- Department of Otolaryngology, University of Pittsburgh School of Medicine, Pittsburgh, PA, USA
This review considers the integration of vestibular and other signals by the central nervous system pathways that participate in balance control and blood pressure regulation, with an emphasis on how this integration may modify posture-related responses in accordance with behavioral context. Two pathways convey vestibular signals to limb motoneurons: the lateral vestibulospinal tract and reticulospinal projections. Both pathways receive direct inputs from the cerebral cortex and cerebellum, and also integrate vestibular, spinal, and other inputs. Decerebration in animals or strokes that interrupt corticobulbar projections in humans alter the gain of vestibulospinal reflexes and the responses of vestibular nucleus neurons to particular stimuli. This evidence shows that supratentorial regions modify the activity of the vestibular system, but the functional importance of descending influences on vestibulospinal reflexes acting on the limbs is currently unknown. It is often overlooked that the vestibulospinal and reticulospinal systems mainly terminate on spinal interneurons, and not directly on motoneurons, yet little is known about the transformation of vestibular signals that occurs in the spinal cord. Unexpected changes in body position that elicit vestibulospinal reflexes can also produce vestibulosympathetic responses that serve to maintain stable blood pressure. Vestibulosympathetic reflexes are mediated, at least in part, through a specialized group of reticulospinal neurons in the rostral ventrolateral medulla that project to sympathetic preganglionic neurons in the spinal cord. However, other pathways may also contribute to these responses, including those that dually participate in motor control and regulation of sympathetic nervous system activity. Vestibulosympathetic reflexes differ in conscious and decerebrate animals, indicating that supratentorial regions alter these responses. However, as with vestibular reflexes acting on the limbs, little is known about the physiological significance of descending control of vestibulosympathetic pathways.
Introduction
Historically, vestibular-elicited reflexes were considered to be stereotyped responses to particular head movements (1–3). However, recent research has shown that vestibular nucleus neurons receive inputs from a variety of sources in addition to the inner ear, such that vestibular-elicited reflexes are shaped in accordance with ongoing movements and behaviors. For example, the work of Cullen et al. showed that some vestibular nucleus neurons compare the planned head movement with feedback sensory signals, and discharge in accordance with deviations from the intended movement (4–6). However, most studies that considered the modification of vestibular responses in a behavioral context focused on the control of eye movements and gaze (4, 7, 8), but not vestibular reflexes acting on the limbs and vestibulosympathetic responses that regulate blood pressure.
This review considers the integration of vestibular and other signals by the central nervous system pathways that participate in balance control and blood pressure regulation, with an emphasis on how this integration may modify posture-related responses in accordance with behavioral context. It starts with an overview of the pathways that relay vestibular signals to limb motoneurons and sympathetic preganglionic neurons, and then considers the integration of signals that occurs in these pathways. Next, evidence is presented that descending signals modify vestibulospinal reflexes acting on the limbs as well as vestibulosympathetic reflexes, presumably to shape the responses in accordance with physiological challenges that are present or anticipated, as well as behaviors and movements that are planned or being implemented. Finally, directions for future research are discussed. In total, the review describes how perspectives on vestibulospinal and vestibulosympathetic responses have evolved, such that these responses are now considered to be components of a hierarchy of systems that are activated to achieve stable movements without disturbances in blood pressure.
Organization of Vestibulospinal and Vestibulosympathetic Pathways
Vestibulospinal Pathways Acting on the Limbs
Two pathways originating in the vestibular nuclei provide inputs to spinal cord segments containing limb motoneurons. The medial vestibulospinal tract (MVST) originates in the rostral portion of the descending vestibular nucleus as well as the bordering areas of the medial and lateral vestibular nuclei (9–11). The main influences of this pathway are on upper-body musculature, particularly neck musculature, although a small fraction of MVST projections provide inputs to segments containing forelimb motoneurons (10–12). The lateral vestibulospinal tract (LVST) originates mainly from the lateral vestibular nucleus, with some contribution from the descending nucleus (9–11). This tract extends the entire length of the spinal cord and provides extensive inputs to spinal cord segments containing motoneurons that innervate forelimb and hindlimb muscles (13, 14). Since the LVST provides much more extensive inputs to the spinal cord segments containing limb motoneurons than does the MVST (10–12), it likely plays a predominant role in controlling postural responses of the limbs. Thus, the LVST will be a focus of this article.
The LVST mainly terminates in Rexed’s laminae VII and VIII in the forelimb and hindlimb segments of the spinal cord, which contain premotor interneurons, and not directly on motoneurons (13–16). Electrophysiological studies have confirmed that most connections of LVST axons with limb α-motoneurons are polysynaptic, although some weak monosynaptic inputs may occur to hindlimb motoneurons (17, 18). These observations suggest that signals conveyed through the LVST are processed and likely modified by spinal interneurons prior to reaching motoneurons. The LVST mainly has excitatory effects on extensor motoneurons, with some inhibitory effects on flexor motoneurons (17, 18). At least in cats, approximately half of LVST axons that terminate in lower cervical and upper thoracic spinal segments (which contain forelimb motoneurons) also have a branch to the lumbar spinal cord, raising the prospect that some LVST neurons simultaneously control muscle activity in both the forelimbs and hindlimbs (19).
Neurons whose axons project to the spinal cord in the pontine and medullary reticulospinal tracts (RST) also receive extensive vestibular inputs (20–23). These vestibular inputs are polysynaptic, indicating that vestibular nucleus neurons and other pathways convey labyrinthine signals to RST neurons, but that RST neurons do not receive direct inputs from vestibular nerve fibers (20, 21). RST neurons have both excitatory and inhibitory effects on flexor and extensor forelimb and hindlimb motoneurons (24–28), as opposed to LVST neurons that tend to excite extensor motoneurons and inhibit flexor motoneurons (17, 18). However, LVST and RST (24, 25, 29) neurons are similar in that their effects on the excitability of limb motoneurons are mainly polysynaptic, via spinal interneurons, and that the axons of both RST (30) and LVST (19) neurons are highly branched. Thus, the two major pathways that convey vestibular signals to limb motoneurons have some similarities, as well as some major differences.
Vestibulosympathetic Pathways
The first key study demonstrating that the vestibular system contributes to cardiovascular regulation was published by Doba and Reis (31). As discussed in detail in a recent review (32), considerable evidence from experiments in both human and animal subjects has shown that the vestibular system participates in regulating sympathetic nervous system activity, to provide for adjustments in blood pressure during movement.
A column of reticulospinal neurons located near the ventral surface of the rostral medulla plays a predominant role in controlling sympathetic nerve activity and blood pressure (33–36). These neurons comprise the rostral ventrolateral medulla (RVLM). Lesions of the RVLM abolished vestibulosympathetic responses (37), suggesting that reticulospinal neurons with cell bodies in the RVLM constitute the major pathway through which vestibular signals are conveyed to sympathetic preganglionic neurons in the thoracic spinal cord. RVLM neurons are components of the baroreceptor reflex arc, which also includes neurons in nucleus tractus solitarius (NTS) that receive baroreceptor inputs, as well as inhibitory neurons in the caudal ventrolateral medulla (CVLM) (38–41). The RVLM receives direct inputs from the caudal aspects of the vestibular nucleus complex (42–47), as does the NTS (42, 48–51) and CVLM (42, 43, 45–47). The connections from the caudal portions of the vestibular nuclei to brainstem regions that regulate sympathetic nervous system activity are shown in Figure 1. In addition, neurons in other areas of the reticular formation that project to the RVLM, including those in the lateral tegmental field, receive labyrinthine inputs (52). Thus, RVLM neurons that control sympathetic nervous system activity receive both direct and multisynaptic inputs from vestibular nucleus neurons.
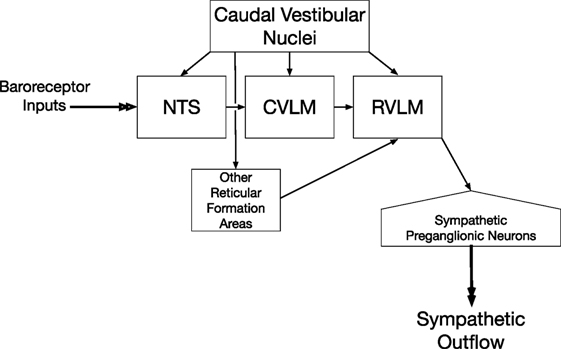
Figure 1. Vestibulosympathetic reflex pathway. Neurons in the caudal portions of the vestibular nuclei provide both direct and multisynaptic inputs to neurons in the rostral ventrolateral medulla (RVLM) with projections to sympathetic preganglionic neurons in the thoracic spinal cord. Multisynaptic pathways from the vestibular nuclei to the RVLM include relays in nucleus tractus solitarius (NTS), caudal ventrolateral medulla (CVLM), and other regions of the reticular formation including the medullary lateral tegmental field.
Pathways for Dual Control of Sympathetic Outflow and Limb Muscle Activity
Although most studies have focused on pathways that independently regulate blood pressure and limb movements, transneuronal tracing studies demonstrated that neurons in the medial medullary reticular formation and adjacent raphe nuclei have collateralized projections to both sympathetic preganglionic neurons and limb motoneurons, as do some brainstem catecholaminergic neurons including those in locus coeruleus and nucleus subcoeruleus (53–56). As noted above, the activity of many medial medullary reticulospinal neurons is modulated by vestibular inputs (20–23). There is also evidence that medullary raphespinal neurons (57, 58), as well as spinally projecting neurons in locus coeruleus (59–61), receive vestibular signals. However, it is not yet known whether the dual-control neurons mediate both vestibulosympathetic and vestibulospinal responses. Moreover, the physiological role of the dual-control pathways is currently unknown, but these pathways should not be ignored when considering vestibular control of motor and sympathetic nervous system activity.
Inputs to Vestibulospinal and Vestibulosympathetic Pathways
Inputs to the Vestibular Nuclei
The vestibular nucleus complex on each side of the brainstem receives a wide variety of inputs. These inputs include vestibular signals from ipsilateral primary vestibular afferents and the contralateral vestibular nuclei, somatosensory inputs from the spinal cord, and modulatory signals from the cerebellum and higher-order brain centers. The convergence of widely dispersed inputs likely contributes, at least in part, to controlling the activity of vestibulospinal and vestibulosympathetic reflex pathways.
Vestibular Inputs
The central projections of vestibular afferents have been studied extensively and delineated in detail in prior reviews and chapters (8, 62), and thus will only be briefly described here. Vestibular afferents encode information about head tilt, translation, and rotations in space and project to the vestibular nuclear complex and to other areas of the nervous system that participate in balance control, such as the cerebellar nodulus and uvula (62–64). Vestibular afferents with peripheral processes innervating otolith and semicircular canal endorgans terminate ipsilaterally in all of the major vestibular nuclei, although projection patterns vary across species (62). Furthermore, the vestibular nuclei, excepting the lateral vestibular nucleus, are strongly interconnected by commissural projections from the contralateral side, and through ipsilateral intrinsic pathways (65, 66). Finally, inputs from multiple vestibular receptors, such as from otolith and semicircular canal inputs, converge onto single vestibular nucleus neurons, producing modulation of neural activity with varying spatial and temporal characteristics (termed spatiotemporal convergence) (67).
Spinal Inputs
Anatomic studies demonstrated that all levels of the spinal cord convey inputs to the vestibular nuclei (68–71). Cervical proprioceptive afferents send collaterals directly to the ipsilateral medial and inferior vestibular nuclei (68, 72, 73). Disynaptic pathways also carry afferent information from the cervical spinal cord to the medial, inferior, and lateral vestibular nuclei (74). Neurons within the lumbar enlargement send direct projections to the medial and inferior vestibular nuclei, and possibly the lateral vestibular nucleus (69, 70). Other indirect pathways, such as through the reticular formation and cerebellum, may also convey information from the spinal cord to the vestibular nuclei (70). Neurophysiologic studies conducted in a variety of species (mice, rat, cat, and cynomolgus monkey) and preparations confirmed that stimulation of somatosensory afferents from the neck and limbs modulates the activity of vestibular nucleus neurons (75–81).
Visual Inputs
Many neurons in the vestibular nuclei have activity that is related to eye position or eye movements [for reviews, see Ref. (4, 7, 8)]. Such neurons are not believed to contribute to vestibulospinal responses acting on the limbs and are largely absent from the caudal aspects of the vestibular nuclei that mediate vestibulosympathetic responses (82). Thus, these neurons will not be discussed further in this article. However, the activities of both neurons with eye-position sensitivity and “vestibular only” (VO) neurons that may contribute to vestibulospinal reflexes are modulated by movement of the visual field (optokinetic stimuli) (83–87). It is well-established that visual inputs contribute to postural control (88–95), and there is evidence that visual signals modulate vestibulosympathetic responses (96). However, it is unknown whether visual contributions to these responses are mediated through optokinetic inputs to the vestibular nuclei, or via other pathways.
Cerebellar Inputs
Cerebellar outflow to the vestibular nuclei is largely through the deep cerebellar nuclei, which are comprised of the fastigial, interposed, and dentate nuclei. Purkinje neurons in the cerebellar cortex send inhibitory projections to the deep cerebellar nuclei (97), which subsequently project to a variety of targets in the brainstem. The fastigial nucleus has been shown in a number of studies to project heavily to the vestibular nuclear complex (98–102); a few studies have also demonstrated projections to the vestibular nuclei from the interposed (103, 104) and dentate nuclei (105).
Some cerebellar cortical Purkinje neurons, particularly those in the vestibulocerebellum, project directly to the vestibular nuclei. Immunostaining studies localizing protein kinase C (PKC), an enzyme found in Purkinje cells, demonstrated that cerebellar Purkinje cells project to the major vestibular nuclei (106, 107). Anterograde and retrograde tracing studies showed that flocculus Purkinje neurons terminate in all of the major vestibular nuclei as well as group y, a cytoarchitecturally distinct extension of the vestibular nuclei located dorsocaudal to the restiform body (108–110). Tracing data also demonstrated that Purkinje cells in the nodulus send axons to the medial, inferior, and superior vestibular nuclei and to group y (111–113). Purkinje cells in the uvula were also shown to project to the superior and medial vestibular nuclei (112). The delta-isoform of PKC, which heavily stains Purkinje cells in the folia of the uvula and nodulus as well as the posterior cerebellum, is found in the caudal vestibular nuclei (inferior and medial vestibular nuclei), indicative of a significant direct cerebellar projection to these regions (107, 114).
Cerebral Inputs
Direct corticovestibular projections have been demonstrated in a number of animal preparations and are best described in cats and non-human primates (115–120), as reviewed by Fukushima (121). In the cat, injection of retrograde tracers into the vestibular nuclei labeled neurons is widely dispersed in areas of cerebral cortex including areas 6, 2, and 3a (115, 117). Electrical stimulation of areas 2 and 3a resulted in excitatory and inhibitory responses of vestibular nucleus neurons (115). This study also showed that vestibular nucleus neurons with spinal projections were particularly likely to have their activity modulated by cortical stimulation (115). Similarly, electrical stimulation of cortical motor areas (pericruciate cortex) resulted in short-latency (possibly monosynaptic) and long-latency excitation and inhibition of vestibular nucleus neurons (116). Studies in a variety of non-human primate species showed that widely dispersed cortical areas, including parieto-insular vestibular cortex, area 3aV, temporal area T3, premotor area 6a, area 7ant in squirrel monkey (corresponding to macaque area 2v), and the anterior cingulate cortex, project to the vestibular nuclei (119–122).
Inputs to Reticulospinal Neurons
As previously discussed, reticulospinal neurons in the pons and medulla [pontomedullary reticulospinal tract neurons (pmRSTn)] that contribute to postural control receive extensive inputs from the vestibular nuclei (20–23). Although all four vestibular nuclei provide inputs to pmRSTn, the distribution, and extent of the projections from each nucleus are not uniform (21). Electrical stimulation of the VIII cranial nerve evoked disynaptic and polysynaptic excitation as well as polysynaptic inhibition of neurons in the pontomedullary reticular formation, confirming anatomical evidence that these cells receive extensive labyrinthine inputs (21, 123). A study in decerebrate cats indicated that pmRSTn receive extensive inputs from the otolith organs, which supports the notion that the RST plays an important role in generating gravity-dependent postural reflexes (22). However, some pmRSTn did not respond to stimulation of the VIII nerve, showing that the function of the RST is not simply to transmit vestibular signals to the spinal cord (21).
Extensive spinoreticular projections convey spinal afferent inputs to the reticular formation (124–128). As noted above, vestibular nucleus neurons receive extensive spinal inputs, such that projections from the vestibular nuclei to the reticular formation are another potential route for transmitting signals from skin and muscle to pmRSTn. Cutaneous inputs to the reticular formation are prevalent (124, 125, 128–131). Drew and Colleagues (131) reported that the majority of medullary RST neurons responded to cutaneous inputs, usually with an excitatory response. There is less evidence that pmRSTn receive inputs from muscle afferents, although one study in decerebrate cats showed that vibration applied to the gastrocnemius–soleus muscle complex altered the firing in 27% of neurons located within the nucleus gigantocellularis (a region of the medullary reticular formation) (132).
Like vestibular nucleus neurons, pmRSTn receive direct inputs from cerebral cortex (133–140). Inputs to pmRSTn are mainly from motor and premotor cortex, whereas vestibular nucleus neurons receive inputs from widespread cortical areas, at least in non-human primates (121). RST neurons, like vestibulospinal neurons, also receive extensive inputs from the cerebellar fastigial nucleus (141–143). However, unlike vestibulospinal neurons, pmRSTn receive little direct input from cerebellar Purkinje cells. Thus, there is a possibility that RST neurons are subject to different cortical and cerebellar control than vestibulospinal neurons, although this notion has not been thoroughly examined experimentally.
As noted above, some of the inputs to pmRSTn resemble those to vestibulospinal neurons. In addition, some reticulospinal neurons receive inputs distinct from those to the vestibular nuclei. For example, a population of spinally projecting neurons in the ventral and caudal aspect of the pontine reticular formation mediates acoustic startle responses (144). Such responses entail the stiffening of the dorsal neck, body wall, and limbs to provide protection from predatory attack before a defensive action can be engaged. The acoustic startle response entails monosynaptic inputs from the cochlear nuclei to reticulospinal neurons (145–148). Although vestibulospinal neurons may also mediate acoustic startle responses (149), the reticulospinal system appears to play a dominant role (150).
Shik et al. discovered in 1966 that electrical stimulation of a constrained region at the junction between the midbrain- and pons-elicited locomotion in animals (151). This area has subsequently been called the “mesencephalic locomotor region” (MLR) and has been identified across vertebrate species including man (152). The MLR does not project to the spinal cord, but provides inputs to pmRSTn (153, 154) that transmit locomotor signals to spinal premotor interneurons (155, 156). Hence, pmRSTn play a unique and pivotal role in controlling locomotion. There is no evidence that vestibulospinal neurons receive inputs from the MLR.
Although it is appreciated that a variety of inputs are conveyed to the reticular formation, little is known about the convergence of these inputs on single neurons (129). Moreover, only a few studies have entailed recordings from pmRSTn in conscious animals, or attempted to ascertain how the activity of these neurons changes during ongoing movements and across behavioral states.
Figure 2 summarizes the inputs to and connections of vestibulospinal and reticulospinal neurons.
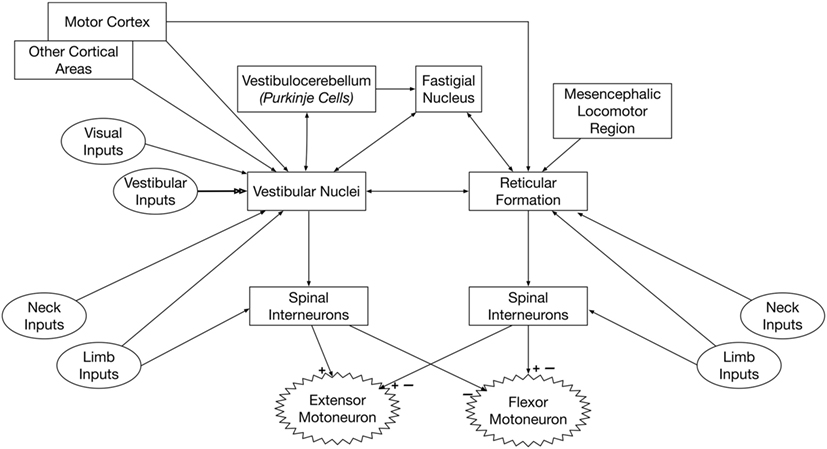
Figure 2. Pathways that convey vestibular signals to limb motoneurons. Spinal premotor interneurons receive inputs from the vestibulospinal and reticulospinal pathways. The vestibulospinal system excites extensor motoneurons and inhibits flexor motoneurons, while the reticulospinal system elicits both excitation and inhibition of flexor and extensor motoneurons. Thus, the spinal interneurons that receive inputs from vestibulospinal and reticulospinal pathways must be at least partially distinct. The neurons of origin of reticulospinal and vestibulospinal projections integrate vestibular, neck, and limb inputs; this integration is modulated by signals from several brain regions, including the cerebral cortex and cerebellum.
Processing of Vestibulospinal and Reticulospinal Signals by Spinal Interneurons
As discussed above, few vestibulospinal and reticulospinal projections provide direct inputs to limb motoneurons, but instead mainly terminate on interneurons in Rexed’s laminae VII and VIII (24, 25, 29). A variety of identified types of spinal interneurons have been reported to receive labyrinthine inputs, including Renshaw cells (157, 158), Ia inhibitory interneurons (159, 160), propriospinal interneurons (161–163), and commissural interneurons (164–166). However, most experiments that characterized the responses of spinal interneurons to natural vestibular stimulation (whole-body rotations) did not identify the physiological role of those interneurons, or the convergent spinal and descending inputs they receive (167–169).
Considering the extensive projections of muscle spindle afferents to Rexed’s lamina VII (170, 171), it seems likely that there is convergence and integration of vestibular and proprioceptive signals in the spinal cord. A variety of descending pathways from the brain terminate in Rexed’s laminae VIII, including corticospinal, vestibulospinal, and reticulospinal projections [for review, see Ref. (172)]. A caveat is that the vestibulospinal system excites extensor motoneurons and inhibits flexor motoneurons (17, 18), while the reticulospinal system elicits both excitation and inhibition of flexor and extensor motoneurons (24–28). Thus, the spinal interneurons that receive inputs from the vestibulospinal and reticulospinal pathways must be at least partially distinct. Unfortunately, little is known about the convergence of signals from descending pathways in the spinal cord, and how the integration of the descending motor commands shapes ongoing and planned movements. Thus, the significance and physiological role of the processing of vestibular signals by spinal cord interneurons remain unclear. One role of the signal integration may be to adjust muscle activity when body orientation changes during locomotion, as while walking uphill (173–175).
It has also been suggested that the vestibulospinal and reticulospinal pathways provide inputs to gamma motoneurons, thereby affecting the gain of the myotatic reflex (176–178). However, some studies in animals (179), as well as recent experiments in humans (180, 181), indicated that vestibular signals do not affect the fusimotor system. Further experiments are needed to address the discrepancies in the conclusions of these studies.
Inputs to Vestibulosympathetic Neurons
Neurons in the RVLM that control sympathetic nervous system activity receive vestibular inputs through a variety of pathways (32), as illustrated in Figure 1. Since many of the relays from the vestibular nuclei to the RVLM are polysynaptic, it is difficult to identify “vestibulosympathetic neurons” during neurophysiologic experiments. Thus, much of what is known about convergence of signals in the vestibulosympathetic pathways was ascertained by determining inputs and behavioral states that modulate sympathetic nerve activity elicited by vestibular stimulation.
Baroreceptors provide a dominant input to sympathetic nervous system neurons that control blood pressure, such that an increase in blood pressure results in a decrease in the activity of RVLM neurons as well as the sympathetic preganglionic neurons they provide inputs to (38–41). It is thus not surprising that stimulation of baroreceptors by increasing blood pressure resulted in an attenuation of vestibulosympathetic responses (182). A number of studies demonstrated the convergence of vestibular and baroreceptor inputs onto RVLM neurons, including putative presympathetic neurons with projections to the thoracic spinal cord (183, 184).
Rostral ventrolateral medulla neurons receive inputs from a variety of brain regions, either directly or indirectly through connections in the baroreceptor reflex pathway. These brain regions include the periaqueductal gray (185), parabrachial nucleus (186–193), several hypothalamic nuclei (190, 194–215), the amygdala (195, 212, 216–219), and prefrontal and insular cortices (206, 212, 220–225). Thus, engagement of a wide variety of descending pathways could potentially modulate vestibulosympathetic responses.
Transformation of Vestibular Reflexes by Descending Pathways
Transformation of Vestibulospinal and Reticulospinal Reflexes: Evidence from Animal Studies
The present body of knowledge regarding descending control of vestibulospinal reflexes largely stems from one of two experimental designs: comparing vestibular nucleus neuronal responses during active and passive movement in intact animals and comparing vestibular nucleus neural responses between decerebrate and intact preparations. While additional studies are clearly needed, in particular to discriminate which higher centers are responsible for modulating vestibulospinal reflexes, some insights can be gained from the present body of knowledge.
A subset of vestibular nucleus neurons identified in non-human primates, termed VO neurons, are thought to mediate vestibulospinal reflexes (226–229). The activity of VO neurons, like other vestibular nucleus neurons and primary vestibular afferents, is modulated by passive (externally applied) head movement with respect to space. However, unlike other classes of vestibular nucleus neurons, the firing rate of VO neurons does not change during eye movements (230, 231). During active (self-generated) head movement, responses of VO neurons are dramatically attenuated (227, 231, 232). Furthermore, proprioceptive feedback must match that signaled by the active motor command (efference copy) to suppress VO neuronal activity during self-motion (233, 234). While the locations of the circuits that compare efference copy with proprioceptive feedback during a movement have not yet been fully elucidated, descending inputs from higher brain centers must play a key role since volitional movement is triggered from cerebral cortex. In addition, it is unknown whether VO neurons responsible for reflex posturing of the limbs respond differently to active and passive (unexpected) limb movements.
Decerebration results in a disconnection of brainstem centers, including the vestibular nuclei and reticular formation, from higher brain centers. In decerebrate animals, the neural circuitry of vestibulospinal pathways is simplified by removing descending cortical influences, permitting investigations in a reduced preparation. The interruption of supratentorial inputs to the lateral vestibular nucleus is thought to produce unsuppressed activation of extensor motoneurons by the LVST, resulting in decerebrate extensor posturing (235–237).
As previously discussed, there is widespread convergence of afferent inputs from multiple sources, including vestibular, somatosensory, and visual signals, in the vestibular nuclei. The effects of hindlimb somatosensory inputs on the activity of vestibular nucleus neurons have been studied in both decerebrate and conscious cats by the same investigators, using the same equipment and methodology during experiments in both preparations, thus permitting comparisons (75, 78, 238, 239). Comparisons in decerebrate and conscious cats of the effects of electrical stimulation of hindlimb nerves on vestibular nucleus neuronal activity revealed some similarities, as well as differences (238, 239). Similarities across preparations include the following: the majority of vestibular nucleus neurons received convergent limb inputs from multiple nerves; response latencies were ~20 ms suggesting that polysynaptic pathways conveyed limb inputs to vestibular nucleus neurons; most responses were excitatory; and the proportion of neurons activated by hindlimb nerve stimulation increased after bilateral labyrinthectomy (238, 239). By contrast, low-intensity stimuli [<twice threshold (T) for eliciting a compound action potential in the stimulated nerve] elicited changes in activity of many vestibular nucleus neurons in decerebrate animals, but such low-intensity stimuli were ineffective in conscious animals. Only high-threshold stimuli (≥3 T) altered the activity of vestibular nucleus neurons in conscious animals. This finding suggests that supratentorial brain regions may suppress the transmission of inputs from large diameter hindlimb afferent fibers (i.e., group Ia and II afferents) to the vestibular nuclei in the conscious animal, or may block the responses of vestibular nucleus neurons to these signals.
Modulation of vestibular nucleus neuronal activity in response to hindlimb movement has also been compared in decerebrate and conscious cats (75, 78). While vestibular nucleus neurons responded to hindlimb movement in both preparations, some important differences were notable (see Figure 3). In decerebrate cats, the majority of vestibular nucleus neurons whose activity was modulated by hindlimb movement encoded the direction of hindlimb movement, and most also encoded hindlimb position signals (78). By contrast, most vestibular nucleus neurons in conscious cats encoded limb movement irrespective of the direction of the movement and did not encode hindlimb position (75). These findings suggest an interesting parallel with that noted above for experiments that made use of electrical nerve stimulation. If large-fiber afferents, such as those from muscle spindles, are responsible for the directional and position-related responses noted in decerebrate animals, the near complete lack of such responses in conscious animals suggests that higher brain centers selectively suppress this afferent feedback.
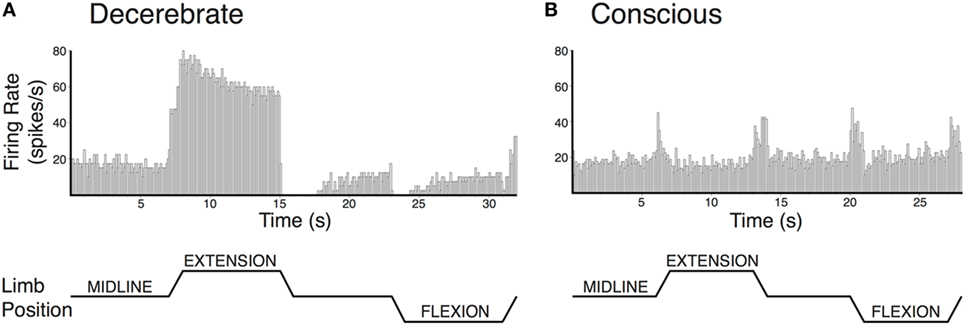
Figure 3. Vestibular nucleus neurons responded to hindlimb movement in decerebrate (A) and conscious (B) cats, but the responses had different characteristics in the two preparations. Vestibular nucleus neuronal responses to hindlimb movement in the decerebrate cat typically included encoding the direction of hindlimb movement and encoding hindlimb position signals. For example, the firing rate of the vestibular nucleus neuron depicted in panel (A) increased with hindlimb movements toward extension and decreased with hindlimb movements toward flexion. Furthermore, when the hindlimb was held in extension, neuronal firing remained elevated. While neurons with such response characteristics were also present in conscious cats, they were much less common than in decerebrate cats. By contrast, the activities of most vestibular nucleus neurons in conscious cats with responses to hindlimb movement were modulated in a similar fashion during all tested directions of hindlimb movement. For example, the activity of the vestibular nucleus neuron depicted in panel (B) increased with each hindlimb movement (midline to extension; extension to midline; midline to flexion; and flexion to midline). In both panels (A,B), hindlimb movements were passively generated (externally applied) at 60°/s, lasted for a duration of 1 s, and were isolated to movements about the hip and knee joints. The hindlimb was held in each position for 7 s in panel (A) and 6 s in panel (B). Unit activity was binned in 0.1 s intervals and averaged over four repetitions in panel (A) and eight repetitions in panel (B). Panel (A) was adapted from Ref. (78); panel (B) was adapted from Ref. (75). Both panels were used with permission of the American Physiological Society.
Similar comparisons of responses to limb movement in decerebrate and conscious cats have been performed for reticular formation neurons (240). In both preparations, the majority of responsive reticular formation neurons encoded limb movement irrespective of the direction of the movement; few encoded limb position or the direction of limb movement. In other words, the responses of reticular formation neurons to limb movement in both decerebrate and conscious animals resembled those of vestibular nucleus neurons in conscious animals. No suppression of limb position signals to reticular formation neurons by supratentorial brain regions was observed (240), as noted for vestibular nucleus neurons (75). These findings raise the possibility that higher brain areas such as cerebral cortex play fundamentally different roles in regulating the activity of the vestibulospinal and reticulospinal systems, although there is no experimental evidence to suggest the nature of the differences in regulation of the two systems.
Transformation of Vestibulospinal and Reticulospinal Reflexes: Evidence from Studies in Human Subjects
As discussed above, transecting the midbrain in animals produces unsuppressed activation of extensor motoneurons, resulting in decerebrate extensor posturing (235–237). In humans, strokes affecting the internal capsule, which damage corticobulbar projections, produce an analogous condition: muscle spasticity (241, 242). Spasticity manifests as a sharply lateralized increase in muscle tone with enhanced tendon jerks (243). Several studies suggested that spasticity in patients, like decerebrate rigidity in animals, results from increased activity of vestibulospinal pathways (244–246). This is in contrast to one study in cats, which provided evidence that spasticity and decerebrate rigidity are differentially mediated through vestibulospinal and reticulospinal projections (247). A recent study in hemispheric stroke subjects supports the notion that spasticity results from disinhibition of vestibulospinal projections. Responses of neck muscles to vestibular stimulation (cervical vestibular-evoked myogenic potentials) were compared on the intact and lesioned sides in stroke survivors with spasticity. The differences on the two sides were proportional to the severity of the spasticity (248); the responses on the lesioned side were amplified, as illustrated in Figure 4. In combination with data from animals discussed previously, these data support the notion that supratentorial regions of the brain regulate the excitability of vestibulospinal neurons.
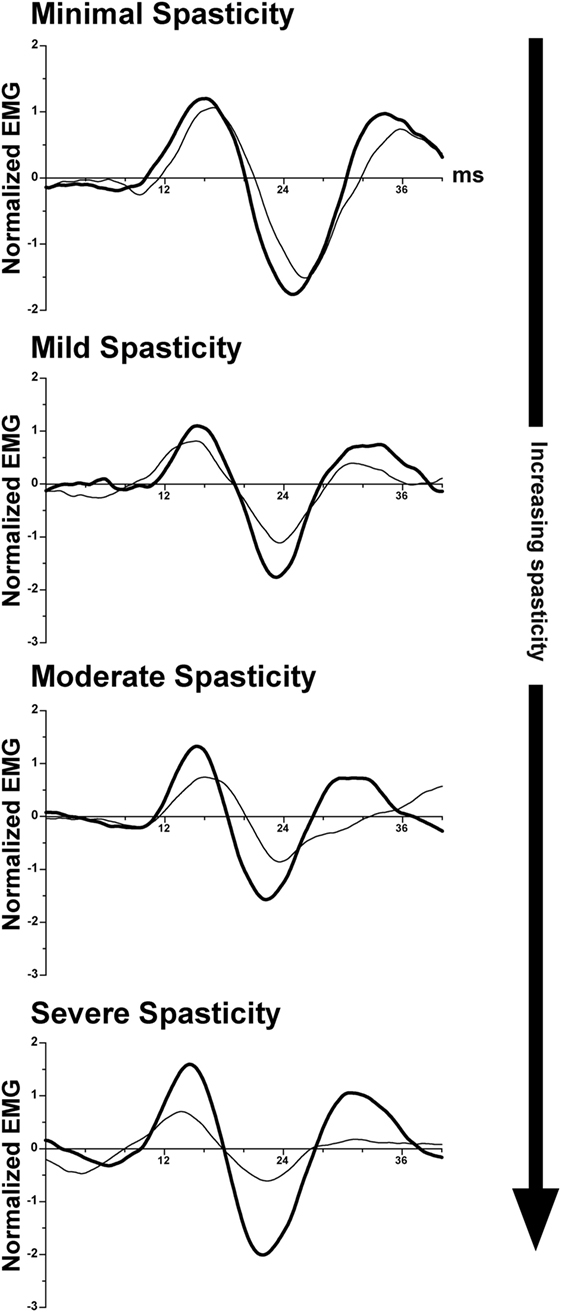
Figure 4. Representative vestibular-evoked reflex responses from four subjects rank ordered as a function of increasing severity of spasticity. Cervical vestibular-evoked myogenic potentials (VEMPs) were measured from the sternocleidomastoid muscles. In most subjects, the VEMP responses on the spastic-paretic side (thick line) were larger than on the contralateral side (thin line). As the degree of spasticity increased, so did the asymmetry in response amplitude between the two sides. Adapted from Ref. (248); used with permission of Elsevier.
Transformation of Vestibulosympathetic Reflexes
Evidence that supratentorial brain regions affect the gain of vestibulosympathetic reflexes comes from a comparison of these responses in conscious and decerebrate animals (184). Whereas about half of RVLM neurons responded to 10–15° tilts in vertical planes in decerebrate felines, the activity of <1% of RVLM neurons was modulated by similar rotations in conscious cats (184). Large rotations are required to generate vestibulosympathetic responses in conscious animals, presumably because small-amplitude tilts (<40%) do not produce peripheral blood pooling that necessitates an increase in sympathetic nervous system activity (249, 250). It has been suggested that higher brain areas adjust the responsiveness of neurons in the vestibulosympathetic reflex pathway to vestibular inputs, so that the gain of the vestibulosympathetic reflex is appropriate for the ensuing movement or postural change (32). Recordings from conscious animals also provided evidence that following a bilateral labyrinthectomy, the gain of the baroreceptor reflex is adjusted by descending signals from supratentorial brain regions (251). As noted above, a number of supratentorial regions provide inputs to neurons that comprise the vestibulosympathetic reflex pathway, and it is unclear which of these regions participates in adjusting the response gain, and where along the pathway (vestibular nuclei, NTS, CVLM, RVLM) the gain adjustments occur.
The notion that sympathetic nerve activity is regulated by higher brain centers during movement is not new. In both animals and humans, adjustments in sympathetic nerve activity and alterations in the set point of the baroreceptor reflex are initiated when exercise begins (252, 253). The changes in the baroreceptor set point are needed to allow blood pressure to increase during exercise. The term “central command,” which was coined by Goodwin et al., refers to the parallel changes in autonomic nervous system activity that accompanies muscle contraction (254). Perhaps the best evidence for feedforward cardiovascular responses during movement comes from a study in paralyzed human subjects, who exhibited increases in blood pressure and heart rate that were graded to the intensity of imagined activity (255). In decerebrate or anesthetized cats, stimulation of regions of the lateral and caudal hypothalamus, fields of Forel, MLR, and midbrain ventral tegmental area elicit parallel changes in motor activity and cardiovascular responses (253, 256, 257). However, little is known about signal processing in these regions that leads to changes in sympathetic nerve activity and the baroreceptor reflex set point.
Conclusion and Directions for Future Research
Although there is a considerable body of data regarding vestibular system contributions to maintenance of stable eye position, much less is known about vestibular reflexes that ensure postural stability and constant blood pressure during movement. This is likely because vestibulospinal pathways that act on the limbs and vestibulosympathetic pathways that affect the cardiovascular system are much more complicated than the three and four neuron arcs that mediate vestibulo-ocular reflexes (3, 8). An often-overlooked aspect of vestibulospinal reflexes acting on the limbs is that they are mediated through premotor interneurons in the spinal cord (24, 25, 29), and not via direct connections of vestibulospinal and reticulospinal neurons with motoneurons. Virtually nothing is known about the convergence of descending motor signals with proprioceptive inputs in the spinal cord, and the role that spinal interneurons play in transforming the motor commands (11). Since there is a potential for spinal interneurons to integrate signals from a variety of descending motor pathways, the interneurons could potentially play an appreciable role in recovery of function if one of the pathways (or inputs to the pathways) is eliminated. Thus, a research focus is certainly warranted on the spinal interneurons that are components of vestibulospinal reflexes that act on the limbs.
Although it has been recognized for decades that both reticulospinal and vestibulospinal neurons convey vestibular signals to the spinal cord, and that these pathways receive somewhat different inputs, it is not yet clear if there is a distinct functional difference between the two pathways. As noted above, it is possible that signals conveyed through reticulospinal and vestibulospinal pathways converge on the same premotor spinal interneurons (11), although this is presently unknown. The reticulospinal pathway may be comprised of a number of parallel pathways with distinct functional roles such as mediating locomotion (153, 154), startle responses (144), and postural stability. Thus, it may be misleading to consider the reticulospinal pathway as a single functional pathway. Moreover, there is a paucity of data regarding the activity of vestibulospinal and reticulospinal neurons in awake, behaving animals, and the lack of this information complicates the determination of the physiologic roles of the pathways.
An experimental hurdle that complicates experiments in conscious animals on vestibulospinal and reticulospinal pathways is the difficulty in identifying neurons that are components of these pathways. Experiments entailing microstimulation in the spinal cord to ascertain the projections of vestibulospinal and reticulospinal neurons are tedious in paralyzed decerebrate or anesthetized animals (19), and even more complicated in conscious animals, since spinal stimulation can induce movement and extraneous inputs to the central nervous system. Consequently, there is a tendency to consider “VO neurons,” which are known to have a projection to the spinal cord, as a single class of neurons with uniform properties (227–230). Yet it is known from experiments in paralyzed animals that the branching pattern of individual vestibulospinal neurons can vary considerably (15, 16, 19). Vestibulospinal neurons that affect neck muscle activity may have dramatically different inputs and changes in activity across behavioral states than those that affect limb extensor activity. Thus, it is crucial to develop new methodology to ascertain the projection patterns of vestibulospinal neurons whose activity is monitored during neurophysiologic experiments, and to discontinue the practice of assuming that all vestibulospinal neurons must have the same properties. The same considerations should be applied to experiments on reticulospinal neurons.
Neurophysiologic experiments on neurons that constitute vestibulosympathetic pathways are in their infancy, and just two studies (184, 251) have monitored in conscious animals the activity of RVLM neurons that play a key role in regulating sympathetic nervous system activity. Little attention has been paid to the contributions of other pathways to vestibulosympathetic responses, including those originating in the medial reticular formation, raphe nuclei, and locus coeruleus that provide monosynaptic or polysynaptic inputs to both limb motoneurons and sympathetic preganglionic neurons (53–56). These pathways were identified in transneuronal tracing studies in rodents, and it is unclear whether they exist in other species, and what their role is in autonomic and motor control. In addition, it is critical that neurophysiologic studies on these neurons include an identification of their projection patterns, to ascertain which outputs they control.
In conclusion, it is now well-demonstrated that vestibulospinal and reticulospinal neurons that contribute to postural stability, and brainstem neurons that adjust blood pressure during postural alterations, receive converging inputs from a variety of sources, including cerebral cortex. Accordingly, the activity and responses to stimuli of the neurons can vary tremendously in conscious and “reduced” preparations, such as anesthetized and decerebrate animal models. In the ongoing efforts of the scientific community to foster the reproducibility of experiments and their translation to treatments for patients, the differences in responses between preparations must be fully considered.
Author Contributions
All the authors co-wrote the manuscript and co-prepared the figures, and approved the final version of the manuscript.
Conflict of Interest Statement
The authors have no conflicts of interest to declare related to this manuscript. No third-party payments were received related to the contents, and the authors have no financial interests that influenced the writing of the article.
Funding
The authors’ work related to this article is supported by the following grants from the National Institutes of Health of the United States: R01-DC013788 to BY, F32-DC015157 and T32-DC011499 to DM, and K08-DC013571 to AM.
References
1. Wilson VJ, Peterson BW. Peripheral and central substrates of vestibulospinal reflexes. Physiol Rev (1978) 58:80–105.
2. Wilson VJ, Peterson BW. Vestibulospinal and reticulospinal systems. In: Brookhart JM, Mountcastle VB, editors. Handbook of Physiology. Section 1: The Nervous System, Volume II, Motor Control, Part 1. Bethesda: American Physiological Society (1981). p. 667–702. doi:10.1002/cphy.cp010214
3. Goldberg JM, Fernandez C. Vestibular mechanisms. Annu Rev Physiol (1975) 37:129–62. doi:10.1146/annurev.ph.37.030175.001021
4. Cullen KE. Physiology of central pathways. Handb Clin Neurol (2016) 137:17–40. doi:10.1016/B978-0-444-63437-5.00002-9
5. Cullen KE. The vestibular system: multimodal integration and encoding of self-motion for motor control. Trends Neurosci (2012) 35:185–96. doi:10.1016/j.tins.2011.12.001
6. Cullen KE, Brooks JX, Jamali M, Carriot J, Massot C. Internal models of self-motion: computations that suppress vestibular reafference in early vestibular processing. Exp Brain Res (2011) 210:377–88. doi:10.1007/s00221-011-2555-9
7. Angelaki DE, Cullen KE. Vestibular system: the many facets of a multimodal sense. Annu Rev Neurosci (2008) 31:125–50. doi:10.1146/annurev.neuro.31.060407.125555
8. Goldberg JM, Wilson VJ, Cullen KE, Angelaki DE, Broussard DM, Buttner-Ennever J, et al. The Vestibular System: A Sixth Sense. New York: Oxford University Press (2012).
9. Akaike T. Comparison of neuronal composition of the vestibulospinal system between cat and rabbit. Exp Brain Res (1973) 18:429–32. doi:10.1007/BF00239110
10. Akaike T. Neuronal organization of the vestibulospinal system in the cat. Brain Res (1983) 259:217–27. doi:10.1016/0006-8993(83)91252-0
11. Shinoda Y, Sugiuchi Y, Izawa Y, Hata Y. Long descending motor tract axons and their control of neck and axial muscles. Prog Brain Res (2006) 151:527–63. doi:10.1016/S0079-6123(05)51017-3
12. Perlmutter SI, Iwamoto Y, Baker JF, Peterson BW. Interdependence of spatial properties and projection patterns of medial vestibulospinal tract neurons in the cat. J Neurophysiol (1998) 79:270–84.
13. Nyberg-Hansen R, Mascitti TA. Sites and mode of termination of fibers of the vestibulospinal tract in the cat. An experimental study with silver impregnation methods. J Comp Neurol (1964) 122:369–83. doi:10.1002/cne.901220307
14. Petras JM. Cortical, tectal and tegmental fiber connections in the spinal cord of the cat. Brain Res (1967) 6:275–324. doi:10.1016/0006-8993(67)90196-5
15. Kuze B, Matsuyama K, Matsui T, Miyata H, Mori S. Segment-specific branching patterns of single vestibulospinal tract axons arising from the lateral vestibular nucleus in the cat: a PHA-L tracing study. J Comp Neurol (1999) 414:80–96. doi:10.1002/(SICI)1096-9861(19991108)414:1<80::AID-CNE7>3.3.CO;2-5
16. Shinoda Y, Ohgaki T, Futami T. The morphology of single lateral vestibulospinal tract axons in the lower cervical spinal cord of the cat. J Comp Neurol (1986) 249:226–41. doi:10.1002/cne.902490208
17. Grillner S, Hongo T, Lund S. The vestibulospinal tract. Effects on alpha-motoneurones in the lumbosacral spinal cord in the cat. Exp Brain Res (1970) 10:94–120. doi:10.1007/BF00340521
18. Wilson VJ, Yoshida M. Comparison of effects of stimulation of Deiters’ nucleus and medial longitudinal fasciculus on neck, forelimb and hindlimb motoneurons. J Neurophysiol (1969) 32:743–58.
19. Abzug C, Maeda M, Peterson BW, Wilson VJ. Cervical branching of lumbar vestibulospinal axons. With an appendix by C.P. Bean. J Physiol (1974) 243:499–522. doi:10.1113/jphysiol.1974.sp010764
20. Peterson BW, Filion M, Felpel LP, Abzug C. Responses of medial reticular neurons to stimulation of the vestibular nerve. Exp Brain Res (1975) 22:335–50. doi:10.1007/BF00234670
21. Peterson BW, Abzug C. Properties of projections from vestibular nuclei to medial reticular formation in the cat. J Neurophysiol (1975) 38:1421–35.
22. Bolton PS, Goto T, Schor RH, Wilson VJ, Yamagata Y, Yates BJ. Response of pontomedullary reticulospinal neurons to vestibular stimuli in vertical planes. Role in vertical vestibulospinal reflexes of the decerebrate cat. J Neurophysiol (1992) 67:639–47.
23. Schor RH, Yates BJ. Horizontal rotation responses of medullary reticular neurons in the decerebrate cat. J Vestib Res (1995) 5:223–8. doi:10.1016/0957-4271(94)00034-Y
24. Engberg I, Lundberg A, Ryall RW. Reticulospinal inhibition of transmission in reflex pathways. J Physiol (1968) 194:201–23. doi:10.1113/jphysiol.1968.sp008402
25. Lloyd DPC. Activity in neurons of the bulbospinal correlation system. J Neurophysiol (1941) 4:115–34.
26. Magoun HW, Rhines R. An inhibitory mechanism in the bulbar reticular formation. J Neurophysiol (1946) 9:165–71.
27. Rhines R, Magoun HW. Brain stem facilitation of cortical motor response. J Neurophysiol (1946) 9:219–29.
28. Sprague JM, Chambers WW. Control of posture by reticular formation and cerebellum in the intract, anesthetized and unanesthetized and in the decerebrated cat. Am J Physiol (1954) 176:52–64.
29. Engberg I, Lundberg A, Ryall RW. Reticulospinal inhibition of transmission through interneurones of spinal reflex pathways. Experientia (1965) 21:612–3. doi:10.1007/BF02151565
30. Peterson BW, Maunz RA, Pitts NG, Mackel RG. Patterns of projections and branching of reticulospinal neurons. Exp Brain Res (1975) 23:333–51. doi:10.1007/BF00238019
31. Doba N, Reis DJ. Role of the cerebellum and vestibular apparatus in regulation of orthostatic reflexes in the cat. Circ Res (1974) 34:9–18. doi:10.1161/01.RES.34.1.9
32. Yates BJ, Bolton PS, Macefield VG. Vestibulo-sympathetic responses. Compr Physiol (2014) 4:851–87. doi:10.1002/cphy.c130041
33. Dampney RA, Goodchild AK, McAllen RM. Vasomotor control by subretrofacial neurones in the rostral ventrolateral medulla. Can J Physiol Pharmacol (1987) 65:1572–9. doi:10.1139/y87-247
34. Dampney RA. The subretrofacial nucleus: its pivotal role in cardiovascular regulation. News Physiol Sci (1990) 5:63–7.
35. Dampney RA. The subretrofacial vasomotor nucleus – anatomical, chemical and pharmacological properties and role in cardiovascular regulation. Prog Neurobiol (1994) 42:197–227. doi:10.1016/0301-0082(94)90064-7
36. Bourassa EA, Sved AF, Speth RC. Angiotensin modulation of rostral ventrolateral medulla (RVLM) in cardiovascular regulation. Mol Cell Endocrinol (2009) 302:167–75. doi:10.1016/j.mce.2008.10.039
37. Yates BJ, Siniaia MS, Miller AD. Descending pathways necessary for vestibular influences on sympathetic and inspiratory outflow. Am J Physiol (1995) 268:R1381–5.
38. Dampney RA. Functional organization of central pathways regulating the cardiovascular system. Physiol Rev (1994) 74:323–64.
39. Abboud FM, Thames MD. Interaction of cardiovascular reflexes in circulatory control. In: Shepherd JT, Abboud FM, editors. Handbook of Physiology. Section 2: Circulation. Volume III: Peripheral Circulation and Organ Blood Flow, Part 2. Bethesda, MD: American Physiological Society (1983). p. 497–555. doi:10.1002/cphy.cp020319
40. Gilbey MP, Spyer KM. Essential organization of the sympathetic nervous system. Baillieres Clin Endocrinol Metab (1993) 7:259–78. doi:10.1016/S0950-351X(05)80177-6
41. Spyer KM. Annual review prize lecture – central nervous mechanisms contributing to cardiovascular control. J Physiol (1994) 474:1–19. doi:10.1113/jphysiol.1994.sp019997
42. Porter JD, Balaban CD. Connections between the vestibular nuclei and brain stem regions that mediate autonomic function in the rat. J Vestib Res (1997) 7:63–76. doi:10.1016/S0957-4271(96)00138-3
43. Stocker SD, Steinbacher BC, Balaban CD, Yates BJ. Connections of the caudal ventrolateral medullary reticular formation in the cat brainstem. Exp Brain Res (1997) 116:270–82. doi:10.1007/PL00005755
44. Holstein GR, Friedrich VL Jr, Kang T, Kukielka E, Martinelli GP. Direct projections from the caudal vestibular nuclei to the ventrolateral medulla in the rat. Neuroscience (2011) 175:104–17. doi:10.1016/j.neuroscience.2010.12.011
45. Holstein GR, Friedrich VL Jr, Martinelli GP. Projection neurons of the vestibulo-sympathetic reflex pathway. J Comp Neurol (2014) 522:2053–74. doi:10.1002/cne.23517
46. Holstein GR, Friedrich VL Jr, Martinelli GP. Imidazoleacetic acid-ribotide in vestibulo-sympathetic pathway neurons. Exp Brain Res (2016) 234:2747–60. doi:10.1007/s00221-016-4725-2
47. Holstein GR, Friedrich VL Jr, Martinelli GP. Glutamate and GABA in vestibulo-sympathetic pathway neurons. Front Neuroanat (2016) 10:7. doi:10.3389/fnana.2016.00007
48. Balaban CD, Beryozkin G. Vestibular nucleus projections to nucleus tractus solitarius and the dorsal motor nucleus of the vagus nerve: potential substrates for vestibulo-autonomic interactions. Exp Brain Res (1994) 98:200–12. doi:10.1007/BF00228409
49. Yates BJ, Grelot L, Kerman IA, Balaban CD, Jakus J, Miller AD. Organization of vestibular inputs to nucleus tractus solitarius and adjacent structures in cat brain stem. Am J Physiol (1994) 267:R974–83.
50. Cai YL, Ma WL, Li M, Guo JS, Li YQ, Wang LG, et al. Glutamatergic vestibular neurons express Fos after vestibular stimulation and project to the NTS and the PBN in rats. Neurosci Lett (2007) 417:132–7. doi:10.1016/j.neulet.2007.01.079
51. Ruggiero DA, Mtui EP, Otake K, Anwar M. Vestibular afferents to the dorsal vagal complex: substrate for vestibular-autonomic interactions in the rat. Brain Res (1996) 743:294–302. doi:10.1016/S0006-8993(96)01099-2
52. Yates BJ, Balaban CD, Miller AD, Endo K, Yamaguchi Y. Vestibular inputs to the lateral tegmental field of the cat: potential role in autonomic control. Brain Res (1995) 689:197–206. doi:10.1016/0006-8993(95)00569-C
53. Kerman IA, Shabrang C, Taylor L, Akil H, Watson SJ. Relationship of presympathetic-premotor neurons to the serotonergic transmitter system in the rat brainstem. J Comp Neurol (2006) 499:882–96. doi:10.1002/cne.21129
54. Kerman IA. Organization of brain somatomotor-sympathetic circuits. Exp Brain Res (2008) 187:1–16. doi:10.1007/s00221-008-1337-5
55. Nam H, Kerman IA. Distribution of catecholaminergic presympathetic-premotor neurons in the rat lower brainstem. Neuroscience (2016) 324:430–45. doi:10.1016/j.neuroscience.2016.02.066
56. Kerman IA, Enquist LW, Watson SJ, Yates BJ. Brainstem substrates of sympatho-motor circuitry identified using trans-synaptic tracing with pseudorabies virus recombinants. J Neurosci (2003) 23:4657–66.
57. Yates BJ, Goto T, Kerman I, Bolton PS. Responses of caudal medullary raphe neurons to natural vestibular stimulation. J Neurophysiol (1993) 70:938–46.
58. Yates BJ, Goto T, Bolton PS. Responses of neurons in the caudal medullary raphe nuclei of the cat to stimulation of the vestibular nerve. Exp Brain Res (1992) 89:323–32. doi:10.1007/BF00228248
59. Pompeiano O. Relationship of noradrenergic locus coeruleus neurones to vestibulospinal reflexes. Prog Brain Res (1989) 80:329–43. doi:10.1016/S0079-6123(08)62228-1
60. Pompeiano O, Manzoni D, Barnes CD, Stampacchia G, d’Ascanio P. Responses of locus coeruleus and subcoeruleus neurons to sinusoidal stimulation of labyrinth receptors. Neuroscience (1990) 35:227–48. doi:10.1016/0306-4522(90)90078-I
61. Pompeiano O, Manzoni D, Barnes CD. Responses of locus coeruleus neurons to labyrinth and neck stimulation. Prog Brain Res (1991) 88:411–34. doi:10.1016/S0079-6123(08)63826-1
62. Newlands SD, Perachio AA. Central projections of the vestibular nerve: a review and single fiber study in the Mongolian gerbil. Brain Res Bull (2003) 60:475–95. doi:10.1016/S0361-9230(03)00051-0
63. Purcell IM, Perachio AA. Peripheral patterns of terminal innervation of vestibular primary afferent neurons projecting to the vestibulocerebellum in the gerbil. J Comp Neurol (2001) 433:48–61. doi:10.1002/cne.1124
64. Korte GE. The brainstem projection of the vestibular nerve in the cat. J Comp Neurol (1979) 184:279–92. doi:10.1002/cne.901840204
65. Epema AH, Gerrits NM, Voogd J. Commissural and intrinsic connections of the vestibular nuclei in the rabbit: a retrograde labeling study. Exp Brain Res (1988) 71:129–46. doi:10.1007/BF00247528
66. Carleton SC, Carpenter MB. Afferent and efferent connections of the medial, inferior and lateral vestibular nuclei in the cat and monkey. Brain Res (1983) 278:29–51. doi:10.1016/0006-8993(83)90223-8
67. Dickman JD, Angelaki DE. Vestibular convergence patterns in vestibular nuclei neurons of alert primates. J Neurophysiol (2002) 88:3518–33. doi:10.1152/jn.00518.2002
68. Neuhuber WL, Zenker W. Central distribution of cervical primary afferents in the rat, with emphasis on proprioceptive projections to vestibular, perihypoglossal, and upper thoracic spinal nuclei. J Comp Neurol (1989) 280:231–53. doi:10.1002/cne.902800206
69. McKelvey-Briggs DK, Saint-Cyr JA, Spence SJ, Partlow GD. A reinvestigation of the spinovestibular projection in the cat using axonal transport techniques. Anat Embryol (1989) 180:281–91. doi:10.1007/BF00315886
70. Pompeiano O. Spinovestibular relations: anatomical and physiological aspects. Prog Brain Res (1972) 37:263–96. doi:10.1016/S0079-6123(08)63907-2
71. Jian BJ, Acernese AW, Lorenzo J, Card JP, Yates BJ. Afferent pathways to the region of the vestibular nuclei that participates in cardiovascular and respiratory control. Brain Res (2005) 1044:241–50. doi:10.1016/j.brainres.2005.03.010
72. Prihoda M, Hiller M-S, Mayr R. Central projections of cervical primary afferent fibers in the guinea pig: an HRP and WGA/HRP tracer study. J Comp Neurol (1991) 308:418–31. doi:10.1002/cne.903080309
73. Bankoul S, Goto T, Yates B, Wilson VJ. Cervical primary afferent input to vestibulospinal neurons projecting to the cervical dorsal horn: an anterograde and retrograde tracing study in the cat. J Comp Neurol (1995) 353:529–38. doi:10.1002/cne.903530405
74. Sato H, Ohkawa T, Uchino Y, Wilson VJ. Excitatory connections between neurons of the central cervical nucleus and vestibular neurons in the cat. Exp Brain Res (1997) 115:381–6. doi:10.1007/PL00005708
75. McCall AA, Miller DM, DeMayo WM, Bourdages GH, Yates BJ. Vestibular nucleus neurons respond to hindlimb movement in the conscious cat. J Neurophysiol (2016) 116:1785–94. doi:10.1152/jn.00414.2016
76. Barresi M, Grasso C, Li Volsi G, Manzoni D. Effects of body to head rotation on the labyrinthine responses of rat vestibular neurons. Neuroscience (2013) 244:134–46. doi:10.1016/j.neuroscience.2013.04.010
77. Medrea I, Cullen KE. Multisensory integration in early vestibular processing in mice: the encoding of passive versus active motion. J Neurophysiol (2013) 110:2704–17. doi:10.1152/jn.01037.2012
78. Arshian MS, Hobson CE, Catanzaro MF, Miller DJ, Puterbaugh SR, Cotter LA, et al. Vestibular nucleus neurons respond to hindlimb movement in the decerebrate cat. J Neurophysiol (2014) 111:2423–32. doi:10.1152/jn.00855.2013
79. Kasper J, Schor RH, Wilson VJ. Response of vestibular neurons to head rotations in vertical planes. II. Response to neck stimulation and vestibular-neck interaction. J Neurophysiol (1988) 60:1765–78.
80. Sadeghi SG, Mitchell DE, Cullen KE. Different neural strategies for multimodal integration: comparison of two macaque monkey species. Exp Brain Res (2009) 195:45–57. doi:10.1007/s00221-009-1751-3
81. Gdowski GT, McCrea RA. Neck proprioceptive inputs to primate vestibular nucleus neurons. Exp Brain Res (2000) 135:511–26. doi:10.1007/s002210000542
82. Miller DM, Cotter LA, Gandhi NJ, Schor RH, Cass SP, Huff NO, et al. Responses of caudal vestibular nucleus neurons of conscious cats to rotations in vertical planes, before and after a bilateral vestibular neurectomy. Exp Brain Res (2008) 188:175–86. doi:10.1007/s00221-008-1359-z
83. Blazquez PM, Highstein SM. Visual-vestibular interaction in vertical vestibular only neurons. Neuroreport (2007) 18:1403–6. doi:10.1097/WNR.0b013e3282cdeedd
84. Boyle R, Buttner U, Markert G. Vestibular nuclei activity and eye movements in the alert monkey during sinusoidal optokinetic stimulation. Exp Brain Res (1985) 57:362–9. doi:10.1007/BF00236542
85. Reisine H, Raphan T. Neural basis for eye velocity generation in the vestibular nuclei of alert monkeys during off-vertical axis rotation. Exp Brain Res (1992) 92:209–26. doi:10.1007/BF00227966
86. Bryan AS, Angelaki DE. Optokinetic and vestibular responsiveness in the macaque rostral vestibular and fastigial nuclei. J Neurophysiol (2009) 101:714–20. doi:10.1152/jn.90612.2008
87. Waespe W, Henn V. Neuronal activity in the vestibular nuclei of the alert monkey during vestibular and optokinetic stimulation. Exp Brain Res (1977) 27:523–38. doi:10.1007/BF00239041
88. Reynard F, Terrier P. Role of visual input in the control of dynamic balance: variability and instability of gait in treadmill walking while blindfolded. Exp Brain Res (2015) 233:1031–40. doi:10.1007/s00221-014-4177-5
89. Raffi M, Piras A, Persiani M, Squatrito S. Importance of optic flow for postural stability of male and female young adults. Eur J Appl Physiol (2014) 114:71–83. doi:10.1007/s00421-013-2750-4
90. Joseph Jilk D, Safavynia SA, Ting LH. Contribution of vision to postural behaviors during continuous support-surface translations. Exp Brain Res (2014) 232:169–80. doi:10.1007/s00221-013-3729-4
91. Bove M, Fenoggio C, Tacchino A, Pelosin E, Schieppati M. Interaction between vision and neck proprioception in the control of stance. Neuroscience (2009) 164:1601–8. doi:10.1016/j.neuroscience.2009.09.053
92. Palm HG, Strobel J, Achatz G, von Luebken F, Friemert B. The role and interaction of visual and auditory afferents in postural stability. Gait Posture (2009) 30:328–33. doi:10.1016/j.gaitpost.2009.05.023
93. Mahboobin A, Loughlin PJ, Redfern MS, Sparto PJ. Sensory re-weighting in human postural control during moving-scene perturbations. Exp Brain Res (2005) 167:260–7. doi:10.1007/s00221-005-0053-7
94. Bent LR, McFadyen BJ, Inglis JT. Visual-vestibular interactions in postural control during the execution of a dynamic task. Exp Brain Res (2002) 146:490–500. doi:10.1007/s00221-002-1204-8
95. Manchester D, Woollacott M, Zederbauer-Hylton N, Marin O. Visual, vestibular and somatosensory contributions to balance control in the older adult. J Gerontol (1989) 44:M118–27. doi:10.1093/geronj/44.4.M118
96. Jian BJ, Cotter LA, Emanuel BA, Cass SP, Yates BJ. Effects of bilateral vestibular lesions on orthostatic tolerance in awake cats. J Appl Physiol (1999) 86:1552–60.
97. Ito M, Yoshida M, Obata K, Kawai N, Udo M. Inhibitory control of intracerebellar nuclei by the Purkinje cell axons. Exp Brain Res (1970) 10:64–80. doi:10.1007/BF00340519
98. Noda H, Sugita S, Ikeda Y. Afferent and efferent connections of the oculomotor region of the fastigial nucleus in the macaque monkey. J Comp Neurol (1990) 302:330–48. doi:10.1002/cne.903020211
99. Homma Y, Nonaka S, Matsuyama K, Mori S. Fastigiofugal projection to the brainstem nuclei in the cat: an anterograde PHA-L tracing study. Neurosci Res (1995) 23:89–102. doi:10.1016/0168-0102(95)90019-5
100. Asanuma C, Thach WT, Jones EG. Brainstem and spinal projections of the deep cerebellar nuclei in the monkey, with observations on the brainstem projections of the dorsal column nuclei. Brain Res (1983) 286:299–322. doi:10.1016/0165-0173(83)90017-6
101. Batton RR, Jayaraman A, Ruggiero D, Carpenter MB. Fastigial efferent projections in the monkey: an autoradiographic study. J Comp Neurol (1977) 174:281–306. doi:10.1002/cne.901740206
102. Teune TM, van der Burg J, van der Moer J, Voogd J, Ruigrok TJ. Topography of cerebellar nuclear projections to the brain stem in the rat. Prog Brain Res (2000) 124:141–72. doi:10.1016/S0079-6123(00)24014-4
103. Compoint C, Buisseret-Delmas C, Diagne M, Buissseret P, Angaut P. Connections between the cerebellar nucleus interpositus and the vestibular nuclei: an anatomical study in the rat. Neurosci Lett (1997) 238:91–4. doi:10.1016/S0304-3940(97)00864-1
104. Gonzalo-Ruiz A, Leichnetz GR. Connections of the caudal cerebellar interpositus complex in a new world monkey (Cebus apella). Brain Res Bull (1990) 25:919–27. doi:10.1016/0361-9230(90)90189-7
105. Delfini C, Diagne M, Angaut P, Buisseret P, Buisseret-Delmas C. Dentatovestibular projections in the rat. Exp Brain Res (2000) 135:285–92. doi:10.1007/s002210000516
106. Barmack NH, Yakhnitsa V. Vestibularly evoked climbing-fiber responses modulate simple spikes in rabbit cerebellar Purkinje neurons. Ann N Y Acad Sci (2002) 978:237–54. doi:10.1111/j.1749-6632.2002.tb07571.x
107. Barmack NH, Qian Z, Yoshimura J. Regional and cellular distribution of protein kinase C in rat cerebellar Purkinje cells. J Comp Neurol (2000) 427:235–54. doi:10.1002/1096-9861(20001113)427:2<235::AID-CNE6>3.0.CO;2-6
108. Umetani T. Efferent projections from the flocculus in the albino rat as revealed by an autoradiographic orthograde tracing method. Brain Res (1992) 586:91–103. doi:10.1016/0006-8993(92)91376-P
109. Balaban CD, Schuerger RJ, Porter JD. Zonal organization of flocculo-vestibular connections in rats. Neuroscience (2000) 99:669–82. doi:10.1016/S0306-4522(00)00232-3
110. Langer T, Fuchs AF, Chubb MC, Scudder CA, Lisberger SG. Floccular efferents in the rhesus macaque as revealed by autoradiography and horseradish peroxidase. J Comp Neurol (1985) 235:26–37. doi:10.1002/cne.902350103
111. Wylie DR, De Zeeuw CI, DiGiorgi PL, Simpson JI. Projections of individual Purkinje cells of identified zones in the ventral nodulus to the vestibular and cerebellar nuclei in the rabbit. J Comp Neurol (1994) 349:448–63. doi:10.1002/cne.903490309
112. Shojaku H, Sato Y, Ikarashi K, Kawasaki T. Topographical distribution of Purkinje cells in the uvula and the nodulus projecting to the vestibular nuclei in cats. Brain Res (1987) 416:100–12. doi:10.1016/0006-8993(87)91501-0
113. Walberg F, Dietrichs E. The interconnection between the vestibular nuclei and the nodulus: a study of reciprocity. Brain Res (1988) 449:47–53. doi:10.1016/0006-8993(88)91022-0
114. Barmack NH. Central vestibular system: vestibular nuclei and posterior cerebellum. Brain Res Bull (2003) 60:511–41. doi:10.1016/S0361-9230(03)00055-8
115. Wilson VJ, Zarzecki P, Schor RH, Isu N, Rose PK, Sato H, et al. Cortical influences on the vestibular nuclei of the cat. Exp Brain Res (1999) 125:1–13. doi:10.1007/s002210050651
116. Troiani D, Draicchio F, Bonci A, Zannoni B. Responses of vestibular neurons to stimulation of cortical sensorimotor areas in the cat. Arch Ital Biol (1993) 131:137–46.
117. Pogossian VI, Fanardjian VV. Organization of afferent projections to the ventral and dorsal regions of the cat lateral vestibular nucleus: an HRP study. J Vestib Res (1992) 2:107–22.
118. Nishiike S, Guldin WO, Baurle J. Corticofugal connections between the cerebral cortex and the vestibular nuclei in the rat. J Comp Neurol (2000) 420:363–72. doi:10.1002/(SICI)1096-9861(20000508)420:3<363::AID-CNE7>3.3.CO;2-O
119. Akbarian S, Grusser OJ, Guldin WO. Corticofugal projections to the vestibular nuclei in squirrel monkeys – further evidence of multiple cortical vestibular fields. J Comp Neurol (1993) 332:89–104. doi:10.1002/cne.903320107
120. Akbarian S, Grusser OJ, Guldin WO. Corticofugal connections between the cerebral cortex and brainstem vestibular nuclei in the macaque monkey. J Comp Neurol (1994) 339:421–37. doi:10.1002/cne.903390309
121. Fukushima K. Corticovestibular interactions: anatomy, electrophysiology, and functional considerations. Exp Brain Res (1997) 117:1–16. doi:10.1007/PL00005786
122. Guldin WO, Mirring S, Grusser OJ. Connections from the neocortex to the vestibular brain stem nuclei in the common marmoset. Neuroreport (1993) 5:113–6. doi:10.1097/00001756-199311180-00004
123. Abzug C, Peterson BW. Antidromic stimulation in the ponto-medullary reticular formation of local axon branches of contralateral vestibular neurons. Brain Res (1973) 64:407–13. doi:10.1016/0006-8993(73)90196-0
124. Fields HL, Clanton CH, Anderson SD. Somatosensory properties of spinoreticular neurons in the cat. Brain Res (1977) 120:49–66. doi:10.1016/0006-8993(77)90497-8
125. Maunz RA, Pitts NG, Peterson BW. Cat spinoreticular neurons: locations, responses and changes in responses during repetitive stimulation. Brain Res (1978) 148:365–79. doi:10.1016/0006-8993(78)90725-4
126. Kevetter GA, Haber LH, Yezierski RP, Chung JM, Martin RF, Willis WD. Cells of origin of the spinoreticular tract in the monkey. J Comp Neurol (1982) 207:61–74. doi:10.1002/cne.902070106
127. Chapman CD, Ammons WS, Foreman RD. Raphe magnus inhibition of feline T1-Tr spinoreticular tract cell responses to visceral and somatic inputs. J Neurophysiol (1985) 53:773–85.
128. Casey KL. Somatic stimuli, spinal pathways, and size of cutaneous fibers influencing unit activity in the medial medulary reticular formation. Exp Neurol (1969) 25:35–56. doi:10.1016/0014-4886(69)90070-3
129. Peterson BW, Anderson ME, Filion M. Responses of pontomedullary reticular neurons to cortical, tectal and cutaneous stimuli. Exp Brain Res (1974) 21:19–44. doi:10.1007/BF00234256
130. Peterson BW, Franck JI, Daunton NG. Changes in responses of medial pontomedullary reticular neurons during repetitive cutaneous, vestibular, cortical, and tectal stimulation. J Neurophysiol (1976) 39:564–81.
131. Drew T, Cabana T, Rossignol S. Responses of medullary reticulospinal neurones to stimulation of cutaneous limb nerves during locomotion in intact cats. Exp Brain Res (1996) 111:153–68. doi:10.1007/BF00227294
132. Pompeiano O, Barnes CD. Response of brain stem reticular neurons to muscle vibration in the decerebrate cat. J Neurophysiol (1971) 24:709–24.
133. Keizer K, Kuypers HG. Distribution of corticospinal neurons with collaterals to lower brain stem reticular formation in cat. Exp Brain Res (1984) 54:107–20. doi:10.1007/BF00235823
134. Keizer K, Kuypers HG. Distribution of corticospinal neurons with collaterals to the lower brain stem reticular formation in monkey (Macaca fascicularis). Exp Brain Res (1989) 74:311–8. doi:10.1007/BF00248864
135. Berrevoets CE, Kuypers HG. Pericruciate cortical neurons projecting to brain stem reticular formation, dorsal column nuclei and spinal cord in the cat. Neurosci Lett (1975) 1:257–62. doi:10.1016/0304-3940(75)90040-3
136. Matsuyama K, Drew T. Organization of the projections from the pericruciate cortex to the pontomedullary brainstem of the cat: a study using the anterograde tracer Phaseolus vulgaris-leucoagglutinin. J Comp Neurol (1997) 389:617–41. doi:10.1002/(SICI)1096-9861(19971229)389:4<617::AID-CNE6>3.0.CO;2-3
137. Rho MJ, Cabana T, Drew T. Organization of the projections from the pericruciate cortex to the pontomedullary reticular formation of the cat: a quantitative retrograde tracing study. J Comp Neurol (1997) 388:228–49. doi:10.1002/(SICI)1096-9861(19971117)388:2<228::AID-CNE4>3.0.CO;2-3
138. Kably B, Drew T. Corticoreticular pathways in the cat. I. Projection patterns and collateralization. J Neurophysiol (1998) 80:389–405.
139. Canedo A, Lamas JA. Pyramidal and corticospinal synaptic effects over reticulospinal neurones in the cat. J Physiol (1993) 463:475–89. doi:10.1113/jphysiol.1993.sp019606
140. Magni F, Willis WD. Cortical control of brain stem reticular neurons. Arch Ital Biol (1964) 102:418–33.
141. Eccles JC, Nicoll RA, Schwarz WF, Taborikova H, Willey TJ. Reticulospinal neurons with and without monosynaptic inputs from cerebellar nuclei. J Neurophysiol (1975) 38:513–30.
142. Takahashi M, Sugiuchi Y, Shinoda Y. Convergent synaptic inputs from the caudal fastigial nucleus and the superior colliculus onto pontine and pontomedullary reticulospinal neurons. J Neurophysiol (2014) 111:849–67. doi:10.1152/jn.00634.2013
143. Matsuyama K, Jankowska E. Coupling between feline cerebellum (fastigial neurons) and motoneurons innervating hindlimb muscles. J Neurophysiol (2004) 91:1183–92. doi:10.1152/jn.00896.2003
144. Yeomans JS, Frankland PW. The acoustic startle reflex: neurons and connections. Brain Res Brain Res Rev (1995) 21:301–14. doi:10.1016/0165-0173(96)00004-5
145. Lingenhohl K, Friauf E. Giant neurons in the rat reticular formation: a sensorimotor interface in the elementary acoustic startle circuit? J Neurosci (1994) 14:1176–94.
146. Davis M, Gendelman DS, Tischler MD, Gendelman PM. A primary acoustic startle circuit: lesion and stimulation studies. J Neurosci (1982) 2:791–805.
147. Lingenhohl K, Friauf E. Giant neurons in the caudal pontine reticular formation receive short latency acoustic input: an intracellular recording and HRP-study in the rat. J Comp Neurol (1992) 325:473–92. doi:10.1002/cne.903250403
148. Nodal FR, Lopez DE. Direct input from cochlear root neurons to pontine reticulospinal neurons in albino rat. J Comp Neurol (2003) 460:80–93. doi:10.1002/cne.10656
149. Li L, Steidl S, Yeomans JS. Contributions of the vestibular nucleus and vestibulospinal tract to the startle reflex. Neuroscience (2001) 106:811–21. doi:10.1016/S0306-4522(01)00324-4
150. Steidl S, Faerman P, Li L, Yeomans JS. Kynurenate in the pontine reticular formation inhibits acoustic and trigeminal nucleus-evoked startle, but not vestibular nucleus-evoked startle. Neuroscience (2004) 126:127–36. doi:10.1016/j.neuroscience.2004.03.020
151. Shik M, Severin F, Orlovskii G. [Control of walking and running by means of electric stimulation of the midbrain]. Biofizika (1966) 11:659–66.
152. Ryczko D, Dubuc R. The multifunctional mesencephalic locomotor region. Curr Pharm Des (2013) 19:4448–70. doi:10.2174/1381612811319240011
154. Garcia-Rill E, Skinner RD. The mesencephalic locomotor region. II. Projections to reticulospinal neurons. Brain Res (1987) 411:13–20. doi:10.1016/0006-8993(87)90676-7
155. Shefchyk SJ, Jordan LM. Excitatory and inhibitory postsynaptic potentials in alpha-motoneurons produced during fictive locomotion by stimulation of the mesencephalic locomotor region. J Neurophysiol (1985) 53:1345–55.
156. Noga BR, Kriellaars DJ, Brownstone RM, Jordan LM. Mechanism for activation of locomotor centers in the spinal cord by stimulation of the mesencephalic locomotor region. J Neurophysiol (2003) 90:1464–78. doi:10.1152/jn.00034.2003
157. Pompeiano O, Wand P, Srivastava UC. Responses of Renshaw cells coupled with hindlimb extensor motoneurons to sinusoidal stimulation of labyrinth receptors in the decerebrate cat. Pflugers Arch (1985) 403:245–57. doi:10.1007/BF00583595
158. Ross HG, Thewissen M. Inhibitory connections of ipsilateral semicircular canal afferents onto Renshaw cells in the lumbar spinal cord of the cat. J Physiol (1987) 388:83–99. doi:10.1113/jphysiol.1987.sp016603
159. Jankowska E, Krutki P, Matsuyama K. Relative contribution of Ia inhibitory interneurones to inhibition of feline contralateral motoneurones evoked via commissural interneurones. J Physiol (2005) 568:617–28. doi:10.1113/jphysiol.2005.088351
160. Hultborn H, Illert M, Santini M. Convergence on interneurones mediating the reciprocal Ia inhibition of motoneurones. III. Effects from supraspinal pathways. Acta Physiol Scand (1976) 96:368–91. doi:10.1111/j.1748-1716.1976.tb10188.x
161. Anker AR, Sadacca BF, Yates BJ. Vestibular inputs to propriospinal interneurons in the feline C1-C2 spinal cord projecting to the C5-C6 ventral horn. Exp Brain Res (2006) 170:39–51. doi:10.1007/s00221-005-0186-8
162. Alstermark B, Lundberg A, Pinter M, Sasaki S. Subpopulations and functions of long C3-C5 propriospinal neurones. Brain Res (1987) 404:395–400. doi:10.1016/0006-8993(87)91400-4
163. Skinner RD, Remmel RS, Minor LB. Monosynaptic activation of long descending propriospinal neurons from the lateral vestibular nucleus and the medial longitudinal fasciculus. Exp Neurol (1984) 86:462–72. doi:10.1016/0014-4886(84)90081-5
164. Bolton PS, Goto T, Wilson VJ. Commissural neurons in the cat upper cervical spinal cord. Neuroreport (1991) 2:743–6. doi:10.1097/00001756-199112000-00003
165. Krutki P, Jankowska E, Edgley SA. Are crossed actions of reticulospinal and vestibulospinal neurons on feline motoneurons mediated by the same or separate commissural neurons? J Neurosci (2003) 23:8041–50.
166. Kasumacic N, Lambert FM, Coulon P, Bras H, Vinay L, Perreault MC, et al. Segmental organization of vestibulospinal inputs to spinal interneurons mediating crossed activation of thoracolumbar motoneurons in the neonatal mouse. J Neurosci (2015) 35:8158–69. doi:10.1523/JNEUROSCI.5188-14.2015
167. Wilson VJ. Convergence of neck and vestibular signals on spinal interneurons. Prog Brain Res (1988) 76:137–43. doi:10.1016/S0079-6123(08)64499-4
168. Schor RH, Suzuki I, Timerick SJ, Wilson VJ. Responses of interneurons in the cat cervical cord to vestibular tilt stimulation. J Neurophysiol (1986) 56:1147–56.
169. Suzuki I, Timerick SJ, Wilson VJ. Body position with respect to the head or body position in space is coded by lumbar interneurons. J Neurophysiol (1985) 54:123–33.
170. Brown AG, Fyffe RE. The morphology of group Ia afferent fibre collaterals in the spinal cord of the cat. J Physiol (1978) 274:111–27. doi:10.1113/jphysiol.1978.sp012137
171. Fyffe RE. The morphology of group II muscle afferent fibre collaterals. J Physiol (1979) 296:39–40.
172. Kuypers HGJM. Anatomy of the descending pathways. In: Brookhart JM, Mountcastle VB, editors. Handbook of Physiology. Section 1: The Nervous System, Volume II, Motor Control, Part 1. Bethesda: American Physiological Society (1981). p. 597–666. doi:10.1002/cphy.cp010213
173. Gottschall JS, Nichols TR. Head pitch affects muscle activity in the decerebrate cat hindlimb during walking. Exp Brain Res (2007) 182:131–5. doi:10.1007/s00221-007-1084-z
174. Gottschall JS, Nichols TR. Neuromuscular strategies for the transitions between level and hill surfaces during walking. Philos Trans R Soc Lond B Biol Sci (2011) 366:1565–79. doi:10.1098/rstb.2010.0355
175. Honeycutt CF, Gottschall JS, Nichols TR. Electromyographic responses from the hindlimb muscles of the decerebrate cat to horizontal support surface perturbations. J Neurophysiol (2009) 101:2751–61. doi:10.1152/jn.91040.2008
176. Carli G, Diete-Spiff K, Pompeiano O. Responses of the muscle spindles and of the extrafusal fibres in an extensor muscle to stimulation of the lateral vestibular nucleus in the cat. Arch Ital Biol (1967) 105:209–42.
177. Diete-Spiff K, Carli G, Pompeiano O. Comparison of the effects of stimulation of the 8th cranial nerve, the vestibular nuclei or the reticular formation on the gastrocnemius muscle and its spindles. Arch Ital Biol (1967) 105:243–72.
178. Pompeiano O, Diete-Spiff K, Carli G. Two pathways transmitting vestibulospinal influences from the lateral vestibular nucleus of Deiters to extensor fusimotor neurones. Pflugers Arch Gesamte Physiol Menschen Tiere (1967) 293:272–5. doi:10.1007/BF00417124
179. Kasper J, Wilson VJ, Yamagata Y, Yates BJ. Neck muscle spindle activity in the decerebrate, unparalyzed cat: dynamics and influence of vestibular stimulation. J Neurophysiol (1989) 62:917–23.
180. Bent LR, Bolton PS, Macefield VG. Vestibular inputs do not influence the fusimotor system in relaxed muscles of the human leg. Exp Brain Res (2007) 180:97–103. doi:10.1007/s00221-006-0836-5
181. Knellwolf TP, Hammam E, Macefield VG. The vestibular system does not modulate fusimotor drive to muscle spindles in relaxed leg muscles of subjects in a near-vertical position. J Neurophysiol (2016) 115:2529–35. doi:10.1152/jn.01125.2015
182. Kerman IA, Yates BJ. Regional and functional differences in the distribution of vestibulosympathetic reflexes. Am J Physiol (1998) 275:R824–35.
183. Yates BJ, Yamagata Y, Bolton PS. The ventrolateral medulla of the cat mediates vestibulosympathetic reflexes. Brain Res (1991) 552:265–72. doi:10.1016/0006-8993(91)90091-9
184. DeStefino VJ, Reighard DA, Sugiyama Y, Suzuki T, Cotter LA, Larson MG, et al. Responses of neurons in the rostral ventrolateral medulla to whole body rotations: comparisons in decerebrate and conscious cats. J Appl Physiol (2011) 110:1699–707. doi:10.1152/japplphysiol.00180.2011
185. Lovick TA. The periaqueductal gray-rostral medulla connection in the defence reaction: efferent pathways and descending control mechanisms. Behav Brain Res (1993) 58:19–25. doi:10.1016/0166-4328(93)90087-7
186. Felder RB, Mifflin SW. Modulation of carotid sinus afferent input to nucleus tractus solitarius by parabrachial nucleus stimulation. Circ Res (1988) 63:35–49. doi:10.1161/01.RES.63.1.35
187. Hamilton RB, Ellenberger H, Liskowsky D, Schneiderman N. Parabrachial area as mediator of bradycardia in rabbits. J Auton Nerv Syst (1981) 4:261–81. doi:10.1016/0165-1838(81)90049-7
188. Herbert H, Moga MM, Saper CB. Connections of the parabrachial nucleus with the nucleus of the solitary tract and the medullary reticular formation in the rat. J Comp Neurol (1990) 293:540–80. doi:10.1002/cne.902930404
189. Krukoff TL, Harris KH, Jhamandas JH. Efferent projections from the parabrachial nucleus demonstrated with the anterograde tracer Phaseolus vulgaris leucoagglutinin. Brain Res Bull (1993) 30:163–72. doi:10.1016/0361-9230(93)90054-F
190. Mifflin SW, Felder RB. Synaptic mechanisms regulating cardiovascular afferent inputs to solitary tract nucleus. Am J Physiol (1990) 259:H653–61.
191. Mraovitch S, Kumada M, Reis DJ. Role of the nucleus parabrachialis in cardiovascular regulation in cat. Brain Res (1982) 232:57–75. doi:10.1016/0006-8993(82)90610-2
192. Paton JF, Silva-Carvalho L, Thompson CS, Spyer KM. Nucleus tractus solitarius as mediator of evoked parabrachial cardiovascular responses in the decerebrate rabbit. J Physiol (1990) 428:693–705. doi:10.1113/jphysiol.1990.sp018235
193. Saper CB, Loewy AD. Efferent connections of the parabrachial nucleus in the rat. Brain Res (1980) 197:291–317. doi:10.1016/0006-8993(80)91117-8
194. Sapru HN. Role of the hypothalamic arcuate nucleus in cardiovascular regulation. Auton Neurosci (2013) 175:38–50. doi:10.1016/j.autneu.2012.10.016
195. Bowman BR, Kumar NN, Hassan SF, McMullan S, Goodchild AK. Brain sources of inhibitory input to the rat rostral ventrolateral medulla. J Comp Neurol (2013) 521:213–32. doi:10.1002/cne.23175
196. Kawabe T, Chitravanshi VC, Kawabe K, Sapru HN. Cardiovascular function of a glutamatergic projection from the hypothalamic paraventricular nucleus to the nucleus tractus solitarius in the rat. Neuroscience (2008) 153:605–17. doi:10.1016/j.neuroscience.2008.02.076
197. Horiuchi J, McDowall LM, Dampney RA. Differential control of cardiac and sympathetic vasomotor activity from the dorsomedial hypothalamus. Clin Exp Pharmacol Physiol (2006) 33:1265–8. doi:10.1111/j.1440-1681.2006.04522.x
198. Cravo SL, Possas OS, Ferreira-Neto ML. Rostral ventrolateral medulla: an integrative site for muscle vasodilation during defense-alerting reactions. Cell Mol Neurobiol (2003) 23:579–95. doi:10.1023/A:1025076130854
199. Fontes MA, Tagawa T, Polson JW, Cavanagh SJ, Dampney RA. Descending pathways mediating cardiovascular response from dorsomedial hypothalamic nucleus. Am J Physiol Heart Circ Physiol (2001) 280:H2891–901.
200. Coote JH, Yang Z, Pyner S, Deering J. Control of sympathetic outflows by the hypothalamic paraventricular nucleus. Clin Exp Pharmacol Physiol (1998) 25:461–3. doi:10.1111/j.1440-1681.1998.tb02235.x
201. Badoer E. Neurons in the hypothalamic paraventricular nucleus that project to the rostral ventrolateral medulla are not activated by hypotension. Brain Res (1998) 801:224–7. doi:10.1016/S0006-8993(98)00560-5
202. Kawano H, Masuko S. Substance P innervation of neurons projecting to the paraventricular hypothalamic nucleus in the rat nucleus tractus solitarius. Brain Res (1995) 689:136–40. doi:10.1016/0006-8993(95)00501-G
203. Martin DS, Haywood JR. Hemodynamic responses to paraventricular nucleus disinhibition with bicuculline in conscious rats. Am J Physiol (1993) 265:H1727–33.
204. Ebihara H, Kawasaki H, Nakamura S, Takasaki K, Wada A. Pressor response to microinjection of clonidine into the hypothalamic paraventricular nucleus in conscious rats. Brain Res (1993) 624:44–52. doi:10.1016/0006-8993(93)90058-U
205. Martin DS, Haywood JR. Sympathetic nervous system activation by glutamate injections into the paraventricular nucleus. Brain Res (1992) 577:261–7. doi:10.1016/0006-8993(92)90282-E
206. Cechetto DF, Chen SJ. Hypothalamic and cortical sympathetic responses relay in the medulla of the rat. Am J Physiol (1992) 263:R544–52.
207. Allen GV, Cechetto DF. Functional and anatomical organization of cardiovascular pressor and depressor sites in the lateral hypothalamic area: I. Descending projections. J Comp Neurol (1992) 315:313–32. doi:10.1002/cne.903150307
208. Markgraf CG, Winters RW, Liskowsky DR, McCabe PM, Green EJ, Schneiderman N. Hypothalamic, midbrain and bulbar areas involved in the defense reaction in rabbits. Physiol Behav (1991) 49:493–500. doi:10.1016/0031-9384(91)90270-X
209. Wible JH Jr, Luft FC, DiMicco JA. Hypothalamic GABA suppresses sympathetic outflow to the cardiovascular system. Am J Physiol (1988) 254:R680–7.
210. Mifflin SW, Spyer KM, Withington-Wray DJ. Baroreceptor inputs to the nucleus tractus solitarius in the cat: modulation by the hypothalamus. J Physiol (1988) 399:369–87. doi:10.1113/jphysiol.1988.sp017085
211. Jordan D, Mifflin SW, Spyer KM. Hypothalamic inhibition of neurones in the nucleus tractus solitarius of the cat is GABA mediated. J Physiol (1988) 399:389–404. doi:10.1113/jphysiol.1988.sp017087
212. van der Kooy D, Koda LY, McGinty JF, Gerfen CR, Bloom FE. The organization of projections from the cortex, amygdala, and hypothalamus to the nucleus of the solitary tract in rat. J Comp Neurol (1984) 224:1–24. doi:10.1002/cne.902240102
213. Kannan H, Yamashita H. Electrophysiological study of paraventricular nucleus neurons projecting to the dorsomedial medulla and their response to baroreceptor stimulation in rats. Brain Res (1983) 279:31–40. doi:10.1016/0006-8993(83)90160-9
214. Berk ML, Finkelstein JA. Efferent connections of the lateral hypothalamic area of the rat: an autoradiographic investigation. Brain Res Bull (1982) 8:511–26. doi:10.1016/0361-9230(82)90009-0
215. Ross CA, Ruggiero DA, Reis DJ. Afferent projections to cardiovascular portions of the nucleus of the tractus solitarius in the rat. Brain Res (1981) 223:402–8. doi:10.1016/0006-8993(81)91155-0
216. Saha S, Drinkhill MJ, Moore JP, Batten TF. Central nucleus of amygdala projections to rostral ventrolateral medulla neurones activated by decreased blood pressure. Eur J Neurosci (2005) 21:1921–30. doi:10.1111/j.1460-9568.2005.04023.x
217. Saha S. Role of the central nucleus of the amygdala in the control of blood pressure: descending pathways to medullary cardiovascular nuclei. Clin Exp Pharmacol Physiol (2005) 32:450–6. doi:10.1111/j.1440-1681.2005.04210.x
218. Schwaber JS, Kapp BS, Higgins GA, Rapp PR. Amygdaloid and basal forebrain direct connections with the nucleus of the solitary tract and the dorsal motor nucleus. J Neurosci (1982) 2:1424–38.
219. Kapp BS, Gallagher M, Underwood MD, McNall CL, Whitehorn D. Cardiovascular responses elicited by electrical stimulation of the amygdala central nucleus in the rabbit. Brain Res (1982) 234:251–62. doi:10.1016/0006-8993(82)90866-6
220. Verberne AJ, Owens NC. Cortical modulation of the cardiovascular system. Prog Neurobiol (1998) 54:149–68. doi:10.1016/S0301-0082(97)00056-7
221. Cechetto DF, Chen SJ. Subcortical sites mediating sympathetic responses from insular cortex in rats. Am J Physiol (1990) 258:R245–55.
222. Shipley MT. Insular cortex projection to the nucleus of the solitary tract and brainstem visceromotor regions in the mouse. Brain Res Bull (1982) 8:139–48. doi:10.1016/0361-9230(82)90040-5
223. Sevoz-Couche C, Comet MA, Bernard JF, Hamon M, Laguzzi R. Cardiac baroreflex facilitation evoked by hypothalamus and prefrontal cortex stimulation: role of the nucleus tractus solitarius 5-HT2A receptors. Am J Physiol Regul Integr Comp Physiol (2006) 291:R1007–15. doi:10.1152/ajpregu.00052.2006
224. Gabbott PL, Warner TA, Jays PR, Salway P, Busby SJ. Prefrontal cortex in the rat: projections to subcortical autonomic, motor, and limbic centers. J Comp Neurol (2005) 492:145–77. doi:10.1002/cne.20738
225. Owens NC, Verberne AJ. Medial prefrontal depressor response: involvement of the rostral and caudal ventrolateral medulla in the rat. J Auton Nerv Syst (2000) 78:86–93. doi:10.1016/S0165-1838(99)00062-4
226. Boyle R. Activity of medial vestibulospinal tract cells during rotation and ocular movement in the alert squirrel monkey. J Neurophysiol (1993) 70:2176–80.
227. Gdowski GT, McCrea RA. Integration of vestibular and head movement signals in the vestibular nuclei during whole-body rotation. J Neurophysiol (1999) 82:436–49.
228. Cullen KE, Roy JE. Signal processing in the vestibular system during active versus passive head movements. J Neurophysiol (2004) 91:1919–33. doi:10.1152/jn.00988.2003
229. McCrea RA, Gdowski GT, Boyle R, Belton T. Firing behavior of vestibular neurons during active and passive head movements: vestibulo-spinal and other non-eye-movement related neurons. J Neurophysiol (1999) 82:416–28.
230. Cullen KE, Minor LB. Semicircular canal afferents similarly encode active and passive head-on-body rotations: implications for the role of vestibular efference. J Neurosci (2002) 22:RC226.
231. Roy JE, Cullen KE. Selective processing of vestibular reafference during self-generated head motion. J Neurosci (2001) 21:2131–42.
232. Roy JE, Cullen KE. Dissociating self-generated from passively applied head motion: neural mechanisms in the vestibular nuclei. J Neurosci (2004) 24:2102–11. doi:10.1523/JNEUROSCI.3988-03.2004
233. Brooks JX, Cullen KE. The primate cerebellum selectively encodes unexpected self-motion. Curr Biol (2013) 23:947–55. doi:10.1016/j.cub.2013.04.029
234. Brooks JX, Cullen KE. Early vestibular processing does not discriminate active from passive self-motion if there is a discrepancy between predicted and actual proprioceptive feedback. J Neurophysiol (2014) 111:2465–78. doi:10.1152/jn.00600.2013
235. Pompeiano O. The vestibulo-ocular and the vestibulospinal reflexes: noradrenergic influences on the plastic changes which affect the cerebellar cortex during vestibular adaptation. Arch Ital Biol (2006) 144:197–253.
236. Lund S, Pompeiano O. Monosynaptic excitation of alpha motoneurones from supraspinal structures in the cat. Acta Physiol Scand (1968) 73:1–21. doi:10.1111/j.1748-1716.1968.tb04075.x
237. Llinas R. Mechanisms of supraspinal actions upon spinal cord activities. differences between reticular and cerebellar inhibitory actions upon alpha extensor motoneurons. J Neurophysiol (1964) 27:1117–26.
238. McCall AA, Moy JD, Puterbaugh SR, DeMayo WM, Yates BJ. Responses of vestibular nucleus neurons to inputs from the hindlimb are enhanced following a bilateral labyrinthectomy. J Appl Physiol (2013) 114:742–51. doi:10.1152/japplphysiol.01389.2012
239. Jian BJ, Shintani T, Emanuel BA, Yates BJ. Convergence of limb, visceral, and vertical semicircular canal or otolith inputs onto vestibular nucleus neurons. Exp Brain Res (2002) 144:247–57. doi:10.1007/s00221-002-1042-8
240. Miller DM, DeMayo WM, Bourdages GH, Wittman S, Yates BJ, McCall AA. Neurons in the pontomedullary reticular formation receive converging inputs from the hindlimb and labyrinth. Exp Brain Res (2017) 235:1195–1207. doi:10.1007/s00221-017-4875-x
241. Wissel J, Manack A, Brainin M. Toward an epidemiology of poststroke spasticity. Neurology (2013) 80:S13–9. doi:10.1212/WNL.0b013e3182762448
242. Urban PP, Wolf T, Uebele M, Marx JJ, Vogt T, Stoeter P, et al. Occurrence and clinical predictors of spasticity after ischemic stroke. Stroke (2010) 41:2016–20. doi:10.1161/STROKEAHA.110.581991
244. Katz RT, Rymer WZ. Spastic hypertonia: mechanisms and measurement. Arch Phys Med Rehabil (1989) 70:144–55.
247. Burke D, Knowles L, Andrews C, Ashby P. Spasticity, decerebrate rigidity and the clasp-knife phenomenon: an experimental study in the cat. Brain (1972) 95:31–48. doi:10.1093/brain/95.1.31
248. Miller DM, Klein CS, Suresh NL, Rymer WZ. Asymmetries in vestibular evoked myogenic potentials in chronic stroke survivors with spastic hypertonia: evidence for a vestibulospinal role. Clin Neurophysiol (2014) 125:2070–8. doi:10.1016/j.clinph.2014.01.035
249. Wilson TD, Cotter LA, Draper JA, Misra SP, Rice CD, Cass SP, et al. Vestibular inputs elicit patterned changes in limb blood flow in conscious cats. J Physiol (2006) 575:671–84. doi:10.1113/jphysiol.2006.112904
250. Yavorcik KJ, Reighard DA, Misra SP, Cotter LA, Cass SP, Wilson TD, et al. Effects of postural changes and removal of vestibular inputs on blood flow to and from the hindlimb of conscious felines. Am J Physiol Regul Integr Comp Physiol (2009) 297:R1777–84. doi:10.1152/ajpregu.00551.2009
251. Barman SM, Sugiyama Y, Suzuki T, Cotter LA, DeStefino VJ, Reighard DA, et al. Rhythmic activity of neurons in the rostral ventrolateral medulla of conscious cats: effect of removal of vestibular inputs. Am J Physiol Regul Integr Comp Physiol (2011) 301:R937–46. doi:10.1152/ajpregu.00265.2011
252. Fadel PJ, Raven PB. Human investigations into the arterial and cardiopulmonary baroreflexes during exercise. Exp Physiol (2012) 97:39–50. doi:10.1113/expphysiol.2011.057554
253. Waldrop TG, Eldridge FL, Iwamoto GA, Mitchell JH. Central neural control of respiration and circulation during exercise. In: Rowell LB, Shepherd JT, editors. Handbook of Physiology, Section 12, Exercise: Regulation and Integration of Multiple Systems. New York: Oxford University Press (1996). p. 333–80. doi:10.1002/cphy.cp120109
254. Goodwin GM, McCloskey DI, Mitchell JH. Cardiovascular and respiratory responses to changes in central command during isometric exercise at constant muscle tension. J Physiol (1972) 226:173–90. doi:10.1113/jphysiol.1972.sp009979
255. Gandevia SC, Killian K, McKenzie DK, Crawford M, Allen GM, Gorman RB, et al. Respiratory sensations, cardiovascular control, kinaesthesia and transcranial stimulation during paralysis in humans. J Physiol (1993) 470:85–107. doi:10.1113/jphysiol.1993.sp019849
256. Matsukawa K. Central command: control of cardiac sympathetic and vagal efferent nerve activity and the arterial baroreflex during spontaneous motor behaviour in animals. Exp Physiol (2012) 97:20–8. doi:10.1113/expphysiol.2011.057661
Keywords: vestibular nuclei, reticular formation, posture, balance, vestibulo-autonomic interactions, sympathetic nervous system
Citation: McCall AA, Miller DM and Yates BJ (2017) Descending Influences on Vestibulospinal and Vestibulosympathetic Reflexes. Front. Neurol. 8:112. doi: 10.3389/fneur.2017.00112
Received: 16 January 2017; Accepted: 09 March 2017;
Published: 27 March 2017
Edited by:
Bernard Cohen, Icahn School of Medicine at Mount Sinai, USAReviewed by:
William Michael King, University of Michigan, USARichard Nichols, Georgia Institute of Technology, USA
Copyright: © 2017 McCall, Miller and Yates. This is an open-access article distributed under the terms of the Creative Commons Attribution License (CC BY). The use, distribution or reproduction in other forums is permitted, provided the original author(s) or licensor are credited and that the original publication in this journal is cited, in accordance with accepted academic practice. No use, distribution or reproduction is permitted which does not comply with these terms.
*Correspondence: Bill J. Yates, YnlhdGVzQHBpdHQuZWR1