- Institute of Physiology and Pathophysiology, Medical Biophysics, Heidelberg University, Heidelberg, Germany
Large portions of the thalamus receive strong driving input from cortical layer 5 (L5) neurons but the role of this important pathway in cortical and thalamic computations is not well understood. L5-recipient “higher-order” thalamic regions participate in cortico-thalamo-cortical (CTC) circuits that are increasingly recognized to be (1) anatomically and functionally distinct from better-studied “first-order” CTC networks, and (2) integral to cortical activity related to learning and perception. Additionally, studies are beginning to elucidate the clinical relevance of these networks, as dysfunction across these pathways have been implicated in several pathological states. In this review, we highlight recent advances in understanding L5 CTC networks across sensory modalities and brain regions, particularly studies leveraging cell-type-specific tools that allow precise experimental access to L5 CTC circuits. We aim to provide a focused and accessible summary of the anatomical, physiological, and computational properties of L5-originating CTC networks, and outline their underappreciated contribution in pathology. We particularly seek to connect single-neuron and synaptic properties to network (dys)function and emerging theories of cortical computation, and highlight information processing in L5 CTC networks as a promising focus for computational studies.
Introduction
The thalamus is a bilateral structure of the diencephalon that serves integral roles in a significant range of neurophysiological functions including sensory information relay, learning and memory, motor control, and regulation of sleep and wakefulness (Herrero et al., 2002; Yuan et al., 2016). Positioned above the midbrain, the thalamus displays widespread connectivity with the cerebral cortex, as well as subcortical and temporal structures (e.g., mammillary bodies, fornix, and hippocampus), acting as a central hub for functional brain networks (Hwang et al., 2017). The thalamus is traditionally fractionated into functional nuclei, each of which participates in feedback and/or feedforward communication with unique cortical areas (Morel et al., 1997; Yuan et al., 2016; Halassa and Kastner, 2017).
The thalamus is massively innervated by deep corticofugal cells in layer 5 (L5) and layer 6 (L6) (Figure 1). While these dense projections have been documented since the early 20th century (Cajal, 1906), in-depth study of the pathways and their functional impact on the thalamus has only recently become experimentally accessible (Guy and Staiger, 2017). Indeed, technological advances in high-yield electrophysiology as well as cell-type-specific optogenetics and anatomical tracing have elucidated interactions between specific cortical layers and thalamic nuclei (Luo et al., 2018). In particular, such approaches have refined our understanding of “higher-order” (HO) thalamic nuclei – those regions of the thalamus that are strongly innervated by cortical L5 pyramidal neurons (Sherman, 2007; Figure 1A). However, this understanding still lags that of “first-order” (FO) thalamic nuclei (Figure 1B), which do not receive L5 input and have proven more tractable with typical sensory physiological experiments.
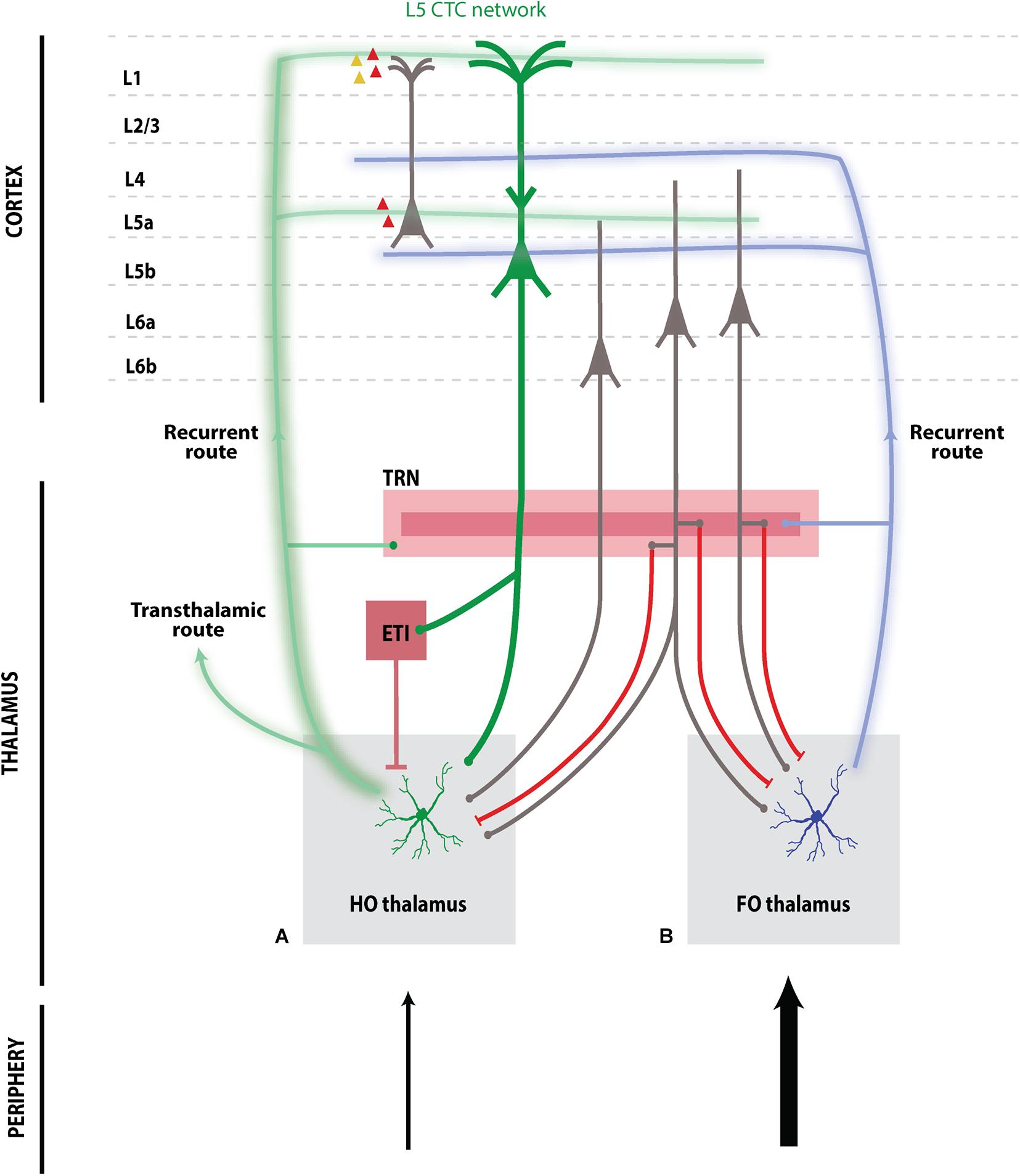
Figure 1. Schematic of higher-order (A) and first-order (B) cortico-thalamo-cortical networks. L5 thick-tufted pyramidal cells in L5B send projections to HO thalamic nuclei and to additional subcortical targets including extrathalamic inhibitory (ETI) sources, e.g., the ZI and APT, which exert strong inhibition on HO nuclei. HO thalamus (A) sends TC projections across transthalamic routes to other cortical regions, or forms a recurrent route back to the same cortical region targeting L5A and upper layers. HO TC projections target excitatory pyramidal cells, as well as PV (red) and 5HT3-positive inhibitory (yellow) interneurons, although there are modality-specific variations (e.g., associative thalamus). In contrast, FO thalamus (B) sends recurrent TC projections to cortical layers L5B and L4. L6 corticothalamic cell subpopulations display distinct connectivity with thalamic nuclei: Upper L6A cells specifically target FO thalamus, while lower L6A cells target both FO and HO thalamus; both populations send collaterals to inhibitory TRN. L6B cells specifically target HO thalamus but do not send collaterals to TRN. Both HO and FO nuclei engage intrathalamic inhibitory feedback loops via excitatory projections to TRN. Outer “shell” TRN sends inhibitory projections to HO thalamus while inner “core” TRN sends inhibitory projections to FO thalamus.
A recent study provides beginning evidence that L5-HO thalamus connectivity may represent a “default” scheme: while each sensory modality (e.g., somatosensation, vision, and audition) retains a distinct FO and HO nucleus, FO and HO nuclei are each genetically homologous across modalities. Intriguingly, the transcriptional identity of FO nuclei depends on the presence of peripheral input, as neonatal ablation of these inputs leads to FO nuclei transitioning to a HO transcriptional program and descending cortical input that is typically HO-directed (Frangeul et al., 2016). In addition, recent studies have highlighted the importance of L5-originating CTC networks in diverse functions, such as permitting selective thalamic-driven modulation, dynamic cortical coupling, sending motor instructions, and informing higher cortical areas about which motor instructions have been issued, as well as roles in learning and plasticity in cortical networks (Sherman and Guillery, 2011; Gambino et al., 2014; Audette et al., 2019; Usrey and Sherman, 2019). However, a synthesized view of HO CTC computation is still elusive, given the diverse nonlinear single-neuron and synaptic properties of these networks, and complex connectivity with other subcortical regions.
In this focused review, we discuss foundational and recent studies of L5-originating HO CTC networks across cortical regions. In particular, we emphasize findings distinguishing HO CTC networks from better-studied FO regions of thalamus that serve as bottom-up relays to the cortex. Many excellent existing reviews summarize the recent rapid progress in understanding anatomical and functional properties of CTC networks (e.g., Usrey and Sherman, 2019; Shepherd and Yamawaki, 2021), particularly contrasting FO and HO CTC connectivity. For context, we review this material but focus mainly on studies relevant to understanding computation within HO CTC networks as well as the emerging importance of these networks in learning and pathology.
Our aim is to present a unified yet comprehensible overview of these increasingly appreciated circuits that is accessible to experimental and computational neuroscientists from outside the thalamocortical field. The schematic in Figure 1 outlines the networks of interest and Figure 2 illustrates available information on synaptic inputs to HO thalamus. Key literature is organized according to modality and methodology (Table 1) and relevance to pathology (Table 2). This review is structured as follows:
1. L5-HO thalamus definitions and network connectivity schemes.
2. HO thalamic nuclei across modalities.
3. Inhibitory control of HO thalamus.
4. Synaptic properties of L5-HO thalamus projections.
5. HO thalamus intrinsic properties and single-cell information processing.
6. HO encoding of L5 cortical information.
7. HO thalamocortical innervation of cortex and roles in sensory processing and cognition.
8. Clinical relevance of L5-originating CTC networks.
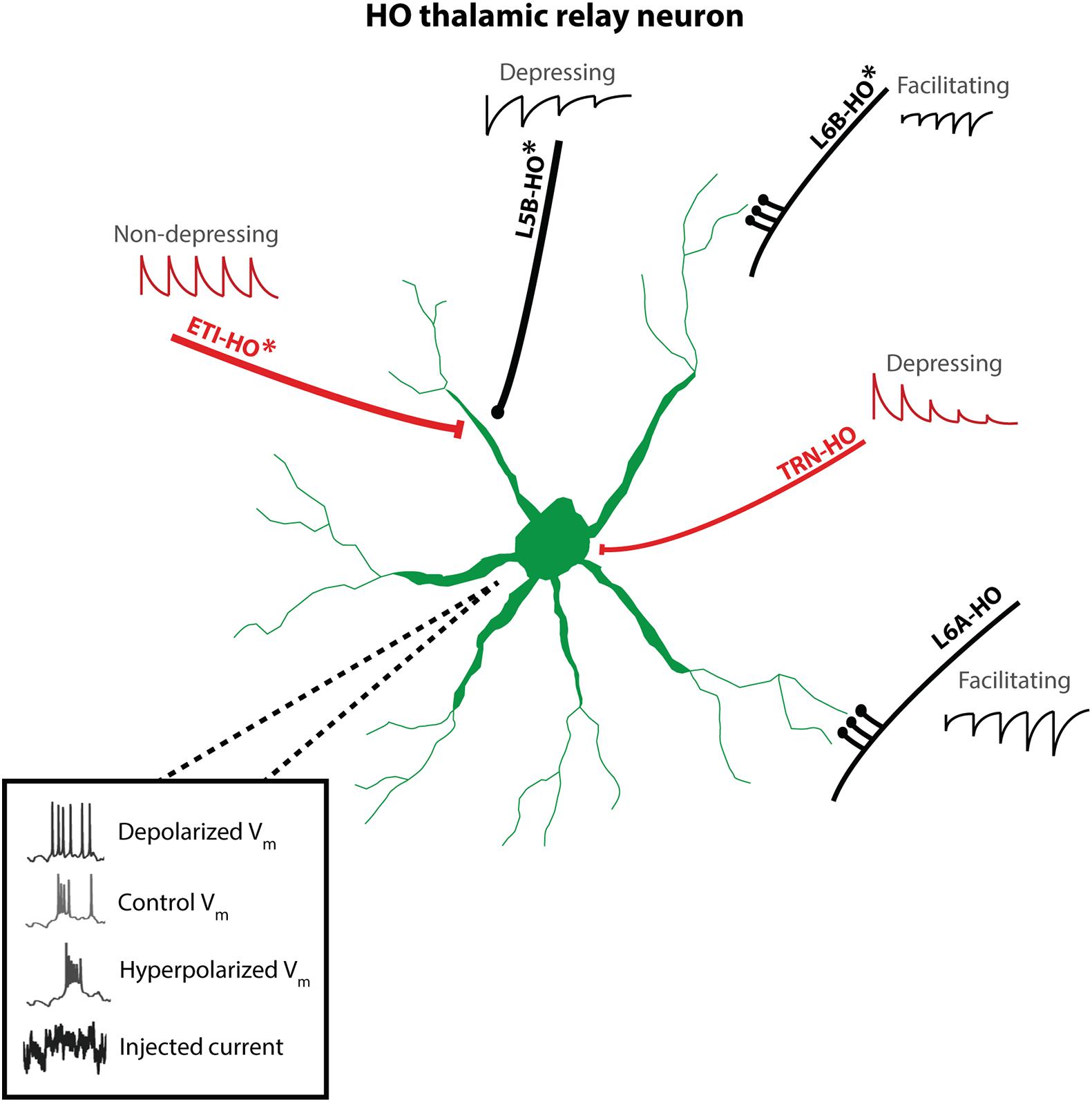
Figure 2. Cartoon of synaptic inputs to HO thalamic relay neurons and intrinsic excitability patterns. Asterisks (*) indicate inputs which target HO thalamus and are not present in FO nuclei. L5tt neurons provide strong, depressing “driving” excitatory input to proximal dendrites via large synapses, while L6A CT en passant synapses provide relatively weaker, facilitating, modulatory excitatory input to distal dendrites. L6B provides en passant excitatory inputs (synaptic dynamics not yet reported). Inhibitory TRN inputs show strong depression. In contrast, powerful inhibitory ETI synapses are located in close proximity to L5tt excitatory inputs and show little depression. Not shown: additional area-specific sources of driver input reported in “convergence” zones of HO thalamus and feedforward inhibitory loops L5tt-ETI-thalamus and L6A-TRN-thalamus. Inset voltage traces: HO thalamic neurons show characteristic voltage-dependent bursting: depolarization inactivates bursting mechanisms and promotes tonic spiking.
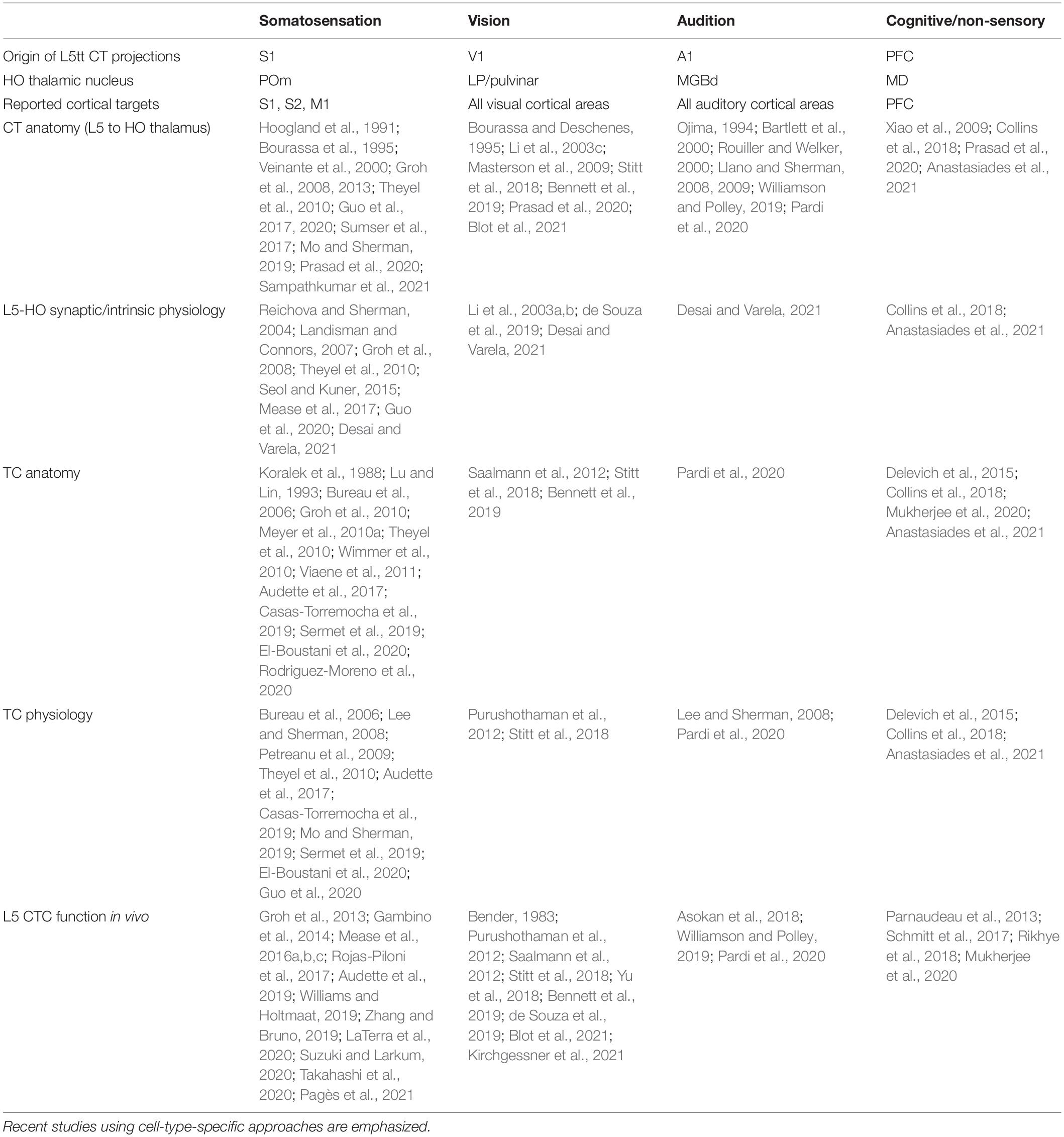
Table 1. Key literature describing anatomical and/or physiological properties of CTC networks across sensory modalities and an example non-sensory modality.
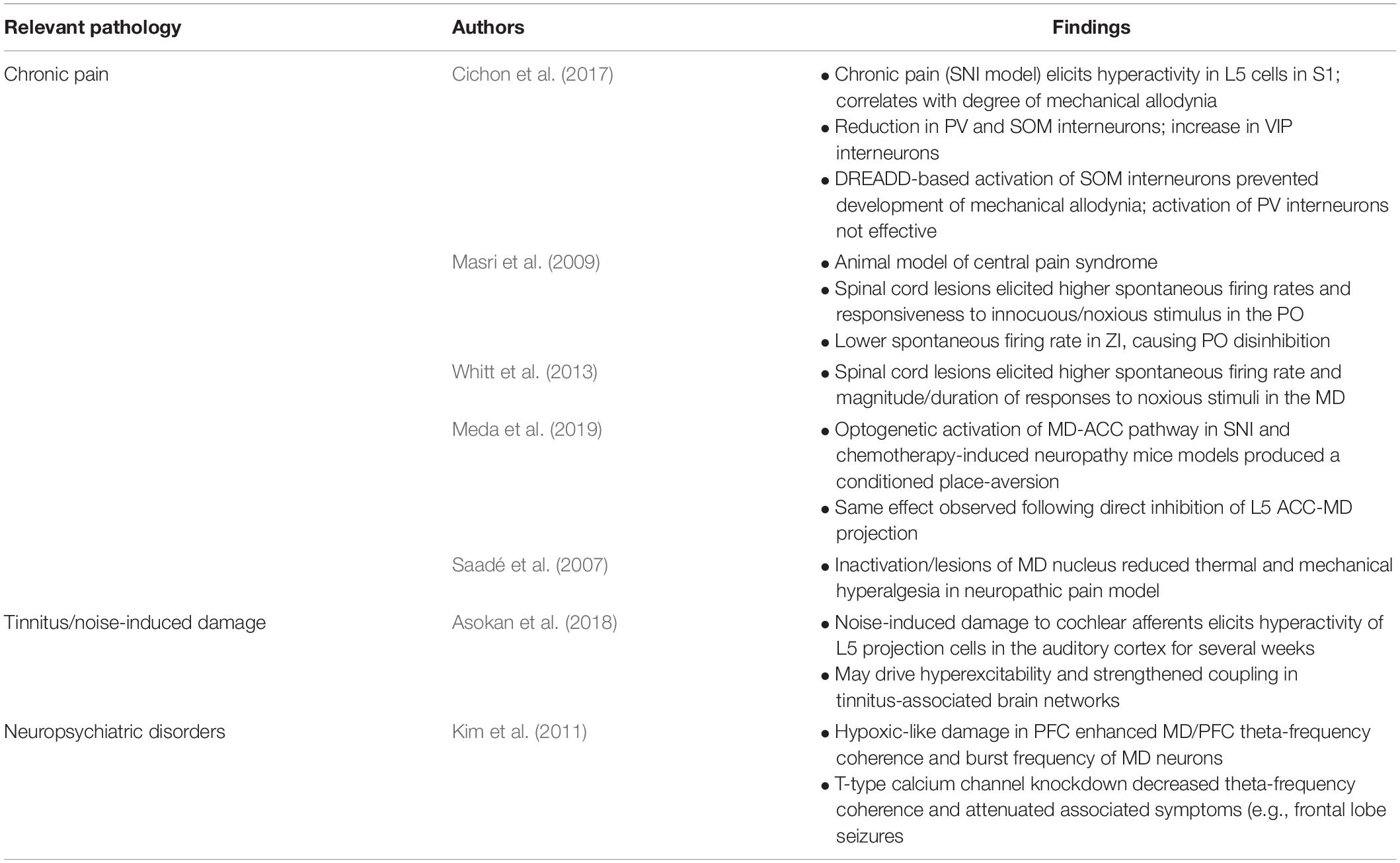
Table 2. Summary of key literature findings on the relevance of higher-order CTC pathway components across pathological states including pain, tinnitus, and neuropsychiatric disorders.
Throughout, we attempt to highlight missing experimental data and theoretical perspectives.
L5 to Higher-Order Thalamus: Corticothalamic Connectivity From the Pyramidal Tract
Pyramidal neurons in L5 of the cortex serve as the major exit point by which cortical signals are directed to subcortical circuits. Aside from L6 corticothalamic (CT) neurons, only L5 neurons are reported to make synapses outside of the neocortex; in contrast to the L6A CT pathway which is restricted largely to thalamus, L5 neurons project to several subcortical targets, including thalamus, superior colliculus, pons, brainstem, and spinal cord. Despite its complexity and computational power, these L5- and L6-originating outputs represent the only means by which the cortex can influence subcortical processes and thereby influence behavior (Sherman and Guillery, 2013; Usrey and Sherman, 2019; Prasad et al., 2020; Sherman and Usrey, 2021). Indeed, innervation by L5 driving inputs is the criterion for the influential and useful “higher-order” terminology introduced by Sherman and colleagues. Presently, several L5-originating CTC networks have been identified across sensory modalities including somatosensation, audition, and vision (Sherman, 2016; Table 1).
Recently, the development of novel transgenic mouse lines has granted refined experimental access to cell-type- and layer-specific observations (Gong et al., 2007; Gerfen et al., 2013; Harris et al., 2014, 2018; Daigle et al., 2018). In conjunction, advancements in optogenetics and electrophysiology have enabled targeted monitoring and manipulation of neuronal circuits, and dissection of physiological and functional circuit properties. These powerful approaches have begun to disentangle the properties of L5-HO thalamus pathways from L6-thalamus pathways, for example, through the use of Rbp4, Npr3, and thy-1 mouse lines (Groh et al., 2013; Daigle et al., 2018; Guo et al., 2020; Prasad et al., 2020; Kirchgessner et al., 2021), establishing key characteristics of L5 projections to the thalamus. Most importantly, L5 inputs to HO nuclei are sparse and display characteristic “driver” properties, in contrast to L6 projections, which target both HO and FO thalamic nuclei and provide modulatory input (Sherman and Guillery, 2002; Reichova and Sherman, 2004; Sherman, 2016).
Higher-order thalamic nuclei are specifically targeted by L5 “thick-tufted” (L5tt) pyramidal tract neurons in L5B (Harris and Shepherd, 2015; Figure 1A, green). As recipients of information from all cortical layers, L5tt neurons are well-suited for integrating cortical signals–particularly characteristic are the elaborately branched apical dendrites that cover nearly a column in width. Notably, L5tt neurons show minimal axonal branching within the cortex, suggesting a main role is to distribute information subcortically and coordinate behavior (Ramaswamy and Markram, 2015). In line with this predicted function, many anatomical studies demonstrate that L5tt neurons send branching collaterals to subcortical targets including the HO thalamus, brainstem, and spinal cord–even at the level of single L5 neurons innervating more than one subcortical region (Veinante et al., 2000; Sherman, 2016; Guo et al., 2017; Rockland, 2019; Prasad et al., 2020). However, there is also evidence that L5tt subpopulations with distinct intrinsic properties innervate specific subcortical targets (Hattox and Nelson, 2007; Rojas-Piloni et al., 2017). This point raises the intriguing possibility that L5tt populations may have excitability and synaptic properties matched to their innervation targets. L5tt axonal branching is a matter of ongoing study and appears to be species-specific (Smith et al., 2014; Rockland, 2019). Reports also depend on specific experimental methodology, with axonal reconstructions reporting more branching versus retrograde tracing. High-throughput reconstruction efforts will be key to sharpening our understanding of L5tt innervation of subcortical targets in the near future.
Transthalamic and Recurrent Pathways
From the perspective of the HO thalamus, L5tt projections provide a strong but sparse drive to individual neurons (discussed in the next section). Where does this information go after being further processed in HO thalamus? (Sherman and Guillery, 2011) suggested a significant revision to our understanding of thalamocortical processing with the “transthalamic” hypothesis, specifically that cortical L5tt neurons in one source region send information to a secondary cortical recipient region via HO thalamus. Recent anatomical and functional data suggest that transthalamic pathways paralleling “direct” cortico-cortical pathways could be a common feature of the thalamocortical system and that these pathways carry distinct rather than redundant information (Sherman, 2016; Bennett et al., 2019; Mo and Sherman, 2019; Blot et al., 2021). However, there is recent evidence that certain HO circuits can also be involved in recurrent “closed-loop” networks, in which the same cortical region providing L5tt input to HO thalamus is itself reciprocally innervated by HO thalamus (Wimmer et al., 2010; Guo et al., 2020). This reciprocal connectivity motif is seen in FO CTC networks, where L6A modulatory CT projections originate from the same cortical region innervated by FO TC projections. However, the transthalamic and recurrent network motifs are not mutually exclusive and may subserve different functions. As we highlight below, based on region-specific descending CT and ascending HO TC connectivity patterns, different combinations of recurrent and transthalamic communication are possible. An important focus for future studies is to understand the distinct signals transmitted by transthalamic and recurrent pathways, and to uncover the computational scheme integrating these information channels with cortico-cortical signals.
Currently, it is understood that HO nuclei, which represent a majority of the thalamus by volume, participate in the generation of activity in distinct cortico-thalamo-cortical (CTC) networks (Guillery, 1995; Sherman and Guillery, 2011; Sherman, 2016). Additionally, these CTC networks serve to integrate a diverse range of cortical and subcortical signals (Groh et al., 2013; Bickford, 2015). While a full assessment of this literature is beyond the scope of this review, we note that these “convergence zones” have been reported across modalities (Groh et al., 2013; Bickford, 2015). For example, projections from the primary visual cortex in the HO lateral posterior (LP) nucleus overlap with terminals originating in the superior colliculus (Li et al., 2003c; Masterson et al., 2009). Elsewhere, Bosch-Bouju et al. (2013) introduce the concept of a “super-integrator,” where motor regions of the thalamus integrate information from the cortex with information from the basal ganglia and cerebellum. Additional convergence zones have been identified by exploiting the differential distribution of type 1 and type 2 vesicular glutamate transporters, which are specific to cortical and subcortical inputs, respectively (Rovó et al., 2012; Bickford, 2015). Recent anatomical evidence points to a role for HO thalamus in integrating information from different cortical regions (Prasad et al., 2020), even at the level of single cells (Sampathkumar et al., 2021).
Higher-Order Nuclei Across Modalities
In this focused review, we center our discussion on HO nuclei (Table 1) involved in primary sensory modalities (somatosensation, audition, and vision), as well as on HO nuclei that have been implicated in pathology (e.g., pain and cognitive dysfunction). A very recent anatomical survey of L5tt-subcortical projections has identified additional HO thalamic regions (Prasad et al., 2020); the functional properties of these projections remain to be characterized.
In the somatosensory system, the medial subdivision of the posterior nucleus (POm) receives L5tt input from the primary somatosensory cortex (S1), and thereafter recurrently targets S1 (Audette et al., 2017; Guo et al., 2020) and relays information trans-thalamically to the secondary somatosensory cortex (S2) (Theyel et al., 2010) and the primary motor cortex (M1) (Mo and Sherman, 2019). As Table 1 reflects, the somatosensory HO CTC circuit has provided a wealth of recent anatomical and functional data on cell-type-specific interactions and the in vivo impact of HO thalamus on cortical function.
In the visual system, the pulvinar nucleus (PuV) [or the homologous LP nucleus in rodents] is the L5tt-receiving HO nucleus, and is connected to all visual cortices (Bender, 1983; Saalmann et al., 2012; Bennett et al., 2019; de Souza et al., 2019). While pulvinar has been largely studied in primates, recent cell-type-specific and optogenetic studies have made increasing use of mouse models (Table 1). Regions within the LP that are reciprocally connected to the cortex are dominated by small terminals, while those that lack reciprocal connectivity are dominated by large terminals (Van Horn and Sherman, 2004). LP projections to higher visual areas have been shown to integrate descending information from L5tt cells in V1 with that from several cortical and subcortical areas, and the information conveyed to higher-order visual areas differs from that conveyed by cortico-cortical projections from V1. For example, while intracortical V1 projections convey information to the anteriolateral higher visual area about visual motion, the transthalamic route to this region (i.e., through the LP nucleus) integrates information about visual motion and the animals’ movement (Purushothaman et al., 2012; Stitt et al., 2018; Blot et al., 2021).
In the auditory system, the dorsal aspect of the medial geniculate nucleus (MGBd) receives L5tt input from the primary auditory cortex (A1), and relays to higher-order auditory regions (e.g., A2) (Lee and Sherman, 2011; Lee, 2015; Sherman, 2017). Cortical projections to the inferior colliculus (IC) have axon collaterals in the MGBd, although the degree and functional relevance of this branching is unclear (Asokan et al., 2018; Williamson and Polley, 2019). Notably, detailed physiological characterizations of the L5-MGBd-cortical pathways are lacking (Table 1).
While these sensory HO nuclei have received significant attention, there also exist “associative” L5tt-receiving HO nuclei that participate in non-sensory modalities. Here we emphasize rodent studies in the mediodorsal (MD) nucleus (Table 1), which contributes to learning, memory, and decision-making processes. MD’s influence on cognitive abilities results from interactions with L5tt-projecting frontal areas such as the prefrontal cortex (PFC) (Mitchell, 2015; Bolkan et al., 2017; Schmitt et al., 2017; Alcaraz et al., 2018; Pergola et al., 2018; Rikhye et al., 2018). Indeed, 20% of PFC projections (mainly from dorsal and medial areas) to MD stem from L5 (Xiao et al., 2009). It has been found that PFC output neurons in fact branch to both MD and the functionally diverse ventromedial (VM) nucleus, which may enable synchronized thalamic spiking across nuclei (Collins et al., 2018). In addition, the MD nucleus is implicated in mediating affective and emotional aspects of pain (Whitt et al., 2013; Mitchell, 2015).
Inhibition of Higher-Order Thalamus
A small but growing body of work demonstrates that HO thalamus is subject to inhibitory effects that either differ or are entirely distinct from those seen in FO circuits. Here we summarize the main points of distinction, and refer readers to Halassa and Acsady (2016) for a more comprehensive treatment of inhibitory control of thalamus.
Intrathalamic Inhibition of HO Thalamus
A central regulator of thalamic function is feedback inhibition via thalamic reticular nucleus (TRN), a thin layer of GABAergic neurons partially encapsulating the relay nuclei which project to cortex (Pinault, 2004). As well as TC afferents to cortex, thalamic relay neurons also send thalamoreticular projections to TRN which in turn provide feedback inhibition to relay neurons (Figure 1); the temporal scale of this inhibition is sensitive to spiking patterns (Figure 2), with high-frequency bursts triggering long-lasting IPSCs due to GABA “spillover” to extrasynaptic receptors, while tonic spiking patterns trigger shorter IPSCs (Halassa and Acsady, 2016). A recent pair of milestone studies in the somatosensory thalamus reveal that properties of HO and FO intrathalamic inhibitory circuitry differ significantly: HO nucleus POm excites and is inhibited by a discrete shell population of TRN neurons; furthermore, the synaptic dynamics of POm-TRN connections as well as the intrinsic properties of POm-connected TRN neurons are functionally distinct from those in VP-TRN circuits (Li et al., 2020; Martinez-Garcia et al., 2020). Thus, it may be that the dynamics of intrathalamic inhibition are matched to the distinct signal processing requirements of HO and FO circuits carrying L5tt and sensory information, respectively.
Given its role in gating thalamocortical transmission as well as its positional and physiological properties, the TRN has been implicated in the regulation of attention in the “searchlight hypothesis” (Crick, 1984; Crabtree, 2018). Regions in the TRN show increased activity in response to attentional stimuli, and the specific region in which this response is found is modality-dependent (McAlonan et al., 2000, 2006). Moreover, limbic TRN projections correlate with arousal states, while sensory TRN projections are suppressed by attentional states (Halassa et al., 2014). Work by Halassa et al. (2011) demonstrates TRN-dependent control of thalamocortical firing mode and state regulation, where selective drive of TRN causes a switch from tonic to burst firing and generates state-dependent neocortical spindles (Halassa et al., 2011).
Likewise, there is evidence for an attentional role of HO thalamus. For example, the MD is activated in humans during tasks requiring a rule-dependent shift in attentional allocation (i.e., set-shifting), such as the Wisconsin card-sorting task (Monchi et al., 2001; Halassa and Kastner, 2017). Human and monkey studies also point to a role of the pulvinar in visual attention. Pulvinar lesions in patients result in impairments in filtering distracting information, while pulvinar inactivation in monkey impairs spatial attention (Danziger et al., 2004; Snow et al., 2009; Wilke et al., 2010; Halassa and Kastner, 2017). In addition, Yu et al. (2008) describe the pulvinar’s role in sustained attention, employing the five-choice serial reaction time task to show that half of recorded units in this nucleus were attention-modulated (Yu et al., 2018). However, TRN control of HO thalamus in the context of attention and arousal has yet to be systematically investigated.
L6 CT Inputs to HO Thalamus
Thalamic reticular nucleus inhibition is also influenced by topographically organized CT projections from L6A which innervate both relay neurons and neurons in TRN (Lam and Sherman, 2010; Figure 1). Thus, these CT inputs simultaneously provide direct excitatory input to relay neurons and exert additional top-down control on inhibition via TRN, providing a cortical signal for task or attention-specific modulation of the thalamus. Overall, the net functional impact of L6A CT signals on thalamic relay neurons is determined by an excitation-inhibition balancing act of rate-dependent synaptic depression and facilitation (Crandall et al., 2015); this dynamic balance is seen in vivo in both HO and FO nuclei (Kirchgessner et al., 2020). It should be noted that HO and FO nuclei are targeted by partially disjoint populations of neurons in L6A (Thomson, 2010; Whilden et al., 2021; Figure 1), perhaps in keeping with the distinct reticular inhibitory circuits discussed above. Finally, aside from the L6A CT pathways, recent studies have identified a HO-thalamus specific pathway from layer 6B (Figure 1) which does not send collaterals to TRN and appears to exert strong excitatory influences on relay neurons (Hoerder-Suabedissen et al., 2018; Ansorge et al., 2020), although detailed physiological measures of these inputs are currently lacking. Notably, L6B neurons are orexin-sensitive (Bayer et al., 2004), suggesting that the cortex may provide HO thalamus with descending excitation based on wakefulness.
Extrathalamic Inhibition
Another key differentiator of HO thalamic circuits is the presence of additional subcortical inhibition from sources of “extrathalamic inhibition” (ETI) (Figures 1, 2), such as the zona incerta (ZI) and the anterior pretectal nucleus (APT) [reviewed in Halassa and Acsady (2016)]. Anatomical and experimental studies have suggested that these ETI sources exert a significantly more powerful inhibitory effect on the thalamus than do intrathalamic inhibition sources (Barthó et al., 2002; Lavallée et al., 2005; Park et al., 2018). In contrast to the TRN, which targets all thalamic nuclei, ETI inputs display greater target selectivity, a property that is evolutionarily highly conserved (Halassa and Acsady, 2016). Across sensory modalities, studies have demonstrated that HO, but not FO nuclei, receive ETI input, and this appears to serve as the inhibitory counterpart of L5tt excitatory inputs. This topographical restriction means that these ETI projections do not interact with ascending sensory relays, nor do they directly impact FO thalamus activity. Overall, ET inhibition of HO nuclei is notably strong, rapid, and precise, rendering it capable of influencing the timing of individual spikes in target cells (Halassa and Acsady, 2016). Further spatial selectivity has been established within subregions of the ZI. For example, Lavallée et al. (2005) found that the posterior (PO) HO nucleus receives inhibitory input from the ventral division of the ZI, which is in fact the same ZI subregion that receives input from the APT (Giber et al., 2008; Murray et al., 2010). Further underscoring the importance of ETI nuclei in sculpting HO thalamus processing of L5tt inputs, ZI and APT are also strongly driven by L5tt collaterals (Figure 1)–thus, HO nuclei are embedded in an additional feedforward inhibitory loop similar in motif to the L6A-TRN loop, but specific to HO nuclei and with different synaptic properties (Barthó et al., 2002, 2007; Halassa and Acsady, 2016).
Properties of PT Corticothalamic Synapses
L5tt-HO thalamus projections form sparse, large glutamatergic synapses electronically close to the soma of target thalamic relay neurons (Figure 2), typically on thick proximal dendrites near branch points (Hoogland et al., 1991; Bourassa et al., 1995; Deschênes et al., 1998; Rouiller and Welker, 2000). Terminals vary widely in size (2–10+μm), notably these distributions include a heavy tail of “giant” synapses reported in somatosensory, visual, and auditory HO nuclei (Llano and Sherman, 2008; Hoerder-Suabedissen et al., 2018; Prasad et al., 2020). “Giant” boutons are glomerular structures containing multiple synapses (Hoerder-Suabedissen et al., 2018) and development of these structures appears to be use-dependent (Hayashi et al., 2021). A recent anatomical comparison of L5tt-HO thalamus terminals (Prasad et al., 2020) reports some pathway-specific variation in size: V1 to pulvinar/LP, and PFC to MD terminals are somewhat smaller than S1 L5tt-POm benchmark “giant” synapses, but still larger than L6A-thalamus terminals.
These “driver” synapses have high release probability and high postsynaptic density of AMPA and NMDA receptors (Hoogland et al., 1991; Li et al., 2003b; Reichova and Sherman, 2004; Groh et al., 2008). While sparse–for example, single L5tt fibers form an average of only three boutons onto single POm neurons–single fiber activation can nevertheless trigger extremely large (∼3 nA in rat, ∼ 800 pA in mouse) excitatory postsynaptic currents (EPSCs), which drive large, unitary EPSPs (>10 mV) (Li et al., 2003b; Groh et al., 2008; Seol and Kuner, 2015). Aside from sheer synaptic strength, the EPSCs have exceptionally fast (<1 ms/2 ms rise/decay in rat) kinetics due to the presence of the GluA4 AMPA subunit (Seol and Kuner, 2015). While strong, L5tt-HO thalamus synapses are characterized by rapid and pronounced frequency-dependent depression due to presynaptic depletion of releasable vesicles (Reichova and Sherman, 2004; Groh et al., 2008; Seol and Kuner, 2015; Figure 2). At the L5tt-POm synapse, frequencies greater than ∼2 Hz induce depression, with paired-pulse ratios of 0.5 at 20 Hz, with similar values reported for L5tt-LP (Li et al., 2003b) and L5tt-MD and L5tt-VM (Collins et al., 2018), although comparable physiological data for the auditory system is lacking. In sum, despite some modality-specific anatomical variations which require further functional characterization (Prasad et al., 2020), L5tt-HO synapses appear to have relatively conserved properties supporting powerful, temporally precise, adaptive information transmission.
Higher-Order Thalamus Single Neuron Computation
Strong synaptic drive by L5 prompts the question of how HO neurons encode descending cortical information. In the context of neural coding, corticothalamic information transfer has been widely studied as L6A’s modulatory influence on FO neurons’ encoding of sensory drivers, in combination with aligned feedforward inhibition from TRN (e.g., Contreras et al., 1996; Wolfart et al., 2005; Béhuret et al., 2013). In HO nuclei, the situation is different: L5tt driver synapses provide a mechanism by which L5tt neurons’ precise spike times reach HO thalamus with minimal temporal distortion as large, fast EPSCs; via synaptic depression, EPSC amplitude will reflect L5’s preceding spiking history. To generate thalamic spikes, these cortically generated EPSCs must be integrated with other driver inputs (if present, e.g., Groh et al., 2013; Bickford, 2015; Blot et al., 2021), and finally transformed to spikes by intrinsic membrane properties.
Bursting
Intrinsic thalamic contributions to a corticothalamic code from L5tt to HO thalamus are expected to be considerable, given the nonlinear bursting properties of thalamic neurons (Llinás and Jahnsen, 1982) and increased appreciation for bursting as a significant mode of information processing (Zeldenrust et al., 2018b). In brief, across modalities, HO neurons display the well-known burst-tonic excitability switch characteristic of thalamic relay neurons (Figure 2). Burst spiking–high-frequency sodium APs superimposed on a transient calcium plateau or “low-threshold spike”–arises from the interplay of slow voltage-dependent currents, namely IT, carried by the Cav3 subfamily of calcium channels, with some contribution from IH, the hyperpolarization-activated cation current (Jahnsen and Llinas, 1984; Destexhe et al., 1993; Sherman, 2001). In particular, the phenomenon of bursting in thalamic neurons is well-studied, but a limitation to understanding HO bursting is that most detailed biophysical measurements come from FO neurons, typically from the visual system.
Several reports indicate that bursting properties differ between HO and FO nuclei: (Ramcharan et al., 2005) report more bursting in primate HO MD and HO pulvinar; cortico-recipient LP in rat shows enhanced bursting (Li et al., 2003a) and likewise, in tree shrew pulvinar (Wei et al., 2011), increased bursting was associated with increased expression of Cav3.2 and SK2, an afterhyperpolarization-generating K+ channel which can support repetitive firing. The somatosensory system appears to be an exception, as (Landisman and Connors, 2007) found that HO POm spikes at lower frequencies relative to FO VPM, both within bursts and during tonic spiking, associated with a higher voltage threshold for AP initiation in HO POm. A recent comparison across sensory modalities (Desai and Varela, 2021) provides a mechanistic framework for understanding some of these differences based on electrophysiology and simulations. Rebound burst size (number of APs) in HO nuclei was comparable across sensory modalities. However, differences between FO and HO bursting were modality specific and in agreement with data previously reported for single modalities: in visual and auditory thalamus, FO nuclei were less bursty than HO nuclei, while in somatosensory thalamus, the FO nucleus was more bursty than HO. This spectrum of bursting properties could be reproduced by changing the voltage-dependence and maximal conductance of IT. Taken together, these studies suggest functionally relevant differences in fast and slow intrinsic excitability mechanisms in HO and FO thalamic neurons.
Indeed, a recent comprehensive report of the thalamus transcriptome (Phillips et al., 2019) found that HO and FO nuclei show distinct transcriptomic profiles based on expression of genes tightly linked to neuronal identity (ion channels, receptors), in line with distinct intrinsic excitability of HO neurons. These points underscore the need for additional basic functional data from HO neurons, particularly voltage-clamp studies of biophysical properties necessary for computational studies, and at minimum, caution in using FO models as stand-ins for HO neurons in simulations.
Intrinsic Transformation of L5tt-HO EPSCs
We emphasize that careful consideration of bursting mechanisms is key to understanding how HO thalamus performs computations on descending cortical signals, as these mechanisms are responsible for nonlinear transformation of L5tt-evoked EPSCs to EPSPs. In the simple case of synaptic input after a period of inactivity, e.g., the beginning of a cortical Up state–large undepressed EPSCs will be further enhanced by activation of IT; functionally, this means that a single L5tt spike can evoke bursts of spikes in a post-synaptic HO neuron (supralinear corticothalamic spike transfer) (Groh et al., 2008; Seol and Kuner, 2015). In the more complicated case of higher input rates, synaptic depression will decrease EPSC size and preceding depolarization will simultaneously inactivate IT, supporting a more linear EPSC→ EPSP transformation. Here, integration of coincident inputs from different L5tt neurons can be required to drive output spikes (Groh et al., 2008). Although L5tt neurons’ drive of HO neurons at the single-neuron level has been most studied in the somatosensory system, the parallel effect of synaptic depression paired with inactivation of bursting is also apparent in data from other modalities (Li et al., 2003b; Collins et al., 2018).
The information processing role of bursts is an active area of study, and our understanding of thalamic burst coding continues to be refined. Recent studies (Elijah et al., 2015; Mease et al., 2017; Zeldenrust et al., 2018a; Park et al., 2019) make it clear that various properties of thalamic bursts (number of spikes, timing of spikes, burst onset, etc.) can convey information about presynaptic inputs, so it may be that the distinct bursting properties of HO thalamus subserve a particular functional role. In HO POm, we found that intrinsic bursting and high spiking threshold of POm neurons provides a mechanism for “multiplexed coding” of low- and high-frequency (∼5 Hz and >100 Hz) information, and that high-frequency encoding channel showed information-preserving adaptation (Mease et al., 2017). Furthermore, the exact bandwidth of the “slow” encoding channel was tuned by depolarization. This finding suggests that POm spike trains could carry information both in burst size and precise spike timing within bursts. However, the implications of this intrinsic code in combination with strong L5tt-HO synaptic depression have yet to be assessed. While it is not clear if these findings can be generalized to other nuclei, the observation that HO bursts seem to show less variation across modalities (Desai and Varela, 2021) suggests that further investigation of a common HO thalamic computational scheme for encoding L5tt inputs may provide new insights beyond those gleaned from studies focusing on computation in FO thalamus.
Neuromodulation
A final unexplored factor in HO thalamic encoding of L5tt inputs is neuromodulation. As membrane potential is a critical mediator of bursting properties, any modulatory input altering baseline depolarization or hyperpolarization would be expected to exert strong control over HO thalamic encoding. While neuromodulation specific to HO thalamus has not been widely studied, evidence suggests that it may dynamically regulate HO CTC network activity. For example, Stitt et al. (2018) suggest that activity in the HO thalamus may be state-dependent and influenced by ongoing levels of arousal. Elsewhere, it was found that the brainstem cholinergic system preferentially suppresses spontaneous activity in the POm-targeting region of ZI, thereby enhancing whisker responses in the POm (Masri et al., 2006; Trageser et al., 2006). Furthermore, Varela and Sherman found neuromodulation may exert differential effects on FO and HO neurons: while all FO and most HO neurons are depolarized by muscarinic and serotonergic activation, a significant fraction (15–20%) of HO neurons are hyperpolarized (Varela and Sherman, 2007, 2009). Taken in combination with attentional control of TRN and arousal-dependent activation of L6B discussed in the previous section, these studies tentatively point to an unexplored state-dependence of HO thalamus’s processing of L5tt drive. Future studies of this topic are warranted, particularly incorporating insight from recent molecular profiling work (Phillips et al., 2019) which may provide specific candidate mechanisms for state-dependent control.
Higher-Order Thalamic Encoding of L5tt Cortical Information
A wealth of evidence demonstrates that HO thalamus spike output is strongly affected or even contingent upon L5tt output, in line with the driver characteristics of this pathway. Manipulations of S1 cortex (Diamond et al., 1992) showed that spiking in HO POm but not FO VPM depend on S1 input; more specific optogenetic drive of L5 in vivo is sufficient to drive large driver EPSPs and bursts in POm, and VGAT optogenetic inhibition of S1 eliminates POm spiking (Groh et al., 2013; Mease et al., 2016c). Similarly, portions of HO LP (Bennett et al., 2019; Kirchgessner et al., 2021) are driven by L5tt inputs from V1. This situation is supported by findings that receptive fields in HO thalamus in vivo are typically broad and less specific for primary sensory drive (Moore et al., 2015; Urbain et al., 2015; Mease et al., 2016b; Williamson and Polley, 2019), suggesting that HO nuclei receive most sensory information after it has been processed by L5. Given the relatively low convergence of L5tt neurons onto HO neurons (Sumser et al., 2017; Rockland, 2019), single HO neurons may integrate the spiking of small cortical ensembles; indeed, in anesthetized mice, there is some evidence that HO thalamus may be driven to spike by just 1–3 active L5tt neurons in vivo (Mease et al., 2016c). Thus, the question becomes: what particular patterns of L5tt activation could be encoded by HO thalamus?
A critical piece of information necessary for understanding what L5tt signals HO neurons transmit back to the cortex is exactly what L5tt neurons encode and what the “raw” cortical input arriving in HO looks like via single fibers and convergent inputs from groups of L5tt neurons. L5tt neurons have been the focus of intense experimental and theoretical research interest over decades (Larkum, 2013; Ramaswamy and Markram, 2015; Sakmann, 2017), but despite this arguably focused attention, the exact signals propagated through L5tt pathways are not yet fully understood, despite being one of the most active neurons during behavior (de Kock et al., 2007; O’Connor et al., 2010; Senzai et al., 2019). The emerging picture is that L5tt spiking on both single-neuron and population levels carries complex information, with L5tt neurons typically showing broad sensory tuning (de Kock et al., 2007; Williamson and Polley, 2019) in line with their integration of multilaminar information in basal and apical dendrites, which is presumably inherited by HO thalamic targets. Extensive discussion of L5’s coding across modalities is beyond our scope; here we attempt to orient two key characteristics of L5tt output–bursting and ensemble synchrony–to what is known about further subcortical processing in HO thalamus.
L5tt Bursting
The active, nonlinear dendritic properties of L5tt neurons [reviewed in Larkum (2013); Ramaswamy and Markram (2015)] provide a single-neuron substrate for the integration of “top-down” and “bottom-up” information streams arriving at different lamina. Excitation of either basal or apical dendrites leads to sparse spiking, but near-coincident excitation of both regions triggers a burst of high-frequency (>100 Hz) APs which depends on a backpropagating AP in the soma triggering a calcium plateau in the apical tuft (Larkum et al., 1999; Larkum, 2013). In this framework, bursts indicate a temporal alignment of internal representation and novel external information. Such high-frequency bursts are characteristic of L5tt neurons in the primary somatosensory (Larkum et al., 1999; de Kock and Sakmann, 2008), visual (Shai et al., 2015), and auditory (Llano and Sherman, 2009; Williamson and Polley, 2019) cortices, and are associated with perception (Takahashi et al., 2016, 2020) and exploratory whisker touch (de Kock et al., 2021). While bursting appears to be an important nonlinear computational property of L5tt neurons, it remains to be determined if these spiking patterns are relevant for postsynaptic targets such as HO thalamus, i.e., are these high-frequency patterns faithfully transferred across the L5tt-HO thalamus synapse?
L5tt Synchrony and Ensemble Activity
Particular patterns of L5tt synchronization often involve inhibitory feedback interactions with interneurons, reviewed in Naka and Adesnik (2016). In particular, L5tt neurons excite both Martinotti cells (a subset of somatostatin-positive interneurons) which inhibit L5tt apical tufts, and PV neurons, which provide perisomatic inhibition to L5tt neurons. Inhibition from Martinotti neurons seems particularly tuned to L5tt bursting, as Martinotti neurons are preferentially recruited by high-frequency inputs from L5tt due to synaptic facilitation. This “frequency-dependent disynaptic inhibition” is a mechanism linking L5tt bursting to population synchrony, as bursting in a handful of L5tt neurons preferentially inhibits and synchronizes neighboring L5tt neurons (Silberberg and Markram, 2007; Hilscher et al., 2017).
Synchronous activity in particular frequency bands is an important mechanism by which cortical circuits transfer information (Buzsáki and Draguhn, 2004) and recent work has begun to relate understanding of cortical rhythms to specific neuronal cell types. For example, Adesnik and Scanziani (2010), Otsuka and Kawaguchi (2021) report that stimulation of L2/3 pyramidal neurons evokes beta/gamma band activity in L5tt neurons. Corticothalamic synchrony has been explored extensively from the perspective of CTL6 inputs to thalamus (e.g., Contreras et al., 1996; Bal et al., 2000; Béhuret et al., 2013), but how L5 synchrony might propagate to the HO thalamus is only beginning to be studied. For example (Stitt et al., 2018) find that alpha and theta band coherence is prominent in deep-layer PPC and pulvinar interactions; while this study did not specifically isolate layer 5, the involvement of HO nuclei suggest that L5tt input could participate in driving these corticothalamic oscillations. In sum, L5 synchronous population oscillations over a wide range (alpha, beta, theta, and gamma) of frequencies suggest that L5tt-HO thalamus synapses will have synchronized activation at diverse temporal scales that will engage different degrees of synaptic depression.
Recent efforts have begun to explore how pyramidal single-cell properties and population activity in the cortex are linked to implement network-level information coding strategies. The recently proposed Burst Ensemble (Naud and Sprekeler, 2018) theory suggests that ensemble event rate in L5tt (spike or burst count/time) reflects somatic input, burst probability reflects apical input, and burst rate reflects coincident somatic and apical input, while a simple spike rate code cannot disambiguate these combinations of inputs. Lipshutz et al. (2020) explored how simple model networks of pyramidal neurons can implement canonical correlation analysis, finding the features in apical and basal inputs which have maximal correlation; in this framework bursts indicate maximal alignment between the two input sources. Lankarany et al. (2019) demonstrate synchrony-division multiplexing: S1 neurons receiving common input can use the rate of asynchronous spiking to encode the intensity of low-contrast stimulus features while using the timing of synchronous spikes to encode the occurrence of high-contrast features. These studies highlight the importance of simultaneously considering cortical bursts and synchronous spikes as putatively informative signals for postsynaptic targets such as HO thalamus.
In sum, L5tt spike trains appear to carry information in spike count and timing and population synchrony; it is not well understood to what degree these information streams are disentangled and further transformed in HO thalamus. Groh et al. (2008) show that stimulating L5tt-POm synapses with in vivo L5tt spiking patterns resulted in single L5tt spikes driving POm spiking or bursting after long periods of silence in contrast to subthreshold EPSPs evoked at higher presynaptic L5tt rates sufficient to induce depression. Similarly, Collins et al. (2018) report that PFC L5tt EPSCs depress and only drive spiking in HO MD at the onset of 10 Hz stimulation. However, Groh et al. (2008) found that coincident activation of separate L5tt inputs served to overcome synaptic depression, and suggested a role for HO thalamus in detecting synchronous firing of L5tt neurons. Within the HO thalamus multiplexing framework (Mease et al., 2017), such coincident L5tt upstream activity could be encoded by the timing and spike count of POm bursts. Such coincidence detection may also work similarly in the case of integration of cortical and subcortical drivers, as in anesthetized animals, POm output reflects the latency between L5 activation and whisker stimulation (Groh et al., 2013).
Abundant evidence highlights L5tt bursts as somewhat privileged spiking patterns, both in terms of selective encoding of inputs and intracortical impact. From the point of view of HO thalamus, bursts would be translated into temporally discrete EPSCs of decreasing size. We hypothesized that the intrinsic properties of POm neurons may allow these EPSCs to influence spike timing within HO bursts, a situation which would preserve much of the temporal information with L5tt bursts (Mease et al., 2017), but this possibility remains to be tested particularly in awake animals during which HO thalamus bursting is less pronounced. An alternative hypothesis is that subsequent spikes in L5tt bursts evoke EPSCs too small to drive spiking in HO thalamus, or require coincident bursts from multiple presynaptic L5tt neurons to drive HO spiking. Combining cell-type-specific approaches with depth-resolved high-yield recordings in cortex (e.g., Senzai et al., 2019) and HO thalamus (e.g., Kirchgessner et al., 2020) will likely provide data to test these hypotheses.
Higher-Order Thalamocortical Projections to Cortex
How do signals computed by HO thalamus functionally impact the cortex? As we have reviewed above, although the exact features in L5tt spiking encoded by HO neurons and sent back to cortex remain to be determined, several recent studies have begun to clarify the function of HO TC inputs within the cortical microcircuit. These insights have built upon foundational anatomy studies demonstrating that HO TC projections follow the “matrix” pattern of TC innervation in that projections are not somatotopically precise and tend to be wide-ranging across cortical areas [reviewed in Harris and Shepherd (2015)]. In this section, we differentiate HO and FO TC pathways and highlight recent advances in understanding cell-type-specific HO TC innervation. We close with a discussion of recent evidence for the functional importance of HO TC inputs in higher-level CTC computations.
The function of HO CTC circuits cannot be viewed in isolation from other components of the cortical microcircuit–we refer to Shepherd and Yamawaki (2021) for a comprehensive review of CTC wiring, functional connectivity, and integration with feed-forward cortico-cortico circuits. Moreover, we also restrict our scope to differentiating HO and FO TC pathways in reciprocally connected circuits (e.g., S1→ POm→ S1). Readers are referred to other sources for discussion of HO properties for identified transthalamic sensory pathways S1-POm-S2 (Theyel et al., 2010; Viaene et al., 2011); there is evidence that TC inputs may have different laminar targets and synaptic properties in transthalamic circuits (e.g., Casas-Torremocha et al., 2019; Rodriguez-Moreno et al., 2020).
In S1, layer-specific HO TC inputs tend to interdigitate with and complement FO TC inputs (Figure 1), with dense HO projections to L5A and L1 (Koralek et al., 1988; Lu and Lin, 1993; Bureau et al., 2006; Meyer et al., 2010b; Wimmer et al., 2010), These distinct innervation patterns predict that HO thalamus provides synaptic input to particular cortical neuronal targets with dendrites in these lamina; the degree of expected TC innervation is often predicted as a function of depth as the TC projection density multiplied by the dendritic reconstruction’s summed cross-section. Optogenetic-aided circuit-mapping methods, (e.g., Bureau et al., 2006; Audette et al., 2017; Sermet et al., 2019) have provided the means to assess these anatomical predictions on a functional level, albeit largely in vitro in brain slices, and it has become clear that HO TC inputs provide input both excitatory and inhibitory neurons distinct from that provided by FO TC inputs.
Cell-Type-Specific Innervation
Excitatory Neurons
HO TC inputs provide direct excitation to both L2/3 and L5 pyramidal neurons (Figure 1 green), although different degrees of cell-type-specific excitation are seen across cortical regions. In S1, HO POm fibers evoke EPSPs in excitatory neurons across all cortical layers (Audette et al., 2017; Sermet et al., 2019), with the largest responses in L5A pyramidal neurons (Bureau et al., 2006; Audette et al., 2017; Sermet et al., 2019), which is sufficient to evoke robust spiking (Audette et al., 2017). Audette et al. (2017) also report significant but smaller input to L2/3 pyramidal neurons in vitro. While earlier reports found little direct HO input to L5tt neurons (Petreanu et al., 2009), small but significant inputs were reported by Audette et al. (2017); Sermet et al. (2019). A main point is that POm only provides weak inputs to L4, the main cortical recipient layer of FO TC inputs (Audette et al., 2017; Figure 1 blue). Similarly, in the auditory system, projections from HO MGd to A1 L1 equally excite pyramidal neurons in L2/3 and L5 (Pardi et al., 2020). In HO nuclei targeted by PFC, the situation is different (Collins et al., 2018): excitatory inputs to L2/3 pyramidal neurons by HO MD are more than ∼3x greater than those to L5A neurons, while inputs from HO VM to both layers are comparably strong, suggesting that MD preferentially activates superficial neurons in L2/3. The relevance of L3 MD-PFC is further evidenced by the finding that the MD also drives disynaptic inhibition in L3 of medial PFC through excitation of PV interneurons, tightening the time window during which PFC pyramidal neurons can fire (Delevich et al., 2015).
Optogenetic manipulations of HO TC inputs in vivo have provided some evidence for how these inputs impact different cortical cell types in the intact brain. Gambino et al. (2014) find that HO POm evokes long-lasting NMDA-dependent plateaus in L2/3 pyramidal neurons, while (Mease et al., 2016a) show that HO POm projections provide long-lasting depolarizations in L5 neurons and enhance sensory responses in vivo, and this effect is even stronger under awake conditions (Zhang and Bruno, 2019). The recently proposed embedded ensemble encoding (Antic et al., 2018) theory suggests that ensembles of neurons experiencing a synchronized somatic depolarization are in a transient “prepared state” to respond with precise spike timing to additional inputs. Given this evidence for HO TC induction of sustained depolarizations, HO thalamus could play a role in coordinating such transient ensembles of “prepared” neurons and sensitizing the cortex to additional synaptic inputs. One experimental difficulty in assessing HO TC’s impact in vivo that mass optogenetic excitation and inhibition does not lend itself to physiological stimulation patterns and it is likely that more naturalistic interventions will reveal nuances of the effect of HO TC projections–for example, the use of step-opsins by Mukherjee et al. (2020) to show that enhancement of MD thalamus led to inhibition dominating activity in PFC.
Interneurons
HO-thalamus innervation of specific interneuron types in cortex (Figure 1) appears to be key to understanding the functional impact of HO TC inputs, with several recent studies taking advantage of molecular markers for different interneuron populations. In particular, in S1, POm HO TC inputs provide strong excitation to PV interneurons in L5a and L2/3 but little direct input to SOM interneurons (Audette et al., 2017; Sermet et al., 2019; Williams and Holtmaat, 2019). Thus HO TC provides disynaptic inhibition via PV to L5a pyramidal neurons, as well as direct excitation (Audette et al., 2017). A future direction will be to understand how PV interneurons encode naturalistic HO inputs in vivo, as PV neurons are particularly excitable, with low membrane time constants and high repetitive firing ability. Intriguingly Cruikshank et al. (2007) showed that, due to these single-cell properties, fast-spiking (presumed PV) interneurons are intensively driven by FO TC inputs. More recently, Jouhanneau et al. (2018) found that precise PV spiking can be evoked by unitary cortico-cortical EPSPs. Although HO TC to PV neuron encoding remains to be assessed, the strong synaptic drive in combination with high post-synaptic temporal precision suggests PV neurons may be able to follow high-frequency information in HO spike trains.
Interneurons in L1 are increasingly appreciated as targets of HO TC inputs, although direct comparison across studies is somewhat challenging due to variations in exact methodology and specificity of classification. A small group of studies has begun to clarify the importance of differential HO TC innervation of 5HT3 neurons in layer 1, which include VIP and NDNF populations (Schuman et al., 2021), particularly suggesting roles in disinhibition of deeper layers. In S1, 5HT3 interneurons receive direct HO POm input which evokes spiking in vitro (Audette et al., 2017). Notably, while somatostatin-positive (SOM) interneurons receive little direct HO TC input (Audette et al., 2017; Sermet et al., 2019; Williams and Holtmaat, 2019) find that PO excitation of VIP interneurons disynaptically inhibits SOM neurons. Similarly, Anastasiades et al. (2021) show that in PFC, HO MD projections directly target VIP neurons in L1, which then inhibit SOM neurons. These studies point to HO TC disinhibition of the pyramidal targets of SOM neurons, with possible implications for network synchronization, i.e., via Martinotti neurons’ interactions with L5tt discussed in the previous section. Finally, results from two different cortical regions demonstrate a role for neurogliaform/NDNF interneurons’ interaction with HO TC inputs in controlling pyramidal neuron excitability. Pardi et al. (2020) find that neurogliaform interneurons provide presynaptic inhibition of the HO MGd terminals involved in learning and triggering long-lasting NMDA potentials. Anastasiades et al. (2021) show that L1 NDNF interneurons in PFC are innervated by HO VM and inhibit SOM interneurons, but also act to block VM’s direct excitation of L5tt neurons’ apical tufts.
HO Thalamus in Sensory Processing and Cognition
Cell-type-specific circuit interventions have revealed roles for HO thalamus in conveying signals important for learning, perception, and behavioral salience. Recent in vivo studies in behaving animals are also providing insight into the qualitative content of these HO thalamus signals, supporting roles for HO TC in promoting awake cortical behavior patterns associated with learning, cognitive flexibility, perception, and even consciousness.
In S1, studies by Holtmaat and colleagues provide a strong line of evidence for HO POm in generating long-term potentiation of intracortical synapses onto L2/3 pyramidal neurons via NMDA-dependent plateau potentials which depend on VIP-mediated disinhibition (Gambino et al., 2014; Williams and Holtmaat, 2019); these plateau potentials also provide a mechanism for cortical map plasticity (Pagès et al., 2021). In a multi-whisker sensory association task, Audette et al. (2019) found that training sequentially induces plasticity at HO POm TC synapses onto L5 and L2 pyramidal neurons, thereby increasing POm-driven spiking without changes in cortical single-cell properties. In an auditory associative learning task, Pardi et al. (2020) also demonstrated learning-related HO TC plasticity, finding that HO TC input to A1 transmits memory-related information which reflects task-specific relevance of sensory stimuli. El-Boustani et al. (2020) find that HO thalamus inputs show goal-directed modulation in mice trained in a whisker discrimination task; similarly (LaTerra et al., 2020) find that HO POm axons in S1 signal correct performance during goal-directed behavior and that inhibition of POm impedes task performance.
In the context of cognition, it is suggested that a generic role for the thalamus may be to coordinate and maintain cortical representations relevant for particular cognitive tasks (Halassa and Kastner, 2017; Nakajima and Halassa, 2017). For example, in an auditory-visual cue-switching attentional task, Schmitt et al. (2017) find that HO MD maintains PFC ensemble representations of task rules by control of functional connectivity. In the same behavioral paradigm, Rikhye et al. (2018) found that interactions between the PFC and HO MD provide a mechanism for cognitive flexibility to switch cortical representations, with MD thalamus encoding behavioral context. Although the specific contributions of L5 inputs to such cognitive tasks remain to be assessed, recent evidence suggests that L5-CTC loops may be key to conscious perception. For example, Suzuki and Larkum (2020) show that HO POm TC input enables L5tt dendro-somatic coupling necessary for awake activity patterns and robust somatic spiking, and that general anesthesia blocks this coupling. Although not specific to L5, Redinbaugh et al. (2020) find that in primates, activity in central lateral (CL) HO thalamus and deep-layer cortical neurons correlate with consciousness level; indeed, gamma stimulation in CL could rouse monkeys from anesthesia. These studies point to diverse but unexplored functions for HO nuclei in higher-level cognitive computations in the cortex.
We emphasize here that a core component of disentangling HO thalamus’s role in critical cortical function is quantification of how patterns of L5tt spiking are selected and transmitted back to the cortex. Finally, understanding the impact of specific patterns of HO neuron activity in cortex will require combining emerging knowledge of cell-type-specific TC synaptic dynamics with the state-dependent, nonlinear dendritic integration properties of cortical neurons, along with detailed microcircuit connectivity patterns.
The Clinical Relevance of L5tt-HO CTC Networks
The Pathological Role of L5-Originating CTC Networks in Pain
While further work is required to assess the underlying mechanisms and functionality of L5-originating CTC networks, recent studies have emphasized their potential clinical relevance (see Table 2). Among these, an increasing number of studies highlight the importance of these CTC networks in processing nociceptive information in both acute and chronic pain states. For example, spared nerve injury (SNI) in rodents- a model of neuropathic pain–results in a three-fold increase in Ca2+ activity in the somata of L5 pyramidal neurons in S1, as well as an increase in dendritic spine Ca2+ activity (Cichon et al., 2017). However, this study did not assess the impact of enhanced cortical activity on HO thalamic nuclei. Indeed, the development of chronic pain is associated with several maladaptive alterations in S1 and other cortical regions (e.g., hyperexcitability, somatotopic reorganization), but there remains a need to characterize how these alterations specifically influence CTC networks, especially given that these cortical alterations typically correlate with the degree of mechanical allodynia (i.e., a painful sensation resulting from typically innocuous mechanical stimuli) (Tan and Kuner, 2021). The relevance of these cortical alterations, and their potential impact on HO nuclei and CTC networks, is further exemplified by the fact that targeting S1 alterations beneficially alters pain trajectories (Flor et al., 2001; Moseley and Flor, 2012; Cichon et al., 2017). Hyperactive states in L5 neurons have also been observed in other cortical areas under inflammatory states–for instance, a study employing a peripheral inflammatory mouse model found transient hyperactivity in L5 pyramidal neurons in the frontal/motor cortex (Odoj et al., 2021). However, this study did not observe L5 hyperactivity in S1, so it is unclear whether the frontal/motor cortex alterations are pain-relevant.
Cichon et al. (2017) further investigated the role of inhibitory circuits in the development of S1 alterations, specifically, the source of L5 hyperactivity in chronic pain. It was shown that 1 month following SNI induction, somatostatin (SOM) interneuron activity, which regulates somatic and dendritic pyramidal cell activity, was reduced by half. Likewise, PV interneuron activity was reduced. In part, these findings were the result of a 90% increase in vasoactive intestinal peptide (VIP)-expressing interneuron activity in SNI animals, which directly inhibit SOM and PV interneurons. The SOM contribution to L5 pyramidal cell hyperactivity was confirmed through selective SOM cell activation, which decreased L5 dendritic and somatic Ca2+ activity and prevented the development of mechanical allodynia (Cichon et al., 2017). However, activation of PV interneurons did not alter mechanical thresholds for pain, perhaps because these cells predominantly synapse perisomatically, or because they provide only brief inhibition of somata (Cichon et al., 2017). Given the specific interaction of HO TC inputs with PV and VIP interneurons covered in the previous section and the plasticity of these connections (Audette et al., 2017, 2019; Williams and Holtmaat, 2019), it may be productive to further assess how HO TC inputs contribute to maladaptive cortical plasticity related to chronic pain.
It may be that L5 alterations in part contribute to changes observed in HO nuclei during chronic pain. For example, the PO nucleus, which is involved in pain processing, displays higher spontaneous firing rates and greater responses to both noxious and innocuous peripheral stimuli in a chronic pain state (Perl and Whitlock, 1961; Whitlock and Perl, 1961; Casey, 1966; Mao et al., 1993; Masri et al., 2009; Park et al., 2018). Another relevant HO nucleus is the MD, which mediates affective aspects of pain and is similarly hyperactive in chronic pain states. However, as with the PO, it is not understood if these changes in part stem from CTC alterations at the level of the cortex (Rinaldi et al., 1991; Wang et al., 2007; Whitt et al., 2013; Mitchell, 2015). Reinforcing its role in affective components of pain, optogenetic activation of MD inputs in the anterior cingulate cortex (ACC) elicits a conditioned place-aversion in the SNI model and chemotherapy-induced neuropathy, but intriguingly, direct inhibition of L5tt ACC projection neurons to the MD nucleus also produces this effect (Meda et al., 2019). In addition, inactivation and lesioning of the MD nucleus both resulted in a reduction in thermal and mechanical hyperalgesia in a rodent model of neuropathic pain (Saadé et al., 2007). Overall, while further effort is required to assess the overall role of CTC networks in pain, evidence suggests that both HO-projecting L5tt cells and HO thalamic nuclei are altered in pain states, and that targeting these alterations may serve to benefit pain trajectories.
The Pathological Role of CTC Networks in Auditory Disorders
In the auditory system, comparable to the findings in chronic pain, Asokan et al. (2018) observed a hyperactive state of L5 projection cells in the auditory cortex following noise-induced damage to cochlear afferents, and this effect was sustained for several weeks (Asokan et al., 2018). Specifically, this L5 potentiation–which represents a form of compensatory plasticity–was observed in projections to the IC, but the same study also found axon collaterals to the HO MGBd nucleus (Asokan et al., 2018). Alterations in inhibitory networks are also implicated in this L5 pathology–for instance, PV interneuron-mediated intracortical inhibition is significantly reduced for at least 45 days following cochlear synaptopathy (Resnik and Polley, 2017). Increased sensory gain is a characteristic finding across noise-induced hearing-loss pathologies (e.g., tinnitus), and it is proposed that L5 projection neurons are responsible for driving hyperexcitability and strong coupling across tinnitus-associated brain networks (Asokan et al., 2018). As with the pain example discussed above, the impact of such pathological L5 activity on auditory HO nuclei remains to be assessed.
The Pathological Role of CTC Networks in Cognitive/Behavioral Dysfunction
In addition to the apparent involvement of CTC networks in sensory modalities, recent work has alluded to their relevance to pathologies characterized by cognitive and behavioral dysfunction, such as schizophrenia (SZ) and Alzheimer’s disease (AD). Studies have demonstrated the involvement of the MD in these dysfunctions, and there is human, rodent and primate evidence that they can be elicited by damaging this thalamic nucleus (Mitchell et al., 2007, 2014; Mitchell and Gaffan, 2008; Mitchell, 2015; Perry et al., 2021). For instance, monkeys with damage in the MD display impairments in complex associated learning tasks (Mitchell et al., 2007). MD damage-associated impairment has been postulated to result from disruption of the influence of the MD nucleus on the PFC, since the MD nucleus and PFC are reciprocally connected within specific subdivisions (e.g., the pars parvicellularis of the MD is reciprocally connected to the dorsolateral PFC), but this view is contested (Mitchell, 2015; Collins et al., 2018). Specifically, the MD has been shown to activate cortico-cortical projections in layers 2 and 3 in the PFC (Collins et al., 2018). Further work on thalamic innervation of associative brain structures has shown that enhanced excitability in the MD elicits suppression of PFC excitatory neurons (Mukherjee et al., 2020).
Moreover, it was found that hypoxic-like damage to the PFC results in enhanced theta-frequency coherence between the MD and the PFC, as well as an increase in the frequency of bursting in MD neurons, while subsequent knockdown of T-type calcium channels (Cav3.1) in the MD nucleus decreased theta-frequency coherence and attenuated associated symptoms (e.g., frontal lobe seizures and locomotor hyperactivity). The authors propose that the observed neurological and behavioral abnormalities result from impaired thalamocortical feedback between the PFC and the MD, driven by the activation of thalamic T-type calcium channels (Kim et al., 2011). Moreover, abnormalities have been reported in HO nuclei in SZ patients, including reductions in the volume and activity of the MD nucleus and the PuV. This may disrupt transthalamic networks and account for schizophrenia-associated cognitive impairments (Sherman, 2017). In fact, Parnaudeau et al. (2013) show that pharmacogenetic inhibition of the MD nucleus disrupts MD-PFC synchrony in the beta range, causing cognitive impairment with relevance to SZ. Similarly, there is evidence that CT dysfunction contributes to cognitive and behavioral impairments observed in AD (Jagirdar and Chin, 2019).
In addition to these findings, it is increasingly understood that dendritic integration in pyramidal neurons, which plays essential roles in sensory processing and cognition, is disrupted in a range of neurodevelopmental disorders (Nelson and Bender, 2021). For example, autism spectrum disorder is associated with genetic changes that elicit functional, morphological, and organizational alterations in L5, but it is not understood if and how these cortical changes affect the rest of the CTC network (Nelson and Bender, 2021).
Discussion
The thalamocortical field has clearly moved on from the historical view of the thalamus as simply a passive provider of input to the cortex, although this view is still surprisingly entrenched in general neuroscience literature. As comprehensively framed by Sherman and Guillery (2006); Sherman (2016), any adequate theory of cortical function must include active, dynamic, and iterative information processing by the thalamus. With unique L5tt input and output connectivity patterns in combination with distinct synaptic and intrinsic properties, HO thalamic nuclei are well-suited to support top-down information transformation and exchange critical for novelty detection and prediction (Keller and Mrsic-Flogel, 2018) or propagation of learning-related signals (Chéreau et al., 2021).
Here, we have sought to present a broad overview of L5tt corticothalamic information transfer to HO thalamus and back to the cortex. Rather than attempting comprehensive coverage, we have highlighted mechanistic properties and functional findings underscoring the point that insights from FO nuclei may not transfer well to HO nuclei as points of orientation for neuroscientists new to the vast, complex thalamocortical literature. Although we have attempted to balance findings across sensory modalities and HO regions where data are present, many recent illuminating studies were done only in single regions–for example, many of the very recent cell-type-specific functional insights about HO TC inputs are from the somatosensory system. Lastly, in focusing largely on studies using cell-type-specific and/or experimental methods tractable in rodents, by necessity most of the presented information comes from experiments in mouse and rat models.
A key point is that in comparison to the relatively rich theoretical framework focused on cortical function, further subcortical processing of L5tt signals has been comparatively neglected. Cortico-centric models have yet to be fully integrated with the transthalamic communication model (Sherman and Guillery, 2011)–we emphasize that a central missing piece is how exactly L5tt signals are processed and encoded in HO thalamus. Whatever the modality-specific information L5tt spike trains send to HO thalamus, these signals will be further transformed by a heady blend of strong synaptic depression, the nonlinear input/output properties of single HO thalamic neurons tuned by dynamic L6A and TRN-driven excitation and inhibition, and finally, integration with other subcortical drivers and strong extrathalamic sources of inhibition.
Cast in this light, it is not particularly surprising that the question of what HO thalamus encodes is currently unanswered–but solving this puzzle appears increasingly central to understanding cortical network function underpinning cognition and perception. We have emphasized the viewpoint that it may be more productive to consider HO thalamus’s encoding of driving cortical signals from L5tt, rather than any particular parameterization of raw sensory stimuli. Given the evidence for HO integration of L5tt inputs with non-cortical drivers and various neuromodulatory signals, this viewpoint is clearly an oversimplification–but possibly still a useful beginning step in linking L5tt drive of HO thalamus to general theories of cortical computation. More specifically, we hypothesize that HO thalamus may be able to simultaneously detect and transmit distinct patterns of L5tt synchrony and high-frequency spiking, and that these signals may have cell-type-specific functions in the cortex depending on HO TC postsynaptic targets. In the future, these ideas could be tested by combining high-yield electrophysiology approaches, cell-type-specific interventions, and recent advances quantifying selective information transfer between brain regions, such as the “communication subspace” scheme described by Semedo et al. (2019).
Although the synaptic and intrinsic mechanistic pieces appear to exist for an adaptive information-dense corticothalamic code from L5 to HO thalamus, there are still significant experimental and theoretical efforts to be made. Computational modeling would expedite understanding of how HO thalamus encodes cortical L5 spike trains, but based on our survey of ModelDB (Hines et al., 2004), very few models of HO thalamic neurons have been published (Golomb et al., 2006; Desai and Varela, 2021) and certainly none with the level of biophysical detail as recent modeling efforts focused on FO neurons (e.g., Connelly et al., 2016; Iavarone et al., 2019). Such efforts could boost further assessment of the relationship between bursting in L5 and HO thalamus, as it is clear that bursts can play privileged roles in both transmitting information and engaging plasticity mechanisms (Larkum, 2013; Crunelli et al., 2018; Zeldenrust et al., 2018b; Payeur et al., 2021). In the future, it will be important to expand existing network models of thalamocortical interactions which are mainly but not always (see Golomb et al., 2006) based on FO data to include HO and FO distinctions in driving input, intrathalamic inhibition, and intrinsic properties which we have attempted to summarize here.
Finally, studies have begun to shed light on the clinical relevance of HO-thalamus CTC pathways, as a range of disruptions along these pathways, especially in HO nuclei, have been implicated in pathologies including chronic pain, SZ, and AD. However, present studies have largely concentrated efforts on characterizing either cortical or HO thalamic dysfunction in pathological contexts, but do not consider the interregional relationships. As such, further efforts are merited to transfer recent fundamental insights from sensory processing and cognition to pathology in L5tt-HO thalamus circuits, in particular studies that assess CTC pathways in their entirety. In the future, improving our understanding of these pathways in both pathological and non-pathological settings may serve to facilitate the identification of novel therapeutic targets and inform clinical strategies.
Author Contributions
RM: conception, planning, and funding acquisition. RM and AG: research, writing, and editing. Both authors contributed to the article and approved the submitted version.
Funding
This work was funded by the Brigitte-Schlieben-Lange-Program, Baden-Württemberg (RM), Deutsche Forschungsgemeinschaft Collaborative Research Center 1158 (RM and AG), and Chica and Heinz Schaller Foundation (RM).
Conflict of Interest
The authors declare that the research was conducted in the absence of any commercial or financial relationships that could be construed as a potential conflict of interest.
Publisher’s Note
All claims expressed in this article are solely those of the authors and do not necessarily represent those of their affiliated organizations, or those of the publisher, the editors and the reviewers. Any product that may be evaluated in this article, or claim that may be made by its manufacturer, is not guaranteed or endorsed by the publisher.
Acknowledgments
The authors thank Alexander Groh for comments on early versions of this manuscript and the reviewers for their constructive suggestions for improvement.
Abbreviations
AHP, afterhyperpolarization; AD, Alzheimer’s disease; ACC, anterior cingulate cortex; APT, anterior pretectal nucleus; CT, corticothalamic; CTC, cortico-thalamo-cortical; MGBd, dorsal aspect of medial geniculate body; EPSP, excitatory postsynaptic potential; EPSC, excitatory postsynaptic current; FO, first-order; HO, higher-order; IH, hyperpolarization-activated cation current; LP, lateral posterior nucleus; L5, layer-V; L6, layer-VI; MD, mediodorsal nucleus; PV, parvalbumin; POm, posterior medial nucleus; PO, posterior nucleus; PPC, posterior parietal cortex; PFC, prefrontal cortex; A1, primary auditory cortex; M1, primary motor cortex; S1, primary somatosensory cortex; V1, primary visual cortex; PuV, pulvinar nucleus; A2, secondary auditory cortex; S2, secondary somatosensory cortex; SOM, somatostatin; SNI, spared nerve injury; SZ, schizophrenia; TT, thick-tufted; IT, transient low-threshold calcium current; VIP, vasoactive intestinal peptide; ZI, zona incerta.
References
Adesnik, H., and Scanziani, M. (2010). Lateral competition for cortical space by layer-specific horizontal circuits. Nature 464, 1155–1160. doi: 10.1038/nature08935
Alcaraz, F., Fresno, V., Marchand, A. R., Kremer, E. J., Coutureau, E., and Wolff, M. (2018). Thalamocortical and corticothalamic pathways differentially contribute to goal-directed behaviors in the rat. Elife 7:e32517.
Anastasiades, P. G., Collins, D. P., and Carter, A. G. (2021). Mediodorsal and ventromedial thalamus engage distinct L1 circuits in the prefrontal cortex. Neuron 109, 314–330.e4.
Ansorge, J., Humanes-Valera, D., Pauzin, F. P., Schwarz, M. K., and Krieger, P. (2020). Cortical layer 6 control of sensory responses in higher-order thalamus. J. Physiol. 598, 3973–4001. doi: 10.1113/JP279915
Antic, S. D., Hines, M., and Lytton, W. W. (2018). Embedded ensemble encoding hypothesis: the role of the “Prepared” cell. J. Neurosci. Res. 96, 1543–1559. doi: 10.1002/jnr.24240
Asokan, M. M., Williamson, R. S., Hancock, K. E., and Polley, D. B. (2018). Sensory overamplification in layer 5 auditory corticofugal projection neurons following cochlear nerve synaptic damage. Nat. Commun. 9:2468.
Audette, N. J., Bernhard, S. M., Ray, A., Stewart, L. T., and Barth, A. L. (2019). Rapid plasticity of higher-order thalamocortical inputs during sensory learning. Neuron 103, 277–291.e4. doi: 10.1016/j.neuron.2019.04.037
Audette, N. J., Urban-Ciecko, J., Matsushita, M., and Barth, A. L. (2017). POm thalamocortical input drives layer-specific microcircuits in somatosensory cortex. Cereb. Cortex 28, 1312–1328. doi: 10.1093/cercor/bhx044
Bal, T., Debay, D., and Destexhe, A. (2000). Cortical feedback controls the frequency and synchrony of oscillations in the visual thalamus. J. Neurosci. 20, 7478–7488. doi: 10.1523/jneurosci.20-19-07478.2000
Barthó, P., Freund, T. F., and Acsády, L. (2002). Selective GABAergic innervation of thalamic nuclei from zona incerta. Eur. J. Neurosci. 16, 999–1014. doi: 10.1046/j.1460-9568.2002.02157.x
Barthó, P., Slézia, A., Varga, V., Bokor, H., Pinault, D., Buzsáki, G., et al. (2007). Cortical control of zona incerta. J. Neurosci. 27, 1670–1681. doi: 10.1523/jneurosci.3768-06.2007
Bartlett, E. L., Stark, J. M., Guillery, R. W., and Smith, P. H. (2000). Comparison of the fine structure of cortical and collicular terminals in the rat medial geniculate body. Neuroscience 100, 811–828. doi: 10.1016/s0306-4522(00)00340-7
Bayer, L., Serafin, M., Eggermann, E., Saint-Mleux, B., Machard, D., Jones, B. E., et al. (2004). Exclusive postsynaptic action of hypocretin-orexin on sublayer 6b cortical neurons. J. Neurosci. 24, 6760–6764. doi: 10.1523/jneurosci.1783-04.2004
Béhuret, S., Deleuze, C., Gomez, L., Frégnac, Y., and Bal, T. (2013). Cortically-controlled population stochastic facilitation as a plausible substrate for guiding sensory transfer across the thalamic gateway. PLoS Comput. Biol. 9:e1003401. doi: 10.1371/journal.pcbi.1003401
Bender, D. B. (1983). Visual activation of neurons in the primate pulvinar depends on cortex but not colliculus. Brain Res. 279, 258–261. doi: 10.1016/0006-8993(83)90188-9
Bennett, C., Gale, S. D., Garrett, M. E., Newton, M. L., Callaway, E. M., Murphy, G. J., et al. (2019). Higher-order thalamic circuits channel parallel streams of visual information in mice. Neuron 102, 477–492.e5.
Bickford, M. E. (2015). Thalamic circuit diversity: modulation of the driver/modulator framework. Front. Neural Circuits 9:86. doi: 10.3389/fncir.2015.00086
Blot, A., Roth, M. M., Gasler, I., Javadzadeh, M., Imhof, F., and Hofer, S. B. (2021). Visual intracortical and transthalamic pathways carry distinct information to cortical areas. Neuron 109, 1996–2008.e6. doi: 10.1016/j.neuron.2021.04.017
Bolkan, S. S., Stujenske, J. M., Parnaudeau, S., Spellman, T. J., Rauffenbart, C., Abbas, A. I., et al. (2017). Thalamic projections sustain prefrontal activity during working memory maintenance. Nat. Neurosci. 20, 987–996. doi: 10.1038/nn.4568
Bosch-Bouju, C., Hyland, B. I., and Parr-Brownlie, L. C. (2013). Motor thalamus integration of cortical, cerebellar and basal ganglia information: implications for normal and parkinsonian conditions. Front. Comput. Neurosci. 7:163. doi: 10.3389/fncom.2013.00163
Bourassa, J., and Deschenes, M. (1995). Corticothalamic projections from the primary visual cortex in rats: a single fiber study using biocytin as an anterograde tracer. Neuroscience 66, 253–263. doi: 10.1016/0306-4522(95)00009-8
Bourassa, J., Pinault, D., and Deschênes, M. (1995). Corticothalamic projections from the cortical barrel field to the somatosensory thalamus in rats: a single-fibre study using biocytin as an anterograde tracer. Eur. J. Neurosci. 7, 19–30. doi: 10.1111/j.1460-9568.1995.tb01016.x
Bureau, I., von Saint Paul, F., and Svoboda, K. (2006). Interdigitated paralemniscal and lemniscal pathways in the mouse barrel cortex. PLoS Biol. 4:e382. doi: 10.1371/journal.pbio.0040382
Buzsáki, G., and Draguhn, A. (2004). Neuronal oscillations in cortical networks. Science 304, 1926–1929. doi: 10.1126/science.1099745
Cajal, S. R. Y. (1906). Santiago Ramón y Cajal – Nobel Lecture. Available online at: https://www.nobelprize.org/uploads/2018/06/cajal-lecture.pdf (accessed December 31, 2017).
Casas-Torremocha, D., Porrero, C., Rodriguez-Moreno, J., García-Amado, M., Lübke, J. H. R., Núñez, Á, et al. (2019). Posterior thalamic nucleus axon terminals have different structure and functional impact in the motor and somatosensory vibrissal cortices. Brain Struct. Funct 224, 1627–1645. doi: 10.1007/s00429-019-01862-4
Casey, K. L. (1966). Unit analysis of nociceptive mechanisms in the thalamus of the awake squirrel monkey. J. Neurophysiol. 29, 727–750. doi: 10.1152/jn.1966.29.4.727
Chéreau, R., Williams, L. E., Bawa, T., and Holtmaat, A. (2021). Circuit mechanisms for cortical plasticity and learning. Semin. Cell Dev. Biol. S1084-9521(21)00199-3. doi: 10.1016/j.semcdb.2021.07.012
Cichon, J., Blanck, T. J. J., Gan, W.-B., and Yang, G. (2017). Activation of cortical somatostatin interneurons prevents the development of neuropathic pain. Nat. Neurosci. 20, 1122–1132. doi: 10.1038/nn.4595
Collins, D. P., Anastasiades, P. G., Marlin, J. J., and Carter, A. G. (2018). Reciprocal circuits linking the prefrontal cortex with dorsal and ventral thalamic nuclei. Neuron 98, 366–379.e4.
Connelly, W. M., Crunelli, V., and Errington, A. C. (2016). Passive synaptic normalization and input synchrony-dependent amplification of cortical feedback in thalamocortical neuron dendrites. J. Neurosci. 36, 3735–3754. doi: 10.1523/jneurosci.3836-15.2016
Contreras, D., Destexhe, A., Sejnowski, T. J., and Steriade, M. (1996). Control of spatiotemporal coherence of a thalamic oscillation by corticothalamic feedback. Science 274, 771–774. doi: 10.1126/science.274.5288.771
Crabtree, J. W. (2018). Functional diversity of thalamic reticular subnetworks. Front. Syst. Neurosci. 12:41. doi: 10.3389/fnsys.2018.00041
Crandall, S. R., Cruikshank, S. J., and Connors, B. W. (2015). A corticothalamic switch: controlling the thalamus with dynamic synapses. Neuron 86, 768–782. doi: 10.1016/j.neuron.2015.03.040
Crick, F. (1984). Function of the thalamic reticular complex: the searchlight hypothesis. Proc. Natl. Acad. Sci. U.S.A. 81, 4586–4590. doi: 10.1073/pnas.81.14.4586
Cruikshank, S. J., Lewis, T. J., and Connors, B. W. (2007). Synaptic basis for intense thalamocortical activation of feedforward inhibitory cells in neocortex. Nat. Neurosci. 10, 462–468. doi: 10.1038/nn1861
Crunelli, V., Lõrincz, M. L., Connelly, W. M., David, F., Hughes, S. W., Lambert, R. C., et al. (2018). Dual function of thalamic low-vigilance state oscillations: rhythm-regulation and plasticity. Nat. Rev. Neurosci. 19, 107–118. doi: 10.1038/nrn.2017.151
Daigle, T. L., Madisen, L., Hage, T. A., Valley, M. T., Knoblich, U., Larsen, R. S., et al. (2018). A suite of transgenic driver and reporter mouse lines with enhanced brain-cell-type targeting and functionality. Cell 174, 465-480.e22.
Danziger, S., Ward, R., Owen, V., and Rafal, R. (2004). Contributions of the human pulvinar to linking vision and action. Cogn. Affect. Behav. Neurosci. 4, 89–99. doi: 10.3758/cabn.4.1.89
de Kock, C. P. J., Pie, J., Pieneman, A. W., Mease, R. A., Bast, A., Guest, J. M., et al. (2021). High-frequency burst spiking in layer 5 thick-tufted pyramids of rat primary somatosensory cortex encodes exploratory touch. Commun. Biol. 4, 1–14.
de Kock, C. P., and Sakmann, B. (2008). High frequency action potential bursts (>or= 100 Hz) in L2/3 and L5B thick tufted neurons in anaesthetized and awake rat primary somatosensory cortex. J. Physiol. 586, 3353–3364. doi: 10.1113/jphysiol.2008.155580
de Kock, C. P., Bruno, R. M., Spors, H., and Sakmann, B. (2007). Layer- and cell-type-specific suprathreshold stimulus representation in rat primary somatosensory cortex. J. Physiol. 581, 139–154. doi: 10.1113/jphysiol.2006.124321
de Souza, B. O. F., Cortes, N., and Casanova, C. (2019). Pulvinar modulates contrast responses in the visual cortex as a function of cortical hierarchy. Cereb. Cortex 30, 1068–1086. doi: 10.1093/cercor/bhz149
Delevich, K., Tucciarone, J., Huang, Z. J., and Li, B. (2015). The mediodorsal thalamus drives feedforward inhibition in the anterior cingulate cortex via parvalbumin interneurons. J. Neurosci. 35, 5743–5753. doi: 10.1523/jneurosci.4565-14.2015
Desai, N. V., and Varela, C. (2021). Distinct burst properties contribute to the functional diversity of thalamic nuclei. J. Comp. Neurol doi: 10.1002/cne.25141
Deschênes, M., Veinante, P., and Zhang, Z. W. (1998). The organization of corticothalamic projections: reciprocity versus parity. Brain Res. Brain Res. Rev. 28, 286–308. doi: 10.1016/s0165-0173(98)00017-4
Destexhe, A., Babloyantz, A., and Sejnowski, T. J. (1993). Ionic mechanisms for intrinsic slow oscillations in thalamic relay neurons. Biophys. J. 65, 1538–1552. doi: 10.1016/s0006-3495(93)81190-1
Diamond, M. E., Armstrong-James, M., Budway, M. J., and Ebner, F. F. (1992). Somatic sensory responses in the rostral sector of the posterior group (POm) and in the ventral posterior medial nucleus (VPM) of the rat thalamus: dependence on the barrel field cortex. J. Comp. Neurol. 319, 66–84. doi: 10.1002/cne.903190108
El-Boustani, S., Sermet, B. S., Foustoukos, G., Oram, T. B., Yizhar, O., and Petersen, C. C. H. (2020). Anatomically and functionally distinct thalamocortical inputs to primary and secondary mouse whisker somatosensory cortices. Nat. Commun. 11, 3342.
Elijah, D. H., Samengo, I., and Montemurro, M. A. (2015). Thalamic neuron models encode stimulus information by burst-size modulation. Front. Comput. Neurosci. 9:113. doi: 10.3389/fncom.2015.00113
Flor, H., Denke, C., Schaefer, M., and Grüsser, S. (2001). Effect of sensory discrimination training on cortical reorganisation and phantom limb pain. Lancet 357, 1763–1764. doi: 10.1016/s0140-6736(00)04890-x
Frangeul, L., Pouchelon, G., Telley, L., Lefort, S., Luscher, C., and Jabaudon, D. (2016). A cross-modal genetic framework for the development and plasticity of sensory pathways. Nature 538, 96–98. doi: 10.1038/nature19770
Gambino, F., Pagès, S., Kehayas, V., Baptista, D., Tatti, R., Carleton, A., et al. (2014). Sensory-evoked LTP driven by dendritic plateau potentials in vivo. Nature 515, 116–119. doi: 10.1038/nature13664
Gerfen, C. R., Paletzki, R., and Heintz, N. (2013). GENSAT BAC cre-recombinase driver lines to study the functional organization of cerebral cortical and basal ganglia circuits. Neuron 80, 1368–1383. doi: 10.1016/j.neuron.2013.10.016
Giber, K., Slézia, A., Bokor, H., Bodor, A. L., Ludányi, A., Katona, I., et al. (2008). Heterogeneous output pathways link the anterior pretectal nucleus with the zona incerta and the thalamus in rat. J. Comp. Neurol. 506, 122–140. doi: 10.1002/cne.21545
Golomb, D., Ahissar, E., and Kleinfeld, D. (2006). Coding of stimulus frequency by latency in thalamic networks through the interplay of GABAB-mediated feedback and stimulus shape. J. Neurophysiol. 95, 1735–1750. doi: 10.1152/jn.00734.2005
Gong, S., Doughty, M., Harbaugh, C. R., Cummins, A., Hatten, M. E., Heintz, N., et al. (2007). Targeting cre recombinase to specific neuron populations with bacterial artificial chromosome constructs. J. Neurosci. 27, 9817–9823. doi: 10.1523/jneurosci.2707-07.2007
Groh, A., Bokor, H., Mease, R. A., Plattner, V. M., Hangya, B., Stroh, A., et al. (2013). Convergence of cortical and sensory driver inputs on single thalamocortical cells. Cereb. Cortex 24, 3167–3179. doi: 10.1093/cercor/bht173
Groh, A., de Kock, C. P. J., Wimmer, V. C., Sakmann, B., and Kuner, T. (2008). Driver or coincidence detector: modal switch of a corticothalamic giant synapse controlled by spontaneous activity and short-term depression. J. Neurosci. 28, 9652–9663. doi: 10.1523/jneurosci.1554-08.2008
Groh, A., Meyer, H. S., Schmidt, E. F., Heintz, N., Sakmann, B., and Krieger, P. (2010). Cell-type specific properties of pyramidal neurons in neocortex underlying a layout that is modifiable depending on the cortical area. Cereb. Cortex 20, 826–836. doi: 10.1093/cercor/bhp152
Guillery, R. W. (1995). Anatomical evidence concerning the role of the thalamus in corticocortical communication: a brief review. J. Anat. 187 (Pt 3), 583–592.
Guo, C., Peng, J., Zhang, Y., Li, A., Li, Y., Yuan, J., et al. (2017). Single-axon level morphological analysis of corticofugal projection neurons in mouse barrel field. Sci. Rep. 7:2846.
Guo, K., Yamawaki, N., Barrett, J. M., Tapies, M., and Shepherd, G. M. G. (2020). Cortico-thalamo-cortical circuits of mouse forelimb S1 are organized primarily as recurrent loops. J. Neurosci. 40, 2849–2858. doi: 10.1523/jneurosci.2277-19.2020
Guy, J., and Staiger, J. F. (2017). The functioning of a cortex without layers. Front. Neuroanat. 11:54. doi: 10.3389/fnana.2017.00054
Halassa, M. M., and Acsady, L. (2016). Thalamic inhibition: diverse sources, diverse scales. Trends Neurosci. 39, 680–693. doi: 10.1016/j.tins.2016.08.001
Halassa, M. M., and Kastner, S. (2017). Thalamic functions in distributed cognitive control. Nat. Neurosci. 20, 1669–1679. doi: 10.1038/s41593-017-0020-1
Halassa, M. M., Chen, Z., Wimmer, R. D., Brunetti, P. M., Zhao, S., Zikopoulos, B., et al. (2014). State-dependent architecture of thalamic reticular subnetworks. Cell 158, 808–821. doi: 10.1016/j.cell.2014.06.025
Halassa, M. M., Siegle, J. H., Ritt, J. T., Ting, J. T., Feng, G., and Moore, C. I. (2011). Selective optical drive of thalamic reticular nucleus generates thalamic bursts and cortical spindles. Nat. Neurosci. 14, 1118–1120. doi: 10.1038/nn.2880
Harris, J. A., Hirokawa, K. E., Sorensen, S. A., Gu, H., Mills, M., Ng, L. L., et al. (2014). Anatomical characterization of Cre driver mice for neural circuit mapping and manipulation. Front. Neural Circuits 8:76. doi: 10.3389/fncir.2014.00076
Harris, J. A., Mihalas, S., Hirokawa, K. E., Whitesell, J. D., Knox, J. E., Bernard, A., et al. (2018). The organization of intracortical connections by layer and cell class in the mouse brain. bioRxiv [Preprint] doi: 10.1101/292961 bioRxiv: 292961,
Harris, K. D., and Shepherd, G. M. (2015). The neocortical circuit: themes and variations. Nat. Neurosci. 18, 170–181. doi: 10.1038/nn.3917
Hattox, A. M., and Nelson, S. B. (2007). Layer V neurons in mouse cortex projecting to different targets have distinct physiological properties. J. Neurophysiol. 98, 3330–3340. doi: 10.1152/jn.00397.2007
Hayashi, S., Hoerder-Suabedissen, A., Kiyokage, E., Maclachlan, C., Toida, K., Knott, G., et al. (2021). Maturation of complex synaptic connections of layer 5 cortical axons in the posterior thalamic nucleus requires SNAP25. Cereb. Cortex 31, 2625–2638. doi: 10.1093/cercor/bhaa379
Herrero, M., Barcia, C., and Navarro, J. M. (2002). Functional anatomy of thalamus and basal ganglia. Childs. Nerv. Syst. 18, 386–404. doi: 10.1007/s00381-002-0604-1
Hilscher, M. M., Leão, R. N., Edwards, S. J., Leão, K. E., and Kullander, K. (2017). Chrna2-martinotti cells synchronize layer 5 type a pyramidal cells via rebound excitation. PLoS Biol. 15:e2001392. doi: 10.1371/journal.pbio.2001392
Hines, M. L., Morse, T., Migliore, M., Carnevale, N. T., and Shepherd, G. M. (2004). ModelDB: a database to support computational neuroscience. J. Comput. Neurosci. 17, 7–11. doi: 10.1023/b:jcns.0000023869.22017.2e
Hoerder-Suabedissen, A., Hayashi, S., Upton, L., Nolan, Z., Casas-Torremocha, D., Grant, E., et al. (2018). Subset of cortical layer 6b neurons selectively innervates higher order thalamic nuclei in mice. Cereb. Cortex 28, 1882–1897. doi: 10.1093/cercor/bhy036
Hoogland, P. V., Wouterlood, F. G., Welker, E., and Van der Loos, H. (1991). Ultrastructure of giant and small thalamic terminals of cortical origin: a study of the projections from the barrel cortex in mice using Phaseolus vulgaris leuco-agglutinin (PHA-L). Exp. Brain Res. 87, 159–172. doi: 10.1007/bf00228517
Hwang, K., Bertolero, M. A., Liu, W. B., and D’Esposito, M. (2017). The human thalamus is an integrative hub for functional brain networks. J. Neurosci. 37, 5594–5607. doi: 10.1523/jneurosci.0067-17.2017
Iavarone, E., Yi, J., Shi, Y., Zandt, B.-J., O’Reilly, C., Van Geit, W., et al. (2019). Experimentally-constrained biophysical models of tonic and burst firing modes in thalamocortical neurons. PLoS Comput. Biol. 15:e1006753. doi: 10.1371/journal.pcbi.1006753
Jagirdar, R., and Chin, J. (2019). Corticothalamic network dysfunction and Alzheimer’s disease. Brain Res. 1702, 38–45. doi: 10.1016/j.brainres.2017.09.014
Jahnsen, H., and Llinas, R. (1984). Ionic basis for the electro-responsiveness and oscillatory properties of guinea-pig thalamic neurones in vitro. J. Physiol. 349, 227–247. doi: 10.1113/jphysiol.1984.sp015154
Jouhanneau, J.-S., Kremkow, J., and Poulet, J. F. A. (2018). Single synaptic inputs drive high-precision action potentials in parvalbumin expressing GABA-ergic cortical neurons in vivo. Nat. Commun. 9, 1540.
Keller, G. B., and Mrsic-Flogel, T. D. (2018). Predictive processing: a canonical cortical computation. Neuron 100, 424–435. doi: 10.1016/j.neuron.2018.10.003
Kim, J., Woo, J., Park, Y.-G., Chae, S., Jo, S., Choi, J. W., et al. (2011). Thalamic T-type Ca2+ channels mediate frontal lobe dysfunctions caused by a hypoxia-like damage in the prefrontal cortex. J. Neurosci. 31, 4063–4073. doi: 10.1523/jneurosci.4493-10.2011
Kirchgessner, M. A., Franklin, A. D., and Callaway, E. M. (2020). Context-dependent and dynamic functional influence of corticothalamic pathways to first- and higher-order visual thalamus. Proc. Natl. Acad. Sci. U. S. A. 117, 13066–13077. doi: 10.1073/pnas.2002080117
Kirchgessner, M. A., Franklin, A. D., and Callaway, E. M. (2021). Distinct “driving” versus “modulatory” influences of different visual corticothalamic pathways. bioRxiv [Preprint] doi: 10.1101/2021.03.30.437715 bioRxiv: 2021.03.30.437715.
Koralek, K. A., Jensen, K. F., and Killackey, H. P. (1988). Evidence for two complementary patterns of thalamic input to the rat somatosensory cortex. Brain Res. 463, 346–351. doi: 10.1016/0006-8993(88)90408-8
Lam, Y.-W., and Sherman, S. M. (2010). Functional organization of the somatosensory cortical layer 6 feedback to the thalamus. Cereb. Cortex 20, 13–24. doi: 10.1093/cercor/bhp077
Landisman, C. E., and Connors, B. W. (2007). VPM and PoM nuclei of the rat somatosensory thalamus: intrinsic neuronal properties and corticothalamic feedback. Cereb. Cortex 17, 2853–2865. doi: 10.1093/cercor/bhm025
Lankarany, M., Al-Basha, D., Ratté, S., and Prescott, S. A. (2019). Differentially synchronized spiking enables multiplexed neural coding. Proc. Natl. Acad. Sci. U. S. A. 116, 201812171. doi: 10.1073/pnas.1812171116
Larkum, M. (2013). A cellular mechanism for cortical associations: an organizing principle for the cerebral cortex. Trends Neurosci. 36, 141–151. doi: 10.1016/j.tins.2012.11.006
Larkum, M. E., Zhu, J. J., and Sakmann, B. (1999). A new cellular mechanism for coupling inputs arriving at different cortical layers. Nature 398, 338–341. doi: 10.1038/18686
LaTerra, D., Petryszyn, S., Rosier, M., and Palmer, L. M. (2020). Higher order thalamus encodes correct goal-directed action. bioRxiv [Preprint]. 2020.07.05.188821. doi: 10.1101/2020.07.05.188821
Lavallée, P., Urbain, N., Dufresne, C., Bokor, H., Acsády, L., and Deschênes, M. (2005). Feedforward inhibitory control of sensory information in higher-order thalamic nuclei. J. Neurosci. 25, 7489–7498. doi: 10.1523/JNEUROSCI.2301-05.2005
Lee, C. C. (2015). Exploring functions for the non-lemniscal auditory thalamus. Front. Neural Circuits 9:69. doi: 10.3389/fncir.2015.00069
Lee, C. C., and Sherman, S. M. (2008). Synaptic properties of thalamic and intracortical inputs to layer 4 of the first- and higher-order cortical areas in the auditory and somatosensory systems. J. Neurophysiol. 100, 317–326. doi: 10.1152/jn.90391.2008
Lee, C. C., and Sherman, S. M. (2011). On the classification of pathways in the auditory midbrain, thalamus, and cortex. Hear. Res. 276, 79–87. doi: 10.1016/j.heares.2010.12.012
Li, J., Bickford, M. E., and Guido, W. (2003a). Distinct firing properties of higher order thalamic relay neurons. J. Neurophysiol. 90, 291–299. doi: 10.1152/jn.01163.2002
Li, J., Guido, W., and Bickford, M. E. (2003b). Two distinct types of corticothalamic EPSPs and their contribution to short-term synaptic plasticity. J. Neurophysiol. 90, 3429–3440. doi: 10.1152/jn.00456.2003
Li, J., Wang, S., and Bickford, M. E. (2003c). Comparison of the ultrastructure of cortical and retinal terminals in the rat dorsal lateral geniculate and lateral posterior nuclei. J. Comp. Neurol. 460, 394–409. doi: 10.1002/cne.10646
Li, Y., Lopez-Huerta, V. G., Adiconis, X., Levandowski, K., Choi, S., Simmons, S. K., et al. (2020). Distinct subnetworks of the thalamic reticular nucleus. Nature. 583, 819–824. doi: 10.1038/s41586-020-2504-5
Lipshutz, D., Bahroun, Y., Golkar, S., Sengupta, A. M., and Chklovskii, D. B. (2020). A biologically plausible neural network for multi-channel Canonical Correlation Analysis. arXiv [q-bio.NC]. Available online at: http://arxiv.org/abs/2010.00525 (accessed March 26, 2021).
Llano, D. A., and Sherman, S. M. (2008). Evidence for nonreciprocal organization of the mouse auditory thalamocortical-corticothalamic projection systems. J. Comp. Neurol. 507, 1209–1227. doi: 10.1002/cne.21602
Llano, D. A., and Sherman, S. M. (2009). Differences in intrinsic properties and local network connectivity of identified layer 5 and layer 6 adult mouse auditory corticothalamic neurons support a dual corticothalamic projection hypothesis. Cereb. Cortex 19, 2810–2826. doi: 10.1093/cercor/bhp050
Llinás, R., and Jahnsen, H. (1982). Electrophysiology of mammalian thalamic neurones in vitro. Nature 297, 406–408. doi: 10.1038/297406a0
Lu, S. M., and Lin, R. C. (1993). Thalamic afferents of the rat barrel cortex: a light- and electron-microscopic study using Phaseolus vulgaris leucoagglutinin as an anterograde tracer. Somatosens. Mot. Res. 10, 1–16. doi: 10.3109/08990229309028819
Luo, L., Callaway, E. M., and Svoboda, K. (2018). Genetic dissection of neural circuits: a decade of progress. Neuron 98, 256–281. doi: 10.1016/j.neuron.2018.03.040
Mao, J., Mayer, D. J., and Price, D. D. (1993). Patterns of increased brain activity indicative of pain in a rat model of peripheral mononeuropathy. J. Neurosci. 13, 2689–2702. doi: 10.1523/jneurosci.13-06-02689.1993
Martinez-Garcia, R. I., Voelcker, B., Zaltsman, J. B., Patrick, S. L., Stevens, T. R., Connors, B. W., et al. (2020). Two dynamically distinct circuits drive inhibition in the sensory thalamus. Nature 583, 813–818. doi: 10.1038/s41586-020-2512-5
Masri, R., Quiton, R. L., Lucas, J. M., Murray, P. D., Thompson, S. M., and Keller, A. (2009). Zona incerta: a role in central pain. J. Neurophysiol. 102, 181–191. doi: 10.1152/jn.00152.2009
Masri, R., Trageser, J. C., Bezdudnaya, T., Li, Y., and Keller, A. (2006). Cholinergic regulation of the posterior medial thalamic nucleus. J. Neurophysiol. 96, 2265–2273. doi: 10.1152/jn.00476.2006
Masterson, S. P., Li, J., and Bickford, M. E. (2009). Synaptic organization of the tectorecipient zone of the rat lateral posterior nucleus. J. Comp. Neurol. 515, 647–663. doi: 10.1002/cne.22077
McAlonan, K., Brown, V. J., and Bowman, E. M. (2000). Thalamic reticular nucleus activation reflects attentional gating during classical conditioning. J. Neurosci. 20, 8897–8901. doi: 10.1523/jneurosci.20-23-08897.2000
McAlonan, K., Cavanaugh, J., and Wurtz, R. H. (2006). Attentional modulation of thalamic reticular neurons. J. Neurosci. 26, 4444–4450. doi: 10.1523/jneurosci.5602-05.2006
Mease, R. A., Kuner, T., Fairhall, A. L., and Groh, A. (2017). Multiplexed spike coding and adaptation in the thalamus. Cell Rep. 19, 1130–1140. doi: 10.1016/j.celrep.2017.04.050
Mease, R. A., Metz, M., and Groh, A. (2016a). Cortical sensory responses are enhanced by the higher-order thalamus. Cell Rep. 14, 208–215. doi: 10.1016/j.celrep.2015.12.026
Mease, R. A., Sumser, A., Sakmann, B., and Groh, A. (2016b). Cortical dependence of whisker responses in posterior medial thalamus in vivo. Cereb. Cortex 26, 3534–3543. doi: 10.1093/cercor/bhw144
Mease, R. A., Sumser, A., Sakmann, B., and Groh, A. (2016c). Corticothalamic spike transfer via the L5B-POm pathway in vivo. Cereb. Cortex 26, 3461–3475. doi: 10.1093/cercor/bhw123
Meda, K. S., Patel, T., Braz, J. M., Malik, R., Turner, M. L., Seifikar, H., et al. (2019). Microcircuit mechanisms through which mediodorsal thalamic input to anterior cingulate cortex exacerbates pain-related aversion. Neuron 102, 944–959.e3.
Meyer, H. S., Wimmer, V. C., Hemberger, M., Bruno, R. M., de Kock, C. P. J., Frick, A., et al. (2010a). Cell type-specific thalamic innervation in a column of rat vibrissal cortex. Cereb. Cortex 20, 2287–2303. doi: 10.1093/cercor/bhq069
Meyer, H. S., Wimmer, V. C., Oberlaender, M., de Kock, C. P. J., Sakmann, B., and Helmstaedter, M. (2010b). Number and laminar distribution of neurons in a thalamocortical projection column of rat vibrissal cortex. Cereb. Cortex 20, 2277–2286. doi: 10.1093/cercor/bhq067
Mitchell, A. S. (2015). The mediodorsal thalamus as a higher order thalamic relay nucleus important for learning and decision-making. Neurosci. Biobehav. Rev. 54, 76–88. doi: 10.1016/j.neubiorev.2015.03.001
Mitchell, A. S., and Gaffan, D. (2008). The magnocellular mediodorsal thalamus is necessary for memory acquisition. But Not Retrieval. J. Neurosci. 28, 258–263. doi: 10.1523/jneurosci.4922-07.2008
Mitchell, A. S., Baxter, M. G., and Gaffan, D. (2007). Dissociable performance on scene learning and strategy implementation after lesions to magnocellular mediodorsal thalamic nucleus. J. Neurosci. 27, 11888–11895. doi: 10.1523/jneurosci.1835-07.2007
Mitchell, A. S., Murray Sherman, S., Sommer, M. A., Mair, R. G., Vertes, R. P., and Chudasama, Y. (2014). Advances in understanding mechanisms of thalamic relays in cognition and behavior. J. Neurosci. 34, 15340–15346. doi: 10.1523/jneurosci.3289-14.2014
Mo, C., and Sherman, S. (2019). A sensorimotor pathway via higher-order thalamus. J. Neurosci. 39, 692–704. doi: 10.1523/jneurosci.1467-18.2018
Monchi, O., Petrides, M., Petre, V., Worsley, K., and Dagher, A. (2001). Wisconsin Card Sorting revisited: distinct neural circuits participating in different stages of the task identified by event-related functional magnetic resonance imaging. J. Neurosci. 21, 7733–7741. doi: 10.1523/jneurosci.21-19-07733.2001
Moore, J. D., Mercer Lindsay, N., Deschênes, M., and Kleinfeld, D. (2015). Vibrissa self-motion and touch are reliably encoded along the same somatosensory pathway from brainstem through thalamus. PLoS Biol. 13:e1002253. doi: 10.1371/journal.pbio.1002253
Morel, A., Magnin, M., and Jeanmonod, D. (1997). Multiarchitectonic and stereotactic atlas of the human thalamus. J. Comp. Neurol. 387, 588–630. doi: 10.1002/(sici)1096-9861(19971103)387:4<588::aid-cne8>3.0.co;2-z
Moseley, G. L., and Flor, H. (2012). Targeting cortical representations in the treatment of chronic pain: a review. Neurorehabil. Neural Repair. 26, 646–652. doi: 10.1177/1545968311433209
Mukherjee, A., Bajwa, N., Lam, N. H., Porrero, C., Clasca, F., and Halassa, M. M. (2020). Variation of connectivity across exemplar sensory and associative thalamocortical loops in the mouse. Elife 9:e62554.
Murray, P. D., Masri, R., and Keller, A. (2010). Abnormal anterior pretectal nucleus activity contributes to central pain syndrome. J. Neurophysiol. 103, 3044–3053. doi: 10.1152/jn.01070.2009
Naka, A., and Adesnik, H. (2016). Inhibitory circuits in cortical layer 5. Front. Neural Circuits 10:35. doi: 10.3389/fncir.2016.00035
Nakajima, M., and Halassa, M. M. (2017). Thalamic control of functional cortical connectivity. Curr. Opin. Neurobiol. 44, 127–131. doi: 10.1016/j.conb.2017.04.001
Naud, R., and Sprekeler, H. (2018). Sparse bursts optimize information transmission in a multiplexed neural code. Proc. Natl. Acad. Sci. U.S.A. 115, E6329–E6338. doi: 10.1073/pnas.1720995115
Nelson, A. D., and Bender, K. J. (2021). Dendritic integration dysfunction in neurodevelopmental disorders. Dev. Neurosci. 43, 1–21. doi: 10.1159/000516657
O’Connor, D. H., Peron, S. P., Huber, D., and Svoboda, K. (2010). Neural activity in barrel cortex underlying vibrissa-based object localization in mice. Neuron 67, 1048–1061. doi: 10.1016/j.neuron.2010.08.026
Odoj, K., Brawek, B., Asavapanumas, N., Mojtahedi, N., Heneka, M. T., and Garaschuk, O. (2021). In vivo mechanisms of cortical network dysfunction induced by systemic inflammation. Brain Behav. Immun. 96, 113–126. doi: 10.1016/j.bbi.2021.05.021
Ojima, H. (1994). Terminal morphology and distribution of corticothalamic fibers originating from layers 5 and 6 of cat primary auditory cortex. Cereb. Cortex 4, 646–663. doi: 10.1093/cercor/4.6.646
Otsuka, T., and Kawaguchi, Y. (2021). Pyramidal cell subtype-dependent cortical oscillatory activity regulates motor learning. Commun. Biol. 4:495.
Pagès, S., Chenouard, N., Chéreau, R., Kouskoff, V., Gambino, F., and Holtmaat, A. (2021). An increase in dendritic plateau potentials is associated with experience-dependent cortical map reorganization. Proc. Natl. Acad. Sci. U.S.A. 118, e2024920118. doi: 10.1073/pnas.2024920118
Pardi, M. B., Vogenstahl, J., Dalmay, T., Spanò, T., Pu, D.-L., Naumann, L. B., et al. (2020). A thalamocortical top-down circuit for associative memory. Science 370, 844–848. doi: 10.1126/science.abc2399
Park, A., Uddin, O., Li, Y., Masri, R., and Keller, A. (2018). Pain after spinal cord injury is associated with abnormal presynaptic inhibition in the posterior nucleus of the thalamus. J. Pain 19, 727.e1–727.e15.
Park, S., Sohn, J.-W., Cho, J., and Huh, Y. (2019). A computational modeling reveals that strength of inhibitory input, E/I balance, and distance of excitatory input modulate thalamocortical bursting properties. Exp. Neurobiol. 28:568. doi: 10.5607/en.2019.28.5.568
Parnaudeau, S., O’Neill, P.-K., Bolkan, S. S., Ward, R. D., Abbas, A. I., Roth, B. L., et al. (2013). Inhibition of mediodorsal thalamus disrupts thalamofrontal connectivity and cognition. Neuron 77, 1151–1162. doi: 10.1016/j.neuron.2013.01.038
Payeur, A., Guerguiev, J., Zenke, F., Richards, B. A., and Naud, R. (2021). Burst-dependent synaptic plasticity can coordinate learning in hierarchical circuits. Nat. Neurosci. 24, 1010–1019. doi: 10.1038/s41593-021-00857-x
Pergola, G., Danet, L., Pitel, A.-L., Carlesimo, G. A., Segobin, S., Pariente, J., et al. (2018). The regulatory role of the human mediodorsal thalamus. Trends Cogn. Sci. 22, 1011–1025.
Perl, E. R., and Whitlock, D. G. (1961). Somatic stimuli exciting spinothalamic projections to thalamic neurons in cat and monkey. Exp. Neurol. 3, 256–296. doi: 10.1016/0014-4886(61)90016-4
Perry, B. A. L., Lomi, E., and Mitchell, A. S. (2021). Thalamocortical interactions in cognition and disease: the mediodorsal and anterior thalamic nuclei. Neurosci. Biobehav. Rev. (in press). doi: 10.1016/j.neubiorev.2021.05.032
Petreanu, L., Mao, T., Sternson, S. M., and Svoboda, K. (2009). The subcellular organization of neocortical excitatory connections. Nature 457, 1142–1145. doi: 10.1038/nature07709
Phillips, J. W., Schulmann, A., Hara, E., Winnubst, J., Liu, C., Valakh, V., et al. (2019). A repeated molecular architecture across thalamic pathways. Nat. Neurosci. 22, 1925–1935. doi: 10.1038/s41593-019-0483-3
Pinault, D. (2004). The thalamic reticular nucleus: structure, function and concept. Brain Res. Brain Res. Rev. 46, 1–31. doi: 10.1016/j.brainresrev.2004.04.008
Prasad, J. A., Carroll, B. J., and Sherman, S. M. (2020). Layer 5 corticofugal projections from diverse cortical areas: variations on a pattern of thalamic and extra-thalamic targets. J. Neurosci. 40, 5785–5796. doi: 10.1523/JNEUROSCI.0529-20.2020
Purushothaman, G., Marion, R., Li, K., and Casagrande, V. A. (2012). Gating and control of primary visual cortex by pulvinar. Nat. Neurosci. 15, 905–912. doi: 10.1038/nn.3106
Ramaswamy, S., and Markram, H. (2015). Anatomy and physiology of the thick-tufted layer 5 pyramidal neuron. Front. Cell. Neurosci. 9:233. doi: 10.3389/fncel.2015.00233
Ramcharan, E. J., Gnadt, J. W., and Sherman, S. M. (2005). Higher-order thalamic relays burst more than first-order relays. Proc. Natl. Acad. Sci. U. S. A. 102, 12236–12241. doi: 10.1073/pnas.0502843102
Redinbaugh, M. J., Phillips, J. M., Kambi, N. A., Mohanta, S., Andryk, S., Dooley, G. L., et al. (2020). Thalamus modulates consciousness via layer-specific control of cortex. Neuron 106, 66–75.e12.
Reichova, I., and Sherman, S. M. (2004). Somatosensory corticothalamic projections: distinguishing drivers from modulators. J. Neurophysiol. 92, 2185–2197. doi: 10.1152/jn.00322.2004
Resnik, J., and Polley, D. B. (2017). Fast-spiking GABA circuit dynamics in the auditory cortex predict recovery of sensory processing following peripheral nerve damage. Elife 6:e21452. doi: 10.7554/eLife.21452
Rikhye, R. V., Gilra, A., and Halassa, M. M. (2018). Thalamic regulation of switching between cortical representations enables cognitive flexibility. Nat. Neurosci. 21, 1753–1763. doi: 10.1038/s41593-018-0269-z
Rinaldi, P. C., Young, R. F., Albe-Fessard, D., and Chodakiewitz, J. (1991). Spontaneous neuronal hyperactivity in the medial and intralaminar thalamic nuclei of patients with deafferentation pain. J. Neurosurg. 74, 415–421. doi: 10.3171/jns.1991.74.3.0415
Rockland, K. S. (2019). Corticothalamic axon morphologies and network architecture. Eur. J. Neurosci. 49, 969–977. doi: 10.1111/ejn.13910
Rodriguez-Moreno, J., Porrero, C., Rollenhagen, A., Rubio-Teves, M., Casas-Torremocha, D., Alonso-Nanclares, L., et al. (2020). Area-specific synapse structure in branched posterior nucleus axons reveals a new level of complexity in thalamocortical networks. J. Neurosci. 40, 2663–2679. doi: 10.1523/jneurosci.2886-19.2020
Rojas-Piloni, G., Guest, J. M., Egger, R., Johnson, A. S., Sakmann, B., and Oberlaender, M. (2017). Relationships between structure, in vivo function and long-range axonal target of cortical pyramidal tract neurons. Nat. Commun. 8:870.
Rouiller, E. M., and Welker, E. (2000). A comparative analysis of the morphology of corticothalamic projections in mammals. Brain Res. Bull. 53, 727–741. doi: 10.1016/s0361-9230(00)00364-6
Rovó, Z., Ulbert, I., and Acsády, L. (2012). Drivers of the primate thalamus. J. Neurosci. 32, 17894–17908. doi: 10.1523/jneurosci.2815-12.2012
Saadé, N. E., Al Amin, H., Abdel Baki, S., Chalouhi, S., Jabbur, S. J., and Atweh, S. F. (2007). Reversible attenuation of neuropathic-like manifestations in rats by lesions or local blocks of the intralaminar or the medial thalamic nuclei. Exp. Neurol. 204, 205–219. doi: 10.1016/j.expneurol.2006.10.009
Saalmann, Y. B., Pinsk, M. A., Wang, L., Li, X., and Kastner, S. (2012). The pulvinar regulates information transmission between cortical areas based on attention demands. Science 337, 753–756. doi: 10.1126/science.1223082
Sakmann, B. (2017). From single cells and single columns to cortical networks: dendritic excitability, coincidence detection and synaptic transmission in brain slices and brains. Exp. Physiol. 102, 489–521.
Sampathkumar, V., Miller-Hansen, A., Sherman, S. M., and Kasthuri, N. (2021). Integration of signals from different cortical areas in higher order thalamic neurons. Proc. Natl. Acad. Sci. U. S. A 118:e2104137118. doi: 10.1073/pnas.2104137118
Schmitt, L. I., Wimmer, R. D., Nakajima, M., Happ, M., Mofakham, S., and Halassa, M. M. (2017). Thalamic amplification of cortical connectivity sustains attentional control. Nature 545, 219–223. doi: 10.1038/nature22073
Schuman, B., Dellal, S., Prönneke, A., Machold, R., and Rudy, B. (2021). Neocortical layer 1: an elegant solution to top-down and bottom-up integration. Annu. Rev. Neurosci. 44, 221–252. doi: 10.1146/annurev-neuro-100520-012117
Semedo, J. D., Zandvakili, A., Machens, C. K., Yu, B. M., and Kohn, A. (2019). Cortical areas interact through a communication subspace. Neuron 102, 249–259.e4.
Senzai, Y., Fernandez-Ruiz, A., and Buzsáki, G. (2019). Layer-specific physiological features and interlaminar interactions in the primary visual cortex of the mouse. Neuron 101, 500–513.e5.
Seol, M., and Kuner, T. (2015). Ionotropic glutamate receptor GluA4 and T-type calcium channel Cav 3.1 subunits control key aspects of synaptic transmission at the mouse L5B-POm giant synapse. Eur. J. Neurosci. 42, 3033–3044. doi: 10.1111/ejn.13084
Sermet, B. S., Truschow, P., Feyerabend, M., Mayrhofer, J. M., Oram, T. B., Yizhar, O., et al. (2019). Pathway-, layer- and cell-type-specific thalamic input to mouse barrel cortex. Elife 8:e52665.
Shai, A. S., Anastassiou, C. A., Larkum, M. E., and Koch, C. (2015). Physiology of layer 5 pyramidal neurons in mouse primary visual cortex: coincidence detection through bursting. PLoS Comput. Biol. 11:e1004090. doi: 10.1371/journal.pcbi.1004090
Shepherd, G. M. G., and Yamawaki, N. (2021). Untangling the cortico-thalamo-cortical loop: cellular pieces of a knotty circuit puzzle. Nat. Rev. Neurosci. 22, 389–406. doi: 10.1038/s41583-021-00459-3
Sherman, S. M. (2001). Tonic and burst firing: dual modes of thalamocortical relay. Trends Neurosci. 24, 122–126. doi: 10.1016/s0166-2236(00)01714-8
Sherman, S. M. (2007). The thalamus is more than just a relay. Curr. Opin. Neurobiol. 17, 417–422. doi: 10.1016/j.conb.2007.07.003
Sherman, S. M. (2016). Thalamus plays a central role in ongoing cortical functioning. Nat. Neurosci. 19, 533–541. doi: 10.1038/nn.4269
Sherman, S. M. (2017). Functioning of circuits connecting thalamus and cortex. Compr. Physiol. 7, 713–739. doi: 10.1002/cphy.c160032
Sherman, S. M., and Guillery, R. W. (2002). The role of the thalamus in the flow of information to the cortex. Philos. Trans. R. Soc. Lond. B Biol. Sci. 357, 1695–1708. doi: 10.1098/rstb.2002.1161
Sherman, S. M., and Guillery, R. W. (2006). Exploring The Thalamus And Its Role In Cortical Function. Cambridge, MA: MIT Press.
Sherman, S. M., and Guillery, R. W. (2011). Distinct functions for direct and transthalamic corticocortical connections. J. Neurophysiol. 106, 1068–1077. doi: 10.1152/jn.00429.2011
Sherman, S. M., and Usrey, W. M. (2021). Cortical control of behavior and attention from an evolutionary perspective. Neuron S0896-6273(21)00462-1. doi: 10.1016/j.neuron.2021.06.021
Sherman, S., and Guillery, R. W. (2013). Functional Connections of Cortical Areas: A New View from the Thalamus. Cambridge, MA: MIT Press.
Silberberg, G., and Markram, H. (2007). Disynaptic inhibition between neocortical pyramidal cells mediated by martinotti cells. Neuron 53, 735–746. doi: 10.1016/j.neuron.2007.02.012
Smith, Y., Wichmann, T., and DeLong, M. R. (2014). Corticostriatal and mesocortical dopamine systems: do species differences matter? Nat. Rev. Neurosci. 15:63. doi: 10.1038/nrn3469-c1
Snow, J. C., Allen, H. A., Rafal, R. D., and Humphreys, G. W. (2009). Impaired attentional selection following lesions to human pulvinar: Evidence for homology between human and monkey. Proc. Natl. Acad. Sci. U.S.A. 106, 4054–4059. doi: 10.1073/pnas.0810086106
Stitt, I., Zhou, Z. C., Radtke-Schuller, S., and Fröhlich, F. (2018). Arousal dependent modulation of thalamo-cortical functional interaction. Nat. Commun. 9, 1–13.
Sumser, A., Mease, R. A., Sakmann, B., and Groh, A. (2017). Organization and somatotopy of corticothalamic projections from L5B in mouse barrel cortex. Proc. Natl. Acad. Sci. U.S.A. 114, 8853–8858. doi: 10.1073/pnas.1704302114
Suzuki, M., and Larkum, M. E. (2020). General anesthesia decouples cortical pyramidal neurons. Cell 180, 666–676.e13.
Takahashi, N., Ebner, C., Sigl-Glöckner, J., Moberg, S., Nierwetberg, S., and Larkum, M. E. (2020). Active dendritic currents gate descending cortical outputs in perception. Nat. Neurosci. 23, 1277–1285. doi: 10.1038/s41593-020-0677-8
Takahashi, N., Oertner, T. G., Hegemann, P., and Larkum, M. E. (2016). Active cortical dendrites modulate perception. Science 354, 1587–1590. doi: 10.1126/science.aah6066
Tan, L. L., and Kuner, R. (2021). Neocortical circuits in pain and pain relief. Nat. Rev. Neurosci 22, 458–471. doi: 10.1038/s41583-021-00468-2
Theyel, B. B., Llano, D. A., and Sherman, S. M. (2010). The corticothalamocortical circuit drives higher-order cortex in the mouse. Nat. Neurosci. 13, 84–88. doi: 10.1038/nn.2449
Thomson, A. M. (2010). Neocortical layer 6, a review. Front. Neuroanat. 4:13. doi: 10.3389/fnana.2010.00013
Trageser, J. C., Burke, K. A., Masri, R., Li, Y., Sellers, L., and Keller, A. (2006). State-dependent gating of sensory inputs by zona incerta. J. Neurophysiol. 96, 1456–1463. doi: 10.1152/jn.00423.2006
Urbain, N., Salin, P. A., Libourel, P. A., Comte, J. C., Gentet, L. J., and Petersen, C. C. (2015). Whisking-related changes in neuronal firing and membrane potential dynamics in the somatosensory thalamus of awake mice. Cell Rep. 13, 647–656. doi: 10.1016/j.celrep.2015.09.029
Usrey, W. M., and Sherman, S. M. (2019). Corticofugal circuits: communication lines from the cortex to the rest of the brain. J. Comp. Neurol. 527, 640–650. doi: 10.1002/cne.24423
Van Horn, S. C., and Sherman, S. M. (2004). Differences in projection patterns between large and small corticothalamic terminals. J. Comp. Neurol. 475, 406–415. doi: 10.1002/cne.20187
Varela, C., and Sherman, S. M. (2007). Differences in response to muscarinic activation between first and higher order thalamic relays. J. Neurophysiol. 98, 3538–3547. doi: 10.1152/jn.00578.2007
Varela, C., and Sherman, S. M. (2009). Differences in response to serotonergic activation between first and higher order thalamic nuclei. Cereb. Cortex 19, 1776–1786. doi: 10.1093/cercor/bhn208
Veinante, P., Lavallée, P., and Deschênes, M. (2000). Corticothalamic projections from layer 5 of the vibrissal barrel cortex in the rat. J. Comp. Neurol. 424, 197–204. doi: 10.1002/1096-9861(20000821)424:2<197::aid-cne1>3.0.co;2-6
Viaene, A. N., Petrof, I., and Murray Sherman, S. (2011). Properties of the thalamic projection from the posterior medial nucleus to primary and secondary somatosensory cortices in the mouse. Proc. Natl. Acad. Sci. U.S.A. 108, 18156–18161. doi: 10.1073/pnas.1114828108
Wang, J.-Y., Chang, J.-Y., Woodward, D. J., Baccalá, L. A., Han, J.-S., and Luo, F. (2007). Corticofugal influences on thalamic neurons during nociceptive transmission in awake rats. Synapse 61, 335–342. doi: 10.1002/syn.20375
Wei, H., Bonjean, M., Petry, H. M., Sejnowski, T. J., and Bickford, M. E. (2011). Thalamic burst firing propensity: a comparison of the dorsal lateral geniculate and pulvinar nuclei in the tree shrew. J. Neurosci. 31, 17287–17299. doi: 10.1523/jneurosci.6431-10.2011
Whilden, C. M., Chevée, M., An, S. Y., and Brown, S. P. (2021). The synaptic inputs and thalamic projections of two classes of layer 6 corticothalamic neurons in primary somatosensory cortex of the mouse. J. Comp. Neurol. doi: 10.1002/cne.25163
Whitlock, D. G., and Perl, E. R. (1961). Thalamic projections of spinothalamic pathways in monkey. Exp. Neurol. 3, 240–255. doi: 10.1016/0014-4886(61)90015-2
Whitt, J. L., Masri, R., Pulimood, N. S., and Keller, A. (2013). Pathological activity in mediodorsal thalamus of rats with spinal cord injury pain. J. Neurosci. 33, 3915–3926. doi: 10.1523/jneurosci.2639-12.2013
Wilke, M., Turchi, J., Smith, K., Mishkin, M., and Leopold, D. A. (2010). Pulvinar inactivation disrupts selection of movement plans. J. Neurosci. 30, 8650–8659. doi: 10.1523/jneurosci.0953-10.2010
Williams, L. E., and Holtmaat, A. (2019). Higher-order thalamocortical inputs gate synaptic long-term potentiation via disinhibition. Neuron 101, 91–102.e4.
Williamson, R. S., and Polley, D. B. (2019). Parallel pathways for sound processing and functional connectivity among layer 5 and 6 auditory corticofugal neurons. Elife 8:e42974. doi: 10.7554/eLife.42974
Wimmer, V. C., Bruno, R. M., de Kock, C. P. J., Kuner, T., and Sakmann, B. (2010). Dimensions of a projection column and architecture of VPM and POm axons in rat vibrissal cortex. Cereb. Cortex 20, 2265–2276. doi: 10.1093/cercor/bhq068
Wolfart, J., Debay, D., Le Masson, G., Destexhe, A., and Bal, T. (2005). Synaptic background activity controls spike transfer from thalamus to cortex. Nat. Neurosci. 8, 1760–1767. doi: 10.1038/nn1591
Xiao, D., Zikopoulos, B., and Barbas, H. (2009). Laminar and modular organization of prefrontal projections to multiple thalamic nuclei. Neuroscience 161, 1067–1081. doi: 10.1016/j.neuroscience.2009.04.034
Yu, C., Li, Y., Stitt, I. M., Zhou, Z. C., Sellers, K. K., and Frohlich, F. (2018). Theta oscillations organize spiking activity in higher-order visual thalamus during sustained attention. eNeuro 5:ENEURO.0384-17.2018. doi: 10.1523/ENEURO.0384-17.2018
Yu, J., Anderson, C. T., Kiritani, T., Sheets, P. L., Wokosin, D. L., Wood, L., et al. (2008). Local-circuit phenotypes of layer 5 neurons in motor-frontal cortex of YFP-H mice. Front. Neural Circuits 2:6. doi: 10.3389/neuro.04.006.2008
Yuan, R., Di, X., Taylor, P. A., Gohel, S., Tsai, Y.-H., and Biswal, B. B. (2016). Functional topography of the thalamocortical system in human. Brain Struct. Funct. 221, 1971–1984. doi: 10.1007/s00429-015-1018-7
Zeldenrust, F., Chameau, P., and Wadman, W. J. (2018a). Spike and burst coding in thalamocortical relay cells. PLoS Comput. Biol. 14:e1005960. doi: Spike and burst coding in thalamocortical relay
Zeldenrust, F., Wadman, W. J., and Englitz, B. (2018b). Neural Coding With Bursts-Current State and Future Perspectives. Front. Comput. Neurosci. 12:48. doi: 10.3389/fncom.2018.00048
Keywords: pyramidal neurons, corticothalamic, higher-order thalamus, bursting, thalamus, layer 5, neural coding, pathology
Citation: Mease RA and Gonzalez AJ (2021) Corticothalamic Pathways From Layer 5: Emerging Roles in Computation and Pathology. Front. Neural Circuits 15:730211. doi: 10.3389/fncir.2021.730211
Received: 24 June 2021; Accepted: 10 August 2021;
Published: 09 September 2021.
Edited by:
Max F. K. Happel, MSB Medical School Berlin, GermanyReviewed by:
Ralf D. Wimmer, Massachusetts Institute of Technology, United StatesMathieu Wolff, Centre National de la Recherche Scientifique (CNRS), France
Copyright © 2021 Mease and Gonzalez. This is an open-access article distributed under the terms of the Creative Commons Attribution License (CC BY). The use, distribution or reproduction in other forums is permitted, provided the original author(s) and the copyright owner(s) are credited and that the original publication in this journal is cited, in accordance with accepted academic practice. No use, distribution or reproduction is permitted which does not comply with these terms.
*Correspondence: Rebecca A. Mease, cmViZWNjYS5tZWFzZUBwaHlzaW9sb2dpZS51bmktaGVpZGVsYmVyZy5kZQ==