- 1Renal Research Unit, Department of Clinical Research and Epidemiology, University of Würzburg, Würzburg, Germany
- 2Clinical Trial Service and Epidemiology Studies Unit, Nuffield Department of Population Health, University of Oxford, Oxford, United Kingdom
- 3Nephrology Section, Department of Internal Medicine and Pediatrics, University Hospital, Ghent, Belgium
- 4European Kidney Health Alliance, Brussels, Belgium
- 5Department of Nephrology and Hypertension, IIS-Fundacion Jimenez Diaz UAM, Madrid, Spain
- 6Departamento de Medicina, Facultad de Medicina, Universidad Autónoma de Madrid, Madrid, Spain
- 7UCL Department of Nephrology, Royal Free Hospital, University College London, London, United Kingdom
- 8School of Medicine, University of Montpellier, Montpellier, France
- 9Department of Nephrology and Hypertension, University Medical Centre Utrecht, Utrecht University, Utrecht, Netherlands
- 10Division of Pharmacology, Utrecht Institute for Pharmaceutical Sciences, Utrecht University, Utrecht, Netherlands
- 11Division of Nephrology, Department of Internal Medicine, Maastricht University Medical Centre+, Maastricht, Netherlands
- 12Department of Internal Medicine, NUTRIM School of Nutrition and Translational Research in Metabolism, Maastricht University, Maastricht, Netherlands
- 13Department of Nephrology, Dialysis and Renal Transplantation, Fondazione IRCCS Ca’ Granda Ospedale Maggiore Policlinico, Milan, Italy
- 14Department of Clinical Sciences and Community Health, University of Milan, Milan, Italy
- 15Advanced Organ Bioengineering and Therapeutics-Technical Medical Centre, University of Twente, Enschede, Netherlands
- 16Department of Nephrology, Radboud University Medical Center, Radboud Institute for Molecular Life Sciences, Nijmegen, Netherlands
- 17Manchester Academy of Health Sciences Centre, Manchester University Hospitals, University of Manchester, Manchester, United Kingdom
- 18Department of Nephrology, Amsterdam UMC, location Vrije Universiteit Amsterdam, Nephrology, Amsterdam, Netherlands
- 19Department of Nephrology, Amsterdam Cardiovascular Sciences, Diabetes & Metabolism, Amsterdam, Netherlands
- 20Department for Biomedical Research, University for Continuing Education Krems, Krem, Austria
- 21Medical Department III - Endocrinology, Nephrology, Rheumatology, University of Leipzig Medical Center, Leipzig, Germany
- 22Division of Biomedical Engineering, Department of Chemical and Biological Engineering, University of Saskatchewan, Saskatoon, SK, Canada
- 23Fresenius SE & Co. KGaA, Bad Homburg, Germany
- 24Division of Renal Medicine, Department of Clinical Science, Intervention and Technology, Karolinska Institutet, Stockholm, Sweden
Purpose of symposium: From September 6 – 8 2022, the Life/2022 Membrane Symposium was held in Frankfurt, Germany, and transmitted live to a worldwide internet audience. The event was part of the Life/Nephrology Campus initiative, a continuous educational platform for the nephrology community to expand knowledge and share expertise on contemporary topics in chronic kidney disease. We describe recent questions and advances in the field, and we underline challenges in the care of dialysis patients and opportunities for integration of new findings into clinical practice to improve patient outcomes in end stage kidney disease patients.
Topics: Most patients with kidney failure are on maintenance hemodialysis (MHD). The scientific program of the symposium was developed around topics about the role, functional determinants, technical aspects, limitations, and clinical implications of membranes presently in use. International experts with clinical or technical expertise as well as scientific recognition within the nephrology community were asked to prepare their presentations based on their own experiences, perceptions, opinions, and sources of information. The symposium devoted a major portion to discussing novel approaches for improving membranes and treatment quality, including updates on innovative concepts that may could potentially transform the landscape of kidney replacement therapy for chronic kidney disease patients in the future.
Implications: The intent was to provide insights into current attention points for healthcare professionals new to the field of MHD, and to test a unique forum for continuing medical education integrating physician and patient experiences to promote changes in clinical practice. Furthermore, the symposium premiered a specifically developed mixed reality holographic 3D model to demonstrate recent dialyzer innovation diminishing protein fouling on membrane surfaces. As a continuous online educational platform for scientific exchange, this Life/2022 event provided online learning opportunities with on-demand content, with all symposium lectures freely available on nephrologycampus.com.
1 Symposium context and objectives
The demand for MHD is projected to increase significantly over the next decade, increasing the burden for healthcare professionals (HCPs) and systems (1, 2). There’s a growing concern regarding the shortage of skilled nephrology professionals, which could impact the quality of dialysis care. Nephrology is a complex medical field due to the multifaceted nature of diseases, technological integration, and cost pressures. When nine different markers of patient complexity were evaluated, Nephrology was ranked as the most complex of all disciplines (3).
To address the reluctance in choosing nephrology as a career, more efficient and time-saving educational methods and utilization of modern online tools, have gained traction. The Life/nephrology campus strives to provide concise, updated educational material collated by experts for newcomers as well as for established MHD care personnel.
The Life/2022 Membrane Symposium focused on recent advances in membrane technology, particularly in uremic toxin removal in dialysis patients. Renowned experts discussed issues revolving around chronic inflammation and the role of dialysis in bioincompatibility. They also highlighted upcoming technical innovations promising improved patient outcomes and quality of life.
This symposium introduced a novel educational approach, combining on-site speaker presentations for healthcare professionals with a real-time online global audience. The content was categorized into six sections (Table 1), available for scientific discussion and continuous education on nephrologycampus.com. The online library also includes visual aids to enhance learning accessibility, such as movies and animated videos elucidating complex membrane science concepts, e.g., a movie explaining the stages of production of both membranes and dialyzers, and an animated video series depicting the membrane perspective of uremic toxins.

Table 1. Key topics of the Membrane Symposium: aspects of progress in dialysis membrane technology aiming for an improvement of clinical results and topics within the Membrane Symposium.
1.1 Delivery of hemodialysis
Patients with end-stage kidney disease (ESKD) undergo MHD with approximately 89% experiencing a substantial disease burden, reduced life expectancy, notable symptom burden, and a low health-related quality of life (2). These patients have a complex clinical profile, commonly presenting multiple comorbidities, especially cardiovascular (CV) pathologies, protein-energy wasting, and diabetes (4), with increasing age exacerbating their health complications (5). Albeit life-saving, clinical outcomes associated with MHD lag behind the general population and other chronic diseases (2). Rather than exclusively prioritizing survival, there’s an increased focus on symptom alleviation and enhancing functional and social rehabilitation (1). The current intermittent dialysis schedule hampers effective uremic solute removal, internal milieu restoration, clinical tolerance, and patient rehabilitation (6, 7). Research suggests more frequent and extended sessions, often referred to as intensive dialysis, could mitigate these challenges, yet concerns about elevated costs impede widespread adoption by payors and healthcare systems (1, 8). Technological advances in extracorporeal circuitry (ECC) have historically improved the efficiency, reliability, safety, and handling of renal replacement therapy procedures (9, 10). The dialysis membrane, while crucial for detoxification in MHD, also contributes to bioincompatible reactions with their entailed dialysis-related morbidity.
MHD facilitates life prolongation by regularly reducing concentrations of harmful compounds (11). A semi-permeable membrane allows for size-dependent elimination of substances to combat the toxic uremic milieu. The membrane’s structure, dialyzer design, and operating conditions determine solute exchange efficiency (12). Each dialyzer/membrane type presents three interrelated functionalities, each of them decisive for therapeutic quality: a ‘bioexchanger,’ eliminating toxins and excess water, a ‘bioreactor,’ initiating biochemical reactions, and a ‘bioselector,’ balancing the removal of unwanted and essential substances (13) (Figure 1). Despite progress, advancements in membrane science and technology remain vital for refining dialysis therapies (14).
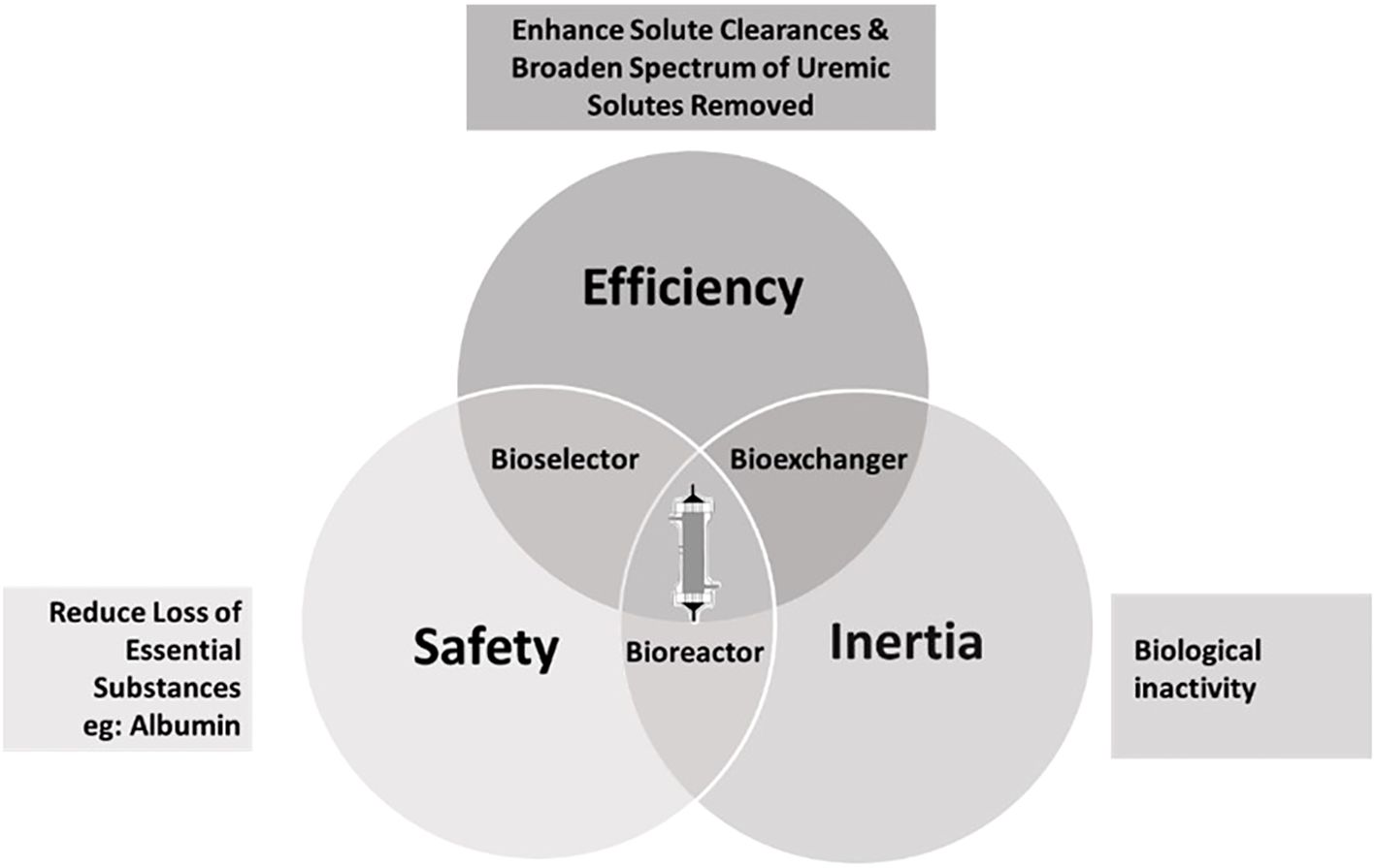
Figure 1. Relationships between the three most characteristic dialyzer functions. Modified from Canaud et al. (13).
The assessment and removal of accumulated uremic retention solutes (URS) during MHD are fundamental for replacing kidney function in ESKD. Traditionally, URS classification focused on size (15), aiding membrane development but limiting clinicians’ ability to reduce circulating uremic toxin levels affecting patients’ well-being (2). A more goal-directed classification based on biological/chemical and clinical toxicity (16) has been proposed to grade key URS compounds (17) (Table 2), identify target molecules for removal, and develop novel elimination methods. However, distinguishing between toxic and beneficial metabolites remains challenging (18), as MHD follows size-exclusion, not toxicity principles. Hence, essential substances (e.g., albumin) can leak through membranes with larger pore size (19), calling for a paradigm shift toward focusing on the dialysis-related loss of beneficial metabolites (20). The uremic milieu entails various biochemical disorders (i.e., inflammation, oxidative and chlorine stresses, and carbamylation reactions) that modify accumulated compounds and increase their toxicity (i.e., beta2-microglobulin and B2M-amyloidosis). Additionally, discussions often overlook the adverse effects of inorganic compounds like water, sodium, potassium, and phosphate (21). impacting patients’ quality of life (QoL) and contributing to hemodynamic instability, CV morbidity, and mortality in MHD patients (22). Tissue sodium accumulation contributes to cardiac and metabolic disorders independently from its osmotic and hemodynamic action (22). Hyperphosphatemia is important in the pathogenesis of secondary hyperparathyroidism, endothelial dysfunction (23), and increased all-cause and CV mortality (24). During MHD, considerable amounts of intracellular phosphate are removed as observed by a significant decrease in intracellular ATP, reflecting MHD-induced cellular stress and/or intracellular phosphate regulation with potentially severe clinical consequences (25, 26). Understanding and managing these compounds’ effects are essential in optimizing treatment strategies for patients undergoing dialysis.
Among the currently available renal replacement modalities, online hemodiafiltration (HDF) is considered the most advanced renal replacement therapy, offering improved clearance of larger uremic toxins and better hemodynamic tolerance (27). It differs from conventional MHD by infusing sterile fluid prepared ‘online’ from standard dialysis fluid into the bloodstream, enhancing blood cleansing (28), (29). Depending on convection volume, high-dosage HDF is associated with better survival rates compared to standard HD (29–32) with survival improving at about 55 to 75 l convection volume per week (29, 33). The CONVINCE trial provides evidence for the superiority of high-volume HDF with a 23% risk reduction in all-cause mortality (34). Mechanisms remain unclear but are likely multifactorial (35). The ongoing UK H4RT study is expected to further support HDF’s superiority over high-flux MHD (36, 37).
1.2 Systemic inflammation
Systemic inflammation is intrinsically linked to chronic kidney disease (CKD) (38), contributing to end-organ damage, particularly in the cardiovascular system and to premature ageing (39). In early CKD stages, over half of patients exhibit increased CRP levels (40), with severity escalating in later stages and complex inflammatory processes persisting in ESKD (41, 42). Uremic toxins contribute to pro-inflammatory effects, potentially catalysing the toxic uremic milieu, promoting risks for wasting and vascular disease (17, 37, 40, 43–46).
The large gap between the health span and lifespan of patients with chronic conditions is a major problem in modern healthcare (47). Lifestyle diseases contribute to senescence and vascular aging in CKD, with factors like uremic toxins, inflammation, and dialysis amplifying oxidative stress and inflammation (47, 48). Loss of gut microbiota biodiversity due to dietary effects further contributes to the allostatic load and aging (‘inflammaging’) (39). A five-year difference in biological and chronological age in patients requiring MHD emphasizes the need for interventions against uremic inflammation (47, 49), eg by reducing inflammatory molecule production and strategies enhancing their removal through improved dialytic clearance (40). Phenotyping according to comorbidities, clinical, social, or cultural and environmental stress factors that add to the global burden of CKD (50) and endotyping for uremic toxins by proteomics, metabolomics, and genetic markers based on selected biomarkers both aim to individualize HD strategies to improve patient outcomes (50–52).
Imbalances between oxidant and antioxidant production, or pro-inflammatory and anti-inflammatory mechanisms, mark the diseased state in CKD (53). Endogenous (i.e. endothelial nitric oxide synthase uncoupling) and exogenous mechanisms (i.e. environmental pollution) contribute to oxidative stress, impacting various organs and pathways. This results in increased proinflammatory cytokines, fuelling CKD progression in a vicious circle (54). Post-translational modifications (PTMs) are bidirectional links between oxidative stress, inflammation, and premature aging in CKD (55). PTMs, mainly phosphorylation, acetylation, and ubiquitination, can alter the structure and function of proteins, exerting adverse or beneficial effects on the kidneys (56, 57). Therapeutic approaches to limit oxidative stress and inflammation and to increase beneficial PTMs are available but need to be tested in clinical trials.
Systemic inflammation associated with chronic kidney disease is part of the uremic milieu disturbances that contribute to the morbidity and mortality of CKD patients. Besides senescence and aging, it is now well established that inflammation and its associated disorders contribute to accelerated cardiovascular disease (58–60), protein-energy wasting (61, 62), vascular calcification (63) and bone disorders, and loss of kidney function (50, 64).
1.3 Bioincompatibility and inflammation axis in MHD therapy
By reducing uremic toxin load and exposure, HD therapy helps to decrease inflammation. However, it introduces other factors, including bioincompatibility at the ECC-patient interface and dialysis fluid contamination, which can amplify inflammation (65–68). Bioincompatibility and inflammation are then inherent to all extracorporeal therapies representing an additional risk factor to MHD patients. Inflammation in MHD results from repetitive interactions between blood, the dialysis membrane, and contaminants in the dialysis fluid. This induces a series of cascade reactions, including complement activation, leukocyte activation, cytokine release, and the release of oxidative stress mediators, which sustain acute phase protein responses and contribute to related organ damage (10, 69). Mitigating bioincompatibility reactions by improving membrane design, circuit components, and dialysis fluid purity is crucial during MHD delivery, as any additional inflammation compounds the existing inflammatory burden of CKD. Despite advances in membrane, dialyzer, and extracorporeal circuit design, bioincompatibility remains a significant issue to address (70). Addressing these issues, e.g. by improving membrane and dialysate purity, helps ameliorate the detrimental effects of the uremic toxin milieu (2, 71).
Independent studies link increased inflammation to factors related to MHD, including ECC components, modality, and patient comorbidities (68). Addressing inflammation in MHD requires patient-individualized approaches due to its multifaceted origins (72)—factors like membrane type, modality, convective volume, and dialysis fluid quality impact MHD-induced inflammation (70). The CONTRAST study revealed that stable CRP values can be maintained over a 3-year online HDF treatment, while CRP levels increased during MHD (73). The use of ultrapure dialysis and sterile substitution fluids in HDF can further reduce inflammation by eliminating endotoxins from dialysis fluid (74) and help preserve residual kidney function in hemodialysis (75, 76).
1.4 Blood-material interactions: unavoidable consequences of maintenance HD
ECC components (needle, blood tubing, air-trap chamber, and dialyzer) interact with blood constituents like plasma proteins and blood cells (70). Without the natural endothelial lining protection, blood encounters polymeric materials and geometries in the dialysis circuit (77), triggering potent reactions and blood component activation. Altered plasma proteins or cells engage multiple biochemical pathways, and the patient’s intrinsic immune response and complement pathways are activated during blood-membrane interaction. Precise characterization and risk stratification guide membrane selection to reduce ECC-induced inflammation, though clinical studies on immune activation in dialysis patients remain scarce.
The 1970s discovery of cellulose-based MHD membranes causing high complement pathways and leukocyte activation sparked the biocompatibility debate. Synthetic membranes induce lower activation and less inflammatory response but don’t eliminate it. Despite membrane biocompatibility advancements, achieving entirely compatible membranes remains challenging (78). Biocompatibility extends beyond membranes to the rest of the ECC. Heparin, commonly used for anticoagulation, is effective but doesn’t fully prevent bioincompatibility or coagulation/complement-leukocyte activation (10), unlike citrate in specific extracorporeal therapies.
As an integral part of the innate immune system, complement plays a crucial role in antibody-mediated immunity (79). Its functions include defending against bacterial infection, bridging innate and adaptive immunity, and eliminating immune complexes. In chronic conditions like CKD, the complement system actively regulates various inflammatory responses. In HD, complement activation induces inflammation, promotes coagulation, and impairs host defense. Overactivation of complement pathways leads to aging-related diseases, e.g. Alzheimer’s disease or age-related macular degeneration (AMD). In CKD, the complement-inflammation axis mimics accelerated kidney aging, involving key players like Klotho expression, C1-inhibitor, pentraxin 3, pericytes, and endothelial-to-mesenchymal transition (74, 75, 80, 81). Understanding HD-induced inflammaging, causing immunological dysfunction and long-term complications that affect mortality (82), could inspire new therapeutic approaches to delay this ‘premature aging phenotype’ and kidney aging processes (83).
Minimizing adverse reactions requires comprehensive characterization of blood-contacting medical devices like dialyzers and membranes (84). Evaluating blood-membrane interactions demands sophisticated methodologies and intricate analytical techniques. Protein adsorption to biomaterials determines subsequent blood reactions, creating a biologically active surface (2-10 nm thick), of protein concentrations up to 1000 times higher than plasma. Proteomic technologies help characterize this protein adsorption/desorption (‘Vroman effect’) (85) creating a ‘blood-material interface’ that then mediates blood cell adhesion and coagulation or complement system activation (86). Platelet and leukocyte activation induce the release of extracellular vesicles, critical markers for cellular activation and intercellular communication (87). Those vesicles are a source of phosphatidylserine and tissue factor, amplifying thrombin generation (88). Flow cytometric techniques prove valuable for studying interactions of extracellular vesicles with biomaterials or with leukocyte subsets (89).
Dialyzer membranes vary in structure and biocompatibility, with different materials and production processes (90, 91). Even membranes from the same base polymer (e.g., polysulfone) may exhibit different biocompatibility and safety profiles (92). Guided by clinical experience, manufacturers aim to minimize adverse reactions, balancing hydrophobic and hydrophilic properties to compromise between complement and coagulation pathway activation. Approaches like adding PVP or thrombomodulin to polysulfone aim to prevent coagulation or platelet adhesion, but data on their success are controversial (93). Vitamin E-coated membranes target oxidative stress and DNA damage reduction (94, 95), but their applicability is limited due to manufacturing constraints and costs.
ECC clotting issues in HD units are common, often stemming from variable and sub-optimal anticoagulation. Assessing clotting relies on subjective visual evaluations of dialyzer color (96). Objective methods such as micro-computed tomography scanning of fibers offer a superior approach to visual scoring, dry weight assessment, or pressure measurement for addressing anticoagulation and fiber patency issues, at least in experimental settings (97).
1.5 Advances in membrane and technology
Several symposium presentations focused on dialysis membrane and dialyzer development, highlighting engineering accomplishments in physiochemistry, thermodynamics, material sciences, and sterilization. The growing demand for dialyzer units necessitates mass production with robotic automation which requires a balance between productivity, recycling, environmental safety, and cost constraints. From conceptualization to delivery, attention is paid to uniformity, quality, clinical performance, and safety. Adjusting membrane ‘spinning’ parameters customizes pore size and porosity for desired sieving properties (98).
However, increasing porosity to remove larger uremic toxins may cause essential substance loss: albumin and amino acid leakage have to be kept to a minimum (19). Achieving optimal membrane structure relies on selecting core polymer–copolymer combinations with lower activation of biological pathways during blood-membrane interaction (98) and higher pro-inflammatory endotoxin retention (99), less protein fouling, and higher hydraulic and solute permeability (100). The development of superflux or super high-flux membranes, utilizing conventional polymers to broaden the range of uremic toxin removal, is a key focus of membrane research and advanced technology in dialysis. Medium Cutoff (MCO) and High Cutoff (HCO) membranes exemplify this research, aimed at improving the clearance of middle- and large-molecular-weight uremic toxins compared to traditional high-flux membranes (13, 101, 102). These advancements are achieved by increasing membrane pore size and enhancing internal filtration and backtransport mechanisms, which together optimize convective clearance. MCO membranes are specifically designed to remove middle- and large-sized uremic toxins while minimizing the loss of essential plasma proteins, such as albumin. MCO-based dialyzers are currently undergoing evaluation in clinical hemodialysis settings for their potential to enhance internal convective clearance, such as through internal hemodiafiltration. HCO membranes, with even larger pore sizes, enable the removal of larger molecules, including inflammatory mediators and nephrotoxic light chains, which are implicated in conditions like myeloma kidney. This technology holds promise for patients with acute kidney injury (AKI) characterized by severe inflammatory responses, as well as those requiring continuous kidney replacement therapy (CKRT). Despite the potential of these advanced membranes, clinical trials aimed at demonstrating their superiority have thus far yielded disappointing results. Further research is needed to fully assess their efficacy and optimize their clinical applications. Microporous silicone membranes, particularly silicon nanopore membranes (SNMs), are under development and evaluation for use in innovative wearable and implantable artificial kidney projects (103, 104). These membranes have uniform nanopores, typically ranging from 5 to 10 nanometers in size, which are critical for effectively filtering uremic toxins from the blood. However, the low porosity of these membranes (≤1%) presents a challenge. To address this, advances such as arrays of nanoslits are being explored to enhance permeability without compromising selectivity for small solutes. SNMs have shown promise in reducing the surface area needed for dialysis, which is a key factor in the miniaturization of artificial kidneys, potentially paving the way for the development of implantable devices. Trilayer interlinked graphene oxide membrane represents a cutting-edge advancement in dialysis technology, capitalizing on graphene’s unique properties to improve separation performance (105). Laboratory tests have demonstrated that these membranes can achieve high permeability and selectivity, offering potential advantages in biocompatibility and toxin removal efficiency compared to traditional polymeric membranes. These emerging membrane technologies present significant disruptive potential for enhancing renal replacement therapy. However, further work is needed in scaling up manufacturing processes and conducting outcome-based studies before these innovations can be fully integrated into the therapeutic arsenal of renal replacement therapy.
Innovative processes aimed at modifying membrane surface reactivity, such as stabilizing polyvinylpyrrolidone (PVP) on the blood-contacting surface, are crucial for reducing protein adsorption and enhancing biocompatibility (106): While conventional membranes elute PVP into the bloodstream (107), a recent approach stabilizes PVP with α-tocopherol (106). This modification creates a stable hydro layer at the inner surface, repelling excessive adsorption of proteins (107), enhancing biocompatibility, and maintaining high solute permeability throughout the treatment session (108).
Clinical evaluation and market introduction of innovations require evidence-based medicine (109). Real-world evidence is often used due to the time and cost challenges of interventional trials (110, 111). ECC and vascular access patency have been an issue (112), (113) that led to the exploration of alternative anticoagulation strategies, such as heparin-free MHD and membrane modification with fluorinated macromolecules (114). Dialyzer fibers present fluorinated end groups to create a surface that minimizes protein adsorption, platelet adhesion, and platelet activation. Evaluation of fluorinated macromolecule-modified dialyzers reveals promising results in reducing thrombus formation and bacterial adhesion (115), (116).
Efforts to improve HD membrane biocompatibility (117) historically relied on trial-and-error methods, often involving costly and time-intensive laboratory evaluations (118). A mathematical model based on morphology, chemistry, and interaction affinity of two membranes, along with in vivo and in vitro data (119), accurately predicted inflammatory biomarker release during HD therapy (120). Incorporating clinical operating conditions, the model´s applicability was extended to various membrane materials and facilitates the prediction and in vitro validation of inflammatory responses associated with synthetic membranes. The model offers a valuable tool to guide the development of novel materials and support evidence-based membrane synthesis (119, 120).
1.6 Emerging trends and future frontiers
Over decades, MHD operated by size-exclusion-based removal of uremic toxins across semipermeable membranes. Various avenues to improve HD strategies have been explored and presented at the symposium.
With a porous inner layer of polyethersulfone/polyvinylpyrrolidone (PES/PVP) (121) and an outer layer with activated carbon, Mixed Matrix fiber Membranes (MMMs) present a promising innovation (121–124) of high-flux membranes that showed limitations in protein-bound uremic toxin (PBUT) removal. MMMs offer effective filtration through diffusion, convection, and adsorption, facilitating MHD with reduced dialysate amounts. They provide high flux, low protein adsorption, low albumin leakage, and excellent hemocompatibility (115, 121), and may protect patients by adsorbing bacterial pyrogens from the dialysate (125). They also show potential for urea removal from dialysate (126) suggesting applications in portable artificial kidney systems.
Efforts to engineer cell-based kidney replacement therapy focus on creating a bioartificial kidney (BAK) replicating key functions of native kidneys, with uremic waste removal as a primary objective (127, 128). Human conditionally immortalized proximal tubular epithelial cell (ciPTEC) lines show promise for BAK development (Figure 2) (124), with the ability of active organic cation transport (130, 131), secretory clearance of albumin-bound uremic toxins and albumin reabsorption. Endocrine functions like secretion of the active form of vitamin D, auto-immune and inflammatory response, and tumorigenic and oncogenic effects have also been investigated (132–134). Absent alloo-stimulatory effects and ciPTEC monolayer stability indicate safety for BAK application, but challenges such as cell sourcing, organ scaffolding, and immune response must be addressed before clinical adaptation for human treatment (135, 136).
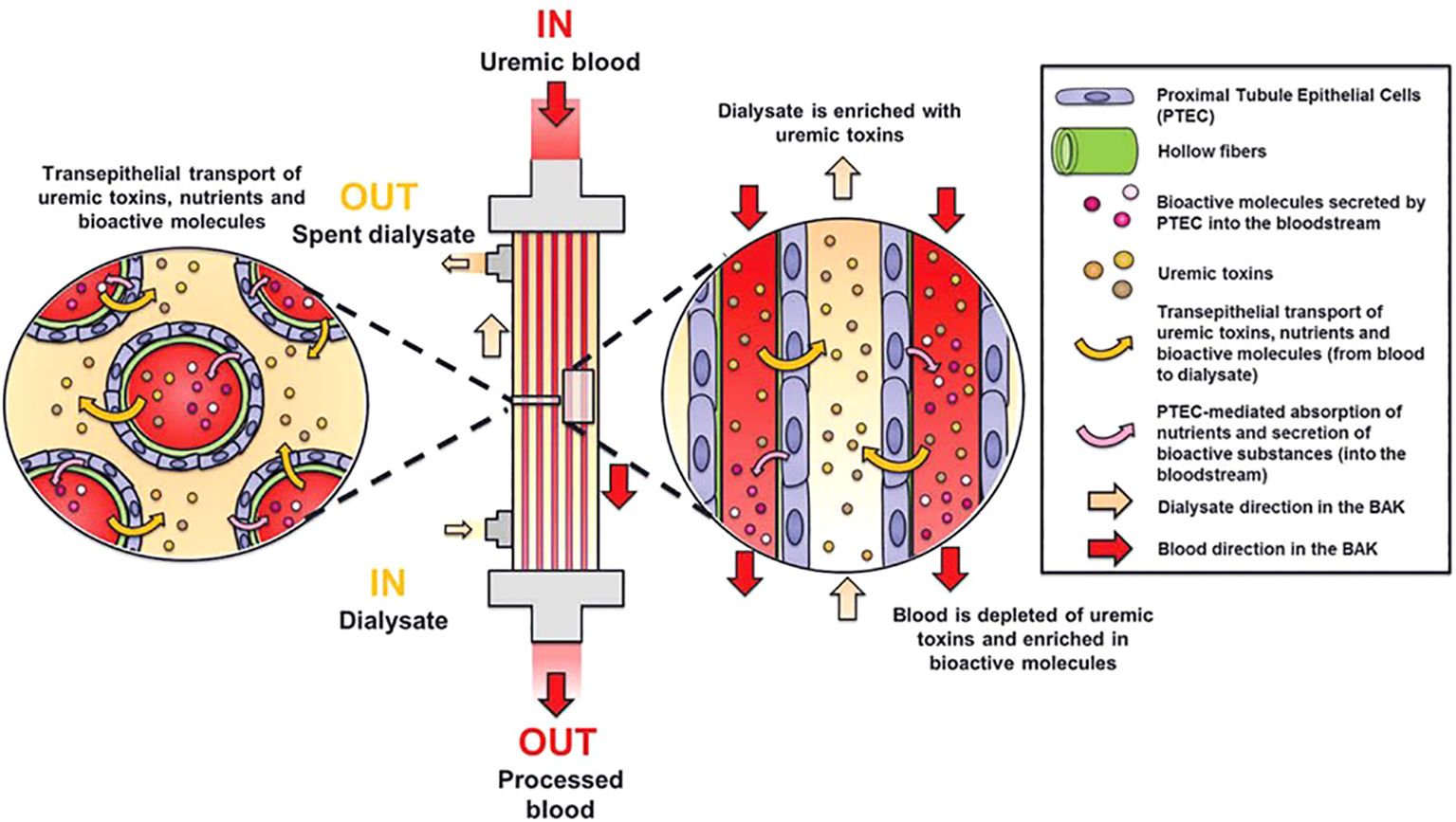
Figure 2. Principle of a bioartificial kidney. The living membrane of the BAK consists of human proximal tubule epithelial cells (PTEC) cultured on hollow membranes. These cells are capable of active transport of uremic toxins and nutrients and secretion of bioactive molecules. Modified from Van Gelder et al.(129).
Several research groups are advancing both wearable and implantable artificial kidneys as viable alternatives to conventional MHD (105). Notable among these efforts is the KidneyX project in the US. Wearable artificial kidneys (WAKs) are designed to be lightweight and portable, offering continuous dialysis and enhanced patient mobility. Implantable artificial kidneys (IAKs) aim to replace failing kidneys through surgical implantation of miniaturized artificial kidney (e.g., silicone nanoporous slit membranes and advanced housing and connecting materials). These devices promise a more permanent and transformative solution. Despite significant technological advancements, challenges such as device size, biocompatibility, vascular access, bladder connection, toxin removal efficiency, and long-term functionality still need to be addressed through clinical trials. Additionally, recent progress in the transplantation of genetically modified porcine kidneys has shown promising results and is approaching clinical application (137, 138).
Artificial intelligence (AI) applications in nephrology, though scarce, show promise (139, 140). Deep learning, a subset of machine learning, involves multi-layered neural networks learning from extensive data (141). The AI application process uses problem definition, data preparation, model building, and data validation steps. In CKD care AI has been successfully applied to predict clinical events, to provide treatment decision aids for optimal drug prescription in anemia control, and to identify patterns for event development (e.g., classification of phenotypical clusters of arteriovenous fistula aneurysms) (142). Optimized machine learning models could enhance risk identification and drive pre-emptive interventions (143). While AI in dialysis practice is in its infancy, more research is needed for consolidated aids in clinical decision-making, and future applications may offer real-time, continuous recommendations for optimal kidney care outcomes (144).
Emerging trends in HD address the growing demand for kidney replacement therapies and the limitations of the current provider system (145). Therapeutic advances were associated with parallel reductions in short-term risk of death and major adverse CV events (143). More frequent and longer MHD sessions show improvements in CV markers (146, 147), and flexible care models, including home or satellite HD and incremental, extended, and shared care, enhance treatment options (135, 148, 149). Wearable artificial kidneys aim for continuous blood purification and patient autonomy, but challenges like electrolyte balance (150) and suitable membrane selection (151) need to be addressed. Further investigation into preserving residual kidney function and modulating gut microbiota are exciting prospects for shaping the future of dialysis.
This future cannot be designed without considering the future of our planet. Dialysis therapies come at an enormous environmental cost due to their water consumption, greenhouse gas emissions, and waste production. Policymakers, manufacturers, providers, healthcare providers, and (future) patients need to put efforts into implementing urgent environmental changes which must include but are not limited to dialysate regeneration, dialysate flow reduction, water distillation systems for dialysate production, and biodegradable and bio-based polymers (150, 152).
Data availability statement
The original contributions presented in the study are included in the article/supplementary material. Further inquiries can be directed to the corresponding author.
Author contributions
CW: Conceptualization, Supervision, Writing – original draft, Writing – review & editing, Investigation, Validation. RV: Conceptualization, Investigation, Validation, Writing – review & editing. AO: Conceptualization, Investigation, Validation, Writing – review & editing. AD: Conceptualization, Investigation, Validation, Writing – review & editing. BC: Conceptualization, Investigation, Supervision, Validation, Writing – review & editing. PB: Conceptualization, Investigation, Validation, Writing – review & editing. RM: Conceptualization, Investigation, Validation, Writing – review & editing. JK: Conceptualization, Investigation, Validation, Writing – review & editing. GC: Conceptualization, Investigation, Validation, Writing – review & editing. DS: Conceptualization, Investigation, Validation, Writing – review & editing. SM: Conceptualization, Investigation, Validation, Writing – review & editing. MG: Conceptualization, Investigation, Supervision, Validation, Writing – review & editing. VW: Conceptualization, Investigation, Validation, Writing – review & editing. TE: Conceptualization, Investigation, Supervision, Validation, Writing – review & editing. AA: Investigation, Validation, Writing – review & editing. SS: Supervision, Writing – review & editing. AS: Conceptualization, Project administration, Resources, Supervision, Visualization, Writing – review & editing. PS: Conceptualization, Investigation, Supervision, Validation, Writing – original draft, Writing – review & editing.
Funding
The author(s) declare financial support was received for the research, authorship, and/or publication of this article. DS acknowledges the financial support of: (i) the Strategic alliance of the University of Twente, University of Utrecht, and University Medical Center Utrecht (2018-2023) (ii) the “European Uremic Toxin Working Group” (EUTox) of the “European Society for Artificial Organs” (ESAO) endorsed by the “European Renal Association-European Dialysis Transplantation Association” (ERA-EDTA). (iii) New-generation high-tech technology (NXTGEN HIGHTECH) growth fund program of the Dutch government (2023-27). RM acknowledges the financial support of: (i) the Strategic alliance of the University of Twente, University of Utrecht, and University Medical Center Utrecht (2018-2023) (ii) the “European Uremic Toxin Working Group” (EUTox) of the “European Society for Artificial Organs” (ESAO) endorsed by the “European Renal Association-European Dialysis Transplantation Association” (ERA-EDTA). (iii) the European Commission (KIDNEW, HORIZON-EIC-2022 Pathfinder program grant agreement no. 101099092). TE was supported by a Novo Nordisk postdoctoral fellowship run in partnership with Karolinska Institutet, Stockholm, Sweden, a Karolinska Institutet Research Foundation grant, the Stiftelsen Stig och Gunborg Westman, the Swedish Kidney Foundation (Njurfonden), the German Diabetes Association (DDG), as well as by the Team Award Nephrology+ 2023 by Otsuka Pharma. T.E. was further funded through the European Fund for Sustainable Development (EFSD) Mentorship Program supported by AstraZeneca. SM is supported by NIHR Health Technology Centre, Devices for Dignity, Sheffield, UK.
Conflict of interest
AO has received grants from Sanofi and consultancy or speaker fees or travel support from Adviccene, Alexion, Astellas, Astrazeneca, Amicus, Amgen, Bioporto, Boehringer Ingelheim, Esteve, Fresenius Medical Care, GSK, Bayer, Sanofi-Genzyme, Sobi, Menarini, Mundipharma, Kyowa Kirin, Lilly, Freeline, Idorsia, Chiesi, Otsuka, Novo-Nordisk, Sysmex and Vifor Fresenius Medical Care Renal Pharma and Spafarma and is Director of the Catedra UAM-Astrazeneca of chronic kidney disease and electrolytes. He has stock in Telara Farma.
Authors SS and AS were employed by Fresenius SE & Co. KGaA.
The remaining authors declare that the research was conducted in the absence of any commercial or financial relationships that could be construed as a potential conflict of interest.
Publisher’s note
All claims expressed in this article are solely those of the authors and do not necessarily represent those of their affiliated organizations, or those of the publisher, the editors and the reviewers. Any product that may be evaluated in this article, or claim that may be made by its manufacturer, is not guaranteed or endorsed by the publisher.
References
1. Himmelfarb J, Vanholder R, Mehrotra R, Tonelli M. The current and future landscape of dialysis. Nat Rev Nephrol. (2020) 16:573–85. doi: 10.1038/s41581-020-0315-4
2. Vanholder R, Annemans L, Bello AK, Bikbov B, Gallego D, Gansevoort RT, et al. Fighting the unbearable lightness of neglecting kidney health: the decade of the kidney. Clin Kidney J. (2021) 14:1719–30. doi: 10.1093/ckj/sfab070
3. Tonelli M, Wiebe N, Manns BJ, Klarenbach SW, James MT, Ravani P, et al. Comparison of the complexity of patients seen by different medical subspecialists in a universal health care system. JAMA network Open. (2018) 1:e184852–e184852. doi: 10.1001/jamanetworkopen.2018.4852
4. Wanner C, Amann K, Shoji T. The heart and vascular system in dialysis. Lancet. (2016) 388:276–84. doi: 10.1016/S0140-6736(16)30508-6
5. Bansal AD, Schell JO. A practical guide for the care of patients with end-stage renal disease near the end of life. Semin Dialysis. (2018) 31(2):170–6. doi: 10.1111/sdi.2018.31.issue-2
6. Ronco C. Dialysis delivery versus dialysis prescription. Int J Artif organs. (1993) 16:628–35. doi: 10.1177/039139889301600902
7. Morfin JA, Fluck RJ, Weinhandl ED, Kansal S, Mccullough PA, Komenda P. Intensive hemodialysis and treatment complications and tolerability. Am J Kidney Dis. (2016) 68:S43–50. doi: 10.1053/j.ajkd.2016.05.021
8. Apel C, Hornig C, Maddux FW, Ketchersid T, Yeung J, Guinsburg A. Informed decision-making in delivery of dialysis: Combining clinical outcomes with sustainability. Clin Kidney J. (2021) 14:i98–i113. doi: 10.1093/ckj/sfab193
9. Lameire N, Van Biesen W, Vanholder R. Did 20 years of technological innovations in hemodialysis contribute to better patient outcomes? Clin J Am Soc Nephrol. (2009) 4(Suppl 1):S30–40. doi: 10.2215/CJN.04000609
10. Cristol JP, Thierry AR, Bargnoux AS, Morena-Carrere M, Canaud B. What is the role of the neutrophil extracellular traps in the cardiovascular disease burden associated with hemodialysis bioincompatibility? Front Med (Lausanne). (2023) 10:1268748. doi: 10.3389/fmed.2023.1268748
11. Duranton F, Cohen G, De Smet R, Rodriguez M, Jankowski J, Vanholder R, et al. Normal and pathologic concentrations of uremic toxins. J Am Soc Nephrol. (2012) 23:1258–70. doi: 10.1681/ASN.2011121175
12. Bowry SK, Kotanko P, Himmele R, Tao X, Anger M. The membrane perspective of uraemic toxins: which ones should, or can, be removed? Clin Kidney J. (2021) 14(Suppl 4):i17–31. doi: 10.1093/ckj/sfab202
13. Canaud B. Recent advances in dialysis membranes. Curr Opin Nephrol Hypertension. (2021) 30:613–22. doi: 10.1097/MNH.0000000000000744
14. Bowry SK, Ortiz AA, Maddux FW. Deciphering the core elements around haemodialysis therapy. Clin Kidney J. (2021) 14(Suppl 4):i1–i4. doi: 10.1093/ckj/sfab224
15. Rosner MH, Reis T, Husain-Syed F, Vanholder R, Hutchison C, Stenvinkel P, et al. Classification of uremic toxins and their role in kidney failure. Clin J Am Soc Nephrol. (2021) 16:1918–28. doi: 10.2215/CJN.02660221
16. Vanholder R. Introduction to the toxins special issue on “Novel Issues in Uremic Toxicity”. Toxins (Basel). (2018) 10(10):388. doi: 10.3390/toxins10100388
17. Vanholder R, Pletinck A, Schepers E, Glorieux G. Biochemical and clinical impact of organic uremic retention solutes: a comprehensive update. Toxins. (2018) 10:33. doi: 10.3390/toxins10010033
18. Vanholder R, Nigam SK, Burtey S, Glorieux G. What if not all metabolites from the uremic toxin generating pathways are toxic? A hypothesis. Toxins. (2022) 14:221. doi: 10.3390/toxins14030221
19. Kalantar-Zadeh K, Ficociello LH, Bazzanella J, Mullon C, Anger MS. Slipping through the pores: hypoalbuminemia and albumin loss during hemodialysis. Int J Nephrol Renovascular Dis. (2021) 2021:14:11–21. doi: 10.2147/IJNRD.S291348
20. Meert N, Eloot S, Schepers E, Lemke H-D, Dhondt A, Glorieux G, et al. Comparison of removal capacity of two consecutive generations of high-flux dialysers during different treatment modalities. Nephrol Dialysis Transplant. (2011) 26:2624–30. doi: 10.1093/ndt/gfq803
21. Canaud B, Collins A, Maddux F. The renal replacement therapy landscape in 2030: reducing the global cardiovascular burden in dialysis patients. Nephrol Dialysis Transplant. (2020) 35:ii51–7. doi: 10.1093/ndt/gfaa005
22. Raimann JG, Ficociello LH, Usvyat LA, Zhang H, Pacelli L, Moore S, et al. Effects of dialysate to serum sodium (Na+) alignment in chronic hemodialysis (HD) patients: retrospective cohort study from a quality improvement project. BMC Nephrol. (2018) 19:1–12. doi: 10.1186/s12882-018-0870-0
23. Shuto E, Taketani Y, Tanaka R, Harada N, Isshiki M, Sato M, et al. Dietary phosphorus acutely impairs endothelial function. J Am Soc Nephrology: JASN. (2009) 20:1504. doi: 10.1681/ASN.2008101106
24. Spalding EM, Chamney PW, Farrington K. Phosphate kinetics during hemodialysis: Evidence for biphasic regulation. Kidney Int. (2002) 61:655–67. doi: 10.1046/j.1523-1755.2002.00146.x
25. Leypoldt JK, Agar BU, Akonur A, Hutchcraft AM, Story KO, Culleton BF. Determinants of phosphorus mobilization during hemodialysis. Kidney Int. (2013) 84:841–8. doi: 10.1038/ki.2013.205
26. Lemoine S, Fournier T, Kocevar G, Belloi A, Normand G, Ibarrola D, et al. Intracellular phosphate dynamics in muscle measured by magnetic resonance spectroscopy during hemodialysis. J Am Soc Nephrol. (2016) 27:2062–8. doi: 10.1681/ASN.2015050546
27. Canaud B, Blankestijn PJ, Grooteman MP, Davenport A. Why and how high volume hemodiafiltration may reduce cardiovascular mortality in stage 5 chronic kidney disease dialysis patients? A comprehensive literature review on mechanisms involved. Semin Dialysis. (2022) 35(2):117–28. doi: 10.1111/sdi.13039
28. Nubé MJ, Peters SAE, Blankestijn PJ, Canaud B, Davenport A, Grooteman MPC, et al. Mortality reduction by post-dilution online-haemodiafiltration: a cause-specific analysis. Nephrol Dial Transplant. (2017) 32(3):548–55. doi: 10.1093/ndt/gfw381
29. Peters SA, Bots ML, Canaud B, Davenport A, Grooteman MP, Kircelli F, et al. Haemodiafiltration and mortality in end-stage kidney disease patients: a pooled individual participant data analysis from four randomized controlled trials. Nephrol Dialysis Transplant. (2016) 31:978–84. doi: 10.1093/ndt/gfv349
30. Grooteman MP, Van Den Dorpel MA, Bots ML, Penne EL, Van Der Weerd NC, Mazairac AH, et al. Effect of online hemodiafiltration on all-cause mortality and cardiovascular outcomes. J Am Soc Nephrol. (2012) 23:1087–96. doi: 10.1681/ASN.2011121140
31. Ok E, Asci G, Toz H, Ok ES, Kircelli F, Yilmaz M, et al. Mortality and cardiovascular events in online haemodiafiltration (OL-HDF) compared with high-flux dialysis: results from the Turkish OL-HDF Study. Nephrol Dial Transplant. (2013) 28:192–202. doi: 10.1093/ndt/gfs407
32. Morena M, Jaussent A, Chalabi L, Leray-Moragues H, Chenine L, Debure A, et al. Treatment tolerance and patient-reported outcomes favor online hemodiafiltration compared to high-flux hemodialysis in the elderly. Kidney Int. (2017) 91:1495–509. doi: 10.1016/j.kint.2017.01.013
33. Canaud B, Barbieri C, Marcelli D, Bellocchio F, Bowry S, Mari F, et al. Optimal convection volume for improving patient outcomes in an international incident dialysis cohort treated with online hemodiafiltration. Kidney Int. (2015) 88:1108–16. doi: 10.1038/ki.2015.139
34. Blankestijn PJ, Vernooij RWM, Hockham C, Strippoli GFM, Canaud B, Hegbrant J, et al. Effect of hemodiafiltration or hemodialysis on mortality in kidney failure. New Engl J Med. (2023) 389(8):700–9. doi: 10.1056/NEJMoa2304820
35. Blankestijn PJ, Fischer KI, Barth C, Cromm K, Canaud B, Davenport A, et al. Benefits and harms of high-dose haemodiafiltration versus high-flux haemodialysis: the comparison of high-dose haemodiafiltration with high-flux haemodialysis (CONVINCE) trial protocol. BMJ Open. (2020) 10:e033228. doi: 10.1136/bmjopen-2019-033228
36. Vernooij RW, Bots ML, Strippoli GF, Canaud B, Cromm K, Woodward M, et al. CONVINCE scientific committee. CONVINCE in the context of existing evidence on haemodiafiltration. Nephrol Dial Transplant. (2022) 37(6):1006–13. doi: 10.1093/ndt/gfac019
37. Akchurin OM, Kaskel F. Update on inflammation in chronic kidney disease. Blood purification. (2015) 39:84–92. doi: 10.1159/000368940
38. Machowska A, Carrero JJ, Lindholm B, Stenvinkel P. Therapeutics targeting persistent inflammation in chronic kidney disease. Trans Res. (2016) 167:204–13. doi: 10.1016/j.trsl.2015.06.012
39. Kooman JP, Dekker MJ, Usvyat LA, Kotanko P, Van Der Sande FM, Schalkwijk CG, et al. Inflammation and premature aging in advanced chronic kidney disease. Am J Physiology-Renal Physiol. (2017) 313:F938–50. doi: 10.1152/ajprenal.00256.2017
40. Cobo G, Lindholm B, Stenvinkel P. Chronic inflammation in end-stage renal disease and dialysis. Nephrol Dialysis Transplant. (2018) 33:iii35–40. doi: 10.1093/ndt/gfy175
41. Carrero JJ, Stenvinkel P. Inflammation in end-stage renal disease—what have we learned in 10 years? Semin Dialysis. (2010) 23(5):498–509. doi: 10.1111/j.1525-139X.2010.00784.x
42. Mihai S, Codrici E, Popescu ID, Enciu A-M, Rusu E, Zilisteanu D, et al. Inflammation-related patterns in the clinical staging and severity assessment of chronic kidney disease. Dis Markers. (2019) 2019. doi: 10.1155/2019/1814304
43. Mihai S, Codrici E, Popescu ID, Enciu A-M, Albulescu L, Necula LG, et al. Inflammation-related mechanisms in chronic kidney disease prediction, progression, and outcome. J Immunol Res. (2018) 2018. doi: 10.1155/2018/2180373
44. Vanholder RC, Glorieux GL, De Smet RV. Back to the future: middle molecules, high flux membranes, and optimal dialysis. Hemodialysis Int. (2003) 7:52–7. doi: 10.1046/j.1492-7535.2003.00004.x
45. Lau WL, Kalantar-Zadeh K, Vaziri ND. The gut as a source of inflammation in chronic kidney disease. Nephron. (2015) 130:92–8. doi: 10.1159/000381990
46. Carrero JJ, Stenvinkel P. Persistent inflammation as a catalyst for other risk factors in chronic kidney disease: a hypothesis proposal. Clin J Am Soc Nephrol. (2009) 4:S49–55. doi: 10.2215/CJN.02720409
47. Hobson S, Arefin S, Witasp A, Hernandez L, Kublickiene K, Shiels P, et al. Accelerated vascular aging in chronic kidney disease: the potential for novel therapies. Circ Res. (2023) 132:950–69. doi: 10.1161/CIRCRESAHA.122.321751
48. Dai L, Qureshi AR, Witasp A, Lindholm B, Stenvinkel P. Early vascular ageing and cellular senescence in chronic kidney disease. Comput Struct Biotechnol J. (2019) 17:721–9. doi: 10.1016/j.csbj.2019.06.015
49. Tian YE, Cropley V, Maier AB, Lautenschlager NT, Breakspear M, Zalesky A. Heterogeneous aging across multiple organ systems and prediction of chronic disease and mortality. Nat Med. (2023) 29:1221–31. doi: 10.1038/s41591-023-02296-6
50. Stenvinkel P, Chertow GM, Devarajan P, Levin A, Andreoli SP, Bangalore S, et al. Chronic inflammation in chronic kidney disease progression: role of nrf2. Kidney Int Rep. (2021) 6:1775–87. doi: 10.1016/j.ekir.2021.04.023
51. Dai L, Schurgers LJ, Shiels PG, Stenvinkel P. Early vascular ageing in chronic kidney disease: impact of inflammation, vitamin K, senescence and genomic damage. Nephrol Dialysis Transplant. (2020) 35:ii31–7. doi: 10.1093/ndt/gfaa006
52. Franco I, Helgadottir HT, Moggio A, Larsson M, Vrtačnik P, Johansson A, et al. Whole genome DNA sequencing provides an atlas of somatic mutagenesis in healthy human cells and identifies a tumor-prone cell type. Genome Biol. (2019) 20:1–22. doi: 10.1186/s13059-019-1892-z
53. Ebert T, Neytchev O, Witasp A, Kublickiene K, Stenvinkel P, Shiels PG. Inflammation and oxidative stress in chronic kidney disease and dialysis patients. Antioxidants Redox Signaling. (2021) 35:1426–48. doi: 10.1089/ars.2020.8184
54. Rapp N, Evenepoel P, Stenvinkel P, Schurgers L. Uremic toxins and vascular calcification–missing the forest for all the trees. Toxins. (2020) 12:624. doi: 10.3390/toxins12100624
55. Gajjala PR, Fliser D, Speer T, Jankowski V, Jankowski J. Emerging role of post-translational modifications in chronic kidney disease and cardiovascular disease. Nephrol Dialysis Transplant. (2015) 30:1814–24. doi: 10.1093/ndt/gfv048
56. Ebert T, Tran N, Schurgers L, Stenvinkel P, Shiels PG. Ageing–Oxidative stress, PTMs and disease. Mol Aspects Med. (2022) 86:101099. doi: 10.1016/j.mam.2022.101099
57. Laget J, Duranton F, Argilés À, Gayrard N. Renal insufficiency and chronic kidney disease–Promotor or consequence of pathological post-translational modifications. Mol Aspects Med. (2022) 101082. doi: 10.1016/j.mam.2022.101082
58. Wang Y, Gao L. Inflammation and cardiovascular disease associated with hemodialysis for end-stage renal disease. Front Pharmacol. (2022) 13:800950. doi: 10.3389/fphar.2022.800950
59. Zoccali C, Mallamaci F, Adamczak M, De Oliveira RB, Massy ZA, Sarafidis P, et al. Cardiovascular complications in chronic kidney disease: a review from the European Renal and Cardiovascular Medicine Working Group of the European Renal Association. Cardiovasc Res. (2023) 119:2017–32. doi: 10.1093/cvr/cvad083
60. Jankowski J, Floege J, Fliser D, Böhm M, Marx N. Cardiovascular disease in chronic kidney disease: pathophysiological insights and therapeutic options. Circulation. (2021) 143:1157–72. doi: 10.1161/CIRCULATIONAHA.120.050686
61. Carrero JJ, Chmielewski M, Axelsson J, Snaedal S, Heimbürger O, Bárány P, et al. Muscle atrophy, inflammation and clinical outcome in incident and prevalent dialysis patients. Clin Nutr. (2008) 27:557–64. doi: 10.1016/j.clnu.2008.04.007
62. Carrero JJ, Stenvinkel P, Cuppari L, Ikizler TA, Kalantar-Zadeh K, Kaysen G, et al. Etiology of the protein-energy wasting syndrome in chronic kidney disease: a consensus statement from the International Society of Renal Nutrition and Metabolism (ISRNM). J Ren Nutr. (2013) 23:77–90. doi: 10.1053/j.jrn.2013.01.001
63. Cannata-Andía JB, Martín-Carro B, Martín-Vírgala J, Rodríguez-Carrio J, Bande-Fernández JJ, Alonso-Montes C, et al. Chronic kidney disease-mineral and bone disorders: pathogenesis and management. Calcif Tissue Int. (2021) 108(4):410–22. doi: 10.1007/s00223-020-00777-1
64. Kadatane SP, Satariano M, Massey M, Mongan K, Raina R. The role of inflammation in CKD. Cells. (2023) 12. doi: 10.3390/cells12121581
65. Lonnemann G. When good water goes bad: how it happens, clinical consequences and possible solutions. Blood purification. (2004) 22:124–9. doi: 10.1159/000074932
66. Rahmati M, Homel P, Hoenich N, Levin R, Kaysen G, Levin N. The role of improved water quality on inflammatory markers in patients undergoing regular dialysis. Int J Artif organs. (2004) 27:723–7. doi: 10.1177/039139880402700811
67. Nystrand R. Microbiology of water and fluids for hemodialysis. J Chin Med Assoc. (2008) 71:223–9. doi: 10.1016/S1726-4901(08)70110-2
68. Jofré R, Rodriguez-Benitez P, López-Gómez JM, Pérez-Garcia R. Inflammatory syndrome in patients on hemodialysis. J Am Soc Nephrol. (2006) 17:S274–80. doi: 10.1681/ASN.2006080926
69. Grooteman MP, Wauters IM, Teerlink T, Twisk JW, Nubé MJ. Plasma dimethylarginine levels in chronic hemodialysis patients are independent of the type of dialyzer applied. Blood purification. (2007) 25:281–9. doi: 10.1159/000104868
70. Bowry SK, Kircelli F, Himmele R, Nigwekar SU. Blood-incompatibility in haemodialysis: alleviating inflammation and effects of coagulation. Clin Kidney J. (2021) 14:i59–71. doi: 10.1093/ckj/sfab185
71. Bouré T, Vanholder R. Biochemical and clinical evidence for uremic toxicity. Artif organs. (2004) 28:248–53. doi: 10.1111/j.1525-1594.2004.47315.x
72. Jiang N, Qian J, Lin A, Lindholm B, Axelsson J, Yao Q. Initiation of glucose-based peritoneal dialysis is associated with increased prevalence of metabolic syndrome in non-diabetic patients with end-stage renal disease. Blood purification. (2008) 26:423–8. doi: 10.1159/000153248
73. Den Hoedt CH, Bots ML, Grooteman MP, Van Der Weerd NC, Mazairac AH, Penne EL, et al. Online hemodiafiltration reduces systemic inflammation compared to low-flux hemodialysis. Kidney Int. (2014) 86:423–32. doi: 10.1038/ki.2014.9
74. Hasegawa T, Nakai S, Masakane I, Watanabe Y, Iseki K, Tsubakihara Y, et al. Dialysis fluid endotoxin level and mortality in maintenance hemodialysis: a nationwide cohort study. Am J Kidney Dis. (2015) 65:899–904. doi: 10.1053/j.ajkd.2014.12.009
75. Schiffl H, Lang SM, Fischer R. Ultrapure dialysis fluid slows loss of residual renal function in new dialysis patients. Nephrol Dial Transplant. (2002) 17:1814–8. doi: 10.1093/ndt/17.10.1814
76. Vilar E, Fry AC, Wellsted D, Tattersall JE, Greenwood RN, Farrington K. Long-term outcomes in online hemodiafiltration and high-flux hemodialysis: a comparative analysis. Clin J Am Soc Nephrol. (2009) 4:1944–53. doi: 10.2215/CJN.05560809
77. Neubauer K, Zieger B. Endothelial cells and coagulation. Cell Tissue Res. (2022) 387:391–8. doi: 10.1007/s00441-021-03471-2
78. Bonomini M, Piscitani L, Di Liberato L, Sirolli V. Biocompatibility of surface-modified membranes for chronic hemodialysis therapy. Biomedicines. (2022) 10. doi: 10.3390/biomedicines10040844
79. Castellano G. The pivotal role of the mentor in triggering the research on Complement system. Mol Immunol. (2015) 68:25–6. doi: 10.1016/j.molimm.2015.06.003
80. Losappio V, Franzin R, Infante B, Godeas G, Gesualdo L, Fersini A, et al. Molecular mechanisms of premature aging in hemodialysis: The complex interplay between innate and adaptive immune dysfunction. Int J Mol Sci. (2020) 21:3422. doi: 10.3390/ijms21103422
81. Castellano G, Intini A, Stasi A, Divella C, Gigante M, Pontrelli P, et al. Complement modulation of anti-aging factor klotho in ischemia/reperfusion injury and delayed graft function. Am J Transplant. (2016) 16:325–33. doi: 10.1111/ajt.13415
82. Poppelaars F, Faria B, Gaya da Costa M, Franssen CFM, van Son WJ, Berger SP, et al. The complement system in dialysis: a forgotten story? Front Immunol. (2018) 9:71. doi: 10.3389/fimmu.2018.00071
83. Ferenbach DA, Bonventre JV. Mechanisms of maladaptive repair after AKI leading to accelerated kidney ageing and CKD. Nat Rev Nephrol. (2015) 11:264–76. doi: 10.1038/nrneph.2015.3
84. Weber M, Steinle H, Golombek S, Hann L, Schlensak C, Wendel HP, et al. Blood-contacting biomaterials: in vitro evaluation of the hemocompatibility. Front bioengineering Biotechnol. (2018) 6:99. doi: 10.3389/fbioe.2018.00099
85. Hirsh SL, Mckenzie DR, Nosworthy NJ, Denman JA, Sezerman OU, Bilek MM. The Vroman effect: competitive protein exchange with dynamic multilayer protein aggregates. Colloids Surfaces B: Biointerfaces. (2013) 103:395–404. doi: 10.1016/j.colsurfb.2012.10.039
86. Sivaraman B, Latour RA. Delineating the roles of the GPIIb/IIIa and GP-Ib-IX-V platelet receptors in mediating platelet adhesion to adsorbed fibrinogen and albumin. Biomaterials. (2011) 32:5365–70. doi: 10.1016/j.biomaterials.2011.04.011
87. Georgatzakou HT, Pavlou EG, Papageorgiou EG, Papassideri IS, Kriebardis AG, Antonelou MH. The multi-faced extracellular vesicles in the plasma of chronic kidney disease patients. Front Cell Dev Biol. (2020) 8:227. doi: 10.3389/fcell.2020.00227
88. Davenport A. What are the anticoagulation options for intermittent hemodialysis? Nat Rev Nephrol. (2011) 7(9):499–508. doi: 10.1038/nrneph.2011.88
89. Weiss R, Gröger M, Rauscher S, Fendl B, Eichhorn T, Fischer MB, et al. Differential interaction of platelet-derived extracellular vesicles with leukocyte subsets in human whole blood. Sci Rep. (2018) 8:6598. doi: 10.1038/s41598-018-25047-x
90. Hoenich NA, Woffindin C, Brennan A, Cox PJ, Matthews J, Goldfinch M. A comparison of three brands of polysulfone membranes. J Am Soc Nephrol. (1996) 7:871–6. doi: 10.1681/ASN.V76871
91. Daugirdas JT, Bernardo AA. Hemodialysis effect on platelet count and function and hemodialysis-associated thrombocytopenia. Kidney Int. (2012) 82:147–57. doi: 10.1038/ki.2012.130
92. Krieter DH, Canaud B, Lemke HD, Rodriguez A, Morgenroth A, Von Appen K, et al. B isphenol A in chronic kidney disease. Artif organs. (2013) 37:283–90. doi: 10.1111/j.1525-1594.2012.01556.x
93. Omichi M, Matsusaki M, Maruyama I, Akashi M. Improvement of blood compatibility on polysulfone–polyvinylpyrrolidone blend films as a model membrane of dialyzer by physical adsorption of recombinant soluble human thrombomodulin (ART-123). J Biomaterials Science Polymer Edition. (2012) 23:593–608. doi: 10.1163/092050611X555669
94. D’Arrigo G, Baggetta R, Tripepi G, Galli F, Bolignano D. Effects of vitamin E-coated versus conventional membranes in chronic hemodialysis patients: a systematic review and meta-analysis. Blood Purification. (2017) 43:101–22. doi: 10.1159/000453444
95. Maitz MF, Zitzmann J, Hanke J, Renneberg C, Tsurkan MV, Sperling C, et al. Adaptive release of heparin from anticoagulant hydrogels triggered by different blood coagulation factors. Biomaterials. (2017) 135:53–61. doi: 10.1016/j.biomaterials.2017.04.044
96. Fazendeiro Matos J, Pinto B, Felix C, Carvalho H, Ponce P, Peralta R. Does subjective assessment of dialyzer appearance reflect dialyzer performance in online hemodiafiltration? Hemodialysis Int. (2020) 24(1):61–70. doi: 10.1111/hdi.12788
97. Vanommeslaeghe F, Van Biesen W, Dierick M, Boone M, Dhondt A, Eloot S. Micro-computed tomography for the quantification of blocked fibers in hemodialyzers. Sci Rep. (2018) 8:1–9. doi: 10.1038/s41598-018-20898-w
98. Bowry S, Ronco C. Surface topography and surface elemental composition analysis of Helixone®, a new high-flux polysulfone dialysis membrane. Int J Artif Organs. (2001) 24:757–64. doi: 10.1177/039139880102401101
99. Bowry S. Dialysis membranes today. Int J Artif organs. (2002) 25:447–60. doi: 10.1177/039139880202500516
100. Vienken J. Polymers in nephrology characteristics and needs. Int J Artif Organs. (2002) 25:470–9. doi: 10.1177/039139880202500518
101. Said N, Lau WJ, Ho YC, Lim SK, Zainol Abidin MN, Ismail AF. A review of commercial developments and recent laboratory research of dialyzers and membranes for hemodialysis application. Membranes (Basel). (2021) 11. doi: 10.3390/membranes11100767
102. Zweigart C, Boschetti-De-Fierro A, Hulko M, Nilsson LG, Beck W, Storr M, et al. Medium cut-off membranes - closer to the natural kidney removal function. Int J Artif Organs. (2017) 40:328–34. doi: 10.5301/ijao.5000603
103. Miller JJ, Carter JA, Hill K, Desormeaux JS, Carter RN, Gaborski TR, et al. Free standing, large-area silicon nitride membranes for high toxin clearance in blood surrogate for small-format hemodialysis. Membranes (Basel). (2020) 10. doi: 10.3390/membranes10060119
104. Kim S, Feinberg B, Kant R, Chui B, Goldman K, Park J, et al. Diffusive silicon nanopore membranes for hemodialysis applications. PloS One. (2016) 11:e0159526. doi: 10.1371/journal.pone.0159526
105. Rode RP, Chung HH, Miller HN, Gaborski TR, Moghaddam S. Trilayer interlinked graphene oxide membrane for wearable hemodialyzer. Adv Mater Interfaces. (2021) 8. doi: 10.1002/admi.202001985
106. Ehlerding G, Erlenkötter A, Gauly A, Griesshaber B, Kennedy J, Rauber L, et al. Performance and hemocompatibility of a novel polysulfone dialyzer: a randomized controlled trial. Kidney360. (2021) 2:937. doi: 10.34067/KID.0000302021
107. Zawada AM, Melchior P, Erlenkoetter A, Delinski D, Stauss-Grabo M, Kennedy JP. Polyvinylpyrrolidone in hemodialysis membranes: Impact on platelet loss during hemodialysis. Hemodialysis Int. (2021) 25:498–506. doi: 10.1111/hdi.12939
108. Melchior P, Erlenkötter A, Zawada AM, Delinski D, Schall C, Stauss-Grabo M, et al. Complement activation by dialysis membranes and its association with secondary membrane formation and surface charge. Artif Organs. (2021) 45:770–8. doi: 10.1111/aor.13887
109. Ioannidis JP. Evidence-based medicine has been hijacked: a report to David Sackett. J Clin Epidemiol. (2016) 73:82–6. doi: 10.1016/j.jclinepi.2016.02.012
110. Concato J, Corrigan-Curay J. Real-world evidence-where are we now? New Engl J Med. (2022) 386(18):1680–2. doi: 10.1056/NEJMp2200089
111. Foreman KJ, Marquez N, Dolgert A, Fukutaki K, Fullman N, Mcgaughey M, et al. Forecasting life expectancy, years of life lost, and all-cause and cause-specific mortality for 250 causes of death: reference and alternative scenarios for 2016–40 for 195 countries and territories. Lancet. (2018) 392:2052–90. doi: 10.1016/S0140-6736(18)31694-5
112. MacEwen C, Watkinson P, Winearls C. Circuit life versus bleeding risk: the impact of achieved activated partial thromboplastin time versus achieved filtration fraction. Ther Apheresis Dialysis. (2015) 19:259–66. doi: 10.1111/tap.2015.19.issue-3
113. Warkentin TE, Greinacher A. Heparin-induced anaphylactic and anaphylactoid reactions: two distinct but overlapping syndromes. Expert Opin Drug Saf. (2009) 8:129–44. doi: 10.1517/14740330902778180
114. Fisher C, Shao H, Ho CH. Improved hemocompatibility of polysulfone hemodialyzers with Endexo® surface modifying molecules. J Biomed Materials Res Part B: Appl Biomaterials. (2022) 110:1335–43. doi: 10.1002/jbm.b.v110.6
115. Meyer JM, Steer D, Weber LA, Zeitone AA, Thakuria M, Ho C-H, et al. Safety of a novel dialyzer containing a fluorinated polyurethane surface-modifying macromolecule in patients with end-stage kidney disease. Blood Purification. (2021) 50:959–67. doi: 10.1159/000514937
116. Higgins M, Zhang L, Ford R, Brownlie J, Kleidon T, Rickard CM, et al. The microbial biofilm composition on peripherally inserted central catheters: a comparison of polyurethane and hydrophobic catheters collected from paediatric patients. J Vasc Access. (2021) 22:388–93. doi: 10.1177/1129729820932423
117. Saadati S, Westphalen H, Eduok U, Abdelrasoul A, Shoker A, Choi P, et al. Biocompatibility enhancement of hemodialysis membranes using a novel zwitterionic copolymer: Experimental, in situ synchrotron imaging, molecular docking, and clinical inflammatory biomarkers investigations. Materials Sci Engineering: C. (2020) 117:111301. doi: 10.1016/j.msec.2020.111301
118. Mollahosseini A, Abdelrasoul A, Shoker A. A critical review of recent advances in hemodialysis membranes hemocompatibility and guidelines for future development. Materials Chem Phys. (2020) 248:122911. doi: 10.1016/j.matchemphys.2020.122911
119. Abdelrasoul A, Westphalen H, Saadati S, Shoker A. Hemodialysis biocompatibility mathematical models to predict the inflammatory biomarkers released in dialysis patients based on hemodialysis membrane characteristics and clinical practices. Sci Rep. (2021) 11:23080. doi: 10.1038/s41598-021-01660-1
120. Westphalen H, Abdelrasoul A, Shoker A. Protein adsorption phenomena in hemodialysis membranes: Mechanisms, influences of clinical practices, modeling, and challenges. Colloid Interface Sci Commun. (2021) 40:100348. doi: 10.1016/j.colcom.2020.100348
121. Geremia I, Pavlenko D, Maksymow K, Rüth M, Lemke H, Stamatialis D. Ex vivo evaluation of the blood compatibility of mixed matrix haemodialysis membranes. Acta biomaterialia. (2020) 111:118–28. doi: 10.1016/j.actbio.2020.05.016
122. Tijink MS, Wester M, Glorieux G, Gerritsen KG, Sun J, Swart PC, et al. Mixed matrix hollow fiber membranes for removal of protein-bound toxins from human plasma. Biomaterials. (2013) 34:7819–28. doi: 10.1016/j.biomaterials.2013.07.008
123. Pavlenko D, Van Geffen E, Van Steenbergen MJ, Glorieux G, Vanholder R, Gerritsen KG, et al. New low-flux mixed matrix membranes that offer superior removal of protein-bound toxins from human plasma. Sci Rep. (2016) 6:34429. doi: 10.1038/srep34429
124. Wilmer MJ, Saleem MA, Masereeuw R, Ni L, Van Der Velden TJ, Russel FG, et al. Novel conditionally immortalized human proximal tubule cell line expressing functional influx and efflux transporters. Cell Tissue Res. (2010) 339:449–57. doi: 10.1007/s00441-009-0882-y
125. Geremia I, Bansal R, Stamatialis D. In vitro assessment of mixed matrix hemodialysis membrane for achieving endotoxin-free dialysate combined with high removal of uremic toxins from human plasma. Acta biomaterialia. (2019) 90:100–11. doi: 10.1016/j.actbio.2019.04.009
126. Geremia I, Jong JA, Van Nostrum CF, Hennink WE, Gerritsen KG, Stamatialis D. New mixed matrix membrane for the removal of urea from dialysate solution. Separation purification Technol. (2021) 277:119408. doi: 10.1016/j.seppur.2021.119408
127. Rizki-Safitri A, Traitteur T, Morizane R. Bioengineered kidney models: Methods and functional assessments. Function. (2021) 2:zqab026. doi: 10.1093/function/zqab026
128. Humes HD, Buffington D, Westover AJ, Roy S, Fissell WH. The bioartificial kidney: current status and future promise. Pediatr Nephrol. (2014) 29:343–51. doi: 10.1007/s00467-013-2467-y
129. van Gelder MK, Mihaila SM, Jansen J, Wester M, Verhaar MC, Joles JA, et al. From portable dialysis to a bioengineered kidney. Expert Rev Med Devices. (2018) 15:323–36.
130. Jansen J, De Napoli I, Fedecostante M, Schophuizen C, Chevtchik N, Wilmer M, et al. Human proximal tubule epithelial cells cultured on hollow fibers: living membranes that actively transport organic cations. Sci Rep. (2015) 5:1–12. doi: 10.1038/srep16702
131. Jansen J, Fedecostante M, Wilmer M, Peters J, Kreuser U, Van Den Broek P, et al. Bioengineered kidney tubules efficiently excrete uremic toxins. Sci Rep. (2016) 6:26715. doi: 10.1038/srep26715
132. Mihajlovic M, Van Den Heuvel LP, Hoenderop JG, Jansen J, Wilmer MJ, Westheim AJ, et al. Allostimulatory capacity of conditionally immortalized proximal tubule cell lines for bioartificial kidney application. Sci Rep. (2017) 7:1–14. doi: 10.1038/s41598-017-07582-1
133. Mihajlovic M, Fedecostante M, Oost MJ, Steenhuis SK, Lentjes EG, Maitimu-Smeele I, et al. Role of vitamin D in maintaining renal epithelial barrier function in uremic conditions. Int J Mol Sci. (2017) 18:2531. doi: 10.3390/ijms18122531
134. Mihajlovic M, Hariri S, Westphal KC, Janssen MJ, Oost MJ, Bongiovanni L, et al. Safety evaluation of conditionally immortalized cells for renal replacement therapy. Oncotarget. (2019) 10:5332. doi: 10.18632/oncotarget.v10i51
135. Basile C, Davenport A, Mitra S, Pal A, Stamatialis D, Chrysochou C, et al. Frontiers in hemodialysis: Innovations and technological advances. Artif Organs. (2021) 45:175–82. doi: 10.1111/aor.13798
136. Chevtchik NV, Fedecostante M, Jansen J, Mihajlovic M, Wilmer M, Rüth M, et al. Upscaling of a living membrane for bioartificial kidney device. Eur J Pharmacol. (2016) 790:28–35. doi: 10.1016/j.ejphar.2016.07.009
137. Porrett PM, Orandi BJ, Kumar V, Houp J, Anderson D, Cozette Killian A, et al. First clinical-grade porcine kidney xenotransplant using a human decedent model. Am J Transplant. (2022) 22:1037–53. doi: 10.1111/ajt.16930
138. Lu T, Yang B, Wang R, Qin C. Xenotransplantation: current status in preclinical research. Front Immunol. (2019) 10:3060. doi: 10.3389/fimmu.2019.03060
139. Loftus TJ, Shickel B, Ozrazgat-Baslanti T, Ren Y, Glicksberg BS, Cao J, et al. Artificial intelligence-enabled decision support in nephrology. Nat Rev Nephrol. (2022) 18:452–65. doi: 10.1038/s41581-022-00562-3
140. Chaudhuri S, Long A, Zhang H, Monaghan C, Larkin JW, Kotanko P, et al. Artificial intelligence enabled applications in kidney disease. Semin Dialysis. (2021) 34(1):5–16. doi: 10.1111/sdi.12915
141. Chaudhuri S, Han H, Usvyat L, Jiao Y, Sweet D, Vinson A, et al. Machine learning directed interventions associate with decreased hospitalization rates in hemodialysis patients. Int J Med Inf. (2021) 153:104541. doi: 10.1016/j.ijmedinf.2021.104541
142. Zhang H, Preddie D, Krackov W, Sor M, Waguespack P, Kuang Z, et al. Deep learning to classify arteriovenous access aneurysms in hemodialysis patients. Clin Kidney J. (2022) 15:829–30. doi: 10.1093/ckj/sfab278
143. Ohara T, Ikeda H, Sugitani Y, Suito H, Huynh VQH, Kinomura M, et al. Artificial intelligence supported anemia control system (AISACS) to prevent anemia in maintenance hemodialysis patients. Int J Med Sci. (2021) 18:1831. doi: 10.7150/ijms.53298
144. Hueso M, Vellido A, Montero N, Barbieri C, Ramos R, Angoso M, et al. Artificial intelligence for the artificial kidney: pointers to the future of a personalized hemodialysis therapy. Kidney Dis. (2018) 4:1–9. doi: 10.1159/000486394
145. Foley RN, Gilbertson DT, Murray T, Collins AJ. Long interdialytic interval and mortality among patients receiving hemodialysis. New Engl J Med. (2011) 365:1099–107. doi: 10.1056/NEJMoa1103313
146. Chazot C, Jean G. The advantages and challenges of increasing the duration and frequency of maintenance dialysis sessions. Nat Clin Pract Nephrol. (2009) 5:34–44. doi: 10.1038/ncpneph0979
147. Fissell R, Hakim RM. Improving outcomes by changing hemodialysis practice patterns. Curr Opin Nephrol Hypertension. (2013) 22:675–80. doi: 10.1097/MNH.0b013e328365b34c
148. Evans M, Xu H, Rydell H, Prütz K-G, Lindholm B, Stendahl M, et al. Association between implementation of novel therapies and improved survival in patients starting haemodialysis: the Swedish Renal Registry 2006–15. Nephrol Dialysis Transplant. (2021) 36:1298–306. doi: 10.1093/ndt/gfaa357
149. Klarenbach S, Manns B. Economic evaluation of dialysis therapies. Semin Nephrol. (2009) 29(5):524–32. doi: 10.1016/j.semnephrol.2009.06.009
150. Vanholder R, Agar J, Braks M, Gallego D, Gerritsen KG, Harber M, et al. The European Green Deal and nephrology: a call for action by the European Kidney Health Alliance. Nephrol Dialysis Transplant. (2023) 38:1080–8. doi: 10.1093/ndt/gfac160
151. Ramada DL, De Vries J, Vollenbroek J, Noor N, Ter Beek O, Mihăilă SM, et al. Portable, wearable and implantable artificial kidney systems: needs, opportunities and challenges. Nat Rev Nephrol. (2023) 19:1–10. doi: 10.1038/s41581-023-00726-9
152. Easy Water For Everyone. home (2023). Available online at: https://www.easywaterforeveryone.org/ (accessed September 23, 2024).
Keywords: dialysis membranes, innovation, biocompatibility, science, technology, clinical impact, chronic kidney disease
Citation: Wanner C, Vanholder R, Ortiz A, Davenport A, Canaud B, Blankestijn PJ, Masereeuw R, Kooman JP, Castellano G, Stamatialis D, Mitra S, Grooteman M, Weber V, Ebert T, Abdelrasoul A, Steppan S, Scheiwe AR and Stenvinkel P (2024) Proceedings of a membrane update symposium: advancements, scientific insights, and future trends for dialysis membranes for enhanced clinical outcomes in end stage kidney disease patients. Front. Nephrol. 4:1455260. doi: 10.3389/fneph.2024.1455260
Received: 26 June 2024; Accepted: 04 September 2024;
Published: 15 October 2024.
Edited by:
Vikram Sabapathy, University of Virginia, United StatesReviewed by:
Murat Dogan, University of Tennessee Health Science Center (UTHSC), United StatesAlexander Kirsch, Medical University of Graz, Austria
Shyam Bihari Bansal, Medanta The Medicity Hospital, India
Copyright © 2024 Wanner, Vanholder, Ortiz, Davenport, Canaud, Blankestijn, Masereeuw, Kooman, Castellano, Stamatialis, Mitra, Grooteman, Weber, Ebert, Abdelrasoul, Steppan, Scheiwe and Stenvinkel. This is an open-access article distributed under the terms of the Creative Commons Attribution License (CC BY). The use, distribution or reproduction in other forums is permitted, provided the original author(s) and the copyright owner(s) are credited and that the original publication in this journal is cited, in accordance with accepted academic practice. No use, distribution or reproduction is permitted which does not comply with these terms.
*Correspondence: Anna Rebecca Scheiwe, cmViZWNjYS5zY2hlaXdlQGZyZXNlbml1cy5jb20=; Rosalinde Masereeuw, ci5tYXNlcmVldXdAdXUubmw=
†ORCID: Thomas Ebert, orcid.org/0000-0003-1683-9276