- 1Department of Neuropharmacology, Graduate School of Pharmaceutical Sciences, Nagoya City University, Nagoya, Japan
- 2Graduate School of Medicine, Tohoku University, Sendai, Japan
- 3Systems Neuroscience Section, Primate Research Institute, Kyoto University, Inuyama, Japan
- 4Department of Anesthesiology, Graduate School of Medicine, Nagoya City University, Nagoya, Japan
- 5Graduate School of Biomedical Engineering, Tohoku University, Sendai, Japan
- 6Division of Health Sciences, Department of Medical Physics and Engineering, Graduate School of Medicine, Osaka University, Suita, Japan
Human brain imaging studies have revealed several regions that are activated in patients with chronic pain. In rodent brains, functional changes due to chronic pain have not been fully elucidated, as brain imaging techniques such as functional magnetic resonance imaging and positron emission tomography (PET) require the use of anesthesia to suppress movement. Consequently, conclusions derived from existing imaging studies in rodents may not accurately reflect brain activity under awake conditions. In this study, we used quantitative activation-induced manganese-enhanced magnetic resonance imaging to directly capture the previous brain activity of awake mice. We also observed and quantified the brain activity of the spared nerve injury (SNI) neuropathic pain model during awake conditions. SNI-operated mice exhibited a robust decrease of mechanical nociceptive threshold 14 days after nerve injury. Imaging on SNI-operated mice revealed increased neural activity in the limbic system and secondary somatosensory, sensory-motor, piriform, and insular cortex. We present the first study demonstrating a direct measurement of awake neural activity in a neuropathic pain mouse model.
Introduction
Chronic pain sensitizes against somatosensory stimuli and induces anxiety and depression in patients (Sah et al., 2003). These physical and psychological changes are caused by chronic alterations in brain activity. Neuropathic pain is caused by abnormal neural excitation elicited by conditions such as nerve injury, diabetes mellitus, herpes simplex virus infection, and human immunodeficiency virus infection. Since neuropathic pain is resistant against many analgesics including opioids, there are very few therapeutic options. It is established that neuropathic pain results in neuroplasticity that can be observed in several brain regions (Jaggi and Singh, 2011). This activation complements the fact that pain is a subjective and multidimensional experiences constructed by a combination of sensory, emotional, and cognitive experiences (Tracey, 2010).
An obvious difference between humans and rodents is the communication methods regarding their physical conditions. Human patients can verbally report pain, while animals may exhibit pain through behavioral changes. Currently, assessing persistent pain using animal models is difficult because the animals often do not exhibit any pain-related behaviors despite experiencing pain. Efforts to measure persistent pain in rodents include the use of ultrasonic vocalizations, facial expressions, altered locomotion, and altered sleep patterns (Jourdan et al., 2002; Wallace et al., 2005; Langford et al., 2010; Mogil et al., 2010; Urban et al., 2011). As these measures of persistent pain did not yield consistent and conclusive results, assessment of neuropathic pain in rodents typically relies on behavioral measures of mechanical and/or thermal thresholds (D’Amour and Smith, 1941; Le Bars et al., 2001). These types of measurements may be difficult to use as interpretations of neuropathic pain in human patients (Gottrup et al., 1998; Backonja and Stacey, 2004; Baron et al., 2009). Based on these reports, it is clear that adequate assessment methods for chronic pain in rodents are still lacking (Borsook et al., 2011).
In humans, imaging studies have revealed brain regions activated by chronic pain, including the primary somatosensory, secondary somatosensory, prefrontal, insular, anterior cingulate cortices and the thalamus (Baliki et al., 2006; Howard et al., 2012). In vivo brain imaging in rodents has revealed activation in homologous brain regions in response to acute noxious stimuli (Borsook et al., 2011; Thompson and Bushnell, 2012). Using positron emission tomography (PET) with the metabolic tracer [18F]fluorodeoxyglucose (FDG) on a rodent model of neuropathic pain, increased brain activity was observed in the somatosensory cortex, a change not seen in rodents assessed under general anesthesia (Thompson et al., 2014). Increased activation was also observed in the prefrontal-limbic-brainstem areas in an awake rat model of neuropathic pain using micro-PET with [18F]FDG (Kim et al., 2014). However, direct measurement of neural activity in awake resting-state rodents has not been successful.
Activation-induced manganese-enhanced magnetic resonance imaging (AIM-MRI) is a method for examining brain activation patterns in rodents (Lin and Koretsky, 1997; Aoki et al., 2004). Manganese ion (Mn2+) is an excellent MRI-detectable T1 contrast agent (Duyn and Koretsky, 2008; Silva and Bock, 2008), because it shortens the longitudinal relaxation time (T1) of proton (H+). Mn2+ can pass through voltage-gated calcium channels (Nelson, 1986; Narita et al., 1990), and accumulates in active neurons (Kikuta et al., 2015). The accumulated Mn2+ in neurons is maintained for more than 48 h (Yu et al., 2005; Tanihira et al., unpublished observation). Kikuta et al. (2015) showed that the amount of accumulated Mn2+ is linearly correlated with neuronal activity. It is important to note that Mn2+ is taken up into brain parenchyma after intraperitoneal (i.p.) administration of MnCl2 (Kikuta et al., 2015; Tanihira et al., unpublished observation). Since the slow and uniform diffusion of Mn2+ into the entire extracellular brain space enables recording of the history of neuronal activity in awake, freely moving animals (Van der Linden et al., 2007), AIM-MRI can map the activated brain regions after MnCl2 administration (Duyn and Koretsky, 2008; Silva and Bock, 2008; Tambalo et al., 2009; Koretsky, 2012; Kikuta et al., 2015).
The present study investigates the steady-state brain activity of neuropathic pain in awake mice. AIM-MRI with quantitative T1 measurement (qAIM-MRI), quantifying T1 values (Tambalo et al., 2009; Kikuta et al., 2015), was used on a spared nerve injury (SNI) model of neuropathic pain (Yamamoto et al., 2016). We found that steady-state brain activity in an awake neuropathic pain mouse model showed increased activity of the prefrontal-limbic-basal ganglia circuit. To our knowledge, this study is first to directly measure neural activity in animals with neuropathic pain under awake conditions.
Materials and Methods
Ethical Issues
All animal studies were approved by the Animal Care Committee of the Graduate School of Pharmaceutical Sciences, Nagoya City University and by the Tohoku University Committee for Animal Experiments. Experiments were conducted in accordance to the guidelines of the National Institute of Health and the Japanese Pharmacological Society.
Animals
We used male C57BL/6J mice (6 weeks old; CLEA Japan, Shizuoka, Japan). All mice were housed in a room maintained at 23 ± 2°C with an alternating 12-h light-dark cycle and had ad libitum access to food and water. Each mouse was used only once.
Spared Nerve Injury Model
The surgical procedure for producing the SNI model was originally described by Decosterd and Woolf (2000). Animals were anesthetized with isoflurane (4% for induction, 2% for maintenance). An incision was made in the skin on the lateral surface of the left thigh, followed by a section through the biceps femoris muscle to expose the sciatic nerves. The common peroneal and tibial nerves were then tightly ligated with 8–0 silk suture, sectioned distal to the ligation, and 1 mm of the distal nerve stump was cut. The sural nerve was left intact, taking care not to stretch it. Sham-operated controls were subjected to exposure of the sciatic nerve and its branches without any lesions.
Tactile Allodynia
Tactile allodynia was evaluated by measuring the hind paw withdrawal responses to von Frey filaments (Touch-test® sensory Evaluators; North Coast Medical, Gilroy, CA, United States), with a pressure ranging from 0.02 to 1.4 g (0.02, 0.04, 0.07, 0.16, 0.4, 0.6, 1.0, and 1.4 g). Mice were placed in cages with wire-mesh floors. The 50% likelihood of a paw withdrawal response (50% threshold) was determined using the up-down method (Dixon, 1965). Testing was initiated with the 0.16 g filament, and each filament was applied perpendicularly within the area innervated by the sural nerve on the lateral plantar surface of the left hind paw with sufficient force to cause slight bending of the filament for a duration of about 3 s (Decosterd and Woolf, 2000). If a positive response (lifting of the hind paw) was elicited, the next weakest filament was used. If a negative response (absence of hind paw withdrawal) was elicited, the next strongest filament was used. We continued until four measurements had been obtained after an initial change in behavior or until four consecutive positive (0.02 g) or five negative (1.4 g) scores had been obtained. The resulting scores were used to calculate the 50% threshold (Chaplan et al., 1994).
Quantitative Activation-Induced, Manganese-Enhanced Magnetic Resonance Imaging, and MR Image Analysis
Two weeks after SNI surgery, mice in both SNI and sham-operated groups were injected with MnCl2 solution (0.2 mmol/kg in saline, intraperitoneally) twice at 24-h intervals (Kikuta et al., 2015). The methods for MRI acquisition were described previously (Kikuta et al., 2015). Brief methods are as follows: MRI acquisition was conducted 48 h after the second MnCl2 administration. The animals were anesthetized with 1–2% isoflurane (Mylan). Body temperature was maintained by circulation of heated water under the body. For T1 measurement of the brain, rapid acquisition with relaxation enhancement (RARE), and variable repetition time (RARE-VTR) pulse sequence with 7 TR values (450, 600, 900, 1500, 2500, 4500, and 7500 ms) was used with effective echo time (TEeff) = 8.1 ms, matrix size = 128 × 128, field-of-view (FOV) = 1.6 cm2 × 1.6 cm2, slice thickness = 0.5 mm, and number of slices = 20. Multislice, fast spin-echo T2-weighted images (RARE, TEeff = 22 ms, TR = 2500 ms) were acquired and used to co-register images to the mouse brain template acquired in advance (Kikuta et al., 2015). All measurements were carried out in a 9.4 T MRI scanner (AV400WB, Bruker) equipped with a 45 G/cm gradient and a 38 mm 1H volume coil (Bruker). The total time required to obtain all magnetic resonance images was approximately 45 min.
MRI analysis methods were also described previously (Kikuta et al., 2015). Brief methods are as follows: after spatial filtering, parametric T1 maps were calculated pixel-by-pixel by fitting with the following equation using Para Vision 5.1 software (Bruker BioSpin).
where SI is signal intensity in each pixel.
The T2-weighted images were registered to the previously described T2-weighted template image (Kikuta et al., 2015), and the T1 maps were co-registered simultaneously using SPM12 software (Wellcome Trust Center for Neuroimaging, University College of London). Using the mouse brain atlas (Lein et al., 2007) Allen Institute for Brain Science. Allen Mouse Brain Atlas1) registered to the T2-weighted template image (Kikuta et al., 2015), we could then identify brain regions by querying structures from the brain atlas. An unpaired Student’s t-test was used to determine which T1 voxels decreased or increased in the SNI group compared with the sham group using SPM12. A parametric map of voxels with statistically significant changes in T1 was created and overlaid on the T2-weighted template image.
ROIs were set in the area where significant changes in T1 values were observed in SPM analysis. The ROI size was 3 × 3 × 3 voxels. Average T1 values in ROIs were calculated for statistical analysis.
Statistical Analyses
Statistical analysis was performed using R, MATLAB (Mathworks), and SPM12 software. For the statistical parametric mapping (SPM) analysis, statistical significance (p < 0.025) was assessed by the unpaired Student’s t-test using SPM12 software. To ascertain the variability of T1 values within each group, we employed the Mann-Whitney U-test, and if a significant difference (p < 0.05) was detected, we confirmed the significance of the mean T1 values by bootstrapping within a 95% confidence interval after 1,000 randomizations. All data are presented as mean ± standard error of mean.
Results
Spared Nerve Injury Model Mice Exhibited Tactile Allodynia at 14 Days After Surgery
Fourteen days after SNI surgery, mice exhibited a prominent decrease of mechanical nociceptive threshold in the injured paw (Figure 1). In contrast, sham-operated mice did not exhibit the reduction of mechanical nociceptive threshold (Figure 1).
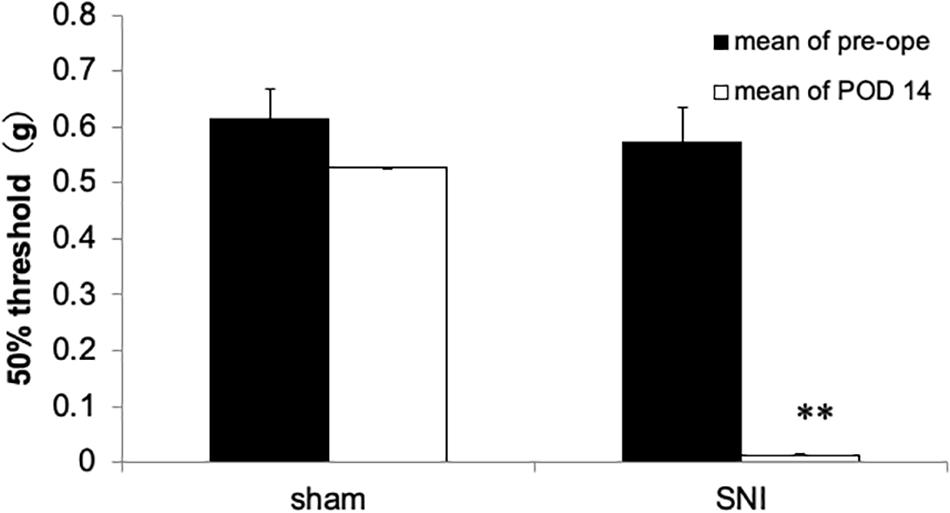
Figure 1. Mechanical nociceptive threshold of the hind paw in spared nerve injury (SNI) and sham-operated mice 14 days after nerve ligation. The SNI group exhibited decreased mechanical nociceptive threshold compared with the sham-operated group. Each column represents the mean ± SEM from 5 (sham group) and 8 (SNI group). Where error bars are not visible, they are smaller than the symbol. ∗∗p < 0.01 vs. before surgery (pre, Student’s t-test).
qAIM-MRI Showed Increased Activation of Several Brain Region in SNI Model Mice
To elucidate the activated brain regions in neuropathic pain, qAIM-MRI was conducted on SNI and sham-operated groups of mice. To visualize regions with significantly elevated activity, the voxels with significant T1-shortening in SNI mice (n = 6) in comparison to sham-operated mice (n = 6) were defined as those with p-values below 0.025 by SPM analysis (Figures 2, 3). Within the limbic structure, the central amygdala (CeA), nucleus accumbens (NAc), caudate putamen (CPu), globus pallidum (GP), posterior cingulate cortex (PCC), and ventral posterolateral nucleus of thalamus (VPL) showed significant shortening of T1 (Figure 2). In the cortex, the regions showing significant T1 shortening were observed in the secondary somatosensory cortex (S2), sensory-motor cortex (S1, M1, and M2), and piriform cortex (Pir), and insular cortex (IC) (Figure 3). None of the brain regions exhibited decreased activity under chronic pain. The t-values and p-values resulting from the Student’s t-test and the brain coordinates are indicated in Table 1. To confirm the variability across animals, we conducted Mann-Whitney U-test and bootstrap analysis of the mean T1 value in each ROI. Significant shortening of T1 values were detected in NAc (Sham; 2.35 ± 0.04, SNI; 2.16 ± 0.03, p < 0.05), CPu (Sham; 2.50 ± 0.06, SNI; 2.35 ± 0.02, p < 0.01), GP (Sham; 2.23 ± 0.04, SNI; 2.09 ± 0.02, p < 0.05), VPL (Sham; 2.20 ± 0.03, SNI; 2.06 ± 0.02, p < 0.005), Pir (Sham; 2.04 ± 0.04, SNI; 1.86 ± 0.04, p < 0.05), right S2 (Sham; 2.52 ± 0.03, SNI; 2.41 ± 0.03, p < 0.05), and left S2 (Sham; 2.67 ± 0.04, SNI; 2.51 ± 0.04, p < 0.05).
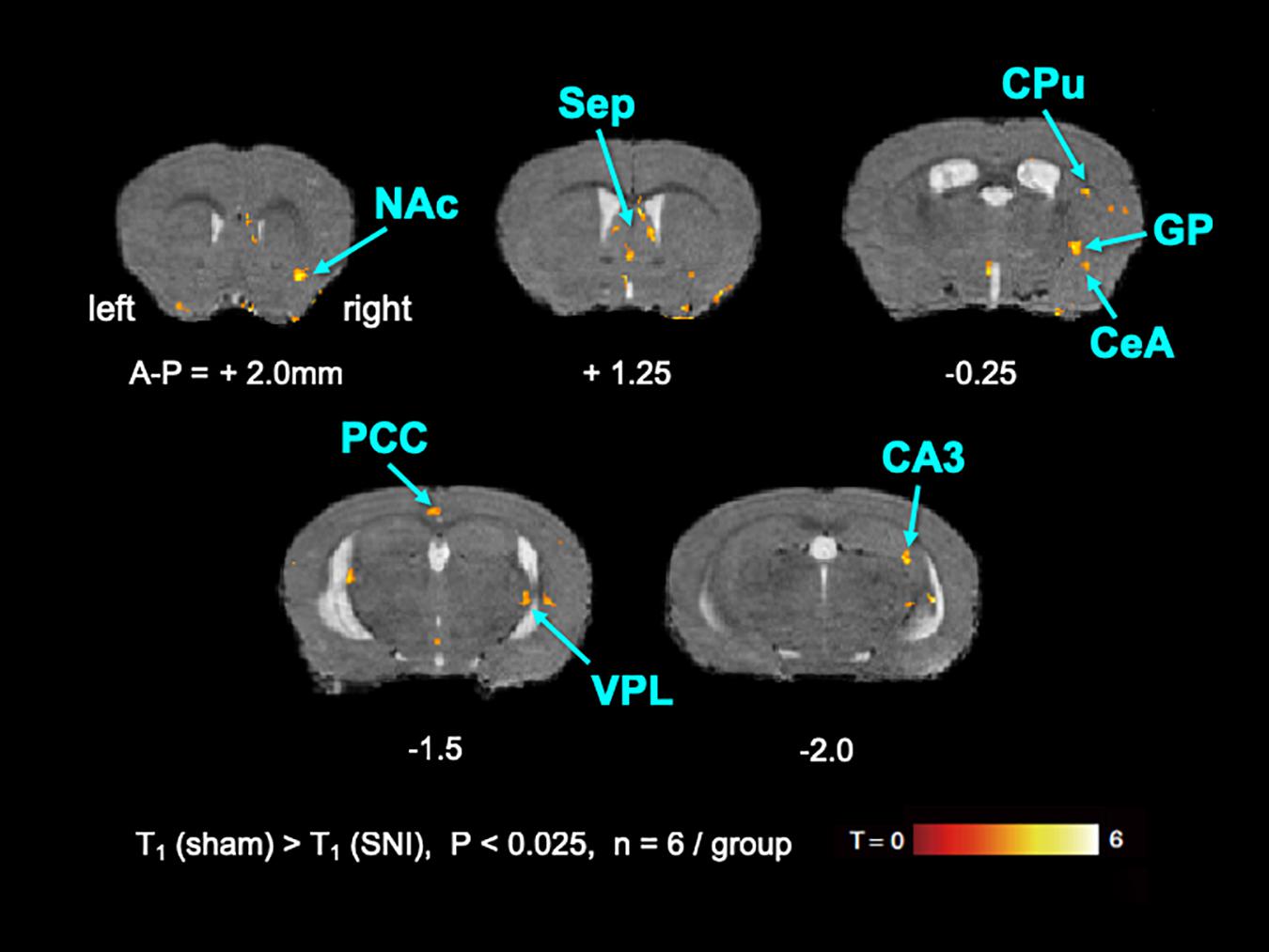
Figure 2. The active regions within the limbic structure in SNI mice compared with sham mice analyzed by AIM-MRI. Regions with significant shortening of T1 in SNI mice are indicated by the pseudo-colored regions over the T2-enhanced brain image template in coronal planes (n = 6 for sham, n = 6 for SNI). The active regions within the limbic structure are defined in text: nucleus accumbens (NAc), caudate-putamen (CPu), globus pallidus (GP), and ventral posterolateral nucleus of thalamus (VPL). The distance from bregma in mm is shown at the bottom. T, t-value; P, p-values.
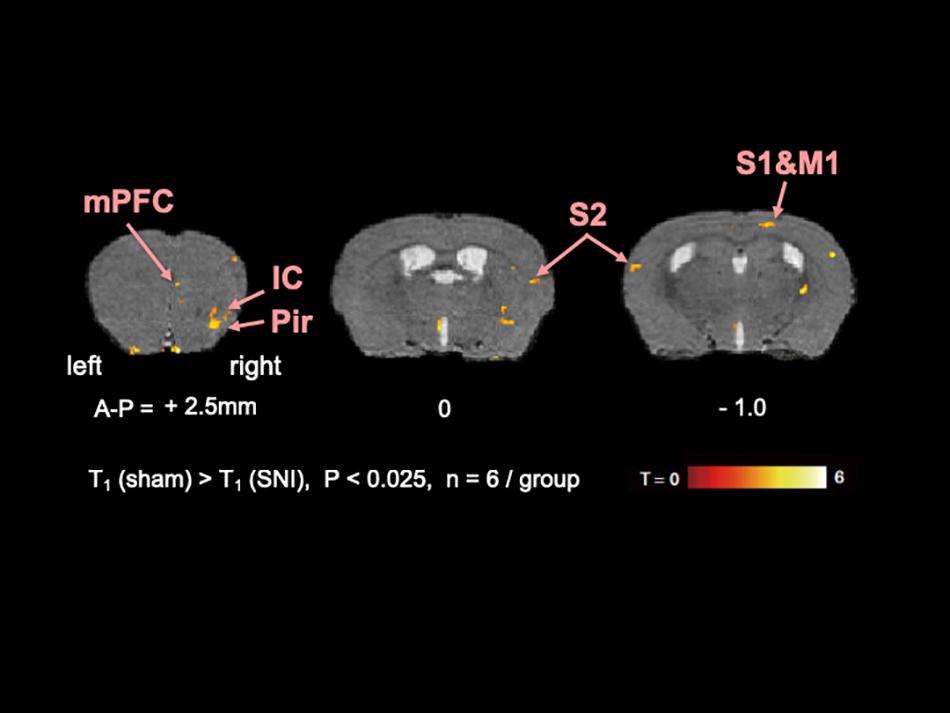
Figure 3. The active regions in the cortex in SNI mice compared with sham mice analyzed by AIM-MRI. Regions with significant shortening of T1 in SNI mice are indicated by the pseudo-colored regions over the T2-enhanced brain image template in coronal planes (n = 6 for sham, n = 6 for SNI). The active regions in the cortex are defined in text: piriform cortex (Pir), insula cortex (IC), and secondary somatosensory area (S2). The distance from bregma in mm is shown at the bottom. T, t-value; P, p-values.
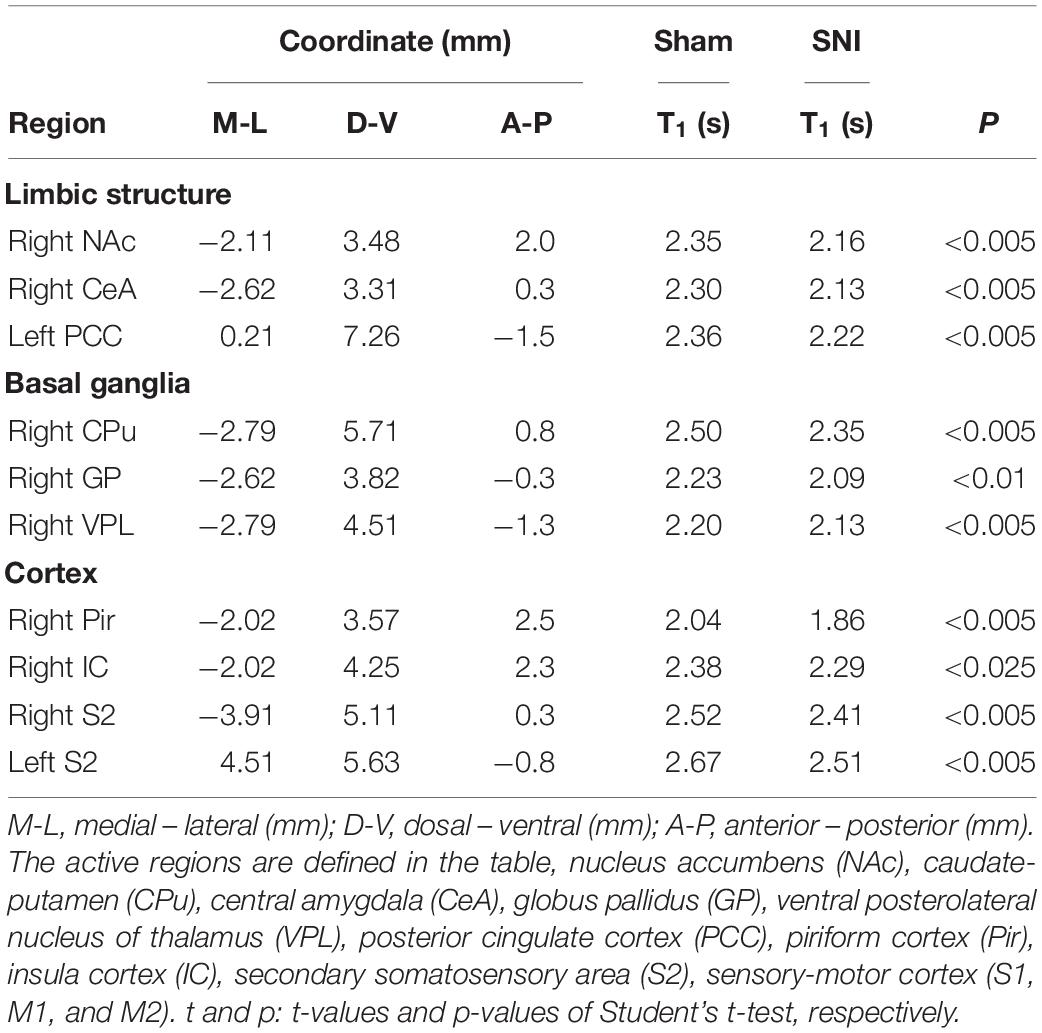
Table 1. Coordinates, statistical values, and T1 value of the active region in SNI compared with sham mice (n = 6) analyzed by AIM-MRI.
Discussion
The present results indicate that the neuropathic pain mouse model experienced an increase of resting-state brain activity and that qAIM-MRI can successfully distinguish neural activity in both the neuropathic pain mouse model and normal control mice.
The objective evaluation of pain is particularly important in basic and clinical research. The most widely used methods for pain assessment are behavioral paradigms such as the tail flick or the hot plate test, which can measure the function of the spinal cord and brain stem but not the cerebral cortex (Vierck et al., 2008). Instead of behavioral assessments, brain imaging is a putative complementary evaluation method for animal studies on pain. Several brain imaging techniques have been used to examine various types of pain models while the animal is under anesthesia (Hess et al., 2007; Seminowicz et al., 2009, 2012; Upadhyay et al., 2013). Because pain perception and related behaviors require consciousness and awareness, assessment of brain activity in awake animals is critical for accurate identification of steady-state brain activity (Chang et al., 2017). The qAIM-MRI method enables quantitative neural activity mapping of an awake animal (Kikuta et al., 2015). In the present study, we injected MnCl2 intraperitoneally without anesthesia. The Mn2+ is taken up into activated neurons while the animal is awake and thus becomes a marker for activity, making it possible to determine previous neuronal activity in awake and free moving animals (Tambalo et al., 2009). As such, the animals could still be anesthetized during MRI for visualization of brain activity. The qAIM-MRI method used in this study enables quantitative neuronal activity mapping over the entire brain and can help characterize changes to brain activity in neuropathic pain.
The present results indicate that the neuropathic pain mouse model have increased activity in several brain regions, including the cortex, limbic system, and basal ganglia (BG). The same brain regions activated in the SNI mice have also been reported in fMRI and PET studies on humans (Peyron et al., 2000; Apkarian et al., 2005), and have been proposed to be the “pain matrix” from brain imaging analysis. The pain matrix includes the primary somatosensory cortex (S1), secondary somatosensory cortex (S2), anterior cingulate cortex (ACC), IC, mPFC, thalamus, amygdala, BG, periaqueductal gray matter (PAG), and cerebellum (Peyron et al., 2000; Apkarian et al., 2005). These brain regions are also activated by nociceptive stimuli in chronic pain patients (Treede et al., 1999; Bosma et al., 2017). There are several reasons behind excluding measurement from the PAG and cerebellum in this study. If data from more slices, including the cerebellum, are acquired, the MRI measurement time increases, which may affect the animal condition. Moreover, since our major focus in this study was to explore the function of the frontal part of cerebral cortex in pain processing, the hind brain regions such as PAG and cerebellum were not evaluated. Further studies are required to reveal the activation of the PAG and cerebellum under chronic pain.
There are several reports wherein fMRI and PET were used to perform whole brain imaging to study pain perception in rodents (Thompson and Bushnell, 2012). Although most studies investigated brain activity after nociceptive stimulation in anesthetized animals, the activated brain regions correlated with the results from human brain imaging studies (Thompson and Bushnell, 2012). In the neuropathic pain model, brain activity in the somatosensory area, cingulate cortex, and thalamus regions are increased after nociceptive stimulation both in anesthetized and awake animals (Baliki et al., 2012; Thompson et al., 2014; Hubbard et al., 2015; Komaki et al., 2016). The resting-state brain activity in neuropathic pain models have also been studied using the [18F]fluorodeoxyglucose (FDG micro-PET) method. Brain regions with increased metabolism were primarily located in prefrontal-limbic-brainstem networks, which engage in cognitive and emotional modulation of pain (Kim et al., 2014). In the current study, we also observed increased activation of the prefrontal-limbic-basal ganglia network during resting-state brain activity in SNI mice using qAIM-MRI. Since the qAIM-MRI method directly determines the neuronal activity in freely moving animals, our present results provide comprehensive evidence that the regions in the nervous system related to emotion might be activated under neuropathic pain.
Recently, AIM-MRI studies were performed in SNI rat and the monosodium iodoacetate (MIA) model rat (Devonshire et al., 2017; Chao et al., 2018). Mn2+ accumulation in the ventral tegmental area (VTA), right CeA, and left cingulate was negatively correlated with pain response in the MIA model rat (Devonshire et al., 2017). The ipsilateral anterior insular cortex was activated in freely moving SNI rats 1 day after surgery using manganese-enhanced MRI (MEMRI) (Chao et al., 2018). Somatosensory cortex S1, cingulate cortex, and insular cortex were also activated 8 days after SNI surgery. In this study, we observed the activation of several limbic structures in SNI mice 14 days after surgery. Since the duration of chronic pain might differentially affect neural plasticity in each brain region, the functional changes across brain regions might be widespread upon prolongation of chronic pain.
The advantage of qAIM-MRI lies in its ability to measure the absolute T1 value, as opposed to several studies using MEMRI that measures the signal intensities obtained from T1-weighted images (Chao et al., 2018). T1-weighted images are quantified by signal intensities that provide relative values, and therefore can be unreliable for comparisons between animals. The absolute T1 value, however, can be used to quantify the Mn2+ concentration. Therefore, our present results reflect absolute neural activation under chronic pain.
It must be noted that Mn2+ itself could alter neuronal activity. In the present study, MnCl2 ⋅ 4H2O was intraperitoneally administered at a dose of 0.2 mmol/kg (39.6 mg/kg), whereas Chao et al. (2018) administered a dose of 75 mg/kg intravenously. Kikuta et al. (2015) reported that the Mn2+ concentrations in the ventricular regions of mice after intraperitoneal administration at a dose of 0.2 mmol/kg are less than 80 μM. The serum Mn2+ concentration after intravenous administration at a dose of 75 mg/kg would be 5.86 mM, because the blood volume in rat weighing 400 g would be 24.77 ml (Lee and Blaufox, 1985). Since the concentrations of Mn2+ over 200 μM could alter neuronal activity (Kostial et al., 1974; Hackett, 1976; Bagust and Kerkut, 1980), intravenous administration of MnCl2 at a dose of 75 mg/kg seems very high to evaluate the brain activity by MEMRI.
There are a couple of limitations and caveats of the qAIM-MRI techniques. The voltage-gated calcium channels (VDCCs) are expressed in neurons and astrocytes (MacVicar and Tse, 1988; Burgos et al., 2007). The ionotropic glutamate receptors, including N-methyl-D-aspartate (NMDA) receptors, that Mn2+ can penetrate through, are also expressed in astrocytes. Since qAIM-MRI utilizes Mn2+ as a contrast agent, excluding the influence of Mn2+ uptake in astrocytes is challenging. Astrocytes, however, can respond to presynaptic neurotransmitter release, and Mn2+ uptake could occur in response to neuronal activity (Kikuta et al., 2015). Therefore, Mn2+ accumulation in astrocytes may correlate with the activity of adjacent neurons.
Conclusion
In conclusion, changes to neural activity in areas that process pain cognition and emotion contribute to the chronification of neuropathic pain.
Ethics Statement
All animal studies were approved by the Animal Care Committee of the Graduate School of Pharmaceutical Sciences, Nagoya City University and the Tohoku University Committee for Animal Experiments. Experiments were conducted in accordance to the guidelines of the National Institute of Health and the Japanese Pharmacological Society.
Author Contributions
CI, HT, SK, OO, MOs, and MOh performed the experiments and data analysis. MOs and MOh calculated the statistical significance and wrote the manuscript. KS and KK supervised the study.
Funding
This study was supported by the KAKENHI Grant Numbers 16H05460 (MOh), 16K08486 (MOs), and 17H05543 (MOs) from the Ministry of Education, Science, Sports and Culture of Japan, and Brain/MINDS [Mapping by Integrated Neurotechnologies for Disease Studies (JP19dm0207001)], AMED (MOs).
Conflict of Interest
The authors declare that the research was conducted in the absence of any commercial or financial relationships that could be construed as a potential conflict of interest.
Acknowledgments
We thank the Institute for Animal Experimentation and Biomedical Research Core, Graduate School of Medicine Tohoku University for the permitting the use of its facilities and their technical assistance.
Footnotes
References
Aoki, I., Naruse, S., and Tanaka, C. (2004). Manganese-enhanced magnetic resonance imaging (MEMRI) of brain activity and applications to early detection of brain ischemia. NMR Biomed. 17, 569–580. doi: 10.1002/nbm.941
Apkarian, A. V., Bushnell, M. C., Treede, R.-D., and Zubieta, J.-K. (2005). Human brain mechanisms of pain perception and regulation in health and disease. Eur. J. Pain 9, 463–484. doi: 10.1016/j.ejpain.2004.11.001
Backonja, M. M., and Stacey, B. (2004). Neuropathic pain symptoms relative to overall pain rating. J. Pain 5, 491–497. doi: 10.1016/j.jpain.2004.09.001
Bagust, J., and Kerkut, G. A. (1980). The use of the transition elements manganese, cobalt and nickel as synaptic blocking agents on isolated, hemisected, mouse spinal cord. Brain Res. 182, 474–477. doi: 10.1016/0006-8993(80)91207-x
Baliki, M. N., Chialvo, D. R., Geha, P. Y., Levy, R. M., Harden, R. N., Parrish, T. B., et al. (2006). Chronic pain and the emotional brain: specific brain activity associated with spontaneous fluctuations of intensity of chronic back pain. J. Neurosci. 26, 12165–12173. doi: 10.1523/JNEUROSCI.3576-06.2006
Baliki, M. N., Petre, B., Torbey, S., Herrmann, K. M., Huang, L., Schnitzer, T. J., et al. (2012). Corticostriatal functional connectivity predicts transition to chronic back pain. Nat. Neurosci. 15, 1117–1119. doi: 10.1038/nn.3153
Baron, R., Tölle, T. R., Gockel, U., Brosz, M., and Freynhagen, R. (2009). A cross-sectional cohort survey in 2100 patients with painful diabetic neuropathy and postherpetic neuralgia: differences in demographic data and sensory symptoms. Pain 146, 34–40. doi: 10.1016/j.pain.2009.06.001
Borsook, D., Becerra, L., and Hargreaves, R. (2011). Biomarkers for chronic pain and analgesia. part 1: the need, reality, challenges, and solutions. Discov. Med. 11, 197–207.
Bosma, R. L., Hemington, K. S., and Davis, K. D. (2017). Using magnetic resonance imaging to visualize the brain in chronic pain. Pain 158, 1192–1193. doi: 10.1097/j.pain.0000000000000941
Burgos, M., Pastor, M. D., González, J. C., Martinez-Galan, J. R., Vaquero, C. F., Fradejas, N., et al. (2007). PKCepsilon upregulates voltage-dependent calcium channels in cultured astrocytes. Glia 55, 1437–1448. doi: 10.1002/glia.20555
Chang, P. C., Centeno, M. V., Procissi, D., Baria, A., and Apkarian, A. V. (2017). Brain activity for tactile allodynia: a longitudinal awake rat functional magnetic resonance imaging study tracking emergence of neuropathic pain. Pain 158, 488–497. doi: 10.1097/j.pain.0000000000000788
Chao, T. H. H., Chen, J. H., and Yen, C. T. (2018). Plasticity changes in forebrain activity and functional connectivity during neuropathic pain development in rats with sciatic spared nerve injury 11 medical and health sciences 1109 neurosciences. Mol. Brain 11, 1–16. doi: 10.1186/s13041-018-0398-z
Chaplan, S. R., Bach, F. W., Pogrel, J. W., Chung, J. M., and Yaksh, T. L. (1994). Quantitative assessment of tactile allodynia in the rat paw. J. Neurosci. Methods 53, 55–63. doi: 10.1016/0165-0270(94)90144-9
D’Amour, F. E., and Smith, D. L. (1941). A method for determining loss of pain sensation. J. Pharmacol. Exp. Ther. 72, 74–79.
Decosterd, I., and Woolf, C. J. (2000). Spared nerve injury: an animal model of persistent peripheral neuropathic pain. Pain 87, 149–158. doi: 10.1016/s0304-3959(00)00276-1
Devonshire, I. M., Burston, J. J., Xu, L., Lillywhite, A., Prior, M. J., Watson, D. J. G., et al. (2017). Manganese-enhanced magnetic resonance imaging depicts brain activity in models of acute and chronic pain: a new window to study experimental spontaneous pain? Neuroimage 157, 500–510. doi: 10.1016/j.neuroimage.2017.06.034
Dixon, W. J. (1965). The up-and-down method for small samples. J. Am. Stat. Assoc. 60, 967–978. doi: 10.1080/01621459.1965.10480843
Duyn, J., and Koretsky, A. P. (2008). Magnetic resonance imaging of neural circuits. Nat. Clin. Pract. Cardiovasc. Med. 5(Suppl. 2), S71–S78. doi: 10.1038/ncpcardio1248
Gottrup, H., Nielsen, J., Arendt-Nielsen, L., and Jensen, T. S. (1998). The relationship between sensory thresholds and mechanical hyperalgesia in nerve injury. Pain 75, 321–329. doi: 10.1016/s0304-3959(98)00011-6
Hackett, J. T. (1976). Selective antagonism of frog cerebellar synaptic transmission by manganese and cobalt ions. Brain Res. 114, 47–52. doi: 10.1016/0006-8993(76)91006-4
Hess, A., Sergejeva, M., Budinsky, L., Zeilhofer, H. U., and Brune, K. (2007). Imaging of hyperalgesia in rats by functional MRI. Eur. J. Pain 11, 109–119. doi: 10.1016/j.ejpain.2006.01.005
Howard, M. A., Sanders, D., Krause, K., O’Muircheartaigh, J., Fotopoulou, A., Zelaya, F., et al. (2012). Alterations in resting-state regional cerebral blood flow demonstrate ongoing pain in osteoarthritis: an arterial spin-labeled magnetic resonance imaging study. Arthritis Rheum. 64, 3936–3946. doi: 10.1002/art.37685
Hubbard, C. S., Khan, S. A., Xu, S., Cha, M., Masri, R., and Seminowicz, D. A. (2015). Behavioral, metabolic and functional brain changes in a rat model of chronic neuropathic pain: a longitudinal MRI study. Neuroimage 107, 333–344. doi: 10.1016/J.NEUROIMAGE.2014.12.024
Jaggi, A. S., and Singh, N. (2011). Role of different brain areas in peripheral nerve injury-induced neuropathic pain. Brain Res. 1381, 187–201. doi: 10.1016/j.brainres.2011.01.002
Jourdan, D., Ardid, D., and Eschalier, A. (2002). Analysis of ultrasonic vocalisation does not allow chronic pain to be evaluated in rats. Pain 95, 165–173. doi: 10.1016/S0304-3959(01)00394-393
Kikuta, S., Nakamura, Y., Yamamura, Y., Tamura, A., Homma, N., Yanagawa, Y., et al. (2015). Quantitative activation-induced manganese-enhanced MRI reveals severity of Parkinson’s disease in mice. Sci. Rep. 5:12800. doi: 10.1038/srep12800
Kim, C.-E., Kim, Y. K., Chung, G., Im, H. J., Lee, D. S., Kim, J., et al. (2014). Identifying neuropathic pain using 18F-FDG micro-PET: a multivariate pattern analysis. Neuroimage 86, 311–316. doi: 10.1016/j.neuroimage.2013.10.001
Komaki, Y., Hikishima, K., Shibata, S., Konomi, T., Seki, F., Yamada, M., et al. (2016). Functional brain mapping using specific sensory-circuit stimulation and a theoretical graph network analysis in mice with neuropathic allodynia. Sci. Rep. 6:37802. doi: 10.1038/srep37802
Koretsky, A. P. (2012). Is there a path beyond bold? Molecular imaging of brain function. Neuroimage 62, 1208–1215. doi: 10.1016/j.neuroimage.2012.02.076
Kostial, K., Landeka, M., and Slat, B. (1974). Manganese ions and synaptic transmission in the superior cervical ganglion of the cat. Br. J. Pharmacol. 51, 231–235. doi: 10.1111/j.1476-5381.1974.tb09652.x
Langford, D. J., Bailey, A. L., Chanda, M. L., Clarke, S. E., Drummond, T. E., Echols, S., et al. (2010). Coding of facial expressions of pain in the laboratory mouse. Nat. Methods 7, 447–449. doi: 10.1038/nmeth.1455
Le Bars, D., Gozariu, M., and Cadden, S. W. (2001). Animal models of nociception. Pharmacol. Rev. 53, 597–652.
Lee, H. B., and Blaufox, M. D. (1985). Blood volume in the rat the estimation of blood volume is important in studies. J. Nucl. Med. 25, 72–76.
Lein, E. S., Hawrylycz, M. J., Ao, N., Ayres, M., Bensinger, A., Bernard, A., et al. (2007). Genome-wide atlas of gene expression in the adult mouse brain. Nature 445, 168–176. doi: 10.1038/nature05453
Lin, Y. J., and Koretsky, A. P. (1997). Manganese ion enhances T1-weighted MRI during brain activation: an approach to direct imaging of brain function. Magn. Reson. Med. 38, 378–388. doi: 10.1002/mrm.1910380305
MacVicar, B. A., and Tse, F. W. (1988). Norepinephrine and cyclic adenosine 3’:5’-cyclic monophosphate enhance a nifedipine-sensitive calcium current in cultured rat astrocytes. Glia 1, 359–365. doi: 10.1002/glia.440010602
Mogil, J. S., Graham, A. C., Ritchie, J., Hughes, S. F., Austin, J. S., Schorscher-Petcu, A., et al. (2010). Hypolocomotion, asymmetrically directed behaviors (licking, lifting, flinching, and shaking) and dynamic weight bearing (gait) changes are not measures of neuropathic pain in mice. Mol. Pain 6, 1–15. doi: 10.1186/1744-8069-6-34
Narita, K., Kawasaki, F., and Kita, H. (1990). Mn and Mg influxes through Ca channels of motor nerve terminals are prevented by verapamil in frogs. Brain Res. 510, 289–295. doi: 10.1016/0006-8993(90)91379-U
Nelson, M. T. (1986). Interactions of divalent cations with single calcium channels from rat brain synaptosomes. J. Gen. Physiol. 87, 201–222. doi: 10.1085/jgp.87.2.201
Peyron, R., Laurent, B., and García-Larrea, L. (2000). Functional imaging of brain responses to pain. a review and meta-analysis (2000). Neurophysiol. Clin. Neurophysiol. 30, 263–288. doi: 10.1016/S0987-7053(00)00227-226
Sah, P., Faber, E. S. L., Lopez De Armentia, M., and Power, J. (2003). The amygdaloid complex: anatomy and physiology. Physiol. Rev. 83, 803–834. doi: 10.1152/physrev.00002.2003
Seminowicz, D. A., Jiang, L., Ji, Y., Xu, S., Gullapalli, R. P., and Masri, R. (2012). Thalamocortical asynchrony in conditions of spinal cord injury pain in rats. J. Neurosci. 32, 15843–15848. doi: 10.1523/JNEUROSCI.2927-12.2012
Seminowicz, D. A., Laferriere, A. L., Millecamps, M., Yu, J. S. C., Coderre, T. J., and Bushnell, M. C. (2009). MRI structural brain changes associated with sensory and emotional function in a rat model of long-term neuropathic pain. Neuroimage 47, 1007–1014. doi: 10.1016/j.neuroimage.2009.05.068
Silva, A. C., and Bock, N. A. (2008). Manganese-enhanced MRI: an exceptional tool in translational neuroimaging. Schizophr. Bull. 34, 595–604. doi: 10.1093/schbul/sbn056
Tambalo, S., Daducci, A., Fiorini, S., Boschi, F., Mariani, M., Marinone, M., et al. (2009). Experimental protocol for activation-induced manganese-enhanced MRI (AIM-MRI) based on quantitative determination of Mn content in rat brain by fast T1mapping. Magn. Reson. Med. 62, 1080–1084. doi: 10.1002/mrm.22095
Thompson, S. J., and Bushnell, M. C. (2012). Rodent functional and anatomical imaging of pain. Neurosci. Lett. 520, 131–139. doi: 10.1016/J.NEULET.2012.03.015
Thompson, S. J., Millecamps, M., Aliaga, A., Seminowicz, D. A., Low, L. A., Bedell, B. J., et al. (2014). Metabolic brain activity suggestive of persistent pain in a rat model of neuropathic pain. Neuroimage 91, 344–352. doi: 10.1016/j.neuroimage.2014.01.020
Tracey, I. (2010). Getting the pain you expect: mechanisms of placebo, nocebo and reappraisal effects in humans. Nat. Med. 16, 1277–1283. doi: 10.1038/nm.2229
Treede, R. D., Kenshalo, D. R., Gracely, R. H., and Jones, A. K. P. (1999). The cortical representation of pain. Pain 79, 105–111. doi: 10.1016/S0304-3959(98)00184-185
Upadhyay, J., Baker, S. J., Rajagovindan, R., Hart, M., Chandran, P., Hooker, B. A., et al. (2013). Pharmacological modulation of brain activity in a preclinical model of osteoarthritis. Neuroimage 64, 341–355. doi: 10.1016/j.neuroimage.2012.08.084
Urban, R., Scherrer, G., Goulding, E. H., Tecott, L. H., and Basbaum, A. I. (2011). Behavioral indices of ongoing pain are largely unchanged in male mice with tissue or nerve injury-induced mechanical hypersensitivity. Pain 152, 990–1000. doi: 10.1016/j.pain.2010.12.003
Van der Linden, A., Van Camp, N., Ramos-Cabrer, P., and Hoehn, M. (2007). Current status of functional MRI on small animals: application to physiology, pathophysiology, and cognition. NMR Biomed. 20, 522–545. doi: 10.1002/nbm.1131
Vierck, C. J., Hansson, P. T., and Yezierski, R. P. (2008). Clinical and pre-clinical pain assessment: are we measuring the same thing? Pain 135, 7–10. doi: 10.1016/j.pain.2007.12.008
Wallace, V. C. J., Norbury, T. A., and Rice, A. S. C. (2005). Ultrasound vocalisation by rodents does not correlate with behavioural measures of persistent pain. Eur. J. Pain 9, 445–452. doi: 10.1016/j.ejpain.2004.10.006
Yamamoto, S., Suzuki, Y., Ono, H., Kume, K., and Ohsawa, M. (2016). N- and L-type calcium channels blocker cilnidipine ameliorates neuropathic pain. Eur. J. Pharmacol. 793, 66–75. doi: 10.1016/j.ejphar.2016.11.001
Keywords: neuropathic pain, MRI, manganese, somatosensory abnormality, emotion
Citation: Inami C, Tanihira H, Kikuta S, Ogasawara O, Sobue K, Kume K, Osanai M and Ohsawa M (2019) Visualization of Brain Activity in a Neuropathic Pain Model Using Quantitative Activity-Dependent Manganese Magnetic Resonance Imaging. Front. Neural Circuits 13:74. doi: 10.3389/fncir.2019.00074
Received: 12 November 2018; Accepted: 31 October 2019;
Published: 26 November 2019.
Edited by:
Edward S. Ruthazer, McGill University, CanadaReviewed by:
Xuan Niu, The First Affiliated Hospital of Medical College, ChinaDario Siniscalco, University of Campania Luigi Vanvitelli, Italy
Copyright © 2019 Inami, Tanihira, Kikuta, Ogasawara, Sobue, Kume, Osanai and Ohsawa. This is an open-access article distributed under the terms of the Creative Commons Attribution License (CC BY). The use, distribution or reproduction in other forums is permitted, provided the original author(s) and the copyright owner(s) are credited and that the original publication in this journal is cited, in accordance with accepted academic practice. No use, distribution or reproduction is permitted which does not comply with these terms.
*Correspondence: Masahiro Ohsawa, b2hzYXdhQHBoYXIubmFnb3lhLWN1LmFjLmpw
†These authors have contributed equally to this work