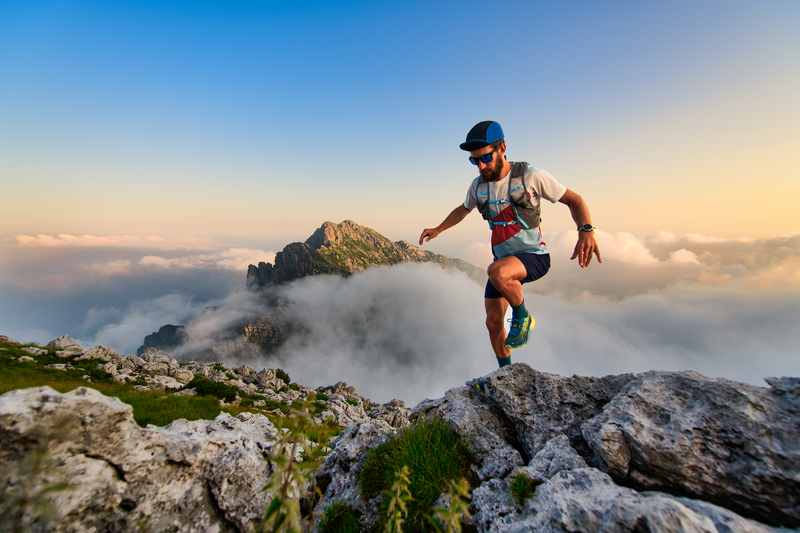
95% of researchers rate our articles as excellent or good
Learn more about the work of our research integrity team to safeguard the quality of each article we publish.
Find out more
MINI REVIEW article
Front. Neural Circuits , 01 November 2022
Volume 16 - 2022 | https://doi.org/10.3389/fncir.2022.939235
This article is part of the Research Topic Prefrontal and Neuromodulatory Circuits in Cognitive Function and Dysfunction View all 5 articles
The prefrontal cortex plays a central role in the control of complex cognitive processes including action control and decision making. It also shows a specific pattern of delayed maturation related to unique behavioral changes during adolescence and allows the development of adult cognitive processes. The adolescent brain is extremely plastic and critically vulnerable to external insults. Related to this vulnerability, adolescence is also associated with the emergence of numerous neuropsychiatric disorders involving alterations of prefrontal functions. Within prefrontal microcircuits, the dopamine and the endocannabinoid systems have widespread effects on adolescent-specific ontogenetic processes. In this review, we highlight recent advances in our understanding of the maturation of the dopamine system and the endocannabinoid system in the prefrontal cortex during adolescence. We discuss how they interact with GABA and glutamate neurons to modulate prefrontal circuits and how they can be altered by different environmental events leading to long-term neurobiological and behavioral changes at adulthood. Finally, we aim to identify several future research directions to help highlight gaps in our current knowledge on the maturation of these microcircuits.
Successful adaptation to a changing environment requires a complex set of cognitive processes known as executive functions including working memory, attentional processes, behavioral flexibility and decision-making (Rangel et al., 2008). The prefrontal cortex (PFC) plays a key role in regulating these processes by integrating multiple signals and controlling the activity of subcortical circuits (Fuster, 2001; Miller and Cohen, 2001). Altered prefrontal functioning is associated with numerous pathological states including substance use disorders, schizophrenia, eating disorders and obsessive compulsive disorders (Paulus, 2007).
In primates including humans the PFC has been historically defined as the part of the cortex that receives projections from the mediodorsal thalamic nucleus (Rose and Woolsey, 1948). In other mammals, especially rodents, the existence of the PFC and its homology to primate PFC are still debated but it is typically defined as the medial prefrontal cortex (mPFC) and the orbitofrontal cortex (see Uylings et al., 2003; Carlén, 2017; Laubach et al., 2018; Kolk and Rakic, 2022 for a complete review). Prefrontal functions are supported by local microcircuits involving long-range glutamatergic pyramidal neurons and diverse populations of GABAergic interneurons (Seamans and Yang, 2004; Lewis and González-Burgos, 2008). Importantly, prefrontal functions are highly dependent on the influence of neuromodulatory inputs (dopamine, serotonin, acetylcholine, and noradrenaline; Hoover and Vertes, 2007) as well as local endocannabinoid signaling (McLaughlin et al., 2014).
Adolescence is an important developmental period between childhood and adulthood characterized by major behavioral and cognitive changes including increased social interactions, novelty-seeking and risk-taking (Spear, 2000; Ernst et al., 2006; Casey et al., 2008; Walker et al., 2017). At the neurobiological level, adolescence is associated with neurophysiological changes, an increase in white matter volume and inverted U-shaped changes in gray matter volume related to synaptic overproduction and subsequent pruning (Lenroot and Giedd, 2006; Caballero et al., 2016; Drzewiecki and Juraska, 2020; Kolk and Rakic, 2022). These changes are especially marked in the PFC, which may participate to the development of adult-like independent behavior (Ernst et al., 2006; Somerville and Casey, 2010). However, adolescence is also associated with the onset of several major neuropsychiatric disorders (Paus et al., 2008) related with prefrontal dysfunction suggesting that prefrontal circuits may be especially vulnerable to external insults during this stage. Thus, there is a growing interest in gaining a better understanding of the maturation processes of prefrontal microcircuits and how their perturbation may lead to long-lasting neurobiological and cognitive alterations.
In this review, we focus on two important elements of prefrontal circuits: the mesocortical dopamine system and the endocannabinoid system. We outline the unique developmental profiles of these systems during adolescence, how they play essential roles in prefrontal physiology, how they interact to control prefrontal functions and how their alteration can lead to long-term changes at adulthood. Finally, we identify future research directions to highlight gaps in our current knowledge on the maturation of these microcircuits.
Adolescence as previously defined is observed in humans but also conserved in other mammals, including rodents (mice and rats) which are commonly used as animal models in neuroscience research (Figure 1A). The exact boundaries of adolescence remain difficult to define. In humans, this is commonly defined as spanning between 12 and 18 years of age. However, these boundaries are not concrete and have been expanded in some studies which report behavioral changes from as early as the age of 10 with the end of adolescent brain maturation occurring as late as the mid-20's (Steinberg, 2005; Ernst et al., 2006; Casey et al., 2008; Blakemore, 2012; Blakemore and Robbins, 2012). Neurocognitive and neurobiological comparisons between primates and rodents have been a somewhat controversial topic in recent years. In one of the most influential reviews on this topic, Spear initially defined adolescence in rodents between post-natal day (PND) 28 and 42 based on the neurobehavioral characteristics reported, however this paper highlighted this may be too restricted (Spear, 2000). Later authors have used diverse age windows to define adolescence and peri-adolescence, based on additional behavioral and neurobiological criteria. Some authors consider rodent adolescence to begin immediately after weaning on PND21 (Schneider, 2008, 2013; Yuan et al., 2015) and to extend all the way until adulthood (PND60-70; Andersen, 2003; McCutcheon and Marinelli, 2009; Brenhouse and Andersen, 2011; Walker et al., 2017). More recently and based on changes in prefrontal anatomy, Caballero and Tseng have classified adolescent periods of development and vulnerability into early (PND35-40), mid (PND40-50), and late (PND50-60) adolescence (Caballero et al., 2016). In the rest of the manuscript, we will use the term adolescence to refer to the broad period between weaning (PND21) and adulthood (PND60-70) in rodents.
Figure 1. (A) Timing of adolescence in humans (top) and rodents (bottom). Adolescence and peri-adolescence are defined as beginning around the age 10 years and continuing until the mid-20's in humans and from post-natal day (PND) 21 to PND 50-60 in mice and rats. (B) Maturation of prefrontal dopamine (DA) and endocannabinoid systems across development. Schematic shows density of prefrontal anandamide (purple), dopamine (DA) tissue concentration (orange), and DA fibers (black) steadily increase from childhood to adulthood. Meanwhile, both CB1 (blue) and dopamine receptors (green) present a transient peak of expression in the prefrontal cortex during early adolescence, paralleled with similar changes in the activity of dopamine neurons in the VTA (red). Finally 2-AG levels (cyan) transiently decreases compared to childhood before returning to stable adult levels.
An important point to note is that although puberty is a part of adolescence, it does not itself fully define adolescence. Puberty is defined as sexual maturity resulting from changes in the secretion of gonadal hormones. In humans the onset of puberty (~10 years old) is often considered as the onset of adolescence, but this can vary depending on sex, gender, ethnicity and socioeconomical status. In rodents, these two phenomena are clearly distinct between sexes. In females, vaginal opening and first ovulation occur early during adolescence (PND30-40), whereas in males increased testosterone and preputial separation occur during mid adolescence (PND40-45; Schneider, 2008; Delevich et al., 2021). Puberty likely contributes to the development of prefrontal anatomy and functions which we will briefly discuss later.
The dopamine system is a central actor in the modulation of behavioral control and conveys various signals related to prediction error, motivation and event salience (Floresco and Magyar, 2006; Berridge, 2007; Schultz, 2007; Wickens et al., 2007; Bromberg-Martin et al., 2010; Berke, 2018). It presents a uniquely late development during postnatal life especially within prefrontal regions, which appears to be fundamental for normal cognitive development (Spear, 2000; Ernst et al., 2006; Somerville and Casey, 2010; Sturman and Moghaddam, 2011; Walker et al., 2017).
Dopaminergic cells mainly located in the midbrain differentially innervate the dorsal striatum (nigrostriatal pathway), the nucleus accumbens (mesolimbic pathway), and prefrontal regions (mesocortical pathway) which we will focus on in the present review (for recent reviews on the anatomical organization of dopamine pathways see Björklund and Dunnett, 2007; Lammel et al., 2014; Morales and Margolis, 2017). The mesocortical pathway originates from the medial part of the ventral tegmental area (VTA) and is comprised of dopamine neurons (30–40%) but also an important fraction of GABAergic and glutamate neurons (Morales and Margolis, 2017). In primates, dopamine neurons innervate prefrontal and sensorimotor cortical regions whereas in rodents this is limited to the mPFC, the agranular insular and the perirhinal cortices (Berger et al., 1991). Dopamine innervation is stronger in ventral parts of the mPFC (prelimbic cortex or Brodmann area 32 / infralimbic cortex or Brodmann area 25; Laubach et al., 2018), and is especially observed in deep cortical layers (layers V–VI; Van Eden et al., 1987; Heidbreder and Groenewegen, 2003). In contrast to the mPFC, rodent orbitofrontal regions are weakly innervated by dopamine neurons. Dopamine neurons rarely send collateral projections to other regions, meaning that mesocortical dopamine neurons represent a distinct population compared to mesolimbic neurons (Fallon, 1988; Beier et al., 2015). Dopamine neurons display two characteristic activity patterns: (1) a “tonic” low frequency mode (2–5 Hz) maintaining basal levels of dopamine in downstream targets and (2) a “phasic” mode with short high frequency (10–20 Hz) bursts transiently increasing dopamine release (Grace and Bunney, 1984a,b; Floresco et al., 2003; Lapish et al., 2007; Rice et al., 2011). This burst firing is especially observed in response to relevant environmental stimuli (Schultz, 2007; Bromberg-Martin et al., 2010). The exact functions of these different modes of dopamine signaling are still debated but through its actions at downstream targets and within local microcircuits, dopamine guides, invigorates and updates complex behaviors (Niv, 2007; Schultz, 2007; Bromberg-Martin et al., 2010; Salamone and Correa, 2012; Berke, 2018).
Mesocortical dopamine neurons respond to both appetitive (Hernandez and Hoebel, 1990; Ahn and Phillips, 1999; Bassareo et al., 2002; St Onge et al., 2012; Ellwood et al., 2017) and aversive stimuli (Abercrombie et al., 1989; Mantz et al., 1989; Vander Weele et al., 2018). Moreover, they have unique properties compared to other dopamine projections including low levels of dopamine transporter DAT and D2 autoreceptors associated with higher basal frequency (Sesack et al., 1998; Lammel et al., 2008). These properties result in different kinetics of dopamine signaling compared to subcortical regions (Garris and Wightman, 1994; Cass and Gerhardt, 1995; Vander Weele et al., 2018). Prefrontal neurons also directly regulate other dopamine circuits by exciting VTA mesocortical and mesolimbic neurons (Carr and Sesack, 2000; Heidbreder and Groenewegen, 2003; Gabbott et al., 2005; Beier et al., 2015) and by modulating dopamine release within subcortical regions including the nucleus accumbens (Taber et al., 1995; Karreman and Moghaddam, 1996; Rice et al., 2011; Nolan et al., 2020).
With its unique characteristics, the mesocortical dopamine pathway plays a key role in prefrontal function (Fuster, 2001; Miller and Cohen, 2001). Current theories postulate a “dual-state” function of prefrontal dopamine signaling. Tonic dopamine levels may act to maintain goal representation and adapted behavioral strategies by stabilizing the activity of specific prefrontal neuronal ensembles. Phasic dopamine release occurs in response to relevant stimuli and would gate new afferent signals from cortical and subcortical regions to update goal representations and behavioral strategies (Montague et al., 2004; Seamans and Yang, 2004; Daw et al., 2006; Durstewitz and Seamans, 2008; Ellwood et al., 2017).
In rodents, the first mesencephalic dopamine neurons appear during embryonic life (E12) and immediately start to extend projections to the developing telencephalic regions (Riddle and Pollock, 2003; Van den Heuvel and Pasterkamp, 2008; Islam et al., 2021). Dopamine fibers are detected in the striatum and the nucleus accumbens at E15-E17 (Voorn et al., 1988). The nigrostriatal and mesolimbic dopamine pathways end their development during the first postnatal week (Kalsbeek et al., 1988; Voorn et al., 1988). Dopamine fibers appear in the mPFC shortly after from E19 and continue to develop during postnatal life (Van Eden et al., 1987; Kalsbeek et al., 1988). In contrast to other neuromodulatory systems in the mPFC, such as noradrenaline or serotonin (Levitt and Moore, 1979; Lidov et al., 1980; Lambe et al., 2000; Naneix et al., 2012), the mesocortical dopamine pathway has a uniquely protracted maturation (Figure 1B). The density of dopamine fibers continues to increase until adulthood in rodents and primates in all mPFC subregions (Kalsbeek et al., 1988; Rosenberg and Lewis, 1994; Benes et al., 1996; Naneix et al., 2012; Hoops and Flores, 2017; Willing et al., 2017; Reynolds and Flores, 2021). This late development parallels increased basal dopamine levels in the mPFC during adolescence and decreased levels of dopamine metabolites (Nomura et al., 1976; Naneix et al., 2012) suggesting a broad increase in dopamine availability and action in prefrontal regions from childhood to adulthood. This is associated with an increase in the proportion of prefrontal cells, especially GABA neurons, in contact with dopamine fibers but also in the absolute number of dopamine varicosities per neuron (Benes et al., 1996), suggesting an increased dopaminergic modulation of these cells.
The continuous growth of dopamine fibers in prefrontal regions likely involves a combination of continued long-range axon growth from the VTA and increased sprouting from dopamine axons already present in the mPFC. There is now considerable evidence that these processes are under the control of several extracellular guidance cues including ephrins, semaphorins, netrins or slits during embryonic and postnatal stages (Van den Heuvel and Pasterkamp, 2008; Brignani and Pasterkamp, 2017; Hoops and Flores, 2017; Islam et al., 2021). The development of the mesocortical dopamine pathway during adolescence seems to be especially dependent of the Netrin-1/DCC (Hoops and Flores, 2017). Dopamine neurons express high levels of the Netrin-1 receptor during embryonic and early post-natal life (pre-weaning) which decrease toward adulthood (Manitt et al., 2010). A proportion of dopamine axons innervating the mPFC at adulthood are still growing through the nucleus accumbens during adolescence (Reynolds et al., 2015, 2018; Reynolds and Flores, 2021). This process is under the control of the Netrin-1 receptor and DCC. Reduced DCC expression in dopamine neurons increases dopamine innervation and dopamine levels in the mPFC (Manitt et al., 2011, 2013; Reynolds et al., 2018). These results suggest that high DCC-expressing dopamine neurons are attracted by brain regions expressing low levels of Netrin-1 such as the nucleus accumbens thus forming the mesolimbic pathway early in development (Manitt et al., 2011). In contrast, prefrontal neurons express high levels of Netrin-1 which attract a specific population of dopamine neurons expressing low levels of DCC during adolescence (Flores et al., 2005; Grant et al., 2007, 2009; Manitt et al., 2011; Reynolds et al., 2018). In addition to guidance cues the refinement of the dopamine system during adolescence may be under the influence of glial cells which contribute to synaptic development and pruning (for review see Béchade et al., 2013; Kettenmann et al., 2013; Brenhouse and Schwarz, 2016). Recent findings show that the downregulation of dopamine receptors in the nucleus accumbens during early adolescence is dependent of microglial activation, especially in males (Kopec et al., 2018). A similar mechanism within the mPFC remains to be demonstrated but microglia cells are already known to regulate the pruning of mPFC glutamatergic synapses during adolescence (Mallya et al., 2019; Blagburn-Blanco et al., 2022).
In addition to the growth of dopamine fibers the activity of dopamine cells also undergoes important changes during adolescence. In vivo and ex vivo recordings have revealed that VTA dopamine neurons in rats have higher tonic firing rates during adolescence compared to adulthood, peaking during late adolescence (PND40-50). In addition, although adolescent rats have a similar proportion of phasic activity episodes, these are consistently longer compared to those of adults (McCutcheon and Marinelli, 2009; McCutcheon et al., 2012; Marinelli and McCutcheon, 2014; but see Kim et al., 2016; McCane et al., 2021). It is currently unknown whether these age-related changes are specific to different defined neuronal populations or pathways. However, in vivo study in mice has demonstrated that the increase in dopamine neuron activity during adolescence may participate specifically to the development of the mesocortical pathway as the phasic activation of dopamine neurons in adolescents, but not in adults, promotes the formation of new synaptic boutons on mesocortical neurons (Mastwal et al., 2014). This is consistent with the role of dopamine in synaptogenesis also demonstrated in vitro (Parish et al., 2001; Fasano et al., 2008, 2010).
At the postsynaptic level, both PFC pyramidal glutamate neurons and GABA interneurons express dopamine D1-like (D1, D5) and D2-like (D2, D3, D4) receptors (Berger et al., 1991; Gaspar et al., 1995; Le Moine and Gaspar, 1998). Dopamine receptor expression during adolescence seems to present a complex reorganization in the cortex. However, data exploring this has produced somewhat conflicting and inconclusive results. For example, autoradiography studies in rats have shown either a steady increase in the expression of D1- and D2-like receptors (Tarazi and Baldessarini, 2000) or a binding peak at weaning followed by a decrease afterwards (Leslie et al., 1991). However immunohistochemistry (Andersen et al., 2000; Brenhouse et al., 2008; Brenhouse and Andersen, 2011) or qPCR studies (Naneix et al., 2012) have shown that the expression of dopamine receptors peaks during mid-adolescence, suggesting a short window of overexpression followed by pruning of dopamine synapses during late adolescence/early adulthood which may be related to the increased activity of dopamine neurons during the same period (McCutcheon and Marinelli, 2009; McCutcheon et al., 2012; Figure 1B). Recent advances in single cell RNA sequencing may clarify this picture by potentially showing different patterns of maturation within mPFC neuronal populations (Bhattacherjee et al., 2019; Tiklová et al., 2019).
Together with the dopamine system, the endocannabinoid (eCB) system is another central neuromodulator of executive functions (Egerton et al., 2006; Pattij et al., 2008) in adults and shares a similar late maturation during adolescence with the dopamine system (Caballero et al., 2016). In the following sections we will discuss how endocannabinoids and their endogenous receptors change across adolescence and how this influences the maturation of the PFC.
The two main endocannabinoids are 2-arachidonoylglycerol (2-AG) and N-arachidonoylethanolamine (AEA or anandamide; Mechoulam et al., 1995; Sugiura et al., 1995). They both bind to two key G-protein coupled receptors (GPCRs): cannabinoid receptor 1 (CB1)—expressed throughout the central nervous system (Herkenham et al., 1990; Mechoulam et al., 1995)—and cannabinoid receptor 2 (CB2) expressed predominantly in immune cells but also found in some neurons and glia (Atwood and Mackie, 2010; Zhang et al., 2014). We will focus here on the CB1 receptor due to its ubiquitous expression throughout the brain including the prefrontal cortex (Herkenham et al., 1990; Marsicano and Lutz, 1999) and its role in PFC development.
CB1 receptors are coupled to inhibitory G-proteins (Gi/o proteins) and their activation reduces cAMP levels, decreasing cAMP-dependent protein kinase activity (Howlett and Mukhopadhyay, 2000; Howlett, 2002). This induces the activation of A-type potassium channels, the inhibition of voltage-gated calcium channels and the disruption of neurotransmitter vesicles (Lovinger, 2008), which decreases the probability of neurotransmitter release. Unlike classical modulatory neurotransmitters which are stored in vesicles, endocannabinoids are synthesized and released de novo in times of sustained neuronal activity (Howlett and Mukhopadhyay, 2000; Freund et al., 2003) acting on presynaptic terminals (Wilson and Nicoll, 2001; Kreitzer and Regehr, 2002). This retrograde action allows neurons to filter and select afferent inputs via negative feedback. The principle psychoactive ingredient in cannabis, Δ9- tetrahydrocannabinol (THC), is a potent CB1 agonist (Gaoni and Mechoulam, 1964; Huestis et al., 2001).
Both 2-AG and AEA are derived from poly-unsaturated fatty acids but have distinct synthesis and metabolic pathways (Ueda et al., 2010; Ueda and Tsuboi, 2012). 2-AG is a major product of the phospholipase Cβ - diacylglycerol lipase pathway synthesized post-synaptically following sustained depolarization and by the subsequent increased calcium influx following activation of voltage-gated Ca2+ channels (Kano et al., 2009). 2-AG is metabolized by monoacylglycerol lipase (MAGL) localized mainly with pre-synaptic CB1 receptors (Häring et al., 2012). AEA synthesis on the other hand is a relatively minor biproduct of fatty acylethanolamine (N-acylethanolamines) species production triggered by increased intracellular calcium or cAMP levels in postsynaptic neurons (Bara et al., 2021). AEA is metabolized by fatty acid amide hydrolase (FAAH) localized post-synaptically to provide feedback inhibition (Häring et al., 2012).
Beyond their different synthetic and metabolic pathways, 2-AG and AEA also differ in terms of their pharmacological and functional actions via CB1 receptors. Pharmacologically 2-AG is a full agonist of the CB1 receptor, whereas AEA is a partial agonist (Sugiura et al., 1995, 2006). Despite binding to CB1 receptors with high affinity, AEA induces relatively poor intracellular signal transduction (Hillard et al., 1995). The slower time course of AEA's action makes it a good candidate for a tonic signal (Hill and Tasker, 2012) and thus AEA is implicated in long-term plasticity (Mackie, 2006). 2-AG on the other hand has a relatively low binding affinity for CB1 receptors but produces a much more robust intracellular response (Hillard et al., 1995). These dynamics combined with 2-AG release following sustained depolarization show a more characteristically phasic signal seen in shorter, activity-dependent synaptic plasticity (Lu and Mackie, 2016). In addition, 2-AG levels are generally several orders of magnitude higher than AEA levels in rodent brain tissue (Bisogno et al., 1999; Ueda and Tsuboi, 2012) which may be related to their different synthesis pathways.
Whilst we focus this review on the function and dysfunction of the eCB system in shaping the PFC through actions at CB1 receptors, it is important to consider that endocannabinoids also interact with other receptors and systems. Whilst predominantly expressed in peripheral tissues CB2 receptors are expressed in some microglia within the brain and their activation may have important effects we do not fully understand (Cabral et al., 2008). In addition, eCBs can activate or facilitate the activation of other receptors. For example, AEA is a TRPV1 receptor agonist and 2-AG can potentiate GABA-A receptors independently of CB1 receptor effects (Piscitelli and Di Marzo, 2012). In fact, eCB signaling has been shown to engage as many as 12 different receptors and involve many biosynthetic and degradative enzymes, transporters and other proteins diversifying the ways eCB signaling may impact local brain circuits (for a comprehensive discussion of the complex interactions between endocannabinoids and other receptors, transporters, and enzymes see Maccarrone, 2020).
The CB1 receptor is the most abundant GPCR in the brain and the eCB system is uniquely positioned to have large scale influence on developing brain circuits. Our current understanding of the functioning and role the eCB system is still relatively new but we know this system is fundamentally important in all phases of brain development especially in controlling long-range axonal guidance and patterning of synaptic contacts (Berghuis et al., 2007; Harkany et al., 2007, 2008; Maccarrone et al., 2014; Bara et al., 2021). In cortical regions CB1 receptors are expressed at higher levels in GABAergic interneurons than in glutamatergic neurons with a higher expression in the PFC compared to motor and sensory cortices (Marsicano and Lutz, 1999). PFC CB1 expression in rodents peaks to its maximal density in early adolescence (Meyer et al., 2018), and then declines into adulthood (Figure 1B; Berrendero et al., 1999; Ellgren et al., 2008; Heng et al., 2011a; Peters et al., 2021c).
During adolescence 2AG and AEA levels also fluctuate. Within the PFC, AEA steadily increases from early to late adolescence, with concentrations reaching three times higher levels in late versus early adolescence before reaching stable levels into adulthood (Ellgren et al., 2008; Tirado-Muñoz et al., 2020). PFC 2-AG levels markedly decrease from early to late adolescence before stabilizing in adulthood (Ellgren et al., 2008; Rubino et al., 2015; Bara et al., 2021). These changes are accompanied by complementary changes in the activity of FAAH and MAGL (Long et al., 2012). Increased FAAH levels in adolescence may reflect in particular the tighter regulation of AEA during this key developmental period (Long et al., 2012).
Changes in the anatomical organization of the endocannabinoid system during adolescence are associated with important functional changes in synaptic plasticity which may also play an important role in long-range axonal pathfinding and patterning of synaptic contacts, both essential processes for prefrontal development (Berghuis et al., 2007; Maccarrone et al., 2014).
There are important sex differences in cortical development in humans, most prominent is the faster maturation of the cortex in females compared to males (Mutlu et al., 2013) as well as early differences in functional connectivity and sex specific age-related changes in resting state activity (Zuo et al., 2010). Similar sex differences are also reported in subcortical regions. Striatal development also occurs faster in females with striatal volume stabilizing around early adolescence, whereas in males this continues to develop until early adulthood (Raznahan et al., 2014; Hammerslag and Gulley, 2016). In rodents, key sex differences have been also reported in the development of the prefrontal cortex (Drzewiecki and Juraska, 2020). Sex and the onset of puberty, with changes in gonadal hormones during adolescence, may be critical factors in the late maturation of dopamine and endocannabinoid systems (Becker and Chartoff, 2019; Zachry et al., 2021). However, until recently most of the data from animal models has come from studies in males.
In the dopamine system there are crucial anatomical and functional sex differences in adulthood including a higher proportion of dopamine neurons in the VTA of female rodents and variation of striatal dopamine concentration with the estrous cycle through the expression of estrogen receptors in both dopamine and striatal GABAergic striatal neurons (for a recent complete review see Zachry et al., 2021). Within the mesocortical pathway in particular, female rats exhibit a higher proportion of dopamine neurons (tyrosine hydroxylase expressing cells) compared to males (>50 vs. 30%; Kritzer and Creutz, 2008). Estrogen receptors are also expressed at both presynaptic and postsynaptic level in the prefrontal cortex (Almey et al., 2015) and in different proportion between males and females (Kritzer and Creutz, 2008) but sex- and estrous cycle-related modulation of dopamine signaling in this region remains poorly understood. So far, studies have found no substantial sex differences in the time course of the development of mesocortical DA innervation (Willing et al., 2017). However, gonadectomy of male rats at adulthood or during the perinatal period alters the organization of the mesocortical dopamine pathway (Kritzer, 1998; Kritzer et al., 1999), suggesting there is a role of puberty in this maturation. Furthermore, there are clear age- and sex-dependent differences in subcortical dopamine release and reuptake in rats in adolescence and adulthood (Pitts et al., 2020). These differences remain to be investigated in the prefrontal cortex.
Consistent with the general earlier maturation of the female brain the increases in CB1 receptor density seen in adolescence begin earlier in female rodents compared to males (de Fonseca et al., 1993). The expression of CB1 receptors also varies across the estrous cycle in female mice and rats, with CB1 mRNA transcripts highest in diestrus and lowest on estrus (González et al., 2000). Levels of AEA are generally higher in female rats and again these fluctuate with the estrous cycle. In contrast, 2-AG does not seem to differ between males and females or as a function of the estrous cycle. In addition, eCB signaling can modulate gonadal hormones in both humans and rodents (Meyer et al., 2018). The relationship between puberty, sex hormones, neuromodulators and the maturation of brain functions during adolescence remains poorly understood and certainly requires a more in-depth investigation (Shansky and Murphy, 2021).
Prefrontal circuits play a central role in action control including working memory processes (Goldman-Rakic et al., 2000; Seamans and Yang, 2004), goal-directed behaviors (Balleine and O'Doherty, 2010; Coutureau and Parkes, 2018; Turner and Parkes, 2020), response inhibition (Winstanley et al., 2006; Turner and Parkes, 2020), and behavioral flexibility (Floresco and Magyar, 2006). In adults, prefrontal dopamine (Floresco and Magyar, 2006; Winstanley et al., 2006) and endocannabinoid (Egerton et al., 2006; Pattij et al., 2008; McLaughlin et al., 2014; Gremel et al., 2016) signaling are important modulators of these executive functions. Several studies have reported alterations of these processes in adolescent rodents including adaptation to action-outcome contingency changes (Naneix et al., 2012, 2013), behavioral inhibition (Andrzejewski et al., 2011), attentional control (Burton and Fletcher, 2012), impulsivity (Doremus-Fitzwater et al., 2012), or behavioral extinction (Sturman et al., 2010). Conversely, adolescent rodents exhibit an increased sensitivity to rewards (Wilmouth and Spear, 2009; Friemel et al., 2010; Doremus-Fitzwater et al., 2012), environmental stimuli driving reward-seeking responses and motivated behaviors (Burton et al., 2011; Marshall et al., 2020), and impulsivity (Adriani and Laviola, 2003). These behavioral changes appear to be essential to the transition from childhood to adulthood by increasing exploration and novelty seeking but may also drive risk-taking behaviors.
Prefrontal functions and physiology are the results of a complex balance between excitatory (glutamatergic) and inhibitory (GABAergic) transmission regulated by different neuromodulation system including the dopamine and endocannabinoid systems (O'Donnell, 2011; Caballero et al., 2016; Figure 2). In the following sections, we will focus on how developmental changes in prefrontal dopamine and eCB systems represent an important mechanism in the emergence of PFC functions by increasing both the stability of PFC microcircuits and the timed filtering of subcortical inputs essential to either maintain or switch behavioral strategies adapted to changing environments (Simon and Moghaddam, 2014; Reichelt, 2016).
Figure 2. Adolescent changes in the modulation of prefrontal circuits by dopamine and endocannabinoids. (1) During adolescence, dopamine (DA, orange) increases the excitability of prefrontal GABAergic interneurons (blue) solely through D1 actions. At adulthood, dopamine facilitates interneurons excitability through both D1 and D2 receptors, which may change GABAergic control of pyramidal neuron (PN) activity. (2) Dopamine actions through D1 receptors enhance glutamatergic NMDA signaling on glutamate (Glu, pink and orange) neurons. This effect is more robust during late adolescence/adulthood, allowing the emergence of persistent depolarizations of pyramidal neurons (PN, orange). (3) eCB retrograde control (green) of glutamate transmission decreases between adolescence and adulthood, likely due to changes in expression levels of CB1 receptors. (4) At adulthood, the combined action of dopamine on D2 receptors and eCB signaling control the activity of plasticity of GABAergic synapses on PN. Changes in this process during adolescence remain to be demonstrated. (5) PN controls the activity of subcortical circuits. Within the VTA for instance, both PN glutamate transmission and local GABAergic transmission control the activity of DA neurons and DA release. Developmental changes in eCB signaling through the expression of CB1 receptors on PN and GABAergic neurons may change this regulation.
Both dopamine and endocannabinoids modulate the activity of pyramidal glutamate neurons and prefrontal glutamatergic plasticity. In adults, dopamine's action on prefrontal circuits is highly dependent of the excitability state of pyramidal neurons and interneurons. Low dopamine levels maintain prefrontal networks, mainly through actions on GABAergic interneurons and D2 receptors. Increases in dopamine levels during phasic activity recruits D1 receptors (Montague et al., 2004; Seamans and Yang, 2004; Durstewitz and Seamans, 2008) required for prefrontal functions such as executive functions (Floresco and Magyar, 2006; Floresco and Jentsch, 2011) and appetitive learning (Baldwin et al., 2002). Dopamine D1 signaling interacts with glutamatergic N-methyl-D-aspartate (NMDA) receptor signaling through D1-dependent mechanisms to induce long-lasting plateau depolarizations (up-state) of pyramidal cells (Tseng and O'Donnell, 2005). Similarly, CB1-dependent eCB signaling regulates prefrontal glutamatergic activity through depolarization-induced suppression of excitation process (Auclair et al., 2000; Kreitzer and Regehr, 2002; Fortin and Levine, 2007; Lovinger, 2008) and long-term depression in deep cortical layers (V–VI; Lafourcade et al., 2007).
As prefrontal D1 expression levels change during adolescence (Leslie et al., 1991; Tarazi and Baldessarini, 2000; Brenhouse et al., 2008; Naneix et al., 2012), ex vivo studies also showed that D1-NMDA interactions emerge during adolescence (Tseng and O'Donnell, 2005; Heng et al., 2011b; Flores-Barrera et al., 2014; Caballero et al., 2016). Despite important anatomical changes on both glutamatergic projections from the amygdala and the hippocampus to the PFC (Cunningham et al., 2002; Johnson et al., 2016; Calabro et al., 2020), only the latter presents a delayed functional maturation during adolescence (Caballero et al., 2014b; Flores-Barrera et al., 2014). Long-term potentiation (LTP) of hippocampal-prefrontal synapses on pyramidal only appear after PND50 in relationship with changes in the expression of GluN2B subunit composition of NMDA receptors. This process parallels the decrease of CB1-related inhibition of glutamatergic transmission (Heng et al., 2011a; Caballero et al., 2016) which may facilitate excitatory transmission from the PFC to subcortical circuits. Interestingly, anatomical studies have shown important changes in D1 expression levels in prefronto-accumbens neurons which may underlie increased incentive salience in response to environmental cues seen in adolescents (Brenhouse et al., 2008; Sonntag et al., 2014).
As for glutamatergic signaling, prefrontal GABAergic transmission is also still undergoing important developmental changes during adolescence. Previous studies demonstrated a progressive increase in the expression levels of calcium binding proteins parvalbumin and a parallel decrease in the expression of calretinin (Caballero et al., 2014a), increasing the proportion of fast-spiking interneurons. This process is dependent on NMDA glutamatergic transmission onto GABAergic interneurons (Flores-Barrera et al., 2014; Caballero et al., 2016; Bogart and O'Donnell, 2018) which may be related to the continuous development of projections from the hippocampus and the amygdala during this period (Cunningham et al., 2002; Johnson et al., 2016; Calabro et al., 2020).
Before adolescence, dopamine facilitates GABAergic transmission by increasing GABA interneurons excitability only through D1 receptor signaling (Gorelova et al., 2003; Tseng et al., 2006). Intriguingly, during late adolescence (PND50), dopamine increases GABA neurons excitability through both D1 and D2 signaling (Tseng et al., 2006; Tseng and O'Donnell, 2007; Caballero et al., 2016). This process is still poorly understood but may involve changes in the expression of D2 receptors and their signaling through β-arrestins which are able to enhance firing of parvalbumin interneurons in the cortex (Urs et al., 2016).
Less is known about the developmental changes of eCB-dependent regulation of GABAergic transmission. In adults, CB1 signaling suppresses GABA release onto glutamatergic cells (Chiu et al., 2010; Hill et al., 2011) and drives depolarization-induced suppression of inhibition at these synapses (Kiritoshi et al., 2013). Moreover, CB1 receptors also colocalize with dopamine D2 receptors on GABA cells, participating in the establishment of long-term depression of GABA synapses (Chiu et al., 2010). It is currently unknown if the changes in prefrontal CB1 receptor expression during adolescence follow the same pattern in glutamatergic and GABAergic neurons (Berrendero et al., 1999; Ellgren et al., 2008; Meyer et al., 2018; Peters et al., 2021c). As for glutamatergic pyramidal neurons, potential changes in CB1 and dopamine modulation of prefrontal GABAergic transmission may contribute to the establishment of excitation/inhibition balance, increasing the selection of specific inputs, decreasing the signal-to-noise ratio and refining prefrontal processing (Montague et al., 2004; Seamans and Yang, 2004; Durstewitz and Seamans, 2008; O'Donnell, 2011; Caballero et al., 2016).
Changes in the prefrontal endocannabinoid system during adolescence may also impact the dopamine system and dopamine signaling in prefrontal subcortical targets, modulating dopamine-related behaviors such as reward prediction error, learning, motivation and event salience (Floresco and Magyar, 2006; Berridge, 2007; Schultz, 2007; Wickens et al., 2007; Bromberg-Martin et al., 2010; Berke, 2018).
In the VTA, dopamine neurons synthesize and release eCBs but do not express CB1 receptors (Julian et al., 2003), VTA eCBs act on local GABA interneurons as well as on GABA and glutamate terminals (Szabo et al., 2002; Melis et al., 2004; Riegel and Lupica, 2004; Wang et al., 2015). This results in the indirect disinhibition of dopamine neurons and the increase of dopamine release in terminal regions including the nucleus accumbens (Cheer et al., 2000, 2007; Oleson et al., 2012; Zlebnik and Cheer, 2016; Covey et al., 2017; Peters et al., 2021a,b) and the prefrontal cortex (Pistis et al., 2002). As in cortical regions, CB1 receptor expression in the VTA increases during early adolescence (PND 40) before decreasing to reach adult levels (de Fonseca et al., 1993) but the neuronal specificity of such changes remains unknown. This may be related to changes in CB1 levels on prefrontal glutamatergic terminals in the VTA controlling the activity of dopamine neurons (Carr and Sesack, 2000; Heidbreder and Groenewegen, 2003; Gabbott et al., 2005; Beier et al., 2015). These complex changes may represent an important mechanism underlying developmental changes in VTA responses to rewarding and aversive environmental stimuli (Kim et al., 2016; McCane et al., 2021) and dopamine-related behaviors (Spear, 2000).
Prefrontal glutamatergic neurons also strongly project to striatal regions (Vertes, 2004; Gabbott et al., 2005) to modulate action control processes (Alexander et al., 1986; Haber, 2003; Voorn et al., 2004). In the nucleus accumbens, cortical glutamatergic terminals control the activity of GABAergic medium spiny neurons (Surmeier et al., 2011) but also modulate local dopamine release through indirect actions on cholinergic interneurons (Cachope et al., 2012; Mateo et al., 2017). In adults, this cortical control of mesolimbic dopamine signal is dependent on CB1 receptors expressed on glutamatergic terminals. Combined with changes in the expression of dopamine D1 receptors by prefronto-accumbens neurons (Brenhouse et al., 2008; Sonntag et al., 2014), changes in CB1 expression in the same neurons may strongly change dopamine release in subcortical regions between adolescents and adults, impacting the control of motivated behaviors. Accordingly, ex vivo and in vivo studies have reported age-related changes in dopamine release in different striatal regions (Matthews et al., 2013; Pitts et al., 2020) and in striatal neuronal responses during instrumental and Pavlovian tasks (Galiñanes et al., 2009; Sturman and Moghaddam, 2012) which may also underlie enhanced reward sensitivity and executive functions deficits during adolescence.
Unfortunately, the late maturation of prefrontal circuits and the associated behavioral changes also creates a window of vulnerability to external insults which may have long-lasting deleterious impact on brain functions. Accordingly, adolescence is the period of life associated with the onset of numerous neuropsychiatric disorders in humans, such as schizophrenia, substance abuse or eating disorders (Paus et al., 2008). As previously stated, adolescents and the adolescent brain are especially sensitive to valence-based stimuli such as drugs of abuse, palatable foods or stressful experience (Spear, 2000; Andersen, 2003; Crews et al., 2007; Reichelt, 2016; Reichelt and Rank, 2017). In this last section, we will focus on two specific examples to illustrate how specific exposure during adolescence may impact prefrontal dopamine and eCB systems and related functions: the effects of cannabis (or CB1 agonists) and the effects of palatable foods.
Cannabis is one of the most commonly used drugs of abuse, especially amongst adolescents (Miech et al., 2020). THC, the principal psychoactive constituent of cannabis, is a potent CB1 agonist (Gaoni and Mechoulam, 1964; Huestis et al., 2001). We do not fully understand how adolescent use in particular impacts the developing brain, however there is emerging evidence that altering the eCB system through such external insults may have important consequences especially at the prefrontal level (Schneider, 2008; Bara et al., 2021). Accordingly, numerous studies in humans and animal models report associations between adolescent cannabis exposure and increased risk of psychiatric conditions which a emerge in adolescence including psychosis, mood disorders and substance use disorders (Laviolette and Grace, 2006; Rubino et al., 2012; Renard et al., 2016).
Chronic CB1 agonist treatment in adolescent animals causes alterations in multiple PFC dependent behaviors, including anxiety (O'Shea et al., 2004), short- and long-term spatial and working memory (Rubino et al., 2009; Renard et al., 2013), motivational and emotional processes (Schneider and Koch, 2003), social behaviors (Schneider et al., 2008), and drug-seeking responses (Ellgren et al., 2007; Higuera-Matas et al., 2008). These alterations are specific to adolescence and are not observed when agonists are administered in adulthood. Importantly, the functioning and vulnerability of the eCB system presents important sex-differences that we do not discuss here (see Ginder et al., 2022 for a complete review of this topic) but which may play a key role in normal and pathological development during adolescence and puberty.
These behavioral phenotypes are associated with profound anatomical and functional alterations of prefrontal circuits (Rubino et al., 2015). These include alterations in mPFC CB1 receptor density and impaired eCB-mediated long-term depression (LTD) of prefrontal excitatory transmission (Rubino et al., 2015; Cuccurazzu et al., 2018). These changes are associated with an increased expression of GluA1 and GluN2B subunits in AMPA and NMDA receptors respectively (Rubino et al., 2015), altering the normal development of PFC glutamatergic transmission (Lovelace et al., 2015; Rubino et al., 2015). Interestingly, these effects are reversed by enhancing AEA levels at adulthood (Cuccurazzu et al., 2018), highlighting the importance of eCB signaling in normative prefrontal development and function.
In addition to its action on prefrontal glutamatergic transmission, there is emerging evidence that prefrontal GABA interneurons may be especially vulnerable to adolescent THC (or synthetic agonist) exposure (Cass et al., 2014; Renard et al., 2018; Peters et al., 2021c). Adolescent, but not adult, exposure to the synthetic CB1 agonist WIN55,212-2 decreases prefrontal GABA signaling resulting in dysregulation of cortical oscillatory activity (Cass et al., 2014; Renard et al., 2017). Conversely, activating prefrontal GABA-A receptors restores behavioral and dopaminergic abnormalities observed following adolescent THC exposure (Renard et al., 2017). Reduced prefrontal GABAergic transmission is associated with hyperdopaminergic state and alterations of prefrontal cortex-related behaviors (Yee et al., 2005; Enomoto et al., 2011), mimicking key behavioral alterations observed for instance in schizophrenia (Carlsson et al., 1997; O'Carroll, 2000; Nakazawa et al., 2012).
Adolescent cannabis exposure also directly impacts the activity of dopamine neurons in the VTA with potential alterations of eCB-mediated inhibition of VTA GABA neurons and glutamate terminals, resulting in a decrease of eCB-dependent disinhibition of dopamine neuron firing and dopamine release in the nucleus accumbens (Scherma et al., 2016), suggesting a broader impact of cannabis during adolescence on dopamine functions. The specific impact of adolescent THC exposure on mesocortical dopamine neurons or on dopamine release and signaling within the prefrontal cortex still needs to be investigated. Similarly, other drugs of abuse seem to have a critical influence on corticolimbic circuits and brain functions if they are used during adolescence compared to adulthood (for a complete view on the impact of drugs on adolescent brains see these recent books edited by Bell and Rahman, 2021a,b).
In parallel to cannabis use by teenagers, in recent decades there has been a dramatic rise of obesity amongst children and adolescents, due to the increased availability of energy-dense and highly palatable foods and drinks (Crews et al., 2007; Wang et al., 2008; Ogden et al., 2016). Moreover, animal studies have shown that adolescents are more sensitive to palatable foods such as high-fat and high-sugar foods (Wilmouth and Spear, 2009; Friemel et al., 2010; Marshall et al., 2017, 2020) which may increase food-seeking behaviors, lead to overconsumption, unbalanced dietary habits and obesity.
The dopamine system is well-known to respond directly to food rewards and modulate food-seeking behaviors by acting in prefrontal and subcortical regions (Hajnal and Norgren, 2001; Bassareo et al., 2002; Hajnal et al., 2004; Berridge, 2007; Avena et al., 2008; de Araujo et al., 2010; Kenny, 2011; McCutcheon, 2015; Alhadeff et al., 2019; Zimmerman and Knight, 2020; Grove et al., 2022). Similarly, eCBs are tightly linked to feeding as they are derived from poly-unsaturated fatty acids and are able to modulate homeostatic and non-homeostatic/hedonic feeding (Mahler et al., 2007; Maccarrone et al., 2010; Lau et al., 2017; Coccurello and Maccarrone, 2018). In humans and rodents, obesity in adults is associated with alteration of both dopamine and eCB circuits (Berthoud and Morrison, 2008; South and Huang, 2008; Volkow et al., 2011; Bello et al., 2012) but also with dysfunction of prefrontal functions (Reichelt, 2016; Lowe et al., 2019). In adolescents, the delayed development of both dopamine and eCB systems may simultaneously support this adolescent sensitivity to overnutrition, but also provides a vulnerability window for the long-term effects of unbalanced dietary habits.
The differential time course in the development of cortical and subcortical dopamine pathways may be responsible of increased reactivity to palatable foods during adolescence, leading to overconsumption and increased food-seeking responses (Wilmouth and Spear, 2009; Friemel et al., 2010; Marshall et al., 2017, 2020). As eCB signaling controls the activity of mesocorticolimbic circuits, increased levels of CB1 receptors during adolescence may also participate to this process by decreasing VTA GABAergic activity and decreasing cortical control on VTA and striatal neurons, all together leading to increased dopamine activity (Lau et al., 2017; Sallam and Borgland, 2021) and food-seeking behaviors (Mateo et al., 2017).
Several studies have also investigated the impact of adolescent exposure to palatable food on related brain functions to understand how vulnerable these circuits may be to natural rewards. Adolescent overnutrition causes complex behavioral alterations which seem to be highly dependent on diet parameters including but not limited the constitution of the diet (e.g. high fat, high sugar, cafeteria diet), the duration of exposure (age at start, length of time exposed, withdrawal period between diet and testing) or other exposure parameters (e.g., continuous or limited access, combined access with control diet or not). We focus here on reward- and prefrontal-related functions but there is also an important literature on the effect of these dietary habits on learning, memory and emotional behaviors (for recent reviews on the topic see Kendig, 2014; Tsan et al., 2021). Taken together, several studies indicate that exposure to highly palatable foods during adolescence, but not at adulthood, strongly impacts reward-related functions including the preference for palatable foods (Vendruscolo et al., 2010b; Carlin et al., 2016; Naneix et al., 2016; Rabasa et al., 2016; Gueye et al., 2018), conditioned place preference for food (Privitera et al., 2011), motivation for food rewards (Frazier et al., 2008; Vendruscolo et al., 2010a; Reichelt et al., 2016; Tantot et al., 2017; Wong et al., 2017; Naneix et al., 2018; Ducrocq et al., 2019), hedonic processing (Naneix et al., 2016; but see Steele et al., 2019), and sensitivity to drugs of abuse (Blanco-Gandía et al., 2017; Naneix et al., 2017). More importantly, several adolescent dietary habits also seem to impact prefrontal-related functions including control of goal-directed behavior (Tantot et al., 2017; but see Kendig et al., 2013), memory extinction (Baker and Reichelt, 2016), and cognitive control (Reichelt et al., 2015; Labouesse et al., 2017; Robertson and Rasmussen, 2017; Steele et al., 2019).
At the neurobiological level, exposure to highly palatable foods during adolescence is associated with broad anatomical and functional alterations of the dopamine system including reduced dopamine clearance in the striatum (Baladi et al., 2015), increased sensitivity of the mesolimbic pathway (Naneix et al., 2017) and changes in the expression of dopamine markers (Teegarden et al., 2009; Carlin et al., 2016; Naneix et al., 2017, 2018) in the prefrontal cortex and the nucleus accumbens. To our knowledge, the specific effects of such adolescent diets on prefrontal dopamine release, transmission and associated plasticity has not been investigated. However, the functioning of prefrontal microcuircuits is strongly impacted by adolescent consumption of palatable foods. As with cannabis exposure, prefrontal GABA signaling seems especially vulnerable, with diet-induced decreased of GABA levels (Sandoval-Salazar et al., 2016) and numbers of parvalbumin interneurons (Reichelt et al., 2015, 2019; Baker and Reichelt, 2016). Moreover, juvenile obesity in mice also impairs prefrontal glutamatergic transmission including NMDA-dependent synaptic plasticity and AMPA postsynaptic currents (Labouesse et al., 2017). Surprisingly, given its role in feeding and appetite, there are no clear data available on the impact of such diet exposure on the eCB system. In adult animals, obesity or the exposure to high-sugar/high-fat foods alters eCB-dependent cortical synaptic plasticity and insulin effects on VTA dopamine neurons to control ingestive behaviors (Labouèbe et al., 2013; Liu and Borgland, 2015; Liu et al., 2016; Lau et al., 2017). As maternal high-fat diet dysregulates the eCB system (Almeida et al., 2022; Urbonaite et al., 2022), we might predict high-fat / high-sugar diet exposure during adolescence would have similar effects. The exact nature of these alterations is not well-understood and the mechanisms which make adolescent PFC circuits particularly vulnerable are yet to be determined. However, altered dopamine and eCB signaling may both contribute to long-lasting diet-induced dysfunctions in executive functions.
Dopamine and eCB signaling are central elements in the regulation of prefrontal microcircuits and their dysregulation is associated with numerous neuropsychiatric disorders that typically first present in adolescence. During adolescence, prefrontal circuits undergo important developmental changes which support the emergence of adaptive adult behaviors. Dopamine and eCB prefrontal systems have a similar delayed maturation during adolescence characterized by transitory increases in receptors expression, gradual increases in neurotransmitter levels and important changes in synaptic effects. Given their interactions with different neuronal populations and effects at different synaptic sites, adolescent remodeling of these systems may play a key role in the successful maturation of prefrontal circuits and their functioning.
Despite a considerable increase in research on this topic during the last few decades, numerous questions remain. By increasing the recruitment of GABAergic interneurons and the sustained activity of pyramidal neurons during the transition to adulthood, prefrontal dopamine signaling increases the selection of specific inputs, decreasing the signal-to-noise ratio and refining prefrontal processing (O'Donnell, 2011; Caballero et al., 2016). Specific guidance cues control the late development of dopamine fibers (Hoops and Flores, 2017) but the mechanisms governing synaptic changes and the emergence of adult-like PFC activity compared to limbic regions remains unclear. Moreover, despite clear effects of external insults (drugs of abuse, palatable foods, and stress) during adolescence on prefrontal functions, anatomical organization of the mesocortical pathway and on the functioning of prefrontal glutamate and GABA transmission, the impact on the functioning of this dopamine pathway in vivo and its interaction with local microcircuits remains poorly explored compared to other dopamine circuits. One of the reasons for this may come from the unique properties of the mesocortical pathway (Sesack et al., 1998; Lammel et al., 2008) which have made it challenging to investigate in vivo until recently.
For the eCB system, most of our knowledge on the maturation of the eCB system comes from studying the effect of drugs including THC or CB1 agonists given during adolescence. The prefrontal eCB system presents important anatomical changes during adolescence (Rubino et al., 2015; Caballero et al., 2016). However, little is known about related neurophysiological changes on different prefrontal populations and the underlying processes governing these changes. Considering the important role of eCB in responses to food and in the control of feeding (Lau et al., 2017), there is also an important lack of information on the impact of unbalanced dietary habits during adolescence on eCB signaling.
Finally, a crucial point to consider is how dopamine and eCB systems interact through development. At adulthood they exhibit strong functional interactions to control diverse motivated behaviors. As they both exhibit a continued maturation throughout adolescence, which is not observed in other neuromodulatory systems, they may represent a crucial milestone in postnatal brain development in health and disease. The recent development of cutting-edge tools such as specific optical sensors for in vivo and ex vivo recordings (Labouesse et al., 2020; Dong et al., 2021) allows us to dissect microcircuits and use longitudinal studies to measure dynamic changes of prefrontal dopamine and eCB signaling from childhood to adulthood which until now were not possible.
All authors conceptualized, wrote and edited the manuscript, designed and produced all figures, and approved the submitted version.
FN was funded by the Royal Society (RGS_R1_211013), the Wellcome Trust Institutional Strategic Support Fund (RG13793-43), and the Tenovus Scotland (G21.11). KZP was funded by the Medical Research Council (project MR/T03260X/1) at the University of Sussex in the lab of Eisuke Koya.
KZP acknowledges the mentorship of Dr. Eisuke Koya in allowing her the time to contribute to this manuscript.
The authors declare that the research was conducted in the absence of any commercial or financial relationships that could be construed as a potential conflict of interest.
All claims expressed in this article are solely those of the authors and do not necessarily represent those of their affiliated organizations, or those of the publisher, the editors and the reviewers. Any product that may be evaluated in this article, or claim that may be made by its manufacturer, is not guaranteed or endorsed by the publisher.
Abercrombie, E. D., Keefe, K. A., DiFrischia, D. S., and Zigmond, M. J. (1989). Differential effect of stress on in vivo dopamine release in striatum, nucleus accumbens, and medial frontal cortex. J. Neurochem. 52, 1655–1658. doi: 10.1111/j.1471-4159.1989.tb09224.x
Adriani, W., and Laviola, G. (2003). Elevated levels of impulsivity and reduced place conditioning with d-amphetamine: two behavioral features of adolescence in mice. Behav. Neurosci. 117, 695–703. doi: 10.1037/0735-7044.117.4.695
Ahn, S., and Phillips, A. G. (1999). Dopaminergic correlates of sensory-specific satiety in the medial prefrontal cortex and nucleus accumbens of the rat. J. Neurosci. Off. J. Soc. Neurosci. 19, RC29. doi: 10.1523/JNEUROSCI.19-19-j0003.1999
Alexander, G. E., DeLong, M. R., and Strick, P. L. (1986). Parallel organization of functionally segregated circuits linking basal ganglia and cortex. Annu. Rev. Neurosci. 9, 357–381. doi: 10.1146/annurev.ne.09.030186.002041
Alhadeff, A. L., Goldstein, N., Park, O., Klima, M. L., Vargas, A., and Betley, J. N. (2019). Natural and drug rewards engage distinct pathways that converge on coordinated hypothalamic and reward circuits. Neuron 103, 891–908.e6. doi: 10.1016/j.neuron.2019.05.050
Almeida, M. M., Dias-Rocha, C. P., Calviño, C., and Trevenzoli, I. H. (2022). Lipid endocannabinoids in energy metabolism, stress and developmental programming. Mol. Cell. Endocrinol. 542, 111522. doi: 10.1016/j.mce.2021.111522
Almey, A., Milner, T. A., and Brake, W. G. (2015). Estrogen receptors in the central nervous system and their implication for dopamine-dependent cognition in females. Horm. Behav. 74, 125–138. doi: 10.1016/j.yhbeh.2015.06.010
Andersen, S. L. (2003). Trajectories of brain development: point of vulnerability or window of opportunity? Neurosci. Biobehav. Rev. 27, 3–18. doi: 10.1016/S0149-7634(03)00005-8
Andersen, S. L., Thompson, A. T., Rutstein, M., Hostetter, J. C., and Teicher, M. H. (2000). Dopamine receptor pruning in prefrontal cortex during the periadolescent period in rats. Synap. N. Y. N 37, 167–169. doi: 10.1002/1098-2396(200008)37:2<167::AID-SYN11>3.0.CO;2-B
Andrzejewski, M. E., Schochet, T. L., Feit, E. C., Harris, R., McKee, B. L., and Kelley, A. E. (2011). A comparison of adult and adolescent rat behavior in operant learning, extinction, and behavioral inhibition paradigms. Behav. Neurosci. 125, 93–105. doi: 10.1037/a0022038
Atwood, B. K., and Mackie, K. (2010). CB2: a cannabinoid receptor with an identity crisis. Br. J. Pharmacol. 160, 467–479. doi: 10.1111/j.1476-5381.2010.00729.x
Auclair, N., Otani, S., Soubrie, P., and Crepel, F. (2000). Cannabinoids modulate synaptic strength and plasticity at glutamatergic synapses of rat prefrontal cortex pyramidal neurons. J. Neurophysiol. 83, 3287–3293. doi: 10.1152/jn.2000.83.6.3287
Avena, N. M., Rada, P., and Hoebel, B. G. (2008). Evidence for sugar addiction: behavioral and neurochemical effects of intermittent, excessive sugar intake. Neurosci. Biobehav. Rev. 32, 20–39. doi: 10.1016/j.neubiorev.2007.04.019
Baker, K. D., and Reichelt, A. C. (2016). Impaired fear extinction retention and increased anxiety-like behaviours induced by limited daily access to a high-fat/high-sugar diet in male rats: implications for diet-induced prefrontal cortex dysregulation. Neurobiol. Learn. Mem. 136, 127–138. doi: 10.1016/j.nlm.2016.10.002
Baladi, M. G., Horton, R. E., Owens, W. A., Daws, L. C., and France, C. P. (2015). Eating high fat chow decreases dopamine clearance in adolescent and adult male rats but selectively enhances the locomotor stimulating effects of cocaine in adolescents. Int. J. Neuropsychopharmacol. 18, pyv024. doi: 10.1093/ijnp/pyv024
Baldwin, A. E., Sadeghian, K., and Kelley, A. E. (2002). Appetitive instrumental learning requires coincident activation of NMDA and dopamine D1 receptors within the medial prefrontal cortex. J. Neurosci. Off. J. Soc. Neurosci. 22, 1063–1071. doi: 10.1523/JNEUROSCI.22-03-01063.2002
Balleine, B. W., and O'Doherty, J. P. (2010). Human and rodent homologies in action control: corticostriatal determinants of goal-directed and habitual action. Neuropsychopharmacol. Off. Publ. Am. Coll. Neuropsychopharmacol. 35, 48–69. doi: 10.1038/npp.2009.131
Bara, A., Ferland, J.-M. N., Rompala, G., Szutorisz, H., and Hurd, Y. L. (2021). Cannabis and synaptic reprogramming of the developing brain. Nat. Rev. Neurosci. 22, 423–438. doi: 10.1038/s41583-021-00465-5
Bassareo, V., De Luca, M. A., and Di Chiara, G. (2002). Differential expression of motivational stimulus properties by dopamine in nucleus accumbens shell vs. core and prefrontal cortex. J. Neurosci. Off. J. Soc. Neurosci. 22, 4709–4719. doi: 10.1523/JNEUROSCI.22-11-04709.2002
Béchade, C., Cantaut-Belarif, Y., and Bessis, A. (2013). Microglial control of neuronal activity. Front. Cell. Neurosci. 7, 32. doi: 10.3389/fncel.2013.00032
Becker, J. B., and Chartoff, E. (2019). Sex differences in neural mechanisms mediating reward and addiction. Neuropsychopharmacol. Off. Publ. Am. Coll. Neuropsychopharmacol. 44, 166–183. doi: 10.1038/s41386-018-0125-6
Beier, K. T., Steinberg, E. E., DeLoach, K. E., Xie, S., Miyamichi, K., Schwarz, L., et al. (2015). Circuit architecture of VTA dopamine neurons revealed by systematic input-output mapping. Cell 162, 622–634. doi: 10.1016/j.cell.2015.07.015
Bell, R. L., and Rahman, S. (2021a). Effects of Peri-Adolescent Licit and Illicit Drug Use on the Developing CNS Part I. 1st Edn. Cambridge, MA: Academic Press.
Bell, R. L., and Rahman, S. (2021b). Effects of Peri-Adolescent Licit and Illicit Drug Use on the Developing CNS: Part II. 1st Edn. Cambridge, MA: Academic Press. doi: 10.1080/21622965.2022.2100821
Bello, N. T., Coughlin, J. W., Redgrave, G. W., Ladenheim, E. E., Moran, T. H., and Guarda, A. S. (2012). Dietary conditions and highly palatable food access alter rat cannabinoid receptor expression and binding density. Physiol. Behav. 105, 720–726. doi: 10.1016/j.physbeh.2011.09.021
Benes, F. M., Vincent, S. L., Molloy, R., and Khan, Y. (1996). Increased interaction of dopamine-immunoreactive varicosities with GABA neurons of rat medial prefrontal cortex occurs during the postweanling period. Synap. N. Y. N 23, 237–245. doi: 10.1002/(SICI)1098-2396(199608)23:4<237::AID-SYN1>3.0.CO;2-8
Berger, B., Gaspar, P., and Verney, C. (1991). Dopaminergic innervation of the cerebral cortex: unexpected differences between rodents and primates. Trends Neurosci. 14, 21–27. doi: 10.1016/0166-2236(91)90179-X
Berghuis, P., Rajnicek, A. M., Morozov, Y. M., Ross, R. A., Mulder, J., Urbán, G. M., et al. (2007). Hardwiring the brain: endocannabinoids shape neuronal connectivity. Science 316, 1212–1216. doi: 10.1126/science.1137406
Berke, J. D. (2018). What does dopamine mean? Nat. Neurosci. 21, 787–793. doi: 10.1038/s41593-018-0152-y
Berrendero, F., Sepe, N., Ramos, J. A., Di Marzo, V., and Fernández-Ruiz, J. J. (1999). Analysis of cannabinoid receptor binding and mRNA expression and endogenous cannabinoid contents in the developing rat brain during late gestation and early postnatal period. Synap. N. Y. N 33, 181–191. doi: 10.1002/(SICI)1098-2396(19990901)33:3<181::AID-SYN3>3.0.CO;2-R
Berridge, K. C. (2007). The debate over dopamine's role in reward: the case for incentive salience. Psychopharmacology 191, 391–431. doi: 10.1007/s00213-006-0578-x
Berthoud, H.-R., and Morrison, C. (2008). The brain, appetite, and obesity. Annu. Rev. Psychol. 59, 55–92. doi: 10.1146/annurev.psych.59.103006.093551
Bhattacherjee, A., Djekidel, M. N., Chen, R., Chen, W., Tuesta, L. M., and Zhang, Y. (2019). Cell type-specific transcriptional programs in mouse prefrontal cortex during adolescence and addiction. Nat. Commun. 10, 4169. doi: 10.1038/s41467-019-12054-3
Bisogno, T., Berrendero, F., Ambrosino, G., Cebeira, M., Ramos, J. A., Fernandez-Ruiz, J. J., et al. (1999). Brain regional distribution of endocannabinoids: implications for their biosynthesis and biological function. Biochem. Biophys. Res. Commun. 256, 377–380. doi: 10.1006/bbrc.1999.0254
Björklund, A., and Dunnett, S. B. (2007). Dopamine neuron systems in the brain: an update. Trends Neurosci. 30, 194–202. doi: 10.1016/j.tins.2007.03.006
Blagburn-Blanco, S. V., Chappell, M. S., De Biase, L. M., and DeNardo, L. A. (2022). Synapse-specific roles for microglia in development: new horizons in the prefrontal cortex. Front. Mol. Neurosci. 15, 965756. doi: 10.3389/fnmol.2022.965756
Blakemore, S.-J. (2012). Imaging brain development: the adolescent brain. NeuroImage 61, 397–406. doi: 10.1016/j.neuroimage.2011.11.080
Blakemore, S.-J., and Robbins, T. W. (2012). Decision-making in the adolescent brain. Nat. Neurosci. 15, 1184–1191. doi: 10.1038/nn.3177
Blanco-Gandía, M. C., Cantacorps, L., Aracil-Fernández, A., Montagud-Romero, S., Aguilar, M. A., Manzanares, J., et al. (2017). Effects of bingeing on fat during adolescence on the reinforcing effects of cocaine in adult male mice. Neuropharmacology 113, 31–44. doi: 10.1016/j.neuropharm.2016.09.020
Bogart, L. J., and O'Donnell, P. (2018). Multiple long-range inputs evoke NMDA currents in prefrontal cortex fast-spiking interneurons. Neuropsychopharmacol. Off. Publ. Am. Coll. Neuropsychopharmacol. 43, 2101–2108. doi: 10.1038/s41386-018-0029-5
Brenhouse, H. C., and Andersen, S. L. (2011). Developmental trajectories during adolescence in males and females: a cross-species understanding of underlying brain changes. Neurosci. Biobehav. Rev. 35, 1687–1703. doi: 10.1016/j.neubiorev.2011.04.013
Brenhouse, H. C., and Schwarz, J. M. (2016). Immunoadolescence: neuroimmune development and adolescent behavior. Neurosci. Biobehav. Rev. 70, 288–299. doi: 10.1016/j.neubiorev.2016.05.035
Brenhouse, H. C., Sonntag, K. C., and Andersen, S. L. (2008). Transient D1 dopamine receptor expression on prefrontal cortex projection neurons: relationship to enhanced motivational salience of drug cues in adolescence. J. Neurosci. Off. J. Soc. Neurosci. 28, 2375–2382. doi: 10.1523/JNEUROSCI.5064-07.2008
Brignani, S., and Pasterkamp, R. J. (2017). Neuronal subset-specific migration and axonal wiring mechanisms in the developing midbrain dopamine system. Front. Neuroanat. 11, 55. doi: 10.3389/fnana.2017.00055
Bromberg-Martin, E. S., Matsumoto, M., and Hikosaka, O. (2010). Dopamine in motivational control: rewarding, aversive, and alerting. Neuron 68, 815–834. doi: 10.1016/j.neuron.2010.11.022
Burton, C. L., and Fletcher, P. J. (2012). Age and sex differences in impulsive action in rats: the role of dopamine and glutamate. Behav. Brain Res. 230, 21–33. doi: 10.1016/j.bbr.2012.01.046
Burton, C. L., Noble, K., and Fletcher, P. J. (2011). Enhanced incentive motivation for sucrose-paired cues in adolescent rats: possible roles for dopamine and opioid systems. Neuropsychopharmacol. Off. Publ. Am. Coll. Neuropsychopharmacol. 36, 1631–1643. doi: 10.1038/npp.2011.44
Caballero, A., Flores-Barrera, E., Cass, D. K., and Tseng, K. Y. (2014a). Differential regulation of parvalbumin and calretinin interneurons in the prefrontal cortex during adolescence. Brain Struct. Funct. 219, 395–406. doi: 10.1007/s00429-013-0508-8
Caballero, A., Granberg, R., and Tseng, K. Y. (2016). Mechanisms contributing to prefrontal cortex maturation during adolescence. Neurosci. Biobehav. Rev. 70, 4–12. doi: 10.1016/j.neubiorev.2016.05.013
Caballero, A., Thomases, D. R., Flores-Barrera, E., Cass, D. K., and Tseng, K. Y. (2014b). Emergence of GABAergic-dependent regulation of input-specific plasticity in the adult rat prefrontal cortex during adolescence. Psychopharmacology 231, 1789–1796. doi: 10.1007/s00213-013-3216-4
Cabral, G. A., Raborn, E. S., Griffin, L., Dennis, J., and Marciano-Cabral, F. (2008). CB2 receptors in the brain: role in central immune function. Br. J. Pharmacol. 153, 240–251. doi: 10.1038/sj.bjp.0707584
Cachope, R., Mateo, Y., Mathur, B. N., Irving, J., Wang, H.-L., Morales, M., et al. (2012). Selective activation of cholinergic interneurons enhances accumbal phasic dopamine release: setting the tone for reward processing. Cell Rep. 2, 33–41. doi: 10.1016/j.celrep.2012.05.011
Calabro, F. J., Murty, V. P., Jalbrzikowski, M., Tervo-Clemmens, B., and Luna, B. (2020). Development of hippocampal–prefrontal cortex interactions through adolescence. Cereb. Cortex 30, 1548–1558. doi: 10.1093/cercor/bhz186
Carlén, M. (2017). What constitutes the prefrontal cortex? Science 358, 478–482. doi: 10.1126/science.aan8868
Carlin, J. L., McKee, S. E., Hill-Smith, T., Grissom, N. M., George, R., Lucki, I., et al. (2016). Removal of high-fat diet after chronic exposure drives binge behavior and dopaminergic dysregulation in female mice. Neuroscience 326, 170–179. doi: 10.1016/j.neuroscience.2016.04.002
Carlsson, A., Hansson, L. O., Waters, N., and Carlsson, M. L. (1997). Neurotransmitter aberrations in schizophrenia: new perspectives and therapeutic implications. Life Sci. 61, 75–94. doi: 10.1016/S0024-3205(97)00228-2
Carr, D. B., and Sesack, S. R. (2000). Projections from the rat prefrontal cortex to the ventral tegmental area: target specificity in the synaptic associations with mesoaccumbens and mesocortical neurons. J. Neurosci. Off. J. Soc. Neurosci. 20, 3864–3873. doi: 10.1523/JNEUROSCI.20-10-03864.2000
Casey, B. J., Jones, R. M., and Hare, T. A. (2008). The adolescent brain. Ann. N. Y. Acad. Sci. 1124, 111–126. doi: 10.1196/annals.1440.010
Cass, D. K., Flores-Barrera, E., Thomases, D. R., Vital, W. F., Caballero, A., and Tseng, K. Y. (2014). CB1 cannabinoid receptor stimulation during adolescence impairs the maturation of GABA function in the adult rat prefrontal cortex. Mol. Psychiatry 19, 536–543. doi: 10.1038/mp.2014.14
Cass, W. A., and Gerhardt, G. A. (1995). In vivo assessment of dopamine uptake in rat medial prefrontal cortex: comparison with dorsal striatum and nucleus accumbens. J. Neurochem. 65, 201–207. doi: 10.1046/j.1471-4159.1995.65010201.x
Cheer, J. F., Aragona, B. J., Heien, M. L. A. V., Seipel, A. T., Carelli, R. M., and Wightman, R. M. (2007). Coordinated accumbal dopamine release and neural activity drive goal-directed behavior. Neuron 54, 237–244. doi: 10.1016/j.neuron.2007.03.021
Cheer, J. F., Kendall, D. A., and Marsden, C. A. (2000). Cannabinoid receptors and reward in the rat: a conditioned place preference study. Psychopharmacology 151, 25–30. doi: 10.1007/s002130000481
Chiu, C. Q., Puente, N., Grandes, P., and Castillo, P. E. (2010). Dopaminergic modulation of endocannabinoid-mediated plasticity at GABAergic synapses in the prefrontal cortex. J. Neurosci. Off. J. Soc. Neurosci. 30, 7236–7248. doi: 10.1523/JNEUROSCI.0736-10.2010
Coccurello, R., and Maccarrone, M. (2018). Hedonic eating and the “delicious circle”: from lipid-derived mediators to brain dopamine and back. Front. Neurosci. 12, 271. doi: 10.3389/fnins.2018.00271
Coutureau, E., and Parkes, S. L. (2018). “Cortical determinants of goal-directed behavior,” in Goal-Directed Decision Making, eds R. Morris, A. Bornstein, and A. Shenhav (Cambridge, MA: Academic Press), 179–197. doi: 10.1016/B978-0-12-812098-9.00008-5
Covey, D., Mateo, Y., Sulzer, D., Cheer, J. F., and Lovinger, D. M. (2017). Endocannabinoid modulation of dopamine neurotransmission. Neuropharmacology 124, 52–61. doi: 10.1016/j.neuropharm.2017.04.033
Crews, F., He, J., and Hodge, C. (2007). Adolescent cortical development: a critical period of vulnerability for addiction. Pharmacol. Biochem. Behav. 86, 189–199. doi: 10.1016/j.pbb.2006.12.001
Cuccurazzu, B., Zamberletti, E., Nazzaro, C., Prini, P., Trusel, M., Grilli, M., et al. (2018). Adult cellular neuroadaptations induced by adolescent THC exposure in female rats are rescued by enhancing anandamide signaling. Int. J. Neuropsychopharmacol. 21, 1014–1024. doi: 10.1093/ijnp/pyy057
Cunningham, M. G., Bhattacharyya, S., and Benes, F. M. (2002). Amygdalo-cortical sprouting continues into early adulthood: Implications for the development of normal and abnormal function during adolescence. J. Comp. Neurol. 453, 116–130. doi: 10.1002/cne.10376
Daw, N. D., O'Doherty, J. P., Dayan, P., Seymour, B., and Dolan, R. J. (2006). Cortical substrates for exploratory decisions in humans. Nature 441, 876–879. doi: 10.1038/nature04766
de Araujo, I. E., Ren, X., and Ferreira, J. G. (2010). Metabolic sensing in brain dopamine systems. Results Probl. Cell Differ. 52, 69–86. doi: 10.1007/978-3-642-14426-4_7
de Fonseca, F. R., Ramos, J. A., Bonnin, A., and Fernández-Ruiz, J. J. (1993). Presence of cannabinoid binding sites in the brain from early postnatal ages. NeuroReport 4, 135–138. doi: 10.1097/00001756-199302000-00005
Delevich, K., Klinger, M., Okada, N. J., and Wilbrecht, L. (2021). Coming of age in the frontal cortex: the role of puberty in cortical maturation. Semin. Cell Dev. Biol. 118, 64–72. doi: 10.1016/j.semcdb.2021.04.021
Dong, A., He, K., Dudok, B., Farrell, J. S., Guan, W., Liput, D. J., et al. (2021). A fluorescent sensor for spatiotemporally resolved imaging of endocannabinoid dynamics in vivo. Nat. Biotechnol. 2021, 329169. doi: 10.1101/2020.10.08.329169
Doremus-Fitzwater, T. L., Barreto, M., and Spear, L. P. (2012). Age-related differences in impulsivity among adolescent and adult Sprague-Dawley rats. Behav. Neurosci. 126, 735–741. doi: 10.1037/a0029697
Drzewiecki, C. M., and Juraska, J. M. (2020). The structural reorganization of the prefrontal cortex during adolescence as a framework for vulnerability to the environment. Pharmacol. Biochem. Behav. 199, 173044. doi: 10.1016/j.pbb.2020.173044
Ducrocq, F., Hyde, A., Fanet, H., Oummadi, A., Walle, R., De Smedt-Peyrusse, V., et al. (2019). Decrease in operant responding under obesogenic diet exposure is not related to deficits in incentive or hedonic processes. Obes. Silver Spring Md 27, 255–263. doi: 10.1002/oby.22358
Durstewitz, D., and Seamans, J. K. (2008). The dual-state theory of prefrontal cortex dopamine function with relevance to catechol-o-methyltransferase genotypes and schizophrenia. Biol. Psychiatry 64, 739–749. doi: 10.1016/j.biopsych.2008.05.015
Egerton, A., Allison, C., Brett, R. R., and Pratt, J. A. (2006). Cannabinoids and prefrontal cortical function: insights from preclinical studies. Neurosci. Biobehav. Rev. 30, 680–695. doi: 10.1016/j.neubiorev.2005.12.002
Ellgren, M., Artmann, A., Tkalych, O., Gupta, A., Hansen, H. S., Hansen, S. H., et al. (2008). Dynamic changes of the endogenous cannabinoid and opioid mesocorticolimbic systems during adolescence: THC effects. Eur. Neuropsychopharmacol. 18, 826–834. doi: 10.1016/j.euroneuro.2008.06.009
Ellgren, M., Spano, S. M., and Hurd, Y. L. (2007). Adolescent cannabis exposure alters opiate intake and opioid limbic neuronal populations in adult rats. Neuropsychopharmacol. Off. Publ. Am. Coll. Neuropsychopharmacol. 32, 607–615. doi: 10.1038/sj.npp.1301127
Ellwood, I. T., Patel, T., Wadia, V., Lee, A. T., Liptak, A. T., Bender, K. J., et al. (2017). Tonic or phasic stimulation of dopaminergic projections to prefrontal cortex causes mice to maintain or deviate from previously learned behavioral strategies. J. Neurosci. Off. J. Soc. Neurosci. 37, 8315–8329. doi: 10.1523/JNEUROSCI.1221-17.2017
Enomoto, T., Tse, M. T., and Floresco, S. B. (2011). Reducing prefrontal gamma-aminobutyric acid activity induces cognitive, behavioral, and dopaminergic abnormalities that resemble schizophrenia. Biol. Psychiatry 69, 432–441. doi: 10.1016/j.biopsych.2010.09.038
Ernst, M., Pine, D. S., and Hardin, M. (2006). Triadic model of the neurobiology of motivated behavior in adolescence. Psychol. Med. 36, 299–312. doi: 10.1017/S0033291705005891
Fallon, J. H. (1988). Topographic organization of ascending dopaminergic projections. Ann. N. Y. Acad. Sci. 537, 1–9. doi: 10.1111/j.1749-6632.1988.tb42093.x
Fasano, C., Kortleven, C., and Trudeau, L.-E. (2010). Chronic activation of the D2 autoreceptor inhibits both glutamate and dopamine synapse formation and alters the intrinsic properties of mesencephalic dopamine neurons in vitro. Eur. J. Neurosci. 32, 1433–1441. doi: 10.1111/j.1460-9568.2010.07397.x
Fasano, C., Poirier, A., DesGroseillers, L., and Trudeau, L.-E. (2008). Chronic activation of the D2 dopamine autoreceptor inhibits synaptogenesis in mesencephalic dopaminergic neurons in vitro. Eur. J. Neurosci. 28, 1480–1490. doi: 10.1111/j.1460-9568.2008.06450.x
Flores, C., Manitt, C., Rodaros, D., Thompson, K. M., Rajabi, H., Luk, K. C., et al. (2005). Netrin receptor deficient mice exhibit functional reorganization of dopaminergic systems and do not sensitize to amphetamine. Mol. Psychiatry 10, 606–612. doi: 10.1038/sj.mp.4001607
Flores-Barrera, E., Thomases, D. R., Heng, L.-J., Cass, D. K., Caballero, A., and Tseng, K. Y. (2014). Late adolescent expression of GluN2B transmission in the prefrontal cortex is input-specific and requires postsynaptic protein kinase A and D1 dopamine receptor signaling. Biol. Psychiatry 75, 508–516. doi: 10.1016/j.biopsych.2013.07.033
Floresco, S. B., and Jentsch, J. D. (2011). Pharmacological enhancement of memory and executive functioning in laboratory animals. Neuropsychopharmacol. Off. Publ. Am. Coll. Neuropsychopharmacol. 36, 227–250. doi: 10.1038/npp.2010.158
Floresco, S. B., and Magyar, O. (2006). Mesocortical dopamine modulation of executive functions: beyond working memory. Psychopharmacology 188, 567–585. doi: 10.1007/s00213-006-0404-5
Floresco, S. B., West, A. R., Ash, B., Moore, H., and Grace, A. A. (2003). Afferent modulation of dopamine neuron firing differentially regulates tonic and phasic dopamine transmission. Nat. Neurosci. 6, 968–973. doi: 10.1038/nn1103
Fortin, D. A., and Levine, E. S. (2007). Differential effects of endocannabinoids on glutamatergic and GABAergic inputs to layer 5 pyramidal neurons. Cereb. Cortex 17, 163–174. doi: 10.1093/cercor/bhj133
Frazier, C. R. M., Mason, P., Zhuang, X., and Beeler, J. A. (2008). Sucrose exposure in early life alters adult motivation and weight gain. PLoS ONE 3, e3221. doi: 10.1371/journal.pone.0003221
Freund, T. F., Katona, I., and Piomelli, D. (2003). Role of endogenous cannabinoids in synaptic signaling. Physiol. Rev. 83, 1017–1066. doi: 10.1152/physrev.00004.2003
Friemel, C. M., Spanagel, R., and Schneider, M. (2010). Reward sensitivity for a palatable food reward peaks during pubertal developmental in rats. Front. Behav. Neurosci. 4, 39. doi: 10.3389/fnbeh.2010.00039
Fuster, J. M. (2001). The prefrontal cortex–an update: time is of the essence. Neuron 30, 319–333. doi: 10.1016/S0896-6273(01)00285-9
Gabbott, P. L. A., Warner, T. A., Jays, P. R. L., Salway, P., and Busby, S. J. (2005). Prefrontal cortex in the rat: projections to subcortical autonomic, motor, and limbic centers. J. Comp. Neurol. 492, 145–177. doi: 10.1002/cne.20738
Galiñanes, G. L., Taravini, I. R. E., and Murer, M. G. (2009). Dopamine-dependent periadolescent maturation of corticostriatal functional connectivity in mouse. J. Neurosci. Off. J. Soc. Neurosci. 29, 2496–2509. doi: 10.1523/JNEUROSCI.4421-08.2009
Gaoni, Y., and Mechoulam, R. (1964). Isolation, structure, and partial synthesis of an active constituent of hashish. J. Am. Chem. Soc. 86, 1646–1647. doi: 10.1021/ja01062a046
Garris, P. A., and Wightman, R. M. (1994). Different kinetics govern dopaminergic transmission in the amygdala, prefrontal cortex, and striatum: an in vivo voltammetric study. J. Neurosci. Off. J. Soc. Neurosci. 14, 442–450. doi: 10.1523/JNEUROSCI.14-01-00442.1994
Gaspar, P., Bloch, B., and Le Moine, C. (1995). D1 and D2 receptor gene expression in the rat frontal cortex: cellular localization in different classes of efferent neurons. Eur. J. Neurosci. 7, 1050–1063. doi: 10.1111/j.1460-9568.1995.tb01092.x
Ginder, D. E., Wright, H. R., and McLaughlin, R. J. (2022). The stoned age: sex differences in the effects of adolescent cannabinoid exposure on prefrontal cortex structure and function in animal models. Int. Rev. Neurobiol. 161, 121–145. doi: 10.1016/bs.irn.2021.07.005
Goldman-Rakic, P. S., Muly, I. E. C., and Williams, G. V. (2000). D1 receptors in prefrontal cells and circuits. Brain Res. Rev. 31, 295–301. doi: 10.1016/S0165-0173(99)00045-4
González, S., Bisogno, T., Wenger, T., Manzanares, J., Milone, A., Berrendero, F., et al. (2000). Sex steroid influence on cannabinoid CB(1) receptor mRNA and endocannabinoid levels in the anterior pituitary gland. Biochem. Biophys. Res. Commun. 270, 260–266. doi: 10.1006/bbrc.2000.2406
Gorelova, N., Seamans, J., Yang, C., Gorelova, N., Seamans, J. K., and Yang, C. R. (2003). Mechanisms of dopamine activation of fast-spiking interneurons that exert inhibition in rat prefrontal cortex. J. Neurophysiol. 88, 3150–3166. doi: 10.1152/jn.00335.2002
Grace, A. A., and Bunney, B. S. (1984a). The control of firing pattern in nigral dopamine neurons: burst firing. J. Neurosci. Off. J. Soc. Neurosci. 4, 2877–2890. doi: 10.1523/JNEUROSCI.04-11-02877.1984
Grace, A. A., and Bunney, B. S. (1984b). The control of firing pattern in nigral dopamine neurons: single spike firing. J. Neurosci. Off. J. Soc. Neurosci. 4, 2866–2876. doi: 10.1523/JNEUROSCI.04-11-02866.1984
Grant, A., Hoops, D., Labelle-Dumais, C., Prévost, M., Rajabi, H., Kolb, B., et al. (2007). Netrin-1 receptor-deficient mice show enhanced mesocortical dopamine transmission and blunted behavioural responses to amphetamine. Eur. J. Neurosci. 26, 3215–3228. doi: 10.1111/j.1460-9568.2007.05888.x
Grant, A., Speed, Z., Labelle-Dumais, C., and Flores, C. (2009). Post-pubertal emergence of a dopamine phenotype in netrin-1 receptor-deficient mice. Eur. J. Neurosci. 30, 1318–1328. doi: 10.1111/j.1460-9568.2009.06919.x
Gremel, C. M., Chancey, J. H., Atwood, B. K., Luo, G., Neve, R., Ramakrishnan, C., et al. (2016). Endocannabinoid modulation of orbitostriatal circuits gates habit formation. Neuron 90, 1312–1324. doi: 10.1016/j.neuron.2016.04.043
Grove, J. C. R., Gray, L. A., La Santa Medina, N., Sivakumar, N., Ahn, J. S., Corpuz, T. V., et al. (2022). Dopamine subsystems that track internal states. Nature 608, 374–380. doi: 10.1038/s41586-022-04954-0
Gueye, A. B., Vendruscolo, L. F., de Ávila, C., Le Moine, C., Darnaudéry, M., and Cador, M. (2018). Unlimited sucrose consumption during adolescence generates a depressive-like phenotype in adulthood. Neuropsychopharmacol. Off. Publ. Am. Coll. Neuropsychopharmacol. 43, 2627–2635. doi: 10.1038/s41386-018-0025-9
Haber, S. N. (2003). The primate basal ganglia: parallel and integrative networks. J. Chem. Neuroanat. 26, 317–330. doi: 10.1016/j.jchemneu.2003.10.003
Hajnal, A., and Norgren, R. (2001). Accumbens dopamine mechanisms in sucrose intake. Brain Res. 904, 76–84. doi: 10.1016/S0006-8993(01)02451-9
Hajnal, A., Smith, G. P., and Norgren, R. (2004). Oral sucrose stimulation increases accumbens dopamine in the rat. Am. J. Physiol. Regul. Integr. Comp. Physiol. 286, R31–37. doi: 10.1152/ajpregu.00282.2003
Hammerslag, L. R., and Gulley, J. M. (2016). Sex differences in behavior and neural development and their role in adolescent vulnerability to substance use. Behav. Brain Res. 298, 15–26. doi: 10.1016/j.bbr.2015.04.008
Häring, M., Guggenhuber, S., and Lutz, B. (2012). Neuronal populations mediating the effects of endocannabinoids on stress and emotionality. Neuroscience 204, 145–158. doi: 10.1016/j.neuroscience.2011.12.035
Harkany, T., Guzmán, M., Galve-Roperh, I., Berghuis, P., Devi, L. A., and Mackie, K. (2007). The emerging functions of endocannabinoid signaling during CNS development. Trends Pharmacol. Sci. 28, 83–92. doi: 10.1016/j.tips.2006.12.004
Harkany, T., Keimpema, E., Barabás, K., and Mulder, J. (2008). Endocannabinoid functions controlling neuronal specification during brain development. Mol. Cell. Endocrinol. 286, S84–90. doi: 10.1016/j.mce.2008.02.011
Heidbreder, C. A., and Groenewegen, H. J. (2003). The medial prefrontal cortex in the rat: evidence for a dorso-ventral distinction based upon functional and anatomical characteristics. Neurosci. Biobehav. Rev. 27, 555–579. doi: 10.1016/j.neubiorev.2003.09.003
Heng, L., Beverley, J. A., Steiner, H., and Tseng, K. Y. (2011a). Differential developmental trajectories for CB1 cannabinoid receptor expression in limbic/associative and sensorimotor cortical areas. Synap. N. Y. N. 65, 278–286. doi: 10.1002/syn.20844
Heng, L., Markham, J. A., Hu, X.-T., and Tseng, K. Y. (2011b). Concurrent upregulation of postsynaptic L-type Ca2+ channel function and protein kinase A signaling is required for the periadolescent facilitation of Ca2+ plateau potentials and dopamine D1 receptor modulation in the prefrontal cortex. Neuropharmacology 60, 953–962. doi: 10.1016/j.neuropharm.2011.01.041
Herkenham, M., Lynn, A. B., Little, M. D., Johnson, M. R., Melvin, L. S., de Costa, B. R., et al. (1990). Cannabinoid receptor localization in brain. Proc. Natl. Acad. Sci. U. S. A. 87, 1932–1936. doi: 10.1073/pnas.87.5.1932
Hernandez, L., and Hoebel, B. G. (1990). Feeding can enhance dopamine turnover in the prefrontal cortex. Brain Res. Bull. 25, 975–979. doi: 10.1016/0361-9230(90)90197-8
Higuera-Matas, A., Soto-Montenegro, M. L., del Olmo, N., Miguéns, M., Torres, I., Vaquero, J. J., et al. (2008). Augmented acquisition of cocaine self-administration and altered brain glucose metabolism in adult female but not male rats exposed to a cannabinoid agonist during adolescence. Neuropsychopharmacol. Off. Publ. Am. Coll. Neuropsychopharmacol. 33, 806–813. doi: 10.1038/sj.npp.1301467
Hill, M. N., McLaughlin, R. J., Pan, B., Fitzgerald, M. L., Roberts, C. J., Lee, T. T.-Y., et al. (2011). Recruitment of prefrontal cortical endocannabinoid signaling by glucocorticoids contributes to termination of the stress response. J. Neurosci. Off. J. Soc. Neurosci. 31, 10506–10515. doi: 10.1523/JNEUROSCI.0496-11.2011
Hill, M. N., and Tasker, J. G. (2012). Endocannabinoid signaling, glucocorticoid-mediated negative feedback, and regulation of the hypothalamic-pituitary-adrenal axis. Neuroscience 204, 5–16. doi: 10.1016/j.neuroscience.2011.12.030
Hillard, C. J., Wilkison, D. M., Edgemond, W. S., and Campbell, W. B. (1995). Characterization of the kinetics and distribution of N-arachidonylethanolamine (anandamide) hydrolysis by rat brain. Biochim. Biophys. Acta 1257, 249–256. doi: 10.1016/0005-2760(95)00087-S
Hoops, D., and Flores, C. (2017). Making dopamine connections in adolescence. Trends Neurosci. 40, 709–719. doi: 10.1016/j.tins.2017.09.004
Hoover, W. B., and Vertes, R. P. (2007). Anatomical analysis of afferent projections to the medial prefrontal cortex in the rat. Brain Struct. Funct. 212, 149–179. doi: 10.1007/s00429-007-0150-4
Howlett, A. C. (2002). The cannabinoid receptors. Prostaglandins Other Lipid Mediat. 68–69, 619–631. doi: 10.1016/S0090-6980(02)00060-6
Howlett, A. C., and Mukhopadhyay, S. (2000). Cellular signal transduction by anandamide and 2-arachidonoylglycerol. Chem. Phys. Lipids 108, 53–70. doi: 10.1016/S0009-3084(00)00187-0
Huestis, M. A., Gorelick, D. A., Heishman, S. J., Preston, K. L., Nelson, R. A., Moolchan, E. T., et al. (2001). Blockade of effects of smoked marijuana by the CB1-selective cannabinoid receptor antagonist SR141716. Arch. Gen. Psychiatry 58, 322–328. doi: 10.1001/archpsyc.58.4.322
Islam, K. U. S., Meli, N., and Blaess, S. (2021). The development of the mesoprefrontal dopaminergic system in health and disease. Front. Neural Circuits 15, 746582. doi: 10.3389/fncir.2021.746582
Johnson, C. M., Loucks, F. A., Peckler, H., Thomas, A. W., Janak, P. H., and Wilbrecht, L. (2016). Long-range orbitofrontal and amygdala axons show divergent patterns of maturation in the frontal cortex across adolescence. Dev. Cogn. Neurosci. 18, 113–120. doi: 10.1016/j.dcn.2016.01.005
Julian, M. D., Martin, A. B., Cuellar, B., Rodriguez De Fonseca, F., Navarro, M., Moratalla, R., et al. (2003). Neuroanatomical relationship between type 1 cannabinoid receptors and dopaminergic systems in the rat basal ganglia. Neuroscience 119, 309–318. doi: 10.1016/S0306-4522(03)00070-8
Kalsbeek, A., Voorn, P., Buijs, R. M., Pool, C. W., and Uylings, H. B. (1988). Development of the dopaminergic innervation in the prefrontal cortex of the rat. J. Comp. Neurol. 269, 58–72. doi: 10.1002/cne.902690105
Kano, M., Ohno-Shosaku, T., Hashimotodani, Y., Uchigashima, M., and Watanabe, M. (2009). Endocannabinoid-mediated control of synaptic transmission. Physiol. Rev. 89, 309–380. doi: 10.1152/physrev.00019.2008
Karreman, M., and Moghaddam, B. (1996). The prefrontal cortex regulates the basal release of dopamine in the limbic striatum: an effect mediated by ventral tegmental area. J. Neurochem. 66, 589–598. doi: 10.1046/j.1471-4159.1996.66020589.x
Kendig, M. D. (2014). Cognitive and behavioural effects of sugar consumption in rodents. A review. Appetite 80, 41–54. doi: 10.1016/j.appet.2014.04.028
Kendig, M. D., Boakes, R. A., Rooney, K. B., and Corbit, L. H. (2013). Chronic restricted access to 10% sucrose solution in adolescent and young adult rats impairs spatial memory and alters sensitivity to outcome devaluation. Physiol. Behav. 120, 164–172. doi: 10.1016/j.physbeh.2013.08.012
Kenny, P. J. (2011). Common cellular and molecular mechanisms in obesity and drug addiction. Nat. Rev. Neurosci. 12, 638–651. doi: 10.1038/nrn3105
Kettenmann, H., Kirchhoff, F., and Verkhratsky, A. (2013). Microglia: new roles for the synaptic stripper. Neuron 77, 10–18. doi: 10.1016/j.neuron.2012.12.023
Kim, Y., Simon, N. W., Wood, J., and Moghaddam, B. (2016). Reward anticipation is encoded differently by adolescent ventral tegmental area neurons. Biol. Psychiatry 79, 878–886. doi: 10.1016/j.biopsych.2015.04.026
Kiritoshi, T., Sun, H., Ren, W., Stauffer, S. R., Lindsley, C. W., Conn, P. J., et al. (2013). Modulation of pyramidal cell output in the medial prefrontal cortex by mGluR5 interacting with CB1. Neuropharmacology 66, 170–178. doi: 10.1016/j.neuropharm.2012.03.024
Kolk, S. M., and Rakic, P. (2022). Development of prefrontal cortex. Neuropsychopharmacology 47, 41–57. doi: 10.1038/s41386-021-01137-9
Kopec, A. M., Smith, C. J., Ayre, N. R., Sweat, S. C., and Bilbo, S. D. (2018). Microglial dopamine receptor elimination defines sex-specific nucleus accumbens development and social behavior in adolescent rats. Nat. Commun. 9, 3769. doi: 10.1038/s41467-018-06118-z
Kreitzer, A. C., and Regehr, W. G. (2002). Retrograde signaling by endocannabinoids. Curr. Opin. Neurobiol. 12, 324–330. doi: 10.1016/S0959-4388(02)00328-8
Kritzer, M. F. (1998). Perinatal gonadectomy exerts regionally selective, lateralized effects on the density of axons immunoreactive for tyrosine hydroxylase in the cerebral cortex of adult male rats. J. Neurosci. Off. J. Soc. Neurosci. 18, 10735–10748. doi: 10.1523/JNEUROSCI.18-24-10735.1998
Kritzer, M. F., Adler, A., Marotta, J., and Smirlis, T. (1999). Regionally selective effects of gonadectomy on cortical catecholamine innervation in adult male rats are most disruptive to afferents in prefrontal cortex. Cereb. Cortex N. Y. N. 9, 507–518. doi: 10.1093/cercor/9.5.507
Kritzer, M. F., and Creutz, L. M. (2008). Region and sex differences in constituent dopamine neurons and immunoreactivity for intracellular estrogen and androgen receptors in mesocortical projections in rats. J. Neurosci. 28, 9525–9535. doi: 10.1523/JNEUROSCI.2637-08.2008
Labouèbe, G., Liu, S., Dias, C., Zou, H., Wong, J. C. Y., Karunakaran, S., et al. (2013). Insulin induces long-term depression of ventral tegmental area dopamine neurons via endocannabinoids. Nat. Neurosci. 16, 300–308. doi: 10.1038/nn.3321
Labouesse, M. A., Cola, R. B., and Patriarchi, T. (2020). GPCR-based dopamine sensors-a detailed guide to inform sensor choice for in vivo imaging. Int. J. Mol. Sci. 21, E8048. doi: 10.3390/ijms21218048
Labouesse, M. A., Lassalle, O., Richetto, J., Iafrati, J., Weber-Stadlbauer, U., Notter, T., et al. (2017). Hypervulnerability of the adolescent prefrontal cortex to nutritional stress via reelin deficiency. Mol. Psychiatry 22, 961–971. doi: 10.1038/mp.2016.193
Lafourcade, M., Elezgarai, I., Mato, S., Bakiri, Y., Grandes, P., and Manzoni, O. J. (2007). Molecular components and functions of the endocannabinoid system in mouse prefrontal cortex. PLoS ONE 2, e709. doi: 10.1371/journal.pone.0000709
Lambe, E. K., Krimer, L. S., and Goldman-Rakic, P. S. (2000). Differential postnatal development of catecholamine and serotonin inputs to identified neurons in prefrontal cortex of rhesus monkey. J. Neurosci. 20, 8780–8787. doi: 10.1523/JNEUROSCI.20-23-08780.2000
Lammel, S., Hetzel, A., Häckel, O., Jones, I., Liss, B., and Roeper, J. (2008). Unique properties of mesoprefrontal neurons within a dual mesocorticolimbic dopamine system. Neuron 57, 760–773. doi: 10.1016/j.neuron.2008.01.022
Lammel, S., Lim, B. K., and Malenka, R. C. (2014). Reward and aversion in a heterogeneous midbrain dopamine system. Neuropharmacology 76, 351–359. doi: 10.1016/j.neuropharm.2013.03.019
Lapish, C. C., Kroener, S., Durstewitz, D., Lavin, A., and Seamans, J. K. (2007). The ability of the mesocortical dopamine system to operate in distinct temporal modes. Psychopharmacology 191, 609–625. doi: 10.1007/s00213-006-0527-8
Lau, B. K., Cota, D., Cristino, L., and Borgland, S. L. (2017). Endocannabinoid modulation of homeostatic and non-homeostatic feeding circuits. Neuropharmacology 124, 38–51. doi: 10.1016/j.neuropharm.2017.05.033
Laubach, M., Amarante, L. M., Swanson, K., and White, S. R. (2018). What, if anything, is rodent prefrontal cortex? eNeuro 5, ENEURO.0315-18.2018. doi: 10.1523/ENEURO.0315-18.2018
Laviolette, S. R., and Grace, A. A. (2006). The roles of cannabinoid and dopamine receptor systems in neural emotional learning circuits: implications for schizophrenia and addiction. Cell. Mol. Life Sci. CMLS 63, 1597–1613. doi: 10.1007/s00018-006-6027-5
Le Moine, C., and Gaspar, P. (1998). Subpopulations of cortical GABAergic interneurons differ by their expression of D1 and D2 dopamine receptor subtypes. Brain Res. Mol. Brain Res. 58, 231–236. doi: 10.1016/S0169-328X(98)00118-1
Lenroot, R. K., and Giedd, J. N. (2006). Brain development in children and adolescents: insights from anatomical magnetic resonance imaging. Neurosci. Biobehav. Rev. 30, 718–729. doi: 10.1016/j.neubiorev.2006.06.001
Leslie, C. A., Robertson, M. W., Cutler, A. J., and Bennett, J. P. (1991). Postnatal development of D1 dopamine receptors in the medial prefrontal cortex, striatum and nucleus accumbens of normal and neonatal 6-hydroxydopamine treated rats: a quantitative autoradiographic analysis. Brain Res. Dev. Brain Res. 62, 109–114. doi: 10.1016/0165-3806(91)90195-O
Levitt, P., and Moore, R. Y. (1979). Development of the noradrenergic innervation of neocortex. Brain Res. 162, 243–259. doi: 10.1016/0006-8993(79)90287-7
Lewis, D. A., and González-Burgos, G. (2008). Neuroplasticity of neocortical circuits in schizophrenia. Neuropsychopharmacol. Off. Publ. Am. Coll. Neuropsychopharmacol. 33, 141–165. doi: 10.1038/sj.npp.1301563
Lidov, H. G., Grzanna, R., and Molliver, M. E. (1980). The serotonin innervation of the cerebral cortex in the rat–an immunohistochemical analysis. Neuroscience 5, 207–227. doi: 10.1016/0306-4522(80)90099-8
Liu, S., and Borgland, S. L. (2015). Regulation of the mesolimbic dopamine circuit by feeding peptides. Neuroscience 289, 19–42. doi: 10.1016/j.neuroscience.2014.12.046
Liu, S., Globa, A. K., Mills, F., Naef, L., Qiao, M., Bamji, S. X., et al. (2016). Consumption of palatable food primes food approach behavior by rapidly increasing synaptic density in the VTA. Proc. Natl. Acad. Sci. U. S. A. 113, 2520–2525. doi: 10.1073/pnas.1515724113
Long, L. E., Lind, J., Webster, M., and Weickert, C. S. (2012). Developmental trajectory of the endocannabinoid system in human dorsolateral prefrontal cortex. BMC Neurosci. 13, 87. doi: 10.1186/1471-2202-13-87
Lovelace, J. W., Corches, A., Vieira, P. A., Mackie, K., and Korzus, E. (2015). An animal model of female adolescent cannabinoid exposure elicits a long-lasting deficit in presynaptic long-term plasticity. Neuropharmacology 99, 242–255. doi: 10.1016/j.neuropharm.2015.04.034
Lovinger, D. M. (2008). Presynaptic modulation by endocannabinoids. Handb. Exp. Pharmacol. 14, 435–477. doi: 10.1007/978-3-540-74805-2_14
Lowe, C. J., Reichelt, A. C., and Hall, P. A. (2019). The prefrontal cortex and obesity: a health neuroscience perspective. Trends Cogn. Sci. 23, 349–361. doi: 10.1016/j.tics.2019.01.005
Lu, H.-C., and Mackie, K. (2016). An introduction to the endogenous cannabinoid system. Biol. Psychiatry 79, 516–525. doi: 10.1016/j.biopsych.2015.07.028
Maccarrone, M. (2020). Missing pieces to the endocannabinoid puzzle. Trends Mol. Med. 26, 263–272. doi: 10.1016/j.molmed.2019.11.002
Maccarrone, M., Gasperi, V., Catani, M. V., Diep, T. A., Dainese, E., Hansen, H. S., et al. (2010). The endocannabinoid system and its relevance for nutrition. Annu. Rev. Nutr. 30, 423–440. doi: 10.1146/annurev.nutr.012809.104701
Maccarrone, M., Guzmán, M., Mackie, K., Doherty, P., and Harkany, T. (2014). Programming of neural cells by (endo)cannabinoids: from physiological rules to emerging therapies. Nat. Rev. Neurosci. 15, 786–801. doi: 10.1038/nrn3846
Mackie, K. (2006). Mechanisms of CB1 receptor signaling: endocannabinoid modulation of synaptic strength. Int. J. Obes. 30, S19–S23. doi: 10.1038/sj.ijo.0803273
Mahler, S. V., Smith, K. S., and Berridge, K. C. (2007). Endocannabinoid hedonic hotspot for sensory pleasure: anandamide in nucleus accumbens shell enhances “liking” of a sweet reward. Neuropsychopharmacol. Off. Publ. Am. Coll. Neuropsychopharmacol. 32, 2267–2278. doi: 10.1038/sj.npp.1301376
Mallya, A. P., Wang, H.-D., Lee, H. N. R., and Deutch, A. Y. (2019). Microglial pruning of synapses in the prefrontal cortex during adolescence. Cereb. Cortex N. Y. N. 29, 1634–1643. doi: 10.1093/cercor/bhy061
Manitt, C., Eng, C., Pokinko, M., Ryan, R. T., Torres-Berrío, A., Lopez, J. P., et al. (2013). DCC orchestrates the development of the prefrontal cortex during adolescence and is altered in psychiatric patients. Transl. Psychiatry 3, e338. doi: 10.1038/tp.2013.105
Manitt, C., Labelle-Dumais, C., Eng, C., Grant, A., Mimee, A., Stroh, T., et al. (2010). Peri-pubertal emergence of UNC-5 homologue expression by dopamine neurons in rodents. PLoS ONE 5, e11463. doi: 10.1371/journal.pone.0011463
Manitt, C., Mimee, A., Eng, C., Pokinko, M., Stroh, T., Cooper, H. M., et al. (2011). The netrin receptor DCC is required in the pubertal organization of mesocortical dopamine circuitry. J. Neurosci. Off. J. Soc. Neurosci. 31, 8381–8394. doi: 10.1523/JNEUROSCI.0606-11.2011
Mantz, J., Thierry, A. M., and Glowinski, J. (1989). Effect of noxious tail pinch on the discharge rate of mesocortical and mesolimbic dopamine neurons: selective activation of the mesocortical system. Brain Res. 476, 377–381. doi: 10.1016/0006-8993(89)91263-8
Marinelli, M., and McCutcheon, J. E. (2014). Heterogeneity of dopamine neuron activity across traits and states. Neuroscience 282, 176–197. doi: 10.1016/j.neuroscience.2014.07.034
Marshall, A. T., Liu, A. T., Murphy, N. P., Maidment, N. T., and Ostlund, S. B. (2017). Sex-specific enhancement of palatability-driven feeding in adolescent rats. PLoS ONE 12, e0180907. doi: 10.1371/journal.pone.0180907
Marshall, A. T., Munson, C. N., Maidment, N. T., and Ostlund, S. B. (2020). Reward-predictive cues elicit excessive reward seeking in adolescent rats. Dev. Cogn. Neurosci. 45, 100838. doi: 10.1016/j.dcn.2020.100838
Marsicano, G., and Lutz, B. (1999). Expression of the cannabinoid receptor CB1 in distinct neuronal subpopulations in the adult mouse forebrain. Eur. J. Neurosci. 11, 4213–4225. doi: 10.1046/j.1460-9568.1999.00847.x
Mastwal, S., Ye, Y., Ren, M., Jimenez, D. V., Martinowich, K., Gerfen, C. R., et al. (2014). Phasic dopamine neuron activity elicits unique mesofrontal plasticity in adolescence. J. Neurosci. 34, 9484–9496. doi: 10.1523/JNEUROSCI.1114-14.2014
Mateo, Y., Johnson, K. A., Covey, D. P., Atwood, B. K., Wang, H.-L., Zhang, S., et al. (2017). Endocannabinoid actions on cortical terminals orchestrate local modulation of dopamine release in the nucleus accumbens. Neuron 96, 1112–1126.e5. doi: 10.1016/j.neuron.2017.11.012
Matthews, M., Bondi, C., Torres, G., and Moghaddam, B. (2013). Reduced presynaptic dopamine activity in adolescent dorsal striatum. Neuropsychopharmacol. Off. Publ. Am. Coll. Neuropsychopharmacol. 38, 1344–1351. doi: 10.1038/npp.2013.32
McCane, A. M., Wegener, M. A., Faraji, M., Rivera-Garcia, M. T., Wallin-Miller, K. G., Costa, V. D., et al. (2021). Adolescent dopamine neurons represent reward differently during action and state guided learning. J. Neurosci. Off. J. Soc. Neurosci. 41, 9419–9430. doi: 10.1523/JNEUROSCI.1321-21.2021
McCutcheon, J. E. (2015). The role of dopamine in the pursuit of nutritional value. Physiol. Behav. 152, 408–415. doi: 10.1016/j.physbeh.2015.05.003
McCutcheon, J. E., Conrad, K. L., Carr, S. B., Ford, K. A., McGehee, D. S., and Marinelli, M. (2012). Dopamine neurons in the ventral tegmental area fire faster in adolescent rats than in adults. J. Neurophysiol. 108, 1620–1630. doi: 10.1152/jn.00077.2012
McCutcheon, J. E., and Marinelli, M. (2009). Age matters. Eur. J. Neurosci. 29, 997–1014. doi: 10.1111/j.1460-9568.2009.06648.x
McLaughlin, R. J., Hill, M. N., and Gorzalka, B. B. (2014). A critical role for prefrontocortical endocannabinoid signaling in the regulation of stress and emotional behavior. Neurosci. Biobehav. Rev. 42, 116–131. doi: 10.1016/j.neubiorev.2014.02.006
Mechoulam, R., Ben-Shabat, S., Hanus, L., Ligumsky, M., Kaminski, N. E., Schatz, A. R., et al. (1995). Identification of an endogenous 2-monoglyceride, present in canine gut, that binds to cannabinoid receptors. Biochem. Pharmacol. 50, 83–90. doi: 10.1016/0006-2952(95)00109-D
Melis, M., Pistis, M., Perra, S., Muntoni, A. L., Pillolla, G., and Gessa, G. L. (2004). Endocannabinoids mediate presynaptic inhibition of glutamatergic transmission in rat ventral tegmental area dopamine neurons through activation of CB1 receptors. J. Neurosci. Off. J. Soc. Neurosci. 24, 53–62. doi: 10.1523/JNEUROSCI.4503-03.2004
Meyer, H. C., Lee, F. S., and Gee, D. G. (2018). The role of the endocannabinoid system and genetic variation in adolescent brain development. Neuropsychopharmacology 43, 21–33. doi: 10.1038/npp.2017.143
Miech, R. A., Patrick, M. E., O'Malley, P. M., Johnston, L. D., and Bachman, J. G. (2020). Trends in reported marijuana vaping among US adolescents, 2017–2019. J. Am. Med. Assoc. 323, 475–476. doi: 10.1001/jama.2019.20185
Miller, E. K., and Cohen, J. D. (2001). An integrative theory of prefrontal cortex function. Annu. Rev. Neurosci. 24, 167–202. doi: 10.1146/annurev.neuro.24.1.167
Montague, P. R., Hyman, S. E., and Cohen, J. D. (2004). Computational roles for dopamine in behavioural control. Nature 431, 760–767. doi: 10.1038/nature03015
Morales, M., and Margolis, E. B. (2017). Ventral tegmental area: cellular heterogeneity, connectivity and behaviour. Nat. Rev. Neurosci. 18, 73–85. doi: 10.1038/nrn.2016.165
Mutlu, A. K., Schneider, M., Debban,é, M., Badoud, D., Eliez, S., and Schaer, M. (2013). Sex differences in thickness, and folding developments throughout the cortex. NeuroImage 82, 200–207. doi: 10.1016/j.neuroimage.2013.05.076
Nakazawa, K., Zsiros, V., Jiang, Z., Nakao, K., Kolata, S., Zhang, S., et al. (2012). GABAergic interneuron origin of schizophrenia pathophysiology. Neuropharmacology 62, 1574–1583. doi: 10.1016/j.neuropharm.2011.01.022
Naneix, F., Darlot, F., Coutureau, E., and Cador, M. (2016). Long-lasting deficits in hedonic and nucleus accumbens reactivity to sweet rewards by sugar overconsumption during adolescence. Eur. J. Neurosci. 43, 671–680. doi: 10.1111/ejn.13149
Naneix, F., Darlot, F., De Smedt-Peyrusse, V., Pape, J.-R., Coutureau, E., and Cador, M. (2018). Protracted motivational dopamine-related deficits following adolescence sugar overconsumption. Neuropharmacology 129, 16–25. doi: 10.1016/j.neuropharm.2017.11.021
Naneix, F., Marchand, A. R., Di Scala, G., Pape, J.-R., and Coutureau, E. (2012). Parallel maturation of goal-directed behavior and dopaminergic systems during adolescence. J. Neurosci. Off. J. Soc. Neurosci. 32, 16223–16232. doi: 10.1523/JNEUROSCI.3080-12.2012
Naneix, F., Marchand, A. R., Pichon, A., Pape, J.-R., and Coutureau, E. (2013). Adolescent stimulation of D2 receptors alters the maturation of dopamine-dependent goal-directed behavior. Neuropsychopharmacol. Off. Publ. Am. Coll. Neuropsychopharmacol. 38, 1566–1574. doi: 10.1038/npp.2013.55
Naneix, F., Tantot, F., Glangetas, C., Kaufling, J., Janthakhin, Y., Boitard, C., et al. (2017). Impact of early consumption of high-fat diet on the mesolimbic dopaminergic system. eNeuro 4, ENEURO.0120-17.2017. doi: 10.1523/ENEURO.0120-17.2017
Niv, Y. (2007). Cost, benefit, tonic, phasic: what do response rates tell us about dopamine and motivation? Ann. N. Y. Acad. Sci. 1104, 357–376. doi: 10.1196/annals.1390.018
Nolan, S. O., Zachry, J. E., Johnson, A. R., Brady, L. J., Siciliano, C. A., and Calipari, E. S. (2020). Direct dopamine terminal regulation by local striatal microcircuitry. J. Neurochem. 155, 475–493. doi: 10.1111/jnc.15034
Nomura, Y., Naitoh, F., and Segawa, T. (1976). Regional changes in monoamine content and uptake of the rat brain during postnatal development. Brain Res. 101, 305–315. doi: 10.1016/0006-8993(76)90271-7
O'Carroll, R. (2000). Cognitive impairment in schizophrenia. Adv. Psychiatr. Treat. 6, 161–168. doi: 10.1192/apt.6.3.161
O'Donnell, P. (2011). Adolescent onset of cortical disinhibition in schizophrenia: insights from animal models. Schizophr. Bull. 37, 484–492. doi: 10.1093/schbul/sbr028
Ogden, C. L., Carroll, M. D., Lawman, H. G., Fryar, C. D., Kruszon-Moran, D., Kit, B. K., et al. (2016). Trends in obesity prevalence among children and adolescents in the United States, 1988–1994 through 2013–2014. J. Am. Med. Assoc. 315, 2292–2299. doi: 10.1001/jama.2016.6361
Oleson, E. B., Beckert, M. V., Morra, J. T., Lansink, C. S., Cachope, R., Abdullah, R. A., et al. (2012). Endocannabinoids shape accumbal encoding of cue-motivated behavior via CB1 receptor activation in the ventral tegmentum. Neuron 73, 360–373. doi: 10.1016/j.neuron.2011.11.018
O'Shea, M., Singh, M. E., McGregor, I. S., and Mallet, P. E. (2004). Chronic cannabinoid exposure produces lasting memory impairment and increased anxiety in adolescent but not adult rats. J. Psychopharmacol. Oxf. Engl. 18, 502–508. doi: 10.1177/026988110401800407
Parish, C. L., Finkelstein, D. I., Drago, J., Borrelli, E., and Horne, M. K. (2001). The role of dopamine receptors in regulating the size of axonal arbors. J. Neurosci. 21, 5147–5157. doi: 10.1523/JNEUROSCI.21-14-05147.2001
Pattij, T., Wiskerke, J., and Schoffelmeer, A. N. M. (2008). Cannabinoid modulation of executive functions. Eur. J. Pharmacol. 585, 458–463. doi: 10.1016/j.ejphar.2008.02.099
Paulus, M. P. (2007). Decision-making dysfunctions in psychiatry–altered homeostatic processing? Science 318, 602–606. doi: 10.1126/science.1142997
Paus, T., Keshavan, M., and Giedd, J. N. (2008). Why do many psychiatric disorders emerge during adolescence? Nat. Rev. Neurosci. 9, 947–957. doi: 10.1038/nrn2513
Peters, K. Z., Cheer, J. F., and Tonini, R. (2021a). Modulating the neuromodulators: dopamine, serotonin, and the endocannabinoid system. Trends Neurosci. 44, 464–477. doi: 10.1016/j.tins.2021.02.001
Peters, K. Z., Oleson, E. B., and Cheer, J. F. (2021b). A brain on cannabinoids: the role of dopamine release in reward seeking and addiction. Cold Spring Harb. Perspect. Med. 11, a039305. doi: 10.1101/cshperspect.a039305
Peters, K. Z., Zlebnik, N. E., and Cheer, J. F. (2021c). “Chapter Three - Cannabis exposure during adolescence: a uniquely sensitive period for neurobiological effects,” in International Review of Neurobiology Effects of Peri-Adolescent Licit and Illicit Drug Use on the Developing CNS Part II, eds R. L. Bell and S. Rahman (Cambridge, MA: Academic Press), 95–120. doi: 10.1016/bs.irn.2021.07.002
Piscitelli, F., and Di Marzo, V. (2012). “Redundancy” of endocannabinoid inactivation: new challenges and opportunities for pain control. ACS Chem. Neurosci. 3, 356–363. doi: 10.1021/cn300015x
Pistis, M., Ferraro, L., Pira, L., Flore, G., Tanganelli, S., Gessa, G. L., et al. (2002). Δ9-Tetrahydrocannabinol decreases extracellular GABA and increases extracellular glutamate and dopamine levels in the rat prefrontal cortex: an in vivo microdialysis study. Brain Res. 948, 155–158. doi: 10.1016/S0006-8993(02)03055-X
Pitts, E. G., Stowe, T. A., Christensen, B. A., and Ferris, M. J. (2020). Comparing dopamine release, uptake, and D2 autoreceptor function across the ventromedial to dorsolateral striatum in adolescent and adult male and female rats. Neuropharmacology 175, 108163. doi: 10.1016/j.neuropharm.2020.108163
Privitera, G. J., Zavala, A. R., Sanabria, F., and Sotak, K. L. (2011). High fat diet intake during pre and periadolescence impairs learning of a conditioned place preference in adulthood. Behav. Brain Funct. 7, 21. doi: 10.1186/1744-9081-7-21
Rabasa, C., Winsa-Jörnulf, J., Vogel, H., Babaei, C. S., Askevik, K., and Dickson, S. L. (2016). Behavioral consequences of exposure to a high fat diet during the post-weaning period in rats. Horm. Behav. 85, 56–66. doi: 10.1016/j.yhbeh.2016.07.008
Rangel, A., Camerer, C., and Montague, P. R. (2008). A framework for studying the neurobiology of value-based decision making. Nat. Rev. Neurosci. 9, 545–556. doi: 10.1038/nrn2357
Raznahan, A., Shaw, P. W., Lerch, J. P., Clasen, L. S., Greenstein, D., Berman, R., et al. (2014). Longitudinal four-dimensional mapping of subcortical anatomy in human development. Proc. Natl. Acad. Sci. U. S. A. 111, 1592–1597. doi: 10.1073/pnas.1316911111
Reichelt, A. C. (2016). Adolescent maturational transitions in the prefrontal cortex and dopamine signaling as a risk factor for the development of obesity and high fat/high sugar diet induced cognitive deficits. Front. Behav. Neurosci. 10, 189. doi: 10.3389/fnbeh.2016.00189
Reichelt, A. C., Abbott, K. N., Westbrook, R. F., and Morris, M. J. (2016). Differential motivational profiles following adolescent sucrose access in male and female rats. Physiol. Behav. 157, 13–19. doi: 10.1016/j.physbeh.2016.01.038
Reichelt, A. C., Gibson, G. D., Abbott, K. N., and Hare, D. J. (2019). A high-fat high-sugar diet in adolescent rats impairs social memory and alters chemical markers characteristic of atypical neuroplasticity and parvalbumin interneuron depletion in the medial prefrontal cortex. Food Funct. 10, 1985–1998. doi: 10.1039/C8FO02118J
Reichelt, A. C., Killcross, S., Hambly, L. D., Morris, M. J., and Westbrook, R. F. (2015). Impact of adolescent sucrose access on cognitive control, recognition memory, and parvalbumin immunoreactivity. Learn. Mem. Cold Spring Harb. N 22, 215–224. doi: 10.1101/lm.038000.114
Reichelt, A. C., and Rank, M. M. (2017). The impact of junk foods on the adolescent brain. Birth Defects Res. 109, 1649–1658. doi: 10.1002/bdr2.1173
Renard, J., Krebs, M.-O., Jay, T. M., and Le Pen, G. (2013). Long-term cognitive impairments induced by chronic cannabinoid exposure during adolescence in rats: a strain comparison. Psychopharmacology 225, 781–790. doi: 10.1007/s00213-012-2865-z
Renard, J., Rushlow, W. J., and Laviolette, S. R. (2016). What can rats tell us about adolescent cannabis exposure? Insights from preclinical research. Can. J. Psychiatry Rev. Can. Psychiatr. 61, 328–334. doi: 10.1177/0706743716645288
Renard, J., Rushlow, W. J., and Laviolette, S. R. (2018). Effects of adolescent THC exposure on the prefrontal GABAergic system: implications for schizophrenia-related psychopathology. Front. Psychiatry 9, 281. doi: 10.3389/fpsyt.2018.00281
Renard, J., Szkudlarek, H. J., Kramar, C. P., Jobson, C. E. L., Moura, K., Rushlow, W. J., et al. (2017). Adolescent THC exposure causes enduring prefrontal cortical disruption of GABAergic inhibition and dysregulation of sub-cortical dopamine function. Sci. Rep. 7, 11420. doi: 10.1038/s41598-017-11645-8
Reynolds, L. M., and Flores, C. (2021). Mesocorticolimbic dopamine pathways across adolescence: diversity in development. Front. Neural Circuits 15, 735625. doi: 10.3389/fncir.2021.735625
Reynolds, L. M., Makowski, C. S., Yogendran, S. V., Kiessling, S., Cermakian, N., and Flores, C. (2015). Amphetamine in adolescence disrupts the development of medial prefrontal cortex dopamine connectivity in a DCC-dependent manner. Neuropsychopharmacology 40, 1101–1112. doi: 10.1038/npp.2014.287
Reynolds, L. M., Pokinko, M., Torres-Berrío, A., Cuesta, S., Lambert, L. C., Del Cid Pellitero, E., et al. (2018). DCC receptors drive prefrontal cortex maturation by determining dopamine axon targeting in adolescence. Biol. Psychiatry 83, 181–192. doi: 10.1016/j.biopsych.2017.06.009
Rice, M. E., Patel, J. C., and Cragg, S. J. (2011). Dopamine release in the basal ganglia. Neuroscience 198, 112–137. doi: 10.1016/j.neuroscience.2011.08.066
Riddle, R., and Pollock, J. D. (2003). Making connections: the development of mesencephalic dopaminergic neurons. Brain Res. Dev. Brain Res. 147, 3–21. doi: 10.1016/j.devbrainres.2003.09.010
Riegel, A. C., and Lupica, C. R. (2004). Independent presynaptic and postsynaptic mechanisms regulate endocannabinoid signaling at multiple synapses in the ventral tegmental area. J. Neurosci. 24, 11070–11078. doi: 10.1523/JNEUROSCI.3695-04.2004
Robertson, S. H., and Rasmussen, E. B. (2017). Effects of a cafeteria diet on delay discounting in adolescent and adult rats: alterations on dopaminergic sensitivity. J. Psychopharmacol. Oxf. Engl. 31, 1419–1429. doi: 10.1177/0269881117735750
Rose, J. E., and Woolsey, C. N. (1948). The orbitofrontal cortex and its connections with the mediodorsal nucleus in rabbit, sheep and cat. Res. Publ. Assoc. Res. Nerv. Ment. Dis. 27, 210–232.
Rosenberg, D. R., and Lewis, D. A. (1994). Changes in the dopaminergic innervation of monkey prefrontal cortex during late postnatal development: a tyrosine hydroxylase immunohistochemical study. Biol. Psychiatry 36, 272–277. doi: 10.1016/0006-3223(94)90610-6
Rubino, T., Prini, P., Piscitelli, F., Zamberletti, E., Trusel, M., Melis, M., et al. (2015). Adolescent exposure to THC in female rats disrupts developmental changes in the prefrontal cortex. Neurobiol. Dis. 73, 60–69. doi: 10.1016/j.nbd.2014.09.015
Rubino, T., Realini, N., Braida, D., Guidi, S., Capurro, V., Vigan,ò, D., et al. (2009). Changes in hippocampal morphology and neuroplasticity induced by adolescent THC treatment are associated with cognitive impairment in adulthood. Hippocampus 19, 763–772. doi: 10.1002/hipo.20554
Rubino, T., Zamberletti, E., and Parolaro, D. (2012). Adolescent exposure to cannabis as a risk factor for psychiatric disorders. J. Psychopharmacol. Oxf. Engl. 26, 177–188. doi: 10.1177/0269881111405362
Salamone, J. D., and Correa, M. (2012). The mysterious motivational functions of mesolimbic dopamine. Neuron 76, 470–485. doi: 10.1016/j.neuron.2012.10.021
Sallam, N. A., and Borgland, S. L. (2021). Insulin and endocannabinoids in the mesolimbic system. J. Neuroendocrinol. 33, e12965. doi: 10.1111/jne.12965
Sandoval-Salazar, C., Ramírez-Emiliano, J., Trejo-Bahena, A., Oviedo-Solís, C. I., and Solís-Ortiz, M. S. (2016). A high-fat diet decreases GABA concentration in the frontal cortex and hippocampus of rats. Biol. Res. 49, 15. doi: 10.1186/s40659-016-0075-6
Scherma, M., Dess,ì, C., Muntoni, A. L., Lecca, S., Satta, V., Luchicchi, A., et al. (2016). Adolescent Δ(9)-tetrahydrocannabinol exposure alters WIN55,212-2 self-administration in adult rats. Neuropsychopharmacol. Off. Publ. Am. Coll. Neuropsychopharmacol. 41, 1416–1426. doi: 10.1038/npp.2015.295
Schneider, M. (2008). Puberty as a highly vulnerable developmental period for the consequences of cannabis exposure. Addict. Biol. 13, 253–263. doi: 10.1111/j.1369-1600.2008.00110.x
Schneider, M. (2013). Adolescence as a vulnerable period to alter rodent behavior. Cell Tissue Res. 354, 99–106. doi: 10.1007/s00441-013-1581-2
Schneider, M., and Koch, M. (2003). Chronic pubertal, but not adult chronic cannabinoid treatment impairs sensorimotor gating, recognition memory, and the performance in a progressive ratio task in adult rats. Neuropsychopharmacol. Off. Publ. Am. Coll. Neuropsychopharmacol. 28, 1760–1769. doi: 10.1038/sj.npp.1300225
Schneider, M., Schömig, E., and Leweke, F. M. (2008). Acute and chronic cannabinoid treatment differentially affects recognition memory and social behavior in pubertal and adult rats. Addict. Biol. 13, 345–357. doi: 10.1111/j.1369-1600.2008.00117.x
Schultz, W. (2007). Multiple dopamine functions at different time courses. Annu. Rev. Neurosci. 30, 259–288. doi: 10.1146/annurev.neuro.28.061604.135722
Seamans, J. K., and Yang, C. R. (2004). The principal features and mechanisms of dopamine modulation in the prefrontal cortex. Prog. Neurobiol. 74, 1–58. doi: 10.1016/j.pneurobio.2004.05.006
Sesack, S. R., Hawrylak, V. A., Matus, C., Guido, M. A., and Levey, A. I. (1998). Dopamine axon varicosities in the prelimbic division of the rat prefrontal cortex exhibit sparse immunoreactivity for the dopamine transporter. J. Neurosci. Off. J. Soc. Neurosci. 18, 2697–2708. doi: 10.1523/JNEUROSCI.18-07-02697.1998
Shansky, R. M., and Murphy, A. Z. (2021). Considering sex as a biological variable will require a global shift in science culture. Nat. Neurosci. 24, 457–464. doi: 10.1038/s41593-021-00806-8
Simon, N. W., and Moghaddam, B. (2014). Neural processing of reward in adolescent rodents. Dev. Cogn. Neurosci. 11, 145–154. doi: 10.1016/j.dcn.2014.11.001
Somerville, L. H., and Casey, B. J. (2010). Developmental neurobiology of cognitive control and motivational systems. Curr. Opin. Neurobiol. 20, 236–241. doi: 10.1016/j.conb.2010.01.006
Sonntag, K. C., Brenhouse, H. C., Freund, N., Thompson, B. S., Puhl, M., and Andersen, S. L. (2014). Viral over-expression of D1 dopamine receptors in the prefrontal cortex increase high-risk behaviors in adults: comparison with adolescents. Psychopharmacology 231, 1615–1626. doi: 10.1007/s00213-013-3399-8
South, T., and Huang, X.-F. (2008). High-fat diet exposure increases dopamine D2 receptor and decreases dopamine transporter receptor binding density in the nucleus accumbens and caudate putamen of mice. Neurochem. Res. 33, 598–605. doi: 10.1007/s11064-007-9483-x
Spear, L. P. (2000). The adolescent brain and age-related behavioral manifestations. Neurosci. Biobehav. Rev. 24, 417–463. doi: 10.1016/S0149-7634(00)00014-2
St Onge, J. R., Ahn, S., Phillips, A. G., and Floresco, S. B. (2012). Dynamic fluctuations in dopamine efflux in the prefrontal cortex and nucleus accumbens during risk-based decision making. J. Neurosci. Off. J. Soc. Neurosci. 32, 16880–16891. doi: 10.1523/JNEUROSCI.3807-12.2012
Steele, C. C., Pirkle, J. R. A., Davis, I. R., and Kirkpatrick, K. (2019). Dietary effects on the determinants of food choice: impulsive choice, discrimination, incentive motivation, preference, and liking in male rats. Appetite 136, 160–172. doi: 10.1016/j.appet.2019.01.023
Steinberg, L. (2005). Cognitive and affective development in adolescence. Trends Cogn. Sci. 9, 69–74. doi: 10.1016/j.tics.2004.12.005
Sturman, D. A., Mandell, D. R., and Moghaddam, B. (2010). Adolescents exhibit behavioral differences from adults during instrumental learning and extinction. Behav. Neurosci. 124, 16–25. doi: 10.1037/a0018463
Sturman, D. A., and Moghaddam, B. (2011). The Neurobiology of Adolescence: changes in brain architecture, functional dynamics, and behavioral tendencies. Neurosci. Biobehav. Rev. 35, 1704–1712. doi: 10.1016/j.neubiorev.2011.04.003
Sturman, D. A., and Moghaddam, B. (2012). Striatum processes reward differently in adolescents versus adults. Proc. Natl. Acad. Sci. U. S. A. 109, 1719–1724. doi: 10.1073/pnas.1114137109
Sugiura, T., Kishimoto, S., Oka, S., and Gokoh, M. (2006). Biochemistry, pharmacology and physiology of 2-arachidonoylglycerol, an endogenous cannabinoid receptor ligand. Prog. Lipid Res. 45, 405–446. doi: 10.1016/j.plipres.2006.03.003
Sugiura, T., Kondo, S., Sukagawa, A., Nakane, S., Shinoda, A., Itoh, K., et al. (1995). 2-Arachidonoylglycerol: a possible endogenous cannabinoid receptor ligand in brain. Biochem. Biophys. Res. Commun. 215, 89–97. doi: 10.1006/bbrc.1995.2437
Surmeier, D. J., Carrillo-Reid, L., and Bargas, J. (2011). Dopaminergic modulation of striatal neurons, circuits and assemblies. Neuroscience 198, 3–18. doi: 10.1016/j.neuroscience.2011.08.051
Szabo, B., Siemes, S., and Wallmichrath, I. (2002). Inhibition of GABAergic neurotransmission in the ventral tegmental area by cannabinoids. Eur. J. Neurosci. 15, 2057–2061. doi: 10.1046/j.1460-9568.2002.02041.x
Taber, M. T., Das, S., and Fibiger, H. C. (1995). Cortical regulation of subcortical dopamine release: mediation via the ventral tegmental area. J. Neurochem. 65, 1407–1410. doi: 10.1046/j.1471-4159.1995.65031407.x
Tantot, F., Parkes, S. L., Marchand, A. R., Boitard, C., Naneix, F., Layé, S., et al. (2017). The effect of high-fat diet consumption on appetitive instrumental behavior in rats. Appetite 108, 203–211. doi: 10.1016/j.appet.2016.10.001
Tarazi, F. I., and Baldessarini, R. J. (2000). Comparative postnatal development of dopamine D(1), D(2) and D(4) receptors in rat forebrain. Int. J. Dev. Neurosci. Off. J. Int. Soc. Dev. Neurosci. 18, 29–37. doi: 10.1016/S0736-5748(99)00108-2
Teegarden, S. L., Scott, A. N., and Bale, T. L. (2009). Early life exposure to a high fat diet promotes long-term changes in dietary preferences and central reward signaling. Neuroscience 162, 924–932. doi: 10.1016/j.neuroscience.2009.05.029
Tiklová, K., Björklund, Å. K., Lahti, L., Fiorenzano, A., Nolbrant, S., Gillberg, L., et al. (2019). Single-cell RNA sequencing reveals midbrain dopamine neuron diversity emerging during mouse brain development. Nat. Commun. 10, 581. doi: 10.1038/s41467-019-08453-1
Tirado-Muñoz, J., Lopez-Rodriguez, A. B., Fonseca, F., Farré, M., Torrens, M., and Viveros, M.-P. (2020). Effects of cannabis exposure in the prenatal and adolescent periods: preclinical and clinical studies in both sexes. Front. Neuroendocrinol. 57, 100841. doi: 10.1016/j.yfrne.2020.100841
Tsan, L., Décarie-Spain, L., Noble, E. E., and Kanoski, S. E. (2021). Western diet consumption during development: setting the stage for neurocognitive dysfunction. Front. Neurosci. 15, 632312. doi: 10.3389/fnins.2021.632312
Tseng, K.-Y., and O'Donnell, P. (2007). Dopamine modulation of prefrontal cortical interneurons changes during adolescence. Cereb. Cortex N. Y. N. 17, 1235–1240. doi: 10.1093/cercor/bhl034
Tseng, K. Y., Mallet, N., Toreson, K. L., Le Moine, C., Gonon, F., and O'Donnell, P. (2006). Excitatory response of prefrontal cortical fast-spiking interneurons to ventral tegmental area stimulation in vivo. Synap. N. Y. N. 59, 412–417. doi: 10.1002/syn.20255
Tseng, K. Y., and O'Donnell, P. (2005). Post-pubertal emergence of prefrontal cortical up states induced by D1-NMDA co-activation. Cereb. Cortex N. Y. N. 15, 49–57. doi: 10.1093/cercor/bhh107
Turner, K. M., and Parkes, S. L. (2020). Prefrontal regulation of behavioural control: evidence from learning theory and translational approaches in rodents. Neurosci. Biobehav. Rev. 118, 27–41. doi: 10.1016/j.neubiorev.2020.07.010
Ueda, N., and Tsuboi, K. (2012). Discrimination between two endocannabinoids. Chem. Biol. 19, 545–547. doi: 10.1016/j.chembiol.2012.05.001
Ueda, N., Tsuboi, K., and Uyama, T. (2010). Enzymological studies on the biosynthesis of N-acylethanolamines. Biochim. Biophys. Acta 1801, 1274–1285. doi: 10.1016/j.bbalip.2010.08.010
Urbonaite, G., Knyzeliene, A., Bunn, F. S., Smalskys, A., and Neniskyte, U. (2022). The impact of maternal high-fat diet on offspring neurodevelopment. Front. Neurosci. 16, 909762. doi: 10.3389/fnins.2022.909762
Urs, N. M., Gee, S. M., Pack, T. F., McCorvy, J. D., Evron, T., Snyder, J. C., et al. (2016). Distinct cortical and striatal actions of a β-arrestin-biased dopamine D2 receptor ligand reveal unique antipsychotic-like properties. Proc. Natl. Acad. Sci. U. S. A. 113, E8178–E8186. doi: 10.1073/pnas.1614347113
Uylings, H. B. M., Groenewegen, H. J., and Kolb, B. (2003). Do rats have a prefrontal cortex? Behav. Brain Res. 146, 3–17. doi: 10.1016/j.bbr.2003.09.028
Van den Heuvel, D. M. A., and Pasterkamp, R. J. (2008). Getting connected in the dopamine system. Prog. Neurobiol. 85, 75–93. doi: 10.1016/j.pneurobio.2008.01.003
Van Eden, C. G., Hoorneman, E. M. D., Buijs, R. M., Matthijssen, M. A. H., Geffard, M., and Uylings, H. B. M. (1987). Immunocytochemical localization of dopamine in the prefrontal cortex of the rat at the light and electron microscopical level. Neuroscience 22, 849–862. doi: 10.1016/0306-4522(87)92964-2
Vander Weele, C. M., Siciliano, C. A., Matthews, G. A., Namburi, P., Izadmehr, E. M., Espinel, I. C., et al. (2018). Dopamine enhances signal-to-noise ratio in cortical-brainstem encoding of aversive stimuli. Nature 563, 397–401. doi: 10.1038/s41586-018-0682-1
Vendruscolo, L. F., Gueye, A. B., Darnaudéry, M., Ahmed, S. H., and Cador, M. (2010a). Sugar overconsumption during adolescence selectively alters motivation and reward function in adult rats. PLoS ONE 5, e9296. doi: 10.1371/journal.pone.0009296
Vendruscolo, L. F., Gueye, A. B., Vendruscolo, J. C. M., Clemens, K. J., Mormède, P., Darnaudéry, M., et al. (2010b). Reduced alcohol drinking in adult rats exposed to sucrose during adolescence. Neuropharmacology 59, 388–394. doi: 10.1016/j.neuropharm.2010.05.015
Vertes, R. P. (2004). Differential projections of the infralimbic and prelimbic cortex in the rat. Synap. N. Y. N. 51, 32–58. doi: 10.1002/syn.10279
Volkow, N. D., Wang, G.-J., and Baler, R. D. (2011). Reward, dopamine and the control of food intake: implications for obesity. Trends Cogn. Sci. 15, 37–46. doi: 10.1016/j.tics.2010.11.001
Voorn, P., Kalsbeek, A., Jorritsma-Byham, B., and Groenewegen, H. J. (1988). The pre- and postnatal development of the dopaminergic cell groups in the ventral mesencephalon and the dopaminergic innervation of the striatum of the rat. Neuroscience 25, 857–887. doi: 10.1016/0306-4522(88)90041-3
Voorn, P., Vanderschuren, L. J. M. J., Groenewegen, H. J., Robbins, T. W., and Pennartz, C. M. A. (2004). Putting a spin on the dorsal-ventral divide of the striatum. Trends Neurosci. 27, 468–474. doi: 10.1016/j.tins.2004.06.006
Walker, D. M., Bell, M. R., Flores, C., Gulley, J. M., Willing, J., and Paul, M. J. (2017). Adolescence and reward: making sense of neural and behavioral changes amid the chaos. J. Neurosci. Off. J. Soc. Neurosci. 37, 10855–10866. doi: 10.1523/JNEUROSCI.1834-17.2017
Wang, H., Treadway, T., Covey, D. P., Cheer, J. F., and Lupica, C. R. (2015). Cocaine-induced endocannabinoid mobilization in the ventral tegmental area. Cell Rep. 12, 1997–2008. doi: 10.1016/j.celrep.2015.08.041
Wang, Y. C., Bleich, S. N., and Gortmaker, S. L. (2008). Increasing caloric contribution from sugar-sweetened beverages and 100% fruit juices among US children and adolescents, 1988–2004. Pediatrics 121, e1604–e1614. doi: 10.1542/peds.2007-2834
Wickens, J. R., Horvitz, J. C., Costa, R. M., and Killcross, S. (2007). Dopaminergic mechanisms in actions and habits. J. Neurosci. Off. J. Soc. Neurosci. 27, 8181–8183. doi: 10.1523/JNEUROSCI.1671-07.2007
Willing, J., Cortes, L. R., Brodsky, J. M., Kim, T., and Juraska, J. M. (2017). Innervation of the medial prefrontal cortex by tyrosine hydroxylase immunoreactive fibers during adolescence in male and female rats. Dev. Psychobiol. 59, 583–589. doi: 10.1002/dev.21525
Wilmouth, C. E., and Spear, L. P. (2009). Hedonic sensitivity in adolescent and adult rats: taste reactivity and voluntary sucrose consumption. Pharmacol. Biochem. Behav. 92, 566–573. doi: 10.1016/j.pbb.2009.02.009
Wilson, R. I., and Nicoll, R. A. (2001). Endogenous cannabinoids mediate retrograde signalling at hippocampal synapses. Nature 410, 588–592. doi: 10.1038/35069076
Winstanley, C. A., Eagle, D. M., and Robbins, T. W. (2006). Behavioral models of impulsivity in relation to ADHD: translation between clinical and preclinical studies. Clin. Psychol. Rev. 26, 379–395. doi: 10.1016/j.cpr.2006.01.001
Wong, A., Dogra, V. R., and Reichelt, A. C. (2017). High-sucrose diets in male rats disrupt aspects of decision making tasks, motivation and spatial memory, but not impulsivity measured by operant delay-discounting. Behav. Brain Res. 327, 144–154. doi: 10.1016/j.bbr.2017.03.029
Yee, B. K., Keist, R., von Boehmer, L., Studer, R., Benke, D., Hagenbuch, N., et al. (2005). A schizophrenia-related sensorimotor deficit links alpha 3-containing GABAA receptors to a dopamine hyperfunction. Proc. Natl. Acad. Sci. U. S. A. 102, 17154–17159. doi: 10.1073/pnas.0508752102
Yuan, M., Cross, S. J., Loughlin, S. E., and Leslie, F. M. (2015). Nicotine and the adolescent brain. J. Physiol. 593, 3397–3412. doi: 10.1113/JP270492
Zachry, J. E., Nolan, S. O., Brady, L. J., Kelly, S. J., Siciliano, C. A., and Calipari, E. S. (2021). Sex differences in dopamine release regulation in the striatum. Neuropsychopharmacol. Off. Publ. Am. Coll. Neuropsychopharmacol. 46, 491–499. doi: 10.1038/s41386-020-00915-1
Zhang, H.-Y., Gao, M., Liu, Q.-R., Bi, G.-H., Li, X., Yang, H.-J., et al. (2014). Cannabinoid CB2 receptors modulate midbrain dopamine neuronal activity and dopamine-related behavior in mice. Proc. Natl. Acad. Sci. U. S. A. 111, E5007–E5015. doi: 10.1073/pnas.1413210111
Zimmerman, C. A., and Knight, Z. A. (2020). Layers of signals that regulate appetite. Curr. Opin. Neurobiol. 64, 79–88. doi: 10.1016/j.conb.2020.03.007
Zlebnik, N. E., and Cheer, J. F. (2016). Drug-induced alterations of endocannabinoid-mediated plasticity in brain reward regions. J. Neurosci. 36, 10230–10238. doi: 10.1523/JNEUROSCI.1712-16.2016
Keywords: prefrontal cortex (PFC), adolescence, dopamine, endocannabinoid, reward, neuromodulators
Citation: Peters KZ and Naneix F (2022) The role of dopamine and endocannabinoid systems in prefrontal cortex development: Adolescence as a critical period. Front. Neural Circuits 16:939235. doi: 10.3389/fncir.2022.939235
Received: 08 May 2022; Accepted: 14 October 2022;
Published: 01 November 2022.
Edited by:
Cecilia Flores, McGill University, CanadaReviewed by:
Kuei Y. Tseng, University of Illinois at Chicago, United StatesCopyright © 2022 Peters and Naneix. This is an open-access article distributed under the terms of the Creative Commons Attribution License (CC BY). The use, distribution or reproduction in other forums is permitted, provided the original author(s) and the copyright owner(s) are credited and that the original publication in this journal is cited, in accordance with accepted academic practice. No use, distribution or reproduction is permitted which does not comply with these terms.
*Correspondence: Fabien Naneix, ZmFiaWVuLm5hbmVpeEBhYmRuLmFjLnVr
Disclaimer: All claims expressed in this article are solely those of the authors and do not necessarily represent those of their affiliated organizations, or those of the publisher, the editors and the reviewers. Any product that may be evaluated in this article or claim that may be made by its manufacturer is not guaranteed or endorsed by the publisher.
Research integrity at Frontiers
Learn more about the work of our research integrity team to safeguard the quality of each article we publish.