- 1Service de Médecine Intensive Réanimation, Centre Hospitalier de Poissy-Saint Germain en Laye, Poissy, France
- 2Service de Réanimation, Hôpital Privé de l’Ouest Parisien, Ramsay Générale de Santé, Trappes, France
- 3INSERM UMR 1018, Equipe Epidémiologie Clinique, CESP, Villejuif, France
Acute kidney injury (AKI) is one of the most frequent causes of organ failure encountered in patients in the intensive care unit (ICU). Because of its predisposition to occur in the most critically ill patients, it is not surprising to observe a high frequency of AKI in patients with acute respiratory distress syndrome (ARDS). However, few studies have been carried out to assess the epidemiology of AKI in subgroups of ARDS patients using recommended KDIGO criteria. Moreover, the mechanisms involved in the physio-pathogenesis of AKI are still poorly understood, in particular the impact of mechanical ventilation on the kidneys. We carried out a review of the literature, focusing on the epidemiology and physiopathology of AKI in patients with ARDS admitted to the ICU. We addressed the importance of clinical management, focusing on mechanical ventilation for improving outcomes, on AKI. Finally, we also propose candidate treatment strategies and management perspectives. Our literature search showed that AKI is particularly common in ICU patients with ARDS. In association with the classic risk factors for AKI, such as comorbidities and iatrogeny, changes in mechanical ventilation parameters, which have been exclusively evaluated for their outcomes on respiratory function and death, must be considered carefully in terms of their impact on the short-term renal prognosis.
Introduction
Acute kidney injury (AKI) is the most common type of organ failure observed in patients in the intensive care unit (ICU) observed in its most severe form in about one-third of ICU patients (1). Surprisingly, few studies have assessed the relation between acute respiratory distress syndrome and AKI, and then only in specific contexts such as trauma (2), influenza (3), after lung transplantation (4), or intoxication (5). In an observational study specifically studied AKI in ARDS patients, 1879 patients were compared with 6150 patients without ARDS. The authors reported that 44% of ARDS patients had AKI and half of these had the most severe form. When compared to non-ARDS patients, this represented a 1.6-fold increase of incidence of AKI, with a strong statistical association between ventilation for ARDS and the occurrence of AKI after adjustment (OR=11[6.8-17.7]) (6). Using the most recent definition of AKI reported in the Kidney Disease Improving Global Outcome (KDIGO) guidelines, Panitchote et al. performed a competing risk survival analysis in a monocentric cohort of 634 ARDS patients. They observed AKI in more than two-thirds of patients and identified severe AKI in 118 (48%). Since December 2019, ICUs around the world have faced a significant increase in admissions for a particular form of ARDS, namely severe COVID-19. Marked by a particularly high rate of AKI [with a reported incidence of >80% in ICU patients receiving mechanical ventilation (MV)] (7) during the first wave in each country, a recent large observational database analysis from the UK observed a decrease in rate of AKI during successive waves in patients hospitalized with COVID-19 (8). Thus, the actual incidence of severe AKI appears to be around 25% and not to be greater than that in ARDS patients without COVID-19 (9). While AKI is associated with poor outcomes in all ICU patients (10, 11), the occurrence of renal failure during ARDS management appears to be a specific unfavorable risk factor for nosocomial pneumonia (12), an event leading to extended ventilation times (13), and for a lower rate of successful weaning from MV (14), which is responsible for an increase in overall mortality (10, 11),
The aim of this mini-review is to outline our current knowledge on the pathophysiological mechanisms involved in the development of AKI during the management of ARDS in ICU patients and to propose therapeutic approaches to limit its consequences.
Methods
We conducted a literature search of available sources describing AKI in patients with ARDS. Research articles were selected on the basis of research topics found in the Web of Science, PubMed, Springer, and Scopus databases. These articles were classified according to their relevancy. The information found in the selected articles was evaluated and is described and discussed in the following sections.
Existing Pathophysiological Models
Patients With ARDS Have a High Baseline Risk of AKI
Epidemiological studies focusing on AKI have highlighted several baseline characteristics as risk factors for renal failure (1, 15). Overall, patients with AKI are frequently male, older, with a history of comorbidities such as arterial hypertension, diabetes, and cardiovascular diseases (16). These characteristics are common to those observed in patients admitted to the ICU for ARDS in the LUNG-SAFE study. Taken together, these data give patients admitted to the ICU with ARDS a high-risk of renal events (Figure 1).
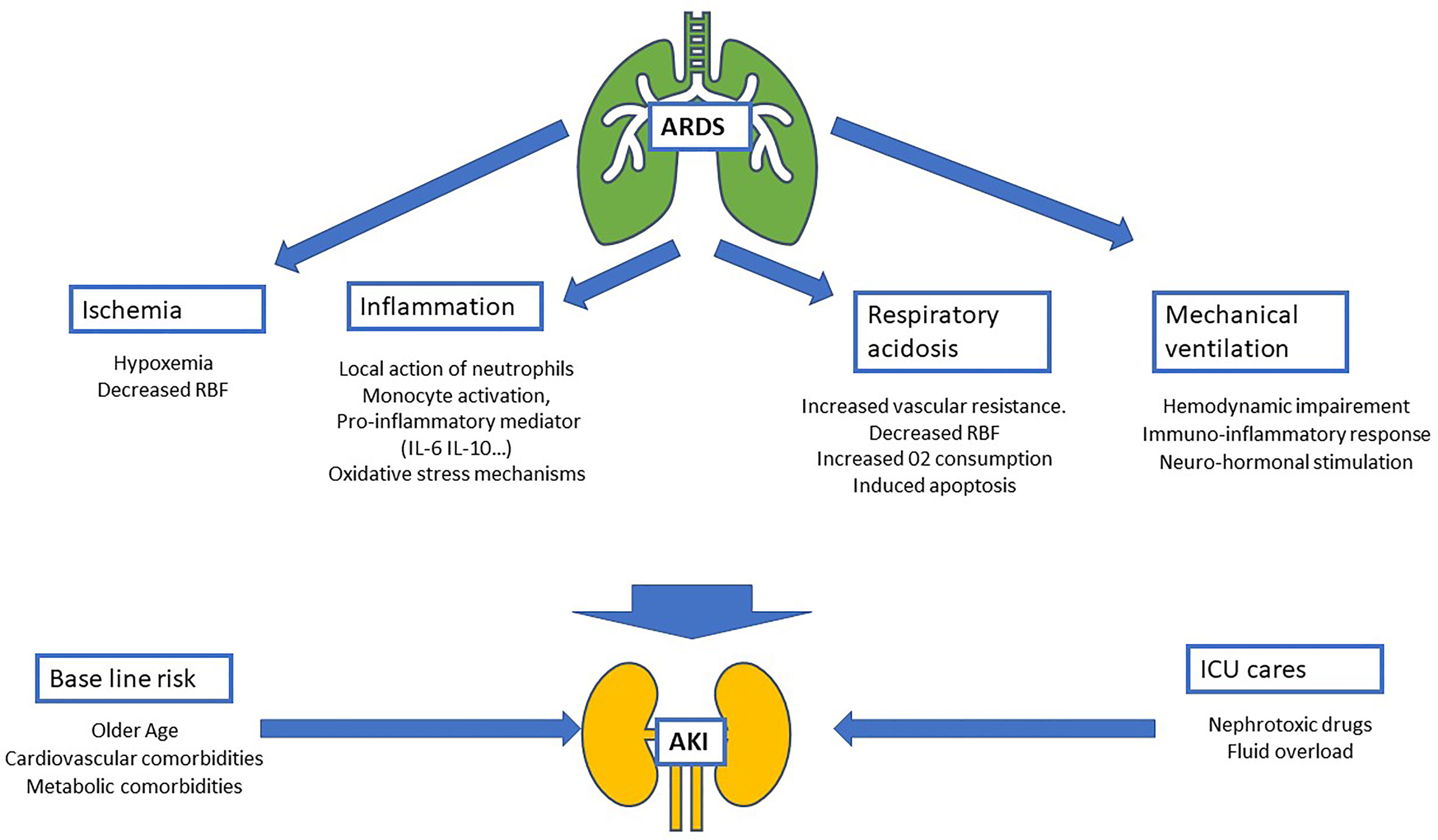
Figure 1 Schematic of physiopathology of acute kidney injury (AKI) during acute respiratory distress syndrome (ARDS). Four mechanisms related to ARDS are identified: ischemia, inflammatory, respiratory acidosis and the impact of mechanical ventilation. These mechanisms added to AKI baseline risk (mostly composed by comorbidities and age) and intensive care unit nephrotoxic treatment and procedures (nephrotoxic drugs, iodinated contrast products…). RBF, renal blood flow; IL-6, interleukin-6; IL-10, interleukin-10.
The “Usual Suspects” for ARDS-Associated AKI: Ischemia, Inflammation, MV, and Respiratory Acidosis
Ischemia
In kidney tissue, tubular proximal cells, which are metabolically hyperactive cells with a high demand for oxygen to reabsorb water and electrolytes, must operate in a low oxygen environment because of the cortex-to-inner oxygen gradient. During ARDS, renal ischemia results principally from hypoxia, defined by an arterial blood oxygen level <75 mmHg. A decrease in oxygen results in a mismatch between local tissue oxygen supply and demand, and the accumulation of metabolic waste products. As a consequence, tubular epithelial cells undergo injury and death by apoptosis and necrosis with functional impairment of the kidney (17).
In addition to this direct effect due to the decrease in blood oxygen levels, ARDS, when associated with shock, could decrease renal blood flow (RBF) leading to renal ischemia by hypoperfusion. Moreover, hypoxemia seems to have a synergistic effect on RBF and is associated with a greater change in renal function (18) (Figure 1).
Systemic Inflammation
It has been suggested that some particular etiologies of ARDS are associated with a higher incidence of AKI. More frequently observed in sepsis (19), and/or in acute pancreatitis (20), and/or burns, and less frequently observed in toxic inhalation and/or viral pneumonia (21, 22), this reinforces the hypothesis that inflammation causes renal injury.
Inflammation has been already identified as one of the major mechanisms leading to the development of AKI, particularly during sepsis with: (i) the local action of neutrophils (PNN) through the secretion of pro-inflammatory cytokines including interleukin (IL)-1β and tumor necrosis factor (TNF)-α (23); (ii) monocyte activation, both pro- and anti-inflammatory, responsible for monocyte infiltration guided by chemokine receptor expression in the kidney (24); and (iii) the impact of a diffuse inflammatory state with significant renal toxicity of the immune response, in particular innate immunity (25).
Two pro-inflammatory mediators, IL-6 and IL-10, have been specifically identified as being involved in the development of renal lesions in murine models of sepsis (26, 27). The link between high IL-6 and IL-10 plasma concentrations and the development of AKI has also been confirmed in humans (25). Other inflammatory biomarkers also seem to participate in the development of renal dysfunction, such as TNF-21 (28), IL-8 (29), and vascular endothelial growth factor (VEGF) hypersecretion, via oxidative stress mechanisms (30).
In ARDS, lung injury is responsible for the release of pro-inflammatory mediators similar to sepsis (IL-6, PAI-1, soluble TNF receptors) (11). Systemic release of these inflammatory mediators has been identified as a pathway involved in AKI development during infectious lung injury (11, 31) (Figure 1).
Mechanical Ventilation
In addition to the impact of MV on pulmonary tissue, positive pressure ventilation also seems to have an impact on the kidney. Originally described in 1947 by the demonstration of a decrease in urinary flow in healthy volunteers (32), several fundamental studies have suggested the involvement of hemodynamic (33, 34), immuno-inflammatory (35–37), and neuro-hormonal (38–40) mechanisms that may alter renal function after the MV initiation.
The hemodynamic impact of MV results from interactions between the heart, kidneys, and lungs. The introduction of positive-end expiratory pressure (PEEP) by MV is not without consequences. The increase in intrathoracic pressure leads to a decrease in heart venous return and, as a consequence, a decrease in cardiac output (41–43). More recently, in a large cohort of ICU patients, a strong link was found between the PEEP level administered through MV and AKI in association with higher venous congestion pressure. The authors hypothesized that high PEEP could disturb renal perfusion via mechanisms linked to an alteration in mean arterial pressure and by an increase in induced venous congestion (44). In addition to the hemodynamic impact, MV was suspected to stimulate the renin angiotensin aldosterone system (RAAS), with a PEEP level-dependent effect (38). In addition to RASS, other hormonal responses are also altered during MV, especially the secretion of antidiuretic hormone and type A natriuretic peptide, without any formal link being established between these responses and the development of AKI (38, 45, 46).
Added to the effects of pulmonary damage related to ARDS, MV also induces pro-inflammatory mechanisms that have a direct impact on renal tissue through neutrophil activation in the intrapulmonary circulation (47) and/or pro-inflammatory cytokine release in animal models (48). In humans, the interaction with initial inflammation associated with ARDS makes it difficult to extrapolate these results, but several pro-inflammatory mediators seem to be increased by MV (TNF-α, IL-6, IL-8, PAI-1, TNF receptor(R)-1, and TNFR) (11, 31, 49) (Figure 1).
Respiratory Acidosis
Over the past 10 years, a consensus has emerged concerning the need to limit plateau pressure during MV while maintaining a lung recruitment strategy with limitation of tidal volume (50). The so-called permissive hypercapnic acidosis observed at the “price” of this strategy has even been suggested to be one of the benefits of lung-protective ventilation (51). However, this point of view was challenged by several studies which suggested that hypercapnia may impair renal hemodynamics. In particular, it has been found that hypercapnia may induce vasoconstriction of the renal vascular bed leading to increased vascular resistance (52–54). A pCO2 threshold of 50 mmHg has even been identified as being responsible for the decrease in RBF (53, 55). In terms of cellular metabolism, respiratory acidosis may induce an increase in oxygen consumption in the proximal tubule (54, 56, 57). Furthermore, the hypercapnia/hypoxia combination has been shown to be synergistic in decreasing RBF and the development of ischemic apoptosis in renal tubule cells (58–61) (Figure 1).
The Special Case of Severe COVID-19
The relationship between renal and pulmonary involvement in a specific model of ARDS has been questioned in the context of the current SARS-COV-2 pandemic. Although renal damage is the second most frequent type of organ damage during COVID-19 (62), a histopathological study showed that acute tubular necrosis was the main lesion observed in ICU patients, without viral material identified in kidney lesions (63), suggesting a predominant ischemic process for AKI. Recently, Sullivan et al. reported a dramatic decrease in rates of AKI after the first wave of COVID-19, and a lower rate of renal failure in older patients (8). Because of recommendations early during the pandemic, in particular warnings of the potential risk of aerosol formation that could increase the risk of contamination for healthcare workers, non-invasive ventilation (NIV) and high-flow nasal cannula oxygen (HFNO) therapy were used in only a minority of patients during the first wave (6). It was later shown that the risk of aerosolization with HFNO and NIV was similar to that with standard oxygen therapy and HFNO was suggested as first-line ventilatory support in COVID-19 patients with acute respiratory failure during subsequent waves of the pandemic (7). The lower rate of AKI observed after the first wave and in older patients, in whom the use of invasive ventilatory support was probably low, suggests the impact of MV on the development of AKI. These results are in line with those reporting MV as a major risk factor for AKI in patients with severe COVID-19 (7, 9, 63).
The Usual Culprit, Septic Shock
Septic shock is frequently complicated by ARDS and is also a risk factor for AKI. Bellani et al. found an incidence of extrapulmonary septic shock in ICU patients with ARDS of 17.3% (16). The diffuse inflammation resulting from sepsis is responsible for a renal toxicity of the immune response (25), via pro-inflammatory cytokines (IL-6, IL-10, IL-1β, TNF-α) (23) and a monocytic infiltration of renal parenchym (24). Furthermore, hemodynamic failure in sepsis is linked to the occurrence of AKI (64). The SEPSIS-PAM trial confirms the importance of the link between blood pressure and AKI in sepsis (65). Vasopressors can improve filtration fraction, and normalize renal hemodynamic (66) but sympathetic overstimulation with high doses of catecholamines could be harmful (67).
Managing AKI in Patients With ARDS
“AKI Is Coming”
The cornerstone of AKI prevention in ICU patients is the management of hemodynamics, including the use of appropriate volumes of fluids, the choice of fluids, and the choice of vasoactive drugs. Although the pathogenesis of AKI in ARDS patients depends on different mechanisms, hemodynamic optimization appears to be essential to prevent alterations in RBF (Table 1).
Hemodynamic Management
Appropriate volume replacement should be performed as early as possible, bearing in mind that fluid overload is reported to be associated with a poor prognosis in ARDS patients (68). Crystalloids are the solutions of choice for ICU patients (69, 70). Because of the supra-physiological concentration of chloride (154 mmol/L; representing 1.5-times normal serum chloride concentrations), 0.9% saline solution had been incriminated in the development of hyperchloremia acidosis in patients receiving high volumes of solution. The results from a recent meta-analysis of 13 trials comprising more than 35000 patients suggested a better global and renal outcome with a balanced solution compared to 0.9% saline solution (OR of major adverse kidney events occurring in the first 30 days=0.78[0.66-0.91]; I2 = 42%) (71).
Further to the choice of solute, the concept of target optimal mean arterial pressure (mABP) has long been advocated. In the EPI-AKI study, the factors associated with AKI included a past medical history of hypertension or shock at ICU admission, with a higher simplified acute physiology score 3 (1). This relationship was demonstrated in physiological studies, which strongly suggested that glomerular filtration rate and RBF can vary widely across mABP and the impact of increasing mABP on renal hemodynamics varies on an individual basis (72) (Table 1).
Improve the Balance Between Oxygen Supply/Needs
Numerous other procedures aimed at improving intra-renal perfusion or oxygenation have been evaluated, including renal vasodilators, control of renal hypercatabolism, anti-inflammatory and antioxidants drugs. Among them, dopamine is undoubtedly the most extensively studied. Its administration at low doses induced a dopaminergic and β-adrenergic effect, resulting in renal vasodilation. However, despite intensive research, the data on preventing the development of AKI remain largely inconclusive (73). Other vasodilators have failed to show any renal benefit (74–77). Erythropoietin, steroids, tight glucose control, and numerous metabolic interventions have also been evaluated for the prevention of kidney damage in various conditions. Except for the control of blood glucose level, for which conflicting results have been obtained (78, 79), no renal benefit has been observed with any of these metabolic interventions (80–82) (Table 1).
Kidney-Lung Protective Ventilation
In the more specific context of ARDS, optimization of ventilation parameters to limit the impact of MV on renal hemodynamics as much as possible, through venous congestion or right-sided heart failure, seem to be an interesting approach to prevent this serious complication, within the limits allowed by the patient’s respiratory severity and oxygenation needs (83). Right ventricular (RV) function is frequently altered in ARDS patients with an incidence of acute cor pulmonale of 25%, resulting in detrimental hemodynamic consequences (84). The choice of PEEP strategy is still under debate. While some authors have observed a respiratory improvement with high level PEEP strategy (85), others have reported that aiming for higher levels of PEEP worsened RV systolic function impairment and a lower PEEP application reduced RV dysfunction, suggesting an association with lower pulmonary vascular resistance (86). In a prospective physiological study, Mekontso Dessap et al. showed that increasing PEEP strategies at constant Pplat during severe ARDS induced marked hypercapnia, lower pH values and was associated with impaired RV function (83). These results suggest that lung protective ventilation should be adopted gradually to limit hypercapnia to improve RV function and renal hemodynamics in at-risk patients (Table 1).
Bundles
Further to a single intervention, “bundles” have been proposed to prevent AKI (87, 88). Bundles are a small, straightforward set of evidence-based practices that have been proven to improve patient outcomes when performed collectively and reliably. This seems to allow better recognition (89) and reduce the risk of AKI progression (90). The implementation of bundles has been shown to result in a lower incidence of AKI in specific settings, such as nephrotoxic AKI or post-cardiac surgery (91, 92). However, it is unknown whether implementing these bundles in general ICU populations or in patients with sepsis could prevent AKI (Table 1).
During AKI: Limiting the Severity and Improving Early Recovery From AKI
Earlier Detection of Renal Damage
Screening, mainly by identifying pre-existing co-morbidities, will help to identify patients who are at risk of renal damage. Early detection of AKI is also necessary so that it can be managed more effectively. Since creatinine levels can increase late in the course of kidney damage, with thresholds being exceeded only after a 50% loss of renal function, the use of more sensitive markers to detect subclinical AKI is required. Early markers of tubular damage (tissue inhibitor of metalloproteinase 2, insulin-like growth factor-binding protein 7, kidney injury molecule-1, neutrophil gelatinase-associated lipocalin, IL-18) have been identified but are not yet available routinely (93). Biomarkers are supplemented by radiological examination with contrast ultrasound techniques to improve the assessment of lesion severity and recovery potential (94).
Activation of the PGC1α-NAD Pathway
While no specific treatment for AKI is currently available, numerous advances in our understanding of the mechanisms leading to AKI in ischemic conditions have been made over the past few years. Among them, the PPAR gamma coactivator 1α nicotinamide adenine dinucleotide (PGC1α-NAD) pathway is one of the most promising targets to prevent AKI. As renal proximal tubular cells are one of the most energy or ATP demanding cells in the body, they are very dependent on mitochondrial function. An increase in the expression of PGC1α in renal epithelial cells subjected to ischemic stress was found to be protective, with an increase in NAD+ (95, 96). Furthermore, decreased expression of PGC1α was observed in kidney biopsies from patients with AKI (96). PPAR agonists have been proposed to prevent AKI induced ischemia-reperfusion (97). Nicotinamide, the amine form of vitamin B3 (niacin), was identified as a potential stimulator of NAD+ production (98). After promising preclinical experiments, nicotinamide administration was evaluated for the prevention of postoperative AKI after in a single center trial with encouraging results (99). In this phase 1 pilot study, 37 patients who had undergone cardiac surgery were randomly assigned to one of three groups: placebo, nicotinamide 1g/day, and nicotinamide 3g/day. The areas under the curve of all longitudinal serum creatinine levels measured after randomization were higher in the placebo group compared to patients who received nicotinamide supplementation. While these results deserve to be reassessed in larger populations with a more suitable outcome, emerging data linking the NAD+ equilibrium to AKI resistance opens a new exciting chapter in AKI research (98, 100).
Renal Replacement Therapy: At the Right Time for the Right Patient
Over the past few years, the timing of renal replacement therapy has received particular attention in critically ill patients (101–105). ARDS patients were studied specifically in a post-hoc analysis of an AKI study, but no significant impact of early renal replacement therapy on 60-day mortality was observed (101). Concerning renal recovery, although higher renal replacement therapy-dependence among survivors at day-90 was observed in the STARRT-AKI study (85/814 (10.4%) for the accelerated group vs. 49/815 (6.0%) for the standard group), these data were observed in unselected ICU patients and are difficult to extrapolate to the ARDS population.
Conclusion
AKI is a frequent complication in ARDS patients and has a significant impact on outcome. MV may favor the emergence of this complication. Although the prevention of renal failure remains difficult, the early identification of high-risk patients, the use of bundles, and personalized ventilation should be an integral part of the management of ARDS patients, in the absence of any new therapeutic targets.
Author Contributions
AM performed review of the literature and designed figure. AM and MJ wrote the manuscript. All authors read and approved the final manuscript. The authors received no financial support for the research, authorship, and publication of this article.
Conflict of Interest
MJ reports lectures fees from Alexion.
The remaining author declares that the research was conducted in the absence of any commercial or financial relationships that could be construed as a potential conflict of interest.
Publisher’s Note
All claims expressed in this article are solely those of the authors and do not necessarily represent those of their affiliated organizations, or those of the publisher, the editors and the reviewers. Any product that may be evaluated in this article, or claim that may be made by its manufacturer, is not guaranteed or endorsed by the publisher.
References
1. Hoste EAJ, Bagshaw SM, Bellomo R, Cely CM, Colman R, Cruz DN, et al. Epidemiology of Acute Kidney Injury in Critically Ill Patients: The Multinational AKI-EPI Study. Intensive Care Med (2015) 41(8):1411–23. doi: 10.1007/s00134-015-3934-7
2. Vivino G, Antonelli M, Moro ML, Cottini F, Conti G, Bufi M, et al. Risk Factors for Acute Renal Failure in Trauma Patients. Intensive Care Med (1998) 24(8):808–14. doi: 10.1007/s001340050670
3. Jung JY, Park BH, Hong SB, Koh Y, Suh GY, Jeon K, et al. Acute Kidney Injury in Critically Ill Patients With Pandemic Influenza A Pneumonia 2009 in Korea: A Multicenter Study. J Crit Care (2011) 26(6):577–85. doi: 10.1016/j.jcrc.2011.02.012
4. Rocha PN, Rocha AT, Palmer SM, Davis RD, Smith SR. Acute Renal Failure After Lung Transplantation: Incidence, Predictors and Impact on Perioperative Morbidity and Mortality. Am J Transplant (2005) 5(6):1469–76. doi: 10.1111/j.1600-6143.2005.00867.x
5. O’Riordan A, Brummell Z, Sizer E, Auzinger G, Heaton N, O’Grady JG, et al. Acute Kidney Injury in Patients Admitted to a Liver Intensive Therapy Unit With Paracetamol-Induced Hepatotoxicity. Nephrol Dial Transplant (2011) 26(11):3501–8. doi: 10.1093/ndt/gfr050
6. Darmon M, Clec’h C, Adrie C, Argaud L, Allaouchiche B, Azoulay E, et al. Acute Respiratory Distress Syndrome and Risk of AKI Among Critically Ill Patients. Clin J Am Soc Nephrol (2014) 9(8):1347–53. doi: 10.2215/CJN.08300813
7. Hirsch JS, Ng JH, Ross DW, Sharma P, Shah HH, Barnett RL, et al. Acute Kidney Injury in Patients Hospitalized With COVID-19. Kidney Int (2020) 98(1):209–18. doi: 10.1016/j.kint.2020.05.006
8. Sullivan MK, Lees JS, Drake TM, Docherty AB, Oates G, Hardwick HE, et al. Acute Kidney Injury in Patients Hospitalized With COVID-19 From the ISARIC WHO CCP-UK Study: A Prospective, Multicentre Cohort Study. Nephrol Dial Transplant (2022) 37(2):271–84. doi: 10.1093/ndt/gfab303
9. Geri G, Darmon M, Zafrani L, Fartoukh M, Voiriot G, Le Marec J, et al. Acute Kidney Injury in SARS-CoV2-Related Pneumonia ICU Patients: A Retrospective Multicenter Study. Ann Intensive Care (2021) 11(1):86. doi: 10.1186/s13613-021-00875-9
10. Cooke CR, Kahn JM, Caldwell E, Okamoto VN, Heckbert SR, Hudson LD, et al. Predictors of Hospital Mortality in a Population-Based Cohort of Patients With Acute Lung Injury. Crit Care Med (2008) 36(5):1412–20. doi: 10.1097/CCM.0b013e318170a375
11. Liu KD, Glidden DV, Eisner MD, Parsons PE, Ware LB, Wheeler A, et al. Predictive and Pathogenetic Value of Plasma Biomarkers for Acute Kidney Injury in Patients With Acute Lung Injury. Crit Care Med (2007) 35(12):2755–61.
12. Mehta RL, Bouchard J, Soroko SB, Ikizler TA, Paganini EP, Chertow GM, et al. Sepsis as a Cause and Consequence of Acute Kidney Injury: Program to Improve Care in Acute Renal Disease. Intensive Care Med (2011) 37(2):241–8. doi: 10.1007/s00134-010-2089-9
13. Dill J, Bixby B, Ateeli H, Sarsah B, Goel K, Buckley R, et al. Renal Replacement Therapy in Patients With Acute Respiratory Distress Syndrome: A Single-Center Retrospective Study. Int J Nephrol Renovasc Dis (2018) 11:249–57. doi: 10.2147/IJNRD.S164628
14. Vieira JM, Castro I, Curvello-Neto A, Demarzo S, Caruso P, Pastore L, et al. Effect of Acute Kidney Injury on Weaning From Mechanical Ventilation in Critically Ill Patients. Crit Care Med (2007) 35(1):184–91. doi: 10.1097/01.CCM.0000249828.81705.65
15. Kellum JA, Sileanu FE, Bihorac A, Hoste EAJ, Chawla LS. Recovery After Acute Kidney Injury. Am J Respir Crit Care Med (2017) 195(6):784–91. doi: 10.1164/rccm.201604-0799OC
16. Bellani G, Laffey JG, Pham T, Fan E, Brochard L, Esteban A, et al. Epidemiology, Patterns of Care, and Mortality for Patients With Acute Respiratory Distress Syndrome in Intensive Care Units in 50 Countries. JAMA (2016) 315(8):788–800. doi: 10.1001/jama.2016.0291
17. Bonventre JV, Yang L. Cellular Pathophysiology of Ischemic Acute Kidney Injury. J Clin Invest (2011) 121(11):4210–21. doi: 10.1172/JCI45161
18. Darmon M, Schortgen F, Leon R, Moutereau S, Mayaux J, Di Marco F, et al. Impact of Mild Hypoxemia on Renal Function and Renal Resistive Index During Mechanical Ventilation. Intensive Care Med (2009) 35(6):1031–8. doi: 10.1007/s00134-008-1372-5
19. Poston JT, Koyner JL. Sepsis Associated Acute Kidney Injury. BMJ (2019) 364:k4891. doi: 10.1136/bmj.k4891
20. Nassar TI, Qunibi WY. AKI Associated With Acute Pancreatitis. Clin J Am Soc Nephrol (2019) 14(7):1106–15. doi: 10.2215/CJN.13191118
21. Dépret F, Hoffmann C, Daoud L, Thieffry C, Monplaisir L, Creveaux J, et al. Association Between Hydroxocobalamin Administration and Acute Kidney Injury After Smoke Inhalation: A Multicenter Retrospective Study. Crit Care (2019) 23(1):421. doi: 10.1186/s13054-019-2706-0
22. Pettilä V, Webb SAR, Bailey M, Howe B, Seppelt IM, Bellomo R. Acute Kidney Injury in Patients With Influenza A (H1N1) 2009. Intensive Care Med (2011) 37(5):763–7. doi: 10.1007/s00134-011-2166-8
23. Edelstein CL, Ling H, Schrier RW. The Nature of Renal Cell Injury. Kidney Int (1997) 51(5):1341–51. doi: 10.1038/ki.1997.183
24. Chousterman BG, Boissonnas A, Poupel L, Baudesson de Chanville C, Adam J, Tabibzadeh N, et al. Ly6Chigh Monocytes Protect Against Kidney Damage During Sepsis via a CX3CR1-Dependent Adhesion Mechanism. J Am Soc Nephrol (2016) 27(3):792–803. doi: 10.1681/ASN.2015010009
25. Payen D, Lukaszewicz AC, Legrand M, Gayat E, Faivre V, Megarbane B, et al. A Multicentre Study of Acute Kidney Injury in Severe Sepsis and Septic Shock: Association With Inflammatory Phenotype and HLA Genotype. PloS One (2012) 7(6):e35838. doi: 10.1371/journal.pone.0035838
26. Nechemia-Arbely Y, Barkan D, Pizov G, Shriki A, Rose-John S, Galun E, et al. IL-6/IL-6R Axis Plays a Critical Role in Acute Kidney Injury. J Am Soc Nephrol (2008) 19(6):1106–15. doi: 10.1681/ASN.2007070744
27. Su H, Lei CT, Zhang C. Interleukin-6 Signaling Pathway and Its Role in Kidney Disease: An Update. Front Immunol (2017) 8:405. doi: 10.3389/fimmu.2017.00405
28. Cunningham PN, Dyanov HM, Park P, Wang J, Newell KA, Quigg RJ. Acute Renal Failure in Endotoxemia Is Caused by TNF Acting Directly on TNF Receptor-1 in Kidney. J Immunol (2002) 168(11):5817–23. doi: 10.4049/jimmunol.168.11.5817
29. Liangos O, Kolyada A, Tighiouart H, Perianayagam MC, Wald R, Jaber BL. Interleukin-8 and Acute Kidney Injury Following Cardiopulmonary Bypass: A Prospective Cohort Study. Nephron Clin Pract (2009) 113(3):c148–54. doi: 10.1159/000232595
30. Xu Y, Jiang W, Zhong L, Li H, Bai L, Chen X, et al. miR-195-5p Alleviates Acute Kidney Injury Through Repression of Inflammation and Oxidative Stress by Targeting Vascular Endothelial Growth Factor A. Aging (Albany NY) (2020) 12(11):10235–45. doi: 10.18632/aging.103160
31. Murugan R, Karajala-Subramanyam V, Lee M, Yende S, Kong L, Carter M, et al. Acute Kidney Injury in Non-Severe Pneumonia Is Associated With an Increased Immune Response and Lower Survival. Kidney Int (2010) 77(6):527–35. doi: 10.1038/ki.2009.502
32. Drury DR, Henry JP, Goodman J. The Effects of Continuous Pressure Breathing on Kidney Function. J Clin Invest (1947) 26(5):945–51. doi: 10.1172/JCI101889
33. Schefold JC, Filippatos G, Hasenfuss G, Anker SD, von Haehling S. Heart Failure and Kidney Dysfunction: Epidemiology, Mechanisms and Management. Nat Rev Nephrol (2016) 12(10):610–23. doi: 10.1038/nrneph.2016.113
34. Koyner JL, Murray PT. Mechanical Ventilation and Lung-Kidney Interactions. Clin J Am Soc Nephrol (2008) 3(2):562–70. doi: 10.2215/CJN.03090707
35. Imai Y, Parodo J, Kajikawa O, de Perrot M, Fischer S, Edwards V, et al. Injurious Mechanical Ventilation and End-Organ Epithelial Cell Apoptosis and Organ Dysfunction in an Experimental Model of Acute Respiratory Distress Syndrome. JAMA (2003) 289(16):2104–12. doi: 10.1001/jama.289.16.2104
36. Ranieri VM, Suter PM, Tortorella C, De Tullio R, Dayer JM, Brienza A, et al. Effect of Mechanical Ventilation on Inflammatory Mediators in Patients With Acute Respiratory Distress Syndrome: A Randomized Controlled Trial. JAMA (1999) 282(1):54–61. doi: 10.1001/jama.282.1.54
37. Gurkan OU, O’Donnell C, Brower R, Ruckdeschel E, Becker PM. Differential Effects of Mechanical Ventilatory Strategy on Lung Injury and Systemic Organ Inflammation in Mice. Am J Physiol Lung Cell Mol Physiol (2003) 285(3):L710–718. doi: 10.1152/ajplung.00044.2003
38. Annat G, Viale JP, Bui Xuan B, Hadj Aissa O, Benzoni D, Vincent M, et al. Effect of PEEP Ventilation on Renal Function, Plasma Renin, Aldosterone, Neurophysins and Urinary ADH, and Prostaglandins. Anesthesiology (1983) 58(2):136–41. doi: 10.1097/00000542-198302000-00006
39. Gauer OH, Henry JP, Sieker HO, Wendt WE. The Effect of Negative Pressure Breathing on Urine Flow. J Clin Invest (1954) 33(2):287–96. doi: 10.1172/JCI102897
40. Farge D, de la Coussaye JE, Beloucif S, Fratacci MD, Payen DM. Interactions Between Hemodynamic and Hormonal Modifications During PEEP-Induced Antidiuresis and Antinatriuresis. Chest (1995) 107(4):1095–100. doi: 10.1378/chest.107.4.1095
41. Ranieri VM, Giunta F, Suter PM, Slutsky AS. Mechanical Ventilation as a Mediator of Multisystem Organ Failure in Acute Respiratory Distress Syndrome. JAMA (2000) 284(1):43–4. doi: 10.1001/jama.284.1.43
42. Slutsky AS, Tremblay LN. Multiple System Organ Failure. Is Mechanical Ventilation a Contributing Factor? Am J Respir Crit Care Med (1998) 157(6 Pt 1):1721–5. doi: 10.1164/ajrccm.157.6.9709092
43. Husain-Syed F, Slutsky AS, Ronco C. Lung-Kidney Cross-Talk in the Critically Ill Patient. Am J Respir Crit Care Med (2016) 194(4):402–14. doi: 10.1164/rccm.201602-0420CP
44. Geri G, Ferrer L, Tran N, Celi LA, Jamme M, Lee J, et al. Cardio-Pulmonary-Renal Interactions in ICU Patients. Role of Mechanical Ventilation, Venous Congestion and Perfusion Deficit on Worsening of Renal Function: Insights From the MIMIC-III Database. J Crit Care (2021) 64:100–7. doi: 10.1016/j.jcrc.2021.03.013
45. Pannu N, Mehta RL. Effect of Mechanical Ventilation on the Kidney. Best Pract Res Clin Anaesthesiol (2004) 18(1):189–203. doi: 10.1016/j.bpa.2003.08.002
46. Hemmer M, Viquerat CE, Suter PM, Vallotton MB. Urinary Antidiuretic Hormone Excretion During Mechanical Ventilation and Weaning in Man. Anesthesiology (1980) 52(5):395–400. doi: 10.1097/00000542-198005000-00004
47. Sugahara S, Suzuki H. Early Start on Continuous Hemodialysis Therapy Improves Survival Rate in Patients With Acute Renal Failure Following Coronary Bypass Surgery. Hemodial Int (2004) 8(4):320–5. doi: 10.1111/j.1492-7535.2004.80404.x
48. von Bethmann AN, Brasch F, Nüsing R, Vogt K, Volk HD, Müller KM, et al. Hyperventilation Induces Release of Cytokines From Perfused Mouse Lung. Am J Respir Crit Care Med (1998) 157(1):263–72. doi: 10.1164/ajrccm.157.1.9608052
49. Meduri GU, Kohler G, Headley S, Tolley E, Stentz F, Postlethwaite A. Inflammatory Cytokines in the BAL of Patients With ARDS. Persistent Elevation Over Time Predicts Poor Outcome. Chest (1995) 108(5):1303–14. doi: 10.1378/chest.108.5.1303
50. Ventilation With Lower Tidal Volumes as Compared With Traditional Tidal Volumes for Acute Lung Injury and the Acute Respiratory Distress Syndrome. N Engl J Med (2000) 342(18):1301–8. doi: 10.1056/NEJM200005043421801
51. Hickling KG. Lung-Protective Ventilation in Acute Respiratory Distress Syndrome: Protection by Reduced Lung Stress or by Therapeutic Hypercapnia? Am J Respir Crit Care Med (2000) 162(6):2021–2. doi: 10.1164/ajrccm.162.6.ed12-00d
52. Thorens JB, Jolliet P, Ritz M, Chevrolet JC. Effects of Rapid Permissive Hypercapnia on Hemodynamics, Gas Exchange, and Oxygen Transport and Consumption During Mechanical Ventilation for the Acute Respiratory Distress Syndrome. Intensive Care Med (1996) 22(3):182–91. doi: 10.1007/BF01712235
53. Sharkey RA, Mulloy EM, Kilgallen IA, O’Neill SJ. Renal Functional Reserve in Patients With Severe Chronic Obstructive Pulmonary Disease. Thorax (1997) 52(5):411–5. doi: 10.1136/thx.52.5.411
54. Barnes T, Zochios V, Parhar K. Re-Examining Permissive Hypercapnia in ARDS: A Narrative Review. Chest (2018) 154(1):185–95. doi: 10.1016/j.chest.2017.11.010
55. Howes TQ, Deane CR, Levin GE, Baudouin SV, Moxham J. The Effects of Oxygen and Dopamine on Renal and Aortic Blood Flow in Chronic Obstructive Pulmonary Disease With Hypoxemia and Hypercapnia. Am J Respir Crit Care Med (1995) 151(2 Pt 1):378–83. doi: 10.1164/ajrccm.151.2.7842195
56. Edwards A, Palm F, Layton AT. A Model of Mitochondrial O2 Consumption and ATP Generation in Rat Proximal Tubule Cells. Am J Physiol Renal Physiol (2020) 318(1):F248–59. doi: 10.1152/ajprenal.00330.2019
57. Aubert V, Kaminski J, Guillaud F, Hauet T, Hannaert P. A Computer Model of Oxygen Dynamics in the Cortex of the Rat Kidney at the Cell-Tissue Level. Int J Mol Sci (2019) 20(24):6246. doi: 10.3390/ijms20246246
58. Rose CE, Kimmel DP, Godine RL, Kaiser DL, Carey RM. Synergistic Effects of Acute Hypoxemia and Hypercapnic Acidosis in Conscious Dogs. Renal Dysfunction and Activation of the Renin-Angiotensin System. Circ Res (1983) 53(2):202–13. doi: 10.1161/01.res.53.2.202
59. Rose CE, Anderson RJ, Carey RM. Antidiuresis and Vasopressin Release With Hypoxemia and Hypercapnia in Conscious Dogs. Am J Physiol (1984) 247(1 Pt 2):R127–134. doi: 10.1152/ajpregu.1984.247.1.R127
60. Rose CE, Peach MJ, Carey RM. Role of Angiotensin II in Renal Vasoconstriction With Acute Hypoxemia and Hypercapnic Acidosis in Conscious Dogs. Ren Fail (1994) 16(2):229–42. doi: 10.3109/08860229409044863
61. Hotter G, Palacios L, Sola A. Low O2 and High CO2 in LLC-PK1 Cells Culture Mimics Renal Ischemia-Induced Apoptosis. Lab Invest (2004) 84(2):213–20. doi: 10.1038/labinvest.3700026
62. COVID-ICU Group on behalf of the REVA Network and the COVID-ICU Investigators. Clinical Characteristics and Day-90 Outcomes of 4244 Critically Ill Adults With COVID-19: A Prospective Cohort Study. Intensive Care Med (2021) 47(1):60–73. doi: 10.1007/s00134-020-06294-x
63. Ferlicot S, Jamme M, Gaillard F, Oniszczuk J, Couturier A, May O, et al. The Spectrum of Kidney Biopsies in Hospitalized Patients With COVID-19, Acute Kidney Injury, and/or Proteinuria. Nephrol Dial Transplant (2021) 2021:gfab042. doi: 10.1093/ndt/gfab042
64. Poukkanen M, Wilkman E, Vaara ST, Pettilä V, Kaukonen K-M, Korhonen A-M, et al. Hemodynamic Variables and Progression of Acute Kidney Injury in Critically Ill Patients With Severe Sepsis: Data From the Prospective Observational FINNAKI Study. Crit Care (2013) 17(6):R295. doi: 10.1186/cc13161
65. Asfar P, Meziani F, Hamel JF, Grelon F, Megarbane B, Anguel N, et al. High Versus Low Blood-Pressure Target in Patients With Septic Shock. N Engl J Med (2014) 370(17):1583–93. doi: 10.1056/NEJMoa1312173
66. Redl-Wenzl EM, Armbruster C, Edelmann G, Fischl E, Kolacny M, Wechsler-Fördös A, et al. The Effects of Norepinephrine on Hemodynamics and Renal Function in Severe Septic Shock States. Intensive Care Med (1993) 19(3):151–4. doi: 10.1007/BF01720530
67. Dünser MW, Hasibeder WR. Sympathetic Overstimulation During Critical Illness: Adverse Effects of Adrenergic Stress. J Intensive Care Med (2009) 24(5):293–316. doi: 10.1177/0885066609340519
68. Vignon P, Evrard B, Asfar P, Busana M, Calfee CS, Coppola S, et al. Fluid Administration and Monitoring in ARDS: Which Management? Intensive Care Med (2020) 46(12):2252–64. doi: 10.1007/s00134-020-06310-0
69. Myburgh JA, Finfer S, Bellomo R, Billot L, Cass A, Gattas D, et al. Hydroxyethyl Starch or Saline for Fluid Resuscitation in Intensive Care. N Engl J Med (2012) 367(20):1901–11. doi: 10.1056/NEJMoa1209759
70. Perner A, Haase N, Guttormsen AB, Tenhunen J, Klemenzson G, Åneman A, et al. Hydroxyethyl Starch 130/0.42 Versus Ringer’s Acetate in Severe Sepsis. N Engl J Med (2012) 367(2):124–34. doi: 10.1056/NEJMoa1204242
71. Hammond DA, Lam SW, Rech MA, Smith MN, Westrick J, Trivedi AP, et al. Balanced Crystalloids Versus Saline in Critically Ill Adults: A Systematic Review and Meta-Analysis. Ann Pharmacother (2020) 54(1):5–13. doi: 10.1177/1060028019866420
72. Redfors B, Bragadottir G, Sellgren J, Swärd K, Ricksten SE. Effects of Norepinephrine on Renal Perfusion, Filtration and Oxygenation in Vasodilatory Shock and Acute Kidney Injury. Intensive Care Med (2011) 37(1):60–7. doi: 10.1007/s00134-010-2057-4
73. Friedrich JO, Adhikari N, Herridge MS, Beyene J. Meta-Analysis: Low-Dose Dopamine Increases Urine Output But Does Not Prevent Renal Dysfunction or Death. Ann Intern Med (2005) 142(7):510. doi: 10.7326/0003-4819-142-7-200504050-00010
74. Bove T, Zangrillo A, Guarracino F, Alvaro G, Persi B, Maglioni E, et al. Effect of Fenoldopam on Use of Renal Replacement Therapy Among Patients With Acute Kidney Injury After Cardiac Surgery: A Randomized Clinical Trial. JAMA (2014) 312(21):2244–53. doi: 10.1001/jama.2014.13573
75. Xiong B, Wang C, Yao Y, Huang Y, Tan J, Cao Y, et al. The Dose-Dependent Effect of Nesiritide on Renal Function in Patients With Acute Decompensated Heart Failure: A Systematic Review and Meta-Analysis of Randomized Controlled Trials. PloS One (2015) 10(6):e0131326. doi: 10.1371/journal.pone.0131326
76. Gordon AC, Perkins GD, Singer M, McAuley DF, Orme RML, Santhakumaran S, et al. Levosimendan for the Prevention of Acute Organ Dysfunction in Sepsis. N Eng J Med (2016) 375(17):1638–48. doi: 10.1056/NEJMoa1609409
77. Landoni G, Lomivorotov VV, Alvaro G, Lobreglio R, Pisano A, Guarracino F, et al. Levosimendan for Hemodynamic Support After Cardiac Surgery. N Eng J Med 376(21):2021–31. doi: 10.1056/NEJMoa1616325
78. Schetz M, Vanhorebeek I, Wouters PJ, Wilmer A, Van den Berghe G. Tight Blood Glucose Control is Renoprotective in Critically Ill Patients. J Am Soc Nephrol (2008) 19(3):571–8. doi: 10.1681/ASN.2006101091
79. Intensive Versus Conventional Glucose Control in Critically Ill Patients: A Meta-Analysis of Randomized Controlled Trials- ClinicalKey. Available at: https://www.clinicalkey.fr/#!/content/playContent/1-s2.0-S0953620512000660?returnurl=https:%2F%2Flinkinghub.elsevier.com%2Fretrieve%2Fpii%2FS0953620512000660%3Fshowall%3Dtrue&referrer=https:%2F%2Flink.springer.com%2Farticle%2F10.1007%2Fs00134-017-4832-y%3Fshared-article-renderer (Accessed February 21, 2020).
80. Guillemet L, Jamme M, Bougouin W, Geri G, Deye N, Vivien B, et al. Effects of Early High-Dose Erythropoietin on Acute Kidney Injury Following Cardiac Arrest: Exploratory Post Hoc Analyses From an Open-Label Randomized Trial. Clin Kidney J (2019) 13(3):413–20. doi: 10.1093/ckj/sfz068
81. Angstwurm MWA, Engelmann L, Zimmermann T, Lehmann C, Spes CH, Abel P, et al. Selenium in Intensive Care (SIC): Results of a Prospective Randomized, Placebo-Controlled, Multiple-Center Study in Patients With Severe Systemic Inflammatory Response Syndrome, Sepsis, and Septic Shock*. Crit Care Med (2007) 35(1):118–26. doi: 10.1097/01.CCM.0000251124.83436.0E
82. Whitlock RP, Devereaux PJ, Teoh KH, Lamy A, Vincent J, Pogue J, et al. Methylprednisolone in Patients Undergoing Cardiopulmonary Bypass (SIRS): A Randomised, Double-Blind, Placebo-Controlled Trial. Lancet (2015) 386(10000):1243–53. doi: 10.1016/S0140-6736(15)00273-1
83. Mekontso Dessap A, Charron C, Devaquet J, Aboab J, Jardin F, Brochard L, et al. Impact of Acute Hypercapnia and Augmented Positive End-Expiratory Pressure on Right Ventricle Function in Severe Acute Respiratory Distress Syndrome. Intensive Care Med (2009) 35(11):1850–8. doi: 10.1007/s00134-009-1569-2
84. Vieillard-Baron A, Schmitt JM, Augarde R, Fellahi JL, Prin S, Page B, et al. Acute Cor Pulmonale in Acute Respiratory Distress Syndrome Submitted to Protective Ventilation: Incidence, Clinical Implications, and Prognosis. Crit Care Med (2001) 29(8):1551–5. doi: 10.1097/00003246-200108000-00009
85. Mercat A, Richard JCM, Vielle B, Jaber S, Osman D, Diehl J-L, et al. Positive End-Expiratory Pressure Setting in Adults With Acute Lung Injury and Acute Respiratory Distress Syndrome: A Randomized Controlled Trial. JAMA (2008) 299(6):646–55. doi: 10.1001/jama.299.6.646
86. Schmitt JM, Vieillard-Baron A, Augarde R, Prin S, Page B, Jardin F. Positive End-Expiratory Pressure Titration in Acute Respiratory Distress Syndrome Patients: Impact on Right Ventricular Outflow Impedance Evaluated by Pulmonary Artery Doppler Flow Velocity Measurements. Crit Care Med (2001) 29(6):1154–8. doi: 10.1097/00003246-200106000-00012
87. Resar R, Pronovost P, Haraden C, Simmonds T, Rainey T, Nolan T. Using a Bundle Approach to Improve Ventilator Care Processes and Reduce Ventilator-Associated Pneumonia. Jt Comm J Qual Patient Saf (2005) 31(5):243–8. doi: 10.1016/s1553-7250(05)31031-2
88. Bhagwanani A, Carpenter R, Yusuf A. Improving the Management of Acute Kidney Injury in a District General Hospital: Introduction of the DONUT Bundle. BMJ Open Qual (2014) 2(2). doi: 10.1136/bmjquality.u202650.w1235
89. Selby NM, Casula A, Lamming L, Stoves J, Samarasinghe Y, Lewington AJ, et al. An Organizational-Level Program of Intervention for AKI: A Pragmatic Stepped Wedge Cluster Randomized Trial. JASN (2019) 30(3):505–15. doi: 10.1681/ASN.2018090886
90. Kolhe NV, Reilly T, Leung J, Fluck RJ, Swinscoe KE, Selby NM, et al. A Simple Care Bundle for Use in Acute Kidney Injury: A Propensity Score-Matched Cohort Study. Nephrol Dial Transplant (2016) 31(11):1846–54. doi: 10.1093/ndt/gfw087
91. Goldstein SL, Dahale D, Kirkendall ES, Mottes T, Kaplan H, Muething S, et al. A Prospective Multi-Center Quality Improvement Initiative (NINJA) Indicates a Reduction in Nephrotoxic Acute Kidney Injury in Hospitalized Children. Kidney Int (2020) 97(3):580–8. doi: 10.1016/j.kint.2019.10.015
92. Meersch M, Schmidt C, Hoffmeier A, Van Aken H, Wempe C, Gerss J, et al. Prevention of Cardiac Surgery-Associated AKI by Implementing the KDIGO Guidelines in High Risk Patients Identified by Biomarkers: The PrevAKI Randomized Controlled Trial. Intensive Care Med (2017) 43(11):1551–61. doi: 10.1007/s00134-016-4670-3
93. Alge JL, Arthur JM. Biomarkers of AKI: A Review of Mechanistic Relevance and Potential Therapeutic Implications. CJASN (2015) 10(1):147–55. doi: 10.2215/CJN.12191213
94. Yoon HE, Kim DW, Kim D, Kim Y, Shin SJ, Shin YR. A Pilot Trial to Evaluate the Clinical Usefulness of Contrast-Enhanced Ultrasound in Predicting Renal Outcomes in Patients With Acute Kidney Injury. Shimosawa T, Ed. PloS One (2020) 15(6):e0235130. doi: 10.1371/journal.pone.0235130
95. Li S, Nagothu KK, Desai V, Lee T, Branham W, Moland C, et al. Transgenic Expression of Proximal Tubule Peroxisome Proliferator-Activated Receptor-Alpha in Mice Confers Protection During Acute Kidney Injury. Kidney Int (2009) 76(10):1049–62. doi: 10.1038/ki.2009.330
96. Tran MT, Zsengeller ZK, Berg AH, Khankin EV, Bhasin MK, Kim W, et al. Pgc1α-Dependent NAD Biosynthesis Links Oxidative Metabolism to Renal Protection. Nature (2016) 531(7595):528–32. doi: 10.1038/nature17184
97. Takahashi K, Kamijo Y, Hora K, Hashimoto K, Higuchi M, Nakajima T, et al. Pretreatment by Low-Dose Fibrates Protects Against Acute Free Fatty Acid-Induced Renal Tubule Toxicity by Counteracting Pparα Deterioration. Toxicol Appl Pharmacol (2011) 252(3):237–49. doi: 10.1016/j.taap.2011.02.012
98. Katsyuba E, Mottis A, Zietak M, De Franco F, van der Velpen V, Gariani K, et al. De Novo NAD+ Synthesis Enhances Mitochondrial Function and Improves Health. Nature (2018) 563(7731):354–9. doi: 10.1038/s41586-018-0645-6
99. Poyan Mehr A, Tran MT, Ralto KM, Leaf DE, Washco V, Messmer J, et al. De Novo NAD+ Biosynthetic Impairment in Acute Kidney Injury in Humans. Nat Med (2018) 24(9):1351–9. doi: 10.1038/s41591-018-0138-z
100. Guan Y, Wang SR, Huang XZ, Xie Q-H, Xu Y-Y, Shang D, et al. Nicotinamide Mononucleotide, an NAD+ Precursor, Rescues Age-Associated Susceptibility to AKI in a Sirtuin 1-Dependent Manner. J Am Soc Nephrol (2017) 28(8):2337–52. doi: 10.1681/ASN.2016040385
101. Gaudry S, Hajage D, Schortgen F, Martin-Lefevre L, Pons B, Boulet E, et al. Initiation Strategies for Renal-Replacement Therapy in the Intensive Care Unit. N Engl J Med (2016) 375(2):122–33. doi: 10.1056/NEJMoa1603017
102. Barbar SD, Clere-Jehl R, Bourredjem A, Hernu R, Montini F, Bruyère R, et al. Timing of Renal-Replacement Therapy in Patients With Acute Kidney Injury and Sepsis. N Engl J Med (2018) 379(15):1431–42. doi: 10.1056/NEJMoa1803213
103. Gaudry S, Hajage D, Benichou N, Chaïbi K, Barbar S, Zarbock A, et al. Delayed Versus Early Initiation of Renal Replacement Therapy for Severe Acute Kidney Injury: A Systematic Review and Individual Patient Data Meta-Analysis of Randomised Clinical Trials. Lancet (2020) 395(10235):1506–15. doi: 10.1016/S0140-6736(20)30531-6
104. STARRT-AKI Investigators, Canadian Critical Care Trials Group, Australian and New Zealand Intensive Care Society Clinical Trials Group, United Kingdom Critical Care Research Group, Canadian Nephrology Trials Network, Irish Critical Care Trials Group, et al. Timing of Initiation of Renal-Replacement Therapy in Acute Kidney Injury. N Engl J Med (2020) 383(3):240–51. doi: 10.1056/NEJMoa2000741
105. Zarbock A, Kellum JA, Schmidt C, Van Aken H, Wempe C, Pavenstädt H, et al. Effect of Early vs Delayed Initiation of Renal Replacement Therapy on Mortality in Critically Ill Patients With Acute Kidney Injury: The ELAIN Randomized Clinical Trial. JAMA (2016) 315(20):2190–9. doi: 10.1001/jama.2016.5828
Keywords: acute kidney injury, acute respiratory distress syndrom (ARDS), lung kidney interaction, mechanical ventilation, COVID
Citation: Marchiset A and Jamme M (2022) When the Renal (Function) Begins to Fall: A Mini-Review of Acute Kidney Injury Related to Acute Respiratory Distress Syndrome in Critically Ill Patients. Front. Nephrol. 2:877529. doi: 10.3389/fneph.2022.877529
Received: 16 February 2022; Accepted: 21 March 2022;
Published: 21 April 2022.
Edited by:
Piergiorgio Messa, University of Milan, ItalyReviewed by:
Carmen Silvia Valente Barbas, University of São Paulo, BrazilCopyright © 2022 Marchiset and Jamme. This is an open-access article distributed under the terms of the Creative Commons Attribution License (CC BY). The use, distribution or reproduction in other forums is permitted, provided the original author(s) and the copyright owner(s) are credited and that the original publication in this journal is cited, in accordance with accepted academic practice. No use, distribution or reproduction is permitted which does not comply with these terms.
*Correspondence: Matthieu Jamme, bWF0LmphbW1lQGdtYWlsLmNvbQ==