- Department of Cell Morphology and Molecular Neurobiology, Ruhr University Bochum, Bochum, Germany
The low-density lipoprotein related protein receptor 1 (LRP1), also known as CD91 or α-Macroglobulin-receptor, is a transmembrane receptor that interacts with more than 40 known ligands. It plays an important biological role as receptor of morphogens, extracellular matrix molecules, cytokines, proteases, protease inhibitors and pathogens. In the CNS, it has primarily been studied as a receptor and clearance agent of pathogenic factors such as Aβ-peptide and, lately, Tau protein that is relevant for tissue homeostasis and protection against neurodegenerative processes. Recently, it was found that LRP1 expresses the Lewis-X (Lex) carbohydrate motif and is expressed in the neural stem cell compartment. The removal of Lrp1 from the cortical radial glia compartment generates a strong phenotype with severe motor deficits, seizures and a reduced life span. The present review discusses approaches that have been taken to address the neurodevelopmental significance of LRP1 by creating novel, lineage-specific constitutive or conditional knockout mouse lines. Deficits in the stem cell compartment may be at the root of severe CNS pathologies.
1 Introduction
1.1 Identification of Lewis-X carrying glycoproteins in radial glia
Radial glia cells represent the principal neural stem and glial progenitor cell (NSPC) compartment during neural development (Malatesta and Gotz, 2013). The development of the CNS is a carefully regulated process where neurogenesis precedes the formation of oligodendrocytes and astrocytes (gliogenesis) (Gotz and Huttner, 2005; Kriegstein and Alvarez-Buylla, 2009; Taverna et al., 2014). Gliogenesis begins around embryonic day (E) 12.5 in the mouse CNS with the specification of oligodendrocyte precursor cells (OPCs) (Bergles and Richardson, 2016). Astrocytes represent an abundant glial population of the nervous system of mammals that emerges at later stages and displays a considerable heterogeneity that has so far not been appreciated (Allen and Barres, 2009; Zhang and Barres, 2010; Clarke and Barres, 2013; Molofsky et al., 2014). Despite their various roles in CNS function and homeostasis their ontogenetic development remains poorly understood (Faissner et al., 2010; Molofsky et al., 2012). Increasing evidence suggests that the extracellular matrix (ECM) microenvironment of the stem cell niche plays an important role for the differentiation of neural stem and glial progenitor cells (Garcion et al., 2004; Faissner and Reinhard, 2015; May et al., 2018; Kjell et al., 2020; Schaberg et al., 2022). In this regard, we have addressed novel glycan markers of the neural stem cell compartment established in our laboratory, in particular LewisX related epitopes (Hennen et al., 2011; Hennen et al., 2013). LewisX (LeX), also known as CD15 or stage specific embryonic antigen-1 (SSEA-1), is a carbohydrate motif associated with glycoproteins and glycolipids. Throughout neurogenesis, LeX is detectable on radial glia that represents the major NSPC population in the developing embryonic CNS (Hennen and Faissner, 2012). Using novel monoclonal antibodies we revealed a considerable microheterogeneity of LeX-related glycans that display differential expression patterns on glial surfaces (Hennen et al., 2011). The LeX-epitopes recognized by the monoclonal antibodies 487LeX and 5750LeX we characterized are shared to some extent by radial glia subpopulations and hiPSC-derived stem cells and partially sensitive to proteolysis (Kandasamy et al., 2017; Roll et al., 2022). In an effort to resolve the apparent heterogeneity of glial surface expression, we characterized LeX-expressing protein cores in the CNS and identified Phosphacan, a secreted splice variant of the protein tyrosine phosphatase receptor-type zeta (Ptprζ1) (Garwood et al., 1999), the ECM glycoprotein tenascin-C (Faissner et al., 2017), the L1-cell adhesion molecule (L1-CAM), and, finally, the lipoprotein receptor-related protein 1 (LRP1) as a novel carrier protein (Hennen et al., 2013). The β1-integrin, lysosomal-associated membrane protein (LAMP-1), CD24 and Thy-1 are further known LeX carriers (Hennen and Faissner, 2012).
1.2 LRP1 is a scavenger expressed by NSPCs and their progeny
LRP1, also known as CD91, is member of the LDL-family of receptors and synthesized as a type 1 transmembrane receptor with 4,525 amino acids that is cleaved by a furin-type protease in the Golgi apparatus (Herz et al., 1988). The cleavage produces an extracellular α-chain of 515 kD and a transmembrane β-chain component of 85 kD which stay both connected by non-covalent interaction (Boucher and Herz, 2011). The structure of LRP1 comprises cysteine-rich egf-type repeats, β-propeller domains, a transmembrane domain and an intracellular domain that contains the NPxY motif (Herz and Strickland, 2001) (Figure 1).
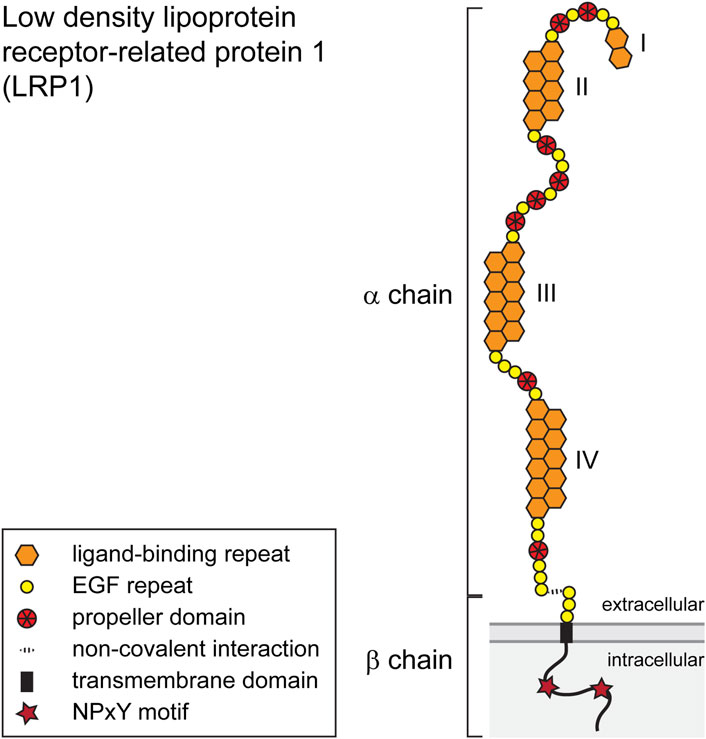
FIGURE 1. Schematic representation of the LRP1 scavenging receptor. The single pass transmembrane receptor protein consists of a 515 kD extracellular α-chain and a transmembrane β-chain of 85 kD which are connected via non-covalent interactions. Both chains are generated from a single-chain precursor protein by a furin-type protease in the Golgi apparatus. The α-chain is characterized by repeat domains including egf-type, egf-precursor-protein type, β-propeller-type repeats and cysteine-rich ligand binding domains I–IV. The intracellular segment of the β-subunit possesses two NPxY motifs and one YxxL motif and two di-leucine motifs. The β-subunit furthermore contains docking sites for PSD-95, Dab-1 and FE-65. For details see text.
LRP1 comprises ligand binding sites for more than 40 binding partners, including ApoE, proteases, protease inhibitors, extracellular matrix components, cytokines, growth factors, bacteria and viruses (Lillis et al., 2008; Boucher and Herz, 2011; Bres and Faissner, 2019b). Its primary function is linked to processes of receptor-mediated endocytosis of ligands, whereupon LRP1 is recycled to the cell membrane surface (Figure 2). A receptor associated protein (RAP) has been discovered that binds to the ligand-binding regions and thereby blocks any receptor-cargo interactions (Bu et al., 1994). The LDL-family of receptors and its member LRP1 have classically been associated with a modulation of lipoprotein metabolism and have been studied for example, with reference to Alzheimer’s disease (Hussain et al., 1999; Jaeger and Pietrzik, 2008; Eggert et al., 2018; Sousa et al., 2023). In addition, diverse functions for this receptor have been uncovered in various aspects of cellular activities, including cell proliferation, migration, differentiation, immune response and survival (Herz and Bock, 2002; Sizova et al., 2023).
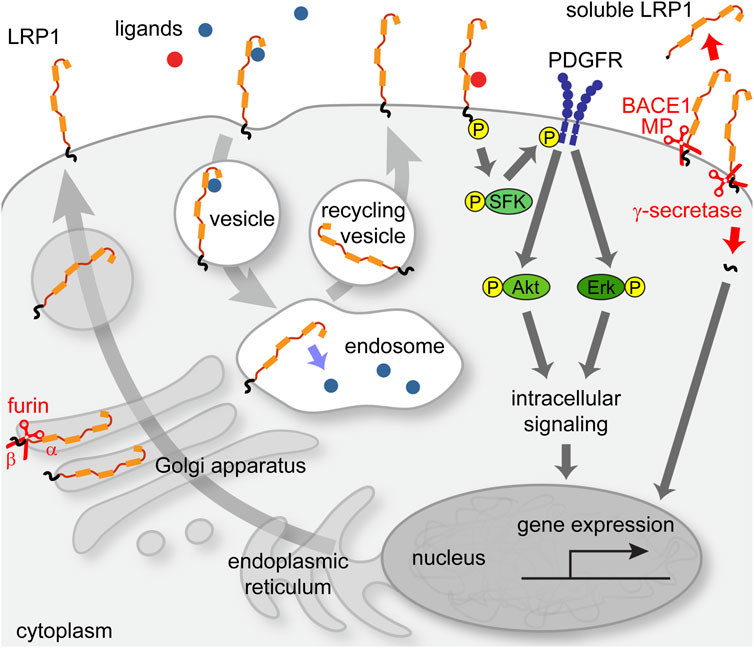
FIGURE 2. Intracellular pathways of LRP1 receptor. LRP1 precursor protein is cleaved by a furin-type protease in the Golgi apparatus and transported to the membrane. Upon ligand binding, an endocytosis process is initiated that leads to transit of LRP1 to the endosomal compartment, from where it is recycled back to the membrane after the release of cargo. In association with other receptors, e.g., PDGFRβ, LRP1 can elicit signal transduction pathways that can impact cellular functions such as gene regulation via the Erk-pathway.
Interestingly, the α-subunit can be released into the extracellular space where it exerts functions independently of the β-subunit. Soluble sLRP1 is the major transport protein for Abeta and prevents the re-entry of the circulating protein from the plasma into the brain (Sagare et al., 2012). The β-subunit that persists in the membrane is cleaved by γ-secretase and the resulting fragment can transit to the nucleus and influence gene transcription (May et al., 2002; Zurhove et al., 2008). In the CNS, LRP1 is expressed by neuronal and glia cell populations (Auderset et al., 2016a). Furthermore, the LRP1 receptor is prominent in the blood-brain-barrier (BBB) where it is present in endothelia, astrocytic end-feet, pericytes and smooth muscle cells (Ma et al., 2018; Storck et al., 2021b; Oue et al., 2023). Interestingly, LRP1 is also expressed in the neural stem cell compartment, by NSPCs (Hennen et al., 2013; Auderset et al., 2016b). However, its potential roles during neural development had not been addressed so far. In order to study its functions in the radial glia stem cell and glial progenitor compartments, we have exploited the Lrp1fl/fl mouse line and deleted the receptor by means of Cre-loxP mediated recombination in vitro using recombinant membrane permeable Cre-recombinase (Nolden et al., 2006). The functional status of LRP1-deficient cells was studied using proliferation, differentiation and apoptosis assays. LRP1-deficient NSPCs from both embryonic spinal cord and cortex demonstrated an altered differentiation potential. The differentiation capacity towards oligodendrocyte precursor cells (OPCs), mature oligodendrocytes and neurons was reduced. In contrast, astrogliogenesis was promoted. Moreover, Lrp1 deletion had a negative effect on NSPC proliferation and survival. Our observations suggested that LRP1 facilitates NSPC differentiation via interaction with ApoE. Upon ApoE4 stimulation wild type NSPCs generated more oligodendrocytes, but LRP1 knockout cells showed no response. The effect of ApoE4 seemed to be independent of cholesterol uptake, but was rather mediated by downstream MAPK and PI3K/Akt activation. In conclusion, in the light of our results LRP1 was characterized as a membrane receptor that controls important signalling pathways in the glial stem and progenitor cell compartment of the developing CNS (Hennen et al., 2013; Safina et al., 2016).
1.3 Emx1 as driver of conditional Lrp1 knockout
In light of the functional roles of LRP1 in the radial glia stem and glial progenitor cell compartments, we decided to investigate its roles in neural stem cells in vivo. To this end, we created a mouse line by crossing the Lrp1fl/fl animals with an Emx1-Cre driver line (Table 1). The mouse empty spiracles homeobox gene family consists of the members Emx1 and empty spiracles homeobox 2 (Emx2). Both regulatory genes are restricted to the forebrain. The Emx1 gene expression in the mouse starts at E9.5 and is confined to the dorsal forebrain that gives rise to the cerebral cortex, olfactory bulbs and hippocampi. The Emx1 gene is found in radial glia, postmitotic projection neurons of the dorsal, lateral and medial pallium forming the neocortex, piriform cortex and hippocampi, respectively, as well as in astrocytes and oligodendrocytes of the pallial corpus callosum and fimbria in the developing brain (Cecchi and Boncinelli, 2000; Gorski et al., 2002). The Emx1-Cre::Lrp1fl/fl line drives the expression of the Cre-recombinase in the telencephalic radial glia compartment and thereby allows for a selective elimination of a gene of interest in the radial glia cells of the forebrain (Iwasato et al., 2000). We obtained viable offspring where the Lrp1 gene had selectively been deleted in the cortical radial glia lineage (Table 1). Emx1 lineage cells do not give rise to GABAergic inhibitory interneurons and subpallial oligodendrocytes. Considering the Emx1Cre distribution, it was expected that in the novel mouse mutant the Lrp1 gene would only be absent in dorsal forebrain radial glia and their progeny. However, the Emx1 expressing cells located in the basal telencephalon can form two distinct populations. The first one gives rise to pyramidal-like, burst-firing, excitatory cells of the amygdala while the second population migrates from the pallium to the subpallium and finally differentiates into medium spiny neurons (Cocas et al., 2009).
1.4 Elimination of Lrp1 from radial glia causes and epileptic seizures
Epilepsy is a neurological disorder that affects up to 1% of the population and leads to severe restrictions of the private and professional lives of those affected (Binder and Steinhauser, 2006). A large range of conditions and pathophysiological developments can cause the disease that is characterized by a shift of the balance between excitation and inhibition (Devinsky et al., 2013; Patel et al., 2019). This imbalance eventually leads to hyperexcitability in distinct brain regions (Stafstrom, 2006; Fritschy, 2008; Steinhäuser and Seifert, 2012; Devinsky et al., 2018; Righes Marafiga et al., 2021). A quest for molecular mechanisms may identify novel, potentially interesting genes as novel targets for therapeutical intervention (Goldberg and Coulter, 2013). In the Emx1-Cre::Lrp1fl/fl knockout mouse pronounced, generalized seizure episodes occurred spontaneously as early as at postnatal day P19 (Bres et al., 2020). An analysis conducted with the c-fos marker revealed that hyperactive neurons were located in the hippocampus, in deep cortical layers and in the thalamus. It is plausible that epileptic activity beginning in the dorsal telencephalon can spread to basal ganglia and higher-order brain regions, resembling the c-fos expression pattern (Constantinople and Bruno, 2013). We have not been able to localize a distinct region of origin of the seizure activity, which would require sophisticated EEG-recording techniques with higher resolution. In a subpopulation of knockout mice the seizures recurred daily and were accompanied by deteriorating health including decreased body weight and, finally, a reduced life span. Indeed, more than 70% of the affected animals did not survive beyond 3 months (Bres et al., 2020). The severity of the phenotype was unexpected and contrasts investigations concerning the role of LRP1 in the neuronal lineage. In fact, ablation of Lrp1 in neurons using CaMKII-Cre mouse lines yielded solely symptoms of hyperexcitability that did not, however, progress to seizure-like symptoms (May et al., 2004; Liu et al., 2010; Maier et al., 2013).
In addition to the presence of seizures, Emx1-Cre::Lrp1fl/fl mice exhibit a clasping reflex when held by the tail. As motor-coordination deficits were detected previously in Lrp1 neuronal knock-out mice (Liu et al., 2010) behavioral tests were performed. The results of a panel of motor tests including the rotarod test, the hangwire test and footprint analysis confirmed that Emx1-Cre::Lrp1fl/fl knock-out mice suffered from cortically induced ataxia (Bres et al., 2020). The cortical circuitry resulting in ataxia in the Emx1-Cre::Lrp1fl/fl line has not been elucidated in detail. The Emx1 lineage medium spiny neurons contribute both to movement facilitation and inhibition, but the majority is involved in movement inhibition (Cocas et al., 2009). In this regard, Emx1-expressing medium spiny neurons which receive dopaminergic input from and provide output to the substantia nigra may contribute to the effect. The prefrontal cortex and the nigrostriatal system are involved in movement initiation and the control of motor activity (Laruelle et al., 2003).
In the Emx1-Cre::Lrp1fl/fl mouse mutant both neuron density and cortical layering were not affected. In this respect the model is similar to the synapsin-Cre::Lrp1fl/fl mutant described earlier (May et al., 2004). Neuron maturation and viability were also not compromised in the Nestin-Cre::Lrp1fl/fl mouse, but reduced neurite growth and branching was observed (Nakajima et al., 2013). This observation corresponds to the reduced length of neurites growing out from Lrp1-deficient neural stem cells in vitro (Safina et al., 2016). Along these lines, blockade of LRP1 by RAP also impaired neurite outgrowth (Qiu et al., 2004). In a neuronal knockout of Lrp1 driven by CaMKII-Cre a reduction of spines has been reported in cortical and in hippocampal neurons (Liu et al., 2010).
The observation of seizures in the Emx1-Cre::Lrp1fl/fl mouse model raises the question whether LRP1 mutations may be a cause of seizures in the human. To date, no reports are available that support an involvement of LRP1 in human epilepsy. However, genetic studies suggest a link between LRP1 truncation variants and autism and pleiotropic effects across psychiatric phenotypes (Torrico et al., 2019). With regard to epilepsy, an enhanced expression of LRP1 was detected in foci of a kainate mouse model of drug-resistant epilepsy (DRE). On that basis, a micellar-based LRP1-targeted paramagnetic Gd3+-LP probe was developed to visualize epileptic foci in vivo. This approach was efficient and informative both in the mouse model and in human DRE patients (Wang et al., 2021). This observation allowed for the application of the MRI probe to circumscribe epileptic foci in the context of therapeutic surgery (Li et al., 2023). This represents an application of the upregulation of LRP1 in seizure territories.
1.5 Ventricular enlargement in the Emx1-Cre::Lrp1fl/fl mouse
A striking phenotype characteristic found in P28 Emx1-Cre::Lrp1fl/fl mice is the enlargement of the volume of the lateral ventricles. Using the magnetic resonance imaging (MRI) technique, it was ascertained that knock-out mice displayed a significant enlargement of the lateral ventricular volume and a compression of the dorsal hippocampus. Remarkably, the expansion of ventricular volume was only mildly visible at P14, but clearly evident after 4 weeks of age, in close correlation with the appearance of seizures and weight loss. The increased ventricular size may translate into the hippocampal compression observed in juvenile Emx1-Cre::Lrp1fl/fl mice. Several pathways may lead to this complex phenotype. One plausible cause may be the augmented production of cerebrospinal fluid (CBF). A possible imbalance of fluid production and removal in the ventricular space addresses the neurovascular unit (NVU). This structure consists of the endothelia that line the lumen of the capillaries, the basement membrane the endothelia appose to, the pericytes that cover the surface of the vessels and the astrocytic processes that abut to the external face of the blood vessel (Kaplan et al., 2020). The diameter of the vessels is in part regulated by vascular smooth muscle cells (VSMC) (Sweeney et al., 2016). These cellular components cooperate to form the blood-brain barrier (BBB) that protects the inner milieu of the CNS and regulates the exchange with the blood stream (Kaplan et al., 2020). Thus, astrocytic function may be disturbed, which has also been discussed as a potential reason for the pathophysiology of epilepsy and BBB integrity (Bedner et al., 2015; Robinson and Jackson, 2016; Liu et al., 2017a).
Astrocytic LRP1 and tPA regulate blood-brain barrier (BBB) permeability and inadequate tPA-LRP1, tPA-neuroserpin or tPA-Mac-1-LRP1-PDGFRβ interactions in the area of the BBB affect the permeability of the neurovascular unit in the context of seizures (Polavarapu et al., 2007; Niego et al., 2012; Nakajima et al., 2014; Fredriksson et al., 2015; Su et al., 2017). The increased ventricular space could reflect malfunctioning of the astrocytic membranes and perivascular astrocytic end feet. The aquaporin 4 (AQP4) transmembrane water channel protein is highly expressed by astrocytes and plays a pivotal role for extracellular space volume regulation and waste clearance by the glymphatic system (Haj-Yasein et al., 2011; Eidsvaag et al., 2017). AQP4 has been implicated in modifications of the BBB in the context of Alzheimer disease and temporal lobe epilepsy (Alvestad et al., 2013; Hubbard et al., 2016; Lan et al., 2017). In agreement with a role of AQP4, mice deficient for the channel suffer from hydrocephalus, accompanied by enlarged ventricles and elevated intracranial pressure (Verkman et al., 2006). Whether AQP4 is significantly changed in our model has not been addressed and is an interesting question for further studies (Bres et al., 2020).
Interestingly, LRP1 has been attributed a role in preventing over-proliferation of vascular smooth muscle cells which are important for blood vessel integrity (Boucher et al., 2003; Boucher et al., 2007; Boucher and Herz, 2011). Along these lines, PDGFRβ signaling intervenes in the recruitment of pericytes in the course of angiogenesis (Zhang et al., 2009) and deficits in blood vessel formation have been associated with temporal lobe epilepsy (Rigau et al., 2007). In this regard it is of interest that the interaction of tPA with PDGFRα is important for BBB integrity (Fredriksson et al., 2015). Because of the impact of the removal of LRP1 on the levels of tPA in the CNS potential modifications of the vascular system in the Emx1-Cre::Lrp1fl/fl mutant merit further investigations (Stefanitsch et al., 2015). Using genetic techniques, the roles of LRP1 expression in the cellular components of the NVU has been studied in more detail (Table 1). Using a Tie2-Cre::Lrp1fl/fl where LRP1 was eliminated in all endothelia the BBB was progressively degraded which resulted in neuronal loss and cognitive deficits (Nikolakopoulou et al., 2021). In an approach that was focused on the endothelia of the CNS the Slco1c1-Cre::Lrp1fl/fl similarly displayed a degradation of the BBB which could be traced to a degradation of the tight junctions of the endothelia and a reduction of the P-glycoprotein (Storck et al., 2021b). These observations are highly relevant in the context of Alzheimer disease, as LRP1 has been attributed an important role in the clearance of Abeta from the CNS (Storck et al., 2016; Storck and Pietrzik, 2017; Petrushanko et al., 2023).
Beyond the endothelia also the pericytes play important roles in the BBB. Thus, pericytes contribute to the integrity of the BBB, are involved in the regulation of angiogenesis, have phagocytic activities, contribute to CBF regulation and to neuroinflammatory responses (Sweeney et al., 2016). The ablation of LRP1 from pericytes in a Cspg4-Cre::Lrp1fl/fl mouse line documented that pericytes can clear Abeta peptide in an LRP1/ApoE-dependent mechanism (Ma et al., 2018). As a further component, the expression of LRP1 in VSMC has been tested in a genetic approach where cell-type restricted deletion was carried out in a sm22α-Cre::Lrp1fl/fl mouse line. The functions of LRP1 were assessed against an APOE3 or APOE4 background. In the latter, the removal of LRP1 led to impaired spatial memory, accompanied by a disruption of the BBB (Oue et al., 2023). These studies clearly emphasize that LRP1 plays important roles in the BBB and partakes in the clearance of Abeta (Cai et al., 2018; Eggert et al., 2018; Storck et al., 2021a; Petrushanko et al., 2023). In several instances the cell-type specific deletion of LRP1 resulted in compromised BBB function and, consequently, increased permeability of the BBB. Whether the BBB is compromised in the Emx1-Cre::Lrp1fl/fl model remains to be seen (Bres et al., 2020). In this context, it is of interest that Alzheimer disease is associated with seizures and epilepsy (Purushotham et al., 2022; van Vliet and Marchi, 2022; Yang et al., 2022). The potential association between BBB deficits, Alzheimer disease and seizures in the available mouse models will have to be explored further.
1.6 LRP1 and glutamate signaling
PSD-95 is a scaffolding protein of the membrane-associated guanylate kinase (MAGUK) that is highly enriched at the postsynaptic density. Among other structural features it comprises three conserved PDZ domains and one Src-homology 3-guanylate kinase module (Cheng et al., 2006). PSD-95 expression was found reduced at stages P28 and P56 both in the Emx1-Cre::Lrp1fl/fl and the CaMKII-Cre::Lrp1fl/fl mutants (Liu et al., 2010; Nakajima et al., 2013; Bres et al., 2020). PSD-95 interacts with a variety of receptors and proteins at the excitatory synapse and is implicated in the tuning of synaptic strength by regulating the number of alpha-amino-3-hydroxy-5-methyl-4-isoxazolepropionic acid (AMPA) receptors (May et al., 2004; Bats et al., 2007; Nakajima et al., 2013). PSD-95 is rather stable and serves to anchor AMPA receptors at the synapse (Chen et al., 2011). The reduction of PSD-95 leads to a reduction of the amount of AMPA receptors (Elias et al., 2006). Surprisingly, the elimination of PSD-95 does not severely affect synaptic transmission, probably because the loss is compensated by other MAGUK-family members (Elias et al., 2006; Chen et al., 2011).
The onset of epileptic seizures in the Emx1-Cre::Lrp1fl/fl mutant corresponds with the period of circuit maturation and maximum NMDAR expression (Szczurowska and Mareš, 2013). LRP1 interacts with PSD-95 and the NMDAR and thereby supposedly affects NMDAR-dependent calcium currents (Gotthardt et al., 2000; Qiu et al., 2002; Martin et al., 2008). It has been proposed that the hyperactivity and dystonia that occur in Synapsin-Cre::Lrp1fl/fl mutants are the consequence of missing modulation of ligand-dependent ion currents caused by the absence of LRP1 (May et al., 2004). In accordance with this assumption, the stimulation of neurons with N-Methyl-D-aspartate (NMDA) causes a reduction of PSD-95-LRP1 complexes, suggesting that LRP1 interacts with the active pool of PSD-95 which is degraded in response to NMDA treatment (Colledge et al., 2003; May et al., 2004).
A link between epilepsy and the downregulation of PSD-95 has been suspected (Wyneken et al., 2003; Sun et al., 2009). NMDARs are ionotropic glutamate channels and play a key role for excitatory synaptic transmission. These tetrameric receptors are composed by two obligatory GluN1 subunits, regulatory GluN2A-D and NMDAR (GluN3)A-B subunits. NMDARs are display high permeability for Ca2+, a voltage-dependent Mg2+ block and require the simultaneous binding of glutamate and d-serine or glycine for channel activation (Traynelis et al., 2010; Hansen et al., 2018). During development the NMDARs switch the subunit composition from GluN2B to preferentially GluN2A comprising combinations (Liu et al., 2004). GluN2A-containing receptors are mostly present at synapses whereas GluN2B are extrasynaptically located (Pal, 2018). In the Emx1-Cre::Lrp1fl/fl knock-out mice, no developmental changes of the obligatory NMDAR subunit GluN1 have been noted in vivo (Bres et al., 2020). However, the level of GluN1 was found increased in the membrane of NSPC-derived neurons in vitro using a cell surface biotinylation technique (Safina et al., 2016). Different from this result, cortical neurons obtained from E15-16 cortex of a Nestin-Cre::Lrp1fl/fl mouse cultured for up to 12 days did not reveal modifications of NMDAR levels (May et al., 2004). However, GluN1 expression was reported reduced in cortical neurons of a CaMKII-Cre::Lrp1fl/fl knockout mouse line (Liu et al., 2010; Gan et al., 2014). Along these lines a reduction of both GluN2B and the AMPA receptor subunits GluA1 and GluA2 was observed in a kainate rat model of epilepsy (Egbenya et al., 2018). These apparent differences may result from the differing promoters driving the Cre-recombinase.
In this regard, it is of interest to consider the opposing strategy, namely, the consequences of knock-in mediated overexpression. There, an upregulation of GluN1 and GluN2B in cultured neurons was noted which translated into behavioral modifications, such as hyperactivity and cognitive deficits (Roebroek et al., 2006; Reekmans et al., 2009; Maier et al., 2013). NMDAR expression is augmented in epileptic patients and in animal models of epilepsy (Mathern et al., 1998; Steffens et al., 2005). A decrease of PSD-95 scaffolding protein is supposedly responsible for a higher mobility of glutamate receptors and an elevation of extra-synaptic NMDARs, which may be relevant for the pathophysiology of epilepsy (Frasca et al., 2011; Gladding and Raymond, 2011; Parsons and Raymond, 2014). The location of NMDARs makes a significant functional difference (Hardingham and Bading, 2010; Bading, 2017). The subunit GluN1 is highly mobile and ligand interactions strongly influence the conformation of the receptor (Zhu et al., 2013). Overall, these results suggest that LRP1 intervenes in the uptake and recycling of glutamate receptors and thereby tunes the sensitivity of neuronal responses (Bading, 2017). However, the resulting changes in network activity are not sufficient to elicit seizures. Because the elimination of Lrp1 in radial glia results in a deletion in both neurons and glia, the glial lineages also have to be considered with regard to the severe phenotype of the Emx1-Cre::Lrp1fl/fl knockout mutant.
1.7 Modulation of glutamate signalling by tPA-LRP1 interactions
Interestingly, the prominent LRP1 ligand tPA interacts with the GluN1 N-terminal domain and can elicit cytotoxicity (Nicole et al., 2001; Samson et al., 2008; Varangot et al., 2023). This notion has been elaborated further in that tPA interaction with GluN1 promotes the extra-synaptic diffusion of NMDARs without increasing the membrane located pool of the receptor (Lesept et al., 2016). The serine protease tPA belongs to the chymotrypsin family and consists of a heavy A-chain and a light B-chain. The A-chain contains a finger domain that mediates binding to fibrin and the membrane receptors Annexin II and LRP1 (Kagitani et al., 1985; Bu et al., 1992; Hajjar et al., 1994). Further structural motifs include an EGF-like domain that interacts with the EGFR and Kringle 1 and 2 domains, where the latter binds to NMDAR (Correa et al., 2011; Lesept et al., 2016). tPA is released as single chain and processed to its active form by plasmin or kallikrein (Leys et al., 2016). High concentrations of tPA can exert neurotoxic effects by stimulating the NMDAR (Bertrand et al., 2015; Chevilley et al., 2015; Wu et al., 2017). tPA has been implicated in a range of CNS pathologies, including Alzheimer disease and psychotic episodes (Fabbro and Seeds, 2009; Hoirisch-Clapauch and Nardi, 2015) and contributes to the integrity of the BBB (Polavarapu et al., 2007; Fredriksson et al., 2015; Stevenson et al., 2022). tPA is expressed in the CNS by excitatory pyramidal glutamatergic neurons, somatostatin expressing neurons of the hippocampus, and astrocytes (Fredriksson et al., 2015; Hebert et al., 2016; Louessard et al., 2016; Stevenson and Lawrence, 2018). The release of tPA affects synaptic activity and acts as a homeostatic regulator of the postsynaptic response, in dependence of activity and Ca2+ concentration (Wu et al., 2015; Jeanneret et al., 2016; Yepes, 2016). In the Emx1-Cre::Lrp1fl/fl mice no salient deviations of tPA concentrations compared to the control were observed (Bres et al., 2020). The exact impact of tPA on seizure generation and epilepsy progression is, however, manifold (Tan et al., 2012). tPA possesses a plethora of functions that are vital for CNS homeostasis (Hebert et al., 2016; Briens et al., 2017; Stevenson et al., 2022). tPA is highly expressed in brain regions undergoing cellular migration and mice deficient for tPA show an increased number of granule neurons that migrate in the molecular layer of the cerebellum (Seeds et al., 1999). tPA influences cortical neuron differentiation (Lee et al., 2014) and is implicated in proper radial glia maturation and neuron migration via interaction with the NMDAR expressed by radial glia (Pasquet et al., 2019). With regard to signaling, it is known that tPA interaction with LRP1 is important for the generation of late LTP in the hippocampus (Madani et al., 1999; Zhuo et al., 2000; Makarova et al., 2003; Wiera and Mozrzymas, 2015). tPA can also modulate NMDAR related signaling via LRP1 and thereby act in a neuroprotective or neurotoxic manner (Nicole et al., 2001; Jullienne et al., 2011; Ng et al., 2012; Parcq et al., 2012; Mantuano et al., 2013; Bertrand et al., 2015; Hedou et al., 2021). In the Emx1-Cre::Lrp1fl/fl mutant we observed by patch-clamp a hyperexcitability of pyramidal neurons in the hippocampus at P28 which was characterized by decreased action potential thresholds and increased action potential firing rates. This phenotype correlated with a decrease of PSD-95 and an elevated surface expression of the GluN1 subunit of the NMDAR (Bres et al., 2020).
1.8 Expression of LRP1 in the astrocyte lineage
LRP1 is expressed by neurons, but also by glial cells, namely, astrocytes and oligodendrocytes (Auderset et al., 2016a). The astrocytes play an important role for synapse formation, synaptic signaling and plasticity, which has led to the concept of the tripartite synapse (Araque et al., 1999; Perea et al., 2009; Faissner et al., 2010; Farhy-Tselnicker and Allen, 2018; Verkhratsky and Nedergaard, 2018). In this perspective, the astrocyte can intervene in synaptic signaling, modulates synaptic activity and has been attributed a role in the progression of epilepsy (Vezzani et al., 2022). For example, astrocytes express glutamate transporters Eaat1 (GLT1) and Eaat2 (GLAST) that serve glutamate clearance at the active excitatory synapse (Danbolt, 2001). This function can be altered in the case of epilepsy (Seifert et al., 2006; Steinhäuser and Seifert, 2012). Therefore, the astrocyte lineage was examined in more detail in the Emx1-Cre::Lrp1fl/fl mutant. When Lrp1 was deleted from NSPCs in vitro, an augmented production of GFAP-expressing astrocytes was detected in differentiation assays (Safina et al., 2016). Also the Emx1-Cre::Lrp1fl/fl mutant displayed a significant increase of GFAP, yet at P28, paralleling the emergence of severe seizures. These were also reflected by a strong increase of c-fos-positive neurons in the epileptic CNS (Bres et al., 2020). This raised the question whether the glutamate transport system was changed, also in light of the fact that GLT-1 is responsible for up to 90% of glutamate uptake in the synaptic region and has been implicated in epilepsy (Kong et al., 2012; Hanson et al., 2015; Petr et al., 2015; Hubbard et al., 2016; Hotz et al., 2022). Small variations of transporter expression were detected at earlier developmental stages, but not at P14 or later, when the seizure activity progresses. Therefore, tampered glutamate transport did not seem to be the major cause of seizure in the Emx1-Cre::Lrp1fl/fl mutant (Bres et al., 2020). It is known that tPA is co-released with glutamate at the glutamatergic synapse and taken up by neighboring astrocytes (Cassé et al., 2012). Therefore, a deletion of Lrp1 from the astrocyte membrane should reduce the clearance of tPA at active synapses. When the Lrp1-deficient astrocytes were tested the ability for tPA uptake was found strongly reduced (Bres and Faissner, 2019a; Bres et al., 2020). This led to the concept that the Emx1-Cre::Lrp1fl/fl mutant is characterized by an elevation of NMDARs, reduced clearance of tPA and transiently compromised glutamate clearance that result in a disturbed tPA-NMDAR signaling axis. This may contribute to or even cause the seizure phenotype that represents a salient feature of the mutant (Bres et al., 2020).
This idea was followed further by the generation of a conditional mutant where Lrp1 was deleted exclusively from the astrocyte lineage (Romeo et al., 2020). To this end, the floxed reporter mouse line GLAST-CreERT2::Rosa26fl/fl was mated with the LRP1fl/fl mice to generate a triple-transgenic line that expresses the Tamoxifen-inducible Cre-recombinase under the control of the astroglial GLAST-promoter (Rohlmann et al., 1996; Mori et al., 2006; Madisen et al., 2010; Romeo et al., 2020). The reporter tdTomato allowed for tracing of the recombined cells (Table 1). The recombination and deletion of Lrp1 could successfully be induced by addition of Tamoxifen to cultivated astrocytes. When the astrocytes obtained from the mutant were co-cultivated with hippocampal neurons in an in vitro coculture model (Geissler and Faissner, 2012; Gottschling et al., 2016), LRP1-deficient astrocytes caused changes in synaptogenesis and a decrease in synaptic activity in hippocampal neuronal networks. This was accompanied by a change of cytokine patterns released by the astrocytes in the coculture setting (Romeo et al., 2020). For further characterization, the recombination and deletion of Lrp1 was induced in astrocytes in vivo, at early postnatal stages. In these mutants, the maturation of astrocytes appeared significantly retarded compared to controls. This resulted in a reduced neuronal activity in vivo, as monitored by the detection of the immediate early gene c-Fos in hippocampal neurons at P21 (Romeo et al., 2021). In accordance with this observation, a recent investigation has pointed out that the genetic knockout of APOE resulted in a decrease of lipid-accumulating reactive astrocytes and reduced seizures in a mouse model of temporal lobe epilepsy (TLE) (Chen et al., 2023). Lipid accumulation in astrocytes thus emerges as a risk factor for seizures and Alzheimer disease (Tcw et al., 2022; Chen et al., 2023). The maturation of astrocytes depends among others on the activation of the Ras/Raf/MEK/Erk signaling pathway (Franzdóttir et al., 2009; Li et al., 2012). This pathway can be activated by a complex of LRP1 with the PDGFβ-receptor (Boucher et al., 2002; Loukinova et al., 2002). Interestingly, the amount of activated Erk was significantly reduced in the LRP1-deficient GLAST-CreERT2::Rosa26 fl/fl::Lrp1fl/fl mouse line at P21, consistent with a delay in astrocyte maturation. Cognitive abilities and motor coordination were also examined in the GLAST-CreERT2::Rosa26fl/fl::Lrp1fl/fl knockout mouse line and found unaltered. In conclusion, the conditional elimination of Lrp1 from the astrocyte lineage revealed a link to astrocyte maturation. In contrast to the Emx1-Cre::Lrp1fl/fl mouse line, however, neither hyperexcitability nor seizures were observed in this model (Romeo et al., 2020; Romeo et al., 2021). This indicates that probably the LRP1 deficiency both in astrocytes and in neurons synergizes to produce the epilepsy phenotype (Bres et al., 2020).
It is of interest here to consider further approaches to elucidate LRP1 functions in astrocytes. The LRP1 and the LRP2 receptor are discussed as important modulators in the context of the Alzheimer disease because they contribute to the clearance of amyloid-beta (Aβ) peptide (Shinohara et al., 2017; Cai et al., 2018; Tachibana et al., 2019; Knopman et al., 2021). The selective knock-out of Lrp1 in astrocytes results in an accumulation of Aβ-peptide in the CNS and exacerbates disease development in APP/PS1 mice, an established model of Alzheimer disease (Kanekiyo et al., 2013; Liu et al., 2017b). This underlines the importance of the LRP1-based clearance system in pathology, an aspect that has recently been emphasized by the report that LRP1 plays also a pivotal role in the clearance of pathogenic Tau-protein (Fearon and Lynch, 2020; Rauch et al., 2020) and of α-synuclein (Chen et al., 2022). However, also in this approach the removal of Lrp1 from the astrocyte lineage did not result in an hyperexcitability phenotype.
1.9 Significance of LRP1 in the oligodendrocyte lineage and in myelin
The ablation of Lrp1 from NSPCs in vitro also resulted in the impaired maturation of oligodendrocytes (Safina et al., 2016). This may reflect the fact that oligodendrocyte maturation heavily relies on the supply of cholesterol (Saher et al., 2005). Myelin malformation may cause both motor deficits and hyperexcitability in neural tissues (Nave and Werner, 2014; Nave and Werner, 2021). Therefore, potential roles of LRP1 for myelin formation were examined in more detail. It is known that LRP1 is expressed by young oligodendrocytes and oligodendrocyte precursors and rapidly lost upon differentiation (Zhang et al., 2014; Auderset et al., 2016a). An important task of LRP1 with reference to myelin is the clearance of debris in the context of lesion (Gaultier et al., 2009; Fernandez-Castaneda et al., 2013; May, 2013; Sharp et al., 2023). Furthermore, LRP1 can bind the myelin-associated glycoprotein (MAG) and expose the inhibitor to neurites (Stiles et al., 2013). Oligodendrocytes are generated in three distinct waves and colonize the developing nervous system through a migratory process (Rowitch and Kriegstein, 2010). Therefore, LRP1 may be of significance for myelin formation during the early developmental stages of oligodendrocyte precursor cell (OPC) formation. In order to explore the role of LRP1 for OPCs, a novel triple transgenic mouse line was elaborated (Table 1). To this end, a triple transgenic mouse model was generated that expressed the inducible Cre-recombinase under the control of the NG2-promoter (Huang et al., 2014). The resulting NG2-CreERT2::R26eGFP::Lrp1fl/fl line enabled the induced recombination and removal of LRP1 from NG2-positive cells. The recombined cells would furthermore express the eGFP reporter, so that the LRP1 deficient cells could efficiently be tracked in vivo and in vitro (Schafer et al., 2019). The knockout of Lrp1 was induced three and 4 days after birth and the resulting mice were examined at P7, P14, P21, P28, P42 and P56. The development of oligodendrocytes and myelin was monitored in the corpus callosum, a strongly myelinated structure of the CNS. The recombination and removal of LRP1 in the oligodendrocyte lineage proved successful. However, no significant alterations of myelin were observed in the mutant in comparison to the control, and no apparent motor deficits could be recorded. In contrast, when the fluorescent marker protein eGFP was set in relation to the Olig2 marker of the oligodendrocyte lineage it appeared that the recombined, fluorescent Olig2-positive cells dramatically decreased during the postnatal period. This was interpreted as indicating that the LRP1-deficient, reporter expressing and Olig2-positive double fluorescent OPCs progressively were eliminated from the system and presumably replaced by non-recombined NG2-and LRP1-positive OPCs (Zhu et al., 2008; Dimou and Gallo, 2015; Schafer et al., 2019). This result suggests a stronger vulnerability of the LRP1-deleted OPCs, in agreement with the previous findings reporting reduced numbers of oligodendrocytes obtained from Lrp1-deficient recombined NSPCs (Safina et al., 2016). Accordingly, in vitro studies using immuno-panned OPCs only recovered a small minority of <3% LRP1-negative, fluorescent recombined cells, much less than expected, which could be explained by the increased vulnerability of the LRP1-deleted OPCs (Schafer et al., 2019). In another approach where LRP1 was deleted from the oligodendrocytes using Olig2-Cre::Lrp1fl/fl mice the mutants showed hypomyelination of the optic nerve and a clear decrease of differentiated oligodendrocytes. Furthermore, in both CAG-CreERTM::Lrp1fl/fl and PDGFRa-CreERTM::Lrp1fl/fl the ability to repair myelin was restricted compared to the wild-type (Lin et al., 2017). This phenotype was correlated with a decrease of peroxisomal biogenesis factor-2 and a reduced number of peroxisomes in oligodendrocyte processes. The deficits could be corrected by a combined treatment with cholesterol and pioglitazone, a drug that promotes peroxisome proliferation (Lin et al., 2017). Recently, a study using the PDGFRa-CreERTM::Rosa26-YFP::Lrp1flflf line addressed the relevance of LRP1 in adult CNS myelin, with focus on the corpus callosum (Table 1). Upon Tamoxifen treatment, Lrp1 could successfully be eliminated from oligodendrocytes in the adult CNS, which, surprisingly, resulted in an increase of OPC proliferation and of the number of new oligodendrocytes added to the adult brain. Furthermore, the number of OPCs recruited upon Cuprizone feeding was also augmented. This correlated with an heightened proportion of OPCs that differentiated in vitro (Auderset et al., 2020). Considering the contrast of these results with the studies of LRP1 ablation in the oligodendrocytes during the postnatal period, the authors hypothesize that postnatal and adult oligodendrocytes may have different properties and requirements (Auderset et al., 2020). Of note, in neither of the reported lineage-restricted eliminations of LRP1 from oligodendrocytes and myelin motor deficits, let alone hyperexcitability or seizures could be detected. Thus, the removal of LRP1 from the radial glia stem cell has a stronger impact than the one obtained by elimination of LRP1 from individual neural lineages (Table 1).
1.10 Conclusion and outlook
The receptor protein LRP1 interacts with more than 40 different ligands and plays a pivotal role for the uptake and clearance of a large variety of molecules. It has so far been considered in the context of disease, in particular Alzheimer disease in view of its role in the clearance of Aβ-peptide (Storck et al., 2016; Eggert et al., 2018; Van Gool et al., 2019). In view of its importance it is not surprising that the constitutive knock-out of Lrp1 is lethal at early embryonic stages (Herz et al., 1992). While the major emphasis in studying LRP1 has been oriented towards its role in the homeostasis of tissues and in protecting the CNS against the accumulation of pathogenic metabolic products, we have discovered a prominent role in the stem cell compartment during early development (Hennen et al., 2013; Safina et al., 2016). Several animal models have been constructed to decipher the functions of LRP1 in distinct cellular lineages of the developing CNS (Table 1). It appears that by far the most dramatic phenotype was obtained when Lrp1 was deleted from the cortical radial glia compartment using the Emx1 promoter as driver of Cre recombinase (Bres et al., 2020). Neither the knockout in neurons (Liu et al., 2010; Maier et al., 2013), nor the knockout in oligodendrocytes (Lin et al., 2017; Schafer et al., 2019; Auderset et al., 2020) or astrocytes (Liu et al., 2017b; Romeo et al., 2020; Romeo et al., 2021) yielded a phenotype of comparable strength. This opens the perspective that seizures may be rooted in a neurodevelopmental deficit affecting the neural stem cell compartment. The identification of the genes and pathways involved may in the future benefit from the application of genomic and transcriptomic strategies to the mouse model (Busch et al., 2022; Lariviere et al., 2022; Pai et al., 2022; Assis-Mendonca et al., 2023; Chung et al., 2023; Huang et al., 2023).
Statement of significance
The low-density lipoprotein related protein receptor 1 (LRP1, also known as CD91) is a transmembrane receptor that interacts with more than 40 known ligands. In the CNS, it has primarily been studied as a receptor and clearance agent of pathogenic factors such as Abeta-peptide and, lately, Tau protein that is relevant for tissue homeostasis and protection against neurodegenerative processes. Recently, we have found that LRP1 is expressed in the neural stem cell compartment and regulates the development of glial lineages. When LRP1 was eliminated from cortical radial glia a strong phenotype with motor coordination deficits and severe seizures resulted. In the present review I discuss the attempts that have been made to understand the link of LRP1 expression in glial lineages to the generation of epilepsy, a burdening human disease. In particular, several conditional mouse lines with lineage-specific ablation of LRP1 have been constructed and described. Taken together, the studies strongly suggest that cooperative effects between neurons and glia are responsible for the phenotype. These observations emphasize LRP1 as an important regulator of tissue homeostasis and highlight the significance of the neural stem cell compartment for neurodevelopmental disorders and CNS pathology.
Author contributions
AF: concept of the article, writing of the drafts, and revision.
Funding
The generation of the mouse lines generated in our laboratory discussed herein was supported by the German Research Foundation (DFG, SPP 1757/1,2, Fa 159/20-1,2, Grant Number 254968232, to AF). The author acknowledges support from the DFG Open Access Publication Funds of the Ruhr-Universität Bochum.
Acknowledgments
The author thanks L. Roll for critical comments and drawing Figures 1, 2.
Conflict of interest
The author declares that the research was conducted in the absence of any commercial or financial relationships that could be construed as a potential conflict of interest.
Publisher’s note
All claims expressed in this article are solely those of the authors and do not necessarily represent those of their affiliated organizations, or those of the publisher, the editors and the reviewers. Any product that may be evaluated in this article, or claim that may be made by its manufacturer, is not guaranteed or endorsed by the publisher.
References
Allen, N. J., and Barres, B. A. (2009). Neuroscience: Glia - more than just brain glue. Nature 457, 675–677. doi:10.1038/457675a
Alvestad, S., Hammer, J., Hoddevik, E. H., Skare, O., Sonnewald, U., Amiry-Moghaddam, M., et al. (2013). Mislocalization of AQP4 precedes chronic seizures in the kainate model of temporal lobe epilepsy. Epilepsy Res. 105, 30–41. doi:10.1016/j.eplepsyres.2013.01.006
Araque, A., Parpura, V., Sanzgiri, R. P., and Haydon, P. G. (1999). Tripartite synapses: Glia, the unacknowledged partner. Trends Neurosci. 22, 208–215. doi:10.1016/s0166-2236(98)01349-6
Assis-Mendonca, G. R., Athie, M. C. P., Tamanini, J. V. G., de Souza, A., Zanetti, G. G., Araujo, P., et al. (2023). Transcriptome analyses of the cortex and white matter of focal cortical dysplasia type II: Insights into pathophysiology and tissue characterization. Front. Neurol. 14, 1023950. doi:10.3389/fneur.2023.1023950
Auderset, L., Cullen, C. L., and Young, K. M. (2016a). Low density lipoprotein-receptor related protein 1 is differentially expressed by neuronal and glial populations in the developing and mature mouse central nervous system. PLoS One 11, e0155878. doi:10.1371/journal.pone.0155878
Auderset, L., Landowski, L. M., Foa, L., and Young, K. M. (2016b). Low density lipoprotein receptor related proteins as regulators of neural stem and progenitor cell function. Stem Cells Int. 2016, 2108495. doi:10.1155/2016/2108495
Auderset, L., Pitman, K. A., Cullen, C. L., Pepper, R. E., Taylor, B. V., Foa, L., et al. (2020). Low-density lipoprotein receptor-related protein 1 (LRP1) is a negative regulator of oligodendrocyte progenitor cell differentiation in the adult mouse brain. Front. Cell Dev. Biol. 8, 564351. doi:10.3389/fcell.2020.564351
Bading, H. (2017). Therapeutic targeting of the pathological triad of extrasynaptic NMDA receptor signaling in neurodegenerations. J. Exp. Med. 214, 569–578. doi:10.1084/jem.20161673
Bats, C., Groc, L., and Choquet, D. (2007). The interaction between Stargazin and PSD-95 regulates AMPA receptor surface trafficking. Neuron 53, 719–734. doi:10.1016/j.neuron.2007.01.030
Bedner, P., Dupper, A., Huttmann, K., Muller, J., Herde, M. K., Dublin, P., et al. (2015). Astrocyte uncoupling as a cause of human temporal lobe epilepsy. Brain 138, 1208–1222. doi:10.1093/brain/awv067
Bergles, D. E., and Richardson, W. D. (2016). Oligodendrocyte development and plasticity. Cold Spring Harb. Perspect. Biol. 8, a020453. doi:10.1101/cshperspect.a020453
Bertrand, T., Lesept, F., Chevilley, A., Lenoir, S., Aimable, M., Briens, A., et al. (2015). Conformations of tissue plasminogen activator (tPA) orchestrate neuronal survival by a crosstalk between EGFR and NMDAR. Cell Death Dis. 6, e1924. doi:10.1038/cddis.2015.296
Binder, D. K., and Steinhauser, C. (2006). Functional changes in astroglial cells in epilepsy. Glia 54, 358–368. doi:10.1002/glia.20394
Boucher, P., Gotthardt, M., Li, W.-P., Anderson, R. G. W., and Herz, J. (2003). Lrp: Role in vascular wall integrity and protection from atherosclerosis. Sci. (New York, N.Y.) 300, 329–332. doi:10.1126/science.1082095
Boucher, P., and Herz, J. (2011). Signaling through LRP1: Protection from atherosclerosis and beyond. Biochem. Pharmacol. 81, 1–5. doi:10.1016/j.bcp.2010.09.018
Boucher, P., Li, W. P., Matz, R. L., Takayama, Y., Auwerx, J., Anderson, R. G., et al. (2007). LRP1 functions as an atheroprotective integrator of TGFbeta and PDFG signals in the vascular wall: Implications for marfan syndrome. PLoS One 2, e448. doi:10.1371/journal.pone.0000448
Boucher, P., Liu, P., Gotthardt, M., Hiesberger, T., Anderson, R. G. W., and Herz, J. (2002). Platelet-derived growth factor mediates tyrosine phosphorylation of the cytoplasmic domain of the low Density lipoprotein receptor-related protein in caveolae. J. Biol. Chem. 277, 15507–15513. doi:10.1074/jbc.M200428200
Bres, E. E., and Faissner, A. (2019a). Detection of protein uptake in in vitro cultured astrocytes exemplified by the uptake of the serine protease, tissue plasminogen activator. Methods Mol. Biol. 1938, 203–217. doi:10.1007/978-1-4939-9068-9_14
Bres, E. E., and Faissner, A. (2019b). Low density receptor-related protein 1 interactions with the extracellular matrix: More than meets the eye. Front. Cell Dev. Biol. 7, 31. doi:10.3389/fcell.2019.00031
Bres, E. E., Safina, D., Muller, J., Bedner, P., Yang, H., Helluy, X., et al. (2020). Lipoprotein receptor loss in forebrain radial glia results in neurological deficits and severe seizures. Glia 68, 2517–2549. doi:10.1002/glia.23869
Briens, A., Bardou, I., Lebas, H., Miles, L. A., Parmer, R. J., Vivien, D., et al. (2017). Astrocytes regulate the balance between plasminogen activation and plasmin clearance via cell-surface actin. Cell Discov. 3, 17001. doi:10.1038/celldisc.2017.1
Bu, G., Maksymovitch, E. A., Nerbonne, J. M., and Schwartz, A. L. (1994). Expression and function of the low density lipoprotein receptor-related protein (LRP) in mammalian central neurons. J. Biol. Chem. 269, 18521–18528. doi:10.1016/s0021-9258(17)32340-2
Bu, G., Williams, S., Strickland, D. K., and Schwartz, A. L. (1992). Low density lipoprotein receptor-related protein/alpha 2-macroglobulin receptor is an hepatic receptor for tissue-type plasminogen activator. Proc. Natl. Acad. Sci. U. S. A. 89, 7427–7431. doi:10.1073/pnas.89.16.7427
Busch, R. M., Yehia, L., Blumcke, I., Hu, B., Prayson, R., Hermann, B. P., et al. (2022). Molecular and subregion mechanisms of episodic memory phenotypes in temporal lobe epilepsy. Brain Commun. 4, fcac285. doi:10.1093/braincomms/fcac285
Cai, Z., Qiao, P. F., Wan, C. Q., Cai, M., Zhou, N. K., and Li, Q. (2018). Role of blood-brain barrier in alzheimer's disease. J. Alzheimers Dis. 63, 1223–1234. doi:10.3233/JAD-180098
Cassé, F., Bardou, I., Danglot, L., Briens, A., Montagne, A., Parcq, J., et al. (2012). Glutamate controls tPA recycling by astrocytes, which in turn influences glutamatergic signals. Official J. Soc. Neurosci. 32, 5186–5199. doi:10.1523/JNEUROSCI.5296-11.2012
Cecchi, C., and Boncinelli, E. (2000). Emx homeogenes and mouse brain development. Trends Neurosci. 23, 347–352. doi:10.1016/s0166-2236(00)01608-8
Chen, K., Martens, Y. A., Meneses, A., Ryu, D. H., Lu, W., Raulin, A. C., et al. (2022). LRP1 is a neuronal receptor for α-synuclein uptake and spread. Mol. Neurodegener. 17, 57. doi:10.1186/s13024-022-00560-w
Chen, X., Nelson, C. D., Li, X., Winters, C. A., Azzam, R., Sousa, A. A., et al. (2011). PSD-95 is required to sustain the molecular organization of the postsynaptic density. J. Neurosci. 31, 6329–6338. doi:10.1523/JNEUROSCI.5968-10.2011
Chen, Z. P., Wang, S., Zhao, X., Fang, W., Wang, Z., Ye, H., et al. (2023). Lipid-accumulated reactive astrocytes promote disease progression in epilepsy. Nat. Neurosci. 26, 542–554. doi:10.1038/s41593-023-01288-6
Cheng, D., Hoogenraad, C. C., Rush, J., Ramm, E., Schlager, M. A., Duong, D. M., et al. (2006). Relative and absolute quantification of postsynaptic density proteome isolated from rat forebrain and cerebellum. Mol. Cell Proteomics 5, 1158–1170. doi:10.1074/mcp.D500009-MCP200
Chevilley, A., Lesept, F., Lenoir, S., Ali, C., Parcq, J., and Vivien, D. (2015). Impacts of tissue-type plasminogen activator (tPA) on neuronal survival. Front. Cell Neurosci. 9, 415. doi:10.3389/fncel.2015.00415
Chung, C., Yang, X., Bae, T., Vong, K. I., Mittal, S., Donkels, C., et al. (2023). Comprehensive multi-omic profiling of somatic mutations in malformations of cortical development. Nat. Genet. 55, 209–220. doi:10.1038/s41588-022-01276-9
Clarke, L. E., and Barres, B. A. (2013). Emerging roles of astrocytes in neural circuit development. Nat. Rev. Neurosci. 14, 311–321. doi:10.1038/nrn3484
Cocas, L. A., Miyoshi, G., Carney, R. S., Sousa, V. H., Hirata, T., Jones, K. R., et al. (2009). Emx1-lineage progenitors differentially contribute to neural diversity in the striatum and amygdala. J. Neurosci. 29, 15933–15946. doi:10.1523/JNEUROSCI.2525-09.2009
Colledge, M., Snyder, E. M., Crozier, R. A., Soderling, J. A., Jin, Y., Langeberg, L. K., et al. (2003). Ubiquitination regulates PSD-95 degradation and AMPA receptor surface expression. Neuron 40, 595–607. doi:10.1016/s0896-6273(03)00687-1
Constantinople, C. M., and Bruno, R. M. (2013). Deep cortical layers are activated directly by thalamus. Science 340, 1591–1594. doi:10.1126/science.1236425
Correa, F., Gauberti, M., Parcq, J., Macrez, R., Hommet, Y., Obiang, P., et al. (2011). Tissue plasminogen activator prevents white matter damage following stroke. J. Exp. Med. 208, 1229–1242. doi:10.1084/jem.20101880
Danbolt, N. C. (2001). Glutamate uptake. Prog. Neurobiol. 65, 1–105. doi:10.1016/s0301-0082(00)00067-8
Devinsky, O., Vezzani, A., Najjar, S., de Lanerolle, N. C., and Rogawski, M. A. (2013). Glia and epilepsy: Excitability and inflammation. Trends Neurosci. 36, 174–184. doi:10.1016/j.tins.2012.11.008
Devinsky, O., Vezzani, A., O'Brien, T. J., Jette, N., Scheffer, I. E., de Curtis, M., et al. (2018). Epilepsy. Nat. Rev. Dis. Prim. 4, 18024. doi:10.1038/nrdp.2018.24
Dimou, L., and Gallo, V. (2015). NG2-glia and their functions in the central nervous system. Glia 63, 1429–1451. doi:10.1002/glia.22859
Egbenya, D. L., Hussain, S., Lai, Y. C., Xia, J., Anderson, A. E., and Davanger, S. (2018). Changes in synaptic AMPA receptor concentration and composition in chronic temporal lobe epilepsy. Mol. Cell Neurosci. 92, 93–103. doi:10.1016/j.mcn.2018.07.004
Eggert, S., Thomas, C., Kins, S., and Hermey, G. (2018). Trafficking in alzheimer's disease: Modulation of APP transport and processing by the transmembrane proteins LRP1, SorLA, SorCS1c, sortilin, and calsyntenin. Mol. Neurobiol. 55, 5809–5829. doi:10.1007/s12035-017-0806-x
Eidsvaag, V. A., Enger, R., Hansson, H. A., Eide, P. K., and Nagelhus, E. A. (2017). Human and mouse cortical astrocytes differ in aquaporin-4 polarization toward microvessels. Glia 65, 964–973. doi:10.1002/glia.23138
Elias, G. M., Funke, L., Stein, V., Grant, S. G., Bredt, D. S., and Nicoll, R. A. (2006). Synapse-specific and developmentally regulated targeting of AMPA receptors by a family of MAGUK scaffolding proteins. Neuron 52, 307–320. doi:10.1016/j.neuron.2006.09.012
Fabbro, S., and Seeds, N. W. (2009). Plasminogen activator activity is inhibited while neuroserpin is up-regulated in the Alzheimer disease brain. J. Neurochem. 109, 303–315. doi:10.1111/j.1471-4159.2009.05894.x
Faissner, A., Pyka, M., Geissler, M., Sobik, T., Frischknecht, R., Gundelfinger, E. D., et al. (2010). Contributions of astrocytes to synapse formation and maturation - potential functions of the perisynaptic extracellular matrix. Brain Res. Rev. 63, 26–38. doi:10.1016/j.brainresrev.2010.01.001
Faissner, A., and Reinhard, J. (2015). The extracellular matrix compartment of neural stem and glial progenitor cells. Glia 63, 1330–1349. doi:10.1002/glia.22839
Faissner, A., Roll, L., and Theocharidis, U. (2017). Tenascin-C in the matrisome of neural stem and progenitor cells. Mol. Cell Neurosci. 81, 22–31. doi:10.1016/j.mcn.2016.11.003
Farhy-Tselnicker, I., and Allen, N. J. (2018). Astrocytes, neurons, synapses: A tripartite view on cortical circuit development. Neural Dev. 13, 7. doi:10.1186/s13064-018-0104-y
Fearon, C., and Lynch, T. (2020). Commentary: LRP1 is a master regulator of tau uptake and spread. Front. Neurol. 11, 557509. doi:10.3389/fneur.2020.557509
Fernandez-Castaneda, A., Arandjelovic, S., Stiles, T. L., Schlobach, R. K., Mowen, K. A., Gonias, S. L., et al. (2013). Identification of the low density lipoprotein (LDL) receptor-related protein-1 interactome in central nervous system myelin suggests a role in the clearance of necrotic cell debris. J. Biol. Chem. 288, 4538–4548. doi:10.1074/jbc.M112.384693
Franzdóttir, S. R., Engelen, D., Yuva-Aydemir, Y., Schmidt, I., Aho, A., and Klämbt, C. (2009). Switch in FGF signalling initiates glial differentiation in the Drosophila eye. Nature 460, 758–761. doi:10.1038/nature08167
Frasca, A., Aalbers, M., Frigerio, F., Fiordaliso, F., Salio, M., Gobbi, M., et al. (2011). Misplaced NMDA receptors in epileptogenesis contribute to excitotoxicity. Neurobiol. Dis. 43, 507–515. doi:10.1016/j.nbd.2011.04.024
Fredriksson, L., Stevenson, T. K., Su, E. J., Ragsdale, M., Moore, S., Craciun, S., et al. (2015). Identification of a neurovascular signaling pathway regulating seizures in mice. Ann. Clin. Transl. Neurol. 2, 722–738. doi:10.1002/acn3.209
Fritschy, J. M. (2008). Epilepsy, E/I balance and GABA(A) receptor plasticity. Front. Mol. Neurosci. 1, 5. doi:10.3389/neuro.02.005.2008
Gan, M., Jiang, P., Mclean, P., Kanekiyo, T., and Bu, G. (2014). Low-density lipoprotein receptor-related protein 1 (LRP1) regulates the stability and function of GluA1 α-amino-3-hydroxy-5-methyl-4-isoxazole propionic acid (AMPA) receptor in neurons. PLoS ONE 9, e113237. doi:10.1371/journal.pone.0113237
Garcion, E., Halilagic, A., Faissner, A., and Ffrench-Constant, C. (2004). Generation of an environmental niche for neural stem cell development by the extracellular matrix molecule tenascin C. Development 131, 3423–3432. doi:10.1242/dev.01202
Garwood, J., Schnadelbach, O., Clement, A., Schutte, K., Bach, A., and Faissner, A. (1999). DSD-1-proteoglycan is the mouse homolog of phosphacan and displays opposing effects on neurite outgrowth dependent on neuronal lineage. J. Neurosci. 19, 3888–3899. doi:10.1523/JNEUROSCI.19-10-03888.1999
Gaultier, A., Wu, X., le Moan, N., Takimoto, S., Mukandala, G., Akassoglou, K., et al. (2009). Low-density lipoprotein receptor-related protein 1 is an essential receptor for myelin phagocytosis. J. Cell Sci. 122, 1155–1162. doi:10.1242/jcs.040717
Geissler, M., and Faissner, A. (2012). A new indirect co-culture set up of mouse hippocampal neurons and cortical astrocytes on microelectrode arrays. J. Neurosci. Methods 204, 262–272. doi:10.1016/j.jneumeth.2011.11.030
Gladding, C. M., and Raymond, L. A. (2011). Mechanisms underlying NMDA receptor synaptic/extrasynaptic distribution and function. Mol. Cell. Neurosci. 48, 308–320. doi:10.1016/j.mcn.2011.05.001
Goldberg, E. M., and Coulter, D. A. (2013). Mechanisms of epileptogenesis: A convergence on neural circuit dysfunction. Nat. Rev. Neurosci. 14, 337–349. doi:10.1038/nrn3482
Gorski, J. A., Talley, T., Qiu, M., Puelles, L., Rubenstein, J. L. R., and Jones, K. R. (2002). Cortical excitatory neurons and glia, but not GABAergic neurons, are produced in the Emx1-expressing lineage. J. Neurosci. official J. Soc. Neurosci. 22, 6309–6314. doi:10.1523/JNEUROSCI.22-15-06309.2002
Gotthardt, M., Trommsdorff, M., Nevitt, M. F., Shelton, J., Richardson, J. A., Stockinger, W., et al. (2000). Interactions of the low density lipoprotein receptor gene family with cytosolic adaptor and scaffold proteins suggest diverse biological functions in cellular communication and signal transduction. J. Biol. Chem. 275, 25616–25624. doi:10.1074/jbc.M000955200
Gottschling, C., Dzyubenko, E., Geissler, M., and Faissner, A. (2016). The indirect neuron-astrocyte coculture assay: An in vitro set-up for the detailed investigation of neuron-glia interactions. J. Vis. Exp. 2016, 54757. doi:10.3791/54757
Gotz, M., and Huttner, W. B. (2005). The cell biology of neurogenesis. Nat. Rev. Mol. Cell Biol. 6, 777–788. doi:10.1038/nrm1739
Haj-Yasein, N. N., Vindedal, G. F., Eilert-Olsen, M., Gundersen, G. A., Skare, O., Laake, P., et al. (2011). Glial-conditional deletion of aquaporin-4 (Aqp4) reduces blood-brain water uptake and confers barrier function on perivascular astrocyte endfeet. Proc. Natl. Acad. Sci. U. S. A. 108, 17815–17820. doi:10.1073/pnas.1110655108
Hajjar, K. A., Jacovina, A. T., and Chacko, J. (1994). An endothelial cell receptor for plasminogen/tissue plasminogen activator I. Identity with annexin II. J. Biol. Chem. 269, 21191–21197. doi:10.1016/s0021-9258(17)31947-6
Hansen, K. B., Yi, F., Perszyk, R. E., Furukawa, H., Wollmuth, L. P., Gibb, A. J., et al. (2018). Structure, function, and allosteric modulation of NMDA receptors. J. Gen. Physiol. 150, 1081–1105. doi:10.1085/jgp.201812032
Hanson, E., Armbruster, M., Cantu, D., Andresen, L., Taylor, A., Danbolt, N. C., et al. (2015). Astrocytic glutamate uptake is slow and does not limit neuronal NMDA receptor activation in the neonatal neocortex. Glia 63, 1784–1796. doi:10.1002/glia.22844
Hardingham, G. E., and Bading, H. (2010). Synaptic versus extrasynaptic NMDA receptor signalling: Implications for neurodegenerative disorders. Nat. Rev. Neurosci. 11, 682–696. doi:10.1038/nrn2911
Hebert, M., Lesept, F., Vivien, D., and Macrez, R. (2016). The story of an exceptional serine protease, tissue-type plasminogen activator (tPA). Rev. Neurol. Paris. 172, 186–197. doi:10.1016/j.neurol.2015.10.002
Hedou, E., Douceau, S., Chevilley, A., Varangot, A., Thiebaut, A. M., Triniac, H., et al. (2021). Two-chains tissue plasminogen activator unifies met and NMDA receptor signalling to control neuronal survival. Int. J. Mol. Sci. 22, 13483. doi:10.3390/ijms222413483
Hennen, E., Czopka, T., and Faissner, A. (2011). Structurally distinct LewisX glycans distinguish subpopulations of neural stem/progenitor cells. J. Biol. Chem. 286, 16321–16331. doi:10.1074/jbc.M110.201095
Hennen, E., and Faissner, A. (2012). LewisX: A neural stem cell specific glycan? Int. J. Biochem. Cell Biol. 44, 830–833. doi:10.1016/j.biocel.2012.02.019
Hennen, E., Safina, D., Haussmann, U., Worsdorfer, P., Edenhofer, F., Poetsch, A., et al. (2013). A LewisX-glycoprotein screen identifies the low density lipoprotein receptor-related protein 1 (LRP1) as a modulator of oligodendrogenesis in mice. J. Biol. Chem. 288, 16538–16545. doi:10.1074/jbc.M112.419812
Herz, J., and Bock, H. H. (2002). Lipoprotein receptors in the nervous system. Annu. Rev. Biochem. 71, 405–434. doi:10.1146/annurev.biochem.71.110601.135342
Herz, J., Clouthier, D. E., and Hammer, R. E. (1992). LDL receptor-related protein internalizes and degrades uPA-PAI-1 complexes and is essential for embryo implantation. Cell 71, 411–421. doi:10.1016/0092-8674(92)90511-a
Herz, J., Hamann, U., Rogne, S., Myklebost, O., Gausepohl, H., and Stanley, K. K. (1988). Surface location and high affinity for calcium of a 500-kd liver membrane protein closely related to the LDL-receptor suggest a physiological role as lipoprotein receptor. EMBO J. 7, 4119–4127. doi:10.1002/j.1460-2075.1988.tb03306.x
Herz, J., and Strickland, D. K. (2001). Lrp: A multifunctional scavenger and signaling receptor. J. Clin. Invest. 108, 779–784. doi:10.1172/JCI13992
Hoirisch-Clapauch, S., and Nardi, A. E. (2015). Improvement of psychotic symptoms and the role of tissue plasminogen activator. Int. J. Mol. Sci. 16, 27550–27560. doi:10.3390/ijms161126053
Hotz, A. L., Jamali, A., Rieser, N. N., Niklaus, S., Aydin, E., Myren-Svelstad, S., et al. (2022). Loss of glutamate transporter eaat2a leads to aberrant neuronal excitability, recurrent epileptic seizures, and basal hypoactivity. Glia 70, 196–214. doi:10.1002/glia.24106
Huang, C., You, Z., He, Y., Li, J., Liu, Y., Peng, C., et al. (2023). Combined transcriptomics and proteomics forecast analysis for potential biomarker in the acute phase of temporal lobe epilepsy. Front. Neurosci. 17, 1145805. doi:10.3389/fnins.2023.1145805
Huang, W., Zhao, N., Bai, X., Karram, K., Trotter, J., Goebbels, S., et al. (2014). Novel NG2-CreERT2 knock-in mice demonstrate heterogeneous differentiation potential of NG2 glia during development. Glia 62, 896–913. doi:10.1002/glia.22648
Hubbard, J. A., Szu, J. I., Yonan, J. M., and Binder, D. K. (2016). Regulation of astrocyte glutamate transporter-1 (GLT1) and aquaporin-4 (AQP4) expression in a model of epilepsy. Exp. Neurol. 283, 85–96. doi:10.1016/j.expneurol.2016.05.003
Hussain, M. M., Strickland, D. K., and Bakillah, A. (1999). The mammalian low-density lipoprotein receptor family. Annu. Rev. Nutr. 19, 141–172. doi:10.1146/annurev.nutr.19.1.141
Iwasato, T., Datwani, A., Wolf, A. M., Nishiyama, H., Taguchi, Y., Tonegawa, S., et al. (2000). Cortex-restricted disruption of NMDAR1 impairs neuronal patterns in the barrel cortex. Nature 406, 726–731. doi:10.1038/35021059
Jaeger, S., and Pietrzik, C. U. (2008). Functional role of lipoprotein receptors in Alzheimer's disease. Curr. Alzheimer Res. 5, 15–25. doi:10.2174/156720508783884675
Jeanneret, V., Wu, F., Merino, P., Torre, E., Diaz, A., Cheng, L., et al. (2016). Tissue-type plasminogen activator (tPA) modulates the postsynaptic response of cerebral cortical neurons to the presynaptic release of glutamate. Front. Mol. Neurosci. 9, 121. doi:10.3389/fnmol.2016.00121
Jullienne, A., Montagne, A., Orset, C., Lesept, F., Jane, D. E., Monaghan, D. T., et al. (2011). Selective inhibition of GluN2D-containing N-methyl-D-aspartate receptors prevents tissue plasminogen activator-promoted neurotoxicity both in vitro and in vivo. Mol. Neurodegener. 6, 68. doi:10.1186/1750-1326-6-68
Kagitani, H., Tagawa, M., Hatanaka, K., Ikari, T., Saito, A., Bando, H., et al. (1985). Expression in E. coli of finger-domain lacking tissue-type plasminogen activator with high fibrin affinity. FEBS Lett. 189, 145–149. doi:10.1016/0014-5793(85)80860-7
Kandasamy, M., Roll, L., Langenstroth, D., Brustle, O., and Faissner, A. (2017). Glycoconjugates reveal diversity of human neural stem cells (hNSCs) derived from human induced pluripotent stem cells (hiPSCs). Cell Tissue Res. 368, 531–549. doi:10.1007/s00441-017-2594-z
Kanekiyo, T., Cirrito, J. R., Liu, C. C., Shinohara, M., Li, J., Schuler, D. R., et al. (2013). Neuronal clearance of amyloid-beta by endocytic receptor LRP1. J. Neurosci. 33, 19276–19283. doi:10.1523/JNEUROSCI.3487-13.2013
Kaplan, L., Chow, B. W., and Gu, C. (2020). Neuronal regulation of the blood-brain barrier and neurovascular coupling. Nat. Rev. Neurosci. 21, 416–432. doi:10.1038/s41583-020-0322-2
Kjell, J., Fischer-Sternjak, J., Thompson, A. J., Friess, C., Sticco, M. J., Salinas, F., et al. (2020). Defining the adult neural stem cell niche proteome identifies key regulators of adult neurogenesis. Cell Stem Cell 26, 277–293. doi:10.1016/j.stem.2020.01.002
Knopman, D. S., Amieva, H., Petersen, R. C., Chetelat, G., Holtzman, D. M., Hyman, B. T., et al. (2021). Alzheimer disease. Nat. Rev. Dis. Prim. 7, 33. doi:10.1038/s41572-021-00269-y
Kong, Q., Takahashi, K., Schulte, D., Stouffer, N., Lin, Y., and Lin, C. L. (2012). Increased glial glutamate transporter EAAT2 expression reduces epileptogenic processes following pilocarpine-induced status epilepticus. Neurobiol. Dis. 47, 145–154. doi:10.1016/j.nbd.2012.03.032
Kriegstein, A., and Alvarez-Buylla, A. (2009). The glial nature of embryonic and adult neural stem cells. Annu. Rev. Neurosci. 32, 149–184. doi:10.1146/annurev.neuro.051508.135600
Lan, Y. L., Chen, J. J., Hu, G., Xu, J., Xiao, M., and Li, S. (2017). Aquaporin 4 in astrocytes is a target for therapy in alzheimer's disease. Curr. Pharm. Des. 23, 4948–4957. doi:10.2174/1381612823666170714144844
Lariviere, S., Royer, J., Rodriguez-Cruces, R., Paquola, C., Caligiuri, M. E., Gambardella, A., et al. (2022). Structural network alterations in focal and generalized epilepsy assessed in a worldwide ENIGMA study follow axes of epilepsy risk gene expression. Nat. Commun. 13, 4320. doi:10.1038/s41467-022-31730-5
Laruelle, M., Kegeles, L. S., and Abi-Dargham, A. (2003). Glutamate, dopamine, and schizophrenia: From pathophysiology to treatment. Ann. N. Y. Acad. Sci. 1003, 138–158. doi:10.1196/annals.1300.063
Lee, S. H., Ko, H. M., Kwon, K. J., Lee, J., Han, S. H., Han, D. W., et al. (2014). tPA regulates neurite outgrowth by phosphorylation of LRP5/6 in neural progenitor cells. Mol. Neurobiol. 49, 199–215. doi:10.1007/s12035-013-8511-x
Lesept, F., Chevilley, A., Jezequel, J., Ladepeche, L., Macrez, R., Aimable, M., et al. (2016). Tissue-type plasminogen activator controls neuronal death by raising surface dynamics of extrasynaptic NMDA receptors. Cell Death Dis. 7, e2466. doi:10.1038/cddis.2016.279
Leys, D., Hommet, Y., Jacquet, C., Moulin, S., Sibon, I., Mas, J. L., et al. (2016). Proportion of single-chain recombinant tissue plasminogen activator and outcome after stroke. Neurology 87, 2416–2426. doi:10.1212/WNL.0000000000003399
Li, X., Newbern, J. M., Wu, Y., Morgan-Smith, M., Zhong, J., Charron, J., et al. (2012). MEK is a key regulator of gliogenesis in the developing brain. Neuron 75, 1035–1050. doi:10.1016/j.neuron.2012.08.031
Li, Z., Sun, W., Duan, W., Jiang, Y., Chen, M., Lin, G., et al. (2023). Guiding epilepsy surgery with an LRP1-targeted SPECT/SERRS dual-mode imaging probe. ACS Appl. Mater Interfaces 15, 14–25. doi:10.1021/acsami.2c02540
Lillis, A. P., van Duyn, L. B., Murphy-Ullrich, J. E., and Strickland, D. K. (2008). LDL receptor-related protein 1: Unique tissue-specific functions revealed by selective gene knockout studies. Physiol. Rev. 88, 887–918. doi:10.1152/physrev.00033.2007
Lin, J. P., Mironova, Y. A., Shrager, P., and Giger, R. J. (2017). LRP1 regulates peroxisome biogenesis and cholesterol homeostasis in oligodendrocytes and is required for proper CNS myelin development and repair. Elife 6, e30498. doi:10.7554/eLife.30498
Liu, C. C., Hu, J., Zhao, N., Wang, J., Wang, N., Cirrito, J. R., et al. (2017b). Astrocytic LRP1 mediates brain Aβ clearance and impacts amyloid deposition. J. Neurosci. 37, 4023–4031. doi:10.1523/JNEUROSCI.3442-16.2017
Liu, C. C., Teschemacher, A. G., and Kasparov, S. (2017a). Neuroprotective potential of astroglia. J. Neurosci. Res. 95, 2126–2139. doi:10.1002/jnr.24140
Liu, Q., Trotter, J., Zhang, J., Peters, M. M., Cheng, H., Bao, J., et al. (2010). Neuronal LRP1 knockout in adult mice leads to impaired brain lipid metabolism and progressive, age-dependent synapse loss and neurodegeneration. J. Neurosci. Official J. Soc. Neurosci. 30, 17068–17078. doi:10.1523/JNEUROSCI.4067-10.2010
Liu, X. B., Murray, K. D., and Jones, E. G. (2004). Switching of NMDA receptor 2A and 2B subunits at thalamic and cortical synapses during early postnatal development. J. Neurosci. 24, 8885–8895. doi:10.1523/JNEUROSCI.2476-04.2004
Louessard, M., Lacroix, A., Martineau, M., Mondielli, G., Montagne, A., Lesept, F., et al. (2016). Tissue plasminogen activator expression is restricted to subsets of excitatory pyramidal glutamatergic neurons. Mol. Neurobiol. 53, 5000–5012. doi:10.1007/s12035-015-9432-7
Loukinova, E., Ranganathan, S., Kuznetsov, S., Gorlatova, N., Migliorini, M. M., Loukinov, D., et al. (2002). Platelet-derived growth factor (PDGF)-induced tyrosine phosphorylation of the low density lipoprotein receptor-related protein (LRP). Evidence for integrated co-receptor function betwenn LRP and the PDGF. J. Biol. Chem. 277, 15499–15506. doi:10.1074/jbc.M200427200
Ma, Q., Zhao, Z., Sagare, A. P., Wu, Y., Wang, M., Owens, N. C., et al. (2018). Blood-brain barrier-associated pericytes internalize and clear aggregated amyloid-β42 by LRP1-dependent apolipoprotein E isoform-specific mechanism. Mol. Neurodegener. 13, 57. doi:10.1186/s13024-018-0286-0
Madani, R., Hulo, S., Toni, N., Madani, H., Steimer, T., Muller, D., et al. (1999). Enhanced hippocampal long-term potentiation and learning by increased neuronal expression of tissue-type plasminogen activator in transgenic mice. EMBO J. 18, 3007–3012. doi:10.1093/emboj/18.11.3007
Madisen, L., Zwingman, T. A., Sunkin, S. M., Oh, S. W., Zariwala, H. A., Gu, H., et al. (2010). A robust and high-throughput Cre reporting and characterization system for the whole mouse brain. Nat. Neurosci. 13, 133–140. doi:10.1038/nn.2467
Maier, W., Bednorz, M., Meister, S., Roebroek, A., Weggen, S., Schmitt, U., et al. (2013). LRP1 is critical for the surface distribution and internalization of the NR2B NMDA receptor subtype. Mol. Neurodegener. 8, 25. doi:10.1186/1750-1326-8-25
Makarova, A., Mikhailenko, I., Bugge, T. H., List, K., Lawrence, D. A., and Strickland, D. K. (2003). The low density lipoprotein receptor-related protein modulates protease activity in the brain by mediating the cellular internalization of both neuroserpin and neuroserpin-tissue-type plasminogen activator complexes. J. Biol. Chem. 278, 50250–50258. doi:10.1074/jbc.M309150200
Malatesta, P., and Gotz, M. (2013). Radial glia - from boring cables to stem cell stars. Development 140, 483–486. doi:10.1242/dev.085852
Mantuano, E., Lam, M. S., and Gonias, S. L. (2013). LRP1 assembles unique co-receptor systems to initiate cell signaling in response to tissue-type plasminogen activator and myelin-associated glycoprotein. J. Biol. Chem. 288, 34009–34018. doi:10.1074/jbc.M113.509133
Martin, A. M., Kuhlmann, C., Trossbach, S., Jaeger, S., Waldron, E., Roebroek, A., et al. (2008). The functional role of the second NPXY motif of the LRP1 beta-chain in tissue-type plasminogen activator-mediated activation of N-methyl-D-aspartate receptors. J. Biol. Chem. 283, 12004–12013. doi:10.1074/jbc.M707607200
Mathern, G. W., Pretorius, J. K., Leite, J. P., Kornblum, H. I., Mendoza, D., Lozada, A., et al. (1998). Hippocampal AMPA and NMDA mRNA levels and subunit immunoreactivity in human temporal lobe epilepsy patients and a rodent model of chronic mesial limbic epilepsy. Epilepsy Res. 32, 154–171. doi:10.1016/s0920-1211(98)00048-5
May, M., Denecke, B., Schroeder, T., Gotz, M., and Faissner, A. (2018). Cell tracking in vitro reveals that the extracellular matrix glycoprotein Tenascin-C modulates cell cycle length and differentiation in neural stem/progenitor cells of the developing mouse spinal cord. Biol. Open 7, bio027730. doi:10.1242/bio.027730
May, P., Reddy, Y. K., and Herz, J. (2002). Proteolytic processing of low density lipoprotein receptor-related protein mediates regulated release of its intracellular domain. J. Biol. Chem. 277, 18736–18743. doi:10.1074/jbc.M201979200
May, P., Rohlmann, A., Bock, H. H., Zurhove, K., Marth, J. D., Schomburg, E. D., et al. (2004). Neuronal LRP1 functionally associates with postsynaptic proteins and is required for normal motor function in mice. Mol. Cell. Biol. 24, 8872–8883. doi:10.1128/MCB.24.20.8872-8883.2004
May, P. (2013). The low-density lipoprotein receptor-related protein 1 in inflammation. Curr. Opin. Lipidol. 24, 134–137. doi:10.1097/MOL.0b013e32835e809c
Molofsky, A. V., Kelley, K. W., Tsai, H. H., Redmond, S. A., Chang, S. M., Madireddy, L., et al. (2014). Astrocyte-encoded positional cues maintain sensorimotor circuit integrity. Nature 509, 189–194. doi:10.1038/nature13161
Molofsky, A. V., Krencik, R., Ullian, E. M., Tsai, H. H., Deneen, B., Richardson, W. D., et al. (2012). Astrocytes and disease: A neurodevelopmental perspective. Genes Dev. 26, 891–907. doi:10.1101/gad.188326.112
Mori, T., Tanaka, K., Buffo, A., Wurst, W., Kuhn, R., and Gotz, M. (2006). Inducible gene deletion in astroglia and radial glia--a valuable tool for functional and lineage analysis. Glia 54, 21–34. doi:10.1002/glia.20350
Nakajima, C., Haffner, P., Goerke, S. M., Zurhove, K., Adelmann, G., Frotscher, M., et al. (2014). The lipoprotein receptor LRP1 modulates sphingosine-1-phosphate signaling and is essential for vascular development. Development 141, 4513–4525. doi:10.1242/dev.109124
Nakajima, C., Kulik, A., Frotscher, M., Herz, J., Schafer, M., Bock, H. H., et al. (2013). Low density lipoprotein receptor-related protein 1 (LRP1) modulates N-methyl-D-aspartate (NMDA) receptor-dependent intracellular signaling and NMDA-induced regulation of postsynaptic protein complexes. J. Biol. Chem. 288, 21909–21923. doi:10.1074/jbc.M112.444364
Nave, K. A., and Werner, H. B. (2021). Ensheathment and myelination of axons: Evolution of glial functions. Annu. Rev. Neurosci. 44, 197–219. doi:10.1146/annurev-neuro-100120-122621
Nave, K. A., and Werner, H. B. (2014). Myelination of the nervous system: Mechanisms and functions. Annu. Rev. Cell Dev. Biol. 30, 503–533. doi:10.1146/annurev-cellbio-100913-013101
Ng, K. S., Leung, H. W., Wong, P. T., and Low, C. M. (2012). Cleavage of the NR2B subunit amino terminus of N-methyl-D-aspartate (NMDA) receptor by tissue plasminogen activator: Identification of the cleavage site and characterization of ifenprodil and glycine affinities on truncated NMDA receptor. J. Biol. Chem. 287, 25520–25529. doi:10.1074/jbc.M112.374397
Nicole, O., Docagne, F., Ali, C., Margaill, I., Carmeliet, P., Mackenzie, E. T., et al. (2001). The proteolytic activity of tissue-plasminogen activator enhances NMDA receptor-mediated signaling. Nat. Med. 7, 59–64. doi:10.1038/83358
Niego, B., Freeman, R., Puschmann, T. B., Turnley, A. M., and Medcalf, R. L. (2012). t-PA-specific modulation of a human blood-brain barrier model involves plasmin-mediated activation of the Rho kinase pathway in astrocytes. Blood 119, 4752–4761. doi:10.1182/blood-2011-07-369512
Nikolakopoulou, A. M., Wang, Y., Ma, Q., Sagare, A. P., Montagne, A., Huuskonen, M. T., et al. (2021). Endothelial LRP1 protects against neurodegeneration by blocking cyclophilin A. J. Exp. Med. 218, e20202207. doi:10.1084/jem.20202207
Nolden, L., Edenhofer, F., Haupt, S., Koch, P., Wunderlich, F. T., Siemen, H., et al. (2006). Site-specific recombination in human embryonic stem cells induced by cell-permeant Cre recombinase. Nat. Methods 3, 461–467. doi:10.1038/nmeth884
Oue, H., Yamazaki, Y., Qiao, W., Yuanxin, C., Ren, Y., Kurti, A., et al. (2023). LRP1 in vascular mural cells modulates cerebrovascular integrity and function in the presence of APOE4. JCI Insight 8, e163822. doi:10.1172/jci.insight.163822
Pai, B., Tome-Garcia, J., Cheng, W. S., Nudelman, G., Beaumont, K. G., Ghatan, S., et al. (2022). High-resolution transcriptomics informs glial pathology in human temporal lobe epilepsy. Acta Neuropathol. Commun. 10, 149. doi:10.1186/s40478-022-01453-1
Pal, B. (2018). Involvement of extrasynaptic glutamate in physiological and pathophysiological changes of neuronal excitability. Cell Mol. Life Sci. 75, 2917–2949. doi:10.1007/s00018-018-2837-5
Parcq, J., Bertrand, T., Montagne, A., Baron, A. F., Macrez, R., Billard, J. M., et al. (2012). Unveiling an exceptional zymogen: The single-chain form of tPA is a selective activator of NMDA receptor-dependent signaling and neurotoxicity. Cell Death Differ. 19, 1983–1991. doi:10.1038/cdd.2012.86
Parsons, M. P., and Raymond, L. A. (2014). Extrasynaptic NMDA receptor involvement in central nervous system disorders. Neuron 82, 279–293. doi:10.1016/j.neuron.2014.03.030
Pasquet, N., Douceau, S., Naveau, M., Lesept, F., Louessard, M., Lebouvier, L., et al. (2019). Tissue-type plasminogen activator controlled corticogenesis through a mechanism dependent of NMDA receptors expressed on radial glial cells. Cereb. Cortex 29, 2482–2498. doi:10.1093/cercor/bhy119
Patel, D. C., Tewari, B. P., Chaunsali, L., and Sontheimer, H. (2019). Neuron-glia interactions in the pathophysiology of epilepsy. Nat. Rev. Neurosci. 20, 282–297. doi:10.1038/s41583-019-0126-4
Perea, G., Navarrete, M., and Araque, A. (2009). Tripartite synapses: Astrocytes process and control synaptic information. Trends Neurosci. 32, 421–431. doi:10.1016/j.tins.2009.05.001
Petr, G. T., Sun, Y., Frederick, N. M., Zhou, Y., Dhamne, S. C., Hameed, M. Q., et al. (2015). Conditional deletion of the glutamate transporter GLT-1 reveals that astrocytic GLT-1 protects against fatal epilepsy while neuronal GLT-1 contributes significantly to glutamate uptake into synaptosomes. J. Neurosci. 35, 5187–5201. doi:10.1523/JNEUROSCI.4255-14.2015
Petrushanko, I. Y., Mitkevich, V. A., and Makarov, A. A. (2023). Effect of beta-amyloid on blood-brain barrier properties and function. Biophys. Rev. 15, 183–197. doi:10.1007/s12551-023-01052-x
Polavarapu, R., Gongora, M. C., Yi, H., Ranganthan, S., Lawrence, D. A., Strickland, D., et al. (2007). Tissue-type plasminogen activator-mediated shedding of astrocytic low-density lipoprotein receptor-related protein increases the permeability of the neurovascular unit. Blood 109, 3270–3278. doi:10.1182/blood-2006-08-043125
Purushotham, M., Tashrifwala, F., Jena, R., Vudugula, S. A., Patil, R. S., and Agrawal, A. (2022). The association between alzheimer's disease and epilepsy: A narrative review. Cureus 14, e30195. doi:10.7759/cureus.30195
Qiu, Z., Hyman, B. T., and Rebeck, G. W. (2004). Apolipoprotein E receptors mediate neurite outgrowth through activation of p44/42 mitogen-activated protein kinase in primary neurons. J. Biol. Chem. 279, 34948–34956. doi:10.1074/jbc.M401055200
Qiu, Z., Strickland, D. K., Hyman, B. T., and Rebeck, G. W. (2002). Alpha 2-Macroglobulin exposure reduces calcium responses to N-methyl-D-aspartate via low density lipoprotein receptor-related protein in cultured hippocampal neurons. J. Biol. Chem. 277, 14458–14466. doi:10.1074/jbc.M112066200
Rauch, J. N., Luna, G., Guzman, E., Audouard, M., Challis, C., Sibih, Y. E., et al. (2020). LRP1 is a master regulator of tau uptake and spread. Nature 580, 381–385. doi:10.1038/s41586-020-2156-5
Reekmans, S. M., Pflanzner, T., Gordts, P. L. S. M., Isbert, S., Zimmermann, P., Annaert, W., et al. (2009). Inactivation of the proximal NPXY motif impairs early steps in LRP1 biosynthesis. Cell. Mol. Life Sci. 67, 135–145. doi:10.1007/s00018-009-0171-7
Rigau, V., Morin, M., Rousset, M. C., de Bock, F., Lebrun, A., Coubes, P., et al. (2007). Angiogenesis is associated with blood-brain barrier permeability in temporal lobe epilepsy. Brain 130, 1942–1956. doi:10.1093/brain/awm118
Righes Marafiga, J., Vendramin Pasquetti, M., and Calcagnotto, M. E. (2021). GABAergic interneurons in epilepsy: More than a simple change in inhibition. Epilepsy Behav. 121, 106935. doi:10.1016/j.yebeh.2020.106935
Robinson, M. B., and Jackson, J. G. (2016). Astroglial glutamate transporters coordinate excitatory signaling and brain energetics. Neurochem. Int. 98, 56–71. doi:10.1016/j.neuint.2016.03.014
Roebroek, A. J. M., Reekmans, S., Lauwers, A., Feyaerts, N., Smeijers, L., and Hartmann, D. (2006). Mutant Lrp1 knock-in mice generated by recombinase-mediated cassette exchange reveal differential importance of the NPXY motifs in the intracellular domain of LRP1 for normal fetal development. Mol. Cell. Biol. 26, 605–616. doi:10.1128/MCB.26.2.605-616.2006
Rohlmann, A., Gotthardt, M., Willnow, T. E., Hammer, R. E., and Herz, J. (1996). Sustained somatic gene inactivation by viral transfer of Cre recombinase. Nat. Biotechnol. 14, 1562–1565. doi:10.1038/nbt1196-1562
Roll, L., Lessmann, K., Brustle, O., and Faissner, A. (2022). Cerebral organoids maintain the expression of neural stem cell-associated glycoepitopes and extracellular matrix. Cells 11, 760. doi:10.3390/cells11050760
Romeo, R., Boden-El Mourabit, D., Scheller, A., Mark, M. D., and Faissner, A. (2021). Low-density lipoprotein receptor-related protein 1 (LRP1) as a novel regulator of early astroglial differentiation. Front. Cell Neurosci. 15, 642521. doi:10.3389/fncel.2021.642521
Romeo, R., Glotzbach, K., Scheller, A., and Faissner, A. (2020). Deletion of LRP1 from astrocytes modifies neuronal network activity in an in vitro model of the tripartite synapse. Front. Cell Neurosci. 14, 567253. doi:10.3389/fncel.2020.567253
Rowitch, D. H., and Kriegstein, A. R. (2010). Developmental genetics of vertebrate glial-cell specification. Nature 468, 214–222. doi:10.1038/nature09611
Safina, D., Schlitt, F., Romeo, R., Pflanzner, T., Pietrzik, C. U., Narayanaswami, V., et al. (2016). Low-density lipoprotein receptor-related protein 1 is a novel modulator of radial glia stem cell proliferation, survival, and differentiation. Glia 64, 1363–1380. doi:10.1002/glia.23009
Sagare, A. P., Deane, R., and Zlokovic, B. V. (2012). Low-density lipoprotein receptor-related protein 1: A physiological Aβ homeostatic mechanism with multiple therapeutic opportunities. Pharmacol. Ther. 136, 94–105. doi:10.1016/j.pharmthera.2012.07.008
Saher, G., Brugger, B., Lappe-Siefke, C., Mobius, W., Tozawa, R., Wehr, M. C., et al. (2005). High cholesterol level is essential for myelin membrane growth. Nat. Neurosci. 8, 468–475. doi:10.1038/nn1426
Samson, A. L., Nevin, S. T., Croucher, D., Niego, B., Daniel, P. B., Weiss, T. W., et al. (2008). Tissue-type plasminogen activator requires a co-receptor to enhance NMDA receptor function. J. Neurochem. 107, 1091–1101. doi:10.1111/j.1471-4159.2008.05687.x
Schaberg, E., Gotz, M., and Faissner, A. (2022). The extracellular matrix molecule tenascin-C modulates cell cycle progression and motility of adult neural stem/progenitor cells from the subependymal zone. Cell Mol. Life Sci. 79, 244. doi:10.1007/s00018-022-04259-5
Schafer, I., Kaisler, J., Scheller, A., Kirchhoff, F., Haghikia, A., and Faissner, A. (2019). Conditional deletion of LRP1 leads to progressive loss of recombined NG2-expressing oligodendrocyte precursor cells in a novel mouse model. Cells 8, 1550. doi:10.3390/cells8121550
Seeds, N. W., Basham, M. E., and Haffke, S. P. (1999). Neuronal migration is retarded in mice lacking the tissue plasminogen activator gene. Proc. Natl. Acad. Sci. U. S. A. 96, 14118–14123. doi:10.1073/pnas.96.24.14118
Seifert, G., Schilling, K., and Steinhauser, C. (2006). Astrocyte dysfunction in neurological disorders: A molecular perspective. Nat. Rev. Neurosci. 7, 194–206. doi:10.1038/nrn1870
Sharp, F. R., Decarli, C. S., Jin, L. W., and Zhan, X. (2023). White matter injury, cholesterol dysmetabolism, and APP/abeta dysmetabolism interact to produce alzheimer's disease (ad) neuropathology: A hypothesis and review. Front. Aging Neurosci. 15, 1096206. doi:10.3389/fnagi.2023.1096206
Shinohara, M., Tachibana, M., Kanekiyo, T., and Bu, G. (2017). Role of LRP1 in the pathogenesis of alzheimer's disease: Evidence from clinical and preclinical studies. J. Lipid Res. 58, 1267–1281. doi:10.1194/jlr.R075796
Sizova, O., John, L. S., Ma, Q., and Molldrem, J. J. (2023). Multi-faceted role of LRP1 in the immune system. Front. Immunol. 14, 1166189. doi:10.3389/fimmu.2023.1166189
Sousa, J. A., Bernardes, C., Bernardo-Castro, S., Lino, M., Albino, I., Ferreira, L., et al. (2023). Reconsidering the role of blood-brain barrier in Alzheimer's disease: From delivery to target. Front. Aging Neurosci. 15, 1102809. doi:10.3389/fnagi.2023.1102809
Stafstrom, C. E. (2006). Epilepsy: A review of selected clinical syndromes and advances in basic science. J. Cereb. Blood Flow. Metab. 26, 983–1004. doi:10.1038/sj.jcbfm.9600265
Stefanitsch, C., Lawrence, A. L., Olverling, A., Nilsson, I., and Fredriksson, L. (2015). tPA deficiency in mice leads to rearrangement in the cerebrovascular tree and cerebroventricular malformations. Front. Cell Neurosci. 9, 456. doi:10.3389/fncel.2015.00456
Steffens, M., Huppertz, H. J., Zentner, J., Chauzit, E., and Feuerstein, T. J. (2005). Unchanged glutamine synthetase activity and increased NMDA receptor density in epileptic human neocortex: Implications for the pathophysiology of epilepsy. Neurochem. Int. 47, 379–384. doi:10.1016/j.neuint.2005.06.001
Steinhäuser, C., and Seifert, G. (2012). “Astrocyte dysfunction in epilepsy,” in Jasper's basic mechanisms of the epilepsies. Editors J. L. NOEBELS, M. AVOLI, M. A. ROGAWSKI, R. W. OLSEN, and A. V. DELGADO-ESCUETA 4th ed. (Bethesda (MD): National Center for Biotechnology Information).
Stevenson, T. K., and Lawrence, D. A. (2018). Characterization of tissue plasminogen activator expression and trafficking in the adult murine brain. eNeuro 5, 0119. doi:10.1523/ENEURO.0119-18.2018
Stevenson, T. K., Moore, S. J., Murphy, G. G., and Lawrence, D. A. (2022). Tissue plasminogen activator in central nervous system Physiology and pathology: From synaptic plasticity to alzheimer's disease. Semin. Thromb. Hemost. 48, 288–300. doi:10.1055/s-0041-1740265
Stiles, T. L., Dickendesher, T. L., Gaultier, A., Fernandez-Castaneda, A., Mantuano, E., Giger, R. J., et al. (2013). LDL receptor-related protein-1 is a sialic-acid-independent receptor for myelin-associated glycoprotein that functions in neurite outgrowth inhibition by MAG and CNS myelin. J. Cell Sci. 126, 209–220. doi:10.1242/jcs.113191
Storck, S. E., Hartz, A. M. S., and Pietrzik, C. U. (2021a). The blood-brain barrier in alzheimer's disease. Handb. Exp. Pharmacol. 2021, 1–10.
Storck, S. E., Kurtyka, M., and Pietrzik, C. U. (2021b). Brain endothelial LRP1 maintains blood-brain barrier integrity. Fluids Barriers CNS 18, 27. doi:10.1186/s12987-021-00260-5
Storck, S. E., Meister, S., Nahrath, J., Meissner, J. N., Schubert, N., Di Spiezio, A., et al. (2016). Endothelial LRP1 transports amyloid-β(1-42) across the blood-brain barrier. J. Clin. Invest. 126, 123–136. doi:10.1172/JCI81108
Storck, S. E., and Pietrzik, C. U. (2017). Endothelial LRP1 - a potential target for the treatment of alzheimer's disease: Theme: Drug discovery, development and delivery in alzheimer's disease guest editor: Davide brambilla. Pharm. Res. 34, 2637–2651. doi:10.1007/s11095-017-2267-3
Su, E. J., Cao, C., Fredriksson, L., Nilsson, I., Stefanitsch, C., Stevenson, T. K., et al. (2017). Microglial-mediated PDGF-CC activation increases cerebrovascular permeability during ischemic stroke. Acta Neuropathol. 134, 585–604. doi:10.1007/s00401-017-1749-z
Sun, Q. J., Duan, R. S., Wang, A. H., Shang, W., Zhang, T., Zhang, X. Q., et al. (2009). Alterations of NR2B and PSD-95 expression in hippocampus of kainic acid-exposed rats with behavioural deficits. Behav. Brain Res. 201, 292–299. doi:10.1016/j.bbr.2009.02.027
Sweeney, M. D., Ayyadurai, S., and Zlokovic, B. V. (2016). Pericytes of the neurovascular unit: Key functions and signaling pathways. Nat. Neurosci. 19, 771–783. doi:10.1038/nn.4288
Szczurowska, E., and Mareš, P. (2013). NMDA and AMPA receptors: Development and status epilepticus. Physiological Res./ Acad. Sci. Bohemoslovaca 62 (1), S21–S38. doi:10.33549/physiolres.932662
Tachibana, M., Holm, M. L., Liu, C. C., Shinohara, M., Aikawa, T., Oue, H., et al. (2019). APOE4-mediated amyloid-beta pathology depends on its neuronal receptor LRP1. J. Clin. Invest. 129, 1272–1277. doi:10.1172/JCI124853
Tan, M. L., Ng, A., Pandher, P. S., Sashindranath, M., Hamilton, J. A., Davis, S. M., et al. (2012). Tissue plasminogen activator does not alter development of acquired epilepsy. Epilepsia 53, 1998–2004. doi:10.1111/j.1528-1167.2012.03635.x
Taverna, E., Gotz, M., and Huttner, W. B. (2014). The cell biology of neurogenesis: Toward an understanding of the development and evolution of the neocortex. Annu. Rev. Cell Dev. Biol. 30, 465–502. doi:10.1146/annurev-cellbio-101011-155801
Tcw, J., Qian, L., Pipalia, N. H., Chao, M. J., Liang, S. A., Shi, Y., et al. (2022). Cholesterol and matrisome pathways dysregulated in astrocytes and microglia. Cell 185, 2213–2233 e25. doi:10.1016/j.cell.2022.05.017
Torrico, B., Shaw, A. D., Mosca, R., Vivo-Luque, N., Hervas, A., Fernandez-Castillo, N., et al. (2019). Truncating variant burden in high-functioning autism and pleiotropic effects of LRP1 across psychiatric phenotypes. J. Psychiatry Neurosci. 44, 350–359. doi:10.1503/jpn.180184
Traynelis, S. F., Wollmuth, L. P., Mcbain, C. J., Menniti, F. S., Vance, K. M., Ogden, K. K., et al. (2010). Glutamate receptor ion channels: Structure, regulation, and function. Pharmacol. Rev. 62, 405–496. doi:10.1124/pr.109.002451
van Gool, B., Storck, S. E., Reekmans, S. M., Lechat, B., Gordts, P., Pradier, L., et al. (2019). LRP1 has a predominant role in production over clearance of Aβ in a mouse model of alzheimer's disease. Mol. Neurobiol. 56, 7234–7245. doi:10.1007/s12035-019-1594-2
van Vliet, E. A., and Marchi, N. (2022). Neurovascular unit dysfunction as a mechanism of seizures and epilepsy during aging. Epilepsia 63, 1297–1313. doi:10.1111/epi.17210
Varangot, A., Lebatard, S., Bellemain-Sagnard, M., Lebouvier, L., Hommet, Y., and Vivien, D. (2023). Modulations of the neuronal trafficking of tissue-type plasminogen activator (tPA) influences glutamate release. Cell Death Dis. 14, 34. doi:10.1038/s41419-022-05543-9
Verkhratsky, A., and Nedergaard, M. (2018). Physiology of astroglia. Physiol. Rev. 98, 239–389. doi:10.1152/physrev.00042.2016
Verkman, A. S., Binder, D. K., Bloch, O., Auguste, K., and Papadopoulos, M. C. (2006). Three distinct roles of aquaporin-4 in brain function revealed by knockout mice. Biochim. Biophys. Acta 1758, 1085–1093. doi:10.1016/j.bbamem.2006.02.018
Vezzani, A., Ravizza, T., Bedner, P., Aronica, E., Steinhauser, C., and Boison, D. (2022). Astrocytes in the initiation and progression of epilepsy. Nat. Rev. Neurol. 18, 707–722. doi:10.1038/s41582-022-00727-5
Wang, C., Zhang, J., Song, S., Li, Z., Yin, S., Duan, W., et al. (2021). Imaging epileptic foci in mouse models via a low-density lipoprotein receptor-related protein-1 targeting strategy. EBioMedicine 63, 103156. doi:10.1016/j.ebiom.2020.103156
Wiera, G., and Mozrzymas, J. W. (2015). Extracellular proteolysis in structural and functional plasticity of mossy fiber synapses in hippocampus. Front. Cell Neurosci. 9, 427. doi:10.3389/fncel.2015.00427
Wu, F., Torre, E., Cuellar-Giraldo, D., Cheng, L., Yi, H., Bichler, E. K., et al. (2015). Tissue-type plasminogen activator triggers the synaptic vesicle cycle in cerebral cortical neurons. J. Cereb. Blood Flow. Metab. 35, 1966–1976. doi:10.1038/jcbfm.2015.155
Wu, Y., Chen, C., Yang, Q., Jiao, M., and Qiu, S. (2017). Endocytosis of GluN2B-containing NMDA receptors mediates NMDA-induced excitotoxicity. Mol. Pain 13, 1744806917701921. doi:10.1177/1744806917701921
Wyneken, U., Marengo, J. J., Villanueva, S., Soto, D., Sandoval, R., Gundelfinger, E. D., et al. (2003). Epilepsy-induced changes in signaling systems of human and rat postsynaptic densities. Epilepsia 44, 243–246. doi:10.1046/j.1528-1157.2003.17602.x
Yang, F., Chen, L., Yu, Y., Xu, T., Chen, L., Yang, W., et al. (2022). Alzheimer's disease and epilepsy: An increasingly recognized comorbidity. Front. Aging Neurosci. 14, 940515. doi:10.3389/fnagi.2022.940515
Yepes, M. (2016). Tissue-type plasminogen activator is a modulator of the synaptic vesicle cycle. Neural Regen. Res. 11, 212–213. doi:10.4103/1673-5374.177712
Zhang, J., Cao, R., Zhang, Y., Jia, T., Cao, Y., and Wahlberg, E. (2009). Differential roles of PDGFR-alpha and PDGFR-beta in angiogenesis and vessel stability. FASEB J. 23, 153–163. doi:10.1096/fj.08-113860
Zhang, Y., and Barres, B. A. (2010). Astrocyte heterogeneity: An underappreciated topic in neurobiology. Curr. Opin. Neurobiol. 20, 588–594. doi:10.1016/j.conb.2010.06.005
Zhang, Y., Chen, K., Sloan, S. A., Bennett, M. L., Scholze, A. R., O'Keeffe, S., et al. (2014). An RNA-sequencing transcriptome and splicing database of glia, neurons, and vascular cells of the cerebral cortex. J. Neurosci. 34, 11929–11947. doi:10.1523/JNEUROSCI.1860-14.2014
Zhu, S., Stroebel, D., Yao, C. A., Taly, A., and Paoletti, P. (2013). Allosteric signaling and dynamics of the clamshell-like NMDA receptor GluN1 N-terminal domain. Nat. Struct. Mol. Biol. 20, 477–485. doi:10.1038/nsmb.2522
Zhu, X., Bergles, D. E., and Nishiyama, A. (2008). NG2 cells generate both oligodendrocytes and gray matter astrocytes. Development 135, 145–157. doi:10.1242/dev.004895
Zhuo, M., Holtzman, D. M., Li, Y., Osaka, H., Demaro, J., Jacquin, M., et al. (2000). Role of tissue plasminogen activator receptor LRP in hippocampal long-term potentiation. J. Neurosci. 20, 542–549. doi:10.1523/JNEUROSCI.20-02-00542.2000
Keywords: astrocyte, Emx1, epilepsy, low-density lipoprotein receptor-related protein-1 (LRP1), NG2 (CSPG4) chondroitin sulfate proteoglycan, radial glia, tissue plasminogen activator (tPA), (GLAST) glutamate aspartate transporter
Citation: Faissner A (2023) Low-density lipoprotein receptor-related protein-1 (LRP1) in the glial lineage modulates neuronal excitability. Front. Netw. Physiol. 3:1190240. doi: 10.3389/fnetp.2023.1190240
Received: 20 March 2023; Accepted: 25 May 2023;
Published: 13 June 2023.
Edited by:
Alexei Verkhratsky, The University of Manchester, United KingdomReviewed by:
Steffen E. Storck, Washington University in St. Louis, United StatesWayne W. Poon, University of California, Irvine, United States
Amal Kaddoumi, Auburn University, United States
Copyright © 2023 Faissner. This is an open-access article distributed under the terms of the Creative Commons Attribution License (CC BY). The use, distribution or reproduction in other forums is permitted, provided the original author(s) and the copyright owner(s) are credited and that the original publication in this journal is cited, in accordance with accepted academic practice. No use, distribution or reproduction is permitted which does not comply with these terms.
*Correspondence: Andreas Faissner, YW5kcmVhcy5mYWlzc25lckBydWIuZGU=