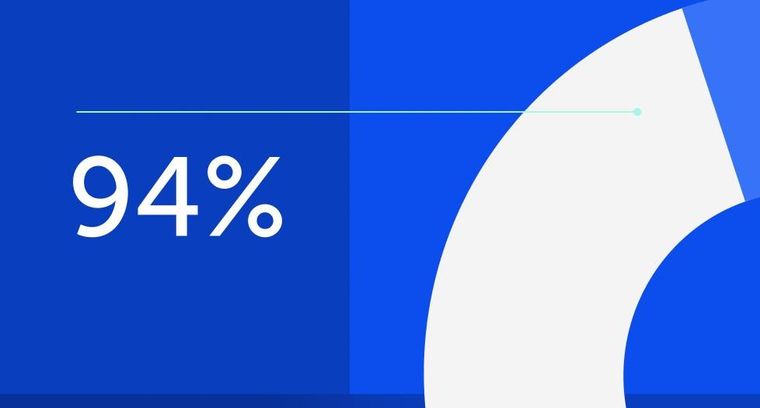
94% of researchers rate our articles as excellent or good
Learn more about the work of our research integrity team to safeguard the quality of each article we publish.
Find out more
REVIEW article
Front. Neural Circuits, 24 March 2023
Volume 17 - 2023 | https://doi.org/10.3389/fncir.2023.1113023
This article is part of the Research TopicHorizons in Neural Circuits, Volume IIView all 7 articles
Major depressive disorder (MDD) is a chronic and disabling disorder affecting roughly 280 million people worldwide. While multiple brain areas have been implicated, dysfunction of prefrontal cortex (PFC) circuitry has been consistently documented in MDD, as well as in animal models for stress-induced depression-like behavioral states. During brain development, axonal guidance cues organize neuronal wiring by directing axonal pathfinding and arborization, dendritic growth, and synapse formation. Guidance cue systems continue to be expressed in the adult brain and are emerging as important mediators of synaptic plasticity and fine-tuning of mature neural networks. Dysregulation or interference of guidance cues has been linked to depression-like behavioral abnormalities in rodents and MDD in humans. In this review, we focus on the emerging role of guidance cues in stress-induced changes in adult prefrontal cortex circuitry and in precipitating depression-like behaviors. We discuss how modulating axonal guidance cue systems could be a novel approach for precision medicine and the treatment of depression.
Major depressive disorder (MDD), a multifactorial disorder with heterogeneous symptomatology, is one of the leading causes of disease and disability worldwide, affecting roughly 280 million people, and manifesting an estimated lifetime prevalence of approximately 17% (Kessler et al., 2003; James et al., 2018; Johnston et al., 2019; Gold et al., 2020; World Health Organization, 2021). Despite decades of research, about 50% of depressed patients are not adequately covered by existing interventions (Akil et al., 2018), and about 30–50% of the patients who receive treatment eventually relapse (de Zwart et al., 2018). Decades of clinical and preclinical studies consistently report that depression is a circuit-level disorder, often induced by chronic stress (Pizzagalli, 2014; Heshmati and Russo, 2015; Bagot et al., 2016; Fischer et al., 2016; Belleau et al., 2018; Hare and Duman, 2020; Spellman and Liston, 2020). Chronic stress disrupts the organization of the prefrontal cortex (PFC) and its connections with cortical, subcortical, and limbic areas–a phenomenon associated with increased susceptibility to depression in humans and to depression-like behavioral abnormalities in rodents (Liston et al., 2009; McEwen and Morrison, 2013; Liu et al., 2017; Hare and Duman, 2020). At the cellular level, chronic stress induces circuitry alterations by impairing signaling essential for the maintenance and formation of neuronal connections, as well as synaptic transmission and plasticity (Duman and Aghajanian, 2012; Chaudhury et al., 2015; Duman et al., 2016; Yan and Rein, 2022). Guidance cues known to be involved in the organization and plasticity of synaptic networks are pre-eminently important in these cellular processes and may act as molecular links between stress and PFC circuitry remodeling (Dickson, 2002; Lanoue and Cooper, 2019).
Axonal guidance cues, and their corresponding receptors, are highly conserved families of proteins that steer growing neurites (e.g., axons, dendrites) to their intended targets, dictating the fine organization of neuronal circuits (Dickson, 2002; Guan and Rao, 2003; Stoeckli, 2018; Lanoue and Cooper, 2019). These cues include classical guidance molecules such as the netrin, ephrin, slit, and semaphorin families, and non-conventional molecules such as morphogens including sonic hedgehog (SHH), bone morphogenetic proteins (BMPs), and the wingless-type family (WNTs). In general, however, not exclusively, axonal guidance cues are secreted, diffusible proteins that are highly expressed during early brain development, both in embryonic and early post-natal life, when they guide long-distance axonal pathfinding required for proper brain wiring and maturation (Dickson, 2002; Guan and Rao, 2003; Stoeckli, 2018). Secreted guidance cues can also bind to the extracellular matrix and function as local haptotactic adhesive signals through one of two main mechanisms: affinity to a component of the extracellular matrix (e.g., Netrin-1 binds to heparan sulfate proteoglycans) (Kennedy et al., 1994; Matsumoto et al., 2007); and surface adhesion to a membrane bound molecule (e.g., Slit-2 adsorbed to GPI-anchored heparan sulfate proteoglycan glypican-1) (Lau and Margolis, 2010; Dominici et al., 2017; Varadarajan et al., 2017; Boyer and Gupton, 2018; Meijers et al., 2019). Expression of axonal guidance cues is at its maximum during embryonic and fetal development, when the forming brain is involved in intensive long-distance wiring. This high expression decreases in post-natal life, with additional evidence from mice of changes between adolescence and adulthood in a number of guidance cue pathways (netrin, ephrin, semaphorin) (Goldman et al., 2013), as the matured brain attenuates large-scale rewiring, but they continue to play a critical role in the organization of local circuits by influencing dendritic structure and synaptic plasticity (Stoeckli, 2018; Lanoue and Cooper, 2019; Glasgow et al., 2020; Torres-Berrío et al., 2020a). Many guidance cues diffuse out from their site of production, often establishing a concentration gradient, and interacting with their specific receptors on the growth cone of the growing neurite. Interaction with specific receptors at this site can induce attraction and neurite growth or can trigger repulsion and neurite collapse, depending on the particular ligand: receptor pair (Dent et al., 2011; McCormick and Gupton, 2020).
Recent studies in rodents have demonstrated that exposure to chronic stress leads to dysregulation of axonal guidance cue expression, which leads to depression-like behavioral states (Torres-Berrío et al., 2020a; Vosberg et al., 2020; Van der Zee et al., 2022). We propose that chronic stress-induced changes in axonal guidance cue function trigger the reorganization of local PFC circuitry increasing incidence of behavioral abnormalities in rodents and the onset and severity of depression in humans. In this review, we discuss axonal guidance cue systems as important mediators of persistent effects of stress exposure in adulthood on PFC neuronal connectivity and behavior, with special consideration given to sex-dependent divergent results, to the extent to which they have been investigated. Within this framework, our focus will mainly be the classical guidance cue families, Netrins, Slits, Ephrins, and Semaphorins, with particular consideration given to Netrin-1, arguably the most studied guidance cue, as well as touching briefly on unconventional guidance cues (morphogens, adhesion proteins). We gather emerging evidence from rodent models for adult chronic stress and from studies in humans indicating altered expression of guidance cues in rodents susceptible to stress and in individuals with MDD, when pertinent, touching on sex differences and their possible protective or predisposing features.
The PFC is significantly larger in humans, compared to rodents, due to the developmental expansion of the dorsolateral PFC and the frontal pole, seen in primates, but absent in rodents (Wise, 2008). The PFC in rodents consists of medial, orbitofrontal and cingulate areas and lacks an anatomical homolog of the primate dorsolateral PFC (Kolb et al., 2012; Kolk and Rakic, 2022). Nonetheless, the rodent prelimbic and infralimbic subregions of the PFC are considered functionally homologous to the human pregenual anterior cingulate cortex (Brodmann area 24) and the subgenual cingulate cortex (Brodmann area 25), both widely shown to be involved in MDD (Uylings et al., 2003; Kolb et al., 2012; Pizzagalli and Roberts, 2022). The PFC is highly implicated in reward, motivated behavior, memory, decision-making, and reinforcement learning (Fuster, 2002; Alexander and Brown, 2011; Euston et al., 2012; Wang et al., 2018) while also required for top-down processing, cognitive control, the internal representation of goals, goal-directed behavior, and planning. Dysfunction of the PFC in humans leads to impulsive, disorganized, and socially inappropriate behavior (Wise et al., 1996; Miller, 1999; Miller and Cohen, 2001; Dalley et al., 2004). As part of the frontal lobes, the PFC is one of the last brain areas to fully mature, with its development completing well into adulthood (Sowell et al., 1999; Fuster, 2002; Gogtay et al., 2004; Parker et al., 2020). It is plausible that, due in part to its protracted critical maturation period, the PFC is uniquely vulnerable to stress-induced insults.
The dysfunction of the PFC is well documented in MDD and in rodent models for chronic stress-induced depression-like behavioral abnormalities, including the adult chronic social defeat paradigm (CSDS) (George et al., 1994; Czéh et al., 2007; Ma et al., 2016; Murrough et al., 2016; Liu et al., 2017; Belleau et al., 2018). CSDS induces alterations in the function of the PFC circuit, which augment the susceptibility to developing social and motivational deficits (Vialou et al., 2014; Bagot et al., 2015; Bonnefil et al., 2019). More precisely, exposure to chronic stress induces loss of dendrites and spines in PFC neurons, and these changes are associated with impairments in PFC functioning and PFC-mediated behaviors such as decision-making and attentional set-shifting (Radley et al., 2005, 2006; Liston et al., 2006; Dias-Ferreira et al., 2009; Hains et al., 2009; Shansky et al., 2009). These structural modifications do not appear to be permanent, since after long periods in the absence of stress, the number of dendrites and spines increases back to baseline levels (Radley et al., 2005). The neuronal changes mentioned are not pleiotropic throughout the PFC, but are circuit specific. For example, Shansky et al. (2009) found that chronic stress induces a reduction in dendritic number in PFC neurons that have cortico-cortical projections but leads to an increase in the number of dendrites of PFC neurons innervating the amygdala. The concentration of circulating blood estrogen as dictated by biological sex has also emerged as an important factor. The same group showed, using a model for pharmacological activation of the stress system, that female rats are more sensitive than males to developing PFC-dependent working memory deficits, but only during the period of high levels of circulating estrogen. Ovariectomized females had lower stress sensitivity, but regained high sensitivity to stress after exogenous estrogen replacement (Shansky et al., 2004, 2010). Sexually dimorphic changes in adult PFC synaptic connectivity induced by chronic stress which are dependent on circulating gonadal hormones have also been shown using various paradigms. Many types of chronic stress ultimately result in removal of PFC synapses, suggesting that different stressors produce a similar outcome, possibly through a shared mechanism (Popoli et al., 2012; Wellman and Moench, 2019; Woo et al., 2021). While the endpoint effector pathway has not yet been identified for the PFC, in the hippocampus, the expression of the cell adhesion protein PSA-NCAM has been proven to decrease in rodent models of depression-like behaviors, while the effectiveness of antidepressants correlates with increased PSA-NCAM in the hippocampus (Wainwright and Galea, 2013). We argue that understanding the molecular players involved in stress-induced synaptic connectivity changes in the PFC is fundamental for developing novel therapeutic approaches.
Much of our understanding of the cellular and molecular processes involved in the development of depression-like behavior comes from studies using rodents. Modeling chronic stress entails exposing rodents to prolonged stress, such as social stress, physical restraint or inescapable foot shock, direct activation of the stress system, or to various types of stressors (wet bedding, loud noise) presented in a variable sequence to prevent habituation effects (Newman et al., 2018). The effect of stress on anhedonia, learned helplessness, social avoidance, appetite and body weight, sleep and circadian rhythms, self-care/grooming, among several other measures, are assessed to determine the impact of stress on depression-like behavioral states (Nestler and Hyman, 2010; Krishnan and Nestler, 2011; Czéh et al., 2016; Wang et al., 2017; Antoniuk et al., 2018; Torres-Berrío et al., 2020a). It should be noted that some of the behavioral and physiological alterations induced in these rodent paradigms reproduce aspects of the traits observed in MDD, helping us understand how brain function and behavior change in response to chronic stress. However, it should be emphasized that these rodent models mimic neither depressive symptoms, as experienced by humans, nor the complex interaction between genetic and environmental factors. Often, following chronic stress exposure, susceptible and resilient phenotypes are assessed in behavioral tests that measure phenotypic traits such as reward-seeking and reward sensitivity, learning, social preference and/or interaction, reward preference, and time spent grooming (Nestler and Hyman, 2010; Krishnan and Nestler, 2011; Czéh et al., 2016; Wang et al., 2017; Antoniuk et al., 2018; Torres-Berrío et al., 2020a). Figure 1 depicts some of the most commonly employed models for chronic stress used in adult male and female mice. Despite the limitations of using rodents, results from stress models have revealed changes in neural circuitry in brain areas implicated in MDD to be associated with resilience/susceptibility to stress (Frazer and Morilak, 2005; Nestler and Hyman, 2010; Wang et al., 2017; Bale et al., 2019; Parekh et al., 2022).
Figure 1. Rodent models for chronic stress-induced depression-like behaviors. (A) These are examples of commonly employed sex non-specific paradigms such as different variations of chronic/subchronic variable (mild) stress (chronically exposing the experimental animals to different stressors in random order), social instability stress (disturbances in an animal’s social hierarchy), social isolation, and corticosterone administration. These can be used in both male and female mice to investigate biological sex-mediated mechanisms in stress vulnerability. In these paradigms animals are not segregated into resilient and susceptible groups, thus examination of individual differences in stress susceptibility is limited. (B) A widely used and validated model of chronic stress in male mice is CSDS. In this procedure, control animals are housed with a conspecific and a divider that allows sensory stimuli. Each experimental male mouse is exposed to a social defeat session (for 5–10 min each day) in which the mouse is socially defeated by an aggressive CD-1 mouse for several (often 10) consecutive days. After the CSDS, all mice are assessed in a social interaction test to evaluate their sociability toward a social target (e.g., a novel CD-1 mouse). This will classify each experimental mouse in either susceptible or resilient phenotype. (C) A major limitation of the CSDS model is that female mice are not readily attacked by an aggressor CD-1 mouse. Thus, the CSDS model has needed to be adapted for female mice. A few different approaches have been described, such as a modified version of CSDS involving Swiss Webster female mice (Newman et al., 2019; Williams et al., 2022) and CSDS with lactating females, (Bourke and Neigh, 2012; Jacobson-Pick et al., 2013) both shown to induce behavioral deficits in female mice. However, the defeated mice are not segregated into resilient and susceptible groups, which limits in-depth assessment. An additional paradigm involves marking female experimental mice with male urine to induce aggression from CD-1 mice within a CSDS framework (Harris et al., 2018). Therefore, it can be used in parallel with classical CSDS in males to investigate social stress vulnerability in both sexes and identify divergent effects either at the behavioral or molecular levels.
While several models for chronic stress can be implemented in either male or female rodents (Figure 1A), some of them have only been applied to males, particularly those developed for mice (Figure 1B). One of these models is the CSDS paradigm in which an adult C57BL/6 experimental male mouse is subjected to repeated physical attacks and submission by an aggressive CD-1 conspecific male. Defeated mice can then be classified as “susceptible” or “resilient” based on their social approach phenotype in a social interaction test performed typically 24 h after the last defeat session (Figure 1B). Susceptible mice also show deficits in other behavioral domains linked to MDD, for example anhedonia (Berton et al., 2006; Krishnan et al., 2007; Golden et al., 2011). A limitation of the standard CSDS model is that adult females are not attacked by an aggressive conspecific, which hinders implementation in females of models designed for male mice. Due to this difference between male-male and male-female aggression, it has been difficult to run social defeat protocols identically for both male and female mice, a known confounding factor in the field. However, to overcome this limitation, new models adapted for females are emerging, including chemogenetically activating the ventrolateral subdivision of the ventromedial hypothalamus of the CD-1 aggressor to induce attacks toward females (Takahashi et al., 2017), applying urine from male mice to experimental females to induce aggression from CD-1 mice, (Harris et al., 2018) vicarious social defeat stress model (Iñiguez et al., 2018), and the chronic non-discriminatory social defeat stress model (Figure 1C; Yohn et al., 2019). Due, in part, to our extensive work with mouse models, we, and others, posit that stress models for females and males do not need to be identical for them to generate meaningful insights into stress pathophysiology, and can be adapted depending on the questions asked (Lopez and Bagot, 2021). For example, standard CSDS in males and chronic variable stress in females can be used to investigate sex-specific molecular mechanisms whereas chronic non-discriminatory social defeat stress can be used both in male and female mice simultaneously to investigate sex-dependent mechanisms and potential sex-differences.
Axonal guidance cue systems play critical roles in the development and maturation of the PFC and its circuitry, with more recent evidence implicating them in dysfunction in the adult brain (Schubert et al., 2015; Yamagishi et al., 2021; Kolk and Rakic, 2022). Multiple neuronal inputs form very selective synaptic connections with local PFC neurons, essential for proper PFC functioning. Classical guidance molecule families such as the netrin, ephrin, slit, and semaphorin (cartoon representation in Figure 2) orchestrate the formation of PFC functional networks across the lifespan and perform maintenance roles of these connections in later life. Apart from conventional axonal guidance cues, morphogens, growth factors and cell adhesion proteins (Figure 2) are also involved in proper target recognition of PFC efferents and in synapse formation (Schubert et al., 2015). Growth factors and morphogens–fibroblast growth factors (FGFs), BMPs, SHH, and WNTs–work in tandem with conventional guidance cue systems and adhesion proteins to pattern and steer PFC formation, including integration of connections of various neurotransmitter systems within the PFC) (Schubert et al., 2015). Disruptions to this coordinated process can lead to altered neuronal connectivity and, in turn, behavior. Therefore, it is known that experiences capable of altering guidance cue function can induce changes in the formation of developing neuronal networks, which can translate into behavioral abnormalities later on in life. To govern neuronal connectivity and plasticity, the adult brain uses the very same axonal guidance cues involved in the proper wiring and pathfinding of developing neurons to control dendritic arborization and synapse formation in adulthood (Glasgow et al., 2020). Since guidance cues continue to be expressed in the adult brain, it is conceivable that exposure to chronic stress alters guidance cue function to modify already established connections. We contend that chronic stress in adulthood could disrupt the fine-tuned orchestra of axonal guidance cues and induce depressive-like behaviors. In the following section, and in Table 1, we list studies and review evidence suggesting that changes in guidance cue function induced by stress lead to depressive states.
Figure 2. Representation of the classical guidance cue systems and adhesion proteins involved in axonal guidance. Different effects of chronic stress ultimately result in removal of synapses and reduction in neurotransmission, suggesting that different stressors may induce the same outcome through shared mechanisms. Here we show cartoon representations of the domain arrangements of the classical guidance cue families (Netrin, Slit, Semaphorin, Ephrin/Ephexin) and their receptors (inserted in bilayer), as well as cell adhesion proteins (laminin, NCAM and laminin receptor). From top to bottom, Netrin consists of a laminin-like domain, 3 epidermal growth factor (EGF) domains, and a Netrin-like domain; DCC consists of 4 Ig (immunoglobulin) domains, 6 fibronectin III-like (FN) domains, a transmembrane region and 3 P-motifs; Robo consists of 5 Ig domains, 3 FN domains, a membrane spanning region and 4 proline-rich regions; Slit consists of 4 leucine-rich repeats, 6 EGF-like domains, a laminin G-like module, 3 EGF-like domains and a cysteine knot; Semaphorin consists of a Sema domain, a PSI (plexin, semaphorin, and integrin) domain and an Ig domain; Neuropilin consists of 2 Sema binding domains, 2 VEGF (vascular endothelial growth factors) binding subdomains, a MAM (meprin, A-5 protein, and receptor protein-tyrosine phosphatase mu) domain, a transmembrane domain and a PDZ [post synaptic density protein (PSD95), drosophila disc large tumor suppressor (Dlg1), and zonula occludens-1 protein (zo-1)] domain; Plexin consist of a Sema domain, 3 PSI domains, 3 IPT (Ig-like, plexins, transcription factors) domains, a transmembrane region, a GAP (GTPase-accelerating proteins) domain, an RBD (Rho-GTPase binding domain) sequence and a GAP domain; the Ephrin receptor A/B consists of an ephrin binding, and EGF-like domain, two FN domains, a membrane spanning region, a kinase domain, a SAM (Sterile alpha motif) domain and a PDZ-binding domain; Ephrin consists of a membrane spanning region and a receptor binding region; Ephexin consists of a DH (Dbl homology) domain, a PH (Pleckstrin homology) domain and a SH3 (SRC Homology 3) domain; laminin consists of 3 laminin domains in the alpha chain, and 2 laminin domains in both the beta and the gamma chains, a coiled coil and 5 laminin G domains; NCAM consists of 5 IgG-like domains and two FN domains; Laminin receptor consists of single-chain variable fragment (scFv) Ig antibody binding domain, a heparin/laminin binding domain, a laminin/PrP binding domain, a transmembrane domain, and a N-terminal domain.
Table 1. Preclinical and human studies linking guidance cues to chronic stress-induced PFC reorganization and MDD (findings from rodent and human studies do not necessarily match).
Netrin-1 is a secreted protein that attracts or repels axons and neurites by binding to transmembrane receptors, most notably deleted in colorectal cancer (DCC) and uncoordinated 5 (UNC5) homologues (Boyer and Gupton, 2018). Although peaking in neuronal cells during embryogenesis, netrin-1 and its receptors continue to be expressed in the adult matured brain, including in the PFC (Manitt et al., 2011; Goldman et al., 2013; Torres-Berrío et al., 2017). In adult mice, exposure to CSDS upregulates DCC receptor expression in PFC neurons, but only in those exhibiting a susceptible phenotype (Torres-Berrío et al., 2017). Experimentally increasing or decreasing DCC levels in PFC pyramidal neurons of adult male mice induces susceptibility or resilience, respectively, indicating a causal role. Altered expression of DCC in the PFC appears to be a persistent trait in MDD, supported by two independent post-mortem brain studies which show increased Dcc mRNA levels in the PFC of adult patients who died by suicide compared to non-psychiatric control subjects (Manitt et al., 2013; Torres-Berrío et al., 2017). A large and increasing number of studies show a tight link between genetic variation in the Netrin-1/DCC system and MDD. For a detailed description of the role of Netrin-1 and DCC receptors in stress-induced behavioral alterations in rodents and in MDD see Torres-Berrío et al. (2020a,b) and Vosberg et al. (2020).
Further confirmation of the Netrin-1/DCC system as a depression risk marker comes from a recent study that integrated multi-omics data. Li et al. (2020) conducted a genome-wide integrative analysis of depression and validated their findings in independent replications across different ethnic populations. The authors found that the DCC gene predicts the risk of depression in both Europeans and Han Chinese, with higher Dcc mRNA expression in the PFC associated with depression-relevant personality traits, cognitive function and putamen volumes in independent samples.
Entailing an additional layer of regulation, microRNA mechanisms mediate stress-induced changes in DCC receptor expression in the PFC of susceptible mice. Exposure to CSDS upregulates Dcc mRNA expression in the PFC by downregulating microRNA miR-218, a potent repressor of DCC receptors. In male mice, manipulating miR-218 in the PFC leads to corresponding changes in DCC levels in local neurons, inducing stress susceptibility or resilience, while in humans, miR-218 dysregulation is implicated in MDD (Manitt et al., 2013; Torres-Berrío et al., 2017). Due to the limitations in performing neurogenetic experiments on humans, the mechanism of how variations of DCC receptor expression in the PFC impact human behavior and psychopathology remains to be established. However, miR-218-mediated remodeling of dendritic spines in local neurons has been reported (Torres-Berrío et al., 2020b), in line with the strong influence of this pathway in the organization of dendritic structure and synaptic plasticity of the adult PFC (Goldman et al., 2013; Glasgow et al., 2020). Taken together, these findings suggest that alterations in the Netrin-1/DCC guidance cue system in the PFC predispose rodents to stress-induced susceptibility and depression-like behaviors, while also being associated with MDD in humans. For this reason, it constitutes a viable target for the development of new treatments for depression.
Slits (SLIT1-3) are secreted proteins that act as a repulsive axon guidance cue, normally repelling growth cones by engaging roundabout (ROBO) class receptors (Gonda et al., 2020). Both netrins and Slits play an important role in midline axon guidance. Once a growing axon has crossed the midline in the developing brain, repulsion by Slits inhibits recrossing (Ypsilanti et al., 2010). Mutations in Slit-receptor ROBO induce midline guidance defects in humans (Battum et al., 2015). Recent studies have implicated Slits and their receptors in depression-related behaviors in mice, as well as in MDD in humans. For example, adult transgenic mice constitutively overexpressing human Slit2 in whole-body exhibited increased depression-/anxiety-like behavior alterations assessed by sucrose preference test, open field test and elevated plus maze, with lower body weights compared to wild-type animals (Huang et al., 2021). Van der Zee et al. (2022) revealed a SLIT1 downregulation in the ventromedial PFC of women with MDD in comparison with healthy controls, an effect not seen in men with depression. Similarly, a sex-specific pattern of Slit1 downregulation was discovered in the ventromedial PFC of female mice exposed to chronic variable stress but not in males. After performing a knockdown of Slit1 expression in the ventromedial PFC of both sexes of mice, female mice had a sex-specific elevation in depression-like behaviors, as well as a decreased dendritic arborization and excitability of ventromedial PFC pyramidal neurons, with both findings absent in males. For a more extensive list of studies implicating Slits in stress-induced depression-like behaviors and MDD, please consult Table 1.
Unlike other conventional guidance cues, which can be either membrane bound or secreted, all ephrins are membrane-bound ligands that activate Eph receptors (Ephs) on the surface of neighboring cells, to induce either attraction or repulsion (Egea and Klein, 2007). In some cases, ephrin- and Eph-expressing cells can engage in “reverse signaling” in which ephrin transduces a signal in its expressing cell while the Eph receptor acts as the ligand (Holland et al., 1996; Lisabeth et al., 2013). Both “forward” and “reverse,” termed “bidirectional signaling,” are important for the proper formation and maintenance of local circuit microarchitecture (Murai and Pasquale, 2003; Lisabeth et al., 2013).
Zhang et al. (2017) investigated the role of the ephrin receptor EphA4– ephexin1 signaling in depressive-like behaviors and examined if systemic administration of rhynchophylline, an EPHA4 inhibitor, has an antidepressant-like effect. Adult male mice were subjected to CSDS, and afterward susceptible mice showed increased levels of phosphorylated EPHA4 and ephexin1 in the PFC and hippocampus. Overexpression of EPHA4 in the PFC induced depressive-like phenotypes and rhynchophylline reversed the phenotypes in these mice. Furthermore, Zhang et al. (2017) corroborates the importance of these findings by showing that individuals with MDD also have a higher level of phosphorylated EPHA4 in the parietal cortex, compared with healthy controls. Chang et al. (2014) conducted meta-clustering of gene expression links in 11 transcriptome studies from post-mortem PFC brain samples of MDD and non-psychiatric control subjects. Further implicating the ephrin pathway in depression, they found that Ephrin receptors (EPHA3, EPHA5) genes were among 88 genes that were significantly associated with MDD by GWAS, and with medical illnesses with increased clinical risk of depression, but not for other illness. These findings suggest that ephrin and ephexin signaling through Eph receptors in the PFC is a potential marker of depressive-like phenotypes and is associated with MDD, making this pathway a potential therapeutic target for depression. For additional studies implicating the ephrin, ephexin/Eph receptor pathway to stress and depressive-like behavior or MDD, please consult Table 1.
Semaphorins represent a family of thirty proteins, grouped into eight classes, seven of which are found in animals (SEMA1-7) and one in viruses (SEMAV). Semaphorins can either be secreted, membrane spanning, or membrane associated and are primarily axonal repellents, activating complexes of cell-surface receptors called Plexin family proteins (Plexin A1-4) and Neuropilin family proteins (Neuropilin-1 and -2) (Kolodkin et al., 1993). For a comprehensive review of semaphorin function in the adult brain, please consult Alto and Terman (2016), Carulli et al. (2021).
SEMA3F, a secreted semaphorin, has been implicated in depressive-like behaviors in male mice. Matsuda et al. (2016) showed that SEMA3F knockout male mice show a reduced score in the social interaction test compared with controls, among multiple other neurological findings. In human psychopathology research, a GWAS study identified a risk variant in the SEMA3A gene associated with comorbid alcohol dependence and depression in African American participants (Zhou et al., 2017). For additional studies implicating chronic stress or MDD to changes in semaphorin signaling, please consult Table 1.
Recent studies highlight the roles of WNT and SHH signaling in depression and depression-like behaviors, possibly via BDNF-mediated processes (Voleti and Duman, 2012; Tayyab et al., 2018). WNT signaling may regulate depression-like behaviors by altering adult neurogenesis, dendritic morphology, synaptic plasticity and synaptic transmission. For a detailed review on the role of WNT signaling, please refer to Voleti and Duman (2012). The role of morphogens on stress-induced alterations in the adult PFC has not been sufficient investigated.
Several non-conventional guidance molecules such as laminin, tenascins, proteoglycans, N-CAM, and L1-CAM participate in axonal guidance. If considered together, a large body of evidence implicate these molecules in depression-like behaviors. For instance, the polysialylated (PSA) form of N-CAM is involved in the development and migration of neurons in the immature vertebrate brain (Quartu et al., 2008), while also participating in neurite and synaptic remodeling (Varea et al., 2007). Although expressed throughout the lifetime, it is required for synaptogenesis and structural plasticity in adulthood, with multiple studies implicating it in the structure and function of the adult male rat PFC (Varea et al., 2005; Castillo-Gómez et al., 2008, 2011; Rutishauser, 2008). PSA-NCAM mediates synaptic plasticity, neurogenesis, signaling by neurotrophic factors and inflammatory messengers in the brain, all relevant processes in depression-like behaviors (Saini et al., 2020). This makes PSA-NCAM a viable candidate for increasing neuroplasticity, a hallmark in the modern hypothesis of treatment for MDD. Chronic treatment with the tricyclic antidepressant imipramine increased the expression of polysialylated N-CAM in the PFC and hippocampus (Sairanen et al., 2007). Similarly, chronic fluoxetine treatment increased the PSA N-CAM expression in the PFC (Varea et al., 2007). However, selective cleaving of polysialylation moieties using endoneuraminidase N inhibited the antidepressant efficacy of fluoxetine in a chronic unpredictable stress model for depression-like behaviors, likely by disrupting the interaction of PSA-NCAM with the D2 dopamine receptor in the medial PFC (Castillo-Gómez et al., 2008, 2011; Wainwright et al., 2016). Due to the structural nature of PSA-NCAM, direct administration using purified protein would be an impediment to its utility as an antidepressant, which led researchers to use peptide mimetics of NCAM to circumvent this barrier (Hansen et al., 2009; Køhler et al., 2010; Xu et al., 2014). Turner et al. (2019) compared three of these peptide mimetics in rats and found that each one had either anxiolytic or antidepressant effects with their own intrinsic kinetics, which consolidates this unconventional area of depression research. These findings suggest that chronic stress downregulates the expression of the PSA-NCAM in the PFC, and that PSA-NCAM-mediated synaptic plasticity is necessary for antidepressant action. For additional work linking cell adhesion molecules to MDD or depression-like behaviors, please read the work of Laifenfeld et al. (2005a,b).
The hippocampus is another brain area in which disruption in axonal guidance signaling can lead to depression-like phenotypes. Notably, neogenin, a multifunctional transmembrane receptor, participates in adult hippocampal neurogenesis. Loss of neogenin reduced dendritic branches and spines, impaired glutamatergic neurotransmission, and mice with depletion of neogenin in adult neural stem cells or neural progenitor cells showed depressive-like behavior (Sun et al., 2018). Gui et al. (2021) compared the hippocampal transcriptional features between four models [i.e., chronic unpredictable mild stress (CUMS), CSDS, learned helplessness and MDD patients]. The authors found that axonal guidance signaling was one of the seven significantly enriched pathways in all four models (Gui et al., 2021). Li et al. (2022) reported that EPHA4 expression is increased in the excitatory neurons in the hippocampus of mice subjected to CUMS, and knockdown of EphA4 prevented depression-like behaviors. EPHA4 levels were significantly higher in MDD samples compared to control individuals (Li et al., 2022). The administration of fluoxetine for 4 weeks restored dysregulated EPHA4 levels in fluoxetine responder rats compared to antidepressant resistant rats (Li et al., 2014). This suggests that ephrin signaling in the hippocampus is implicated in the antidepressant effect.
Semaphorin 3B in the hippocampus may play a role in inducing depression-like behaviors. Levels of SEMA3B protein are decreased in the hippocampus and serum of chronic mild stress (CMS)-treated mice (Du et al., 2022). Increasing the levels of SEMA3B in the hippocampus or the lateral ventricles, improved CMS-induced depression-like behaviors and increased resilience to acute stress by increasing dendritic spine density in hippocampal neurons (Du et al., 2022).
Emerging findings suggest that Shh is involved in adult hippocampal neurogenesis and may have an antidepressant effect. Yao et al. (2016) investigated the role of Shh in electroconvulsive (ECT) therapy in rats. ECT is often used in treatment-resistant depression and severe cases of depression. ECT induced the proliferation of hippocampal neural progenitor cells in rats, and blockade of Shh signaling with cyclopamine completely inhibited ECT-induced neural progenitor cell proliferation (Yao et al., 2016). However, it is yet to be investigated how Shh signaling mechanisms induce depression-like behaviors.
Measuring subtle changes in axonal guidance cue signaling in the PFC could offer a novel approach to precision medicine and the treatment of depression. MicroRNAs are promising molecules that fit this description, as their expression level has previously been well correlated with structural and functional changes in the PFC, as exemplified by mir-218 in a previous section, and they can readily be detected in peripheral fluids. It is conceivable that changes in these biomarkers could correlate between the brain and peripheral fluids under normal circumstances and could also be used as a readout of treatment efficacy. Chronic stress-induced changes in the PFC in rodents, often in axonal guidance signaling pathways, may coincide with changes in levels of biomarkers (other than mir-218) in peripheral fluids, such as saliva, and peripheral blood (Torres-Berrío et al., 2020b; Morgunova and Flores, 2021). For example, Fan et al. (2014) reported that compared to age and gender-matched control subjects, in depressed patients, 25 of 26 identified miRNAs were upregulated. These miRNAs are all involved in pathways related to axon guidance, WNT signaling, ERBB signaling, mTOR signaling, VEGF signaling, and long-term potentiation (Lopizzo et al., 2019).
Depression is a chronic disabling disease, often induced by chronic stress. It is paramount to unravel the cellular and molecular pathophysiology of depression that could guide strategies to prevent its onset and help accelerate the development of novel therapeutics. In this review, we gathered accumulating evidence of the roles of axonal guidance cues in depression-like behaviors in rodents. We also discussed supporting data from human studies emphasizing the importance of further elucidating the involvement of guidance cues in MDD. We highlight the need of conducting translational research connecting human and rodent observations, that can help us better determine how and when alteration and disruption in the signaling pathways of various axonal guidance cues can affect vulnerability. Depression is twice more likely in women than men, and there is a great need to understand how and why this sex-difference arises. Since most preclinical studies have been conducted primarily on male rodents, relatively little is known about how biological sex, together with different behavioral coping strategies, induce male and female vulnerability. To move forward, rodent models should always include females or carefully justify the use of only one sex. If existing models do not work in females, new models need to be devised.
AM, RGA, and CF wrote the manuscript. All authors contributed to the article and approved the submitted version.
The present research was supported by the National Institute on Drug Abuse (# DA037911 to CF), the Canadian Institutes of Health Research (# FRN 156272 to CF), and the Natural Sciences and Engineering Research Council of Canada (# RGPIN-2020-04703 to CF). AM was supported by the Laszlo and Etelka Kollar Fellowship from the Faculty of Medicine and Health Sciences (McGill University) and by a Postgraduate Scholarship-Doctoral (PGS D) from the Natural Sciences and Engineering Research Council of Canada (NSERC).
The authors declare that the research was conducted in the absence of any commercial or financial relationships that could be construed as a potential conflict of interest.
All claims expressed in this article are solely those of the authors and do not necessarily represent those of their affiliated organizations, or those of the publisher, the editors and the reviewers. Any product that may be evaluated in this article, or claim that may be made by its manufacturer, is not guaranteed or endorsed by the publisher.
Akil, H., Gordon, J., Hen, R., Javitch, J., Mayberg, H., McEwen, B., et al. (2018). Treatment resistant depression: A multi-scale, systems biology approach. Neurosci. Biobehav. Rev. 84, 272–288. doi: 10.1016/j.neubiorev.2017.08.019
Alexander, W. H., and Brown, J. W. (2011). Medial prefrontal cortex as an action-outcome predictor. Nat. Neurosci. 14, 1338–1344. doi: 10.1038/nn.2921
Alto, L. T., and Terman, J. R. (2016). Semaphorin signaling, methods and protocols. Methods Mol. Biol. 1493, 1–25. doi: 10.1007/978-1-4939-6448-2_1
Antoniuk, S., Bijata, M., Ponimaskin, E., and Wlodarczyk, J. (2018). Chronic unpredictable mild stress for modeling depression in rodents: Meta-analysis of model reliability. Neurosci. Biobehav. Rev. 99, 101–116. doi: 10.1016/j.neubiorev.2018.12.002
Bagot, R. C., Cates, H. M., Purushothaman, I., Lorsch, Z. S., Walker, D. M., Wang, J., et al. (2016). Circuit-wide transcriptional profiling reveals brain region-specific gene networks regulating depression susceptibility. Neuron 90, 969–983. doi: 10.1016/j.neuron.2016.04.015
Bagot, R. C., Parise, E. M., Peña, C. J., Zhang, H.-X., Maze, I., Chaudhury, D., et al. (2015). Ventral hippocampal afferents to the nucleus accumbens regulate susceptibility to depression. Nat. Commun. 6:7062. doi: 10.1038/ncomms8062
Bale, T. L., Abel, T., Akil, H., Carlezon, W. A. Jr., Moghaddam, B., Nestler, E. J., et al. (2019). The critical importance of basic animal research for neuropsychiatric disorders. Neuropsychopharmacology 44, 1349–1353. doi: 10.1038/s41386-019-0405-9
Battum, E. Y. V., Brignani, S., and Pasterkamp, R. J. (2015). Axon guidance proteins in neurological disorders. Lancet Neurol. 14, 532–546. doi: 10.1016/s1474-4422(14)70257-1
Belleau, E. L., Treadway, M. T., and Pizzagalli, D. A. (2018). The impact of stress and major depressive disorder on hippocampal and medial prefrontal cortex morphology. Biol. Psychiatry 85, 443–453. doi: 10.1016/j.biopsych.2018.09.031
Berton, O., McClung, C. A., DiLeone, R. J., Krishnan, V., Renthal, W., Russo, S. J., et al. (2006). Essential role of BDNF in the mesolimbic dopamine pathway in social defeat stress. Science 311, 864–868. doi: 10.1126/science.1120972
Bondar, N., Bryzgalov, L., Ershov, N., Gusev, F., Reshetnikov, V., Avgustinovich, D., et al. (2018). molecular adaptations to social defeat stress and induced depression in mice. Mol. Neurobiol. 55, 3394–3407. doi: 10.1007/s12035-017-0586-3
Bonnefil, V., Dietz, K., Amatruda, M., Wentling, M., Aubry, A. V., Dupree, J. L., et al. (2019). Region-specific myelin differences define behavioral consequences of chronic social defeat stress in mice. Elife 8:e40855. doi: 10.7554/elife.40855
Bourke, C. H., and Neigh, G. N. (2012). Exposure to repeated maternal aggression induces depressive-like behavior and increases startle in adult female rats. Behav. Brain Res. 227, 270–275. doi: 10.1016/j.bbr.2011.11.001
Boyer, N. P., and Gupton, S. L. (2018). Revisiting netrin-1: One who guides (Axons). Front. Cell Neurosci. 12:221. doi: 10.3389/fncel.2018.00221
Carulli, D., de Winter, F., and Verhaagen, J. (2021). Semaphorins in adult nervous system plasticity and disease. Front. Synaptic Neurosci. 13:672891. doi: 10.3389/fnsyn.2021.672891
Castillo-Gómez, E., Gómez-Climent, M. Á, Varea, E., Guirado, R., Blasco-Ibáñez, J. M., Crespo, C., et al. (2008). Dopamine acting through D2 receptors modulates the expression of PSA-NCAM, a molecule related to neuronal structural plasticity, in the medial prefrontal cortex of adult rats. Exp. Neurol. 214, 97–111. doi: 10.1016/j.expneurol.2008.07.018
Castillo-Gómez, E., Varea, E., Blasco-Ibáñez, J. M., Crespo, C., and Nacher, J. (2011). Polysialic acid is required for dopamine D2 receptor-mediated plasticity involving inhibitory circuits of the rat medial prefrontal cortex. PLoS One 6:e29516. doi: 10.1371/journal.pone.0029516
Chang, L.-C., Jamain, S., Lin, C.-W., Rujescu, D., Tseng, G. C., and Sibille, E. (2014). A conserved BDNF, glutamate-and GABA-enriched gene module related to human depression identified by coexpression meta-analysis and DNA variant genome-wide association studies. PLoS One 9:e90980. doi: 10.1371/journal.pone.0090980
Chaudhury, D., Liu, H., and Han, M.-H. (2015). Neuronal correlates of depression. Cell Mol. Life Sci. 72, 4825–4848. doi: 10.1007/s00018-015-2044-6
Czéh, B., Fuchs, E., Wiborg, O., and Simon, M. (2016). Animal models of major depression and their clinical implications. Prog. Neuro Psychopharmacol. Biol. Psychiatry 64, 293–310. doi: 10.1016/j.pnpbp.2015.04.004
Czéh, B., Müller-Keuker, J. I. H., Rygula, R., Abumaria, N., Hiemke, C., Domenici, E., et al. (2007). Chronic social stress inhibits cell proliferation in the adult medial prefrontal cortex: Hemispheric asymmetry and reversal by fluoxetine treatment. Neuropsychopharmacology 32, 1490–1503. doi: 10.1038/sj.npp.1301275
Dalley, J. W., Cardinal, R. N., and Robbins, T. W. (2004). Prefrontal executive and cognitive functions in rodents: Neural and neurochemical substrates. Neurosci. Biobehav. Rev. 28, 771–784. doi: 10.1016/j.neubiorev.2004.09.006
de Zwart, P. L., Jeronimus, B. F., and de Jonge, P. (2018). Empirical evidence for definitions of episode, remission, recovery, relapse and recurrence in depression: A systematic review. Epidemiol. Psychiatr. Sci. 28, 1–19. doi: 10.1017/s2045796018000227
Dent, E. W., Gupton, S. L., and Gertler, F. B. (2011). The growth cone cytoskeleton in axon outgrowth and guidance. Cold Spring Harb. Perspect. Biol. 3:a001800. doi: 10.1101/cshperspect.a001800
Dias-Ferreira, E., Sousa, J. C., Melo, I., Morgado, P., Mesquita, A. R., Cerqueira, J. J., et al. (2009). Chronic stress causes frontostriatal reorganization and affects decision-making. Science 325, 621–625. doi: 10.1126/science.1171203
Dickson, B. J. (2002). Molecular mechanisms of axon guidance. Science 298, 1959–1964. doi: 10.1126/science.1072165
Dominici, C., Moreno-Bravo, J. A., Puiggros, S. R., Rappeneau, Q., Rama, N., Vieugue, P., et al. (2017). Floor-plate-derived netrin-1 is dispensable for commissural axon guidance. Nature 545, 350–354. doi: 10.1038/nature22331
Du, Y., Shi, Y., Wang, X., Song, H., Wang, X., Hao, Y., et al. (2022). Hippocampal semaphorin 3B improves depression-like behaviours in mice by upregulating synaptic plasticity and inhibiting neuronal apoptosis. J. Neurochem. 163, 133–148. doi: 10.1111/jnc.15680
Duman, R. S., Aghajanian, G. K., Sanacora, G., and Krystal, J. H. (2016). Synaptic plasticity and depression: New insights from stress and rapid-acting antidepressants. Nat. Med. 22, 238–249. doi: 10.1038/nm.4050
Duman, R. S., and Aghajanian, G. K. (2012). Synaptic dysfunction in depression: Potential therapeutic targets. Science 338, 68–72. doi: 10.1126/science.1222939
Egea, J., and Klein, R. (2007). Bidirectional Eph–ephrin signaling during axon guidance. Trends Cell Biol. 17, 230–238. doi: 10.1016/j.tcb.2007.03.004
Euston, D. R., Gruber, A. J., and McNaughton, B. L. (2012). The role of medial prefrontal cortex in memory and decision making. Neuron 76, 1057–1070. doi: 10.1016/j.neuron.2012.12.002
Fan, H., Sun, X., Guo, W., Zhong, A., Niu, W., Zhao, L., et al. (2014). Differential expression of microRNA in peripheral blood mononuclear cells as specific biomarker for major depressive disorder patients. J. Psychiatr. Res. 59, 45–52. doi: 10.1016/j.jpsychires.2014.08.007
Fischer, A. S., Keller, C. J., and Etkin, A. (2016). The clinical applicability of functional connectivity in depression: Pathways toward more targeted intervention. Biol. Psychiatry Cogn. Neurosci. Neuroimaging 1, 262–270. doi: 10.1016/j.bpsc.2016.02.004
Frazer, A., and Morilak, D. A. (2005). What should animal models of depression model? Neurosci. Biobehav. Rev. 29, 515–523. doi: 10.1016/j.neubiorev.2005.03.006
Fuster, J. M. (2002). Frontal lobe and cognitive development. J. Neurocytol. 31, 373–385. doi: 10.1023/a:1024190429920
George, M. S., Ketter, T. A., and Post, R. M. (1994). Prefrontal cortex dysfunction in clinical depression. Depression 2, 59–72. doi: 10.1002/depr.3050020202
Glasgow, S. D., Ruthazer, E. S., and Kennedy, T. E. (2020). Guiding synaptic plasticity: Novel roles for netrin-1 in synaptic plasticity and memory formation in the adult brain. J. Physiol. 599, 493–505. doi: 10.1113/jp278704
Gogtay, N., Giedd, J. N., Lusk, L., Hayashi, K. M., Greenstein, D., Vaituzis, A. C., et al. (2004). Dynamic mapping of human cortical development during childhood through early adulthood. Biol. Sci. 101, 8174–8179. doi: 10.1073/pnas.0402680101
Gold, S. M., Köhler-Forsberg, O., Moss-Morris, R., Mehnert, A., Miranda, J. J., Bullinger, M., et al. (2020). Comorbid depression in medical diseases. Nat. Rev. Dis. Primers 6:69. doi: 10.1038/s41572-020-0200-2
Golden, S. A., Covington, H. E., Berton, O., and Russo, S. J. (2011). A standardized protocol for repeated social defeat stress in mice. Nat. Protoc. 6, 1183–1191. doi: 10.1038/nprot.2011.361
Goldman, J. S., Ashour, M. A., Magdesian, M. H., Tritsch, N. X., Harris, S. N., Christofi, N., et al. (2013). Netrin-1 promotes excitatory synaptogenesis between cortical neurons by initiating synapse assembly. J. Neurosci. 33, 17278–17289. doi: 10.1523/jneurosci.1085-13.2013
Gonda, Y., Namba, T., and Hanashima, C. (2020). Beyond axon guidance: Roles of Slit-Robo signaling in neocortical formation. Front. Cell Dev. Biol. 8:607415. doi: 10.3389/fcell.2020.607415
Goswami, D. B., Jernigan, C. S., Chandran, A., Iyo, A. H., May, W. L., Austin, M. C., et al. (2013). Gene expression analysis of novel genes in the prefrontal cortex of major depressive disorder subjects. Prog. Neuro Psychopharmacol. Biol. Psychiatry 43, 126–133. doi: 10.1016/j.pnpbp.2012.12.010
Guan, K.-L., and Rao, Y. (2003). Signalling mechanisms mediating neuronal responses to guidance cues. Nat. Rev. Neurosci. 4, 941–956. doi: 10.1038/nrn1254
Gui, S., Liu, Y., Pu, J., Song, X., Chen, X., Chen, W., et al. (2021). Comparative analysis of hippocampal transcriptional features between major depressive disorder patients and animal models. J. Affect. Disord. 293, 19–28. doi: 10.1016/j.jad.2021.06.007
Guo, H., Huang, Z., Wang, W., Zhang, S., Li, J., Cheng, K., et al. (2017). iTRAQ-based proteomics suggests Ephb6 as a potential regulator of the ERK pathway in the prefrontal cortex of chronic social defeat stress model mice. Proteomics Clin. Appl. 11:1700115. doi: 10.1002/prca.201700115
Hains, A. B., Vu, M. A. T., Maciejewski, P. K., van Dyck, C. H., Gottron, M., and Arnsten, A. F. T. (2009). Inhibition of protein kinase C signaling protects prefrontal cortex dendritic spines and cognition from the effects of chronic stress. Proc. Natl. Acad. Sci. U.S.A. 106, 17957–17962. doi: 10.1073/pnas.0908563106
Hansen, S. M., Li, S., Bock, E., and Berezin, V. (2009). Structure and function of the neural cell adhesion molecule NCAM. Adv. Exp. Med. Biol. 663, 355–372. doi: 10.1007/978-1-4419-1170-4_22
Hare, B. D., and Duman, R. S. (2020). Prefrontal cortex circuits in depression and anxiety: Contribution of discrete neuronal populations and target regions. Mol. Psychiatry 25, 2742–2758. doi: 10.1038/s41380-020-0685-9
Harris, A. Z., Atsak, P., Bretton, Z. H., Holt, E. S., Alam, R., Morton, M. P., et al. (2018). A novel method for chronic social defeat stress in female mice. Neuropsychopharmacology 43, 1276–1283. doi: 10.1038/npp.2017.259
Heshmati, M., and Russo, S. J. (2015). Anhedonia and the brain reward circuitry in depression. Curr. Behav. Neurosci. Rep. 2, 146–153. doi: 10.1007/s40473-015-0044-3
Holland, S. J., Gale, N. W., Mbamalu, G., Yancopoulos, G. D., Henkemeyer, M., and Pawson, T. (1996). Bidirectional signalling through the EPH-family receptor Nuk and its transmembrane ligands. Nature 383, 722–725. doi: 10.1038/383722a0
Huang, G., Wang, S., Yan, J., Li, C., Feng, J., Chen, Q., et al. (2021). Depression-/anxiety-like behavior alterations in adult Slit2 transgenic mice. Front. Behav. Neurosci. 14:622257. doi: 10.3389/fnbeh.2020.622257
Hüls, A., Robins, C., Conneely, K. N., Jager, P. L. D., Bennett, D. A., Epstein, M. P., et al. (2020). Association between DNA methylation levels in brain tissue and late-life depression in community-based participants. Transl. Psychiatry 10:262. doi: 10.1038/s41398-020-00948-6
Iñiguez, S. D., Flores-Ramirez, F. J., Riggs, L. M., Alipio, J. B., Garcia-Carachure, I., Hernandez, M. A., et al. (2018). Vicarious social defeat stress induces depression-related outcomes in female mice. Biol. Psychiatry 83, 9–17. doi: 10.1016/j.biopsych.2017.07.014
Jacobson-Pick, S., Audet, M.-C., McQuaid, R. J., Kalvapalle, R., and Anisman, H. (2013). Social agonistic distress in male and female mice: Changes of behavior and brain monoamine functioning in relation to acute and chronic challenges. PLoS One 8:e60133. doi: 10.1371/journal.pone.0060133
Jahan, M. S., Tsuzuki, T., Ito, T., Bhuiyan, E. R., Takahashi, I., Takamatsu, H., et al. (2022). PlexinA1-deficient mice exhibit decreased cell density and augmented oxidative stress in parvalbumin-expressing interneurons in the medial prefrontal cortex. IBRO Neurosci. Rep. 13, 500–512. doi: 10.1016/j.ibneur.2022.11.002
James, S. L., Abate, D., Abate, K. H., Abay, S. M., Abbafati, C., Abbasi, N., et al. (2018). Global, regional, and national incidence, prevalence, and years lived with disability for 354 diseases and injuries for 195 countries and territories, 1990–2017: A systematic analysis for the global burden of disease study 2017. Lancet 392, 1789–1858. doi: 10.1016/s0140-6736(18)32279-7
Johnston, K. M., Powell, L. C., Anderson, I. M., Szabo, S., and Cline, S. (2019). The burden of treatment-resistant depression: A systematic review of the economic and quality of life literature. J. Affect. Disord. 242, 195–210. doi: 10.1016/j.jad.2018.06.045
Kennedy, T. E., Serafini, T., de la Torre, J. R., and Tessier-Lavigne, M. (1994). Netrins are diffusible chemotropic factors for commissural axons in the embryonic spinal cord. Cell 78, 425–435. doi: 10.1016/0092-8674(94)90421-9
Kessler, R. C., Berglund, P., Demler, O., Jin, R., Koretz, D., Merikangas, K. R., et al. (2003). The epidemiology of major depressive disorder: Results from the national comorbidity survey replication (NCS-R). JAMA 289, 3095–3105. doi: 10.1001/jama.289.23.3095
Køhler, L. B., Soroka, V., Korshunova, I., Berezin, V., and Bock, E. (2010). A peptide derived from a trans-homophilic binding site in neural cell adhesion molecule induces neurite outgrowth and neuronal survival. J. Neurosci. Res. 88, 2165–2176. doi: 10.1002/jnr.22380
Kolb, B., Mychasiuk, R., Muhammad, A., Li, Y., Frost, D. O., and Gibb, R. (2012). Experience and the developing prefrontal cortex. Proc. Natl. Acad. Sci. U.S.A. 109, 17186–17193. doi: 10.1073/pnas.1121251109
Kolk, S. M., and Rakic, P. (2022). Development of prefrontal cortex. Neuropsychopharmacology 47, 41–57. doi: 10.1038/s41386-021-01137-9
Kolodkin, A. L., Matthes, D. J., and Goodman, C. S. (1993). The semaphorin genes encode a family of transmembrane and secreted growth cone guidance molecules. Cell 75, 1389–1399. doi: 10.1016/0092-8674(93)90625-z
Krishnan, V., and Nestler, E. J. (2011). Molecular and functional models in neuropsychiatry. Curr. Top. Behav. Neurosci. 7, 121–147. doi: 10.1007/7854_2010_108
Krishnan, V., Han, M.-H., Graham, D. L., Berton, O., Renthal, W., Russo, S. J., et al. (2007). Molecular adaptations underlying susceptibility and resistance to social defeat in brain reward regions. Cell 131, 391–404. doi: 10.1016/j.cell.2007.09.018
Laifenfeld, D., Karry, R., Klein, E., and Ben-Shachar, D. (2005b). Alterations in cell adhesion molecule L1 and functionally related genes in major depression: A postmortem study. Biol. Psychiatry 57, 716–725. doi: 10.1016/j.biopsych.2004.12.016
Laifenfeld, D., Karry, R., Grauer, E., Klein, E., and Ben-Shachar, D. (2005a). Antidepressants and prolonged stress in rats modulate CAM-L1, laminin, and pCREB, implicated in neuronal plasticity. Neurobiol. Dis. 20, 432–441. doi: 10.1016/j.nbd.2005.03.023
Lanoue, V., and Cooper, H. M. (2019). Branching mechanisms shaping dendrite architecture. Dev. Biol. 451, 16–24. doi: 10.1016/j.ydbio.2018.12.005
Lau, E., and Margolis, R. U. (2010). Inhibitors of slit protein interactions with the heparan sulphate proteoglycan glypican-1: Potential agents for the treatment of spinal cord injury. Clin. Exp. Pharmacol. 37, 417–421. doi: 10.1111/j.1440-1681.2009.05318.x
Li, H.-J., Qu, N., Hui, L., Cai, X., Zhang, C.-Y., Zhong, B.-L., et al. (2020). Further confirmation of netrin 1 receptor (DCC) as a depression risk gene via integrations of multi-omics data. Transl. Psychiatry 10:98. doi: 10.1038/s41398-020-0777-y
Li, Y., Su, P., Chen, Y., Nie, J., Yuan, T.-F., Wong, A. H. C., et al. (2022). The Eph receptor A4 plays a role in demyelination and depression-related behavior. J. Clin. Invest. 132:e161559. doi: 10.1172/jci161559
Li, Y., Wang, H., Wang, X., Liu, Z., Wan, Q., and Wang, G. (2014). Differential expression of hippocampal EphA4 and ephrinA3 in anhedonic-like behavior, stress resilience, and antidepressant drug treatment after chronic unpredicted mild stress. Neurosci. Lett. 566, 292–297. doi: 10.1016/j.neulet.2014.03.008
Lisabeth, E. M., Falivelli, G., and Pasquale, E. B. (2013). Eph receptor signaling and ephrins. Cold Spring Harb. Perspect. Biol. 5:a009159. doi: 10.1101/cshperspect.a009159
Liston, C., McEwen, B. S., and Casey, B. J. (2009). Psychosocial stress reversibly disrupts prefrontal processing and attentional control. Proc. Natl. Acad. Sci. U.S.A. 106, 912–917. doi: 10.1073/pnas.0807041106
Liston, C., Miller, M. M., Goldwater, D. S., Radley, J. J., Rocher, A. B., Hof, P. R., et al. (2006). Stress-induced alterations in prefrontal cortical dendritic morphology predict selective impairments in perceptual attentional set-shifting. J. Neurosci. 26, 7870–7874. doi: 10.1523/jneurosci.1184-06.2006
Liu, W., Ge, T., Leng, Y., Pan, Z., Fan, J., Yang, W., et al. (2017). The role of neural plasticity in depression: From hippocampus to prefrontal cortex. Neural Plast. 2017:6871089. doi: 10.1155/2017/6871089
Lopez, J., and Bagot, R. C. (2021). Defining valid chronic stress models for depression with female rodents. Biol. Psychiatry 90, 226–235. doi: 10.1016/j.biopsych.2021.03.010
Lopizzo, N., Zonca, V., Cattane, N., Pariante, C. M., and Cattaneo, A. (2019). miRNAs in depression vulnerability and resilience: Novel targets for preventive strategies. J. Neural Transm. 126, 1241–1258. doi: 10.1007/s00702-019-02048-2
Ma, K., Guo, L., Xu, A., Cui, S., and Wang, J.-H. (2016). Molecular mechanism for stress-induced depression assessed by sequencing miRNA and mRNA in medial prefrontal cortex. PLoS One 11:e0159093. doi: 10.1371/journal.pone.0159093
Manitt, C., Eng, C., Pokinko, M., Ryan, R. T., Torres-Berrío, A., Lopez, J. P., et al. (2013). dcc orchestrates the development of the prefrontal cortex during adolescence and is altered in psychiatric patients. Transl. Psychiatry 3:e338. doi: 10.1038/tp.2013.105
Manitt, C., Mimee, A., Eng, C., Pokinko, M., Stroh, T., Cooper, H. M., et al. (2011). The netrin receptor DCC is required in the pubertal organization of mesocortical dopamine circuitry. J. Neurosci. 31, 8381–8394. doi: 10.1523/jneurosci.0606-11.2011
Matsuda, I., Shoji, H., Yamasaki, N., Miyakawa, T., and Aiba, A. (2016). Comprehensive behavioral phenotyping of a new Semaphorin 3 F mutant mouse. Mol. Brain 9:15. doi: 10.1186/s13041-016-0196-4
Matsumoto, Y., Irie, F., Inatani, M., Tessier-Lavigne, M., and Yamaguchi, Y. (2007). Netrin-1/DCC signaling in commissural axon guidance requires cell-autonomous expression of heparan sulfate. J. Neurosci. 27, 4342–4350. doi: 10.1523/jneurosci.0700-07.2007
McCormick, L. E., and Gupton, S. L. (2020). Mechanistic advances in axon pathfinding. Curr. Opin. Cell Biol. 63, 11–19. doi: 10.1016/j.ceb.2019.12.003
McEwen, B. S., and Morrison, J. H. (2013). The brain on stress: Vulnerability and plasticity of the prefrontal cortex over the life course. Neuron 79, 16–29. doi: 10.1016/j.neuron.2013.06.028
Meijers, R., Smock, R. G., Zhang, Y., and Wang, J.-H. (2019). Netrin synergizes signaling and adhesion through DCC. Trends Biochem. Sci. 45, 6–12. doi: 10.1016/j.tibs.2019.10.005
Miller, E. K. (1999). The prefrontal cortex complex neural properties for complex behavior. Neuron 22, 15–17. doi: 10.1016/s0896-6273(00)80673-x
Miller, E. K., and Cohen, J. D. (2001). An integrative theory of prefrontal cortex function. Annu. Rev. Neurosci. 24, 167–202. doi: 10.1146/annurev.neuro.24.1.167
Morgunova, A., and Flores, C. (2021). MicroRNA regulation of prefrontal cortex development and psychiatric risk in adolescence. Semin. Cell Dev. Biol. 118, 83–91. doi: 10.1016/j.semcdb.2021.04.011
Murai, K. K., and Pasquale, E. B. (2003). ‘Eph’ective signaling: Forward, reverse and crosstalk. J. Cell Sci. 116, 2823–2832. doi: 10.1242/jcs.00625
Murrough, J. W., Abdallah, C. G., Anticevic, A., Collins, K. A., Geha, P., Averill, L. A., et al. (2016). Reduced global functional connectivity of the medial prefrontal cortex in major depressive disorder. Hum. Brain Mapp. 37, 3214–3223. doi: 10.1002/hbm.23235
Musaelyan, K., Yildizoglu, S., Bozeman, J., Preez, A. D., Egeland, M., Zunszain, P. A., et al. (2020). Chronic stress induces significant gene expression changes in the prefrontal cortex alongside alterations in adult hippocampal neurogenesis. Brain Commun. 2:fcaa153. doi: 10.1093/braincomms/fcaa153
Mychasiuk, R., Muhammad, A., and Kolb, B. (2016). Chronic stress induces persistent changes in global DNA methylation and gene expression in the medial prefrontal cortex, orbitofrontal cortex, and hippocampus. Neuroscience 322, 489–499. doi: 10.1016/j.neuroscience.2016.02.053
Nestler, E. J., and Hyman, S. E. (2010). Animal models of neuropsychiatric disorders. Nat. Neurosci. 13, 1161–1169. doi: 10.1038/nn.2647
Newman, E. L., Covington, H. E., Suh, J., Bicakci, M. B., Ressler, K. J., DeBold, J. F., et al. (2019). Fighting females: Neural and behavioral consequences of social defeat stress in female mice. Biol. Psychiatry 86, 657–668. doi: 10.1016/j.biopsych.2019.05.005
Newman, E. L., Leonard, M. Z., Arena, D. T., de Almeida, R. M. M., and Miczek, K. A. (2018). Social defeat stress and escalation of cocaine and alcohol consumption: Focus on CRF. Neurobiol. Stress 9, 151–165. doi: 10.1016/j.ynstr.2018.09.007
Parekh, P. K., Johnson, S. B., and Liston, C. (2022). Synaptic mechanisms regulating mood state transitions in depression. Annu. Rev. Neurosci. 45, 581–601. doi: 10.1146/annurev-neuro-110920-040422
Parker, N., Patel, Y., Jackowski, A. P., Pan, P. M., Salum, G. A., Pausova, Z., et al. (2020). Assessment of neurobiological mechanisms of cortical thinning during childhood and adolescence and their implications for psychiatric disorders. JAMA Psychiatry 77, 1127–1136. doi: 10.1001/jamapsychiatry.2020.1495
Pizzagalli, D. A. (2014). Depression, stress, and anhedonia: Toward a synthesis and integrated model. Clin. Psychol. 10, 393–423. doi: 10.1146/annurev-clinpsy-050212-185606
Pizzagalli, D. A., and Roberts, A. C. (2022). Prefrontal cortex and depression. Neuropsychopharmacology 47, 225–246. doi: 10.1038/s41386-021-01101-7
Popoli, M., Yan, Z., McEwen, B. S., and Sanacora, G. (2012). The stressed synapse: The impact of stress and glucocorticoids on glutamate transmission. Nat. Rev. Neurosci. 13, 22–37. doi: 10.1038/nrn3138
Quartu, M., Serra, M. P., Boi, M., Ibba, V., Melis, T., and Fiacco, M. D. (2008). Polysialylated-neural cell adhesion molecule (PSA-NCAM) in the human trigeminal ganglion and brainstem at prenatal and adult ages. BMC Neurosci. 9:108. doi: 10.1186/1471-2202-9-108
Radley, J. J., Rocher, A. B., Janssen, W. G. M., Hof, P. R., McEwen, B. S., and Morrison, J. H. (2005). Reversibility of apical dendritic retraction in the rat medial prefrontal cortex following repeated stress. Exp. Neurol. 196, 199–203. doi: 10.1016/j.expneurol.2005.07.008
Radley, J. J., Rocher, A. B., Miller, M., Janssen, W. G. M., Liston, C., Hof, P. R., et al. (2006). Repeated stress induces dendritic spine loss in the rat medial prefrontal cortex. Cereb. Cortex 16, 313–320. doi: 10.1093/cercor/bhi104
Romero-Pimentel, A. L., Almeida, D., Muñoz-Montero, S., Rangel, C., Mendoza-Morales, R., Gonzalez-Saenz, E. E., et al. (2021). Integrative DNA methylation and gene expression analysis in the prefrontal cortex of Mexicans who died by suicide. Int. J. Neuropsychopharmacol. 24:yab042. doi: 10.1093/ijnp/pyab042
Rutishauser, U. (2008). Polysialic acid in the plasticity of the developing and adult vertebrate nervous system. Nat. Rev. Neurosci. 9, 26–35. doi: 10.1038/nrn2285
Saini, V., Kaur, T., Kalotra, S., and Kaur, G. (2020). The neuroplasticity marker PSA-NCAM: Insights into new therapeutic avenues for promoting neuroregeneration. Pharmacol. Res. 160:105186. doi: 10.1016/j.phrs.2020.105186
Sairanen, M., O’Leary, O. F., Knuuttila, J. E., and Castrén, E. (2007). Chronic antidepressant treatment selectively increases expression of plasticity-related proteins in the hippocampus and medial prefrontal cortex of the rat. Neuroscience 144, 368–374. doi: 10.1016/j.neuroscience.2006.08.069
Schubert, D., Martens, G. J. M., and Kolk, S. M. (2015). Molecular underpinnings of prefrontal cortex development in rodents provide insights into the etiology of neurodevelopmental disorders. Mol. Psychiatry 20, 795–809. doi: 10.1038/mp.2014.147
Shansky, R. M., Glavis-Bloom, C., Lerman, D., McRae, P., Benson, C., Miller, K., et al. (2004). Estrogen mediates sex differences in stress-induced prefrontal cortex dysfunction. Mol. Psychiatry 9, 531–538. doi: 10.1038/sj.mp.4001435
Shansky, R. M., Hamo, C., Hof, P. R., Lou, W., McEwen, B. S., and Morrison, J. H. (2010). Estrogen promotes stress sensitivity in a prefrontal cortex–amygdala pathway. Cereb. Cortex 20, 2560–2567. doi: 10.1093/cercor/bhq003
Shansky, R. M., Hamo, C., Hof, P. R., McEwen, B. S., and Morrison, J. H. (2009). Stress-induced dendritic remodeling in the prefrontal cortex is circuit specific. Cereb. Cortex 19, 2479–2484. doi: 10.1093/cercor/bhp003
Sowell, E. R., Thompson, P. M., Holmes, C. J., Jernigan, T. L., and Toga, A. W. (1999). In vivo evidence for post-adolescent brain maturation in frontal and striatal regions. Nat. Neurosci. 2, 859–861. doi: 10.1038/13154
Spellman, T., and Liston, C. (2020). Toward circuit mechanisms of pathophysiology in depression. Am J. Psychiatry 177, 381–390. doi: 10.1176/appi.ajp.2020.20030280
Stoeckli, E. T. (2018). Understanding axon guidance: Are we nearly there yet? Development 145:dev151415. doi: 10.1242/dev.151415
Sun, X.-D., Chen, W.-B., Sun, D., Huang, J., Li, Y.-Q., Pan, J.-X., et al. (2018). Neogenin in amygdala for neuronal activity and information processing. J. Neurosci. 38, 9600–9613. doi: 10.1523/jneurosci.0433-18.2018
Sun, Y., Ma, L., Chen, J., Wang, W., Peng, S., Cheng, Y., et al. (2021). RNA-seq co-expression network analysis reveals anxiolytic behavior of mice with Efnb2 knockout in parvalbumin+ neurons. Mol. Brain 14:118. doi: 10.1186/s13041-021-00829-z
Takahashi, A., Chung, J.-R., Zhang, S., Zhang, H., Grossman, Y., Aleyasin, H., et al. (2017). Establishment of a repeated social defeat stress model in female mice. Sci. Rep. 7:12838. doi: 10.1038/s41598-017-12811-8
Tayyab, M., Shahi, M. H., Farheen, S., Mariyath, M. P. M., Khanam, N., Castresana, J. S., et al. (2018). Sonic hedgehog, Wnt, and brain-derived neurotrophic factor cell signaling pathway crosstalk: Potential therapy for depression. J. Neurosci. Res. 96, 53–62. doi: 10.1002/jnr.24104
Theriault, R.-K., Manduca, J., and Perreault, M. (2021). A female-specific role for the EphA2 Receptor within the prefrontal cortex in mediating depression-like behaviours in rats. J. Psychiatry Neurosci. 46, E258–E270.
Tochigi, M., Iwamoto, K., Bundo, M., Sasaki, T., Kato, N., and Kato, T. (2008). Gene expression profiling of major depression and suicide in the prefrontal cortex of postmortem brains. Neurosci. Res. 60, 184–191. doi: 10.1016/j.neures.2007.10.010
Torres-Berrío, A., Hernandez, G., Nestler, E. J., and Flores, C. (2020a). The Netrin-1/DCC guidance cue pathway as a molecular target in depression: Translational evidence. Biol. Psychiatry 88, 611–624. doi: 10.1016/j.biopsych.2020.04.025
Torres-Berrío, A., Nouel, D., Cuesta, S., Parise, E. M., Restrepo-Lozano, J. M., Larochelle, P., et al. (2020b). MiR-218: A molecular switch and potential biomarker of susceptibility to stress. Mol. Psychiatry 25, 951–964. doi: 10.1038/s41380-019-0421-5
Torres-Berrío, A., Lopez, J. P., Bagot, R. C., Nouel, D., Bo, G. D., Cuesta, S., et al. (2017). DCC confers susceptibility to depression-like behaviors in humans and mice and is regulated by miR-218. Biol. Psychiatry 81, 306–315. doi: 10.1016/j.biopsych.2016.08.017
Turner, C. A., Lyons, D. M., Buckmaster, C. L., Aurbach, E. L., Watson, S. J., Schatzberg, A. F., et al. (2019). Neural cell adhesion molecule peptide mimetics modulate emotionality: Pharmacokinetic and behavioral studies in rats and non-human primates. Neuropsychopharmacology 44, 356–363. doi: 10.1038/s41386-018-0052-6
Uylings, H. B. M., Groenewegen, H. J., and Kolb, B. (2003). Do rats have a prefrontal cortex? Behav. Brain Res. 146, 3–17. doi: 10.1016/j.bbr.2003.09.028
Van der Zee, Y. Y., Lardner, C. K., Parise, E. M., Mews, P., Ramakrishnan, A., Patel, V., et al. (2022). Sex-specific role for SLIT1 in regulating stress susceptibility. Biol. Psychiatry 91, 81–91. doi: 10.1016/j.biopsych.2021.01.019
Varadarajan, S. G., Kong, J. H., Phan, K. D., Kao, T.-J., Panaitof, S. C., Cardin, J., et al. (2017). Netrin1 Produced by neural progenitors, not floor plate cells, is required for axon guidance in the spinal cord. Neuron 94, 790–799.e3. doi: 10.1016/j.neuron.2017.03.007
Varea, E., Castillo-Gómez, E., Gómez-Climent, M. A., Blasco-Ibáñez, J. M., Crespo, C., Martínez-Guijarro, F. J., et al. (2007). Chronic antidepressant treatment induces contrasting patterns of synaptophysin and PSA-NCAM expression in different regions of the adult rat telencephalon. Eur. Neuropsychopharmacol. 17, 546–557. doi: 10.1016/j.euroneuro.2007.01.001
Varea, E., Nácher, J., Blasco-Ibáñez, J. M., Gómez-Climent, M. Á, Castillo-Gómez, E., Crespo, C., et al. (2005). PSA-NCAM expression in the rat medial prefrontal cortex. Neuroscience 136, 435–443. doi: 10.1016/j.neuroscience.2005.08.009
Vassilev, P., Pantoja-Urban, A. H., Giroux, M., Nouel, D., Hernandez, G., Orsini, T., et al. (2021). Unique effects of social defeat stress in adolescent male mice on the Netrin-1/DCC pathway, prefrontal cortex dopamine and cognition. Eneuro 8. doi: 10.1523/eneuro.0045-21.2021
Vialou, V., Bagot, R. C., Cahill, M. E., Ferguson, D., Robison, A. J., Dietz, D. M., et al. (2014). Prefrontal cortical circuit for depression- and anxiety-related behaviors mediated by cholecystokinin: Role of ΔFosB. J. Neurosci. 34, 3878–3887. doi: 10.1523/jneurosci.1787-13.2014
Voleti, B., and Duman, R. S. (2012). The roles of neurotrophic factor and wnt signaling in depression. Clin. Pharmacol. Ther. 91, 333–338. doi: 10.1038/clpt.2011.296
Vosberg, D. E., Leyton, M., and Flores, C. (2020). The Netrin-1/DCC guidance system: Dopamine pathway maturation and psychiatric disorders emerging in adolescence. Mol. Psychiatry 25, 297–307. doi: 10.1038/s41380-019-0561-7
Wainwright, S. R., and Galea, L. A. M. (2013). The neural plasticity theory of depression: Assessing the roles of adult neurogenesis and PSA-NCAM within the hippocampus. Neural Plast. 2013:805497. doi: 10.1155/2013/805497
Wainwright, S. R., Barha, C. K., Hamson, D. K., Epp, J. R., Chow, C., Lieblich, S. E., et al. (2016). Enzymatic depletion of the polysialic acid moiety associated with the neural cell adhesion molecule inhibits antidepressant efficacy. Neuropsychopharmacology 41, 1670–1680. doi: 10.1038/npp.2015.337
Wang, J. X., Kurth-Nelson, Z., Kumaran, D., Tirumala, D., Soyer, H., Leibo, J. Z., et al. (2018). Prefrontal cortex as a meta-reinforcement learning system. Nat. Neurosci. 21, 860–868. doi: 10.1038/s41593-018-0147-8
Wang, Q., Timberlake, M. A., Prall, K., and Dwivedi, Y. (2017). The recent progress in animal models of depression. Prog. Neuro Psychopharmacol. Biol. Psychiatry 77, 99–109. doi: 10.1016/j.pnpbp.2017.04.008
Wellman, C. L., and Moench, K. M. (2019). Preclinical studies of stress, extinction, and prefrontal cortex: Intriguing leads and pressing questions. Psychopharmacology 236, 59–72. doi: 10.1007/s00213-018-5023-4
Williams, A. V., Peña, C. J., Ramos-Maciel, S., Laman-Maharg, A., Ordoñez-Sanchez, E., Britton, M., et al. (2022). Comparative transcriptional analyses in the nucleus accumbens identifies RGS2 as a key mediator of depression-related behavior. Biol. Psychiatry 92, 942–951. doi: 10.1016/j.biopsych.2022.06.030
Wise, S. P. (2008). Forward frontal fields: Phylogeny and fundamental function. Trends Neurosci. 31, 599–608. doi: 10.1016/j.tins.2008.08.008
Wise, S. P., Murray, E. A., and Gerfen, C. R. (1996). The frontal cortex-basal ganglia system in primates. Crit. Rev. Neurobiol. 10, 317–356. doi: 10.1615/critrevneurobiol.v10.i3-4.30
Woo, E., Sansing, L. H., Arnsten, A. F. T., and Datta, D. (2021). Chronic stress weakens connectivity in the prefrontal cortex: Architectural and molecular changes. Chron. Stress 5:24705470211029256. doi: 10.1177/24705470211029254
Xu, R., Feyeux, M., Julien, S., Nemes, C., Albrechtsen, M., Dinnyés, A., et al. (2014). Screening of bioactive peptides using an embryonic stem cell-based neurodifferentiation assay. AAPS J. 16, 400–412. doi: 10.1208/s12248-014-9578-7
Yamagishi, S., Bando, Y., and Sato, K. (2021). Involvement of netrins and their receptors in neuronal migration in the cerebral cortex. Front. Cell Dev. Biol. 8:590009. doi: 10.3389/fcell.2020.590009
Yan, Z., and Rein, B. (2022). Mechanisms of synaptic transmission dysregulation in the prefrontal cortex: Pathophysiological implications. Mol. Psychiatry 27, 445–465. doi: 10.1038/s41380-021-01092-3
Yao, P. J., Petralia, R. S., and Mattson, M. P. (2016). Sonic hedgehog signaling and hippocampal neuroplasticity. Trends Neurosci. 39, 840–850. doi: 10.1016/j.tins.2016.10.001
Yohn, C. N., Dieterich, A., Bazer, A. S., Maita, I., Giedraitis, M., and Samuels, B. A. (2019). Chronic non-discriminatory social defeat is an effective chronic stress paradigm for both male and female mice. Neuropsychopharmacology 44, 2220–2229. doi: 10.1038/s41386-019-0520-7
Ypsilanti, A. R., Zagar, Y., and Chédotal, A. (2010). Moving away from the midline: New developments for Slit and Robo. Development 137, 1939–1952. doi: 10.1242/dev.044511
Yuan, N., Tang, K., Da, X., Gan, H., He, L., Li, X., et al. (2021). Integrating clinical and genomic analyses of hippocampal-prefrontal circuit disorder in depression. Front. Genet. 11:565749. doi: 10.3389/fgene.2020.565749
Zhang, J., Yao, W., Qu, Y., Nakamura, M., Dong, C., Yang, C., et al. (2017). Increased EphA4-ephexin1 signaling in the medial prefrontal cortex plays a role in depression-like phenotype. Sci. Rep. 7:7133. doi: 10.1038/s41598-017-07325-2
Zhang, R.-X., Han, Y., Chen, C., Xu, L.-Z., Li, J.-L., Chen, N., et al. (2016). EphB2 in the medial prefrontal cortex regulates vulnerability to stress. Neuropsychopharmacology 41, 2541–2556. doi: 10.1038/npp.2016.58
Keywords: Netrin-1, social defeat, post-mortem, MDD, ephrin, slit, DCC, semaphorin
Citation: Mahmud A, Avramescu RG, Niu Z and Flores C (2023) Awakening the dormant: Role of axonal guidance cues in stress-induced reorganization of the adult prefrontal cortex leading to depression-like behavior. Front. Neural Circuits 17:1113023. doi: 10.3389/fncir.2023.1113023
Received: 30 November 2022; Accepted: 09 March 2023;
Published: 24 March 2023.
Edited by:
Jacob Raber, Oregon Health & Science University, United StatesReviewed by:
Cortney Ann Turner, University of Michigan, United StatesCopyright © 2023 Mahmud, Avramescu, Niu and Flores. This is an open-access article distributed under the terms of the Creative Commons Attribution License (CC BY). The use, distribution or reproduction in other forums is permitted, provided the original author(s) and the copyright owner(s) are credited and that the original publication in this journal is cited, in accordance with accepted academic practice. No use, distribution or reproduction is permitted which does not comply with these terms.
*Correspondence: Cecilia Flores, Y2VjaWxpYS5mbG9yZXNAbWNnaWxsLmNh
†These authors have contributed equally to this work and share first authorship
Disclaimer: All claims expressed in this article are solely those of the authors and do not necessarily represent those of their affiliated organizations, or those of the publisher, the editors and the reviewers. Any product that may be evaluated in this article or claim that may be made by its manufacturer is not guaranteed or endorsed by the publisher.
Research integrity at Frontiers
Learn more about the work of our research integrity team to safeguard the quality of each article we publish.