- Neural Plasticity and Neurorehabilitation Laboratory, Chan Division of Occupational Science and Occupational Therapy, Division of Biokinesiology and Physical Therapy, Department of Neurology, Stevens Neuroimaging and Informatics Institute, University of Southern California, Los Angeles, CA, USA
Transcranial direct current stimulation (tDCS) is a non-invasive brain stimulation method to modulate the local field potential in neural tissue and consequently, cortical excitability. As tDCS is relatively portable, affordable, and accessible, the applications of tDCS to probe brain–behavior connections have rapidly increased in the last 10 years. One of the most promising applications is the use of tDCS to modulate excitability in the motor cortex after stroke and promote motor recovery. However, the results of clinical studies implementing tDCS to modulate motor excitability have been highly variable, with some studies demonstrating that as many as 50% or more of patients fail to show a response to stimulation. Much effort has therefore been dedicated to understand the sources of variability affecting tDCS efficacy. Possible suspects include the placement of the electrodes, task parameters during stimulation, dosing (current amplitude, duration of stimulation, frequency of stimulation), individual states (e.g., anxiety, motivation, attention), and more. In this review, we first briefly review potential sources of variability specific to stroke motor recovery following tDCS. We then examine how the anatomical variability in tDCS placement [e.g., neural target(s) and montages employed] may alter the neuromodulatory effects that tDCS exerts on the post-stroke motor system.
Introduction
Stroke is a neurological deficit induced by the interruption of the blood flow to the brain due to either a vessel occlusion or less frequently an intracerebral hemorrhage (1). Both may induce direct damage of brain tissue at the site of the lesion, along with potential for additional damage in the surrounding tissue, and long-range dysfunction through the interruption of structural and functional pathways in the brain. This also leads to a deregulation of cortical excitability (2–4) and abnormal interhemispheric interactions. Stroke may thus induce many neurological deficits and could result in death. According to the World Stroke Organization, one out of six people will suffer from a stroke, making stroke a leading cause of adult long-term disability worldwide (5–7). Importantly, one of the main challenges after stroke is the loss of one’s functional motor abilities. Research suggests that only 12% of stroke survivors achieve complete motor recovery by 6 months after the stroke (8). In addition, older individuals are more vulnerable to stroke and thus the incidence of stroke is expected to continue rising over the next few decades (9, 10). Accordingly, there is a need to find new potential therapeutic tools to enhance post-stroke motor recovery. Rebalancing interhemispheric interactions and/or restoring excitability in the ipsilesional hemisphere is thought to be beneficial for post-stroke motor recovery (11–17). Thus, techniques aimed at restoring functional brain activity are a promising way to enhance neural recovery after injury. Most of the literature on stroke recovery focuses on the recovery of upper limb motor function. Since the neural mechanisms involved in motor recovery of upper versus lower limbs may differ, in this review, we focus only on upper limb motor recovery after stroke.
Non-invasive brain stimulation (NIBS) techniques show strong therapeutic potential for post-stroke motor rehabilitation due to their ability to modulate cortical excitability (18–21). In particular, transcranial direct current stimulation (tDCS) has emerged as a viable neurorehabilitation tool due to its limited side-effects (22, 23) and safety [e.g., no known risk of neural damage or induction of seizures, as can be found in other NIBS methods like repetitive transcranial magnetic stimulation (rTMS) (24, 25)]. In addition, tDCS stimulators are commercially available and relatively affordable, on the order of several hundred dollars, and application of tDCS is considered relatively simple. By delivering a low-intensity direct current (between 0.5 and 2 mA) to the scalp via two saline-soaked electrodes—an anode and a cathode—tDCS can modulate the transmembrane potential of neurons, modifying cortical excitability and inducing changes in neural plasticity (see Figure 1) (26–30). In addition, recent work has attempted to enhance the spatial resolution of tDCS stimulation, using a new technique called high-definition tDCS (HD-tDCS) (31–34). With this technique, brain regions are more focally targeted using arrays of smaller electrodes arranged on the scalp (Figure 2), using multiple anodes and cathodes (see section on Focal versus Broad Stimulation for a more detailed description). Recently, there has also been increased interest in combining tDCS with imaging methods, such as fMRI or EEG, in order to better understand the local and global effects of tDCS on neural plasticity throughout the brain (35). These methods have all contributed to the growth and interest of tDCS as a viable neuromodulatory method for stroke.
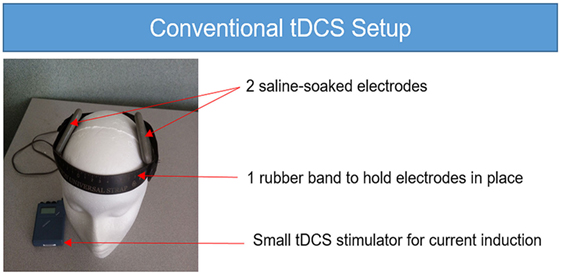
Figure 1. Conventional transcranial direct current stimulation (tDCS) setup. The conventional tDCS setup requires a small tDCS stimulator with a 9-V battery, two saline-soaked sponge electrodes and one rubber band to hold the electrodes in place on the head. While there are many options for convention tDCS, the unit shown here is the Chattanooga Iontophoresis device.
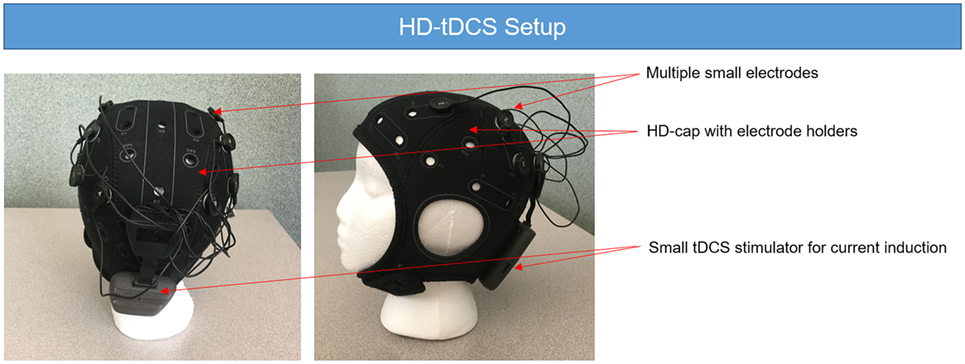
Figure 2. High-definition tDCS (HD-tDCS) setup. The HD-tDCS setup requires the use of several small electrodes to build the desire montage. The electrodes are fixed on the HD-cap. The parameters of the stimulation are stored in the small transcranial direct current stimulation (tDCS) stimulator attached at the back of the head and usually modeled using a computer program. While there are several options for HD-tDCS, the unit shown here is the Neuroelectrics Starstim device.
Accordingly, the use of tDCS to enhance motor recovery after stroke has grown rapidly in the last 10 years (see Figure 3). Despite the increase in research on tDCS for stroke motor recovery, there is much variability in the reliability of this method, with some studies finding that up to 50% or more of patients do not show a change in behavior or cortical excitability following stimulation (36–38). Several stimulation parameters may have an influence on efficacy of tDCS in individuals with stroke, including (1) the placement of the electrodes (e.g., the montage and the neural targets), (2) the intensity of the current, (3) the duration of the stimulation, (4) the timing of the stimulation (e.g., when the stimulation should be applied), (5) the use of a concomitant task (and the nature of the task), (6) the time since stroke, and (7) the stroke lesion size and location, among many other variables. Several provocative and informative reviews provide more insight into these topics (39–43). While little is known overall about the optimal stimulation parameters to enhance motor recovery, work is being done on various aspects of this question. For instance, several researchers are focusing on the optimal tDCS dose (i.e., current intensity, duration, and timing of the stimulation) (44, 45). However, the first question one might ask when designing a tDCS study is not the dose of the stimulation but which brain areas should be targeted and with which kind of stimulation (i.e., excitatory or inhibitory). While there are many conceptual models from which neural targets can be derived to potentially enhance stroke recovery, in the current review, we focus on the motor network, comprised of regions in the primary motor, premotor, supplementary motor, and cerebellar areas, as well as tangential regions, such as the dorsolateral prefrontal cortex (DLPFC). We discuss the potential of targeting each region in relation to stroke recovery. We also touch briefly on the potential reasons for the observed variability in stroke tDCS studies, and then focus more in-depth on the placement of the electrodes.
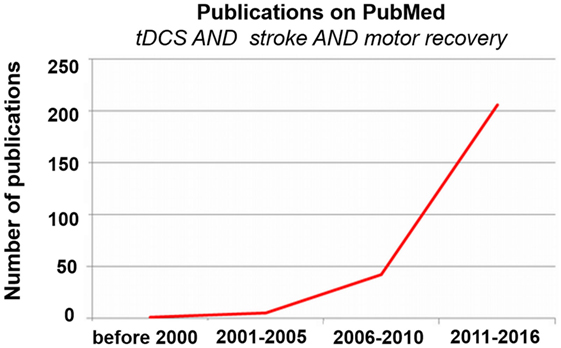
Figure 3. Publications on transcranial direct current stimulation (tDCS), stroke, and motor recovery. Exponentially increasing number of publications on tDCS, stroke, and motor recovery via PubMed over time.
Variability and Lack of Reliability of tDCS Studies in Individuals with Stroke
The variable effects of tDCS on motor performance may be due to the lack of standardization of the technical parameters used across sites. More likely, however, it is also due to (1) the heterogeneity of the populations involved in the studies (43) and (2) the variability of the motor paradigms used.
Heterogeneity of the Involved Populations
The heterogeneity of each study population is a potential source of variability for tDCS effects. However, researchers have had difficulty in finding consistent sources of variability. Studies typically examine the effects of (1) individual biological variability (e.g., metabolic/genetic variants), or, factors specific to the stroke such as, (2) time since stroke, (3) the nature and location of the stroke, and finally (4) the level of motor impairment.
Individual Biological Variability
Intrinsic individual differences in neurotransmitter levels, metabolic factors, and genetic variants provide a complex source of variability when it comes to tDCS responsiveness. For instance, one study found a relationship between individual differences in baseline measures of GABA and anodal stimulation outcomes. However, this relationship was not found between GABA and cathodal or bi-hemispheric stimulation outcomes (46). Another study showed that individual differences in the level of dopamine receptor (D1) activity influenced the excitatory effects of anodal tDCS on M1 (47). These studies suggest that there is a complex, but influential, relationship between biological factors and stimulation response.
Another factor that has been widely explored is the role of an individual’s genetic variability on tDCS response. The secretion of brain-derived neurotrophic factor (BDNF), which is involved in the synaptic plasticity, neuronal growth, long-term potentiation (LTP) formation, and long-term memory storage (48–50), seems to be enhanced by the application of tDCS (51). Moreover, an individuals’ level of BDNF seems to influence the response to NIBS and especially to tDCS (28, 52). The most common form of BDNF polymorphisms, in which an amino acid substitution between a Valine and a Methionine at position 66 on the BDNF gene [Val66Met (53)], modulate the level of BDNF expression and are thus associated with a differential modulation of brain plasticity and altered motor plasticity (54). The Val66Met polymorphism, which induces less BDNF expression, has also been shown to be associated with less-efficient motor learning and reduced responsiveness to all forms of NIBS (28, 54, 55).
However, this finding is not always consistent. For example, one study did not find any impact of the BDNF polymorphism on the neuroplastic changes induced by anodal tDCS in older adults (56), while a second one failed to find any impact of the BDNF polymorphism on the neuroplastic-induced changes associated with either tDCS, transcranial random noise stimulation, or theta burst stimulation (57). Another study demonstrated that the BDNF polymorphism did not predict responses to tDCS in patients suffering from depression but that a serotonin transporter polymorphism did (58). This suggests that the impact of the BDNF polymorphism, or more globally, genetic polymorphisms on NIBS-related effects, may be disease or task dependent.
Time after Stroke
There are also a number of factors specific to individuals with stroke that may affect stimulation efficacy. One variable is that stimulation may be delivered at different times after stroke, during which different neural plasticity mechanisms (59, 60) are active, making it difficult to compare effects across time points. In addition, the categorical definitions of “time after stroke” may be variable as well. For instance, the “chronic phase” of stroke is defined across studies as patients anywhere from 3 months or more (up to many years) after stroke (61, 62). This means that within one “category” of patients with stroke, there may be enormous variability in the plasticity processes and thus the potential tDCS effects and interactions with those processes. More precise clarification of time after stroke, using absolute terms such as months, may be useful for comparing post-stroke tDCS results.
Nature and Location of the Stroke
The location of the stroke lesion (i.e., cortical or subcortical lesion) is similarly mixed or omitted in some tDCS studies (61, 63–66). However, neural plasticity processes and cortical reorganization inducing differential recruitment of different brain regions may be dependent of the size and location of the stroke (67). Another source of stroke-specific variability is the nature of the stroke (i.e., ischemic or hemorrhagic), with some studies mixing both populations together (18, 65, 66, 68). This could also be a potential confound as the deficits and brain plasticity processes associated with cortical/subcortical localization or ischemic or hemorrhagic stroke (69–71) could be different.
Level of Motor Impairment after Stroke
Finally, the level of impairment after stroke should be carefully considered. There is a wealth of literature that suggests that patterns of neural recovery may differ for individuals based on the severity of their stroke (72–76). The recruitment of different brain regions, such as the contralesional hemisphere, may also play a different role based on the level of motor impairment. Most of the tDCS studies in patients after stroke mixed patients with different level of impairments (from mild to severe) (18, 65, 77). However, it may be important to consider an individual’s level of motor impairment and optimal pattern of recovery when applying tDCS.
Interim Conclusion
These are just a few of the factors that may contribute to the variability of tDCS responsiveness in individuals after stroke. Such wide and complex factors present challenges to reproducibility in tDCS studies of stroke motor rehabilitation. In addition to the parameters mentioned here that may contribute to variability, it is also possible that other parameters that have not yet been explored may affect the efficacy of tDCS. For instance, fluctuating state-dependent interactions (78–81) with stimulation (e.g., attention, fatigue, mood, cognitive load) may be a source of intra-individual variability that is not well-studied or controlled for. Another factor is the design of the study, and particularly the use (or lack) of a sham control group. Studies that compare the effects of tDCS to another stimulation condition (e.g., anodal stimulation to cathodal stimulation), rather than to a sham or true baseline condition, may introduce further confounds when interpreting the effects of tDCS.
Motor Paradigms during tDCS
In addition to sources of variability from the individual and stroke-specific factors, there are also many other experimental paradigm factors that could affect tDCS outcomes. While beyond the scope of the current review, there are many sources of noise that arise from the measurement of outcomes. For instance, the measurement of cortical excitability using TMS has been shown to be highly variable (82) and there is also noise within the measurement of performance on motor tasks (83, 84). In addition to measurement noise, there are also three additional sources of paradigm-related variability, specifically (1) the timing of the stimulation (e.g., before, during, or after training), (2) the motor task used, and (3) the emphasis on motor performance versus motor learning.
Time of Stimulation
Is the ideal time to apply tDCS before training to modulate the brain into an optimal state for learning, during training to reinforce training-induced plasticity, or after training to improve consolidation?
This is another question and potential source of variability when understanding the effects of tDCS on motor behavior. In healthy subjects, conflicting results show that tDCS can either be applied during motor training (85), or before training in order to increase corticospinal excitability (86). Other studies have examined stimulation after training to improve consolidation and retention (87, 88). However, the exact time during which tDCS can be applied to maximize neuroplasticity and evoke behavioral changes is still undetermined both in healthy individuals and in individuals with stroke. For instance, a single study with controversial results demonstrated that only tDCS applied before training was able to improve movement kinematics whereas tDCS applied during or after training induced degradation of the motor performance (36). However, other studies show that tDCS during training evokes similar or enhanced results compared to tDCS before motor training (63, 65, 66, 77, 89, 90).
Despite conflicting results in the literature, the most commonly used paradigm in stroke motor rehabilitation is to perform tDCS concomitantly with training on a motor task (see Table 1 for a list of studies that examined the effects of tDCS in individuals with stroke for upper limb motor recovery). Thus, while more research should be done in this area, another key question to consider is what type of task, and what parameters for the task should be implemented for optimal enhancement of function?
Motor Tasks
It seems that tDCS induces different outcomes depending on the nature of the motor task. For instance, one study showed that healthy subjects had different effects of tDCS depending on what task they performed. When they received tDCS during motor practice of a sequential finger tapping motor task, they showed online improvements. By contrast, when they received the same tDCS dose during motor practice of a visual isometric pinch force task, they only showed delayed, but not online, improvements (109). In individuals with stroke, tDCS applied during motor practice induces a strong and immediate improvement in dexterity of the fingers of the paretic hand (e.g., continuous improvement from the start of the tDCS application up until 30 min after the end of the stimulation). However, only a small delayed effect was reported on the kinematics of a paretic precision grip task (89). In addition, sensory feedback during motor performance and the bidirectional relationships within perceptual-motor tasks may also modulate the effects of tDCS on motor abilities after stroke (110, 111). These results suggest that the choice of and nature of a motor task is of crucial importance to maximize tDCS effects.
Depending on the goal or on the severity of the patients’ impairment, several tasks may be used concurrently with tDCS (Table 1). These may include simple motor tasks or more challenging tasks that engage motor, attentional and cognitive resources. The choice of task should be carefully considered in light of the variability it may introduce when used with tDCS. For more severely impaired patients who are not able to perform a motor task due to a lack of mobility or strength in the paretic hand/arm, several other paradigms have been developed to try to improve upper limb motor function. Motor imagery is one such task in which the mental representation of a movement (in individuals with stroke, a movement of the paretic upper limb) without any real/physical movement is used (112). tDCS has shown an improvement in the effects of motor imagery training in individuals with stroke (96). Another option is mirror therapy, in which a patient moves their unaffected limb and watches the movement in a mirror as though the moving limb were their affected limb. This is another alternative paradigm for more impaired patients, and, combined with tDCS, this paradigm has also demonstrated an ability to restore brain activation in the ipsilesional motor network and to enhance paretic upper limb motor function (113). Related to this, as well as the idea of perceptual-motor codes discussed previously, action observation therapy may also be useful for individuals after stroke by linking sensory and motor information to generate super-additive responses across the brain (110). These results suggest that tDCS can be an efficient add-on therapy even in the most impaired patients, although the choice of the motor task should be carefully considered.
Motor Performance versus Motor Learning in Stroke
Finally, another major source of variability when evaluating the effects of tDCS on upper limb motor recovery after stroke is the outcome being measured. In particular, two terms that are often confused are motor skill learning and motor performance. They are often used interchangeably and not well delineated in the literature, introducing variability when understanding the effects of tDCS on stroke motor recovery. By definition, improvements in motor performance result in temporary improvement, whereas motor (skill) learning is a relatively permanent change leading to the acquisition of a new motor ability (114, 115). The effects of tDCS on motor learning versus motor performance are thought to involve different neural mechanisms. Immediate tDCS effects on brain activity/excitability, measured as motor performance, are thought to be mediated through the activation of sodium and calcium channels on the neuronal membrane (116). Polarity-dependent changes of cortical excitability after tDCS have already been documented (117). tDCS induces a depolarization or a hyperpolarization of the local membrane potential, thereby modulating the rate of action potentials and thus neuronal firing (27, 118–120), which are known to be associated with the modulation of motor performance in animals (121).
By contrast, the mechanisms associated with the effects of tDCS on long-term motor learning are thought to be mediated by the activation/insertion of glutamate, NMDA/AMPA, and γ-aminobutyric (GABA) receptors in the post-synaptic membrane (22, 116, 122, 123). These receptors mediate the induction of LTP and long-term depression (LTD) and are affected during tDCS and motor learning (124). The modulation of the glutamatergic system also leads to the synthesis of key proteins such as BDNF (28, 125), which is also involved in LTP formation (126, 127). Finally, there is also evidence that tDCS directly modulates LTP and LTD (128) and BDNF expression (51), which are the basic mechanisms involved in (motor) learning (50, 129–133).
Many researchers believe that motor learning, and especially motor skill learning, plays a key role in post-stroke recovery and neurorehabilitation (134–138). Thus, enhancement of motor skill learning is an attractive option for improving post-stroke motor recovery since it can lead to faster recovery, augment the effects of neurorehabilitation, and generalize to long-term, functional gains. Moreover, coupling tDCS and motor skill learning is likely to show long-term changes in stroke due to the similar molecular mechanisms between the two processes. For instance, both induce a change in membrane potential, increase the likelihood of neurons to fire, induce brain plasticity, and induce brain-derived neurotropic factor secretion (28, 139, 140). More plasticity during training leads to more learning and that leads to long-term gains. Currently, there are only three studies exploring the impact of tDCS on motor skill learning in individuals with stroke (Table 1). Two of them used bi-hemispheric tDCS over M1 during training on a visuomotor skill learning task and demonstrated an improvement of motor skill learning during the tDCS application. In addition, the improvement in motor skill was maintained 1 week later as shown in a delayed retention test (66, 77). The third study used cathodal tDCS over contralesional M1 during a serial reaction time task and demonstrated an improvement during a delayed retention test (90). More studies are of course needed to explore the effect of tDCS on motor skill learning specifically at the long-term retention level.
One reason for the small amount of studies exploring this topic is due to the interchangeable, but often incorrect, use of motor skill learning and simple motor performance in the literature, as well as the increased time and rigor required to carefully study long-term motor learning. However, there are more studies exploring the impact of tDCS on motor performance. These studies are split in two groups (see Table 1). The first group explored only immediate effects of tDCS on motor performance, typically in a single visit. The second group explored the repeated application of tDCS during daily neurorehabilitation therapy, such as occupational therapy, with the intent to enhance motor skill learning. However, oftentimes the “conventional therapy” is not in fact “conventional” and may be more or less rigorous than that which actually occurs in normal clinical practice. The use of this term without a careful description of what was actually done leads to a large amount of variability in the design of the therapy (intensity, regularity, amount of stimulation received) and the selected task [if a skilled movement is actually defined and trained (141, 142)]. Moreover, these studies did not specifically select a motor learning task and instead used general therapy, which could consist of diverse, goal-directed actions, or practice. This makes it difficult to measure actual changes in motor learning, as opposed to improvements in motor impairment or function. While these studies generally show positive effects that translate to real-world gains, it is difficult to say whether the tDCS was effective due to its role in driving motor learning, another mechanism for improvement. Given the extreme variability, it is not surprising that no clear and consistent impacts of tDCS have emerged.
Electrode Placement
In addition to the aforementioned sources of variability, one of the key considerations in using tDCS to improve upper limb motor performance after stroke is where to stimulate the brain (e.g., neural target) and how (e.g., excitatory or inhibitory stimulation). The localization and polarity of the electrodes are especially critical in individuals with stroke due to the lesion location and potential spread of functional reorganization in the post-stroke brain (143). tDCS requires the use of at least two electrodes—one anode and one cathode. Under the anode, the resting membrane potential of the tissue depolarizes, leading to an increase in neuronal excitability. Under the cathode, the resting membrane potential of the tissue hyperpolarizes, leading to an inhibition of neural activity (27). In this next section, we will discuss the choice of the neural targets to improve motor behavior after stroke and the potential montage options.
Neural Targets
Neuroimaging studies have identified sensorimotor and premotor areas as key targets associated with functional motor recovery after stroke (17, 144, 145). NIBS allows researchers to take these neuroimaging findings and test the causal relationship between identified regions and functional recovery by stimulating the regions and observing the effects. In this section, we discuss key neural targets for tDCS to enhance stroke motor recovery.
Primary Motor Cortex
The primary motor cortex (M1) is located in the dorsal portion of the frontal lobe along the precentral gyrus. It is one of the principal brain areas involved in motor function and works to plan and execute movements (146, 147). Signals from M1 cross the body’s midline to activate the opposite side of the body (i.e., the left hemisphere controls movements on the right side of the body). Every part of the body is represented in M1, and these representations are arranged somatotopically. The amount of brain matter dedicated to any body part is related to the amount of control that M1 has over that body part (148). Motor neurons that originate in the motor cortex aggregate in fibers that travel from the cerebral cortex to the brain stem. This tract is named the corticospinal tract. A stroke that affects the corticospinal tract can induce motor impairments. The following sections describe why M1 has been and still is a neural target of choice in order to improve motor recovery after stroke.
In healthy individuals, each hemisphere provides reciprocal inhibitory connections to the other, which are thought to promote coordination between the two hemispheres (19, 149). However, after a stroke, these normal inhibitory connections can become abnormal and lead to greater dysfunction. The interhemispheric rivalry hypothesis suggests that the contralesional M1 may exert an abnormal increase in interhemispheric inhibition of the ipsilesional M1 (3, 14, 15, 150), inducing an additional dysfunction in the ipsilesional hemisphere. Motor impairments may thus arise from the lesion itself as well as from the additional abnormal inhibition patterns coming from the contralesional hemisphere. This can lead to a decrease in excitatory output from the ipsilesional M1 and interfere with functional motor recovery on the affected side. Accordingly, excitation of ipsilesional M1 and/or inhibition of contralesional M1 may be viable options in stroke studies to enhance motor function (151).
Since Priori and collaborators in 1998 described the tDCS as a potential way to modify cortical excitability (26), the primary motor cortex (M1) has become a choice target for most stroke tDCS paradigms. It is worth noting that the emphasis on M1, even in healthy volunteers, may also reflect a bias due to the ease of targeting M1. Stimulation of M1 by TMS can evoke a measurable, and visually observable, movement in the contralateral hand or arm. Typically, this effect is measured using surface electromyography in the contralateral hand and revealed as a motor-evoked potential (MEP). Stimulation over M1 allows for the measurement of amplitude and width of the MEP as a measure of changes in cortical excitability, with an increased MEP amplitude typically indicative of increased cortical excitability. M1 is thus an ideal target as it allows for both easy localization of the target area and quantifiable, measurable effects of stimulation. For other areas, alternative approaches like localization by the EEG 10-20 system (152–157) or by neuronavigation systems (158) must be used, and effects of stimulation are typically measured by performance on various behavioral tasks.
As M1 is a common target for enhancing post-stroke motor function, and specifically behavioral changes, numerous studies have examined this paradigm. Many studies have shown positive results in enhancing motor function in patients with a large range of motor deficits using tDCS (77, 90, 159). Despite positive findings, other recent studies have shown negative or null findings with M1 stimulation, revealing the high variability of observed effects of tDCS over M1 stimulation (37, 46, 102, 106). These studies suggest that some inter-individual variability could influence the response of tDCS stimulation over M1. These conflicting results (see Table 1) also suggest that a one-size-fits-all method is not the best approach for tDCS in stroke recovery, and that potentially adapting the choice of the target area, among other parameters, to each individual could lead to better gains.
Dorsal Premotor Cortex (PMd)
The PMd is part of the premotor regions and is involved in movement selection (160–163), another well-known area in the field of motor recovery. In a middle cerebral artery stroke, major portions of the primary motor and sensory cortices can be damaged, leading to motor impairments. Due to the distribution of the vasculature, PMd is often spared and is one of the remote motor areas recruited in the ipsilesional hemisphere during movements with the paretic hand after a stroke (164–166). PMd recruitment is thus thought to be a compensatory response of the brain, which supports enhanced paretic hand function (166–169). Recent studies suggest that successful motor skill learning is strongly correlated with PMd recruitment in individuals with stroke (77, 170). Accordingly, PMd is a strong tDCS target candidate for enhancing post-stroke motor recovery (145). Some studies using rTMS demonstrated an enhancement of motor function in individuals with stroke either when targeting ipsilesional (excitation) (171) or contralesional (inhibition) (172) PMd, and recently, one study confirmed the positive impact of stimulating the premotor area with tDCS in enhancing motor function after stroke (68).
The small number of studies using PMd as neural target in order to improve motor function might be explained by the assumption that given the large size of tDCS electrodes and the current spread (173), PMd could also have been peripherally stimulated during tDCS with electrodes centered over M1. The use of HD-tDCS and more focal stimulation paradigms may provide a way to disentangle these contributions.
Supplementary Motor Area (SMA)
The SMA is medial to the premotor cortex and is involved in movement control, bilateral movement coordination, and sequential motor learning (174–176). Similarly to PMd, the SMA is a region that is known to be involved with the post-stroke compensatory motor network (177, 178). Moreover, it seems that SMA recruitment is highly associated with an increased recovery of motor function (i.e., individuals with good recovery show reductions in task-evoked activation in the SMA over time, which is associated with an improvement in motor function) (179). This pattern of increased recruitment during the early stages of learning, and a reduction of this recruitment when the task has been consistently learned (180), is seen both in individuals with stroke and in healthy individuals when they learn a new motor skill. Promising results from studies on healthy individuals suggest that excitatory NIBS [both rTMS (181) and tDCS (182, 183)] over the SMA is associated with an improvement of motor skill learning. To date, there are no published studies exploring the impact of tDCS directly over the SMA to enhance motor function after stroke. However, the SMA was thought to also have been stimulated during PMd stimulation (68), which showed gains in motor function and dexterity of the paretic arm from excitatory stimulation over PMd/SMA. Moreover, in healthy individuals, SMA activation seems highly correlated with successful motor skill learning suggesting a key role of the SMA in the correct acquisition of a new motor skill (184). Since the more similar the reconfigured network is to the original undamaged network, the better the recovery (17, 185, 186), stimulating areas that are spontaneously involved during motor skill learning in healthy individuals such as the SMA could be an effective option for enhancing motor recovery.
Cerebellum
Historically, the cerebellum has been described as a part of the motor system because cerebellum lesions can lead to errors in movement control or coordination (187). A recent study also demonstrated the key role of the cerebellum and especially of lobules V and VI in sequential motor skill learning (188). Several observations have provided evidence that the cerebellum is part of the motor recovery network after stroke as well. At the metabolic level, immediately after a stroke, blood flow in the cerebellar hemisphere contralateral to the lesion (i.e., ipsilateral to the paretic arm) decreases, even if there is no lesion in the cerebellum (189). Interestingly, as the patient regains motor abilities in the first few weeks after the stroke, blood flow in the cerebellum likewise increases (190). That is, the rate of increase in blood flow in the contralesional cerebellum correlates with the rate of motor recovery in the patient, suggesting a relationship between the two. Moreover, at a functional level, the intensity of cerebellar recruitment during a motor task performed with the paretic hand is correlated with motor recovery. After several months, there is a reduction of the recruitment in this area during task practice of the affected hand, suggesting a transient use of spared neural resources in the cerebellum during the recovery process (191). Nevertheless, it has to be noted that these results suggest only a correlation between cerebellum function and motor recovery and not a causal relationship.
Recently, the ability of tDCS to modify cerebellar excitability has been demonstrated in combined tDCS–TMS experiments (192). In a visuomotor transformation paradigm, anodal tDCS over the cerebellum was shown to enhance adaptation learning (193, 194). While tDCS over the cerebellum seems to enhance post-stroke cognitive functions (195), as yet there have been no studies demonstrating that modifying cerebellar excitability with tDCS also improves post-stroke motor function.
Other Areas
In addition to these areas, several other regions of the brain could be promising targets as well. The primary and secondary motor areas have strong connections with the parietal cortex, and it may be possible to perturb the motor network through stimulation over the parietal cortex. The fronto-parietal network is largely associated with deficits in motor planning (e.g., apraxia) (196–198), and the parietal cortex is also involved in motor skill learning (199) in individuals with stroke (170). In addition, tDCS over the parietal cortex has been shown to improve hemi-neglect in individuals with stroke, which may have tangential effects on motor recovery (200). However, currently no studies have used this montage to examine post-stroke motor recovery.
The DLPFC is another region that plays an important role in working memory and may be a key component of the motor learning network both in healthy individuals (201) and individuals with stroke (202), especially for complex tasks in which there is a cognitive component (203). More studies are needed to explore the ability of tDCS on DLPFC to enhance post-stroke motor recovery.
Finally, some studies have also demonstrated an involvement of the primary somatosensory cortex (S1) in post-stroke motor recovery (204, 205). tDCS over S1 leads to variable improvements in healthy subjects and in individuals with stroke on performance in the somatosensory domain. For instance, bi-hemispheric tDCS enhanced post-stroke tactile discrimination in individuals after stroke and anodal tDCS enhanced tactile discrimination in healthy subjects. However, no effects were observed after cathodal tDCS in healthy subjects (206–208). Previous studies have shown improvements in motor learning associated with excitatory rTMS over ipsilesional S1 (209) or with continuous theta burst stimulation over the contralesional S1 (210). However, again there have not been any published studies exploring the use of tDCS directly over S1 to enhance post-stroke motor function, although it is likely also stimulated during M1 stimulation with larger electrodes. More targeted stimulation of only S1 thus represents an interesting option for future research.
Overall, it seems that while studies exploring tDCS for motor recovery are growing exponentially, they have been mainly limited in their neuroanatomical targets primarily to M1 [except for one study targeting PMd/SMA (68)]. It is possible that the lack of published studies in stroke recovery on the use of tDCS over regions besides M1 is biased due to a potential lack of any positive, significant results. If this is the case, it would be beneficial for the field of stroke recovery to encourage the publication of all tDCS studies, even the ones with no significant or negative results, to at least understand what has been tried. It is likely that stimulation over different motor regions with tDCS may lead to specific gains in specific post-stroke behavioral conditions with specific populations. In this regard, the field would benefit from better understanding where it does, and does not, work.
Monocephalic or Bi-Hemispheric Montage
In a conventional tDCS setup, tDCS is applied using two electrodes (one anode and one cathode). The position of the electrodes is typically driven by the goal of the study. Depending on this goal, tDCS can be applied in two distinct montages (monocephalic/bi-hemispheric). Nevertheless, the applied current is not restricted over the targeted area but is mode widely distributed with a hotspot around the active electrode (see next paragraph) (173).
When tDCS is applied to change excitability by targeting a single area, this is considered a monocephalic montage. In this case, one small electrode (the active electrode) (up to 50 cm2) is placed over the desired neural target (i.e., the brain area in which the excitability changes induced by the stimulation is thought to be the more useful regarding the goal of the study) and a larger neutral electrode called the “return electrode” (up to 100 cm2) is placed over the contra-orbital area. Increasing the size of the electrode (and keeping its current strength constant over time) will reduce the overall current density flowing through that electrode and thus eliminate its functional efficacy (211). To restore excitability in the ipsilesional hemisphere or rebalance interhemispheric interactions in order to enhance motor function, two different monocephalic montages are typically used: (1) upregulating the excitability of the ipsilesional hemisphere using a monocephalic montage with the anode as the active electrode over the ipsilesional hemisphere and the cathode as the return electrode (20, 91) and (2) downregulating the excitability of the contralesional hemisphere, using a monocephalic montage with the cathode as the active electrode over the contralesional hemisphere and the anode as return electrode (63, 101, 212).
tDCS can also be applied in a bi-hemispheric manner, permitting simultaneous coupling of excitatory and inhibitory effects. The bi-hemispheric montage uses two electrodes of the same size in order to modify brain excitability by targeting two neural targets. Notably, one electrode should be the anode and the other the cathode. With this montage, the effects of both monocephalic montages previously mentioned are coupled with the anode over the ipsilesional hemisphere and the cathode over the contralesional hemisphere (18, 61, 89). The effect of the bi-hemispheric montage is supposed to be linked to a modulation of interhemispheric inhibition. While interhemispheric inhibition as a result of the bi-hemispheric montage has been directly demonstrated in healthy subjects (213), to our knowledge, such a direct demonstration has not yet been performed in stroke patients. In healthy individuals, several direct comparisons have demonstrated that the use of bi-hemispheric tDCS drives at least as much improvement of motor performance as using a monocephalic montage (214, 215) and induces a stronger modulation of the connectivity between the two M1 regions compared to the monocephalic montage (216, 217). However, a single study in individuals with stroke did not demonstrate improved efficiency of bi-hemispheric tDCS in increasing post-stroke motor function compared to a monocephalic montage (46). Furthermore, a deterioration of motor function in the paretic upper limb has been observed after monocephalic cathodal tDCS (101, 218) over the contralesional hemisphere in more impaired patients whereas an improvement is observed in the less impaired patients, suggesting that the optimal parameters may depend on the severity of the stroke. This may, again, potentially explain some of the variability in tDCS stroke findings.
Finally, HD-tCDS refers to the use of more than two electrodes, usually smaller in size, in order to distribute the positive and negative current flow to target neural regions with greater spatial resolution. This is discussed further in Section “Focal versus Broad Stimulation” below.
To date, there is little clear evidence regarding the best tDCS montage to improve post-stroke motor function. Once again, the same montage may likely not work for all patients and might be tailored based on lesion location, severity of motor impairment, desired outcomes, or other factors.
Return Electrode Positioning
A related issue is the placement of the return electrode for the “monocephalic” montages. tDCS involves the flow of current through the brain, which means that there must always be a positive pole and a negative pole. However, when one wishes to only induce a positive or negative effect across the brain, the second electrode is often referred to as the “return” electrode. However, the return electrode can still evoke an effect on the tissue underneath. The primary way to avoid an effect of the return electrode is to use a larger electrode, as the larger an electrode is, the more inactive/passive it will be, since it will reduce current density induced by it (211). Many studies incorrectly mention the use of a control/return electrode when in reality, studies that use 35 cm2 electrodes (or smaller) as return electrodes are implementing bi-hemispheric tDCS and inducing a modulation over the brain area for this “return” electrode. Unfortunately, the primary way to avoid this effect is to use a large return electrode, which is not always easy and/or feasible. However, not doing this introduces the risk of an undesirable current from the return electrode.
To circumvent this problem, one option is to use an extracephalic return electrode (e.g., on the shoulder or neck). In the past, this option has been excluded to avoid stimulation of the brainstem due to safety concerns that arose from early studies (219, 220). Recently, however, several studies have demonstrated safety using extracephalic montages (221, 222). To date, only a single study testing the impact of tDCS on motor performance using an extracephalic electrode has been implemented (92). There is also one study testing the impact of tDCS with an extracephalic electrode on motor skill learning (223). While in this study, the use of tDCS did not improve motor function, future studies should continue to explore both the feasibility and the potential benefits of using an extracephalic return electrode for tDCS in the stroke population.
Focal versus Broad Stimulation
One point that is often considered a major drawback in using tDCS is the low spatial resolution of the induced current (211). In contrast to rTMS, which provides a more focal stimulation on the order of millimeters (224), the current flow delivered by conventional tDCS is not limited to the area under the electrodes for several reasons: (1) the size of the used electrodes (15–50 cm2) is oftentimes larger than the target region of interest and more likely spread to adjacent cortical areas and (2) as previously mentioned, the tDCS induced-current flow is not limited to the neural target but widely distributed contiguously between the two electrodes.
High-definition tDCS is appealing option to use tDCS in a more focal way. HD-tDCS also leads to an increase in cortical excitability, as measured by an increased MEP amplitude up to 2 h after the stimulation, compared to conventional tDCS (30). While the impact of HD-tDCS has shown an improvement of the naming accuracy on aphasia in patients with stroke (225), there are not yet any published studies showing the impact of HD-tDCS on motor function in individuals with stroke.
However, in stroke rehabilitation, the spread of current to affect many regions within a network may in fact be beneficial. While more research is needed to compare focal to broad stimulation in individuals after stroke, the effects of conventional (broad) stimulation in stroke recovery have been generally positive. The conventional tDCS setup modulates functional connectivity (FC) and regions distal to the stimulated region (226, 227) [for a review, see Liew and colleagues (35)], which is important as FC is often disrupted in individuals with stroke (4). Several studies show interesting results of connectivity changes following tDCS in stroke: (1) bi-hemispheric tDCS over Broca’s area (anode over the left hemisphere) increased FC in the left hemisphere after treatment for 3 weeks, (2) 10 sessions of conventional bi-hemispheric tDCS over M1 combined with occupational therapy induced an improvement of the FC between ipsilesional M1 and contralesional premotor cortex (62), and (3) bi-hemispheric tDCS over M1 applied in a single session combined with training on a motor skill learning task induced a reorganization of FC in the motor network with a specific improvement in FC between PMd and M1 in the ipsilesional hemisphere (228). An appealing option, made possible by HD-tDCS, could be to stimulate not only a part of the network (such as M1), but to try to specifically stimulate the entire motor network in order to enhance post-stroke motor network connectivity and subsequently motor function.
From a neurophysiological point of view, focal stimulation is thought to be superior as it allows researchers to directly target the region of interest with less stimulation on other regions. However, it is feasible that, from a neurorehabilitation point of view, concomitant stimulation of adjacent cortical areas of the motor network by conventional tDCS with larger electrodes is beneficial (135, 210). Further research is needed in these areas.
Lesioned Regions
Finally, according to modeling studies, when applying tDCS, the current flow is dependent on the electrode montage (i.e., the position and size of electrodes), the conduction of the different tissues (e.g., skin, skull, white and gray matter, cerebrospinal fluid, etc.), and on individual variation in anatomical features (229–231). Thus, an important question to address when using tDCS in participants after stroke is whether the lesion itself may perturb the current flow. This question is important since it is possible that the stroke lesions induce perturbations during stimulation, as found with rTMS over the post-stroke brain (232). Studies exploring the influence of anisotropic conductivity in the skull and white matter have demonstrated that the spatial distribution of current density is altered by changes in white matter anisotropy (233, 234) and also by variations in the gyri and sulci of each brain (235). This suggests that inter-individual variation in brain structure and integrity need to be taken into account when positioning electrodes. However, in individuals with stroke, it seems that even though there is a perturbation due to the lesion (with an increase in current density at the lesion borders), the global current density remains relatively unchanged compared to the healthy brain (236). Yet again, additional studies are needed to better understand and model current flow in the post-stroke brain.
Summary
As demonstrated in this review, the field of investigation remains wide and varied, and the path to find an efficient and well-defined therapeutic tool is still far ahead. It is possible that one of the neural targets described above may drive stronger and more reliable effects, although it is more likely that there are complex interactions between many of these stimulation parameters, including neural target, task choice, and timing and duration of stimulation. Additional studies are required to determine the best tDCS target region in order to improve post-stroke motor function and the best way to stimulate its function. On the other hand, targeting any of the areas inside the motor learning network may drive the same enhancement effect through their connections to one another. The regions noted here may all be entryways into modulation of the motor network, which are likely to induce both changes specific to each stimulated site as well as similar global changes regardless of the site. Moreover, the one-size-fits-all approach used in most studies may not be an optimal approach. As recently suggested (237), an individual neural target selection based on the functional recruitment during a specific behavior may better permit enhancement in each individual. In other words, at the individual level, the key area involved in a specific task could be selected as a neural target for tDCS application. To do this, the precise and individually based selection of the neural target using a neuronavigation system, as often used with TMS studies, would be beneficial.
Conclusion
In recent years, there has been an increase in the number of studies using tDCS to enhance post-stroke motor recovery. Despite the positive results observed in many studies, there is large variability and a lack of reliability in the literature. The optimal parameters, such as the neural target, the nature of the stimulation, the timing of stimulation in relation to a task, and more, need further exploration before tDCS can readily be used as a therapeutic tool in clinical settings. Moreover as suggested by Grefkes and Fink (238), large, randomized, multicenter controlled trials are now needed to determine the optimal design to enhance motor function after stroke using tDCS. The present review does not question the ability of tDCS to modify brain excitability, restore interhemispheric interaction, and enhance motor learning but rather suggests that to best promote neurorehabilitation processes, a better understanding of how we can maximize each parameter of use is needed.
Author Contributions
SL: performed the review and drafted the manuscript. S-LL: performed the review and drafted the manuscript. All authors edited and revised the manuscript.
Conflict of Interest Statement
The authors declare that the research was conducted in the absence of any commercial or financial relationships that could be construed as a potential conflict of interest.
Acknowledgments
The authors thank Kaori Ito for comments on an early draft.
Funding
This work was supported by the NIH Rehabilitation Research Career Development Program Grant K12 (HD055929). Its contents are solely the responsibility of the author and do not necessarily represent the official views of the NIH.
References
1. Lloyd-Jones D, Adams R, Carnethon M, De Simone G, Ferguson TB, Flegal K, et al. Heart disease and stroke statistics – 2009 update: a report from the American Heart Association Statistics Committee and Stroke Statistics Subcommittee. Circulation (2009) 119(3):480–6. doi: 10.1161/CIRCULATIONAHA.108.191259
2. Kirton A, Deveber G, Gunraj C, Chen R. Cortical excitability and interhemispheric inhibition after subcortical pediatric stroke: plastic organization and effects of rTMS. Clin Neurophysiol (2010) 121(11):1922–9. doi:10.1016/j.clinph.2010.04.021
3. Butefisch CM, Wessling M, Netz J, Seitz RJ, Homberg V. Relationship between interhemispheric inhibition and motor cortex excitability in subacute stroke patients. Neurorehabil Neural Repair (2008) 22(1):4–21. doi:10.1177/1545968307301769
4. Carter AR, Astafiev SV, Lang CE, Connor LT, Rengachary J, Strube MJ, et al. Resting interhemispheric functional magnetic resonance imaging connectivity predicts performance after stroke. Ann Neurol (2010) 67(3):365–75. doi:10.1002/ana.21905
5. Mackay J, Mensah G. WHO publishes definitive atlas on global heart disease and stroke epidemic. Atlas of Heart Disease and Stroke. Indian J Med Sci (2004) 58(9):405–6.
6. Seshadri S, Wolf PA. Lifetime risk of stroke and dementia: current concepts, and estimates from the Framingham Study. Lancet Neurol (2007) 6(12):1106–14. doi:10.1016/S1474-4422(07)70291-0
7. Seshadri S, Beiser A, Kelly-Hayes M, Kase CS, Au R, Kannel WB, et al. The lifetime risk of stroke: estimates from the Framingham study. Stroke (2006) 37(2):345–50. doi:10.1161/01.STR.0000199613.38911.b2
8. Kwakkel G, Kollen BJ, van der Grond J, Prevo AJ. Probability of regaining dexterity in the flaccid upper limb: impact of severity of paresis and time since onset in acute stroke. Stroke (2003) 34(9):2181–6. doi:10.1161/01.STR.0000087172.16305.CD
9. Donnan GA, Fisher M, Macleod M, Davis SM. Stroke. Lancet (2008) 371(9624):1612–23. doi:10.1016/S0140-6736(08)60694-7
10. Lopez AD, Mathers CD, Ezzati M, Jamison DT, Murray CJ. Global and regional burden of disease and risk factors, 2001: systematic analysis of population health data. Lancet (2006) 367(9524):1747–57. doi:10.1016/S0140-6736(06)68770-9
11. Nudo RJ. Mechanisms for recovery of motor function following cortical damage. Curr Opin Neurobiol (2006) 16(6):638–44. doi:10.1016/j.conb.2006.10.004
12. Nudo RJ, Wise BM, SiFuentes F, Milliken GW. Neural substrates for the effects of rehabilitative training on motor recovery after ischemic infarct. Science (1996) 272(5269):1791–4. doi:10.1126/science.272.5269.1791
13. Plautz EJ, Barbay S, Frost SB, Friel KM, Dancause N, Zoubina EV, et al. Post-infarct cortical plasticity and behavioral recovery using concurrent cortical stimulation and rehabilitative training: a feasibility study in primates. Neurol Res (2003) 25(8):801–10. doi:10.1179/016164103771953880
14. Murase N, Duque J, Mazzocchio R, Cohen LG. Influence of interhemispheric interactions on motor function in chronic stroke. Ann Neurol (2004) 55(3):400–9. doi:10.1002/ana.10848
15. Sauerbrei R, Liepert J. Support of the concept of interhemispheric rivalry by two consecutive strokes occurring in both hemispheres: a case study. J Neurol (2012) 259(11):2484–5. doi:10.1007/s00415-012-6560-0
16. Kirton A, Chen R, Friefeld S, Gunraj C, Pontigon AM, Deveber G. Contralesional repetitive transcranial magnetic stimulation for chronic hemiparesis in subcortical paediatric stroke: a randomised trial. Lancet Neurol (2008) 7(6):507–13. doi:10.1016/S1474-4422(08)70096-6
17. Ward NS, Brown MM, Thompson AJ, Frackowiak RS. Neural correlates of outcome after stroke: a cross-sectional fMRI study. Brain (2003) 126(Pt 6):1430–48. doi:10.1093/brain/awg145
18. Bolognini N, Vallar G, Casati C, Latif LA, El-Nazer R, Williams J, et al. Neurophysiological and behavioral effects of tDCS combined with constraint-induced movement therapy in poststroke patients. Neurorehabil Neural Repair (2011) 25(9):819–29. doi:10.1177/1545968311411056
19. Nowak DA, Grefkes C, Ameli M, Fink GR. Interhemispheric competition after stroke: brain stimulation to enhance recovery of function of the affected hand. Neurorehabil Neural Repair (2009) 23(7):641–56. doi:10.1177/1545968309336661
20. Hummel F, Celnik P, Giraux P, Floel A, Wu WH, Gerloff C, et al. Effects of non-invasive cortical stimulation on skilled motor function in chronic stroke. Brain (2005) 128:490–9. doi:10.1093/brain/awh369
21. Adeyemo BO, Simis M, Macea DD, Fregni F. Systematic review of parameters of stimulation, clinical trial design characteristics, and motor outcomes in non-invasive brain stimulation in stroke. Front Psychiatry (2012) 3:88. doi:10.3389/fpsyt.2012.00088
22. Brunoni AR, Nitsche MA, Bolognini N, Bikson M, Wagner T, Merabet L, et al. Clinical research with transcranial direct current stimulation (tDCS): challenges and future directions. Brain Stimul (2012) 5(3):175–95. doi:10.1016/j.brs.2011.03.002
23. Poreisz C, Boros K, Antal A, Paulus W. Safety aspects of transcranial direct current stimulation concerning healthy subjects and patients. Brain Res Bull (2007) 72(4–6):208–14. doi:10.1016/j.brainresbull.2007.01.004
24. Nitsche MA, Paulus W. Sustained excitability elevations induced by transcranial DC motor cortex stimulation in humans. Neurology (2001) 57(10):1899–901. doi:10.1212/WNL.57.10.1899
25. Merrill DR, Bikson M, Jefferys JG. Electrical stimulation of excitable tissue: design of efficacious and safe protocols. J Neurosci Methods (2005) 141(2):171–98. doi:10.1016/j.jneumeth.2004.10.020
26. Priori A, Berardelli A, Rona S, Accornero N, Manfredi M. Polarization of the human motor cortex through the scalp. Neuroreport (1998) 9(10):2257–60. doi:10.1097/00001756-199807130-00020
27. Nitsche MA, Paulus W. Excitability changes induced in the human motor cortex by weak transcranial direct current stimulation. J Physiol (2000) 527(Pt 3):633–9. doi:10.1111/j.1469-7793.2000.t01-1-00633.x
28. Fritsch B, Reis J, Martinowich K, Schambra HM, Ji Y, Cohen LG, et al. Direct current stimulation promotes BDNF-dependent synaptic plasticity: potential implications for motor learning. Neuron (2010) 66(2):198–204. doi:10.1016/j.neuron.2010.03.035
29. Cambiaghi M, Velikova S, Gonzalez-Rosa JJ, Cursi M, Comi G, Leocani L. Brain transcranial direct current stimulation modulates motor excitability in mice. Eur J Neurosci (2010) 31(4):704–9. doi:10.1111/j.1460-9568.2010.07092.x
30. Kuo HI, Bikson M, Datta A, Minhas P, Paulus W, Kuo MF, et al. Comparing cortical plasticity induced by conventional and high-definition 4 x 1 ring tDCS: a neurophysiological study. Brain Stimul (2013) 6(4):644–8. doi:10.1016/j.brs.2012.09.010
31. Datta A, Bansal V, Diaz J, Patel J, Reato D, Bikson M. Gyri-precise head model of transcranial direct current stimulation: improved spatial focality using a ring electrode versus conventional rectangular pad. Brain Stimul (2009) 2(4):201–7,7e1. doi:10.1016/j.brs.2009.03.005
32. Caparelli-Daquer EM, Zimmermann TJ, Mooshagian E, Parra LC, Rice JK, Datta A, et al. A pilot study on effects of 4x1 high-definition tDCS on motor cortex excitability. Conf Proc IEEE Eng Med Biol Soc (2012) 2012:735–8. doi:10.1109/EMBC.2012.6346036
33. Dmochowski JP, Datta A, Bikson M, Su Y, Parra LC. Optimized multi-electrode stimulation increases focality and intensity at target. J Neural Eng (2011) 8(4):046011. doi:10.1088/1741-2560/8/4/046011
34. Villamar MF, Volz MS, Bikson M, Datta A, Dasilva AF, Fregni F. Technique and considerations in the use of 4x1 ring high-definition transcranial direct current stimulation (HD-tDCS). J Vis Exp (2013) (77):e50309. doi:10.3791/50309
35. Liew SL, Santarnecchi E, Buch ER, Cohen LG. Non-invasive brain stimulation in neurorehabilitation: local and distant effects for motor recovery. Front Hum Neurosci (2014) 8:378. doi:10.3389/fnhum.2014.00378
36. Giacobbe V, Krebs HI, Volpe BT, Pascual-Leone A, Rykman A, Zeiarati G, et al. Transcranial direct current stimulation (tDCS) and robotic practice in chronic stroke: the dimension of timing. NeuroRehabilitation (2013) 33(1):49–56. doi:10.3233/NRE-130927
37. Straudi S, Fregni F, Martinuzzi C, Pavarelli C, Salvioli S, Basaglia N. tDCS and robotics on upper limb stroke rehabilitation: effect modification by stroke duration and type of stroke. Biomed Res Int (2016) 2016:5068127. doi:10.1155/2016/5068127
38. Di Lazzaro V, Dileone M, Capone F, Pellegrino G, Ranieri F, Musumeci G, et al. Immediate and late modulation of interhemispheric imbalance with bilateral transcranial direct current stimulation in acute stroke. Brain Stimul (2014) 7(6):841–8. doi:10.1016/j.brs.2014.10.001
39. Marquez J, van Vliet P, McElduff P, Lagopoulos J, Parsons M. Transcranial direct current stimulation (tDCS): does it have merit in stroke rehabilitation? A systematic review. Int J Stroke (2015) 10(3):306–16. doi:10.1111/ijs.12169
40. Bestmann S, de Berker AO, Bonaiuto J. Understanding the behavioural consequences of noninvasive brain stimulation. Trends Cogn Sci (2015) 19(1):13–20. doi:10.1016/j.tics.2014.10.003
41. Horvath JC, Carter O, Forte JD. Transcranial direct current stimulation: five important issues we aren’t discussing (but probably should be). Front Syst Neurosci (2014) 8:2. doi:10.3389/fnsys.2014.00002
42. de Aguiar V, Paolazzi CL, Miceli G. tDCS in post-stroke aphasia: the role of stimulation parameters, behavioral treatment and patient characteristics. Cortex (2015) 63:296–316. doi:10.1016/j.cortex.2014.08.015
43. Li LM, Uehara K, Hanakawa T. The contribution of interindividual factors to variability of response in transcranial direct current stimulation studies. Front Cell Neurosci (2015) 9:181. doi:10.3389/fncel.2015.00181
44. Chhatbar PY, Ramakrishnan V, Kautz S, George MS, Adams RJ, Feng W. Transcranial direct current stimulation post-stroke upper extremity motor recovery studies exhibit a dose-response relationship. Brain Stimul (2016) 9(1):16–26. doi:10.1016/j.brs.2015.09.002
45. Feng W, Enabore A, Kautz S, Adams RJ, Chhatbar P. Dose response relationship in transcranial direct current stimulation stroke motor recovery studies. Stroke (2015) 46(Suppl 1):A7.
46. O’Shea J, Boudrias MH, Stagg CJ, Bachtiar V, Kischka U, Blicher JU, et al. Predicting behavioural response to TDCS in chronic motor stroke. Neuroimage (2014) 85(Pt 3):924–33. doi:10.1016/j.neuroimage.2013.05.096
47. Fresnoza S, Paulus W, Nitsche MA, Kuo MF. Nonlinear dose-dependent impact of D1 receptor activation on motor cortex plasticity in humans. J Neurosci (2014) 34(7):2744–53. doi:10.1523/JNEUROSCI.3655-13.2014
48. Acheson A, Conover JC, Fandl JP, DeChiara TM, Russell M, Thadani A, et al. A BDNF autocrine loop in adult sensory neurons prevents cell death. Nature (1995) 374(6521):450–3. doi:10.1038/374450a0
49. Huang EJ, Reichardt LF. Neurotrophins: roles in neuronal development and function. Annu Rev Neurosci (2001) 24:677–736. doi:10.1146/annurev.neuro.24.1.677
50. Bekinschtein P, Cammarota M, Katche C, Slipczuk L, Rossato JI, Goldin A, et al. BDNF is essential to promote persistence of long-term memory storage. Proc Natl Acad Sci U S A (2008) 105(7):2711–6. doi:10.1073/pnas.0711863105
51. Podda MV, Cocco S, Mastrodonato A, Fusco S, Leone L, Barbati SA, et al. Anodal transcranial direct current stimulation boosts synaptic plasticity and memory in mice via epigenetic regulation of BDNF expression. Sci Rep (2016) 6:22180. doi:10.1038/srep22180
52. Wang HY, Crupi D, Liu J, Stucky A, Cruciata G, Di Rocco A, et al. Repetitive transcranial magnetic stimulation enhances BDNF-TrkB signaling in both brain and lymphocyte. J Neurosci (2011) 31(30):11044–54. doi:10.1523/JNEUROSCI.2125-11.2011
53. Lu B. BDNF and activity-dependent synaptic modulation. Learn Mem (2003) 10(2):86–98. doi:10.1101/lm.54603
54. McHughen SA, Rodriguez PF, Kleim JA, Kleim ED, Marchal Crespo L, Procaccio V, et al. BDNF val66met polymorphism influences motor system function in the human brain. Cereb Cortex (2010) 20(5):1254–62. doi:10.1093/cercor/bhp189
55. Kleim JA, Chan S, Pringle E, Schallert K, Procaccio V, Jimenez R, et al. BDNF val66met polymorphism is associated with modified experience-dependent plasticity in human motor cortex. Nat Neurosci (2006) 9(6):735–7. doi:10.1038/nn1699
56. Fujiyama H, Hyde J, Hinder MR, Kim SJ, McCormack GH, Vickers JC, et al. Delayed plastic responses to anodal tDCS in older adults. Front Aging Neurosci (2014) 6:115. doi:10.3389/fnagi.2014.00115
57. Antal A, Chaieb L, Moliadze V, Zarrouki DB, Moneef S, Paulus W. BDNF gene polymorphisms and motor cortical plasticity in healthy humans: when should we consider it? J Neurosci Rehabil (2014) 1(2):1–13. doi:10.17653/2374-9091.SS0004
58. Brunoni AR, Kemp AH, Shiozawa P, Cordeiro Q, Valiengo LC, Goulart AC, et al. Impact of 5-HTTLPR and BDNF polymorphisms on response to sertraline versus transcranial direct current stimulation: implications for the serotonergic system. Eur Neuropsychopharmacol (2013) 23(11):1530–40. doi:10.1016/j.euroneuro.2013.03.009
59. Cramer SC. Repairing the human brain after stroke: I. Mechanisms of spontaneous recovery. Ann Neurol (2008) 63(3):272–87. doi:10.1002/ana.21412
60. Carmichael ST. Brain excitability in stroke: the yin and yang of stroke progression. Arch Neurol (2012) 69(2):161–7. doi:10.1001/archneurol.2011.1175
61. Lindenberg R, Renga V, Zhu LL, Nair D, Schlaug G. Bihemispheric brain stimulation facilitates motor recovery in chronic stroke patients. Neurology (2010) 75(24):2176–84. doi:10.1212/WNL.0b013e318202013a
62. Chen JL, Schlaug G. Increased resting state connectivity between ipsilesional motor cortex and contralesional premotor cortex after transcranial direct current stimulation with physical therapy. Sci Rep (2016) 6:23271. doi:10.1038/srep23271
63. Fregni F, Boggio PS, Mansur CG, Wagner T, Ferreira MJ, Lima MC, et al. Transcranial direct current stimulation of the unaffected hemisphere in stroke patients. Neuroreport (2005) 16(14):1551–5. doi:10.1097/01.wnr.0000177010.44602.5e
64. Hummel F, Cohen LG. Improvement of motor function with noninvasive cortical stimulation in a patient with chronic stroke. Neurorehabil Neural Repair (2005) 19(1):14–9. doi:10.1177/1545968304272698
65. Stagg CJ, Bachtiar V, O’Shea J, Allman C, Bosnell RA, Kischka U, et al. Cortical activation changes underlying stimulation-induced behavioural gains in chronic stroke. Brain (2012) 135(Pt 1):276–84. doi:10.1093/brain/awr313
66. Lefebvre S, Laloux P, Peeters A, Desfontaines P, Jamart J, Vandermeeren Y. Dual-tDCS enhances online motor skill learning and long-term retention in chronic stroke patients. Front Hum Neurosci (2013) 6:343. doi:10.3389/fnhum.2012.00343
67. Zemke AC, Heagerty PJ, Lee C, Cramer SC. Motor cortex organization after stroke is related to side of stroke and level of recovery. Stroke (2003) 34(5):e23–8. doi:10.1161/01.STR.0000065827.35634.5E
68. Cunningham DA, Varnerin N, Machado A, Bonnett C, Janini D, Roelle S, et al. Stimulation targeting higher motor areas in stroke rehabilitation: a proof-of-concept, randomized, double-blinded placebo-controlled study of effectiveness and underlying mechanisms. Restor Neurol Neurosci (2015) 33(6):911–26. doi:10.3233/RNN-150574
69. Saulle MF, Schambra HM. Recovery and rehabilitation after intracerebral hemorrhage. Semin Neurol (2016) 36(3):306–12. doi:10.1055/s-0036-1581995
70. Kong KH, Lee J. Temporal recovery of activities of daily living in the first year after ischemic stroke: a prospective study of patients admitted to a rehabilitation unit. NeuroRehabilitation (2014) 35(2):221–6. doi:10.3233/NRE-141110
71. Kelly PJ, Furie KL, Shafqat S, Rallis N, Chang Y, Stein J. Functional recovery following rehabilitation after hemorrhagic and ischemic stroke. Arch Phys Med Rehabil (2003) 84(7):968–72. doi:10.1016/S0003-9993(03)00040-6
72. Prabhakaran S, Zarahn E, Riley C, Speizer A, Chong JY, Lazar RM, et al. Inter-individual variability in the capacity for motor recovery after ischemic stroke. Neurorehabil Neural Repair (2008) 22(1):64–71. doi:10.1177/1545968307305302
73. Kwakkel G, Veerbeek JM, van Wegen EE, Nijland R, Harmeling-van der Wel BC, Dippel DW, et al. Predictive value of the NIHSS for ADL outcome after ischemic hemispheric stroke: does timing of early assessment matter? J Neurol Sci (2010) 294(1–2):57–61. doi:10.1016/j.jns.2010.04.004
74. Nijland RH, van Wegen EE, Harmeling-van der Wel BC, Kwakkel G, EPOS Investigators. Presence of finger extension and shoulder abduction within 72 hours after stroke predicts functional recovery: early prediction of functional outcome after stroke: the EPOS cohort study. Stroke (2010) 41(4):745–50. doi:10.1161/STROKEAHA.109.572065
75. Kwakkel G, Veerbeek JM, Harmeling-van der Wel BC, van Wegen E, Kollen BJ, Early Prediction of functional Outcome after Stroke (EPOS) Investigators. Diagnostic accuracy of the Barthel Index for measuring activities of daily living outcome after ischemic hemispheric stroke: does early poststroke timing of assessment matter? Stroke (2011) 42(2):342–6. doi:10.1161/STROKEAHA.110.599035
76. Feng W, Wang J, Chhatbar PY, Doughty C, Landsittel D, Lioutas VA, et al. Corticospinal tract lesion load: an imaging biomarker for stroke motor outcomes. Ann Neurol (2015) 78(6):860–70. doi:10.1002/ana.24510
77. Lefebvre S, Dricot L, Laloux P, Gradkowski W, Desfontaines P, Evrard F, et al. Neural substrates underlying stimulation-enhanced motor skill learning after stroke. Brain (2015) 138(Pt 1):149–63. doi:10.1093/brain/awu336
78. Roe JM, Nesheim M, Mathiesen NC, Moberget T, Alnaes D, Sneve MH. The effects of tDCS upon sustained visual attention are dependent on cognitive load. Neuropsychologia (2016) 80:1–8. doi:10.1016/j.neuropsychologia.2015.11.005
79. Learmonth G, Thut G, Benwell CS, Harvey M. The implications of state-dependent tDCS effects in aging: behavioural response is determined by baseline performance. Neuropsychologia (2015) 74:108–19. doi:10.1016/j.neuropsychologia.2015.01.037
80. Dutta A. Bidirectional interactions between neuronal and hemodynamic responses to transcranial direct current stimulation (tDCS): challenges for brain-state dependent tDCS. Front Syst Neurosci (2015) 9:107. doi:10.3389/fnsys.2015.00107
81. Krause B, Cohen Kadosh R. Not all brains are created equal: the relevance of individual differences in responsiveness to transcranial electrical stimulation. Front Syst Neurosci (2014) 8:25. doi:10.3389/fnsys.2014.00025
82. Schambra HM, Ogden RT, Martinez-Hernandez IE, Lin X, Chang YB, Rahman A, et al. The reliability of repeated TMS measures in older adults and in patients with subacute and chronic stroke. Front Cell Neurosci (2015) 9:335. doi:10.3389/fncel.2015.00335
83. Sosnoff JJ, Newell KM. Aging and motor variability: a test of the neural noise hypothesis. Exp Aging Res (2011) 37(4):377–97. doi:10.1080/0361073X.2011.590754
84. Hasson CJ, Gelina O, Woo G. Neural control adaptation to motor noise manipulation. Front Hum Neurosci (2016) 10:59. doi:10.3389/fnhum.2016.00059
85. Stagg CJ, Jayaram G, Pastor D, Kincses ZT, Matthews PM, Johansen-Berg H. Polarity and timing-dependent effects of transcranial direct current stimulation in explicit motor learning. Neuropsychologia (2011) 49(5):800–4. doi:10.1016/j.neuropsychologia.2011.02.009
86. Cabral ME, Baltar A, Borba R, Galvao S, Santos L, Fregni F, et al. Transcranial direct current stimulation: before, during, or after motor training? Neuroreport (2015) 26(11):618–22. doi:10.1097/WNR.0000000000000397
87. Tecchio F, Zappasodi F, Assenza G, Tombini M, Vollaro S, Barbati G, et al. Anodal transcranial direct current stimulation enhances procedural consolidation. J Neurophysiol (2010) 104(2):1134–40. doi:10.1152/jn.00661.2009
88. Rumpf JJ, Wegscheider M, Hinselmann K, Fricke C, King BR, Weise D, et al. Enhancement of motor consolidation by post-training transcranial direct current stimulation in older people. Neurobiol Aging (2016) 49:1–8. doi:10.1016/j.neurobiolaging.2016.09.003
89. Lefebvre S, Thonnard JL, Laloux P, Peeters A, Jamart J, Vandermeeren Y. Single session of dual-tDCS transiently improves precision grip and dexterity of the paretic hand after stroke. Neurorehabil Neural Repair (2014) 28(2):100–10. doi:10.1177/1545968313478485
90. Zimerman M, Heise KF, Hoppe J, Cohen LG, Gerloff C, Hummel FC. Modulation of training by single-session transcranial direct current stimulation to the intact motor cortex enhances motor skill acquisition of the paretic hand. Stroke (2012) 43(8):2185–91. doi:10.1161/STROKEAHA.111.645382
91. Hummel FC, Voller B, Celnik P, Floel A, Giraux P, Gerloff C, et al. Effects of brain polarization on reaction times and pinch force in chronic stroke. BMC Neurosci (2006) 7:73. doi:10.1186/1471-2202-7-73
92. Mahmoudi H, Borhani Haghighi A, Petramfar P, Jahanshahi S, Salehi Z, Fregni F. Transcranial direct current stimulation: electrode montage in stroke. Disabil Rehabil (2011) 33(15–16):1383–8. doi:10.3109/09638288.2010.532283
93. Hesse S, Werner C, Schonhardt EM, Bardeleben A, Jenrich W, Kirker SG. Combined transcranial direct current stimulation and robot-assisted arm training in subacute stroke patients: a pilot study. Restor Neurol Neurosci (2007) 25(1):9–15.
94. Boggio PS, Nunes A, Rigonatti SP, Nitsche MA, Pascual-Leone A, Fregni F. Repeated sessions of noninvasive brain DC stimulation is associated with motor function improvement in stroke patients. Restor Neurol Neurosci (2007) 25(2):123–9.
95. Ochi M, Saeki S, Oda T, Matsushima Y, Hachisuka K. Effects of anodal and cathodal transcranial direct current stimulation combined with robotic therapy on severely affected arms in chronic stroke patients. J Rehabil Med (2013) 45(2):137–40. doi:10.2340/16501977-1099
96. Ang KK, Guan C, Phua KS, Wang C, Zhao L, Teo WP, et al. Facilitating effects of transcranial direct current stimulation on motor imagery brain-computer interface with robotic feedback for stroke rehabilitation. Arch Phys Med Rehabil (2015) 96(3 Suppl):S79–87. doi:10.1016/j.apmr.2014.08.008
97. Cha HK, Ji SG, Kim MK, Chang JS. Effect of transcranial direct current stimulation of function in patients with stroke. J Phys Ther Sci (2014) 26(3):363–5. doi:10.1589/jpts.26.363
98. Khedr EM, Shawky OA, El-Hammady DH, Rothwell JC, Darwish ES, Mostafa OM, et al. Effect of anodal versus cathodal transcranial direct current stimulation on stroke rehabilitation: a pilot randomized controlled trial. Neurorehabil Neural Repair (2013) 27(7):592–601. doi:10.1177/1545968313484808
99. Lee SJ, Chun MH. Combination transcranial direct current stimulation and virtual reality therapy for upper extremity training in patients with subacute stroke. Arch Phys Med Rehabil (2014) 95(3):431–8. doi:10.1016/j.apmr.2013.10.027
100. Nair DG, Renga V, Lindenberg R, Zhu L, Schlaug G. Optimizing recovery potential through simultaneous occupational therapy and non-invasive brain-stimulation using tDCS. Restor Neurol Neurosci (2011) 29(6):411–20. doi:10.3233/RNN-2011-0612
101. Bradnam LV, Stinear CM, Barber PA, Byblow WD. Contralesional hemisphere control of the proximal paretic upper limb following stroke. Cereb Cortex (2012) 22(11):2662–71. doi:10.1093/cercor/bhr344
102. Yao J, Drogos J, Veltink F, Anderson C, Concha Urday Zaa J, Hanson LI, et al. The effect of transcranial direct current stimulation on the expression of the flexor synergy in the paretic arm in chronic stroke is dependent on shoulder abduction loading. Front Hum Neurosci (2015) 9:262. doi:10.3389/fnhum.2015.00262
103. Goh HT, Chan HY, Abdul-Latif L. Aftereffects of 2 noninvasive brain stimulation techniques on corticospinal excitability in persons with chronic stroke: a pilot study. J Neurol Phys Ther (2015) 39(1):15–22. doi:10.1097/NPT.0000000000000064
104. Fusco A, Iosa M, Venturiero V, De Angelis D, Morone G, Maglione L, et al. After vs. priming effects of anodal transcranial direct current stimulation on upper extremity motor recovery in patients with subacute stroke. Restor Neurol Neurosci (2014) 32(2):301–12. doi:10.3233/RNN-130349
105. Kim DY, Lim JY, Kang EK, You DS, Oh MK, Oh BM, et al. Effect of transcranial direct current stimulation on motor recovery in patients with subacute stroke. Am J Phys Med Rehabil (2010) 89(11):879–86. doi:10.1097/PHM.0b013e3181f70aa7
106. Hesse S, Waldner A, Mehrholz J, Tomelleri C, Pohl M, Werner C. Combined transcranial direct current stimulation and robot-assisted arm training in subacute stroke patients: an exploratory, randomized multicenter trial. Neurorehabil Neural Repair (2011) 25(9):838–46. doi:10.1177/1545968311413906
107. Rossi C, Sallustio F, Di Legge S, Stanzione P, Koch G. Transcranial direct current stimulation of the affected hemisphere does not accelerate recovery of acute stroke patients. Eur J Neurol (2013) 20(1):202–4. doi:10.1111/j.1468-1331.2012.03703.x
108. Viana RT, Laurentino GE, Souza RJ, Fonseca JB, Silva Filho EM, Dias SN, et al. Effects of the addition of transcranial direct current stimulation to virtual reality therapy after stroke: a pilot randomized controlled trial. NeuroRehabilitation (2014) 34(3):437–46. doi:10.3233/NRE-141065
109. Saucedo Marquez CM, Zhang X, Swinnen SP, Meesen R, Wenderoth N. Task-specific effect of transcranial direct current stimulation on motor learning. Front Hum Neurosci (2013) 7:333. doi:10.3389/fnhum.2013.00333
110. Pazzaglia M, Galli G. Translating novel findings of perceptual-motor codes into the neuro-rehabilitation of movement disorders. Front Behav Neurosci (2015) 9:222. doi:10.3389/fnbeh.2015.00222
111. Garrison KA, Aziz-Zadeh L, Wong SW, Liew SL, Winstein CJ. Modulating the motor system by action observation after stroke. Stroke (2013) 44(8):2247–53. doi:10.1161/STROKEAHA.113.001105
112. Dickstein R, Deutsch JE. Motor imagery in physical therapist practice. Phys Ther (2007) 87(7):942–53. doi:10.2522/ptj.20060331
113. Cho HS, Cha HG. Effect of mirror therapy with tDCS on functional recovery of the upper extremity of stroke patients. J Phys Ther Sci (2015) 27(4):1045–7. doi:10.1589/jpts.27.1045
114. Shmuelof L, Krakauer JW, Mazzoni P. How is a motor skill learned? Change and invariance at the levels of task success and trajectory control. J Neurophysiol (2012) 108(2):578–94. doi:10.1152/jn.00856.2011
116. Gomez Palacio Schjetnan A, Faraji J, Metz GA, Tatsuno M, Luczak A. Transcranial direct current stimulation in stroke rehabilitation: a review of recent advancements. Stroke Res Treat (2013) 2013:170256. doi:10.1155/2013/170256
117. Di Lazzaro V, Ranieri F, Profice P, Pilato F, Mazzone P, Capone F, et al. Transcranial direct current stimulation effects on the excitability of corticospinal axons of the human cerebral cortex. Brain Stimul (2013) 6(4):641–3. doi:10.1016/j.brs.2012.09.006
118. Terzuolo CA, Bullock TH. Measurement of imposed voltage gradient adequate to modulate neuronal firing. Proc Natl Acad Sci U S A (1956) 42(9):687–94. doi:10.1073/pnas.42.9.687
119. Bikson M, Inoue M, Akiyama H, Deans JK, Fox JE, Miyakawa H, et al. Effects of uniform extracellular DC electric fields on excitability in rat hippocampal slices in vitro. J Physiol (2004) 557(Pt 1):175–90. doi:10.1113/jphysiol.2003.055772
120. Islam N, Aftabuddin M, Moriwaki A, Hattori Y, Hori Y. Increase in the calcium level following anodal polarization in the rat brain. Brain Res (1995) 684(2):206–8. doi:10.1016/0006-8993(95)00434-R
121. Li CS, Padoa-Schioppa C, Bizzi E. Neuronal correlates of motor performance and motor learning in the primary motor cortex of monkeys adapting to an external force field. Neuron (2001) 30(2):593–607. doi:10.1016/S0896-6273(01)00301-4
122. Stagg CJ, Best JG, Stephenson MC, O’Shea J, Wylezinska M, Kincses ZT, et al. Polarity-sensitive modulation of cortical neurotransmitters by transcranial stimulation. J Neurosci (2009) 29(16):5202–6. doi:10.1523/JNEUROSCI.4432-08.2009
123. Nitsche MA, Seeber A, Frommann K, Klein CC, Rochford C, Nitsche MS, et al. Modulating parameters of excitability during and after transcranial direct current stimulation of the human motor cortex. J Physiol (2005) 568(Pt 1):291–303. doi:10.1113/jphysiol.2005.092429
124. Malenka RC, Nicoll RA. Long-term potentiation – a decade of progress? Science (1999) 285(5435):1870–4. doi:10.1126/science.285.5435.1870
125. Clarkson AN, Overman JJ, Zhong S, Mueller R, Lynch G, Carmichael ST. AMPA receptor-induced local brain-derived neurotrophic factor signaling mediates motor recovery after stroke. J Neurosci (2011) 31(10):3766–75. doi:10.1523/JNEUROSCI.5780-10.2011
126. Ying SW, Futter M, Rosenblum K, Webber MJ, Hunt SP, Bliss TV, et al. Brain-derived neurotrophic factor induces long-term potentiation in intact adult hippocampus: requirement for ERK activation coupled to CREB and upregulation of Arc synthesis. J Neurosci (2002) 22(5):1532–40.
127. Panja D, Bramham CR. BDNF mechanisms in late LTP formation: a synthesis and breakdown. Neuropharmacology (2014) 76(Pt C):664–76. doi:10.1016/j.neuropharm.2013.06.024
128. Ranieri F, Podda MV, Riccardi E, Frisullo G, Dileone M, Profice P, et al. Modulation of LTP at rat hippocampal CA3-CA1 synapses by direct current stimulation. J Neurophysiol (2012) 107(7):1868–80. doi:10.1152/jn.00319.2011
129. Cunha C, Brambilla R, Thomas KL. A simple role for BDNF in learning and memory? Front Mol Neurosci (2010) 3:1. doi:10.3389/neuro.02.001.2010
130. Sanes JN, Donoghue JP. Plasticity and primary motor cortex. Annu Rev Neurosci (2000) 23:393–415. doi:10.1146/annurev.neuro.23.1.393
131. Rioult-Pedotti MS, Friedman D, Hess G, Donoghue JP. Strengthening of horizontal cortical connections following skill learning. Nat Neurosci (1998) 1(3):230–4. doi:10.1038/678
132. Rioult-Pedotti MS, Friedman D, Donoghue JP. Learning-induced LTP in neocortex. Science (2000) 290(5491):533–6. doi:10.1126/science.290.5491.533
133. Rioult-Pedotti MS, Donoghue JP, Dunaevsky A. Plasticity of the synaptic modification range. J Neurophysiol (2007) 98(6):3688–95. doi:10.1152/jn.00164.2007
134. Shmuelof L, Krakauer JW. Are we ready for a natural history of motor learning? Neuron (2011) 72(3):469–76. doi:10.1016/j.neuron.2011.10.017
135. Kantak SS, Stinear JW, Buch ER, Cohen LG. Rewiring the brain: potential role of the premotor cortex in motor control, learning, and recovery of function following brain injury. Neurorehabil Neural Repair (2012) 26(3):282–92. doi:10.1177/1545968311420845
136. Krakauer JW. Motor learning: its relevance to stroke recovery and neurorehabilitation. Curr Opin Neurol (2006) 19(1):84–90. doi:10.1097/01.wco.0000200544.29915.cc
137. Dipietro L, Krebs HI, Volpe BT, Stein J, Bever C, Mernoff ST, et al. Learning, not adaptation, characterizes stroke motor recovery: evidence from kinematic changes induced by robot-assisted therapy in trained and untrained task in the same workspace. IEEE Trans Neural Syst Rehabil Eng (2012) 20(1):48–57. doi:10.1109/TNSRE.2011.2175008
138. Matthews PM, Johansen-Berg H, Reddy H. Non-invasive mapping of brain functions and brain recovery: applying lessons from cognitive neuroscience to neurorehabilitation. Restor Neurol Neurosci (2004) 22(3–5):245–60.
139. Cheeran B, Talelli P, Mori F, Koch G, Suppa A, Edwards M, et al. A common polymorphism in the brain-derived neurotrophic factor gene (BDNF) modulates human cortical plasticity and the response to rTMS. J Physiol (2008) 586(Pt 23):5717–25. doi:10.1113/jphysiol.2008.159905
140. Li Voti P, Conte A, Suppa A, Iezzi E, Bologna M, Aniello MS, et al. Correlation between cortical plasticity, motor learning and BDNF genotype in healthy subjects. Exp Brain Res (2011) 212(1):91–9. doi:10.1007/s00221-011-2700-5
141. Lang CE, Macdonald JR, Reisman DS, Boyd L, Jacobson Kimberley T, Schindler-Ivens SM, et al. Observation of amounts of movement practice provided during stroke rehabilitation. Arch Phys Med Rehabil (2009) 90(10):1692–8. doi:10.1016/j.apmr.2009.04.005
142. Kimberley TJ, Samargia S, Moore LG, Shakya JK, Lang CE. Comparison of amounts and types of practice during rehabilitation for traumatic brain injury and stroke. J Rehabil Res Dev (2010) 47(9):851–62. doi:10.1682/JRRD.2010.02.0019
143. Datta A, Baker JM, Bikson M, Fridriksson J. Individualized model predicts brain current flow during transcranial direct-current stimulation treatment in responsive stroke patient. Brain Stimul (2011) 4(3):169–74. doi:10.1016/j.brs.2010.11.001
144. Lotze M, Markert J, Sauseng P, Hoppe J, Plewnia C, Gerloff C. The role of multiple contralesional motor areas for complex hand movements after internal capsular lesion. J Neurosci (2006) 26(22):6096–102. doi:10.1523/JNEUROSCI.4564-05.2006
145. Plow EB, Cunningham DA, Varnerin N, Machado A. Rethinking stimulation of the brain in stroke rehabilitation: why higher motor areas might be better alternatives for patients with greater impairments. Neuroscientist (2015) 21(3):225–40. doi:10.1177/1073858414537381
146. Cheney PD. Role of cerebral cortex in voluntary movements. A review. Phys Ther (1985) 65(5):624–35.
147. Evarts EV. Role of motor cortex in voluntary movements in primates. Compr Physiol (2011) 2:1083–120. doi:10.1002/cphy.cp010223
148. Hlustik P, Solodkin A, Gullapalli RP, Noll DC, Small SL. Somatotopy in human primary motor and somatosensory hand representations revisited. Cereb Cortex (2001) 11(4):312–21. doi:10.1093/cercor/11.4.312
149. Bloom JS, Hynd GW. The role of the corpus callosum in interhemispheric transfer of information: excitation or inhibition? Neuropsychol Rev (2005) 15(2):59–71. doi:10.1007/s11065-005-6252-y
150. Duque J, Hummel F, Celnik P, Murase N, Mazzocchio R, Cohen LG. Transcallosal inhibition in chronic subcortical stroke. Neuroimage (2005) 28(4):940–6. doi:10.1016/j.neuroimage.2005.06.033
151. Ward NS, Cohen LG. Mechanisms underlying recovery of motor function after stroke. Arch Neurol (2004) 61(12):1844–8. doi:10.1001/archneur.61.12.1844
152. DaSilva AF, Volz MS, Bikson M, Fregni F. Electrode positioning and montage in transcranial direct current stimulation. J Vis Exp (2011) (51). doi:10.3791/2744
153. Borckardt JJ, Reeves ST, Robinson SM, May JT, Epperson TI, Gunselman RJ, et al. Transcranial direct current stimulation (tDCS) reduces postsurgical opioid consumption in total knee arthroplasty (TKA). Clin J Pain (2013) 29(11):925–8. doi:10.1097/AJP.0b013e31827e32be
154. Ladeira A, Fregni F, Campanha C, Valasek CA, De Ridder D, Brunoni AR, et al. Polarity-dependent transcranial direct current stimulation effects on central auditory processing. PLoS One (2011) 6(9):e25399. doi:10.1371/journal.pone.0025399
155. Garin P, Gilain C, Van Damme JP, de Fays K, Jamart J, Ossemann M, et al. Short- and long-lasting tinnitus relief induced by transcranial direct current stimulation. J Neurol (2011) 258(11):1940–8. doi:10.1007/s00415-011-6037-6
156. Dubois PE, Ossemann M, de Fays K, De Bue P, Gourdin M, Jamart J, et al. Postoperative analgesic effect of transcranial direct current stimulation in lumbar spine surgery: a randomized control trial. Clin J Pain (2013) 29(8):696–701. doi:10.1097/AJP.0b013e31826fb302
157. Teo F, Hoy KE, Daskalakis ZJ, Fitzgerald PB. Investigating the role of current strength in tDCS modulation of working memory performance in healthy controls. Front Psychiatry (2011) 2:45. doi:10.3389/fpsyt.2011.00045
158. Herwig U, Padberg F, Unger J, Spitzer M, Schonfeldt-Lecuona C. Transcranial magnetic stimulation in therapy studies: examination of the reliability of “standard” coil positioning by neuronavigation. Biol Psychiatry (2001) 50(1):58–61. doi:10.1016/S0006-3223(01)01153-2
159. Lee DG, Lee DY. Effects of adjustment of transcranial direct current stimulation on motor function of the upper extremity in stroke patients. J Phys Ther Sci (2015) 27(11):3511–3. doi:10.1589/jpts.27.3511
160. di Pellegrino G, Wise SP. A neurophysiological comparison of three distinct regions of the primate frontal lobe. Brain (1991) 114(Pt 2):951–78. doi:10.1093/brain/114.2.951
161. Schluter ND, Rushworth MF, Passingham RE, Mills KR. Temporary interference in human lateral premotor cortex suggests dominance for the selection of movements. A study using transcranial magnetic stimulation. Brain (1998) 121(Pt 5):785–99.
162. Schluter ND, Rushworth MF, Mills KR, Passingham RE. Signal-, set-, and movement-related activity in the human premotor cortex. Neuropsychologia (1999) 37(2):233–43. doi:10.1016/S0028-3932(98)00098-0
163. Grafton ST, Fagg AH, Arbib MA. Dorsal premotor cortex and conditional movement selection: a PET functional mapping study. J Neurophysiol (1998) 79(2):1092–7.
164. Jaillard A, Martin CD, Garambois K, Lebas JF, Hommel M. Vicarious function within the human primary motor cortex? A longitudinal fMRI stroke study. Brain (2005) 128(Pt 5):1122–38. doi:10.1093/brain/awh456
165. Carey LM, Abbott DF, Egan GF, Bernhardt J, Donnan GA. Motor impairment and recovery in the upper limb after stroke: behavioral and neuroanatomical correlates. Stroke (2005) 36(3):625–9. doi:10.1161/01.STR.0000155720.47711.83
166. Johansen-Berg H, Rushworth MF, Bogdanovic MD, Kischka U, Wimalaratna S, Matthews PM. The role of ipsilateral premotor cortex in hand movement after stroke. Proc Natl Acad Sci U S A (2002) 99(22):14518–23. doi:10.1073/pnas.222536799
167. Ward NS, Brown MM, Thompson AJ, Frackowiak RS. Neural correlates of motor recovery after stroke: a longitudinal fMRI study. Brain (2003) 126(Pt 11):2476–96. doi:10.1093/brain/awg145
168. O’Shea J, Johansen-Berg H, Trief D, Gobel S, Rushworth MF. Functionally specific reorganization in human premotor cortex. Neuron (2007) 54(3):479–90. doi:10.1016/j.neuron.2007.04.021
169. Fridman EA, Hanakawa T, Chung M, Hummel F, Leiguarda RC, Cohen LG. Reorganization of the human ipsilesional premotor cortex after stroke. Brain (2004) 127(Pt 4):747–58. doi:10.1093/brain/awh082
170. Lefebvre S, Dricot L, Laloux P, Gradkowski W, Desfontaines P, Evrard F, et al. Neural substrates underlying motor skill learning in chronic hemiparetic stroke patients. Front Hum Neurosci (2015) 9:320. doi:10.3389/fnhum.2015.00320
171. Yozbatiran N, Alonso-Alonso M, See J, Demirtas-Tatlidede A, Luu D, Motiwala RR, et al. Safety and behavioral effects of high-frequency repetitive transcranial magnetic stimulation in stroke. Stroke (2009) 40(1):309–12. doi:10.1161/STROKEAHA.108.522144
172. Ludemann-Podubecka J, Bosl K, Nowak DA. Inhibition of the contralesional dorsal premotor cortex improves motor function of the affected hand following stroke. Eur J Neurol (2016) 23(4):823–30. doi:10.1111/ene.12949
173. Bikson M, Rahman A, Datta A. Computational models of transcranial direct current stimulation. Clin EEG Neurosci (2012) 43(3):176–83. doi:10.1177/1550059412445138
174. Lee D. Behavioral context and coherent oscillations in the supplementary motor area. J Neurosci (2004) 24(18):4453–9. doi:10.1523/JNEUROSCI.0047-04.2004
175. Lewis PA, Wing AM, Pope PA, Praamstra P, Miall RC. Brain activity correlates differentially with increasing temporal complexity of rhythms during initialisation, synchronisation, and continuation phases of paced finger tapping. Neuropsychologia (2004) 42(10):1301–12. doi:10.1016/j.neuropsychologia.2004.03.001
176. VanMier JSP, SE P. Functional changes in brain activity during acquisition and practice of movement sequences. Motor Control (2004) 8(4):500–20. doi:10.1123/mcj.8.4.500
177. Loubinoux I, Carel C, Pariente J, Dechaumont S, Albucher JF, Marque P, et al. Correlation between cerebral reorganization and motor recovery after subcortical infarcts. Neuroimage (2003) 20(4):2166–80. doi:10.1016/j.neuroimage.2003.08.017
178. Feydy A, Carlier R, Roby-Brami A, Bussel B, Cazalis F, Pierot L, et al. Longitudinal study of motor recovery after stroke: recruitment and focusing of brain activation. Stroke (2002) 33(6):1610–7. doi:10.1161/01.STR.0000017100.68294.52
179. Carey LM, Abbott DF, Egan GF, O’Keefe GJ, Jackson GD, Bernhardt J, et al. Evolution of brain activation with good and poor motor recovery after stroke. Neurorehabil Neural Repair (2006) 20(1):24–41. doi:10.1177/1545968305283053
180. Karni A, Meyer G, Jezzard P, Adams MM, Turner R, Ungerleider LG. Functional MRI evidence for adult motor cortex plasticity during motor skill learning. Nature (1995) 377(6545):155–8. doi:10.1038/377155a0
181. Kim YK, Shin SH. Comparison of effects of transcranial magnetic stimulation on primary motor cortex and supplementary motor area in motor skill learning (randomized, cross over study). Front Hum Neurosci (2014) 8:937. doi:10.3389/fnhum.2014.00937
182. Carlsen AN, Eagles JS, MacKinnon CD. Transcranial direct current stimulation over the supplementary motor area modulates the preparatory activation level in the human motor system. Behav Brain Res (2015) 279:68–75. doi:10.1016/j.bbr.2014.11.009
183. Vollmann H, Conde V, Sewerin S, Taubert M, Sehm B, Witte OW, et al. Anodal transcranial direct current stimulation (tDCS) over supplementary motor area (SMA) but not pre-SMA promotes short-term visuomotor learning. Brain Stimul (2013) 6(2):101–7. doi:10.1016/j.brs.2012.03.018
184. Lefebvre S, Dricot L, Gradkowski W, Laloux P, Vandermeeren Y. Brain activations underlying different patterns of performance improvement during early motor skill learning. Neuroimage (2012) 62(1):290–9. doi:10.1016/j.neuroimage.2012.04.052
185. Denny-Brown D. Disintegration of motor function resulting from cerebral lesions. J Nerv Ment Dis (1950) 112(1):1–45. doi:10.1097/00005053-195007000-00001
186. Krakauer JW. Functional imaging of motor recovery after stroke: remaining challenges. Curr Neurol Neurosci Rep (2004) 4(1):42–6. doi:10.1007/s11910-004-0010-z
187. Purves D, Augustine GJ, Fitzpatrick D, Katz LC, LaMantia A-S, McNamara JO, et al. Consequences of cerebellar lesions. 2nd ed. In: Associates SMS, editor. Neurosciences. (2001).
188. Bernard JA, Seidler RD. Cerebellar contributions to visuomotor adaptation and motor sequence learning: an ALE meta-analysis. Front Hum Neurosci (2013) 7:27. doi:10.3389/fnhum.2013.00027
189. Weiller C, Chollet F, Friston KJ, Wise RJ, Frackowiak RS. Functional reorganization of the brain in recovery from striatocapsular infarction in man. Ann Neurol (1992) 31(5):463–72. doi:10.1002/ana.410310502
190. Azari NP, Binkofski F, Pettigrew KD, Freund HJ, Seitz RJ. Enhanced regional cerebral metabolic interactions in thalamic circuitry predicts motor recovery in hemiparetic stroke. Hum Brain Mapp (1996) 4(4):240–53. doi:10.1002/(SICI)1097-0193(1996)4:4
191. Small SL, Hlustik P, Noll DC, Genovese C, Solodkin A. Cerebellar hemispheric activation ipsilateral to the paretic hand correlates with functional recovery after stroke. Brain (2002) 125(Pt 7):1544–57. doi:10.1093/brain/awf148
192. Galea JM, Jayaram G, Ajagbe L, Celnik P. Modulation of cerebellar excitability by polarity-specific noninvasive direct current stimulation. J Neurosci (2009) 29(28):9115–22. doi:10.1523/JNEUROSCI.2184-09.2009
193. Galea JM, Vazquez A, Pasricha N, de Xivry JJ, Celnik P. Dissociating the roles of the cerebellum and motor cortex during adaptive learning: the motor cortex retains what the cerebellum learns. Cereb Cortex (2011) 21(8):1761–70. doi:10.1093/cercor/bhq246
194. Grimaldi G, Argyropoulos GP, Bastian A, Cortes M, Davis NJ, Edwards DJ, et al. Cerebellar transcranial direct current stimulation (ctDCS): a novel approach to understanding cerebellar function in health and disease. Neuroscientist (2016) 22(1):83–97. doi:10.1177/1073858414559409
195. Pope PA, Miall RC. Restoring cognitive functions using non-invasive brain stimulation techniques in patients with cerebellar disorders. Front Psychiatry (2014) 5:33. doi:10.3389/fpsyt.2014.00033
196. Sathian K, Buxbaum LJ, Cohen LG, Krakauer JW, Lang CE, Corbetta M, et al. Neurological principles and rehabilitation of action disorders: common clinical deficits. Neurorehabil Neural Repair (2011) 25(5 Suppl):21S–32S. doi:10.1177/1545968311410941
197. Gross RG, Grossman M. Update on apraxia. Curr Neurol Neurosci Rep (2008) 8(6):490–6. doi:10.1007/s11910-008-0078-y
198. Niessen E, Fink GR, Weiss PH. Apraxia, pantomime and the parietal cortex. Neuroimage Clin (2014) 5:42–52. doi:10.1016/j.nicl.2014.05.017
199. Inoue K, Kawashima R, Satoh K, Kinomura S, Goto R, Sugiura M, et al. Activity in the parietal area during visuomotor learning with optical rotation. Neuroreport (1997) 8(18):3979–83. doi:10.1097/00001756-199712220-00026
200. Sparing R, Thimm M, Hesse MD, Kust J, Karbe H, Fink GR. Bidirectional alterations of interhemispheric parietal balance by non-invasive cortical stimulation. Brain (2009) 132(Pt 11):3011–20. doi:10.1093/brain/awp154
201. Tanji J, Hoshi E. Behavioral planning in the prefrontal cortex. Curr Opin Neurobiol (2001) 11(2):164–70. doi:10.1016/S0959-4388(00)00192-6
202. Meehan SK, Randhawa B, Wessel B, Boyd LA. Implicit sequence-specific motor learning after subcortical stroke is associated with increased prefrontal brain activations: an fMRI study. Hum Brain Mapp (2011) 32(2):290–303. doi:10.1002/hbm.21019
203. Seidler RD, Bo J, Anguera JA. Neurocognitive contributions to motor skill learning: the role of working memory. J Mot Behav (2012) 44(6):445–53. doi:10.1080/00222895.2012.672348
204. Liu H, Song L, Zhang T. Changes in brain activation in stroke patients after mental practice and physical exercise: a functional MRI study. Neural Regen Res (2014) 9(15):1474–84. doi:10.4103/1673-5374.139465
205. Seitz RJ, Kleiser R, Butefisch CM. Reorganization of cerebral circuits in human brain lesion. Acta Neurochir Suppl (2005) 93:65–70. doi:10.1007/3-211-27577-0_9
206. Fujimoto S, Kon N, Otaka Y, Yamaguchi T, Nakayama T, Kondo K, et al. Transcranial direct current stimulation over the primary and secondary somatosensory cortices transiently improves tactile spatial discrimination in stroke patients. Front Neurosci (2016) 10:128. doi:10.3389/fnins.2016.00128
207. Ragert P, Vandermeeren Y, Camus M, Cohen LG. Improvement of spatial tactile acuity by transcranial direct current stimulation. Clin Neurophysiol (2008) 119(4):805–11. doi:10.1016/j.clinph.2007.12.001
208. Labbe S, Meftah el M, Chapman CE. Effects of transcranial direct current stimulation of primary somatosensory cortex on vibrotactile detection and discrimination. J Neurophysiol (2016) 115(4):1978–87. doi:10.1152/jn.00506.2015
209. Brodie SM, Meehan S, Borich MR, Boyd LA. 5 Hz repetitive transcranial magnetic stimulation over the ipsilesional sensory cortex enhances motor learning after stroke. Front Hum Neurosci (2014) 8:143. doi:10.3389/fnhum.2014.00143
210. Meehan SK, Dao E, Linsdell MA, Boyd LA. Continuous theta burst stimulation over the contralesional sensory and motor cortex enhances motor learning post-stroke. Neurosci Lett (2011) 500(1):26–30. doi:10.1016/j.neulet.2011.05.237
211. Nitsche MA, Doemkes S, Karakose T, Antal A, Liebetanz D, Lang N, et al. Shaping the effects of transcranial direct current stimulation of the human motor cortex. J Neurophysiol (2007) 97(4):3109–17. doi:10.1152/jn.01312.2006
212. Takeuchi N, Chuma T, Matsuo Y, Watanabe I, Ikoma K. Repetitive transcranial magnetic stimulation of contralesional primary motor cortex improves hand function after stroke. Stroke (2005) 36(12):2681–6. doi:10.1161/01.STR.0000189658.51972.34
213. Tazoe T, Endoh T, Kitamura T, Ogata T. Polarity specific effects of transcranial direct current stimulation on interhemispheric inhibition. PLoS One (2014) 9(12):e114244. doi:10.1371/journal.pone.0114244
214. Kidgell DJ, Goodwill AM, Frazer AK, Daly RM. Induction of cortical plasticity and improved motor performance following unilateral and bilateral transcranial direct current stimulation of the primary motor cortex. BMC Neurosci (2013) 14(1):64. doi:10.1186/1471-2202-14-64
215. Vines BW, Cerruti C, Schlaug G. Dual-hemisphere tDCS facilitates greater improvements for healthy subjects’ non-dominant hand compared to uni-hemisphere stimulation. BMC Neurosci (2008) 9:103. doi:10.1186/1471-2202-9-103
216. Sehm B, Kipping J, Schafer A, Villringer A, Ragert PA. Comparison between uni- and bilateral tDCS effects on functional connectivity of the human motor cortex. Front Hum Neurosci (2013) 7:183. doi:10.3389/fnhum.2013.00183
217. Lindenberg R, Nachtigall L, Meinzer M, Sieg MM, Floel A. Differential effects of dual and unihemispheric motor cortex stimulation in older adults. J Neurosci (2013) 33(21):9176–83. doi:10.1523/JNEUROSCI.0055-13.2013
218. Bradnam LV, Stinear CM, Byblow WD. Ipsilateral motor pathways after stroke: implications for non-invasive brain stimulation. Front Hum Neurosci (2013) 7:184. doi:10.3389/fnhum.2013.00184
219. Redfearn JW, Lippold OC, Costain R. A preliminary account of the clinical effects of polarizing the brain in certain psychiatric disorders. Br J Psychiatry (1964) 110:773–85. doi:10.1192/bjp.110.469.773
220. Lippold OC, Redfearn JW. Mental changes resulting from the passage of small direct currents through the human brain. Br J Psychiatry (1964) 110:768–72. doi:10.1192/bjp.110.469.768
221. Vandermeeren Y, Jamart J, Ossemann M. Effect of tDCS with an extracephalic reference electrode on cardio-respiratory and autonomic functions. BMC Neurosci (2010) 11:38. doi:10.1186/1471-2202-11-38
222. Im CH, Park JH, Shim M, Chang WH, Kim YH. Evaluation of local electric fields generated by transcranial direct current stimulation with an extracephalic reference electrode based on realistic 3D body modeling. Phys Med Biol (2012) 57(8):2137–50. doi:10.1088/0031-9155/57/8/2137
223. Schambra HM, Abe M, Luckenbaugh DA, Reis J, Krakauer JW, Cohen LG. Probing for hemispheric specialization for motor skill learning: a transcranial direct current stimulation study. J Neurophysiol (2011) 106(2):652–61. doi:10.1152/jn.00210.2011
224. Bolognini N, Ro T. Transcranial magnetic stimulation: disrupting neural activity to alter and assess brain function. J Neurosci (2010) 30(29):9647–50. doi:10.1523/JNEUROSCI.1990-10.2010
225. Richardson J, Datta A, Dmochowski J, Parra LC, Fridriksson J. Feasibility of using high-definition transcranial direct current stimulation (HD-tDCS) to enhance treatment outcomes in persons with aphasia. NeuroRehabilitation (2015) 36(1):115–26. doi:10.3233/NRE-141199
226. Antal A, Polania R, Schmidt-Samoa C, Dechent P, Paulus W. Transcranial direct current stimulation over the primary motor cortex during fMRI. Neuroimage (2011) 55(2):590–6. doi:10.1016/j.neuroimage.2010.11.085
227. Stagg CJ, Lin RL, Mezue M, Segerdahl A, Kong Y, Xie J, et al. Widespread modulation of cerebral perfusion induced during and after transcranial direct current stimulation applied to the left dorsolateral prefrontal cortex. J Neurosci (2013) 33(28):11425–31. doi:10.1523/JNEUROSCI.3887-12.2013
228. Lefebvre S, Dricot L, Laloux P, Desfontaines P, Evrard F, Peeters A, et al. Increased functional connectivity one week after motor learning and tDCS in stroke patients. Neuroscience (2017) 340:424–35. doi:10.1016/j.neuroscience.2016.10.066
229. Wagner S, Rampersad SM, Aydin U, Vorwerk J, Oostendorp TF, Neuling T, et al. Investigation of tDCS volume conduction effects in a highly realistic head model. J Neural Eng (2014) 11(1):016002. doi:10.1088/1741-2560/11/1/016002
230. Faria P, Hallett M, Miranda PC. A finite element analysis of the effect of electrode area and inter-electrode distance on the spatial distribution of the current density in tDCS. J Neural Eng (2011) 8(6):066017. doi:10.1088/1741-2560/8/6/066017
231. Opitz A, Paulus W, Will S, Antunes A, Thielscher A. Determinants of the electric field during transcranial direct current stimulation. Neuroimage (2015) 109:140–50. doi:10.1016/j.neuroimage.2015.01.033
232. Wagner T, Fregni F, Eden U, Ramos-Estebanez C, Grodzinsky A, Zahn M, et al. Transcranial magnetic stimulation and stroke: a computer-based human model study. Neuroimage (2006) 30(3):857–70. doi:10.1016/j.neuroimage.2005.04.046
233. Suh HS, Lee WH, Kim TS. Influence of anisotropic conductivity in the skull and white matter on transcranial direct current stimulation via an anatomically realistic finite element head model. Phys Med Biol (2012) 57(21):6961–80. doi:10.1088/0031-9155/57/21/6961
234. Shahid SS, Bikson M, Salman H, Wen P, Ahfock T. The value and cost of complexity in predictive modelling: role of tissue anisotropic conductivity and fibre tracts in neuromodulation. J Neural Eng (2014) 11(3):036002. doi:10.1088/1741-2560/11/3/036002
235. Datta A, Truong D, Minhas P, Parra LC, Bikson M. Inter-Individual variation during transcranial direct current stimulation and normalization of dose using MRI-derived computational models. Front Psychiatry (2012) 3:91. doi:10.3389/fpsyt.2012.00091
236. Wagner T, Fregni F, Fecteau S, Grodzinsky A, Zahn M, Pascual-Leone A. Transcranial direct current stimulation: a computer-based human model study. Neuroimage (2007) 35(3):1113–24. doi:10.1016/j.neuroimage.2007.01.027
237. Ulm L, McMahon K, Copland D, de Zubicaray GI, Meinzer M. Neural Mechanisms underlying perilesional transcranial direct current stimulation in aphasia: a feasibility study. Front Hum Neurosci (2015) 9:550. doi:10.3389/fnhum.2015.00550
Keywords: tDCS, stroke, motor recovery, electrode placement, optimal stimulation parameters
Citation: Lefebvre S and Liew S-L (2017) Anatomical Parameters of tDCS to Modulate the Motor System after Stroke: A Review. Front. Neurol. 8:29. doi: 10.3389/fneur.2017.00029
Received: 19 November 2016; Accepted: 23 January 2017;
Published: 09 February 2017
Edited by:
Mary Ellen Stoykov, Rush University, USAReviewed by:
Mariella Pazzaglia, Sapienza University of Rome, ItalyHeidi M. Schambra, New York University, USA
Amit Sethi, University of Pittsburgh, USA
Copyright: © 2017 Lefebvre and Liew. This is an open-access article distributed under the terms of the Creative Commons Attribution License (CC BY). The use, distribution or reproduction in other forums is permitted, provided the original author(s) or licensor are credited and that the original publication in this journal is cited, in accordance with accepted academic practice. No use, distribution or reproduction is permitted which does not comply with these terms.
*Correspondence: Sook-Lei Liew, c2xpZXcmI3gwMDA0MDt1c2MuZWR1