping circadian clock. The circadian oscillation of intracellular clock is driven by transcription/translation-based feedback/feedforward loops, composed of a set of clock genes, as well as kinases and phosphatases that regulate the localization and stability of the clock gene protein products. Positive regulatory elements are BMAL1 and CLOCK, which form heterodimer and regulate the rhythmic transcription of Period (Per1 and Per2) and Cryptochrome (Cry1 and Cry2) genes. The PER and CRY proteins interact and translocate to the nucleus, where they act as negative regulators inhibiting further transcriptional activation. In addition to the transcriptional regulation, posttranslational mechanisms, such as phosphorylation of core clock proteins, play an important role in the regulation of the circadian clock. The casein kinases and glycogen synthase kinase-3 beta (GSK3β) have a critical function in the control of circadian period length by phosphorylating several core clock proteins, regulating their degradation, protein stability, and nuclear translocation (1–5).
In mammals, the circadian master pacemaker is located in the suprachiasmatic nucleus (SCN) of the ventral hypothalamus (6, 7). The SCN synchronize numerous biochemical, physiological, and behavioral processes in the peripheral organs with an approximate 24 h periodicity. An important feature of circadian clockwork is the ability to be reset by light, thus, allowing animals to adjust their biological rhythms to changes in the length of daytime and nighttime (6).
Recently, we demonstrated that the circadian and photic activation of the small GTPase Ras is an important modulator of the clockwork in the SCN. Ras activity fine tunes the period length and modulates photoentrainment of the circadian clock (8). The main focus of this review is to offer an overview of the function of Ras signaling in the circadian rhythm and its potential role in learning and memory consolidation.
Ras is a universal eukaryotic intracellular membrane-anchored protein, which cycles between inactive GDP-bound and the signaling competent GTP-bound conformation. Several extracellular signals from multiple receptor types and intracellular second messengers converge onto the activation of Ras, including neurotrophin tyrosine kinase receptors, G-protein-coupled receptors, and local increase of intracellular Ca2+ concentration or Ca-calmodulin kinase II, resulting in the activation of NMDA receptors or voltage-gated Ca2+ channels (9, 10). Ras once activated transduces signals to several signaling pathways, including the major mitogen-activated protein kinase (MAPK)/extracellular-regulated kinase (ERK) cascade and phosphatidylinositol-3 kinase/Akt pathway. Ras plays a central role as a regulator of structural and functional synaptic plasticity in the adult mammalian brain modulating neuronal architecture and synaptic connectivity and tuning synaptic efficacy (11–14).
In order to study the role of Ras and its specific downstream effectors, we have generated a transgenic mouse model, which expresses constitutively active V12-H-Ras selectively in neurons via synapsin I promoter (synRas mice) (15). The synRas mice have brain hypertrophy, which results from an increased cell size and changed morphology of the pyramidal neurons (14, 15). The constitutively activated Ras increases the dendritic length, complexity, and spine density leading to a change in synaptic connectivity in the synRas mice cortex (12–14, 16). The investigation of the signal transduction in the synRas neurons showed that the expression of the constitutively activated V12-H-Ras leads to drastic increase of Ras activity and corresponding elevation of the phosphorylation level of MAPK (ERK1,2) in the cortex and hippocampus. No such changes have been observed in PI(3)K/Akt activity in adult synRas mice (15). In addition, we found increased total expression level of GSK3β (17), which might be result of enhanced Ras–MAPK signaling and ETS-p300 transcriptional complex activation (18). Furthermore, specific increases of pCREB and brain-derived neurotrophic factor (BDNF) levels in the cortex of synRas mice during the developmental stages—postnatal day 7—have been described (19).
The potential involvement of Ras signaling in the regulation of circadian clock has been proposed in numerous studies (8, 20–27). The small GTPase Ras appears to be the major effector of BDNF-mediated signaling and one of the main upstream regulators of ERK pathway resulting in elevated levels of CREB phosphorylation (19) (Figure 1). Indeed, the activation of MAPK pathway and particularly ERK1,2 and its coupling to the activation of transcription factors Elk-1 and CREB (28, 29) is an important molecular mechanism for photoentrainment of the SCN (Figure 1). In vivo studies have shown that inhibition of ERK1,2 in mouse SCN attenuates both the phase shifting effects of light (28, 30) and immediate early gene expression (31). BDNF and its receptor, TrkB, are also necessary for photic resetting. BDNF protein levels oscillate in the SCN with high levels at night, when photic stimulation and glutamate can reset the circadian clockwork (32). The inhibition of TrkB receptors blocks photic- and glutamate-induced clock resetting (33, 34).
Figure 1. Schematic outline of intracellular Ras signaling pathways in the suprachiasmatic nucleus (SCN) regulating circadian clockwork. Solid lines show the signal pathways observed in the SCN, and broken lines indicate hypothetical pathways observed in other neuronal systems. Glutamate/NMDA and brain-derived neurotrophic factor (BDNF)/TrkB are the major ligand–receptor systems within SCN involved in the light-induced phase shifting circadian clock. The light stimuli at night induce glutamate and BDNF release, which result in activation of NMDA receptors (with a subsequent influx of Ca2+, activating the Ca2+-calmodulin kinase II) and TrkB receptor that in turn stimulates Ras. Ras is also negatively regulated by the circadian protein SCN circadian oscillatory protein (SCOP). Ras activates ERK1,2 pathway, which couples to transcriptional factors CREB and Elk-1 phosphorylation, that regulate the transcription of the immediate early genes sFos, JunB, and EGR1, clock protein Per1, the regulator of ERK1,2 pathway MAPK phosphatase 1 (MKP-1). Enhanced Ras signaling via ERK1,2 also activates ETS-p300 transcriptional complex, which in turn regulates circadian clock proteins modulator glycogen synthase kinase-3 beta (GSK-3β). Other abbreviations are explained in manuscript. Please note: Ras downstream effector pathways other than RAF kinase, such as PI3 kinase and Ral/GDF have been omitted for reasons of simplicity and lack of specific information in the SCN.
Consistently, photic stimulation at early and late subjective night activates Ras in the SCN (8) and Ras activation correlates with the length of the light exposure (20), suggesting a direct involvement of Ras in the signaling pathways, coupling photic input to the SCN clock. The light stimuli induce glutamate release from the nerve terminals of the retino-hypothalamic tract, which results in activation of NMDA receptors with a subsequent influx of Ca2+ (35, 36), activating the Ca2+-calmodulin kinase II that in turn stimulates Ras (9, 10) (Figure 1).
Direct evidences for the involvement of Ras in the molecular mechanisms that adjust the circadian clock to the light/dark cycle come from the synRas mice (8, 25). The enhanced Ras activation in the SCN of synRas mice leads to potentiation of the light-induced phase delays at early night and total inhibition of the light-induced phase advances at late night of spontaneous locomotor activity (8). The magnitude of Ras-regulated ERK phosphorylation correlates with the extent of the phase delays at early subjective night—with stronger ERK activation leading to larger phase delays in circadian behavior (20, 37). ERK1,2 phosphorylates p90 ribosomal S6 kinase, which in turn phosphorylates CREB, required for the photic resetting of the SCN (38, 39). In addition, ERK1,2/CREB pathway couples light to immediate early genes expression c-Fos, a robust marker of SCN activation by photic stimuli, and the induction of the clock gene PER1 (31, 40, 41). Therefore, the enhanced activation of the Ras/ERK1,2/CREB pathway in the SCN of synRas mice at early subjective night leads to increased phase delays and enhanced photic induction of c-Fos protein expression in the SCN. Though several reports have shown that the photic stimulation of ERK1,2/CREB phosphorylation is an essential event for the clock photoentrainment, the activation of this pathway is not sufficient to induce c-Fos expression and phase advance the clock of synRas mice at late subjective night (8, 37). Thus, the enhanced basal levels of activation of Ras/ERK in the SCN of synRas mice at early subjective night phase delay the circadian clock and compensate the photic-induced resetting in the late subjective night.
Several reports demonstrated circadian oscillation of Ras activity in various brain regions and peripheral organs, including chick pineal gland (23), mouse hippocampus (22), liver (26), and SCN (8). Potential regulator of Ras activity in the brain is the SCN circadian oscillatory protein (SCOP) (42). The expression of SCOP reach peak levels during late subjective night to inhibit the Ras/ERK pathway by binding to the nucleotide-free state of Ras and preventing the binding of GTP (42, 43) (Figure 1). Furthermore, Ras is one of the main targets for neurotrophins (44). BDNF mRNA and protein levels show a circadian oscillation in different regions of the brain (32, 33, 45–48). Given that multiple extracellular signals such as growth factors and cytokines can stimulate Ras activation in a context-dependent manner, circadian oscillations of circulating humoral factors may lead to rhythmic Ras activation in the liver.
Numerous studies support a model that the circadian activation of ERK1,2 is regulated by the oscillating activation of Ras via the classical Ras–MAPK pathway, which is commonly involved in numerous intracellular events. The Ras-mediated regulation of ERK1,2 activity is conserved mechanism used in many clock-containing tissues, such as the mammalian SCN, as well as in other regions, like hippocampus, pineal gland, and liver (8, 22, 23, 26, 30). Indeed, the data from synRas mice show that the enhanced Ras activity increases ERK1,2 phosphorylation at early subjective night (8). However, the circadian regulation of pERK1,2 levels was preserved, as result of the rhythmic oscillation of the endogenous Ras and the rhythmic expression of MAPK phosphatase 1 (MKP-1) (8, 20) (Figure 1). MKP-1 shows circadian oscillation with the peak time at night in mouse liver and the SCN (8, 26).
It has been recently shown that modulation of Ras activity affects the period length (τ) of circadian oscillation (8). Enhanced activation of Ras in the SCN of synRas mice results of shortening of τ, while in vitro inhibition of Ras activity lengthens the circadian oscillation of BMAL1 promoter-driven luciferase activity (8). By contrast, inducible overexpression of Ras in cancer cell lines disrupts the circadian clock enhances the circadian period, while Ras inhibition leads to a shortening of period length, as mathematically predicted by simulations of BMAL1-mediated transcription (24). However, the mechanism of Ras-mediated modulation of the circadian period length is not well investigated yet. Though, the fine tuning of the molecular clock might have a tumor suppressive role in Ras-driven lung cancer (49).
Within the regulation of τ, phosphorylation of core clock proteins plays an important role, as it determines their stability and degradation (1–3). GSK3β acts as one of the upstream kinases phosphorylating several clock proteins, such as CLOCK, BMAL1, PER 2, Rev-erbα, and CRY2 (1, 4, 5, 50–53) (Figure 1). Enhanced Ras activity results in high total protein expression and low levels of deactivating phosphorylation of GSK3β in the SCN (8), as well as increased GSK3β activity in the cortex of synRas mice (17). Moreover, the inhibition of Ras activity decreases protein expression and increases inhibitory phosphorylation level of GSK3β in vitro (8). Thus, the increased GSK3β activity leads to a shortening, whereas a decreased function leads to substantial lengthening, of the circadian period length (1, 51, 52). By contrast, other studies show that GSK3β inhibition shortens the circadian period in vitro (50), while its chronic activation lengthens the period of mice (54). Interestingly, Ras-mediated regulation of τ may also be a result of the direct influence of Ras on the MAPK pathway. Reduction of MAPK signaling by deletion of MSK1, a target kinase of ERK1,2, results in a lengthened period of circadian behavior (55). In addition, downregulation of ERK1,2 activity inhibits the rhythm and dampens the basal level of the expression of several clock genes (56). Taken together, these data suggest that changes in GTP-Ras levels influence τ via modulation of ERK1,2 and GSK3β activity.
The circadian clocks regulate various neural functions, including cognitive performance. Several studies have demonstrated diurnal modulation of learning and memory in different paradigms, such as Morris water maze task (57), novel object recognition task (58), and fear-related tasks (59). Many investigators have linked Ras and ERK1/2 to learning and memory, since temporal modulation of ERK1/2 activation by Ras is known to play a critical role in several forms of neuroplasticity (60). Spatial and declarative memories are processed in the hippocampus (61), where Ras/MAPK pathway and the downstream CREB transcriptional pathway play an important role. Indeed, it is reported that Ras, ERK, and CREB activities show daily (basal) fluctuations in the mouse hippocampus (22). It has been recently shown that the consolidation of long-term recognition memory is a circadian-regulated process, mediated by the Ras-inhibitory protein SCOP (62). On the other hand, synRas mice showed impaired spatial short-term memory associated also with a reduced proliferation of newborn cells in the dentate gyrus of the hippocampus (63, 64) and decreased short-term recognition memory (65, 66). All these studies suggest that modulation of Ras activity is critical for memory performance, but a question remains as to whether and how circadian regulation of Ras is associated in this process.
Several reports suggest that a disordered circadian system is implicated in the etiology and symptomatology of many psychiatric disorders. Interestingly, the therapeutic action of lithium, an effective mood stabilizer for bipolar affective disorder, may be related to direct effects on the circadian clock via the inhibition of GSK-3β (51, 67). Although this enzyme has a number of functions that could potentially mediate the therapeutic effects of lithium (68), one possibility is via its function as a central regulator of the circadian clock. Consistently, it has been shown recently that activation of GSK-3β may link to the activity in the SCN neurons by regulating their persistent sodium currents (69). However, in order to understand how timing of action potentials is coupled to the pacemaker activity in the SCN, it still needs to be investigated how Ras signaling encodes electrical activity specifically in the SCN neurons.
The small GTPase Ras activity plays a role as a central regulator of structural and functional synaptic plasticity in the nervous system, where it mediates neuronal responses to various extracellular cues allowing the organism to adapt to complex environmental stimuli. Thus, the circadian and photic regulation of Ras activity in the SCN is an important modulator of the clockwork influencing the light-induced phase resetting of the clock and fine tuning the circadian period length. Furthermore, the circadian modulation of Ras signaling might have potential role in memory consolidation and mood regulation (Figure 2). However, the involvement of Ras-controlled GSK-3β expression and its mechanism of regulation in the pathophysiology of bipolar disorder still remain to be investigated.
Figure 2. The role of Ras in the regulation of circadian clockwork and learning and memory. The circadian and photic regulation of Ras activity in the suprachiasmatic nucleus modulates the light-induced phase resetting of the clock and fine tunes the circadian period length. The circadian modulation of Ras signaling might have potential role in learning and memory.
TS wrote the manuscript with assistance from RH.
The authors declare that the research was conducted in the absence of any commercial or financial relationships that could be construed as a potential conflict of interest.
TS is supported by German Research Council (DFG) grant SE 2666/2-1. RH is supported by RUBION providing animal facilities.
1. Harada Y, Sakai M, Kurabayashi N, Hirota T, Fukada Y. Ser-557-phosphorylated mCRY2 is degraded upon synergistic phosphorylation by glycogen synthase kinase-3 beta. J Biol Chem (2005) 280:31714–21. doi: 10.1074/jbc.M506225200
2. Kloss B, Price JL, Saez L, Blau J, Rothenfluh A, Wesley CS, et al. The Drosophila clock gene double-time encodes a protein closely related to human casein kinase Iepsilon. Cell (1998) 94:97–107. doi:10.1016/S0092-8674(00)81225-8
3. Price JL, Blau J, Rothenfluh A, Abodeely M, Kloss B, Young MW. Double-time is a novel Drosophila clock gene that regulates PERIOD protein accumulation. Cell (1998) 94:83–95. doi:10.1016/S0092-8674(00)81224-6
4. Spengler ML, Kuropatwinski KK, Schumer M, Antoch MP. A serine cluster mediates BMAL1-dependent CLOCK phosphorylation and degradation. Cell Cycle (2009) 8(24):4138–46. doi:10.4161/cc.8.24.10273
5. Yin L, Wang J, Klein PS, Lazar MA. Nuclear receptor Rev-erbalpha is a critical lithium-sensitive component of the circadian clock. Science (2006) 311(5763):1002–5. doi:10.1126/science.1121613
6. Albrecht U. Timing to perfection: the biology of central and peripheral circadian clocks. Neuron (2012) 74:246–60. doi:10.1016/j.neuron.2012.04.006
7. Reppert SM, Weaver DR. Coordination of circadian timing in mammals. Nature (2002) 418:935–41. doi:10.1038/nature00965
8. Serchov T, Jilg A, Wolf CT, Radtke I, Stehle JH, Heumann R. Ras activity oscillates in the mouse suprachiasmatic nucleus and modulates circadian clock dynamics. Mol Neurobiol (2016) 53:1843–55. doi:10.1007/s12035-015-9135-0
9. Farnsworth CL, Freshney NW, Rosen LB, Ghosh A, Greenberg ME, Feig LA. Calcium activation of Ras mediated by neuronal exchange factor Ras-GRF. Nature (1995) 376:524–7. doi:10.1038/376524a0
10. Wang JQ, Tang Q, Parelkar NK, Liu Z, Samdani S, Choe ES, et al. Glutamate signaling to Ras-MAPK in striatal neurons: mechanisms for inducible gene expression and plasticity. Mol Neurobiol (2004) 29:1–14. doi:10.1385/MN:29:1:01
11. Zhu JJ, Qin Y, Zhao M, Van Aelst L, Malinow R. Ras and Rap control AMPA receptor trafficking during synaptic plasticity. Cell (2002) 110:443–55. doi:10.1016/S0092-8674(02)00897-8
12. Arendt T, Gartner U, Seeger G, Barmashenko G, Palm K, Mittmann T, et al. Neuronal activation of Ras regulates synaptic connectivity. Eur J Neurosci (2004) 19:2953–66. doi:10.1111/j.0953-816X.2004.03409.x
13. Gartner U, Alpar A, Reimann F, Seeger G, Heumann R, Arendt T. Constitutive Ras activity induces hippocampal hypertrophy and remodeling of pyramidal neurons in synRas mice. J Neurosci Res (2004) 77:630–41. doi:10.1002/jnr.20194
14. Gartner U, Alpar A, Seeger G, Heumann R, Arendt T. Enhanced Ras activity in pyramidal neurons induces cellular hypertrophy and changes in afferent and intrinsic connectivity in synRas mice. Int J Dev Neurosci (2004) 22:165–73. doi:10.1016/j.ijdevneu.2004.02.001
15. Heumann R, Goemans C, Bartsch D, Lingenhohl K, Waldmeier PC, Hengerer B, et al. Transgenic activation of Ras in neurons promotes hypertrophy and protects from lesion-induced degeneration. J Cell Biol (2000) 151:1537–48. doi:10.1083/jcb.151.7.1537
16. Alpar A, Gartner U, Seeger G, Hartig W, Brauer K, Arendt T. Constitutive expression of p21H-ras(Val12) in pyramidal neurons results in reorganization of mouse neocortical afferents. J Neurobiol (2004) 60:263–74. doi:10.1002/neu.20019
17. Holzer M, Gartner U, Klinz FJ, Narz F, Heumann R, Arendt T. Activation of mitogen-activated protein kinase cascade and phosphorylation of cytoskeletal proteins after neurone-specific activation of p21ras. I. Mitogen-activated protein kinase cascade. Neuroscience (2001) 105:1031–40. doi:10.1016/S0306-4522(01)00245-7
18. Zhang JS, Koenig A, Harrison A, Ugolkov AV, Fernandez-Zapico ME, Couch FJ, et al. Mutant K-Ras increases GSK-3beta gene expression via an ETS-p300 transcriptional complex in pancreatic cancer. Oncogene (2011) 30(34):3705–15. doi:10.1038/onc.2011.90
19. Hansen HH, Briem T, Dzietko M, Sifringer M, Voss A, Rzeski W, et al. Mechanisms leading to disseminated apoptosis following NMDA receptor blockade in the developing rat brain. Neurobiol Dis (2004) 16:440–53. doi:10.1016/j.nbd.2004.03.013
20. Butcher GQ, Lee B, Obrietan K. Temporal regulation of light-induced extracellular signal-regulated kinase activation in the suprachiasmatic nucleus. J Neurophysiol (2003) 90:3854–63. doi:10.1152/jn.00524.2003
21. Cheng P, He Q, He Q, Wang L, Liu Y. Regulation of the Neurospora circadian clock by an RNA helicase. Genes Dev (2005) 19:234–41. doi:10.1101/gad.1266805
22. Eckel-Mahan KL, Phan T, Han S, Wang H, Chan GC, Scheiner ZS, et al. Circadian oscillation of hippocampal MAPK activity and cAmp: implications for memory persistence. Nat Neurosci (2008) 11:1074–82. doi:10.1038/nn.2174
23. Hayashi Y, Sanada K, Fukada Y. Circadian and photic regulation of MAP kinase by Ras- and protein phosphatase-dependent pathways in the chick pineal gland. FEBS Lett (2001) 491:71–5. doi:10.1016/S0014-5793(01)02153-6
24. Relogio A, Thomas P, Medina-Perez P, Reischl S, Bervoets S, Gloc E, et al. Ras-mediated deregulation of the circadian clock in cancer. PLoS Genet (2014) 10:e1004338. doi:10.1371/journal.pgen.1004338
25. Serchov T, Heumann R. Constitutive activation of ras in neurons: implications for the regulation of the mammalian circadian clock. Chronobiol Int (2006) 23:191–200. doi:10.1080/07420520500521970
26. Tsuchiya Y, Minami I, Kadotani H, Todo T, Nishida E. Circadian clock-controlled diurnal oscillation of Ras/ERK signaling in mouse liver. Proc Jpn Acad Ser B Phys Biol Sci (2013) 89:59–65. doi:10.2183/pjab.89.59
27. Weber F, Hung HC, Maurer C, Kay SA. Second messenger and Ras/MAPK signalling pathways regulate CLOCK/CYCLE-dependent transcription. J Neurochem (2006) 98:248–57. doi:10.1111/j.1471-4159.2006.03865.x
28. Coogan AN, Piggins HD. Circadian and photic regulation of phosphorylation of ERK1/2 and Elk-1 in the suprachiasmatic nuclei of the Syrian hamster. J Neurosci (2003) 23:3085–93.
29. Obrietan K, Impey S, Smith D, Athos J, Storm DR. Circadian regulation of cAMP response element-mediated gene expression in the suprachiasmatic nuclei. J Biol Chem (1999) 274:17748–56. doi:10.1074/jbc.274.25.17748
30. Butcher GQ, Doner J, Dziema H, Collamore M, Burgoon PW, Obrietan K. The p42/44 mitogen-activated protein kinase pathway couples photic input to circadian clock entrainment. J Biol Chem (2002) 277:29519–25. doi:10.1074/jbc.M203301200
31. Dziema H, Oatis B, Butcher GQ, Yates R, Hoyt KR, Obrietan K. The ERK/MAP kinase pathway couples light to immediate-early gene expression in the suprachiasmatic nucleus. Eur J Neurosci (2003) 17:1617–27. doi:10.1046/j.1460-9568.2003.02592.x
32. Liang FQ, Sohrabji F, Miranda R, Earnest B, Earnest D. Expression of brain-derived neurotrophic factor and its cognate receptor, TrkB, in the rat suprachiasmatic nucleus. Exp Neurol (1998) 151:184–93. doi:10.1006/exnr.1998.6804
33. Liang FQ, Allen G, Earnest D. Role of brain-derived neurotrophic factor in the circadian regulation of the suprachiasmatic pacemaker by light. J Neurosci (2000) 20:2978–87.
34. Michel S, Clark JP, Ding JM, Colwell CS. Brain-derived neurotrophic factor and neurotrophin receptors modulate glutamate-induced phase shifts of the suprachiasmatic nucleus. Eur J Neurosci (2006) 24:1109–16. doi:10.1111/j.1460-9568.2006.04972.x
35. Ding JM, Chen D, Weber ET, Faiman LE, Rea MA, Gillette MU. Resetting the biological clock: mediation of nocturnal circadian shifts by glutamate and NO. Science (1994) 266:1713–7. doi:10.1126/science.7527589
36. Tischkau SA, Gallman EA, Buchanan GF, Gillette MU. Differential cAMP gating of glutamatergic signaling regulates long-term state changes in the suprachiasmatic circadian clock. J Neurosci (2000) 20:7830–7.
37. Hainich EC, Pizzio GA, Golombek DA. Constitutive activation of the ERK-MAPK pathway in the suprachiasmatic nuclei inhibits circadian resetting. FEBS Lett (2006) 580:6665–8. doi:10.1016/j.febslet.2006.11.019
38. Butcher GQ, Lee B, Hsieh F, Obrietan K. Light- and clock-dependent regulation of ribosomal S6 kinase activity in the suprachiasmatic nucleus. Eur J Neurosci (2004) 19:907–15. doi:10.1111/j.0953-816X.2004.03155.x
39. Travnickova-Bendova Z, Cermakian N, Reppert SM, Sassone-Corsi P. Bimodal regulation of mPeriod promoters by CREB-dependent signaling and CLOCK/BMAL1 activity. Proc Natl Acad Sci U S A (2002) 99:7728–33. doi:10.1073/pnas.102075599
40. Kornhauser JM, Nelson DE, Mayo KE, Takahashi JS. Photic and circadian regulation of c-fos gene expression in the hamster suprachiasmatic nucleus. Neuron (1990) 5(2):127–34. doi:10.1016/0896-6273(90)90303-W
41. Abe H, Rusak B. Physiological mechanisms regulating photic induction of Fos-like protein in hamster suprachiasmatic nucleus. Neurosci Biobehav Rev (1994) 18(4):531–6. doi:10.1016/0149-7634(94)90007-8
42. Shimizu K, Okada M, Nagai K, Fukada Y. Suprachiasmatic nucleus circadian oscillatory protein, a novel binding partner of K-Ras in the membrane rafts, negatively regulates MAPK pathway. J Biol Chem (2003) 278:14920–5. doi:10.1074/jbc.M213214200
43. Shimizu K, Phan T, Mansuy IM, Storm DR. Proteolytic degradation of SCOP in the hippocampus contributes to activation of MAP kinase and memory. Cell (2007) 128:1219–29. doi:10.1016/j.cell.2006.12.047
44. Heumann R. Neurotrophin signalling. Curr Opin Neurobiol (1994) 4:668–79. doi:10.1016/0959-4388(94)90008-6
45. Berchtold NC, Oliff HS, Isackson P, Cotman CW. Hippocampal BDNF mRNA shows a diurnal regulation, primarily in the exon III transcript. Brain Res Mol Brain Res (1999) 71:11–22. doi:10.1016/S0169-328X(99)00137-0
46. Bova R, Micheli MR, Qualadrucci P, Zucconi GG. BDNF and trkB mRNAs oscillate in rat brain during the light-dark cycle. Brain Res Mol Brain Res (1998) 57:321–4. doi:10.1016/S0169-328X(98)00092-8
47. Dolci C, Montaruli A, Roveda E, Barajon I, Vizzotto L, Grassi Zucconi G, et al. Circadian variations in expression of the trkB receptor in adult rat hippocampus. Brain Res (2003) 994:67–72. doi:10.1016/j.brainres.2003.09.018
48. Schaaf MJ, Duurland R, de Kloet ER, Vreugdenhil E. Circadian variation in BDNF mRNA expression in the rat hippocampus. Brain Res Mol Brain Res (2000) 75:342–4. doi:10.1016/S0169-328X(99)00314-9
49. Papagiannakopoulos T, Bauer MR, Davidson SM, Heimann M, Subbaraj L, Bhutkar A, et al. Circadian rhythm disruption promotes lung tumorigenesis. Cell Metab (2016) 24(2):324–31. doi:10.1016/j.cmet.2016.07.001
50. Hirota T, Lewis WG, Liu AC, Lee JW, Schultz PG, Kay SA. A chemical biology approach reveals period shortening of the mammalian circadian clock by specific inhibition of GSK-3beta. Proc Natl Acad Sci U S A (2008) 105:20746–51. doi:10.1073/pnas.0811410106
51. Iitaka C, Miyazaki K, Akaike T, Ishida N. A role for glycogen synthase kinase-3beta in the mammalian circadian clock. J Biol Chem (2005) 280:29397–402. doi:10.1074/jbc.M503526200
52. Lavoie J, Hebert M, Beaulieu JM. Glycogen synthase kinase-3beta haploinsufficiency lengthens the circadian locomotor activity period in mice. Behav Brain Res (2013) 253:262–5. doi:10.1016/j.bbr.2013.08.001
53. Sahar S, Zocchi L, Kinoshita C, Borrelli E, Sassone-Corsi P. Regulation of BMAL1 protein stability and circadian function by GSK3beta-mediated phosphorylation. PLoS One (2010) 5:e8561. doi:10.1371/journal.pone.0008561
54. Paul JR, Johnson RL, Jope RS, Gamble KL. Disruption of circadian rhythmicity and suprachiasmatic action potential frequency in a mouse model with constitutive activation of glycogen synthase kinase 3. Neuroscience (2012) 226:1–9. doi:10.1016/j.neuroscience.2012.08.047
55. Cao R, Butcher GQ, Karelina K, Arthur JS, Obrietan K. Mitogen- and stress-activated protein kinase 1 modulates photic entrainment of the suprachiasmatic circadian clock. Eur J Neurosci (2013) 37:130–40. doi:10.1111/ejn.12028
56. Akashi M, Hayasaka N, Yamazaki S, Node K. Mitogen-activated protein kinase is a functional component of the autonomous circadian system in the suprachiasmatic nucleus. J Neurosci (2008) 28:4619–23. doi:10.1523/JNEUROSCI.3410-07.2008
57. Valentinuzzi VS, Menna-Barreto L, Xavier GF. Effect of circadian phase on performance of rats in the Morris water maze task. J Biol Rhythms (2004) 19:312–24. doi:10.1177/0748730404265688
58. Ruby NF, Hwang CE, Wessells C, Fernandez F, Zhang P, Sapolsky R, et al. Hippocampal-dependent learning requires a functional circadian system. Proc Natl Acad Sci U S A (2008) 105:15593–8. doi:10.1073/pnas.0808259105
59. Ralph MR, Sam K, Rawashdeh OA, Cain SW, Ko CH. Memory for time of day (time memory) is encoded by a circadian oscillator and is distinct from other context memories. Chronobiol Int (2013) 30:540–7. doi:10.3109/07420528.2012.754449
60. Ohno M, Frankland PW, Chen AP, Costa RM, Silva AJ. Inducible, pharmacogenetic approaches to the study of learning and memory. Nat Neurosci (2001) 4:1238–43. doi:10.1038/nn771
61. Broadbent NJ, Squire LR, Clark RE. Spatial memory, recognition memory, and the hippocampus. Proc Natl Acad Sci U S A (2004) 101:14515–20. doi:10.1073/pnas.0406344101
62. Shimizu K, Kobayashi Y, Nakatsuji E, Yamazaki M, Shimba S, Sakimura K, et al. SCOP/PHLPP1beta mediates circadian regulation of long-term recognition memory. Nat Commun (2016) 7:12926. doi:10.1038/ncomms12926
63. Manns M, Bichler Z, Leske O, Heumann R. Neuronal Ras activation inhibits adult hippocampal progenitor cell division and impairs spatial short-term memory. Genes Brain Behav (2010) 9:525–36. doi:10.1111/j.1601-183X.2010.00584.x
64. Manns M, Leske O, Gottfried S, Bichler Z, Lafenetre P, Wahle P, et al. Role of neuronal ras activity in adult hippocampal neurogenesis and cognition. Front Neurosci (2011) 5:18. doi:10.3389/fnins.2011.00018
65. Lafenetre P, Leske O, Ma-Hogemeie Z, Haghikia A, Bichler Z, Wahle P, et al. Exercise can rescue recognition memory impairment in a model with reduced adult hippocampal neurogenesis. Front Behav Neurosci (2010) 3:34. doi:10.3389/neuro.08.034.2009
66. Lafenetre P, Leske O, Wahle P, Heumann R. The beneficial effects of physical activity on impaired adult neurogenesis and cognitive performance. Front Neurosci (2011) 5:51. doi:10.3389/fnins.2011.00051
67. Gould TD, Manji HK. Glycogen synthase kinase-3: a putative molecular target for lithium mimetic drugs. Neuropsychopharmacology (2005) 30:1223–37. doi:10.1038/sj.npp.1300731
68. Quiroz JA, Singh J, Gould TD, Denicoff KD, Zarate CA, Manji HK. Emerging experimental therapeutics for bipolar disorder: clues from the molecular pathophysiology. Mol Psychiatry (2004) 9:756–76. doi:10.1038/sj.mp.4001521
Keywords: Ras, circadian, glycogen synthase kinase-3 beta, synRas mice, ERK1/2
Citation: Serchov T and Heumann R (2017) Ras Activity Tunes the Period and Modulates the Entrainment of the Suprachiasmatic Clock. Front. Neurol. 8:264. doi: 10.3389/fneur.2017.00264
Received: 13 January 2017; Accepted: 24 May 2017;
Published: 09 June 2017
Edited by:
Daniela D. Pollak, Medical University of Vienna, AustriaReviewed by:
Brian James Altman, University of Pennsylvania, United StatesCopyright: © 2017 Serchov and Heumann. This is an open-access article distributed under the terms of the Creative Commons Attribution License (CC BY). The use, distribution or reproduction in other forums is permitted, provided the original author(s) or licensor are credited and that the original publication in this journal is cited, in accordance with accepted academic practice. No use, distribution or reproduction is permitted which does not comply with these terms.
*Correspondence: Tsvetan Serchov, dHN2ZXRhbi5zZXJjaG92QHVuaWtsaW5pay1mcmVpYnVyZy5kZQ==;
Rolf Heumann, cm9sZi5oZXVtYW5uQHJ1aHItdW5pLWJvY2h1bS5kZQ==
Disclaimer: All claims expressed in this article are solely those of the authors and do not necessarily represent those of their affiliated organizations, or those of the publisher, the editors and the reviewers. Any product that may be evaluated in this article or claim that may be made by its manufacturer is not guaranteed or endorsed by the publisher.
Research integrity at Frontiers
Learn more about the work of our research integrity team to safeguard the quality of each article we publish.