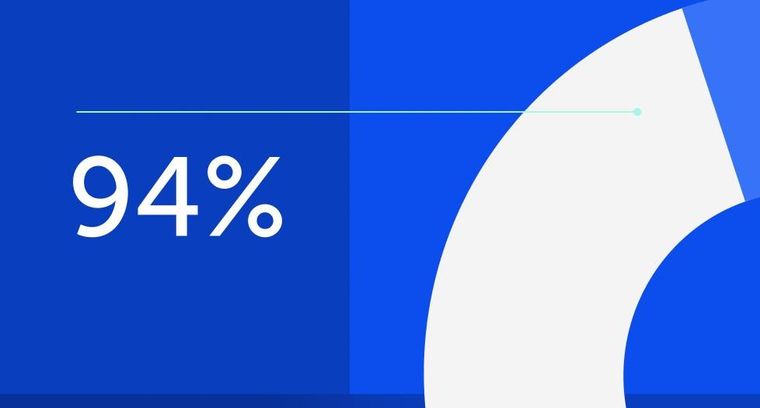
94% of researchers rate our articles as excellent or good
Learn more about the work of our research integrity team to safeguard the quality of each article we publish.
Find out more
MINI REVIEW article
Front. Neural Circuits, 08 July 2024
Volume 18 - 2024 | https://doi.org/10.3389/fncir.2024.1430783
This article is part of the Research TopicNeural Circuit Formation and Sensory InputsView all 21 articles
Early life experiences shape physical and behavioral outcomes throughout lifetime. Sensory circuits are especially susceptible to environmental and physiological changes during development. However, the impact of different types of early life experience are often evaluated in isolation. In this mini review, we discuss the specific effects of postnatal sensory experience, sleep, social isolation, and substance exposure on barrel cortex development. Considering these concurrent factors will improve understanding of the etiology of atypical sensory perception in many neuropsychiatric and neurodevelopmental disorders.
Early life experiences can have profound and life-long physical, emotional, and behavioral consequences (Felitti et al., 1998; Hughes et al., 2017; Lokhandwala and Spencer, 2022). During postnatal development, maturation of circuits involved in sensory perception occurs first, and this process is particularly sensitive to physiological and environmental influences (Chang and Merzenich, 2003; Maitre et al., 2017; Badde et al., 2020; reviews: Hensch, 2004; Fox et al., 2010). In rodents, whisker-mediated tactile input is one of the earliest developed sensory modalities (Akhmetshina et al., 2016; Smirnov and Sitnikova, 2019). Tactile inputs are present at birth, while visual and auditory inputs do not occur until the second postnatal week in mice (Colonnese et al., 2010; Akhmetshina et al., 2016; Makarov et al., 2021). This feature allows tactile sensory inputs to interact with intrinsic programs to uniquely shape sensorimotor circuit maturation (Bragg-Gonzalo et al., 2021).
The developing somatosensory cortex is dominated by synchronous neural activity until the end of the second postnatal week (Khazipov et al., 2004; Minlebaev et al., 2007; Golshani et al., 2009; Tolner et al., 2012; Yang et al., 2013). Its precise patterns and timing are essential for many activity-dependent processes during early postnatal development (Winnubst et al., 2015; Wong et al., 2018; Duan et al., 2020; review: Leighton and Lohmann, 2016). Such activity has been suggested to originate from at least four sources: (1) external, passive tactile sensory inputs onto the whiskers from the dam, littermates, and nesting materials (Akhmetshina et al., 2016); (2) sensorimotor feedback generated by involuntary whisker and limb twitches (Khazipov et al., 2004; Tiriac et al., 2014; Dooley et al., 2020); (3) cross-modal sensory inputs, such as odor-driven activity via direct early excitatory connections from the olfactory cortex to somatosensory cortex (Henschke et al., 2018; Cai et al., 2024); 4) internally generated, spontaneous neural activity independent from the periphery or movement (Moreno-Juan et al., 2017; Mizuno et al., 2018; Nakazawa et al., 2020; Banerjee et al., 2022). Multiple lines of evidence support that activity from these different sources co-exist in the first two postnatal weeks. While spontaneously occurring and whisker stimulation-induced oscillatory activity are both present already at birth, inactivation of the tactile sensory periphery with lidocaine, which occludes tactile sensory inputs and sensorimotor feedback from whisker twitches, reduces but does not abolish synchronous activity (Yang et al., 2009). In addition, barrel column-related patchwork activity in L4 excitatory neurons is uncorrelated with whisker movement and does not disappear after infraorbital nerve transection at P4 (Mizuno et al., 2018; Nakazawa et al., 2018). There is still considerable ongoing debate over whether the four sources of activity mentioned above are distinct – for example, what proportion of activity that has been attributed as “internally-generated activity” is due to sensorimotor feedback given that the circuitry enabling movement-related sensory feedback is already functional in the thalamus embryonically (Antón-Bolaños et al., 2019; Dooley et al., 2020; Blumberg et al., 2022). Latest data demonstrating that synchronous activity in the barrel cortex can be driven by chemosensation also raises the possibility that the remaining synchronous activity after inactivation of tactile sensory periphery could have, at least in part, originated from other sensory modalities (Cai et al., 2024; Wang et al., 2024). Further investigations will be required to confirm or resolve potential overlaps between sources of early neural activity in the barrel cortex.
Given the complexity of early neural activity as discussed above, in this minireview we will focus on summarizing the role of the following early-life sensory experiences on the development of somatosensory cortex: (1) external tactile sensory inputs; (2) sleep in the context of permitting sensorimotor feedback; (3) recently discovered cross-modal olfactory sensory inputs. While there might be overlap in mechanisms, we will not focus on internally generated intrinsic activity here (review: Leighton and Lohmann, 2016). We will also discuss recent findings that have uncovered the impact of other early-life factors, including isolation and substance exposure (Figure 1).
Figure 1. Types of Early Life Experiences and Susceptible Developmental Programs in the Barrel Cortex. In rodents, early sensory experience, sleep, social isolation, and perinatal substance exposure can alter the development of the barrel cortex. Different developmental programs may be affected by these factors across varying sensitive periods. These programs include thalamocortical innervation, intracortical circuit assembly (including maturation of excitatory and inhibitory circuits), experience-dependent synaptic remodeling, and synchronous cortical activity. Figure created with Biorender.com.
In the whisker sensory system, tactile sensory input to the whiskers is transduced by mechanoreceptors in each whisker follicle on the snout, then carried by afferent fibers to the trigeminal nucleus of the brainstem, the ventroposteromedial nucleus of the thalamus (VPM) and finally to the somatosensory cortex (reviews: Petersen, 2007; Diamond et al., 2008). In layer 4 (L4) of the barrel cortex, cytoarchitectonic cylindrical structures known as “barrels” correspond to individual whiskers (Woolsey and van der Loos, 1970). Synaptic connections of within barrel cortex have been extensively characterized, making it an excellent model for investigating experience-dependent circuit maturation. Peripheral manipulations have been widely used and demonstrated to produce marked alterations in the structure and function of the developing barrel cortex. These studies provide a wealth of information on the age-, layer-, cell type-, and synaptic connection-specific effects of postnatal sensory experience (review: Erzurumlu and Gaspar, 2012). Below we will summarize effects of tactile sensory deprivation via peripheral manipulations on barrel cortex synaptic connectivity (thalamocortical and intracortical), neuronal maturation, and behavior.
Thalamocortical afferents (TCAs) to the barrel cortex play a critical role in instructing barrel formation and organization, dendritic remodeling, and neuronal maturation (Li et al., 2013; Matsui et al., 2013; Pouchelon et al., 2014; Young et al., 2023). Disruption of presynaptic vesicle fusion, glutamatergic neurotransmission, or cAMP signaling at thalamocortical synapses alters barrel patterning (Abdel-Majid et al., 1998; Iwasato et al., 2000; Inan et al., 2006; Ballester-Rosado et al., 2010; Narboux-Nême et al., 2012; Li et al., 2013; Suzuki et al., 2015). The TCAs are also particularly sensitive to sensory deprivation. When sensory input is eliminated by infra-orbital nerve transection or whisker follicle cauterization before postnatal day (P) 3, TCA arbors no longer cluster and cylindrical barrels are replaced by elongated fused bands that extend through L4 of the barrel field (Van der Loos and Woolsey, 1973; Killackey and Belford, 1979; Wong-Riley and Welt, 1980; Jeanmonod et al., 1981; Jensen and Killackey, 1987). Similar manipulations that result in permanent peripheral damage at later timepoints, between P4 and P6, lead to TCA disorganization but not a complete loss of barrel structure, while normal barrel patterns are preserved when manipulations occur after P6 (Woolsey and Wann, 1976; Belford and Killackey, 1980; Jeanmonod et al., 1981; Bates and Killackey, 1985; Higashi et al., 1999). Neonatal whisker trimming or plucking, which limits tactile sensory experience without causing peripheral structural damage, does not alter the cytoarchitectonic barrel structure; instead, it leads to changes in the strength of thalamocortical and intracortical synapses (Finnerty et al., 1999; Allen et al., 2003; Sadaka et al., 2003; Schierloh et al., 2004; Bender et al., 2006; Chittajallu and Isaac, 2010; Simons et al., 2015). For instance, the density and efficacy of thalamocortical synapses decrease following chronic whisker trimming (Sadaka et al., 2003). Following extended whisker regrowth, thalamic drive to excitatory neurons rebounds to above control levels, suggesting that sensory inputs can restore and even heighten the efficacy of thalamocortical synapses after early-life manipulations (Simons et al., 2015). Sensory deprivation also weakens intracortical synapses at L4-to-L2/3 connections and increases lateral synaptic transmission across barrel columns, resulting in increased neuronal responses to deflection of surrounding non-principal whiskers (Fox, 1992; Finnerty et al., 1999; Lendvai et al., 2000; Stern et al., 2001; Allen et al., 2003; Shepherd et al., 2003; Schierloh et al., 2004; Bender et al., 2006; Lee et al., 2007). When whisker regrowth is allowed, L4 neurons continue to show broadened sensory-evoked responses (Simons and Land, 1987; Shoykhet et al., 2005). In summary, these studies establish that sensory experience from the periphery plays a central role in regulating thalamocortical and intracortical circuit assembly during development.
During the early postnatal period, cortical GABAergic interneurons also receive significant thalamic inputs and shape circuit function in a sensory experience-dependent manner. In the first postnatal week, direct TCAs from the VPM preferentially form synaptic connections with select interneuron subtypes, such as somatostatin-expressing (SST) interneurons in L5 and Reelin-expressing (Re) interneurons in L1 (Marques-Smith et al., 2016; Tuncdemir et al., 2016; Che et al., 2018; Pouchelon et al., 2021). These thalamic inputs to interneurons are dynamically remodeled during development−VPM inputs to SST, Re, and Vasoactive intestinal peptide-expressing (VIP) interneurons weaken by the end of the second postnatal week, while VPM inputs to parvalbumin-expressing (PV) interneurons and PV-driven feed-forward inhibition continue to strengthen (Daw et al., 2007; Che et al., 2018; Kastli et al., 2020; Modol et al., 2020). The transient TCA connections are required for the maturation of stable circuit connectivity that persists into adulthood (Anastasiades et al., 2016; Marques-Smith et al., 2016; Tuncdemir et al., 2016). It has been hypothesized that interneurons are more sensitive to early-life sensory experience due to their protracted postnatal development (review: Micheva and Beaulieu, 1997). This hypothesis is supported by recent studies demonstrating that sensory deprivation specifically reduces VPM to interneuron inputs and interneuron activity, resulting in delayed cell maturation and alterations in intracortical connectivity (Chittajallu and Isaac, 2010; Marques-Smith et al., 2016; Che et al., 2018; Duan et al., 2020; Bollmann et al., 2023). Whisker plucking from the first postnatal week to adulthood decreases L4 surround inhibition, inhibitory synaptic strength, and the total number of interneurons (Micheva and Beaulieu, 1995a,b; Shoykhet et al., 2005; Gainey et al., 2016). Taken together, these studies demonstrate that developing interneurons are uniquely positioned to relay thalamic inputs and regulate responses to early sensory experiences, thus playing a significant role in shaping cortical circuit formation and function.
Early sensory deprivation also has profound behavioral consequences. Infant-trimmed rodents can differentiate between rough and smooth textures but show difficulty distinguishing between two distinct rough textures (Carvell and Simons, 1996). This is thought to be a consequence of permanent deficits in surround inhibition, which decreases the animal’s perceptual ability in tasks that require information from multiple whiskers (Carvell and Simons, 1996; Shoykhet et al., 2005). Trimming also alters explorative whisking and behavioral strategies during gap-crossing tasks (Lee et al., 2009; Papaioannou et al., 2013). Intriguingly, the effects of early sensory deprivation are especially evident in socio-cognitive behavioral tasks. Animals that have been vibrissectomized are more social and explorative, displaying less emotional reactivity and diminished early withdrawal response from novel tactile stimuli (Shishelova, 2006; Lee et al., 2009; Soumiya et al., 2016; Wang et al., 2022). Furthermore, sensory deprivation through whisker removal reduces excitatory synaptic transmission as well as the synthesis and secretion of the neuropeptide oxytocin, while both oxytocin injection and increased sensory experience rescues excitatory synaptic transmission (Zheng et al., 2014). Nevertheless, it remains to be determined through what specific mechanisms early tactile sensory experience influences the development of higher-order social, emotional, and cognitive function.
Similar to human infants, neonatal rodents spend the majority of their time sleeping (Blumberg et al., 2022). The younger the animal, the more time it spends in active (rapid-eye-movement, REM) sleep states (Jouvet-Mounier et al., 1969). During the first two weeks of life, rodents spend between 45 to 80% of their time in REM sleep, 20 to 35% in wakeful states, and up to 25% in non-REM sleep, while in adult rodents, REM sleep time decreases to 8.5% and time spent awake rises to 43% (Jouvet-Mounier et al., 1969; Blumberg et al., 2022). As discussed previously, sensorimotor feedback generated by myoclonic twitches is a main source of early synchronous activity in the barrel cortex (Blumberg et al., 2022). Recent studies correlating electrophysiological recordings of barrel cortex activity with whisker motion show that up to 75% of spontaneous somatosensory cortical activity is directly related to passive whisker movement (Akhmetshina et al., 2016; Dooley et al., 2020). These twitches during REM sleep are more likely to generate network activity in the barrel cortex than movements during wakefulness (Dooley et al., 2020). Therefore, REM sleep during development serves to enable muscle twitches important for activity-dependent circuit maturation in the barrel cortex. In support of this, REM sleep during development has been shown to support heightened synaptic remodeling, in particular synaptic elimination (Yang and Gan, 2012; Li et al., 2017; Zhou et al., 2020; review: Sun et al., 2020), a process essential for the development of mature and functional circuits (Faust et al., 2021). In addition, REM sleep increases coherence between distant brain regions, facilitating the formation of long-range connections that direct complex sensory-based behaviors in adulthood (Rio-Bermudez et al., 2020).
Early-life sleep deprivation may also alter inhibitory circuits – persistent decrease in PV interneuron number has been reported in adult voles whose sleep was disrupted between P14 and P21 (Jones et al., 2019). Behaviorally, prairie voles that were sleep-deprived as neonates display aberrant exploratory behavior and pair bond formation during adulthood (Jones et al., 2019). Together, these findings indicate that REM sleep is essential for synaptic remodeling during early life, and therefore may have far-reaching behavioral effects.
While multisensory integration via direct thalamocortical and intracortical connections across modalities in adults is well appreciated (review: Driver and Noesselt, 2008), less is known about when multisensory connectivity is established, and whether it contributes to the maturation of and the early activity in primary sensory cortices. In developing Mongolian gerbils, several thalamic nuclei project to two or more sensory cortices between P1-P9, and the non-matched modality projections are pruned away after P15 (Henschke et al., 2018). Direct connections between primary visual, auditory, and somatosensory cortices occur later than multisensory interconnections at the thalamic level, occurring at the onset of sensory experience around P15. In addition, the number of multisensory connections drastically increases following the loss of early sensory experience (Henschke et al., 2018), suggesting that sensory experience from other modalities is able to alter the development and function of the somatosensory cortex during early postanal development. In support of this notion, whisker deprivation or dark rearing reduces excitatory synaptic transmission in the correspondent sensory cortex and cross-modally in other sensory cortices through an oxytocin-dependent mechanism (Zheng et al., 2014).
Direct intracortical excitatory connections have also been demonstrated to trigger early synchronous activity in the somatosensory cortex. In mouse slice culture between embryonic day (E)18-P12, waves of spontaneous electrical activity initiate in the septum and ventral cortex and propagate dorsally across the cortex, including the somatosensory region (Conhaim et al., 2011). This is consistent with the recent finding that there is direct, transient excitatory connectivity from the olfactory cortex to the somatosensory cortex, and in vivo odor-driven activity propagates broadly across the cortex during the first postnatal week (Cai et al., 2024). This odor-evoked activity enhances whisker-evoked activity in the barrel cortex, while neonatal odor deprivation leads to somatosensory defects in adult mice, suggesting that there is a cross-modal critical window for nasal chemosensation-dependent somatosensory function maturation (Cai et al., 2024). Interestingly, recent work quantifying prenatally active neurons in mice using Targeted Recombination in Active Populations (TRAP) identified the piriform cortex as the most abundantly TRAPed region, indicating that early piriform neurons may represent an interconnected hub-like population whose activity promotes recurrent connectivity in the developing cortex (Wang et al., 2024). In summary, early cross-modal sensory experience, in particular olfactory inputs, can have large impact on the proper maturation and function of the somatosensory cortex.
During early postnatal life, social isolation is a well-documented stressor that can alter cortical synaptic function. Social isolation in pups has been shown to affect synaptic spine density in the barrel cortex, though the effects vary with different isolation protocols (Bock et al., 2005; Takatsuru et al., 2009). When neonatal rodents are isolated for 6 hours daily during 3-day-long periods in the first and second postnatal week, AMPA receptor trafficking in L4-to-L2/3 synapses in the barrel cortex is significantly reduced in isolated pups when compared to pups that are allowed to remain with littermates (Miyazaki et al., 2012; Miyazaki et al., 2013). These synaptic effects are at least in part attributed to the instability of synapses – increased mushroom spine turnover has been observed as a result of social isolation in pups (Takatsuru et al., 2009; Takatsuru et al., 2015). On the other hand, 3-hour daily isolation from P2 to P14 has been shown to increase neuronal activity in the adult barrel cortex and raise baseline glutamate levels, which, in a pattern not observed in control animals, rises even further following exposure to physical stressors (Toya et al., 2014). These deficits persist after limiting peripheral inputs, which suggests that mechanisms distinct from sensory experience are responsible for the circuit changes associated with social isolation.
Social isolation activates glucocorticoid signaling widely across the brain (Mccormick et al., 1998; review: Cacioppo et al., 2015). Rodents that underwent neonatal isolation show long-term increases in basal corticosterone levels in the barrel cortex (Toya et al., 2014). Elevated glucocorticoid levels have been shown to increase glutamate release in frontal cortical areas (Treccani et al., 2014). Although similar mechanisms have not been directly demonstrated in the barrel cortex, high glucocorticoid levels may also contribute to aberrant glutamatergic homeostasis observed in this region. Indeed, repeated brief exposure to maternal separation results in significantly enhanced spine density in L2/3 pyramidal neurons in the somatosensory cortex (Bock et al., 2005). Acute administration of corticosterone induces higher spine turnover rate in the juvenile barrel cortex compared to control juvenile animals and the turnover rate observed in glucocorticoid treated adults (Liston and Gan, 2011). Pharmacologically decreasing endogenous glucocorticoid signaling through repeated dexamethasone injections decreases spine turnover, and chronic steroid administration eliminates dendritic spines formed prior, in earlier developmental periods (Liston and Gan, 2011). These findings suggest that stress hormones, released in response to a wide variety of stressors including social isolation, can highjack mechanisms of synaptic plasticity during development and have long-term social and cognitive behavioral consequences (Moriceau et al., 2009; review: Sullivan and Holman, 2010).
Recent evidence has identified glial regulation of synaptic function as another potential mechanism through which early social isolation can affect sensory circuit development. Early sensory experience alters cortical synaptic pruning, a process mediated by microglia and astrocytes (Kalambogias et al., 2020; Gesuita et al., 2022). Neonatal social isolation leads to increased microglial process motility both at baseline and in response to sensory stimulation (Takatsuru et al., 2015). Additionally, astrocytes contribute to the developmental changes in L4-to-L2/3 synaptic plasticity by regulating the switch from long-term depression (LTD) to long-term potentiation (LTP) (Martinez-Gallego et al., 2022). The precise mechanistic connections between early life stress, glucocorticoid signaling, microglial and/or astrocytic dysfunction, and long-term synaptic instability are still unclear. Further investigations of glial development in the barrel cortex will be informative for understanding experience-dependent maturation of somatosensory circuits.
Perinatal exposure to psychoactive substances has also been shown to alter barrel cortex development. The most studied of these substances is ethanol. In humans, consumption of alcohol during pregnancy can cause Fetal Alcohol Syndrome (FAS), a developmental disorder characterized by intellectual disability, facial anomalies, and behavioral deficits (review: Popova et al., 2023). Preclinical rodent models of FAS show that both prenatal and early postnatal (P4-P10) alcohol exposure decrease the area size of the barrel cortex (Miller and Potempa, 1990; Margret et al., 2005, 2006; Powrozek and Zhou, 2005; Chappell et al., 2007). Prenatal ethanol exposure also decreases the number of glia and neurons in the barrel cortex (Miller and Potempa, 1990; Powrozek and Zhou, 2005). This is likely due to the broad apoptotic effect of alcohol exposure in the neonatal brain (Smiley et al., 2019). Between P4 and P7, when alcohol-induced apoptosis reaches maximum levels, intraperitoneal administration of alcohol suppresses spontaneous activity in the barrel cortex and decreases body movements (Lebedeva et al., 2017). Perinatal alcohol exposure decreases the excitability of L5 pyramidal neurons and the cell density of PV interneurons (Granato et al., 2012; Saito et al., 2019). On a behavioral level, adolescent mice exposed to alcohol prenatally show diminished tactile sensitivity (Delatour et al., 2019). These data suggest that early ethanol exposure disrupts normal barrel cortex development and sensory perception by inhibiting cortical activity and increasing apoptosis, which could lead to impaired circuit maturation and organization.
The impact of other psychoactive substances on somatosensory circuit development is less characterized. Prenatal and postnatal opioid exposure has also been shown to increase apoptosis in cortical neurons and microglia, and methadone may specifically decrease the number of GABAergic synapses (Bajic et al., 2013; Grecco et al., 2022). Postnatal (P2-P7) injection of Δ(9)-tetrahydrocannabinol (THC), the primary psychoactive component of cannabis, results in premature retraction and pruning of TCAs during the first postnatal week (Itami et al., 2016). Nicotine, at concentrations similar to those produced by maternal tobacco smoking, desensitizes nicotinic acetylcholine (ACh) receptors in subplate neurons thus diminishing the ACh-driven spontaneous activity in the barrel cortex during the first postnatal week (Hanganu and Luhmann, 2004; Dupont et al., 2006). Overall, these substances may exert long-term effects through pathways that converge upon the disruption of early cortical activity required for sensory circuit development.
Various types of early life experience—including but not limited to sensory inputs, sleep, social interactions, and substance exposure—exert effects on postnatal circuit organization and contribute to the development of normal or dysfunctional somatosensation. The physiological and environmental factors discussed here will contribute to the understanding of the etiology of atypical sensory perception in many neurodevelopmental and neuropsychiatric disorders, providing considerations on the timing of therapeutic interventions.
IN: Writing−review and editing, Writing−original draft. AC: Writing−review and editing, Writing−original draft.
The authors declare financial support was received for the research, authorship, and/or publication of this article. This research was supported by the grants from NINDS 1R00NS114166 (AC), 1R01NS133434 (AC), NIDA 1R01DA059378 (AC), and State of Connecticut, Department of Mental Health and Addiction Services (AC). This publication does not express the views of the Department of Mental Health and Addiction Services or the State of Connecticut.
The authors declare that the research was conducted in the absence of any commercial or financial relationships that could be construed as a potential conflict of interest.
All claims expressed in this article are solely those of the authors and do not necessarily represent those of their affiliated organizations, or those of the publisher, the editors and the reviewers. Any product that may be evaluated in this article, or claim that may be made by its manufacturer, is not guaranteed or endorsed by the publisher.
Abdel-Majid, R. M., Leong, W. L., Schalkwyk, L. C., Smallman, D. S., Wong, S. T., Storm, D. R., et al. (1998). Loss of adenylyl cyclase I activity disrupts patterning of mouse somatosensory cortex. Nat. Genet. 19, 289–291. doi: 10.1038/980
Akhmetshina, D., Nasretdinov, A., Zakharov, A., Valeeva, G., and Khazipov, R. (2016). The nature of the sensory input to the neonatal rat barrel cortex. J. Neurosci. 36:9922. doi: 10.1523/JNEUROSCI.1781-16.2016
Allen, C. B., Celikel, T., and Feldman, D. E. (2003). Long-term depression induced by sensory deprivation during cortical map plasticity in vivo. Nat. Neurosci. 6, 291–299. doi: 10.1038/NN1012
Anastasiades, P. G., Marques-Smith, A., Lyngholm, D., Lickiss, T., Raffiq, S., Katzel, D., et al. (2016). GABAergic interneurons form transient layer-specific circuits in early postnatal neocortex. Nat. Commun. 7, 1–13. doi: 10.1038/ncomms10584
Antón-Bolaños, N., Sempere-Ferràndez, A., Guillamón-Vivancos, T., Martini, F. J., Pérez-Saiz, L., Gezelius, H., et al. (2019). Prenatal activity from thalamic neurons governs the emergence of functional cortical maps in mice. Science 364, 987–990. doi: 10.1126/science.aav7617
Badde, S., Ley, P., Rajendran, S. S., Shareef, I., Kekunnaya, R., and Röder, B. (2020). Sensory experience during early sensitive periods shapes cross-modal temporal biases. Elife 9:1238. doi: 10.7554/ELIFE.61238
Bajic, D., Commons, K. G., and Soriano, S. G. (2013). Morphine-enhanced apoptosis in selective brain regions of neonatal rats. Int. J. Dev. Neurosci. 31, 258–266. doi: 10.1016/J.IJDEVNEU.2013.02.009
Ballester-Rosado, C. J., Albright, M. J., Wu, C. S., Liao, C. C., Zhu, J., Xu, J., et al. (2010). mGluR5 in cortical excitatory neurons exerts both cell-autonomous and -nonautonomous influences on cortical somatosensory circuit formation. J. Neurosci. 30:16896. doi: 10.1523/JNEUROSCI.2462-10.2010
Banerjee, P., Kubo, F., Nakaoka, H., Ajima, R., Sato, T., Hirata, T., et al. (2022). Spontaneous activity in whisker-innervating region of neonatal mouse trigeminal ganglion. Sci. Rep. 12, 1–17. doi: 10.1038/s41598-022-20068-z
Bates, C. A., and Killackey, H. P. (1985). The organization of the neonatal rat’s brainstem trigeminal complex and its role in the formation of central trigeminal patterns. J. Comp. Neurol. 240, 265–287. doi: 10.1002/CNE.902400305
Belford, G. R., and Killackey, H. P. (1980). The sensitive period in the development of the trigeminal system of the neonatal rat. J. Comp. Neurol. 193, 335–350. doi: 10.1002/cne.901930203
Bender, K. J., Allen, C. B., Bender, V. A., and Feldman, D. E. (2006). Synaptic basis for whisker deprivation-induced synaptic depression in rat somatosensory cortex. J. Neurosci. 26, 4155–4165. doi: 10.1523/jneurosci.0175-06.2006
Blumberg, M. S., Dooley, J. C., and Tiriac, A. (2022). Sleep, plasticity, and sensory neurodevelopment. Neuron 110, 3230–3242. doi: 10.1016/J.NEURON.2022.08.005
Bock, J., Gruss, M., Becker, S., and Braun, K. (2005). Experience-induced changes of dendritic spine densities in the prefrontal and sensory cortex: Correlation with developmental time windows. Cereb. Cortex 15, 802–808. doi: 10.1093/CERCOR/BHH181
Bollmann, Y., Modol, L., Tressard, T., Vorobyev, A., Dard, R., Brustlein, S., et al. (2023). Prominent in vivo influence of single interneurons in the developing barrel cortex. Nat. Neurosci. 26, 1555–1565. doi: 10.1038/S41593-023-01405-5
Bragg-Gonzalo, L., Reyes, N. S. D. L., and Nieto, M. (2021). Genetic and activity dependent-mechanisms wiring the cortex: Two sides of the same coin. Semin. Cell Dev. Biol. 118, 24–34. doi: 10.1016/J.SEMCDB.2021.05.011
Cacioppo, J. T., Cacioppo, S., Capitanio, J. P., and Cole, S. W. (2015). The neuroendocrinology of social isolation. Annu. Rev. Psychol. 66, 733–767.
Cai, L., Argunşah, A. Ö, Damilou, A., and Karayannis, T. (2024). A nasal chemosensation-dependent critical window for somatosensory development. Science 384, 652–660.
Carvell, G. E., and Simons, D. J. (1996). Abnormal tactile experience early in life disrupts active touch. J. Neurosci. 16, 2750–2757. doi: 10.1523/JNEUROSCI.16-08-02750.1996
Chang, E. F., and Merzenich, M. M. (2003). Environmental noise retards auditory cortical development. Science 300, 498–502. doi: 10.1126/SCIENCE.1082163
Chappell, T. D., Margret, C. P., Li, C. X., and Waters, R. S. (2007). LONG TERM EFFECTS OF PRENATAL ALCOHOL EXPOSURE (PAE) ON THE SIZE OF THE WHISKER REPRESENTATION IN JUVENILE AND ADULT RAT BARREL CORTEX. Alcohol 41:239. doi: 10.1016/J.ALCOHOL.2007.03.005
Che, A., Babij, R., Iannone, A. F., Fetcho, R. N., Ferrer, M., Liston, C., et al. (2018). Layer I interneurons sharpen sensory maps during neonatal development. Neuron 99:98–116.e7. doi: 10.1016/J.NEURON.2018.06.002
Chittajallu, R., and Isaac, J. T. R. (2010). Emergence of cortical inhibition by coordinated sensory-driven plasticity at distinct synaptic loci. Nat. Neurosci. 13, 1240–1248. doi: 10.1038/nn.2639
Colonnese, M. T., Kaminska, A., Minlebaev, M., Milh, M., Bloem, B., Lescure, S., et al. (2010). A conserved switch in sensory processing prepares developing neocortex for vision. Neuron 67:480. doi: 10.1016/J.NEURON.2010.07.015
Conhaim, J., Easton, C. R., Becker, M. I., Barahimi, M., Cedarbaum, E. R., Moore, J. G., et al. (2011). Developmental changes in propagation patterns and transmitter dependence of waves of spontaneous activity in the mouse cerebral cortex. J. Physiol. 589, 2529–2541. doi: 10.1113/JPHYSIOL.2010.202382
Daw, M. I., Ashby, M. C., and Isaac, J. T. R. (2007). Coordinated developmental recruitment of latent fast spiking interneurons in layer IV barrel cortex. Nat. Neurosci. 10, 453–461. doi: 10.1038/nn1866
Del Rio-Bermudez, C., and Blumberg, M. S. (2018). Active sleep promotes functional connectivity in developing sensorimotor networks. BioEssays 40:e1700234. doi: 10.1002/BIES.201700234
Delatour, L. C., Yeh, P. W., and Yeh, H. H. (2019). Ethanol exposurein uterodisrupts radial migration and pyramidal cell development in the somatosensory cortex. Cereb. Cortex 29, 2125–2139. doi: 10.1093/cercor/bhy094
Diamond, M. E., Heimendahl, M. V., Knutsen, P. M., Kleinfeld, D., and Ahissar, E. (2008). “Where” and “what” in the whisker sensorimotor system. Nat. Rev. Neurosci. 9, 601–612. doi: 10.1038/nrn2411
Dooley, J. C., Glanz, R. M., Sokoloff, G., and Blumberg, M. S. (2020). Self-generated whisker movements drive state-dependent sensory input to developing barrel cortex. Curr. Biol. 30:2404–2410.e4. doi: 10.1016/J.CUB.2020.04.045
Driver, J., and Noesselt, T. (2008). Multisensory interplay reveals crossmodal influences on “sensory-specific” brain regions, neural responses, and judgments. Neuron 57, 11–23. doi: 10.1016/J.NEURON.2007.12.013
Duan, Z. R. S., Che, A., Chu, P., Modol, L., Bollmann, Y., Babij, R., et al. (2020). GABAergic restriction of network dynamics regulates interneuron survival in the developing cortex. Neuron 105:75–92.e5. doi: 10.1016/J.NEURON.2019.10.008
Dupont, E., Hanganu, I. L., Kilb, W., Hirsch, S., and Luhmann, H. J. (2006). Rapid developmental switch in the mechanisms driving early cortical columnar networks. Nature 439, 79–83. doi: 10.1038/NATURE04264
Erzurumlu, R. S., and Gaspar, P. (2012). Development and critical period plasticity of the barrel cortex. Eur. J. Neurosci. 35, 1540–1553. doi: 10.1111/J.1460-9568.2012.08075.X
Faust, T. E., Gunner, G., and Schafer, D. P. (2021). Mechanisms governing activity-dependent synaptic pruning in the mammalian CNS. Nat. Rev. Neurosci. 22:657. doi: 10.1038/S41583-021-00507-Y
Felitti, V. J., Anda, R. F., Nordenberg, D., Williamson, D. F., Spitz, A. M., Edwards, V., et al. (1998). Relationship of childhood abuse and household dysfunction to many of the leading causes of death in adults: The adverse childhood experiences (ACE) study. Am. J. Prev. Med. 14, 245–258. doi: 10.1016/S0749-3797(98)00017-8
Finnerty, G. T., Roberts, L. S. E., and Connors, B. W. (1999). Sensory experience modifies the short-term dynamics of neocortical synapses. Nature 400, 367–371. doi: 10.1038/22553
Fox, K. (1992). A critical period for experience-dependent synaptic plasticity in rat barrel cortex. J. Neurosci. 12:1826. doi: 10.1523/JNEUROSCI.12-05-01826.1992
Fox, S. E., Levitt, P., and Nelson, C. A. (2010). How the timing and quality of early experiences influence the development of brain architecture. Child Dev. 81, 28–40. doi: 10.1111/J.1467-8624.2009.01380.X
Gainey, M. A., Wolfe, R., Pourzia, O., and Feldman, D. E. (2016). Whisker deprivation drives two phases of inhibitory synapse weakening in layer 4 of rat somatosensory cortex. PLoS One 11:e0148227. doi: 10.1371/JOURNAL.PONE.0148227
Gesuita, L., Cavaccini, A., Argunsah, A. Ö, Favuzzi, E., Ibrahim, L. A., Stachniak, T. J., et al. (2022). Microglia contribute to the postnatal development of cortical somatostatin-positive inhibitory cells and to whisker-evoked cortical activity. Cell Rep. 40:209. doi: 10.1016/J.CELREP.2022.111209
Golshani, P., Gonçalves, J. T., Khoshkhoo, S., Mostany, R., Smirnakis, S., and Portera-Cailliau, C. (2009). Internally mediated developmental desynchronization of neocortical network activity. J. Neurosci. 29:10890. doi: 10.1523/JNEUROSCI.2012-09.2009
Granato, A., Palmer, L. M., Giorgio, A., de, Tavian, D., and Larkum, M. E. (2012). Early exposure to alcohol leads to permanent impairment of dendritic excitability in neocortical pyramidal neurons. J. Neurosci. 32, 1377–1382. doi: 10.1523/JNEUROSCI.5520-11.2012
Grecco, G. G., Huang, J. Y., Muñoz, B., Doud, E. H., Hines, C. D., Gao, Y., et al. (2022). Sex-dependent synaptic remodeling of the somatosensory cortex in mice with prenatal methadone exposure. Adv. Drug Alcohol Res. 2:10400. doi: 10.3389/ADAR.2022.10400
Hanganu, I. L., and Luhmann, H. J. (2004). Functional nicotinic acetylcholine receptors on subplate neurons in neonatal rat somatosensory cortex. J. Neurophysiol. 92, 189–198. doi: 10.1152/JN.00010.2004
Hensch, T. K. (2004). CRITICAL PERIOD REGULATION. Annu. Rev. Neurosci. 27, 549–579. doi: 10.1146/annurev.neuro.27.070203.144327
Henschke, J. U., Oelschlegel, A. M., Angenstein, F., Ohl, F. W., Goldschmidt, J., Kanold, P. O., et al. (2018). Early sensory experience influences the development of multisensory thalamocortical and intracortical connections of primary sensory cortices. Brain Struct. Funct. 223:1165. doi: 10.1007/S00429-017-1549-1
Higashi, S., Crair, M. C., Kurotani, T., Inokawa, H., and Toyama, K. (1999). Altered spatial patterns of functional thalamocortical connections in the barrel cortex after neonatal infraorbital nerve cut revealed by optical recording. Neuroscience 91, 439–452. doi: 10.1016/S0306-4522(98)00666-6
Hughes, K., Bellis, M. A., Hardcastle, K. A., Sethi, D., Butchart, A., Mikton, C., et al. (2017). The effect of multiple adverse childhood experiences on health: A systematic review and meta-analysis. Lancet Public Health 2, e356–e366. doi: 10.1016/S2468-2667(17)30118-4
Inan, M., Lu, H. C., Albright, M. J., She, W. C., and Crair, M. C. (2006). Barrel map development relies on protein kinase A regulatory subunit IIβ-mediated cAMP signaling. J. Neurosci. 26, 4338–4349. doi: 10.1523/JNEUROSCI.3745-05.2006
Itami, C., Huang, J. Y., Yamasaki, M., Watanabe, M., Lu, H. C., and Kimura, F. (2016). Developmental switch in spike timing-dependent plasticity and cannabinoid-dependent reorganization of the thalamocortical projection in the barrel cortex. J. Neurosci. 36, 7039–7054. doi: 10.1523/JNEUROSCI.4280-15.2016
Iwasato, T., Datwani, A., Wolf, A. M., Nishiyama, H., Taguchi, Y., Tonegawa, S., et al. (2000). Cortex-restricted disruption of NMDAR1 impairs neuronal patterns in the barrel cortex. Nature 406, 726–731. doi: 10.1038/35021059
Jeanmonod, D., Rice, F. L., Loos, H. V., and der. (1981). Mouse somatosensory cortex: Alterations in the barrelfield following receptor injury at different early postnatal ages. Neuroscience 6, 1503–1535. doi: 10.1016/0306-4522(81)90222-0
Jensen, K. F., and Killackey, H. P. (1987). Terminal arbors of axons projecting to the somatosensory cortex of the adult rat. I. The normal morphology of specific thalamocortical afferents. J. Neurosci. 7, 3529–3543. doi: 10.1523/JNEUROSCI.07-11-03529.1987
Jones, C. E., Opel, R. A., Kaiser, M. E., Chau, A. Q., Quintana, J. R., Nipper, M. A., et al. (2019). Early-life sleep disruption increases parvalbumin in primary somatosensory cortex and impairs social bonding in prairie voles. Sci. Adv. 5:5188. doi: 10.1126/SCIADV.AAV5188
Jouvet-Mounier, D., Astic, L., and Lacote, D. (1969). Ontogenesis of the states of sleep in rat, cat, and guinea pig during the first postnatal month. Dev. Psychobiol. 2, 216–239. doi: 10.1002/DEV.420020407
Kalambogias, J., Chen, C. C., Khan, S., Son, T., Wercberger, R., Headlam, C., et al. (2020). Development and sensory experience dependent regulation of microglia in barrel cortex. J. Comp. Neurol. 528, 559–573. doi: 10.1002/CNE.24771
Kastli, R., Vighagen, R., van der Bourg, A., Argunsah, A., Iqbal, A., Voigt, F. F., et al. (2020). Developmental divergence of sensory stimulus representation in cortical interneurons. Nat. Commun. 11:5729. doi: 10.1038/S41467-020-19427-Z
Khazipov, R., Sirota, A., Leinekugel, X., Holmes, G. L., Ben-Ari, Y., and Buzsáki, G. (2004). Early motor activity drives spindle bursts in the developing somatosensory cortex. Nature 432, 758–761. doi: 10.1038/NATURE03132
Killackey, H. P., and Belford, G. R. (1979). The formation of afferent patterns in the somatosensory cortex of the neonatal rat. J. Comp. Neurol. 183, 285–303. doi: 10.1002/CNE.901830206
Lebedeva, J., Zakharov, A., Ogievetsky, E., Minlebaeva, A., Kurbanov, R., Gerasimova, E., et al. (2017). Inhibition of cortical activity and apoptosis caused by ethanol in neonatal rats in vivo. Cereb. Cortex 27, 1068–1082. doi: 10.1093/CERCOR/BHV293
Lee, L. J., Chen, W. J., Chuang, Y. W., and Wang, Y. C. (2009). Neonatal whisker trimming causes long-lasting changes in structure and function of the somatosensory system. Exp. Neurol. 219, 524–532. doi: 10.1016/J.EXPNEUROL.2009.07.012
Lee, S. H., Land, P. W., and Simons, D. J. (2007). Layer- and cell-type-specific effects of neonatal whisker-trimming in adult rat barrel cortex. J. Neurophysiol. 97, 4380–4385. doi: 10.1152/JN.01217.2006
Leighton, A. H., and Lohmann, C. (2016). The wiring of developing sensory circuits–From patterned spontaneous activity to synaptic plasticity mechanisms. Front. Neural Circuits 10:214646. doi: 10.3389/FNCIR.2016.00071
Lendvai, B., Stern, E. A., Chen, B., and Svoboda, K. (2000). Experience-dependent plasticity of dendritic spines in the developing rat barrel cortex in vivo. Nature 404, 876–881. doi: 10.1038/35009107
Li, H., Fertuzinhos, S., Mohns, E., Hnasko, T. S., Verhage, M., Edwards, R., et al. (2013). Laminar and columnar development of barrel cortex relies on thalamocortical neurotransmission. Neuron 79, 970–986. doi: 10.1016/J.NEURON.2013.06.043
Li, W., Ma, L., Yang, G., and Gan, W. B. (2017). REM sleep selectively prunes and maintains new synapses in development and learning. Nat. Neurosci. 20, 427–437. doi: 10.1038/nn.4479
Liston, C., and Gan, W. B. (2011). Glucocorticoids are critical regulators of dendritic spine development and plasticity in vivo. Proc. Natl. Acad. Sci. U.S.A. 108, 16074–16079.
Lokhandwala, S., and Spencer, R. M. C. (2022). Relations between sleep patterns early in life and brain development: A review. Dev. Cogn. Neurosci. 56:101130. doi: 10.1016/J.DCN.2022.101130
Maitre, N. L., Key, A. P., Chorna, O. D., Slaughter, J. C., Matusz, P. J., Wallace, M. T., et al. (2017). The dual nature of early-life experience on somatosensory processing in the human infant brain. Curr. Biol. 27, 1048–1054. doi: 10.1016/J.CUB.2017.02.036
Makarov, R., Sintsov, M., Valeeva, G., Starikov, P., Negrov, D., and Khazipov, R. (2021). Bone conducted responses in the neonatal rat auditory cortex. Sci. Rep. 11, 1–16. doi: 10.1038/s41598-021-96188-9
Margret, C. P., Li, C. X., Chappell, T. D., Elberger, A. J., Matta, S. G., and Waters, R. S. (2006). Prenatal alcohol exposure delays the development of the cortical barrel field in neonatal rats. Exp. Brain Res. 172, 1–13. doi: 10.1007/s00221-005-0319-0
Margret, C. P., Li, C. X., Elberger, A. J., Matta, S. G., Chappell, T. D., and Waters, R. S. (2005). Prenatal alcohol exposure alters the size, but not the pattern, of the whisker representation in neonatal rat barrel cortex. Exp. Brain Res. 165, 167–178.
Marques-Smith, A., Lyngholm, D., Kaufmann, A. K., Stacey, J. A., Hoerder-Suabedissen, A., Becker, E. B. E., et al. (2016). A transient translaminar gabaergic interneuron circuit connects thalamocortical recipient layers in neonatal somatosensory cortex. Neuron 89, 536–549. doi: 10.1016/J.NEURON.2016.01.015
Martinez-Gallego, I., Perez-Rodriguez, M., Coatl-Cuaya, H., Flores, G., and Rodriguez-Moreno, A. (2022). Adenosine and astrocytes determine the developmental dynamics of spike timing-dependent plasticity in the somatosensory cortex. J. Neurosci. 42:6038. doi: 10.1523/JNEUROSCI.0115-22.2022
Matsui, A., Tran, M., Yoshida, A. C., Kikuchi, S. S., Mami, U., Ogawa, M., et al. (2013). BTBD3 controls dendrite orientation toward active axons in mammalian neocortex. Science 342, 1114–1118.
Mccormick, C. M., Kehoe, P., and Kovacs, S. (1998). Corticosterone release in response to repeated, short episodes of neonatal isolation: Evidence of sensitization. Int. J. Dev. Neurosci. 16, 175–185. doi: 10.1016/S0736-5748(98)00026-4
Micheva, K. D., and Beaulieu, C. (1995a). Neonatal sensory deprivation induces selective changes in the quantitative distribution of GABA-immunoreactive neurons in the rat barrel field cortex. J. Comp. Neurol. 361, 574–584. doi: 10.1002/cne.903610403
Micheva, K. D., and Beaulieu, C. (1995b). Postnatal development of GABA neurons in the rat somatosensory barrel cortex: A quantitative study. Eur. J. Neurosci. 7, 419–430. doi: 10.1111/J.1460-9568.1995.TB00338.X
Micheva, K. D., and Beaulieu, C. (1997). Development and plasticity of the inhibitory neocortical circuitry with an emphasis on the rodent barrel field cortex: a review. Can. J. Physiol. Pharmacol. 75, 380.
Miller, M. W., and Potempa, G. (1990). Numbers of neurons and glia in mature rat somatosensory cortex: Effects of prenatal exposure to ethanol. J. Comp. Neurol. 293, 92–102. doi: 10.1002/CNE.902930108
Minlebaev, M., Ben-Ari, Y., and Khazipov, R. (2007). Network mechanisms of spindle-burst oscillations in the neonatal rat barrel cortex in vivo. J. Neurophysiol. 97, 692–700. doi: 10.1152/JN.00759.2006
Miyazaki, T., Kunii, M., Jitsuki, S., Sano, A., Kuroiwa, Y., and Takahashi, T. (2013). Social isolation perturbs experience-driven synaptic glutamate receptor subunit 4 delivery in the developing rat barrel cortex. Eur. J. Neurosci. 37, 1602–1609. doi: 10.1111/EJN.12188
Miyazaki, T., Kunii, M., Tada, H., Sano, A., Kuroiwa, Y., Goto, T., et al. (2012). Developmental AMPA receptor subunit specificity during experience-driven synaptic plasticity in the rat barrel cortex. Brain Res. 1435, 1–7. doi: 10.1016/J.BRAINRES.2011.11.033
Mizuno, H., Ikezoe, K., Nakazawa, S., Sato, T., Kitamura, K., and Iwasato, T. (2018). Patchwork-type spontaneous activity in neonatal barrel cortex layer 4 transmitted via thalamocortical projections. Cell Rep. 22, 123–135. doi: 10.1016/J.CELREP.2017.12.012
Modol, L., Bollmann, Y., Tressard, T., Baude, A., Che, A., Duan, Z. R. S., et al. (2020). Assemblies of Perisomatic GABAergic neurons in the developing barrel cortex. Neuron 105:93–105.e4. doi: 10.1016/J.NEURON.2019.10.007
Moreno-Juan, V., Filipchuk, A., Antón-Bolaños, N., Mezzera, C., Gezelius, H., Andrés, B., et al. (2017). Prenatal thalamic waves regulate cortical area size prior to sensory processing. Nat. Commun. 8, 1–14. doi: 10.1038/ncomms14172
Moriceau, S., Shionoya, K., Jakubs, K., and Sullivan, R. M. (2009). Early-life stress disrupts attachment learning: The role of amygdala corticosterone, locus ceruleus corticotropin releasing hormone, and olfactory bulb norepinephrine. J. Neurosci. 29, 15745–15755. doi: 10.1523/JNEUROSCI.4106-09.2009
Nakazawa, S., Mizuno, H., and Iwasato, T. (2018). Differential dynamics of cortical neuron dendritic trees revealed by long-term in vivo imaging in neonates. Nat. Commun. 9:3106. doi: 10.1038/S41467-018-05563-0
Nakazawa, S., Yoshimura, Y., Takagi, M., Mizuno, H., and Iwasato, T. (2020). Developmental phase transitions in spatial organization of spontaneous activity in postnatal barrel cortex layer 4. J. Neurosci. 40, 7637–7650. doi: 10.1523/JNEUROSCI.1116-20.2020
Narboux-Nême, N., Evrard, A., Ferezou, I., Erzurumlu, R. S., Kaeser, P. S., Lainé, J., et al. (2012). Neurotransmitter release at the thalamocortical synapse instructs barrel formation but not axon patterning in the somatosensory cortex. J. Neurosci. 32:6183. doi: 10.1523/JNEUROSCI.0343-12.2012
Papaioannou, S., Brigham, L., and Krieger, P. (2013). Sensory deprivation during early development causes an increased exploratory behavior in a whisker-dependent decision task. Brain Behav. 3, 24–34. doi: 10.1002/BRB3.102
Petersen, C. C. H. (2007). The functional organization of the barrel cortex. Neuron 56, 339–355. doi: 10.1016/J.NEURON.2007.09.017
Popova, S., Charness, M. E., Burd, L., Crawford, A., Hoyme, H. E., Mukherjee, R. A. S., et al. (2023). Fetal alcohol spectrum disorders. Nat. Rev. Dis. Prim. 9:11. doi: 10.1038/S41572-023-00420-X
Pouchelon, G., Dwivedi, D., Bollmann, Y., Agba, C. K., Xu, Q., Mirow, A. M. C., et al. (2021). The organization and development of cortical interneuron presynaptic circuits are area specific. Cell Rep. 37:993. doi: 10.1016/J.CELREP.2021.109993
Pouchelon, G., Gambino, F., Bellone, C., Telley, L., Vitali, I., Lüscher, C., et al. (2014). Modality-specific thalamocortical inputs instruct the identity of postsynaptic L4 neurons. Nature 511, 471–474. doi: 10.1038/nature13390
Powrozek, T. A., and Zhou, F. C. (2005). Effects of prenatal alcohol exposure on the development of the vibrissal somatosensory cortical barrel network. Brain Res. Dev. Brain Res. 155, 135–146. doi: 10.1016/J.DEVBRAINRES.2005.01.003
Rio-Bermudez, C. D., Kim, J., Sokoloff, G., and Blumberg, M. S. (2020). Active sleep promotes coherent oscillatory activity in the Cortico-hippocampal system of infant rats. Cereb. Cortex 30, 2070–2082. doi: 10.1093/cercor/bhz223
Sadaka, Y., Weinfeld, E., Lev, D. L., and White, E. L. (2003). Changes in mouse barrel synapses consequent to sensory deprivation from birth. J. Comp. Neurol. 457, 75–86. doi: 10.1002/CNE.10518
Saito, M., Smiley, J. F., Hui, M., Masiello, K., and Betz, J. (2019). Neonatal ethanol disturbs the normal maturation of parvalbumin interneurons surrounded by subsets of perineuronal nets in the cerebral cortex: Partial reversal by lithium. Cereb. Cortex 29, 1383–1397. doi: 10.1093/CERCOR/BHY034
Schierloh, A., Eder, M., Zieglgänsberger, W., and Dodt, H. U. (2004). Effects of sensory deprivation on columnar organization of neuronal circuits in the rat barrel cortex. Eur. J. Neurosci. 20, 1118–1124. doi: 10.1111/J.1460-9568.2004.03557.X
Shepherd, G. M. G., Pologruto, T. A., and Svoboda, K. (2003). Circuit analysis of experience-dependent plasticity in the developing rat barrel cortex. Neuron 38, 277–289. doi: 10.1016/S0896-6273(03)00152-1
Shishelova, A. Y. (2006). Effect of whisker removal on defensive behavior in rats during early ontogenesis. Neurosci. Behav. Physiol. 36, 883–888. doi: 10.1007/S11055-006-0102-0
Shoykhet, M., Land, P. W., and Simons, D. J. (2005). Whisker trimming begun at birth or on postnatal day 12 affects excitatory and inhibitory receptive fields of layer IV barrel neurons. J. Neurophysiol. 94, 3987–3995.
Simons, D. J., Carvell, G. E., and Kyriazi, H. T. (2015). Alterations in functional thalamocortical connectivity following neonatal whisker trimming with adult regrowth. J. Neurophysiol. 114, 1912–1922. doi: 10.1152/jn.00488.2015
Simons, D. J., and Land, P. W. (1987). Early experience of tactile stimulation influences organization of somatic sensory cortex. Nature 326, 694–697. doi: 10.1038/326694a0
Smiley, J. F., Bleiwas, C., Masiello, K., Petkova, E., Betz, J., Hui, M., et al. (2019). Effects of neonatal ethanol on cerebral cortex development through adolescence. Brain Struct. Funct. 224, 1871–1884.
Smirnov, K., and Sitnikova, E. (2019). Developmental milestones and behavior of infant rats: The role of sensory input from whiskers. Behav. Brain Res. 374:112143. doi: 10.1016/J.BBR.2019.112143
Soumiya, H., Godai, A., Araiso, H., Mori, S., Furukawa, S., and Fukumitsu, H. (2016). Neonatal whisker trimming impairs fear/anxiety-related emotional systems of the amygdala and social behaviors in adult mice. PLoS One 11:e0158583. doi: 10.1371/JOURNAL.PONE.0158583
Stern, E. A., Maravall, M., and Svoboda, K. (2001). Rapid development and plasticity of layer 2/3 maps in rat barrel cortex in vivo. Neuron 31, 305–315. doi: 10.1016/S0896-6273(01)00360-9
Sullivan, R. M., and Holman, P. J. (2010). Transitions in sensitive period attachment learning in infancy: The role of corticosterone. Neurosci. Biobehav. Rev. 34, 835–844. doi: 10.1016/J.NEUBIOREV.2009.11.010
Sun, L., Zhou, H., Cichon, J., and Yang, G. (2020). Experience and sleep-dependent synaptic plasticity: From structure to activity. Philos. Trans. R. Soc. B Biol. Sci. 375:234. doi: 10.1098/RSTB.2019.0234
Suzuki, A., Lee, L. J., Hayashi, Y., Muglia, L., Itohara, S., Erzurumlu, R. S., et al. (2015). THALAMIC ADENYLYL CYCLASE 1 IS REQUIRED FOR BARREL FORMATION IN THE SOMATOSENSORY CORTEX. Neuroscience 290:518. doi: 10.1016/J.NEUROSCIENCE.2015.01.043
Takatsuru, Y., Nabekura, J., Ishikawa, T., Kohsaka, S., and Koibuchi, N. (2015). Early-life stress increases the motility of microglia in adulthood. J. Physiol. Sci. 65, 187–194. doi: 10.1007/s12576-015-0361-z
Takatsuru, Y., Yoshitomo, M., Nemoto, T., Eto, K., and Nabekura, J. (2009). Maternal separation decreases the stability of mushroom spines in adult mice somatosensory cortex. Brain Res. 1294, 45–51. doi: 10.1016/J.BRAINRES.2009.07.092
Tiriac, A., Rio-Bermudez, C. D., and Blumberg, M. S. (2014). Self-generated movements with “unexpected” sensory consequences. Curr. Biol. 24, 2136–2141. doi: 10.1016/J.CUB.2014.07.053
Tolner, E. A., Sheikh, A., Yukin, A. Y., Kaila, K., and Kanold, P. O. (2012). Subplate neurons promote spindle bursts and thalamocortical patterning in the neonatal rat somatosensory cortex. J. Neurosci. 32, 692–702. doi: 10.1523/JNEUROSCI.1538-11.2012
Toya, S., Takatsuru, Y., Kokubo, M., Amano, I., Shimokawa, N., and Koibuchi, N. (2014). Early-life-stress affects the homeostasis of glutamatergic synapses. Eur. J. Neurosci. 40, 3627–3634. doi: 10.1111/EJN.12728
Treccani, G., Musazzi, L., Perego, C., Milanese, M., Nava, N., Bonifacino, T., et al. (2014). Stress and corticosterone increase the readily releasable pool of glutamate vesicles in synaptic terminals of prefrontal and frontal cortex. Mol. Psychiatry 19, 433–443. doi: 10.1038/MP.2014.5
Tuncdemir, S. N., Wamsley, B., Stam, F. J., Osakada, F., Goulding, M., Callaway, E. M., et al. (2016). Early somatostatin interneuron connectivity mediates the maturation of deep layer cortical circuits. Neuron 89, 521–535. doi: 10.1016/J.NEURON.2015.11.020
Van der Loos, H., and Woolsey, T. A. (1973). Somatosensory cortex: Structural alterations following early injury to sense organs. Science 179, 395–398. doi: 10.1126/SCIENCE.179.4071.395
Wang, D. C., Santos-Valencia, F., Song, J. H., Franks, K. M., and Luo, L. (2024). Embryonically active piriform cortex neurons promote intracortical recurrent connectivity during development. bioRxiv [Preprint]. doi: 10.1101/2024.05.08.593265
Wang, J., Shan, W., Chen, X., and Zuo, Z. (2022). Whisker trimming induces anti-anxiety like status via activation of dorsomedial hypothalamus nucleus in mice. Brain Res. 1789:147946. doi: 10.1016/J.BRAINRES.2022.147946
Winnubst, J., Cheyne, J. E., Niculescu, D., and Lohmann, C. (2015). Spontaneous activity drives local synaptic plasticity in vivo. Neuron 87, 399–410. doi: 10.1016/J.NEURON.2015.06.029
Wong, F. K., Bercsenyi, K., Sreenivasan, V., Portalés, A., Fernández-Otero, M., and Marín, O. (2018). Pyramidal cell regulation of interneuron survival sculpts cortical networks. Nature 557, 668–673. doi: 10.1038/S41586-018-0139-6
Wong-Riley, M. T. T., and Welt, C. (1980). Histochemical changes in cytochrome oxidase of cortical barrels after vibrissal removal in neonatal and adult mice. Proc. Natl. Acad. Sci. U.S.A. 77, 2333–2337. doi: 10.1073/PNAS.77.4.2333
Woolsey, T. A., and van der Loos, H. (1970). The structural organization of layer IV in the somatosensory region (S I) of mouse cerebral cortex: The description of a cortical field composed of discrete cytoarchitectonic units. Brain Res. 17, 205–242. doi: 10.1016/0006-8993(70)90079-X
Woolsey, T. A., and Wann, J. R. (1976). Areal changes in mouse cortical barrels following vibrissal damage at different postnatal ages. J. Comp. Neurol. 170, 53–66. doi: 10.1002/CNE.901700105
Yang, G., and Gan, W. B. (2012). Sleep contributes to dendritic spine formation and elimination in the developing mouse somatosensory cortex. Dev. Neurobiol. 72, 1391–1398. doi: 10.1002/DNEU.20996
Yang, J. W., An, S., Sun, J. J., Reyes-Puerta, V., Kindler, J., Berger, T., et al. (2013). Thalamic network oscillations synchronize ontogenetic columns in the newborn rat barrel cortex. Cereb. Cortex 23, 1299–1316. doi: 10.1093/CERCOR/BHS103
Yang, J. W., Hanganu-Opatz, I. L., Sun, J. J., and Luhmann, H. J. (2009). Three patterns of oscillatory activity differentially synchronize developing neocortical networks in vivo. J. Neurosci. 29:9011. doi: 10.1523/JNEUROSCI.5646-08.2009
Young, T. R., Yamamoto, M., Kikuchi, S. S., Yoshida, A. C., Abe, T., Inoue, K., et al. (2023). Thalamocortical control of cell-type specificity drives circuits for processing whisker-related information in mouse barrel cortex. Nat. Commun. 14, 1–20. doi: 10.1038/s41467-023-41749-x
Zheng, J. J., Li, S. J., Zhang, X. D., Miao, W. Y., Zhang, D., Yao, H., et al. (2014). Oxytocin mediates early experience-dependent cross-modal plasticity in the sensory cortices. Nat. Neurosci. 17, 391–399. doi: 10.1038/NN.3634
Keywords: somatosensory perception, barrel cortex development, early life experience, sensory deprivation, REM Sleep, early social isolation, cross-modal sensory experience
Citation: Nwabudike I and Che A (2024) Early-life maturation of the somatosensory cortex: sensory experience and beyond. Front. Neural Circuits 18:1430783. doi: 10.3389/fncir.2024.1430783
Received: 10 May 2024; Accepted: 20 June 2024;
Published: 08 July 2024.
Edited by:
Kensaku Mori, RIKEN, JapanReviewed by:
Takuji Iwasato, National Institute of Genetics, JapanCopyright © 2024 Nwabudike and Che. This is an open-access article distributed under the terms of the Creative Commons Attribution License (CC BY). The use, distribution or reproduction in other forums is permitted, provided the original author(s) and the copyright owner(s) are credited and that the original publication in this journal is cited, in accordance with accepted academic practice. No use, distribution or reproduction is permitted which does not comply with these terms.
*Correspondence: Ijeoma Nwabudike, aWplb21hLm53YWJ1ZGlrZUB5YWxlLmVkdQ==; Alicia Che, YWxpY2lhLmNoZUB5YWxlLmVkdQ==
Disclaimer: All claims expressed in this article are solely those of the authors and do not necessarily represent those of their affiliated organizations, or those of the publisher, the editors and the reviewers. Any product that may be evaluated in this article or claim that may be made by its manufacturer is not guaranteed or endorsed by the publisher.
Research integrity at Frontiers
Learn more about the work of our research integrity team to safeguard the quality of each article we publish.