- 1Bioengineering Department, Volgenau School of Engineering, George Mason University, Fairfax, VA, United States
- 2MedStar National Rehabilitation Hospital, Washington, DC, United States
- 3Interdisciplinary Program in Neuroscience, Georgetown University, Washington, DC, United States
Motor practice is an essential part of upper limb motor recovery following stroke. To be effective, it must be intensive with a high number of repetitions. Despite the time and effort required, gains made from practice alone are often relatively limited, and substantial residual impairment remains. Using non-invasive brain stimulation to modulate cortical excitability prior to practice could enhance the effects of practice and provide greater returns on the investment of time and effort. However, determining which cortical area to target is not trivial. The implications of relevant conceptual frameworks such as Interhemispheric Competition and Bimodal Balance Recovery are discussed. In addition, we introduce the STAC (Structural reserve, Task Attributes, Connectivity) framework, which incorporates patient-, site-, and task-specific factors. An example is provided of how this framework can assist in selecting a cortical region to target for priming prior to reaching practice poststroke. We suggest that this expanded patient-, site-, and task-specific approach provides a useful model for guiding the development of more successful approaches to neuromodulation for enhancing motor recovery after stroke.
Poststroke Arm Impairment
Upper limb motor impairment following stroke is highly prevalent and often persists even after intensive rehabilitation efforts (1–4). It is also one of the most disabling of stroke sequela, limiting functional independence and precluding return to work and other roles (5).
Upper extremity motor control relies heavily on input transmitted via the corticospinal tract (CST). The CST descends through the posterior limb of the internal capsule, an area vulnerable to middle cerebral artery stroke and in which CST fibers are densely packed. Thus, even a small lesion in this location can have devastating effects on motor function (6–9). A loss of voluntary wrist and finger extension is particularly common and appears to be related to the extent of CST damage (10). There is also evidence that those who retain wrist extension and have considerable CST sparing are more likely to be responsive to existing therapies (7, 8, 11).
However, even individuals who lack voluntary wrist and finger extension often retain some ability to move the shoulder and elbow. Unfortunately, only a few stereotyped movement patterns can be performed and these are often not functional. The combination of shoulder flexion with elbow extension that is required for most functional reaching tasks, for example, is frequently lost. Nevertheless, previous studies have demonstrated that reaching practice with trunk restraint can improve unconstrained reaching ability, even in patients who lack wrist and finger extension (12–15). Still, a great deal of time and effort is required and the improvements are relatively small.
Non-Invasive Brain Stimulation
Non-invasive brain stimulation offers a potential method of enhancing the effects of practice and thus giving patients greater returns on their investment of time and effort. Approaches to non-invasive brain stimulation are rapidly expanding but generally fall into two major categories: transcranial magnetic stimulation (TMS) and transcranial electrical stimulation [TES; see Ref. (16) for overview of non-invasive techniques for neuromodulation]. These modalities are applied to the scalp overlying a specific cortical area that is being targeted. The level of spatial specificity varies depending on many factors including the modality used (TMS is generally more precise than TES), the stimulation intensity (higher intensity results in a more widespread effect), and the architecture of the underlying tissue. The excitability of the underlying pool of neurons can be modulated by varying stimulation parameters such as the frequency and temporal pattern of the stimuli. Therefore, stimulation can be used to temporarily inhibit or facilitate the underlying cortical area for a sustained period of time after the stimulation ends (usually 20–40 min). In this way, non-invasive brain stimulation could be used to “prime” relevant cortical areas before a bout of practice, potentially enhancing the effects of practice. However, there is little guidance for how such cortical sites might be selected and in which direction (inhibition or facilitation) their activity should be modulated. Conceptual models that could offer such guidance are considered below.
Mechanistic Models to Guide Neuromodulation
Vicariation
Vicariation refers to an intact cortical area taking on a function previously performed by the lesioned area. The famous neurologist, Hughlings Jackson, suggested that recovery from brain injury may be dependent on the “re-weighting” of intact areas to increase their role in controlling the affected limb (17, 18). The neural reorganization that occurs following stroke could be considered a kind of vicariation, in which areas spared by the lesion take on (or increase their involvement in) functions previously performed by the lesioned area. Perilesional areas, for example, have been shown to undergo this sort of transformation (19–23).
Another possible site for a vicariation-like process is homologous areas of the non-lesioned hemisphere. There is evidence, for example, that the right-hemisphere homolog to Broca’s area could contribute to poststroke recovery from aphasia (24). Interestingly, in the intact motor system, there is evidence of compensation between left and right dorsal premotor cortex (PMd). When rTMS inhibition of left PMd produced no effect on right hand motor performance, functional imaging confirmed suppression of left PMd activity, but also revealed increased activation of right PMd, ipsilateral to the moving hand (25). Only when this compensatory ipsilateral activation was also disrupted were decrements in task performance observed. This suggests that ipsilateral PMd increased its contribution to the movement when the function of contralateral PMd was compromised.
Could an analogous process occur following stroke? Perhaps the disruption of function induced by the stroke could trigger a compensatory vicariation-like process in homologous areas of the non-lesioned hemisphere. This possibility remains controversial, however, as it is difficult to rectify with the many studies that have demonstrated a negative relationship between non-lesioned hemisphere activation and motor recovery (26–29).
Interhemispheric Competition
The notion that non-lesioned hemisphere activity has a detrimental effect on motor recovery is supported not by a model of interhemispheric compensation, as described above, but of interhemispheric competition. Central to this model is the phenomenon of interhemispheric inhibition. Homologous primary motor cortex (M1) representations are interconnected via dense transcallosal projections that have primarily inhibitory effects. Thus, a motor representation in one hemisphere tends to inhibit its contralateral homolog, and vice versa, in a relatively balanced manner (30, 31). The interhemispheric competition framework suggests that the negative relationship between non-lesioned hemisphere activation and motor recovery observed poststroke is due to an imbalance in interhemispheric inhibition (32). Damage induced by the stroke is thought to result in a loss of interhemispheric inhibition from the lesioned to the non-lesioned hemisphere. Because of this disinhibition, the non-lesioned hemisphere is thought to have abnormally high excitability; which in turn results in stronger interhemispheric inhibition of the lesioned hemisphere. Numerous intervention strategies focused on “rebalancing” this interhemispheric disparity (e.g., decreasing excitability of the non-lesioned hemisphere and/or increasing excitability of the lesioned hemisphere) have been tested, with mixed results (33–38).
Although this model has proven useful for guiding initial approaches to neuromodulation for motor priming poststroke, it does have some notable limitations. First, it relies heavily on data from and characteristics of M1. It is not clear if or how findings from M1 may apply to the physiological interactions and characteristics of other cortical motor areas. The potential role of the non-lesioned hemisphere in recovery is likely dependent on the site, since it is unlikely that all areas of the non-lesioned hemisphere are over-inhibiting their contralateral counterparts. In the study of bilateral premotor cortices referenced above (25), for example, when premotor activity in one hemisphere was suppressed, the increased activity in the opposite “non-lesioned” hemisphere site did not impair performance, but was shown to be supporting it. Thus, the interhemispheric competition model may be less useful when considering the roles of cortical areas other than M1.
Bimodal Balance Recovery
The Bimodal Balance Recovery Model (16) holds that the relevance of the interhemispheric competition framework depends on the amount of “structural reserve,” or the proportion of the lesioned hemisphere motor system that remains intact following the stroke. According to this model, for those with enough sparing of the lesioned hemisphere motor system, interhemispheric rebalancing may be the best model for driving recovery. However, in those with little structural reserve in the lesioned hemisphere, it is suggested that vicariation within non-lesioned hemisphere motor areas may provide the best option for supporting recovery (34, 39, 40). The concept of “structural reserve” is likely to be captured largely by CST integrity, and indeed, CST integrity is a strong predictor of response to existing treatments and overall recovery (7, 8, 10, 11, 41).
Structural reserve, Task Attributes, Connectivity (STAC)
As suggested by the Bimodal Balance Recovery Model, CST integrity is a key consideration in selecting a cortical site to target with neuromodulatory priming prior to practice. There are two additional (and closely related) factors that we suggest should also be considered: (1) the characteristics of the motor task and (2) the projection patterns of the candidate cortical sites.
Task Attributes
For maximum benefit to be realized, it is necessary to match the targeted cortical site with not only lesion characteristics but also with the type of task that will be practiced in the subsequent treatment session. The cortical site should be selected by considering the neural network that underlies performance of the task to be practiced. Since the neural network underlying a movement varies with certain attributes of the task, it is necessary to carefully consider the attributes of the treatment task(s) that will be trained in the subsequent practice or treatment session (42–45).
One important task attribute to consider is the limb segments and corresponding muscle groups that are involved. The structural and functional characteristics of projections to, for example, proximal and distal or flexor and extensor muscles, can differ (46–48). Perhaps most notably, there are more direct corticospinal projections from M1 to distal muscles than to proximal muscles of the contralateral arm (49, 50), and M1 more strongly influences distal movements (48). Cortical maps of proximal vs. distal limb segments, while overlapping, are still clearly segregated; and representations for distal muscles are larger (51–53) and produce stronger facilitation (54, 55) than those for proximal muscles. Projections to proximal muscles, on the other hand, are more polysynaptic, with cortical projections often synapsing initially in the brainstem or intermediate zone of the spinal cord. In addition, cortical contributions to proximal arm movements such as reaching, originate from a widespread network that includes M1, premotor cortex, supplemental motor area, posterior parietal cortex, and brainstem nuclei. Cortical inputs to proximal muscles are also less strongly lateralized to the contralateral hemisphere than are those controlling distal movements (56, 57), and proximal arm movements thus receive a greater contribution from the ipsilateral hemisphere (58). Other reports have shown differences in the physiological characteristics of proximal vs. distal representations (59), including the physiological mechanisms by which use-dependent reorganization occurs (60).
Notably, much of what we currently understand about mechanisms of poststroke motor recovery in humans is limited to distal hand muscles. Cortical sites contributing to recovery at more proximal joints and muscles are likely to differ from those that underlie recovery of finger movements, due in large part to differences in the sources of neural input to proximal vs. distal muscles outlined above.
Other task attributes to consider include force, speed, or dexterity requirements; whether unimanual or bimanual task performance is required; the level of visual, haptic, or other sensory feedback processing required; whether there is an action selection component, a need to inhibit certain movements, or to produce a specific sequence of movements or movements that are timed to an external cue; to name a few. The neural networks underlying task performance differ based on these attributes, making them an important consideration when selecting a target site for neuromodulatory priming. Prior to practicing a task meant to improve, for example, stereognosis or haptic perception, one might target somatosensory cortex or other sensory processing areas for priming. Similarly, a task that requires precisely timed or sequential movements might suggest supplementary motor area as a target.
Connectivity
It is also necessary to consider the structural and physiological connections of candidate sites. Rather than inhibition or facilitation of the entire lesioned or non-lesioned hemisphere, the unique connectivity patterns and computational roles of specific cortical sites within each hemisphere should be considered. Information about structural connectivity is obtained from such sources as transneuronal tracer studies in animal models [e.g., Ref. (61)] and tractography analyses in humans [e.g., Ref. (62)]. Physiological or “functional” connectivity refers to pathways created through the strengthening of synaptic connections. Information about physiological connectivity can be gained by intracellular stimulation and recording studies in animal models [e.g., Ref. (54, 57)]; TMS and electro- and/or magneto-encephalography recordings [e.g., Ref. (63)], and functional and effective connectivity analyses of BOLD imaging [e.g., Ref. (64)] in humans.
Importantly, the connectivity of a candidate region includes its intra- and inter-hemispheric connectivity with other cortical regions as well as its pattern of projections to peripheral targets. One type of connectivity that is often considered is that of transcallosal connections between M1 representations, as discussed above. Note how an understanding of both structural connectivity (i.e., transcallosal projections linking homologous motor areas) and effective connectivity (i.e., the primarily inhibitory effect of activity within these projections) inform the choice for applying inhibitory or facilitatory neuromodulation to non-lesioned or lesioned hemisphere M1, respectively.
Connectivity analyses of functional neuroimaging data have demonstrated that poststroke impairments are frequently related not only to damage of specific cortical sites but also to disruption of the entire network to which those sites belong (65, 66). Intriguingly, there is emerging evidence that non-invasive priming of lesioned hemisphere M1 prior to rehabilitation treatment can prevent the development of abnormal connectivity and enhance beneficial connectivity (67, 68).
While it may not always be possible to directly affect abnormal connectivity via non-invasive priming, it is still important to understand the overall connectivity of the system so that alternative pathways may be identified. If the lesion results in a loss of facilitatory input to a key node in the network, for example, another area with facilitatory synaptic connections with that area may be considered as a possible target for neuromodulation. Similarly, modulation of one node in the network can alter connectivity patterns elsewhere within the network [e.g., Ref. (67)].
In addition to intracortical connections, in the motor system, it is critical to consider the available output pathways through which neural drive could be transmitted to the musculoskeletal system. One such consideration is the degree to which transmissions from a candidate area rely upon the structural integrity of areas affected by the stroke. In other words, to what degree does the candidate site rely upon the lesioned area to transmit the desired output? In the case of severe CST damage, for instance, targeting lesioned hemisphere motor areas may not be beneficial if their influence upon the affected limb must be transmitted via the CST. Similarly, if CST from the lesioned hemisphere is severely damaged, targeting the non-lesioned hemisphere to improve the affected arm requires a target site with relatively strong projections to the ipsilateral (affected) limb.
In summary, we suggest three main considerations in selecting a cortical site for neuromodulation prior to motor practice: Structural reserve, Task Attributes, Connectivity (STAC). For structural sparing, the key factor seems to be CST integrity; which is reflected, among other things, in voluntary wrist and finger extension. The next consideration is the attributes of the task(s) to be practiced during the subsequent activity-based session. This is important because the neural networks that contribute to the task are determined by specific task attributes. Finally, it is necessary to consider the network connections (both structural and functional) of the candidate sites, depending on the pathway we are attempting to influence.
Putting it all Together: A Cortical Perturbation Study
Using the STAC framework, we have examined the roles of different candidate brain areas in the control of goal-directed reaching movements performed by chronic stroke patients. The following provides an overview of how the STAC approach was applied and initial results from this ongoing investigation.
Structural Sparing
According to the Bimodal Balance Recovery model, in patients with greater structural reserve, the lesioned hemisphere would be the primary contributor to affected arm movement, while those with less structural reserve would rely more on the non-lesioned hemisphere (16). Structural reserve is probably best defined as the integrity of the CST. Studies in non-human primates have demonstrated that CST transection primarily results in impaired finger movements (69–71). Therefore, we defined “good” vs. “poor” structural reserve as the presence or absence, respectively, of voluntary finger extension. The presence of good vs. poor structural reserve, then, would point toward the lesioned and non-lesioned hemisphere, respectively, as possible contributors to recovery and potential sites for neuromodulation.
Task Attributes
In this case, the task is forward reaching. Key attributes of this task are that it is a targeted, goal-directed movement, performed unilaterally using proximal arm muscles and under visual guidance. Visually guided movements to specific locations in the extrapersonal space are known to involve dorsal stream structures, such as PMd (72, 73). Similarly, while M1 projects strongly to distal muscles, PMd has stronger projections to proximal arm muscles (46–48, 70, 71, 74–76). These task attributes raise the possibility of PMd as a potentially important area to target.
Connectivity of Candidate Areas
Cortical projections to proximal arm muscles originate from multiple cortical motor areas and are often bilaterally organized, in many cases, by way of synapses with brainstem motor areas (77). PMd, in particular, projects to brainstem areas involved in the coordination of visually guided reaching (78, 79). Functionally, PMd is involved in the control of visually guided reaching movements of the ipsilateral arm (57). And, as described above, there is evidence that left and right PMd can compensate for one another, and that PMd can provide necessary input to control the ipsilateral limb (25). While M1 projects most strongly to and is most active during contralateral arm movements, PMd is less strongly lateralized and, therefore, more likely to be able to influence the ipsilateral arm.
Based on these considerations, we predicted that non-lesioned hemisphere PMd would have a greater role in reaching movements of patients with poor vs. those with good structural reserve. We also anticipated that in patients with poor structural reserve, non-lesioned hemisphere PMd would have a larger role than non-lesioned hemisphere M1, given the difference in their connectivity patterns (M1: lateralized projections to distal muscles; PMd: projections to proximal arm muscles and projections to ipsilateral arm).
Patients performed a seated, visually guided reaching task to a flat contact sensor placed on a table at 80% of their maximum forward reaching distance [described in detail elsewhere, (15, 80)]. In response to a visual cue, patients were instructed to “quickly reach out to contact the target.” Notably, the target could be contacted with any part of the hand or fist; no finger movement was required. At varying intervals following the visual cue but prior to movement onset, TMS pulses (two pulses with 25 msec inter-stimulus interval) were delivered to the scalp overlying the targeted cortical site. This stimulation produced a momentary perturbation of the neural processing occurring in that area. If the targeted area was performing a task-relevant computation at the time that the perturbation was applied, this should produce an observable effect on the resulting movement when compared to trials with no perturbation.
In an initial analysis, we compared the effects of TMS perturbation applied to lesioned vs. non-lesioned hemisphere PMd in patients with good vs. poor levels of structural reserve. As described above, good vs. poor structural reserve was determined by the presence or absence of voluntary finger extension. Figure 1 displays preliminary results from 10 patients with good structural reserve and 10 with poor structural reserve.
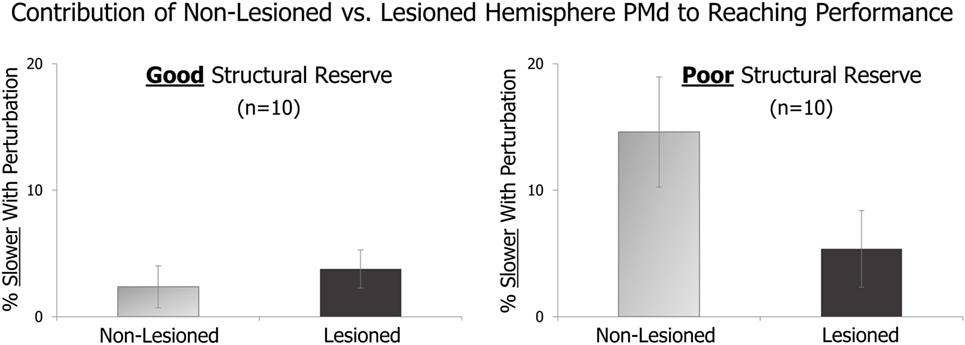
Figure 1. On randomly delivered trials, transcranial magnetic stimulation (TMS) perturbation was applied just after a “Go” cue. The effect of this pre-movement perturbation on the speed of the subsequent reaching movement is expressed relative to that in trials with no TMS perturbation. The amount of slowing due to TMS perturbation of the lesioned vs. non-lesioned hemispheres is shown for patients with good structural reserve (left) and patients with poor structural reserve (right).
Comparing the light gray bars of the figure, it appears that perturbation of non-lesioned hemisphere PMd had a larger effect on patients with poor (right side) vs. good (left side) structural reserve. This suggests that non-lesioned hemisphere PMd may have a larger role in the movements of those with poorer structural reserve. Similarly, within patients with poor structural reserve (right side of figure), the role of non-lesioned hemisphere PMd (light gray bar) appears greater than that of lesioned hemisphere PMd (dark gray bar).
Additionally, the effects of this perturbation do not appear to be generalized across the entire cortical hemisphere. Instead, the effect appears to differ depending on which cortical site within the hemisphere is targeted.
In Figure 2, a comparison between cortical sites within the same hemisphere (M1 vs. PMd) appears indicative of a difference in the amount of movement slowing that occurred with perturbation of each site (M1 vs. PMd) in patients with poor structural reserve (right side of figure). Specifically, the movement appears to be influenced more when PMd is targeted than when M1 is targeted, in line with our prediction.
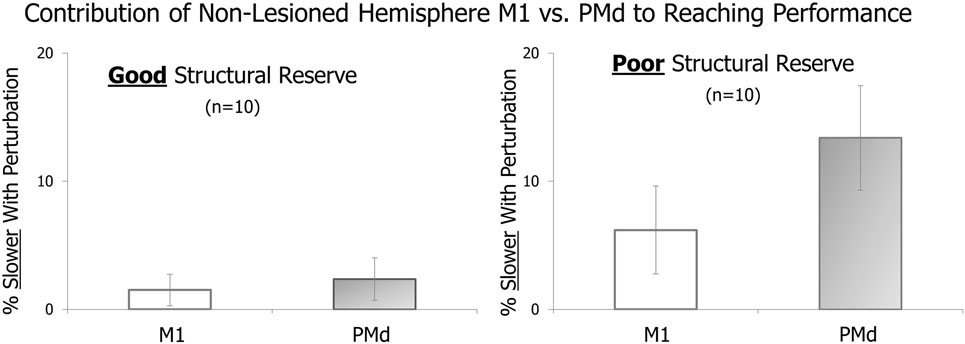
Figure 2. Effect of pre-movement cortical perturbation using transcranial magnetic stimulation (TMS) on the speed of the subsequent reaching movement. The amount of movement slowing with perturbation of non-lesioned hemisphere primary motor cortex (M1) vs. dorsal premotor cortex (PMd) is shown for patients with good structural reserve (left) and those with poor structural reserve (right).
The well-known correlation between non-lesioned hemisphere activation and level of motor recovery does not provide information about the functional role, if any, of that activation. In contrast, directly perturbing the sites of interest, as we have done here, provides causal evidence for their involvement in this task. Using a similar approach, previous studies demonstrated a functional role of non-lesioned hemisphere PMd in affected hand movements (20, 81). The data shown here suggest that this area may also have a role (1) in more severely impaired patients who lack hand movement, (2) in the performance of a multi-joint reaching task that requires activation of proximal muscle groups, and (3) that differs depending on the severity of CST damage. Thus, using the STAC approach and these preliminary findings, one might choose to target non-lesioned hemisphere PMd for motor priming prior to practice of a reaching task in patients with poor structural reserve. Importantly, priming of a different site would be indicated for a different type of task practice in patients with a higher level of structural reserve.
Summary and Recommendations
To move poststroke neuromodulation to the next level of development, a more specific model is needed for identifying cortical areas that could contribute to motor recovery and be targeted for motor priming. Thus, in addition to the amount of CST damage (i.e., structural sparing) that has been sustained, we suggest consideration of the attributes of the task to be practiced and the connectivity patterns of the candidate sites. This “structure, task attributes, and connectivity” (STAC) approach provides a patient-, task-, and site-specific framework for identifying a cortical area to be primed prior to practice. Our initial investigations in patients with different levels of structural sparing support the utility and validity of this approach.
Ethics Statement
This study was carried out in accordance with the recommendations of MedStar Health Research Institute Institutional Review Board with written informed consent from all subjects. All subjects gave written informed consent in accordance with the Declaration of Helsinki. The protocol was approved by the Institutional Review Board.
Author Contributions
MH-L conceptualized, designed, and wrote this submission, critically revised it, had final approval of the version to be published, and is accountable for all aspects of the work. RH contributed to design of this paper, collected data presented, helped critically revise it, had final approval of the version to be published, and is accountable for all aspects of the work.
Conflict of Interest Statement
The authors declare that the research was conducted in the absence of any commercial or financial relationships that could be construed as a potential conflict of interest.
Funding
This work was supported by the National Institutes of Health [K01 HD060886 (PI: MH-L)] and the American Heart Association [14GRNT20460001 (PI: MH-L); 15PRE24920006 (PI: RH)].
References
1. Jørgensen HS, Nakayama H, Raaschou HO, Olsen TS. Stroke. Neurologic and functional recovery the Copenhagen Stroke Study. Phys Med Rehabil Clin N Am (1999) 10(4):887–906.
2. Nakayama H, Jørgensen HS, Raaschou HO, Olsen TS. Recovery of upper extremity function in stroke patients: the Copenhagen Stroke Study. Arch Phys Med Rehabil (1994) 75(4):394–8. doi: 10.1016/0003-9993(94)90161-9
3. Kwakkel G, Kollen BJ, van der Grond J, Prevo AJH. Probability of regaining dexterity in the flaccid upper limb: impact of severity of paresis and time since onset in acute stroke. Stroke (2003) 34(9):2181–6. doi:10.1161/01.STR.0000087172.16305.CD
4. Mozaffarian D, Benjamin EJ, Go AS, Arnett DK, Blaha MJ, Cushman M, et al. Heart disease and stroke statistics-2016 update: a report from the American Heart Association. Circulation (2016) 133(4):e38–360. doi:10.1161/CIR.0000000000000350
5. Nichols-Larsen DS, Clark PC, Zeringue A, Greenspan A, Blanton S. Factors influencing stroke survivors’ quality of life during subacute recovery. Stroke (2005) 36(7):1480–4. doi:10.1161/01.STR.0000170706.13595.4f
6. Macciocchi SN, Diamond PT, Alves WM, Mertz T. Ischemic stroke: relation of age, lesion location, and initial neurologic deficit to functional outcome. Arch Phys Med Rehabil (1998) 79(10):1255–7. doi:10.1016/S0003-9993(98)90271-4
7. Schiemanck SK, Kwakkel G, Post MWM, Kappelle LJ, Prevo AJH. Impact of internal capsule lesions on outcome of motor hand function at one year post-stroke. J Rehabil Med (2008) 40(2):96–101. doi:10.2340/16501977-0130
8. Shelton FN, Reding MJ. Effect of lesion location on upper limb motor recovery after stroke. Stroke (2001) 32(1):107–12. doi:10.1161/01.STR.32.1.107
9. Chen C-L, Tang F-T, Chen H-C, Chung C-Y, Wong M-K. Brain lesion size and location: effects on motor recovery and functional outcome in stroke patients. Arch Phys Med Rehabil (2000) 81(4):447–52. doi:10.1053/mr.2000.3837
10. Cho S-H, Kim DG, Kim D-S, Kim Y-H, Lee C-H, Jang SH. Motor outcome according to the integrity of the corticospinal tract determined by diffusion tensor tractography in the early stage of corona radiata infarct. Neurosci Lett (2007) 426(2):123–7. doi:10.1016/j.neulet.2007.08.049
11. Burke Quinlan E, Dodakian L, See J, McKenzie A, Le V, Wojnowicz M, et al. Neural function, injury, and stroke subtype predict treatment gains after stroke: predicting gains after stroke. Ann Neurol (2015) 77(1):132–45. doi:10.1002/ana.24309
12. Michaelsen SM, Luta A, Roby-Brami A, Levin MF. Effect of trunk restraint on the recovery of reaching movements in hemiparetic patients. Stroke (2001) 32(8):1875–83. doi:10.1161/01.STR.32.8.1875
13. Michaelsen SM, Dannenbaum R, Levin MF. Task-specific training with trunk restraint on arm recovery in stroke: randomized control trial. Stroke (2006) 37(1):186–92. doi:10.1161/01.STR.0000196940.20446.c9
14. Michaelsen SM, Levin MF. Short-term effects of practice with trunk restraint on reaching movements in patients with chronic stroke: a controlled trial. Stroke (2004) 35(8):1914–9. doi:10.1161/01.STR.0000132569.33572.75
15. Harris-Love ML, Morton SM, Perez MA, Cohen LG. Mechanisms of short-term training-induced reaching improvement in severely hemiparetic stroke patients: a TMS study. Neurorehabil Neural Repair (2011) 25(5):398–411. doi:10.1177/1545968310395600
16. Di Pino G, Pellegrino G, Assenza G, Capone F, Ferreri F, Formica D, et al. Modulation of brain plasticity in stroke: a novel model for neurorehabilitation. Nat Rev Neurol (2014) 10(10):597–608. doi:10.1038/nrneurol.2014.162
18. Jackson JH. Case of disease of the brain – left hemiplegia – mental affection. Med Times Gaz (1872) 1:513–4.
19. Cramer SC, Nelles G, Benson RR, Kaplan JD, Parker RA, Kwong KK, et al. A functional MRI study of subjects recovered from hemiparetic stroke. Stroke (1997) 28(12):2518–27. doi:10.1161/01.STR.28.12.2518
20. Johansen-Berg H, Rushworth MFS, Bogdanovic MD, Kischka U, Wimalaratna S, Matthews PM. The role of ipsilateral premotor cortex in hand movement after stroke. Proc Natl Acad Sci U S A (2002) 99(22):14518–23. doi:10.1073/pnas.222536799
21. Cao Y, Vikingstad EM, Huttenlocher PR, Towle VL, Levin DN. Functional magnetic resonance studies of the reorganization of the human hand sensorimotor area after unilateral brain injury in the perinatal period. Proc Natl Acad Sci U S A (1994) 91(20):9612–6. doi:10.1073/pnas.91.20.9612
22. Levy CE, Nichols DS, Schmalbrock PM, Keller P, Chakeres DW. Functional MRI evidence of cortical reorganization in upper-limb stroke hemiplegia treated with constraint-induced movement therapy. Am J Phys Med Rehabil (2001) 80(1):4–12. doi:10.1097/00002060-200101000-00003
23. Fridman EA, Hanakawa T, Chung M, Hummel F, Leiguarda RC, Cohen LG. Reorganization of the human ipsilesional premotor cortex after stroke. Brain (2004) 127(4):747–58. doi:10.1093/brain/awh082
24. Xing S, Lacey EH, Skipper-Kallal LM, Jiang X, Harris-Love ML, Zeng J, et al. Right hemisphere grey matter structure and language outcomes in chronic left hemisphere stroke. Brain (2016) 139(1):227–41. doi:10.1093/brain/awv323
25. O’Shea J, Johansen-Berg H, Trief D, Göbel S, Rushworth MFS. Functionally specific reorganization in human premotor cortex. Neuron (2007) 54(3):479–90. doi:10.1016/j.neuron.2007.04.021
26. Sasaki K, Matsunaga T, Tomite T, Yoshikawa T, Shimada Y. Effect of electrical stimulation therapy on upper extremity functional recovery and cerebral cortical changes in patients with chronic hemiplegia. Biomed Res (Tokyo, Japan) (2012) 33(2):89–96. doi:10.2220/biomedres.33.89
27. Feydy A, Carlier R, Roby-Brami A, Bussel B, Cazalis F, Pierot L, et al. Longitudinal study of motor recovery after stroke: recruitment and focusing of brain activation. Stroke (2002) 33(6):1610–7. doi:10.1161/01.STR.0000017100.68294.52
28. Schaechter JD, Kraft E, Hilliard TS, Dijkhuizen RM, Benner T, Finklestein SP, et al. Motor recovery and cortical reorganization after constraint-induced movement therapy in stroke patients: a preliminary study. Neurorehabil Neural Repair (2002) 16(4):326–38. doi:10.1177/154596830201600403
29. Ward NS, Brown MM, Thompson AJ, Frackowiak RSJ. Neural correlates of motor recovery after stroke: a longitudinal fMRI study. Brain (2003) 126(Pt 11):2476–96. doi:10.1093/brain/awg245
30. Ferbert A, Priori A, Rothwell JC, Day BL, Colebatch JG, Marsden CD. Interhemispheric inhibition of the human motor cortex. J Physiol (1992) 453:525–46. doi:10.1113/jphysiol.1992.sp019243
31. Duque J, Murase N, Celnik P, Hummel F, Harris-Love M, Mazzocchio R, et al. Intermanual differences in movement-related interhemispheric inhibition. J Cogn Neurosci (2007) 19(2):204–13. doi:10.1162/jocn.2007.19.2.204
32. Ward NS, Cohen LG. Mechanisms underlying recovery of motor function after stroke. Arch Neurol (2004) 61(12):1844–8. doi:10.1001/archneur.61.12.1844
33. Ackerley SJ, Stinear CM. Stimulating stimulation: can we improve motor recovery following stroke using repetitive transcranial magnetic stimulation? Phys Ther Rev (2010) 15(4):302–8. doi:10.1179/174328810X12719009060263
34. Bradnam LV, Stinear CM, Byblow WD. Ipsilateral motor pathways after stroke: implications for non-invasive brain stimulation. Front Hum Neurosci (2013) 7:184. doi:10.3389/fnhum.2013.00184
35. Corti M, Patten C, Triggs W. Repetitive transcranial magnetic stimulation of motor cortex after stroke: a focused review. Am J Phys Med Rehabil (2012) 91(3):254–70. doi:10.1097/PHM.0b013e318228bf0c
36. Hsu W-Y, Cheng C-H, Liao K-K, Lee I-H, Lin Y-Y. Effects of repetitive transcranial magnetic stimulation on motor functions in patients with stroke: a meta-analysis. Stroke (2012) 43(7):1849–57. doi:10.1161/STROKEAHA.111.649756
37. Nowak DA, Grefkes C, Ameli M, Fink GR. Interhemispheric competition after stroke: brain stimulation to enhance recovery of function of the affected hand. Neurorehabil Neural Repair (2009) 23(7):641–56. doi:10.1177/1545968309336661
38. Takeuchi N, Chuma T, Matsuo Y, Watanabe I, Ikoma K. Repetitive transcranial magnetic stimulation of contralesional primary motor cortex improves hand function after stroke. Stroke (2005) 36(12):2681–6. doi:10.1161/01.STR.0000189658.51972.34
39. Bäumer T, Bock F, Koch G, Lange R, Rothwell JC, Siebner HR, et al. Magnetic stimulation of human premotor or motor cortex produces interhemispheric facilitation through distinct pathways: interhemispheric facilitation through motor and premotor pathways. J Physiol (2006) 572(3):857–68. doi:10.1113/jphysiol.2006.104901
40. Pundik S, McCabe JP, Hrovat K, Fredrickson AE, Tatsuoka C, Feng IJ, et al. Recovery of post stroke proximal arm function, driven by complex neuroplastic bilateral brain activation patterns and predicted by baseline motor dysfunction severity. Front Hum Neurosci (2015) 9:394. doi:10.3389/fnhum.2015.00394
41. Stinear CM, Barber PA, Smale PR, Coxon JP, Fleming MK, Byblow WD. Functional potential in chronic stroke patients depends on corticospinal tract integrity. Brain (2006) 130(1):170–80. doi:10.1093/brain/awl333
42. Graziano MS, Taylor CS, Moore T. Complex movements evoked by microstimulation of precentral cortex. Neuron (2002) 34(5):841–51. doi:10.1016/S0896-6273(02)00698-0
43. Rizzolatti G, Luppino G, Matelli M. The organization of the cortical motor system: new concepts. Electroencephalogr Clin Neurophysiol (1998) 106(4):283–96. doi:10.1016/S0013-4694(98)00022-4
44. Rizzolatti G, Luppino G. The cortical motor system. Neuron (2001) 31(6):889–901. doi:10.1016/S0896-6273(01)00423-8
45. Chouinard PA, Paus T. What have we learned from “perturbing” the human cortical motor system with transcranial magnetic stimulation? Front Hum Neurosci (2010) 4:173. doi:10.3389/fnhum.2010.00173
46. Colebatch JG, Gandevia SC. The distribution of muscular weakness in upper motor neuron lesions affecting the arm. Brain (1989) 112(Pt 3):749–63. doi:10.1093/brain/112.3.749
47. Colebatch JG, Rothwell JC, Day BL, Thompson PD, Marsden CD. Cortical outflow to proximal arm muscles in man. Brain (1990) 113(Pt 6):1843–56. doi:10.1093/brain/113.6.1843
48. Turton A, Lemon RN. The contribution of fast corticospinal input to the voluntary activation of proximal muscles in normal subjects and in stroke patients. Exp Brain Res (1999) 129(4):559–72. doi:10.1007/s002210050926
49. Palmer E, Ashby P. Corticospinal projections to upper limb motoneurones in humans. J Physiol (1992) 448:397. doi:10.1113/jphysiol.1992.sp019048
50. Porter R, Lemon R. Corticospinal Function and Voluntary Movement. Oxford, UK: Oxford University Press (1993). doi:10.1093/acprof:oso/9780198523758.001.0001
51. Penfield W, Boldrey E. Somatic motor and sensory representation in the cerebral cortex of man as studied by electrical stimulation. Brain (1937) lx:389. doi:10.1093/brain/60.4.389
52. Wassermann EM, McShane LM, Hallett M, Cohen LG. Noninvasive mapping of muscle representations in human motor cortex. Electroencephalogr Clin Neurophysiol (1992) 85(1):1–8. doi:10.1016/0168-5597(92)90094-R
53. Plow EB, Arora P, Pline MA, Binenstock MT, Carey JR. Within-limb somatotopy in primary motor cortex–revealed using fMRI. Cortex (2010) 46(3):310–21. doi:10.1016/j.cortex.2009.02.024
54. Park MC, Belhaj-Saïf A, Gordon M, Cheney PD. Consistent features in the forelimb representation of primary motor cortex in rhesus macaques. J Neurosci (2001) 21(8):2784–92.
55. Park MC, Belhaj-Saif A, Cheney PD. Properties of primary motor cortex output to forelimb muscles in rhesus macaques. J Neurophysiol (2004) 92(5):2968–84. doi:10.1152/jn.00649.2003
56. Nirkko A, Ozdoba C, Redmond S, Bürki M, Schroth G, Hess C, et al. Different ipsilateral representations for distal and proximal movements in the sensorimotor cortex: activation and deactivation patterns. Neuroimage (2001) 13(5):825–35. doi:10.1006/nimg.2000.0739
57. Cisek P, Crammond DJ, Kalaska JF. Neural activity in primary motor and dorsal premotor cortex in reaching tasks with the contralateral versus ipsilateral arm. J Neurophysiol (2003) 89(2):922–42. doi:10.1152/jn.00607.2002
58. Brinkman J, Kuypers H. Cerebral control of contralateral and ipsilateral arm, hand and finger movements in the split-brain rhesus monkey. Brain (1973) 96(4):653–74. doi:10.1093/brain/96.4.653
59. Abbruzzese G, Assini A, Buccolieri A, Schieppati M, Trompetto C. Comparison of intracortical inhibition and facilitation in distal and proximal arm muscles in humans. J Physiol (1999) 514(3):895–903. doi:10.1111/j.1469-7793.1999.895ad.x
60. Krutky MA, Perreault EJ, Motor cortical measures of use-dependent plasticity are graded from distal to proximal in the human upper limb. J Neurophysiol (2007) 98(6):3230–41. doi:10.1152/jn.00750.2007
61. Rathelot J-A, Strick PL. Subdivisions of primary motor cortex based on cortico-motoneuronal cells. Proc Natl Acad Sci U S A (2009) 106(3):918–23. doi:10.1073/pnas.0808362106
62. Tomassini V, Jbabdi S, Klein JC, Behrens TEJ, Pozzilli C, Matthews PM, et al. Diffusion-weighted imaging tractography-based parcellation of the human lateral premotor cortex identifies dorsal and ventral subregions with anatomical and functional specializations. J Neurosci (2007) 27(38):10259–69. doi:10.1523/JNEUROSCI.2144-07.2007
63. Ferreri F, Rossini PM. TMS and TMS-EEG techniques in the study of the excitability, connectivity, and plasticity of the human motor cortex. Rev Neurosci (2013) 24(4):431–42. doi:10.1515/revneuro-2013-0019
64. Yeo BTT, Krienen FM, Sepulcre J, Sabuncu MR, Lashkari D, Hollinshead M, et al. The organization of the human cerebral cortex estimated by intrinsic functional connectivity. J Neurophysiol (2011) 106(3):1125–65. doi:10.1152/jn.00338.2011
65. Rehme AK, Grefkes C. Cerebral network disorders after stroke: evidence from imaging-based connectivity analyses of active and resting brain states in humans. J Physiol (2013) 591(1):17–31. doi:10.1113/jphysiol.2012.243469
66. Siegel JS, Ramsey LE, Snyder AZ, Metcalf NV, Chacko RV, Weinberger K, et al. Disruptions of network connectivity predict impairment in multiple behavioral domains after stroke. Proc Natl Acad Sci U S A (2016) 113:E4367–76. doi:10.1073/pnas.1521083113
67. Grefkes C, Nowak DA, Wang LE, Dafotakis M, Eickhoff SB, Fink GR. Modulating cortical connectivity in stroke patients by rTMS assessed with fMRI and dynamic causal modeling. Neuroimage (2010) 50(1):233–42. doi:10.1016/j.neuroimage.2009.12.029
68. Volz LJ, Rehme AK, Michely J, Nettekoven C, Eickhoff SB, Fink GR, et al. Shaping early reorganization of neural networks promotes motor function after stroke. Cereb Cortex (2016) 26(6):2882–94. doi:10.1093/cercor/bhw034
69. Bernhard CG, Bohm E. Cortical representation and functional significance of the corticomotoneuronal system. AMA Arch Neurol Psychiatry (1954) 72(4):473–502. doi:10.1001/archneurpsyc.1954.02330040075006
70. Lawrence DG, Kuypers HG. The functional organization of the motor system in the monkey. I. The effects of bilateral pyramidal lesions. Brain (1968) 91(1):1–14. doi:10.1093/brain/91.1.1
71. Lawrence DG, Kuypers HG. The functional organization of the motor system in the monkey. II. The effects of lesions of the descending brain-stem pathways. Brain (1968) 91(1):15–36. doi:10.1093/brain/91.1.1
72. Goodale MA, Milner AD. Separate visual pathways for perception and action. Trends Neurosci (1992) 15(1):20–5. doi:10.1016/0166-2236(92)90344-8
73. Culham JC, Danckert SL, Souza JFXD, Gati JS, Menon RS, Goodale MA. Visually guided grasping produces fMRI activation in dorsal but not ventral stream brain areas. Exp Brain Res (2003) 153(2):180–9. doi:10.1007/s00221-003-1591-5
74. Dum RP, Strick PL. The origin of corticospinal projections from the premotor areas in the frontal lobe. J Neurosci (1991) 11(3):667–89.
75. Dum RP, Strick PL. Motor areas in the frontal lobe of the primate. Physiol Behav (2002) 77(4–5):677–82. doi:10.1016/S0031-9384(02)00929-0
76. Turton A, Wroe S, Trepte N, Fraser C, Lemon RN. Contralateral and ipsilateral EMG responses to transcranial magnetic stimulation during recovery of arm and hand function after stroke. Electroencephalogr Clin Neurophysiol (1996) 101(4):316–28. doi:10.1016/0924-980X(96)95560-5
77. von Monakow KH, Akert K, Künzle H. Projections of precentral and premotor cortex to the red nucleus and other midbrain areas in macaca fascicularis. Exp Brain Res (1979) 34(1):91–105. doi:10.1007/BF00238343
78. Zaaimi B, Edgley SA, Soteropoulos DS, Baker SN. Changes in descending motor pathway connectivity after corticospinal tract lesion in macaque monkey. Brain (2012) 135(7):2277–89. doi:10.1093/brain/aws115
79. Baker SN. The primate reticulospinal tract, hand function and functional recovery: primate reticulospinal tract. J Physiol (2011) 589(23):5603–12. doi:10.1113/jphysiol.2011.215160
80. Mohapatra S, Harrington R, Chan E, Dromerick AW, Breceda EY, Harris-Love M. Role of contralesional hemisphere in paretic arm reaching in patients with severe arm paresis due to stroke: a preliminary report. Neurosci Lett (2016) 617:52–8. doi:10.1016/j.neulet.2016.02.004
Keywords: stroke, transcranial magnetic stimulation, motor cortex, rehabilitation, upper extremity
Citation: Harris-Love ML and Harrington RM (2017) Non-Invasive Brain Stimulation to Enhance Upper Limb Motor Practice Poststroke: A Model for Selection of Cortical Site. Front. Neurol. 8:224. doi: 10.3389/fneur.2017.00224
Received: 20 January 2017; Accepted: 09 May 2017;
Published: 29 May 2017
Edited by:
Sangeetha Madhavan, University of Illinois at Chicago, United StatesReviewed by:
Svetlana Pundik, Case Western Reserve University, United StatesShailesh S. Kantak, Moss Rehabilitation Hospital, United States
Copyright: © 2017 Harris-Love and Harrington. This is an open-access article distributed under the terms of the Creative Commons Attribution License (CC BY). The use, distribution or reproduction in other forums is permitted, provided the original author(s) or licensor are credited and that the original publication in this journal is cited, in accordance with accepted academic practice. No use, distribution or reproduction is permitted which does not comply with these terms.
*Correspondence: Michelle L. Harris-Love, bWhhcnJpMzYmI3gwMDA0MDtnbXUuZWR1