- Département d’informatique, Université du Québec à Montréal, Montreal, QC, Canada
The notion of gating as a mechanism capable of controlling the flow of information from one set of neurons to another, has been studied in many regions of the central nervous system. In the nucleus accumbens, where evidence is especially clear, gating seems to rely on the action of bistable neurons, i.e., of neurons that oscillate between a quiescent “down” state and a firing “up” state, and that act as AND-gates relative to their entries. Independently from these observations, a growing body of evidence now indicates that bistable neurons are also quite abundant in the cortex, although their exact functions in the dynamics of the brain remain to be determined. Here, we propose that at least some of these bistable cortical neurons are part of circuits devoted to gating information flow within the cortex. We also suggest that currently available structural, electrophysiological, and imaging data support the existence of at least three different types of gating architectures. The first architecture involves gating directly by the cortex itself. The second architecture features circuits spanning the cortex and the thalamus. The third architecture extends itself through the cortex, the basal ganglia, and the thalamus. These propositions highlight the variety of mechanisms that could regulate the passage of action potentials between cortical neurons sets. They also suggest that gating mechanisms require larger-scale neural circuitry to control the state of the gates themselves, in order to fit in the overall wiring of the brain and complement its dynamics.
Introduction
The concept of gating in the cortex, i.e., of mechanisms that are capable of interrupting, or allowing, the passage of action potentials between distinct sets of cortical neurons, is rapidly generating increasing amounts of interest.
This interest is probably largely motivated by the growing number of electrophysiological and magnetic resonance imaging studies bringing support to the idea of gating. Electrophysiological data gathered in the nineties by Chelazzi et al. (1993) on primates performing the delayed matching-to-sample task, where subjects are required to retain some visual information during a delay, already suggested the presence of mechanisms that manage information propagation between the inferior temporal and the prefrontal cortex. It was later shown by Sakai et al. (2002), using a magnetic resonance imaging experimental setup requiring that subjects keep in mind information while performing a non-related distractor task, that area 46 of the prefrontal cortex seems able to implement a type of shielding mechanism allowing different information to be simultaneously kept in mind. More recent electrophysiological investigations have revealed that activity in the mediodorsal nucleus of the thalamus seems capable of gating information transmission from the hippocampus to the prefrontal cortex (Floresco and Grace, 2003). These studies also indicate that activity in entorhinal cortex may influence the information flow through the medial prefrontal cortex (Valenti and Grace, 2009). Moreover, imaging (McNab and Klingberg, 2008) and theoretical (Frank et al., 2001) studies have suggested a role for the basal ganglia in controlling information access to working memory during certain cognitive tasks. In addition, several theoretical studies have also proposed potential mechanisms that would allow a given group of cells to regulate the propagation of action potentials between distinct sets of cortical neurons. Some of these studies place the bulk of these mechanisms within the connectivity of the cortex (Vogels and Abbott, 2009), while others (Kepecs and Raghavachari, 2007) suggest instead a central role for a class of neurons whose membrane potentials oscillate between two discrete (so-called “up” and “down”) values (Cowan and Wilson, 1994).
At a larger scale, it has also been proposed that gating mechanisms, by their ability to manage the flow of information traveling between distinct cortical regions, might play a key role in the creation of the brain activity coding for behaviors (Bressler, 1995; Gisiger and Kerszberg, 2007). According to this viewpoint, the coordinated action of gating mechanisms would select the cortical regions required for a given behavior, put them in interaction with each other in a timely fashion, and produce the streams of neural computations necessary to accomplish the behavior at hand (Bressler, 1995). Support for this proposal was provided by studies featuring neural network models equipped with gating mechanisms, and designed to perform simple memory tasks. Simulations showed that these models were able to perform such memory tasks while at the same time successfully reproducing electrophysiological data gathered on the cortex of primates performing these same tasks (Gisiger et al., 2005; Gisiger and Kerszberg, 2006, 2007). It was also proposed by Olshausen et al. (1993, 1995) that gating might play a key role in the neural processes involved in spatial attention.
The next frontier in this fast-growing subject, we argue, is to try to connect gating mechanisms at the neural level, with the large-scale computations observed in the cortex during behaviors. Several questions are awaiting an answer, and among them the following: What neural circuits control the mechanisms gating the flow of information in the cortex? Which brain structures are involved in these control circuits? How do circuits featuring gating mechanisms interact with each other? And, also, how do gating events become embedded in the overall dynamics of the cortex and the brain?
Here, we propose tentative answers to these questions using currently available data on cortical neurons, and on the circuitry linking the cortex, the thalamus, and the basal ganglia. We start this article by considering various possible neural mechanisms that would allow control of information transmission between two populations of cortical neurons. We then propose some features that a general gating circuit should possess in order to fit in the overall circuitry of the brain. We next put forward several neural architectures spanning the cortex, the thalamus, and the basal ganglia that might support cortical gating mechanisms. We end this paper by comparing these propositions to already published gating mechanisms, and outlining future directions of research.
Analysis
Bistable Neurons and Gating in the Brain
To our knowledge, the most detailed and far reaching experimental observations on gating in neural systems were published by Grace, O’Donnell and colleagues (O’Donnell and Grace, 1995; Grace, 2000; Goto and O’Donnell, 2001). Their results are especially interesting as they might form a prototype for understanding gating mechanisms in other regions of the central nervous system, and particularly in the cortex.
The system studied by these authors is the nucleus accumbens (Nacc), and more specifically those of its neurons that exhibit distinctive oscillations between two states characterized by specific membrane potential values (O’Donnell and Grace, 1995; Grace, 2000). One state, labeled “down state,” is associated with a hyperpolarized membrane potential, and the complete absence of action potential production by the cell. The other state, labeled “up state,” is characterized by a membrane potential that is just below the cell’s firing threshold and the occurrence of action potentials (Figure 1A).
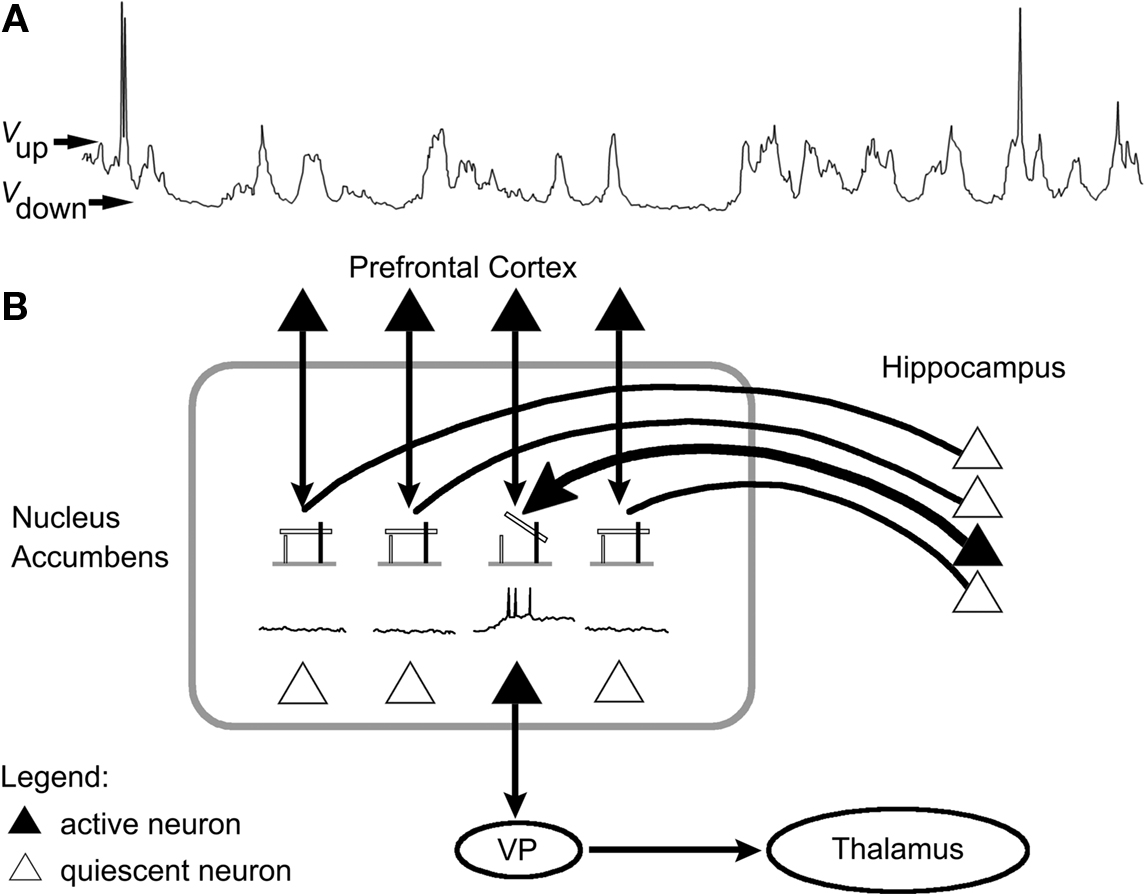
Figure 1. Hippocampus gating of information flow from the prefrontal cortex via modulation of nucleus accumbens neuronal activity. (A) Recording of the membrane potential of a Nacc neuron showing oscillations between a quiescent “down” state (Vdown membrane potential) and a firing “up” state (Vup membrane potential). (B) Projections from the hippocampus and the prefrontal cortex converge onto Nacc neurons, which in turn project on the ventral pallidum, which itself sends projections onto the thalamus. Hippocampus input appears to shift Nacc neurons from their down to their up state, thus efficiently gating prefrontal input to the Nacc (modified with permission from Grace, 2000). VP: ventral pallidum.
Studies have shown that Nacc neurons receive projections from both the hippocampus and the prefrontal cortex. O’Donnell and Grace demonstrated that these two inputs have dramatically different influences on Nacc neuron firing (O’Donnell and Grace, 1995; Grace, 2000). They showed that stimulation of the prefrontal cortex while Nacc neurons are in their down state has very little chance of triggering action potentials in these cells. Stimulation of hippocampus while Nacc neurons are in their down state also fails to produce action potentials, but instead has the effect of shifting these neurons from their down to their up state. Once in this up state, however, prefrontal cortex stimulation produces action potentials in these cortical neurons with very high probability.
These findings seem to suggest that Nacc neurons are part of a gating mechanism in which hippocampal input is required to “arm” the gate by shifting Nacc neurons to their up state. Once this gate is open, subsequent input from the prefrontal cortex faithfully triggers action potentials in Nacc neurons, and is therefore further relayed to other brain structures like the ventral pallidum (Figure 1B). Bringing Nacc neurons back to their down state, where they cannot produce action potentials, closes the gate (Grace, 2000). These cells therefore implement a type of biological AND gate relative to the inputs from the hippocampus and prefrontal cortex.
A growing body of experimental evidence indicates that neurons with membrane potential fluctuating between two subthreshold values and that can only produce action potentials in their “up” state, which we will call “up/down neurons” for short, are quite common in the brain. Indeed, they have been observed in subcortical structures (Wilson, 1993; Wilson and Kawaguchi, 1996), in the cerebellum (Loewenstein et al., 2005), as well as in various regions of the cortex (see Cossart et al., 2003; Shu et al., 2003 and references therein). In the cortex per se, up/down neurons appear to be quite numerous and to come in several types: MacLean et al. (2005) found that between 20 and 50% of all recorded cells in layer IV of mouse cortex were up/down neurons, and identified them as either pyramidal neurons, spiny stellate cells, or interneurons in roughly similar proportions. The location of these cells also seems to hold some sort of strategic significance. Indeed, it was observed by Cossart et al. (2003) that up/down neurons can be scattered in all cortical layers, concentrated in clusters, lined up in a single cortical layer, or distributed along a column spanning all cortical layers.
Two different origins have been proposed for the peculiar bistability of up/down cortical neurons. The first one is at the circuit level: the observed bistability would mainly be a consequence of the wiring of these cells to other neurons of cortex (Cossart et al., 2003; Shu et al., 2003; Parga and Abbott, 2007). The second option, proposed by Kepecs and Raghavachari (2007), places the origin of the bistability mainly within the up/down neurons themselves, i.e., at the cellular or even dendritic levels. The authors propose a model where two information routes coexist on this type of neuron, each being implemented by a different type of glutamate receptor: one involves mainly the NMDA receptor and shifts the cell from its down to its up state; the other involves mainly the AMPA receptor and triggers actual action potentials in the neuron. The result is a neuron that, through its own intrinsic dynamics, implements a type of biological AND gate. Experimental data also seems to support a role for glutamate and NMDA receptors in the overall dynamics generating the up and down states observed in cortical neurons (see Wilson, 2008 for a review). It has been further suggested that dopamine might act as a modulator that tends to stabilize up states, particularly when it binds onto D1 receptors (see Lewis and O’Donnell, 2000 and references therein).
If individual neurons indeed can implement AND gates, as suggested by Kepecs and Raghavachari (2007), then the recent experimental findings on gatings (O’Donnell and Grace, 1995) and bistable neurons (Cossart et al., 2003; Shu et al., 2003; Loewenstein et al., 2005) might motivate a renewed examination of the classical view of neurotransmission. Indeed, classical neurotransmission states that the various excitatory inputs afferent onto a neuron are roughly summed in the dendritic tree, and therefore play qualitatively similar roles in the decision of the postsynaptic neuron to fire. The findings by Grace and colleagues might suggest on the contrary that, in certain cases, some entries are privileged in that decision, being mandatory for the activity of the postsynaptic neuron to take place, but at the same time being insufficient to trigger that firing by themselves (see Katz and Frost, 1996 for a similar point on the expansion of classical neurotransmission to include the concept of neuromodulation).
So far, little seems to be known about the temporal aspects of the dynamics of up/down neurons and of the gating mechanisms they contribute to. Indeed, although the statistical distribution of the time spent by neurons in their up and down states have been presented in numerous studies, the actual duration of the transition itself between these states remains to be investigated. So far, to our knowledge, only Kepecs and Raghavachari (2007) have discussed this aspect of the dynamics, describing this transition as “rapid” and lasting on the order of tens of milliseconds. Similarly, the time interval separating the moment when the neuron reaches its up state, and when it is actually ready to fire action potentials, also has yet to be characterized.
In the present work, we will not make any supposition on which mechanisms are actually responsible for the bistability observed in cortical neurons, as this is still an open question. We will however make an assumption on the function of cells exhibiting this bistability: we will postulate that a sizable proportion of the up/down cells observed in the cortex either individually act as AND gates relative to their entries, or are part of circuits that collectively act as AND gates.
Basic Gating Mechanisms
Gating mechanisms featuring up/down neurons
Based on the assumption, as well as on the experimental and theoretical data presented above, we propose the circuits illustrated in Figure 2, as mechanisms relying on up/down neurons for gating information transmission in the cortex.
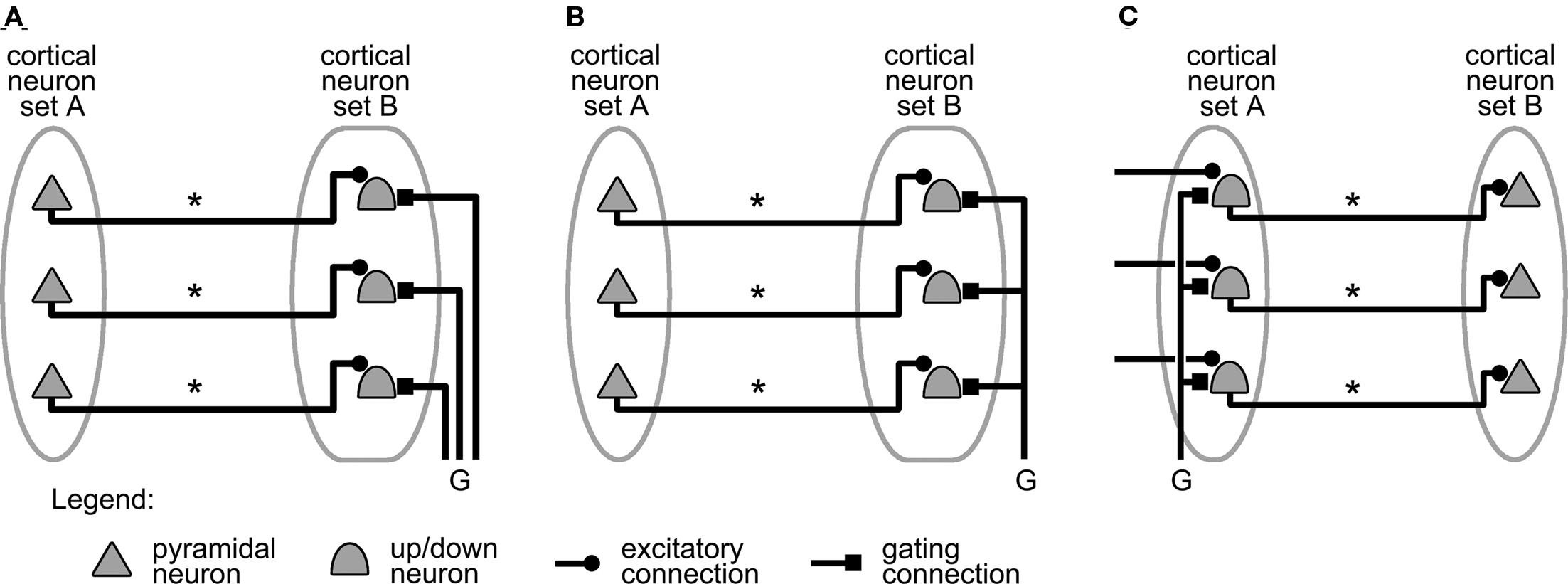
Figure 2. Mechanisms relying on up/down neurons to gate information transmission between two cortical neuron sets. (A) Individual gating of B-set neurons. (B) Simultaneous gating of all B-set neurons. (C) Simultaneous gating of all A-set neurons. Projections labeled “*” carry action potentials from A-set neurons to B-set neurons. Projections labeled “G,” when active, shift up/down neurons to their up state (see text). The neuron symbols represent either individual cortical neurons, or small, interconnected assemblies of cortical neurons exhibiting similar responses (see text).
For simplicity, the system consists of only two sets of cortical neurons labeled A and B. It could however be straightforwardly generalized to a larger number of neuron sets. Set A contains neurons whose firing represents the information that needs to be transmitted to the neurons of set B. This information is carried from A to B by connections, labeled “*” in Figure 2, that neurons in A project onto neurons in B. The projections illustrated in Figure 2 are one-to-one for simplicity, but they could equally well follow divergent or convergent patterns from A to B.
The system is completed by a set of projections, labeled “G” in Figure 2 for gating connection or projection, whose role is to carry a “gating signal,” i.e., action potentials that shift up/down neurons into their up state. These connections therefore gate the transmission of activity from neurons in set A to neurons in set B.
The neurons in sets A and B can be seen as input and output of the system, respectively, or alternatively as sender and receiver of information following the notation introduced by Vogels and Abbott (2009). The two sets could represent groups of biological cells located anywhere in the cortex (as long as the two groups of cells are connected to each other by direct projections). For instance, set A might represent a population of neurons in the sensory cortex, and B might represent cells in the motor cortex. The “*” projections from A to B would then implement a type of cortical reflex circuit, i.e., a mapping of sensory stimulations onto a set of motor responses.
As is shown in Figures 2A–C, such a system can be configured in several ways. Figure 2A is the direct analog of the system studied by O’Donnell and Grace (O’Donnell and Grace, 1995; Grace, 2000; compare with Figure 1B). Set A represents prefrontal cortex neurons and set B corresponds to Nacc cells, which are therefore up/down neurons. Finally, the axons carrying the gating signal represent the projections from the hippocampus. When set A neurons are firing, action potentials are transmitted to the up/down neurons in set B. However, the individual neurons remain silent as long as their respective gating signals are not received, and have not shifted them from their down to their up state.
Figure 2B shows a generalization of this mechanism where the signal that gates access to set B neurons arises from a single axon’s collaterals. The gating now allows the simultaneous shifting of all set B neurons, and is therefore unspecific. This contrasts with Figure 2A where distinct gating signals act on certain set B neurons and not others, and where gating is consequently specific.
Figure 2C illustrates yet another variant. Activity propagation from set A to set B is still gated in an unspecific manner, but the gating projections target set A neurons this time. The gate now controls whether action potentials are transmitted from A to B, instead of just controlling the output of set B neurons.
For simplicity, we focus in the rest of this paper on unspecific gating that targets set B neurons (Figure 2B). However, the results so obtained can be straightforwardly generalized to the specific gating mechanism shown in Figure 2A, or to the unspecific gating mechanism targeting set A sender neurons (Figure 2C).
It should be noted that in both the text and figure illustrations of this paper, the different neurons are meant more in a functional than a strict biological sense. Therefore, the neurons represented in the figures could represent either individual biological cells or small, interconnected assemblies of biological neurons. Similarly, the up/down neuron symbols could represent either individual bistable neurons (Kepecs and Raghavachari, 2007), population of individual bistable cells, or groups of interconnected neurons with some cells exhibiting bistable behavior (Cossart et al., 2003).
Gating mechanisms featuring inhibitory neurons
Current theoretical and experimental data also suggest the possibility of gating mechanisms relying on inhibitory interneurons (Anderson and Van Essen, 1987; Olshausen et al., 1993; Burchell et al., 1998; Floresco and Grace, 2003; Vogels and Abbott, 2005, 2009; Cardin et al., 2009) rather than on up/down neurons.
A first possible gating mechanism of this type is illustrated in Figure 3A.
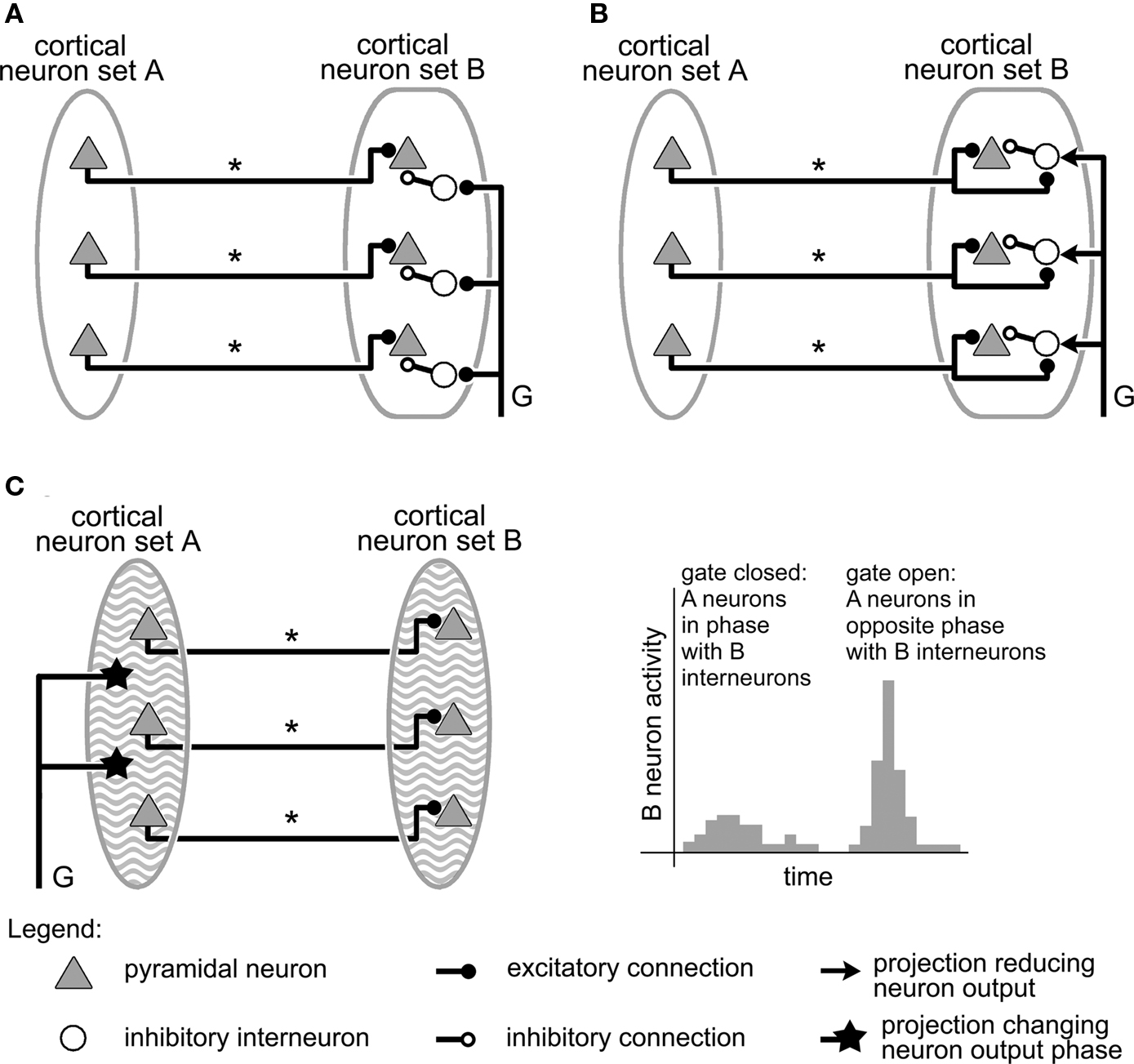
Figure 3. Gating mechanisms involving inhibition. As in Figure 2, in all circuits, information is transmitted from cortical area A to area B by projections labeled “*,” while the gating signal controlling the mechanism is conveyed by an axon labeled “G.” (A) Gating by shunting inhibition. The gating signal triggers inhibitory interneurons that shunt area B pyramidal neuron activity, keeping set A input from triggering set B neurons, and therefore closing the gate. (B) Gating by inhibition modulation in a network where excitation and inhibition compensate each other. The gate is closed by default, but can be opened by the gating signal, which reduces the strength of the inhibition in B, and allows excitatory neurons present there to fire (Modified from Vogels and Abbott, 2009). (C) Gating by network rhythms. Cortical sets A and B, in addition to sender and receiver neurons, also contain densely interconnected inhibitory, fast-spiking interneurons capable of producing stable oscillatory activity at a frequency of about 40 Hz (represented on the figure by a wavy background). By changing the phase of the oscillations in group A relative to those in group B, a gating axon could control the amount of activity evoked in B by input from A, as is illustrated by the histogram on the right (data in histogram modified from Cardin et al., 2009).
This system is identical to the circuit of Figure 2B, except that each up/down neuron has been replaced by a pyramidal neuron and an inhibitory interneuron. These interneurons might correspond, for instance, to basket or chandelier neurons, which were observed in the cortex to make synapses on cell bodies, proximal dendrites, or axon initial segments of pyramidal neurons (Barbas and Zikopoulos, 2007). When active, these cells exercise a powerful inhibition on their targets, even shunting them (Fatt and Katz, 1953; Torre and Poggio, 1978; Koch et al., 1983; Borg-Graham et al., 1998). In the particular circuit illustrated in Figure 3A, these shunting neurons project synapses on the pyramidal neurons in set B, and they are triggered by the action potentials coding for the gating signal (axon “G”). Without the gating signal, there is no inhibition in the system, and action potentials from set A neurons trigger their counterparts in set B: the gate between A and B is open. When the gating signal is present, however, the inhibitory interneurons fire, suppressing activity in set B neurons, and closing the gate to information from set A to set B. The response of the gate to the gating signal is therefore exactly the opposite to that of the mechanism of Figure 2B, where the gate was open/closed when the gating signal was on/off (Olshausen et al., 1993).
To our knowledge, virtually no experimental studies have focused on gating mechanisms of this type in the cortex (although some of the findings of Floresco and Grace, 2003 seem consistent with such a system). Such mechanisms have however been featured or discussed in several theoretical investigations (Anderson and Van Essen, 1987; Olshausen et al., 1993; Vogels and Abbott, 2005). No explicit mention is made in these studies of the neurotransmitters involved, or of the time scale characterizing the dynamics of the gating mechanism. Previous work on shunting inhibition in the cortex, however, suggests a possible role for GABA, which typically operates on a longer time scale than, e.g., glutamate. It also underlines the need for a large temporal overlap between inhibitory and excitatory signals for shunting to actually take place (Koch et al., 1983; Borg-Graham et al., 1998).
Another mechanism also relying on inhibition was recently proposed by Vogels and Abbott (2009). Its main features are summarized in Figure 3B. Here also, there is a sender group A and a receiver group B. Group A consists essentially of excitatory neurons that project long-range connections onto neurons in receiver group B, while group B contains both excitatory and inhibitory neurons. The particularity of this system resides mainly in the values of its synaptic weights, which obey the concept of “detailed balance”: these values are selected so that, when group A neurons are firing, the resulting excitation and inhibition in group B cancel each other, only allowing low, random activity there. In this state, the gate between groups A and B is then closed. The door can be opened, however, if this inhibition is lifted, leaving the excitatory cells in B free to fire. Vogels and Abbott (2009) do not explicitly model the neural mechanisms by which this inhibition lifting might take place. Instead, they open the gate by simultaneously decreasing the responsiveness of all inhibitory neurons in group B. The authors however propose cholinergic modulation as a possible biological implementation for this mechanism. No explicit mention is made of the time scale upon which this type of gating mechanism operates or of the neurotransmitters involved. However, the parameters used in the model have values that fit generic modeling of GABAergic and glutamatergic transmission (see Vogels and Abbott, 2009 for details).
Vogels and Abbott (2005) also showed that similar networks can support forms of signal processing and propagation that go beyond gating. Indeed, by selectively modifying the strength of certain neural pathways of a randomly, but sparsely, interconnected network, they demonstrate that it is possible for neural circuits to implement Not, Switch, and Xor logic gates, as well as Flip-Flop gates that switch between two stable sets of neural activity. The interested reader is referred to their original work for details (Vogels and Abbott, 2005).
We end this subsection by discussing the concept of network rhythms as gating mechanism. Indeed, all the gating mechanisms presented so far rely on specific connection patterns (shunting or balanced inhibition – Figures 3A,B, respectively) or particular neural properties (bistable neurons – Figure 2) to operate. However, it has also been proposed that the oscillations exhibited by neural circuits in certain brain regions might produce a gating effect on afferent information (Burchell et al., 1998; Cardin et al., 2009; Paik and Glaser, 2010).
Indeed, individual neurons behaving in many respects like little “oscillators” when stimulated, it is therefore not surprising that the highly interconnected neural networks of the brain are observed to exhibit a whole range of resonant modes, oscillation frequencies, and synchronization abilities, among others types of dynamics (see for instance Destexhe, 2007; Singer, 2007 and references therein). Current data suggests that the main driving force behind the existence of cortical gamma oscillations, a particular type of oscillation with frequencies ranging from 20 to 80 Hz, is the presence in the cortex of a network of interconnected fast-spiking, inhibitory interneurons. By their activity, these cells appear to stabilize and regulate the activity of neighboring pyramidal neurons into oscillations in the gamma range (Cardin et al., 2009).
It has also been observed experimentally (Burchell et al., 1998; Cardin et al., 2009) and theoretically (Paik and Glaser, 2010), that the effect of an input on a neural circuit exhibiting such an oscillatory state depends strongly on the timing of the input relative to the oscillations: if the input arrives near the peak of the inhibitory oscillation, it is suppressed and triggers very little response (Figure 3C, left part of histogram). On the other hand, if the input arrives near the lowest part of the cycle of inhibition, it generates a much larger response (Figure 3C, right part of histogram). Oscillations, and the narrow window for effective excitation that results from them, could therefore provide an effective way for gating information entry into certain brain regions (Burchell et al., 1998). It has also been proposed that cortical oscillations, and the gating mechanism they implement, might play a central role in how working memory stores several items simultaneously (Lisman and Idiart, 1995; Koene and Hasselmo, 2005), or retains temporal patterns (Fukai, 1999).
Figure 3C puts forward a simple mechanism through which network rhythms might gate information traveling between two neuron sets A and B. This construction is very similar to those of Figures 2 and 3A,B, with sets A and B containing sender and receiver neurons, respectively. Here, however, sets A and B also feature dense networks of inhibitory, fast-spiking interneurons that produce stable oscillatory activity with identical frequencies in the gamma range for both regions. The gating signal that is conveyed to set A neurons by the axon innervating them (axon “G”– Figure 3C), acts to modify the phase (or alternatively the frequency) of oscillations taking place in set A. As a result, the oscillatory input projected by sender neurons of A onto B cells can be tuned to either resonate with oscillations taking place in region B (Figure 3C, right part of histogram – gate open), or instead dissipate through interference (Figure 3C, left part of histogram – gate closed).
A summary of the various mechanisms reviewed in this section is presented in Table 1. All the circuits presented from now on in this article will feature up/down neurons as the central mechanism gating action potential propagation from one cortical region to another. However, all these propositions can also be straightforwardly generalized to the gating mechanisms involving inhibition reviewed earlier.
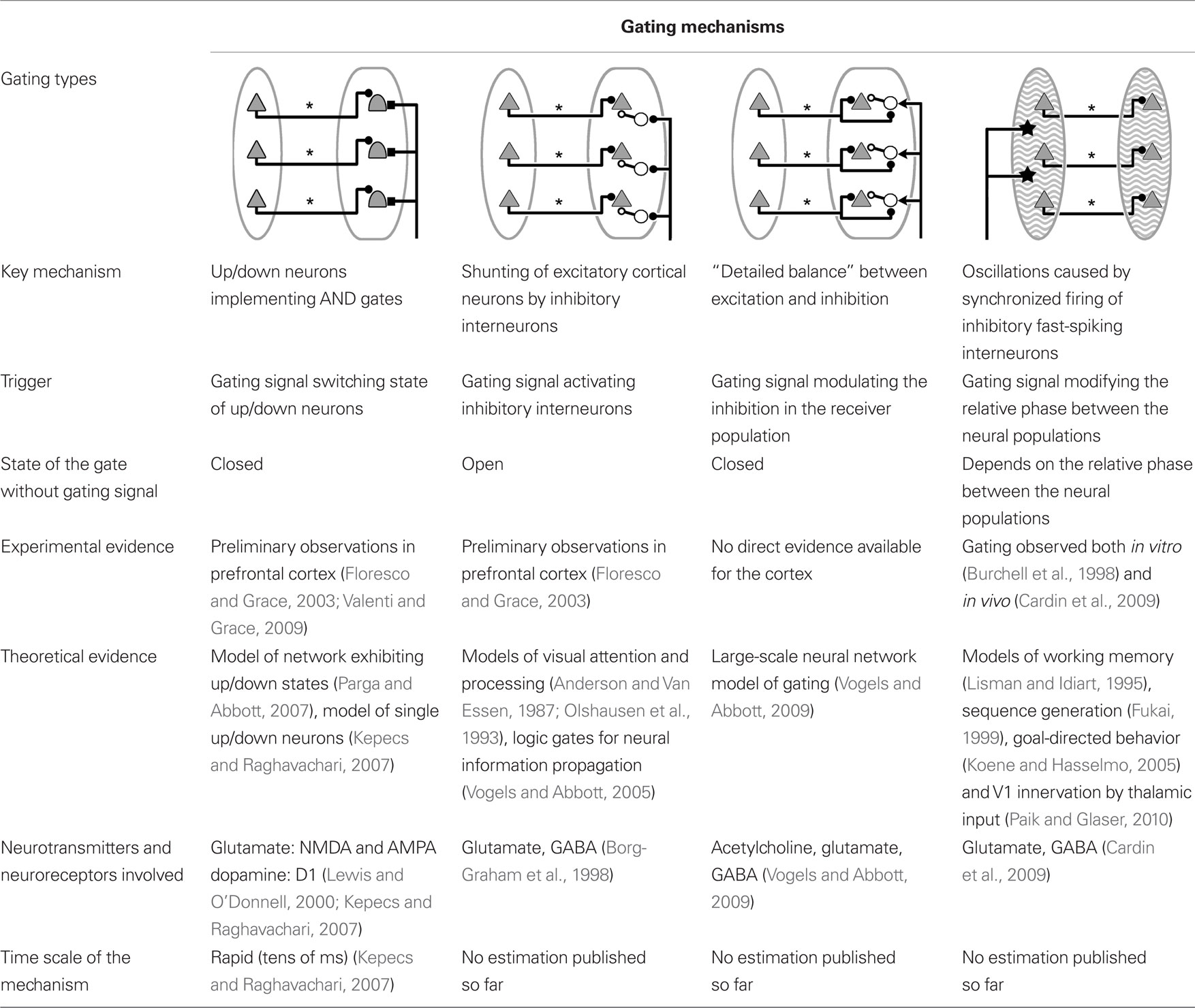
Table 1. Summary of basic gating mechanisms managing information transfer between two groups of cortical neurons.
Generating the Gating Signal
If the current discussion was limited to the mechanisms represented in Figures 2 and 3, then we would be left with a homunculus problem. Indeed, if gates controlling information propagation within the cortex exist in the brain, then, as Katz (2003) put it for gating at the level of synapses, what and where are the gatekeepers? The obvious answer is that the brain should contain neural circuitry that is responsible for deciding when the gate needs to be open or closed, and for producing the corresponding action potentials of the gating signal itself.
Figure 4 illustrates some of the general features characterizing such a gatekeeping circuit in the case of the gating mechanism introduced in Figure 2 for neural groups A and B. Specific proposals for gatekeeping circuits involving the cortex, the thalamus, and the basal ganglia will be presented in the remaining sections of this paper.
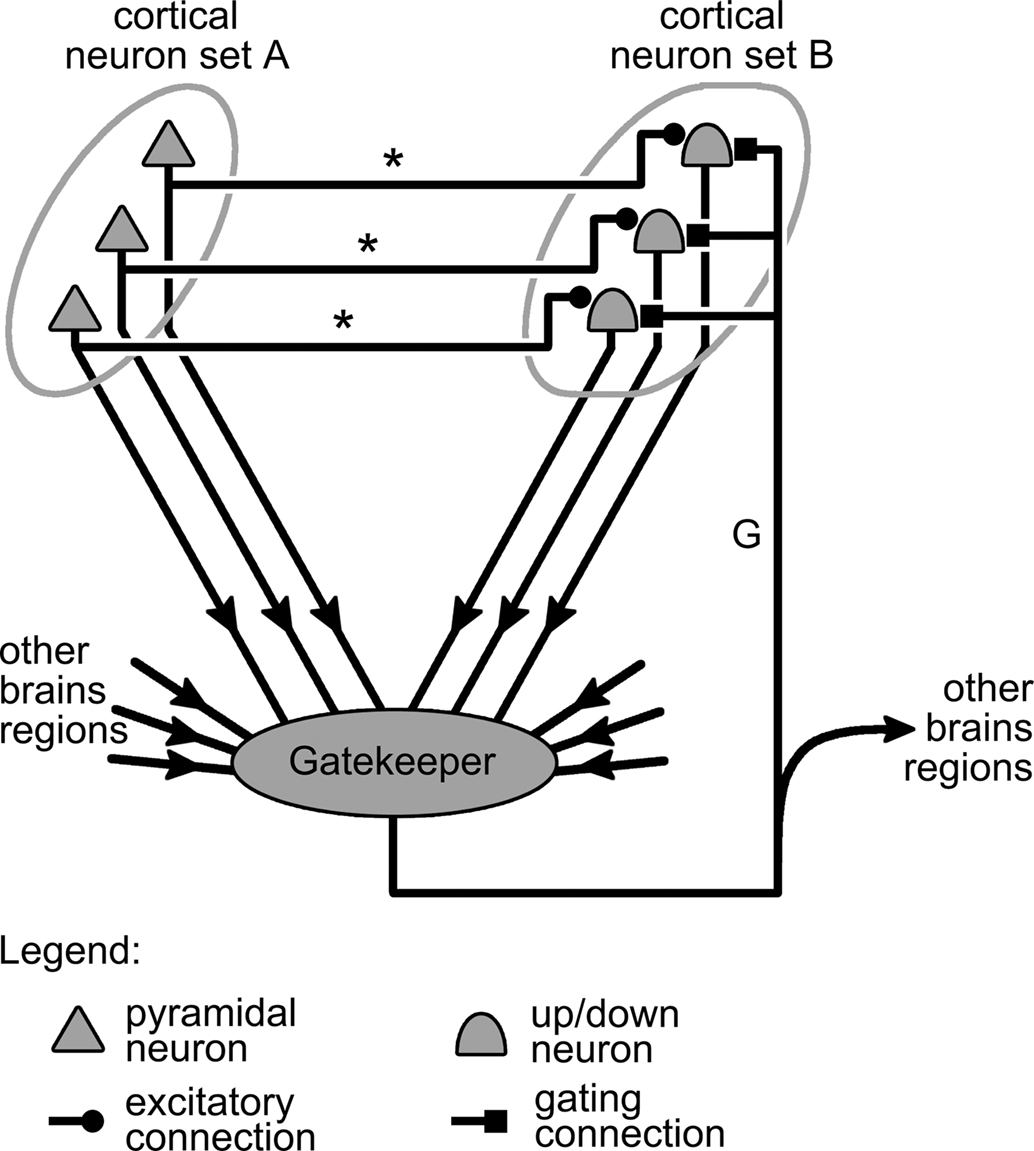
Figure 4. Representation of a complete gating mechanism. This mechanism generalizes that presented in Figure 2B by the addition of a gatekeeping circuit, or controller region (represented by a gray blob) that integrates information from various brain regions, including neuron sets A and B, and generates the gating signal carried by axon “G.”
Generalizing the discussion on gating of synapses by Katz and Frost (1996) to the case of gating in the cortex, gatekeeper circuits can receive two types of inputs: intrinsic and extrinsic. Intrinsic input corresponds to information originating within the system that is being gated, so in our case, from the cortical neuron groups A and B (Figure 4). Extrinsic input, by contrast, comes from brain regions outside of the gated system but that nonetheless influence the state of the gate. All these inputs converging onto the gatekeeper are then integrated to produce a train of action potential representing the gating signal. This signal is then propagated back to neuron group B by the gating axon projected by neurons in the gatekeeper circuits. The gating axon might also branch out to carry a copy of the gating signal to other parts of the brain, informing them on the current state of the gating system (Figure 4).
Going back to the example where sets A and B respectively represent cortical sensory and motor neurons (see above), the various projections afferent onto the gate-triggering mechanism of Figure 4 might take the following biological interpretations. The excitatory projections from set A neurons onto neurons in the gate-triggering mechanism might allow gate opening only if a sufficient level of activity is present in A, i.e., when sensory stimulation is strong enough. Alternatively, projections from set B neurons onto inhibitory interneurons in the gate-triggering mechanism, could close the gate once sufficient activity is present in B, i.e., when a motor response has been initiated. This would cut off further input from the sensory neurons, thereby keeping them from interfering with the movement being executed. The projections from other brain regions onto the gate-triggering circuit might give structures such as premotor cortex a degree of control over the gating system, and consequently over the organism’s motor reactions to sensory stimulation. Finally, the collaterals projected by the gating connection toward other brain regions (Figure 4) might be interpreted as axons transmitting signals to motor centers involved in maintaining the posture of the organism. Indeed, as opening the gate from A to B triggers movements, information on the state of this gate should be useful to motor centers for adjusting the organism’s posture during execution of these movements.
Generalizing further, it is also theoretically possible for the copy of the gating signal from one gating circuit to act as an extrinsic input to another gating circuit, as illustrated in Figure 5. This would then allow the state of a gating mechanism to influence the dynamics of other gates, and to produce complex dynamics such as for instance sequences of gating events (see Discussion).
Another particularity of the circuit shown in Figure 5 is its illustration of how neural networks featuring several interconnected gating circuits present two distinct types of neural pathways. The first type conveys the information processed by the network, implements its various computations, and produces the neural activity coding for its response; it is represented in Figure 5 by the projections that link cortical neural sets A and B, as well as sets C and D together. The second pathway runs parallel to the first, and is formed by the gatekeeping mechanisms from all the gating circuits and by the projections that interconnect them (Figure 5). Being distinct from the first, this pathway is free to convey other types of information and could play various roles.
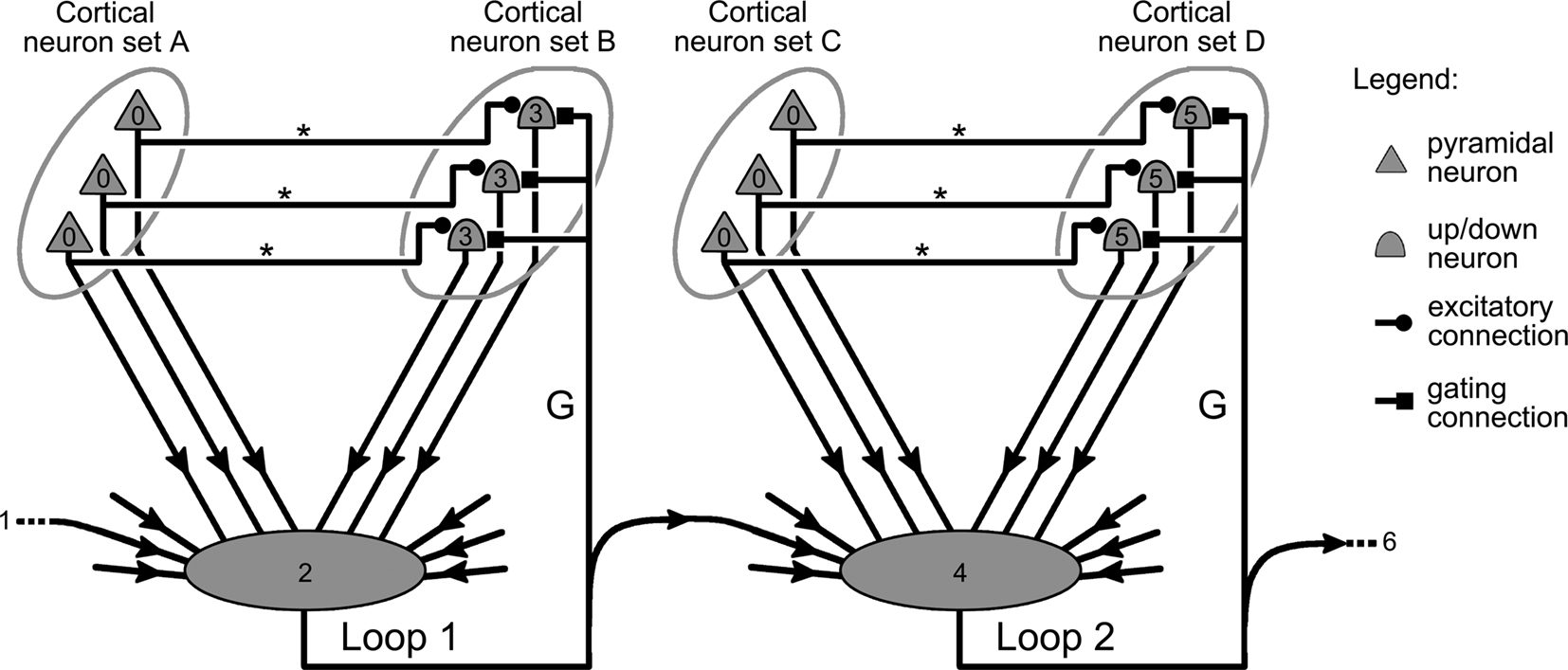
Figure 5. Interactions between gating mechanisms. Illustration of how individual gating mechanisms such as those depicted in Figure 4, can be linked together in a structure capable of producing a sequence of gating events in the cortex. Small numbers within the symbols of the figure indicate the order of the neurons’ firing in the system. The activity of cortical neurons in sets A and C (0) is confined to their respective area until an external signal (1) triggers the gate keeping mechanism of loop 1 (2). As the gate of loop 1 opens up, neurons in B are now free to respond to input from A, and start to fire (3). Simultaneously, the gating signal of loop 1 activates the gate keeping mechanism of loop 2 (4), finally allowing information harbored in C (0) to trigger neurons located in D (5).
An interesting example of this dichotomy in neural pathways is the theoretical work published by Koene and Hasselmo (2005). Here, the authors present a network aiming at reproducing the role of orbitofrontal cortex neurons during tasks where subjects have to associate decisions with stimuli in order to maximize the amount of reward received. The proposed model presents two distinct pathways. The first one conveys information from the network’s sensory units to its action units, and then onward to its reward units. The second pathway of the network spans a set of gating mechanisms that manage access to the sensory, motor, and reward units through the first pathway. However, this secondary pathway connects the network’s units in the reverse direction from the first pathway, starting from the reward unit, then going to the action units, and finally reaching the sensory units. This original structure allows information to simultaneously travel in opposite directions through the network, associating not only responses with stimuli, but also simulating reward-motivated drive. It also enables the model to reproduce certain electrophysiological data gathered on monkeys that are out of reach of traditional feedforward neural network models (see Koene and Hasselmo, 2005 for details).
Gating Information in the Cerebral Cortex
We now present several candidate neural architectures implementing the gating mechanism shown in Figure 4. We limit our study to the simplest possible circuits compatible with current experimental data on vertebrate brain connectivity. Other versions featuring more complex circuitry and a larger number of neurons and interneurons can also be envisioned, but they are not necessary at this point.
Gating by the Cortex
Studies examining the effect of the neural activity in a given cortical area on information transfer between other cortical areas have just started to appear in the literature. One such study by Valenti and Grace (2009) centers on the medial prefrontal cortex, its afferent projections from the hippocampus and the entorhinal cortex, and the efferent projections it sends to other cortical and subcortical regions.
Valenti and Grace (2009) observed that stimulation of the entorhinal cortex led to an increase in the duration of up states in medial prefrontal neurons. This led the authors to suggest that entorhinal cortex might gate the output of prefrontal neurons to other brain regions. Figure 6 illustrates a possible interpretation of these results, where up/down neurons in the medial prefrontal cortex are targeted by gating connections projected by the entorhinal cortex.
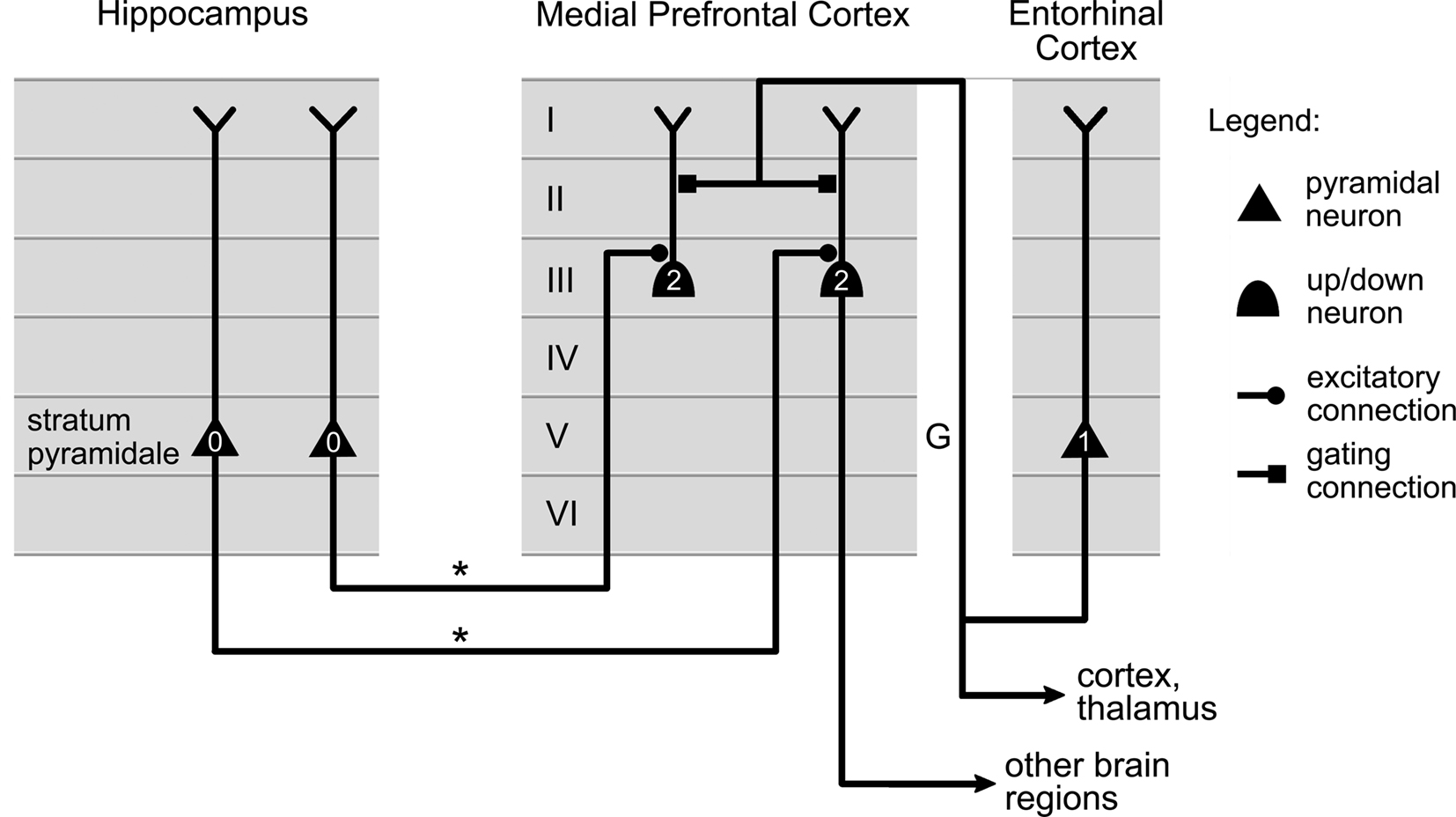
Figure 6. Possible circuit implementing gating of medial prefrontal activity by the entorhinal cortex. Axons (labeled “*”) projected by neurons in the hippocampus carry information to up/down neurons in the medial prefrontal cortex. Axons (labeled “G”) originating in entorhinal cortex influence the state of the up/down neurons, and their ability to relay information to other cortical regions. The firing order for the cells is indicated by small numbers: active cells in the hippocampus (0) can only activate medial prefrontal cells (2) once entorhinal neurons have first fired (1). The location of the cells and the connections in the cortical layers were selected using data published in Valenti and Grace (2009) and Dégenètais et al. (2003).
All these proposals are consistent with one of the roles proposed for entorhinal cortex in contributing to the regulation of memory-guided events (Valenti and Grace, 2009). Indeed, the hippocampus is a key structure in the acquisition of new information and the consolidation of memories, while the prefrontal cortex is involved in nearly every aspect of behavior (Fuster, 1997). By gating prefrontal neurons activity, in particular in relation to the input they receive from the hippocampus, entorhinal cortex could then control the influence of information stored in the hippocampus on behavior. The entorhinal cortex receiving itself projections from multiple brain regions, the gating mechanism would be tributary of the information contained in all these structures.
Gating by the thalamus
Located in the central part of the brain, the thalamus has direct access to the whole cortical mantle through a dense array of two-way projections. Most thalamic nuclei fall in one of two categories: sensory or association. Sensory thalamic nuclei have the particularity of directly receiving information from sensory nerves, which they then communicate to primary sensory cortex. The classical example of this type of thalamic nucleus is the lateral geniculate nucleus (LGN), represented in Figure 7, which relays visual input from the retina to the primary visual cortex and which, like all sensory thalamic nuclei, also receives profuse backprojections from the cortex (Guillery and Sherman, 2002).
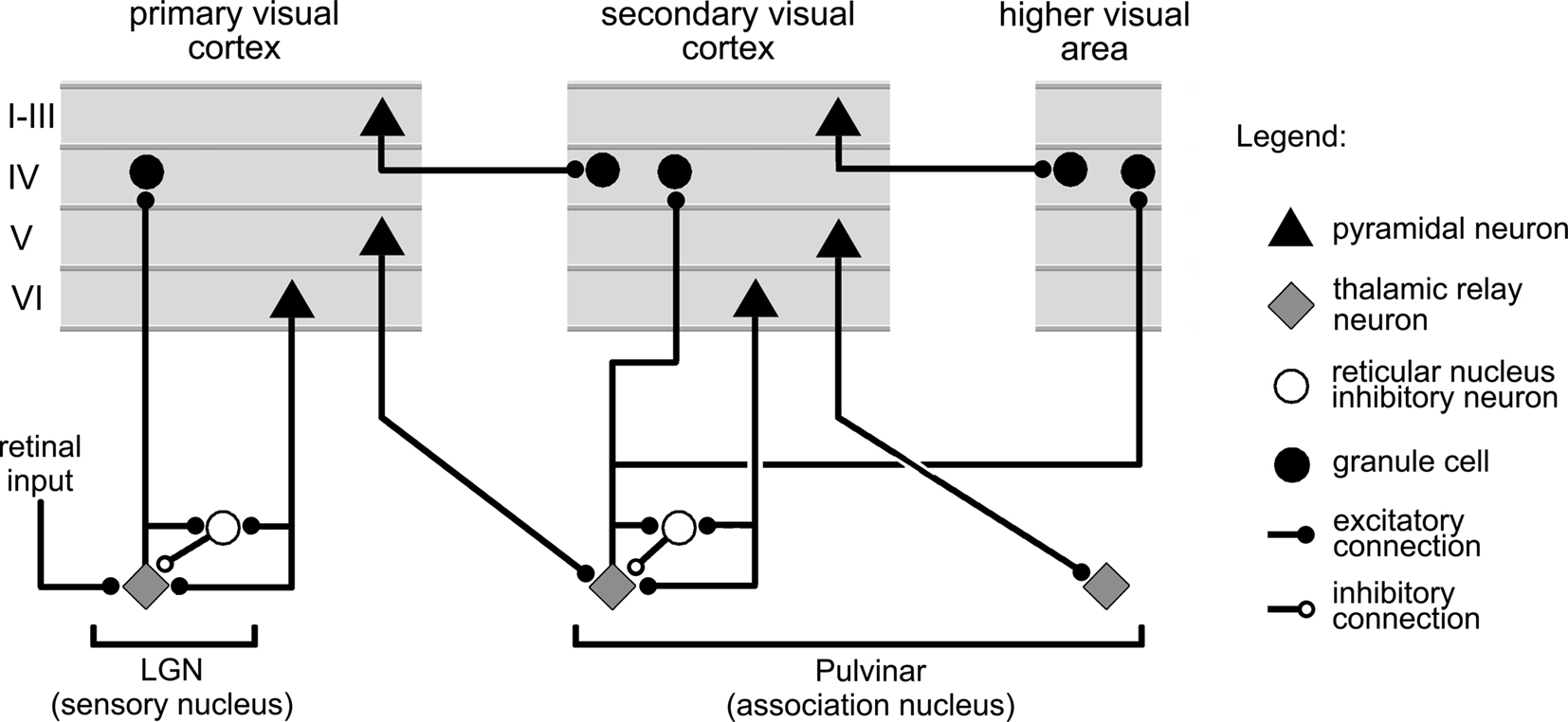
Figure 7. Main features of the connections between the cortex, and thalamic sensory, association, and reticular nuclei. The figure summarizes connectivity features illustrated in Figure 6 of Sherman and Guillery (1996), and Figure 12 of Reichova and Sherman (2004).
The vast majority of thalamic nuclei, however, are association nuclei. They receive no direct input from sensory nerves and, instead, mainly exchange projections with the cortex and other subcortical structures (Figure 7). A typical example of an associative thalamic nucleus in the visual system is the pulvinar, a nucleus several times larger than the LGN, which exchanges connections with virtually all the regions in the visual cortex. As illustrated in Figure 7, the projections from an association nucleus to the cortex are widespread and can simultaneously target several distinct cortical areas (Guillery and Sherman, 2002).
The thalamus also comprises the reticular nucleus, a thin structure that drapes over most of the rest of the thalamic complex, like a shell. This formation only contains inhibitory neurons that send connections to the cells contained in the sensory and association nuclei. Almost all the axons linking the cortex to the thalamus pass through the reticular nucleus, some of them making synapses there in the process (see Sherman and Guillery, 1996; Figure 7).
Currently, there is no general consensus on the relative roles of the cortex and the thalamus in visual, or for that matter, any sensory processing. Present viewpoints range from a mostly cortical (Van Essen, 2005) to a mostly thalamic (Guillery and Sherman, 2002) interpretation of visual information processing in the brain. Here, we propose that certain thalamic association nuclei might be part of circuits that gate information flow in the cortex. Indeed, when comparing the gating mechanism of Figure 4 and the summary of the connection linking the thalamus and the cortex system in Figure 7, it appears that the links between the associative nuclei and the cortex are well suited to give these nuclei a role in controlling the flow of information between distinct cortical areas. A similar idea has been already proposed by Olshausen et al. (1993, 1995) for the pulvinar in the context of spatial attention (see Discussion).
The proposition we are presenting here is supported by two sets of experimental results. The first is the observation by MacLean et al. (2005) that the stimulation of sensory thalamic nuclei can shift up/down neurons in certain layers of the primary sensory cortex from their down to their up state. The second evidence is provided by Floresco and Grace (2003) who found that input from the mediodorsal nucleus, a large association nucleus of the thalamus, can gate the firing of prefrontal cortex neurons in response to action potentials from the hippocampus: stimulation of mediodorsal nucleus reduced the reaction of prefrontal neurons to input from the hippocampus in some of the cells tested, but it also reinforced this reaction in the remaining cells.
Figure 8 presents a possible circuit for how an association nucleus of the thalamus might gate information transmitted between cortical areas A and B. This circuit comprises the same structures described in Figure 4, starting with the pathway formed by the axons (labeled “*”) projected by area A neurons onto up/down neurons in area B. The gate-triggering mechanism here is a single thalamic neuron or a small group of such neurons. This cell is the target of excitatory projections from cortical areas A and B, and possibly from a third area labeled C. It can also be subjected to inhibitory inputs from the cortex through the inhibitory neurons of the reticular nucleus. As can be seen by comparing Figures 7 and 8, all these projections are compatible with current data on the thalamus (Sherman and Guillery, 1996; Guillery and Sherman, 2002).
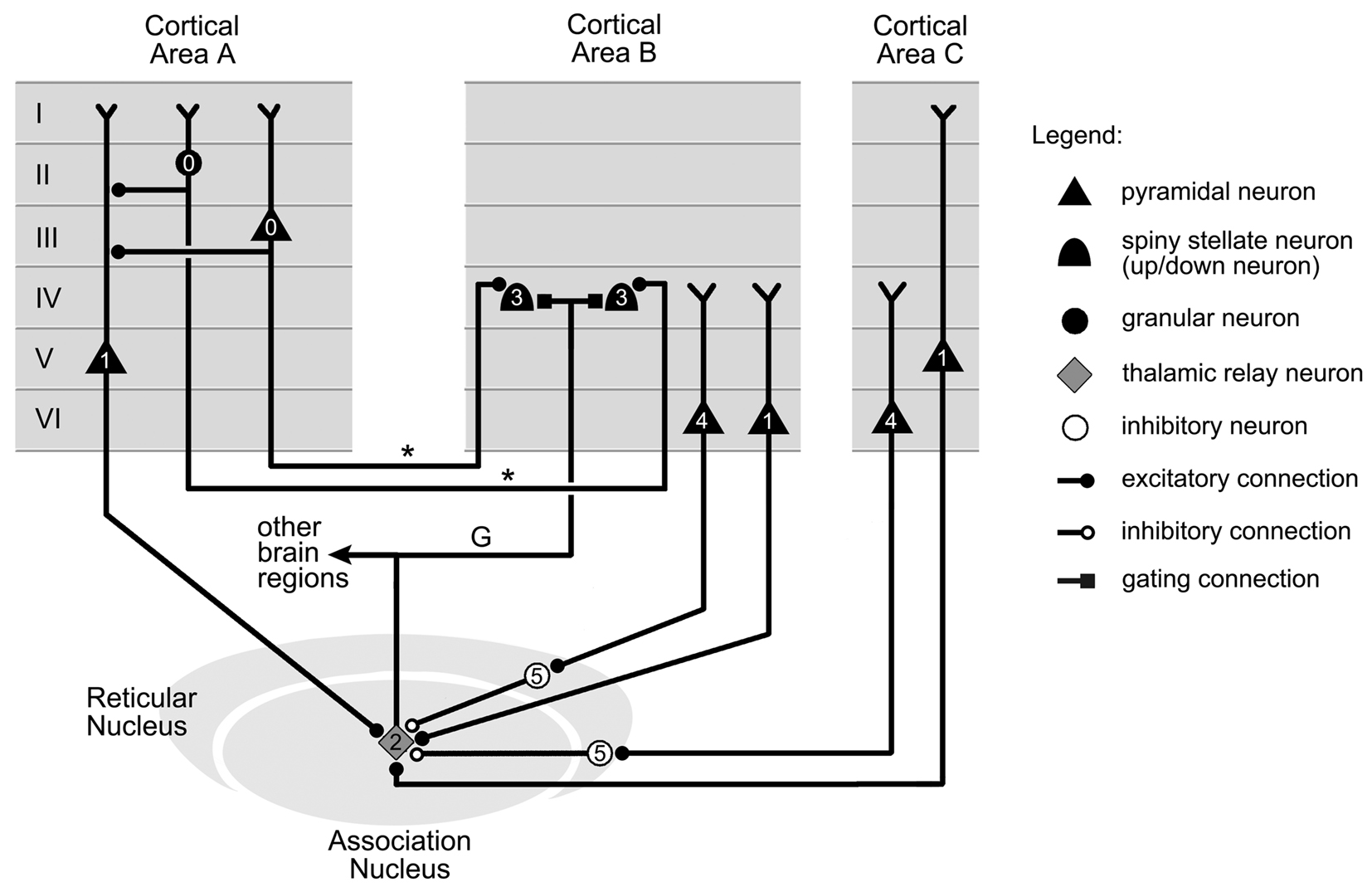
Figure 8. Thalamic gating of information flow between two cortical areas. Information in cortical area A is conveyed to up/down neurons in cortical area B by axons labeled “*.” The up/down neurons are shifted to their up state by gating axon “G” projected by a neuron in an association thalamic nucleus. The remaining connections represented target the thalamic cell. The firing order for the cells, indicated on the figure by small numbers, is as follows. Initially, the activity in cortical area A (0) is confined to this region. However, appropriate firing of neurons in areas A and C (1) can trigger the thalamic projection cell (2), which then switches area B neurons from their down to their up state (3), therefore opening the gate from area A to area B. The activity in areas B and C (4) can however close this gate by silencing the thalamic neuron through inhibitory projections from thalamic reticular nucleus neurons (5). Cell location and connections between cortical layers selected from data in Felleman and Van Essen (1991), Guillery and Sherman (2002), Zikopoulos and Barbas (2006), and Xiao et al. (2009).
The state of the gate is decided by the thalamic cell, which integrates all its afferent inputs, and produces action potentials that are relayed to up/down neurons in cortical area B. This signal shifts the up/down neurons in area B into their up state, therefore opening the gate between areas A and B. The axon (labeled “G”), which carries this gating signal, can also branch out to send copies of it to other brain regions (Figure 8).
Gating involving the basal ganglia
Directly interconnected with thalamic association nuclei are the basal ganglia, a complex of nuclei comprising the caudate nucleus (CD), the substantia nigra pars reticulata (SNr), and also the putamen, the globus pallidus (consisting of an internal segment labeled GPi, and an external segment) and the subthalamic nucleus. The structure of these nuclei, how they connect together, to the cortex and to the thalamus, and their dynamics are still under intense scrutiny. There is presently no real consensus on the role of the basal ganglia in brain functions. This role, however, seems important as evidenced by the deep motor, cognitive, and emotional deficits exhibited by patients affected by Parkinson’s disease.
A particularly interesting development in this respect is the recently published experimental work by McNab and Klingberg (2008), which indicates a role for the basal ganglia in controlling information access to working memory. Working memory is the psychological concept introduced to designate the memory used to remember a chunk of information, such as a name, for immediate use. Recordings on subjects performing working memory tasks show neural activity distributed in sensory cortex, and also in association cortex where sustained firing seems to represent certain aspects of the memorized information (Fuster and Alexander, 1971; Fuster, 2002). Many studies point to a central role in the implementation of working memory for the prefrontal cortex (Fuster, 1997) and for the massive connections linking sensory and association cortical areas (Fuster et al., 1985).
The notion of gating was introduced in this framework to explain the brain’s ability, on one hand, to give pertinent information access to working memory and, on the other, to deny access to irrelevant information: a gate into working memory would open to let the information that needs to be stored in, but would close itself afterward to keep out inappropriate information (Frank et al., 2001; Gisiger et al., 2005). Here we propose that the role played by the basal ganglia in controlling access to working memory (McNab and Klingberg, 2008) might originate from their implication in gating information propagation from sensory cortex to the prefrontal cortex (Gisiger et al., 2005). Figure 9 illustrates this scenario in the case of visual working memory. It proposes a circuit for gating visual information propagating from area TE, a higher visual area of cortex, to areas 45 and 46 of the prefrontal cortex, which are apparently implicated in working memory (Fuster, 1997).
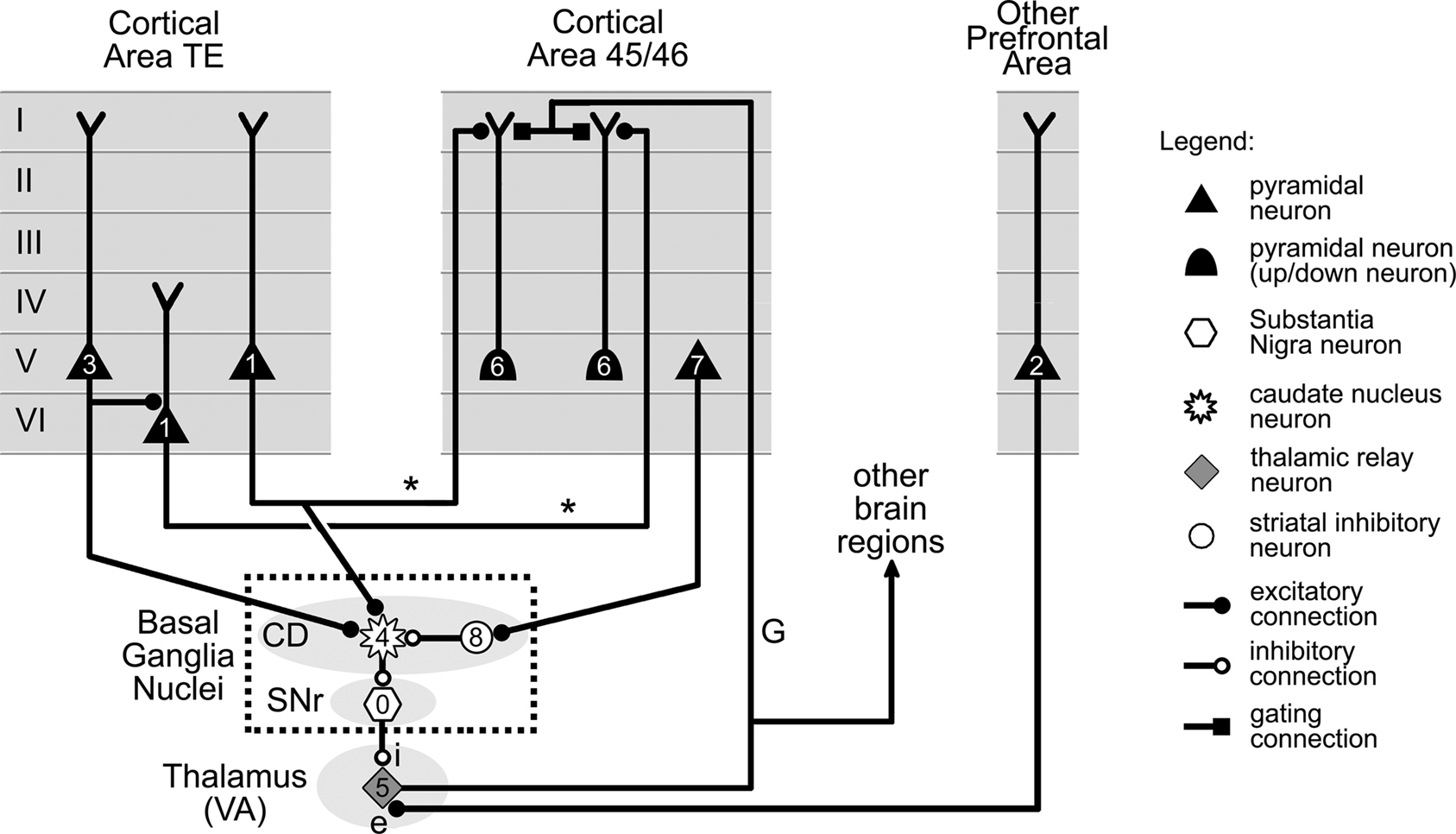
Figure 9. Basal ganglia and thalamic gating of information flow from cortical area TE to area 45/46. Information in cortical area TE is transmitted to up/down neurons in area 45/46 by axons labeled “*.” The up/down neurons are shifted to their up state by a gating axon labeled “G” projected by a VA nucleus thalamic neuron. Firing of the thalamic neuron is controlled by two distinct sets of projections. The first (labeled “i”) involves the double inhibition of the basal ganglia, and is under the control of neurons in cortical areas TE and 45/46 via CD and SNr. The second set of projections (labeled “e”) is excitatory and originates from another area of prefrontal cortex (see text). The firing order for the cells, which is indicated by small numbers, is as follows. Tonic firing of SNr neuron (0) keeps the activity in area TE (1) from reaching cells in area 45/46, despite excitation from cells (2) located in other parts of prefrontal cortex. However, suitable further activity in area TE (3) can trigger neurons in the CD (4), which then reduce the firing of SNr cells. This allows VA thalamic cells to fire (5), switching area 45/46 neurons from the down to their up state, and therefore permitting them to fire (6) in response to their TE input. Additional activity in area 45/46 (7) can still close the gate by triggering local inhibitory interneurons located in CD (8). CD: caudate nucleus, SNr: substantia nigra pars reticulata. VA: ventral anterior. Cell location and connections between cortical layers selected from data in Xiao et al. (2009), Webster et al. (1994), and Middleton and Strick (2000).
Despite the higher number of structures involved, the overall organization of the circuit is still similar to that in Figure 4, and features two pathways. The “*”pathway represents axons projected by area TE neurons onto area 45/46 neurons. It thus carries visual information from area TE into circuits in area 45/46. The second pathway involves a thalamic projection neuron belonging to the ventral anterior (VA) association nucleus of the thalamus. Similarly to the gating mechanism of the previous section (Figure 8), this neuron projects an axon (labeled “G”) to the cortex, whose role is to control the gate by shifting up/down neurons in area 45/46 to their up state.
The main difference between this gating mechanism and the previous one, however, is that the thalamic neuron is now the target of excitatory projections from a distinct area of the prefrontal cortex (labeled “e” – Figure 9), and of inhibitory projections from the basal nuclei (labeled “i” – Figure 9). Whether the gate between areas TE and 45/46 is open or closed depends on the activity of these two projections.
For simplicity, we only represented in Figure 9 two basal ganglia nuclei, i.e., the CD and the SNr, and the direct circuit that connects them together. We make the assumption here, like many other authors, that this so-called “direct pathway” of the system provides a fair approximation to the complete system for present intents and purposes (see Bar-Gad et al., 2003) for a review of theoretical models of the basal ganglia).
The key elements of this simplified system are the neurons located in the SNr. These have the particularity of being inhibitory pacemaker cells with a very high baseline firing frequency. These cells, therefore, exert a strong continuous inhibition on VA thalamic neurons, preventing them from firing even when stimulated by excitatory projections from the cortex (Figure 9). This tonic inhibition can however be temporarily lifted by neurons in the CD, which are also inhibitory, and project onto SNr neurons. The firing of CD neurons, as illustrated in Figure 9, is to a large extent controlled by projections from cortical neurons. Consequently, the gate between areas TE and 45/46 is closed by default due to the tonic activity of SNr neurons. However, adequate activity in the cortex can trigger CD neurons to inhibit SNr cell activity and thus allow VA neurons to fire and open the gate between areas TE and 45/46. It should also be noted that according to Person and Perkel (2005), just momentarily releasing the tonic inhibition of SNr neurons might also be sufficient to trigger VA neurons activity directly through the phenomenon of postinhibitory rebound, and therefore to open the gate between areas TE and 45/46.
The circuit illustrated in Figure 9 puts forward a role for the basal ganglia in managing the flow of information transmitted between area TE and prefrontal cortex. However, it should be noted that the main features of these particular pathways are not unique to areas TE, 45, and 46. Indeed, tracing studies have shown that most of the cortex projects either onto the CD, or its neighboring structure the putamen. It has also been established that output from the basal ganglia through the thalamus targets in return vast parts of the cortex (Middleton and Strick, 2000). We therefore propose the existence of other circuits with a general structure similar to that presented in Figure 9, but whose role would be to gate activity propagating between sets of cortical neurons located in other regions of the cortex, particularly in the frontal lobe (Middleton and Strick, 2000).
Discussion
Alternate Gating Architectures
A mechanism gating the access to working memory involving the basal ganglia, but differing from that presented above (Figure 9), has been proposed by the group of Frank and O’Reilly(Frank et al., 2001). Their work follows the viewpoint that information held in working memory is not sustained in circuitry contained in the prefrontal cortex, but rather maintained by “reverberating loops” harbored in the reciprocal connectivity linking the prefrontal cortex and the thalamus.
As we saw earlier, neurons in the SNr are able to exercise a strong inhibition on thalamic neurons (Figure 9). By their activity, SNr neurons can then eliminate any activity already present in these reverberating loops, or suppress any attempt to create new one there, thereby implementing a form of working memory gating (Frank et al., 2001). This gating mechanism however differs from the one presented in this article in that it does not gate information propagating between groups of neurons in different cortical regions, but rather information propagating between cortical and thalamic neurons (Frank et al., 2001).
Gating mechanisms involving the pulvinar have also been proposed in the context of visual attention (see Olshausen et al., 1993, 1995 and references therein). These authors presented a model that simulates a “window of attention” that can be directed to the parts of the visual field containing objects of interest. They achieve this by using control units that modulate the synaptic strength of the connections linking the various cortical areas of the visual system, therefore enabling the model to flexibly map parts of the visual field onto larger areas of the cortex. The model respects many features of the visual system and makes a variety of experimentally testable predictions. It however does not suggest a precise neural substrate for these control units, or the circuits that control them.
Future Developments and Lines of Research
In this article, we follow up on earlier work proposing that mechanisms gating the flow of information in the cortex are key elements of brain circuitry (Bressler, 1995; Gisiger et al., 2005; Gisiger and Kerszberg, 2006). We started by reviewing work by Grace and colleagues (O’Donnell and Grace, 1995; Grace, 2000; Goto and O’Donnell, 2001) linking bistable neurons with the notion of gating of information flow in the brain. We then reviewed several mechanisms gating information in the cortex (Figures 2 and 3). We next put forward a general architecture for mechanisms gating information transmission between two cortical regions, and listed some of the features that would allow such a system to fit in the overall circuitry of the brain (Figures 4 and 5). We then showed that current data on the connectivity of the cortex, thalamus, and basal ganglia might support at least three types of gating circuits responding to the established features: one that is controlled by the cortex (Figure 6), one that is controlled by association nuclei in the thalamus (Figure 8), and one that is controlled by circuits spanning the basal ganglia, and the thalamic nuclei that are interconnected with them (Figure 9). Table 2 summarizes these findings.
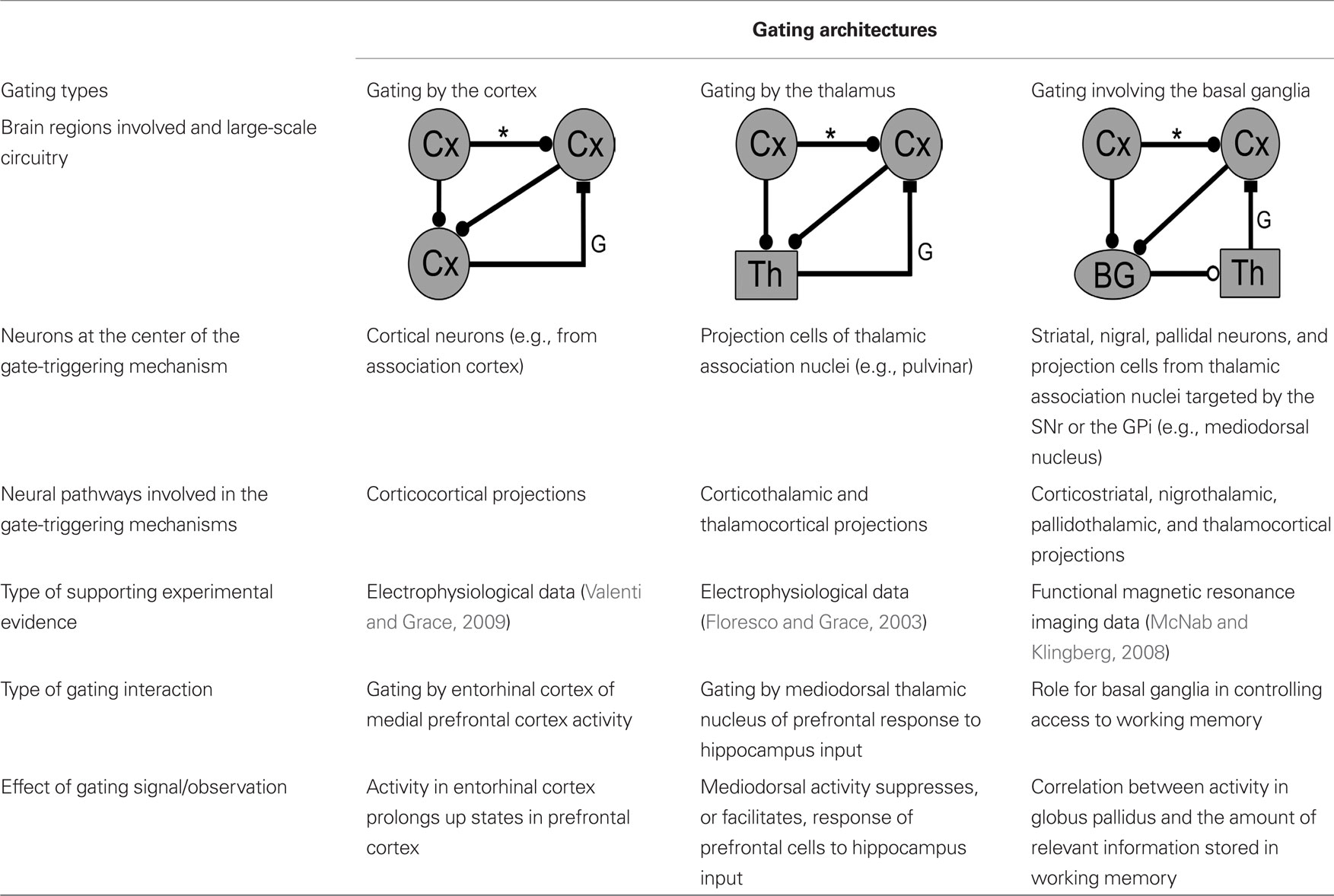
Table 2. Summary of currently available data on gating mechanisms controlled by the cortex, the thalamus and the basal ganglia (same graphic conventions as in Figure 4).
Experimental data upholding the notion of gating in the cortex by the cortex itself is currently incomplete. Indeed, while it has been observed that projections from one cortical area can influence the state of up/down neurons located in another cortical region (Valenti and Grace, 2009), it remains to be shown that neurons in one cortical region can gate information transmission between neurons located in two other cortical areas.
Evidence supporting gating by the thalamus is more solid, with the direct observation that activity in the mediodorsal thalamic nucleus influences the response of prefrontal cells to input from the hippocampus (Floresco and Grace, 2003). In some cases, this response is enhanced, as it would be expected from a mechanisms such as that depicted in Figure 8, while in others it is suppressed, consistently with the inhibitory mechanism of Figure 3A. Also, as was discussed earlier, the connectivity linking thalamic association nuclei with the cortex seems well suited to gating information transmission between cortical areas. However, more studies are needed to better characterize the cellular mechanisms at play when input from the mediodorsal nucleus interacts with information processing in the cortex, and to investigate whether other thalamic association nuclei have similar effect on the transmission of cortical information.
The third proposed gating architecture involved the thalamus and the basal ganglia. Here, due to the size and complexity of the circuitry involved, evidence comes mostly from imaging studies on the role of the basal ganglia in managing access to working memory (McNab and Klingberg, 2008). Further investigations will be needed to test this observation. Of particular importance will be the determination of whether thalamic nuclei receiving input from the basal ganglia, such as the VA nucleus, can gate information transmission in the cortex. As mentioned earlier, the mediodorsal nucleus, which is also the target of projections from the basal ganglia, has already been shown to act in this manner (Floresco and Grace, 2003).
A better understanding of the nature of bistable cortical neurons and of their role in cortical dynamics would also be needed. Indeed, study of bistable neurons in the Nacc has provided extremely precise information on their role as gates relative to information from the hippocampus and the prefrontal cortex. However, it has not yet been clearly demonstrated whether the bistable neurons observed in the cortex also implement a gating function there and, if so, what the mechanisms underlying this function may be.
Also, while many studies have focused on mechanisms that can shift bistable neurons to their up state, few results have been published thus far on the reverse processes: allowing bistable neurons to return to their down state. This is a crucial point since, for acting in a timely fashion and to be useful to an organism, a gating circuit should also include powerful reset mechanisms to close the gate when required. Shu et al. (2003) showed that stimulation of slices of the prefrontal cortex can shift neurons into a sustained, firing up state, while a subsequent similar stimulation brings them back to a down, quiescent state. These results seem to argue for a role of inhibitory interneurons in the termination of sustained activity. These preliminary results however only considered up/down state transitions as means to sustain information in neural circuits. Loewenstein et al. (2005), working on the cerebellum, found similarly induced transtions between up and down states for Purkinje cells relative to their climbing fibers inputs. Comparable studies focusing on these transitions in the context of gating of cortical information are needed.
We end this article with some considerations on the role of gating in brain dynamics. As was initially proposed by Bressler (1995), and later confirmed by numerical simulations (Gisiger et al., 2005; Gisiger and Kerszberg, 2006), by opening and closing the lines of communications linking neural assemblies in a timely fashion, sequences of computations can be produced, which then amount to structured behavior such as that required by simple memory tasks. However, it was not mentioned by Bressler (1995) how these gating units would obtain their activity. In numerical studies (Gisiger et al., 2005; Gisiger and Kerszberg, 2006), the firing patterns of the gating units in the model were set by hand at the start of simulation, and were therefore part of the initial parameters of the networks. The question is then whether circuits such as those illustrated in Figures 4, 6, 8, and 9 could generate the sequences of gating events necessary to produce structured behavior.
As was illustrated in Figure 5, the axon carrying the gating signal, and therefore information on the current state of the gating mechanism, can branch out and send a copy of this information to other regions of the brain. It is therefore possible for this axon to contact neurons in the gate-triggering circuit of other gating mechanisms, and to take part in the decision to open or close these other gates. Gating mechanisms such as those illustrated in Figures 4, 6, 8, and 9 could then in theory interact with each other, and be linked together so as to produce ordered sequences of gating events (Figure 5). Interestingly, it has been demonstrated that the basal ganglia are critically implicated in the performance of specific innate sequences of grooming gestures in the rodents (Aldridge et al., 2004). Circuits spanning the basal ganglia such as those depicted in Figure 9 and linked as illustrated in Figure 5, might propose a possible implementation for how these precise motor sequences are hardwired.
Alternatively, these circuits could also be wired so as to inhibit one another, produce feedback loops, or to form other more complex architectures. Altogether, the gating mechanisms described here, and the way they interact with one another, might provide new insight into how the brain integrates neural computations occurring in various cortical regions, and produces structured behavior.
Conflict of Interest Statement
The authors declare that the research was conducted in the absence of any commercial or financial relationships that could be construed as a potential conflict of interest.
Acknowledgments
We thank A. and M. Parent, and M. Deschênes for many interesting and stimulating discussions. Thomas Gisiger also thanks R.-M. and V. Gisiger for their support throughout this work, as well as I. Igreja for insightful discussions.
References
Aldridge, J. W., Berridge, K. C., and Rosen, A. R. (2004). Basal ganglia neural mechanism of natural movement sequences. Can. J. Physiol. Pharmacol. 82, 732–739.
Anderson, C. H., and Van Essen, D. C. (1987). Shifter circuits: a computational strategy for dynamic aspects of visual processing. Proc. Natl. Acad. Sci. U.S.A. 84, 6297–6301.
Barbas, H., and Zikopoulos, B. (2007). The prefrontal cortex and flexible behavior. Neuroscientist 13, 532–545.
Bar-Gad, I., Morris, G., and Bergman, H. (2003). Information processing, dimensionality reduction and reinforcement learning in the basal ganglia. Prog. Neurobiol. 71, 439–473.
Borg-Graham, L. J., Monier, C., and Frégnac, Y. (1998). Visual input evokes transient and strong shunting inhibition in visual cortical neurons. Nature 393, 369–373.
Burchell, T. R., Faulkner, H. J., and Whittington, M. A. (1998). Gamma frequency oscillations gate temporally coded afferent inputs in the rat hippocampal slice. Neurosci. Lett. 255, 151–154.
Cardin, J. A., Carlén, M., Meletis, K., Knoblich, U., Zhang, F., Deisseroth, K., Tsai, L.-H., and Moore, C. I. (2009). Driving fast-spiking cells induces gamma rhythm and controls sensory responses. Nature 459, 663–667.
Chelazzi, L., Miller, E. K., Duncan, J., and Desimone, R. (1993). A neural basis for visual search in inferior temporal cortex. Nature 363, 345–347.
Cossart, R., Aronov, D., and Yuste, R. (2003). Attractor dynamics of network UP states in the neocortex. Nature 423, 283–288.
Cowan, R. L., and Wilson, C. W. (1994). Spontaneous firing patterns and axonal projections of single corticostriatal neurons in the rat medial agranular cortex. J. Neurophysiol. 71, 17–32.
Dégenètais, E., Thierry, A.-M., Glowinski, J., and Gioanni, Y. (2003). Synaptic influence of hippocampus on pyramidal cells of the rat prefrontal cortex: an in vivo intracellular recording study. Cereb. Cortex 13, 782–792.
Fatt, P., and Katz, B. (1953). The effect of inhibitory nerve impulses on a crustacean muscle fiber. J. Physiol. 121, 374–389.
Felleman, D. J., and Van Essen, D. C. (1991). Distributed hierarchical processing in the primate cerebral cortex. Cereb. Cortex 1, 1–47.
Floresco, S. B., and Grace, A. A. (2003). Gating of hippocampal-evoked activity in prefrontal cortical neurons by inputs from the mediodorsal thalamus and ventral tegmental area. J. Neurosci. 23, 3930–3943.
Frank, M. J., Loughry, B., and O’Reilly, R. C. (2001). Interactions between frontal cortex and basal ganglia in working memory: a computational model. Cogn. Affect. Behav. Neurosci. 1, 137–160.
Fukai, T. (1999). Sequence generation in arbitrary temporal patterns from theta-nested gamma oscillations: a model of the basal ganglia–thalamo-cortical loops. Neural Netw. 12, 975–987.
Fuster, J. M. (1997). The Prefrontal Cortex: Anatomy, Physiology and Neuropsychology of the Frontal Lobe. Philadelphia, NY: Lippincott-Raven Publishers.
Fuster, J. M., and Alexander, G. E. (1971). Neuron activity related to short-term memory. Science 173, 652–654.
Fuster, J. M., Bauer, R. H., and Jervey, J. P. (1985). Functional interactions between inferotemporal and prefrontal cortex in a cognitive task. Brain Res. 25, 299–307.
Gisiger, T., and Kerszberg, M. (2006). A model for integrating elementary neural functions into delayed-response behavior. PLoS Comput. Biol. 2, e25. doi: 10.1371/journal.pcbi.0020025
Gisiger, T., and Kerszberg, M. (2007). From a representation of behavior to the concept of cognitive syntax: a theoretical framework. Prog. Brain Res. 165, 463–474.
Gisiger, T., Kerszberg, M., and Changeux, J.-P. (2005). Acquisition and performance of delayed-response tasks: a neural network model. Cereb. Cortex 15, 489–506.
Goto, Y., and O’Donnell, P. (2001). Synchronous activity in the hippocampus and nucleus accumbens in vivo. J. Neurosci. 21, RC131.
Grace, A. A. (2000). Gating of information flow within the limbic system and the pathophysiology of schizophrenia. Brain Res. Rev. 31, 330–341.
Guillery, R. W., and Sherman, S. M. (2002). Thalamic relay functions and their role in corticocortical communication: generalizations from the visual system. Neuron 33, 163–175.
Katz, P. S., and Frost, W. N. (1996). Instrinsic neuromodulation: altering neuronal circuits from within. Trends Neurosci. 19, 54–61.
Kepecs, A., and Raghavachari, S. (2007). Gating information by two-state membrane potential fluctuations. J. Neurophysiol. 97, 3015–3023.
Koch, C., Poggio, T., and Torre, V. (1983). Nonlinear interactions in a dendritic tree: localization, timing, and role in information processing. Proc. Natl. Acad. Sci. U.S.A. 80, 2799–2802.
Koene, R. A., and Hasselmo, M. E. (2005). An integrate-and-fire model of prefrontal cortex neuronal activity during performance of goal-directed decision making. Cereb. Cortex 15, 1964–1981.
Lewis, B. L., and O’Donnell, P. (2000). Ventral tegmental area afferents to the prefrontal cortex maintain membrane potential “Up” states in pyramidal neurons via D1 dopamine receptors. Cereb. Cortex 10, 1168–1175.
Lisman, J. E., and Idiart, M. A. (1995). Storage of 7± 2 short-term memories in oscillatory subcycles. Science 267, 1512–1515.
Loewenstein, Y., Mahon, S., Chadderton, P., Kitamura, K., Sompolinsky, H., Yarom, Y., and Häusser, M. (2005). Bistability of cerebellar Purkinje cells modulated by sensory stimulation. Nat. Neurosci. 8, 202–211.
MacLean, J. N., Watson, B. O., Aaron, G. B., and Yuste, R. (2005). Internal dynamics determine the cortical response to thalamic stimulation. Neuron 48, 811–823.
McNab, F., and Klingberg, T. (2008). Prefrontal cortex and basal ganglia control access to working memory. Nat. Neurosci. 11, 103–107.
Middleton, F. A., and Strick, P. L. (2000). Basal ganglia and cerebellar loops: motor and cognitive circuits. Brain Res. Rev. 31, 236–250.
O’Donnell, P., and Grace, A. A. (1995). Synaptic interactions among excitatory afferents to nucleus accumbens neurons: hippocampal gating of prefrontal cortical input. J. Neurosci. 15, 3622–3639.
Olshausen, B. A., Anderson, C. H., and Van Essen, D. C. (1993). A neurobiological model of visual attention and invariant pattern recognition based on dynamic routing of information. J. Neurosci. 13, 4700–4719.
Olshausen, B. A., Anderson, C. H., and Van Essen, D. C. (1995). A multiscale dynamic routing circuit for forming size- and position-invariant object representations. J. Comput. Neurosci. 2, 45–62.
Paik, S.-B., and Glaser, D. A. (2010). Synaptic plasticity controls sensory responses through frequency-dependent gamma oscillation resonance. PLoS Comput. Biol. 6, e1000927. doi: 10.1371/journal.pcbi.1000927
Parga, N., and Abbott, L. F. (2007). Network model of spontaneous activity exhibiting synchronous transitions between up and down states. Front. Neurosci. 1:1. doi: 10.3389/neuro.01.1.1.004.2007
Person, A. L., and Perkel, D. J. (2005). Unitary IPSPs drive precise thalamic spiking in a circuit required for learning. Neuron 46, 129–140.
Reichova, I., and Sherman, S. M. (2004). Somatosensory corticothalamic projections: drivers from modulators. J. Neurophysiol. 92, 2185–2197.
Sakai, K., Rowe, J. B., and Passingham, R. E. (2002). Active maintenance in prefrontal area 46 creates distractor-resistant memory. Nat. Neurosci. 5, 479–484.
Sherman, S. M., and Guillery, R. W. (1996). Functional organization of thalamocortical relays. J. Neurophysiol. 76, 1367–1395.
Shu, Y., Hasenstaub, A., and McCormick, D. A. (2003). Turning on and off recurrent balanced cortical activity. Nature 423, 288–293.
Torre, V., and Poggio, T. (1978). A synaptic mechanism possibly underlying directional selectivity to motion. Proc. R. Soc. Lond. (Biol.) 202, 409–416.
Valenti, O., and Grace, A. A. (2009). Entorhinal cortex inhibits medial prefrontal cortex and modulates the activity states of electrophysiologically characterized pyramidal neurons in vivo. Cereb. Cortex 19, 658–674.
Van Essen, D. C. (2005). Corticocortical and thalamocortical information flow in the primate visual system. Prog. Brain Res. 149, 173–185.
Vogels, T. P., and Abbott, L. F. (2005). Signal propagation and logic gating in networks of integrate-and-fire neurons. J. Neurosci. 25, 10786–10795.
Vogels, T. P., and Abbott, L. F. (2009). Gating multiple signals through detailed balance of excitation and inhibition in spiking networks. Nat. Neurosci. 12, 483–491.
Webster, M. J., Bachevalier, J., and Ungerleider, L. G. (1994). Connections of inferior temporal areas TEO and TE with parietal and frontal cortex in macaque monkeys. Cereb. Cortex 5, 470–483.
Wilson, C. J. (1993). The generation of natural firing patterns in neostriatal neurons. Prog. Brain Res. 991, 277–297.
Wilson, C. J., and Kawaguchi, Y. (1996). The origin of two-state spontaneous membrane potential fluctuations of neostriatal spiny neurons. J. Neurosci. 16, 2397–2410.
Xiao, D., Zikopoulos, B., and Barbas, H. (2009). Laminar and modular organization of prefrontal projections to multiple thalamic nuclei. Neuroscience 161, 1067–1081.
Keywords: gating, bistable neurons, up and down states, cortex, thalamus, basal ganglia, information flow, neural circuits
Citation: Gisiger T and Boukadoum M (2011) Mechanisms gating the flow of information in the cortex: what they might look like and what their uses may be. Front. Comput. Neurosci. 5:1. doi: 10.3389/fncom.2011.00001
Received: 29 April 2010;
Paper pending published: 26 August 2010;
Accepted: 04 January 2011;
Published online: 19 January 2011.
Edited by:
Nicolas Brunel, Centre National de la Recherche Scientifique, FranceReviewed by:
Adam Kepecs, Cold Spring Harbor Laboratory, USATim P. Vogels, école Polytechnique Fédérale de Lausanne, Switzerland.
Copyright: © 2011 Gisiger and Boukadoum. This is an open-access article subject to an exclusive license agreement between the authors and Frontiers Media SA, which permits unrestricted use, distribution, and reproduction in any medium, provided the original authors and source are credited.
*Correspondence: Thomas Gisiger, Département d’informatique, Université du Québec à Montréal, Case Postale 8888, Succursale Centre-Ville, Montréal, QC, Canada H3C 3P8. e-mail: gisiger.thomas@uqam.ca