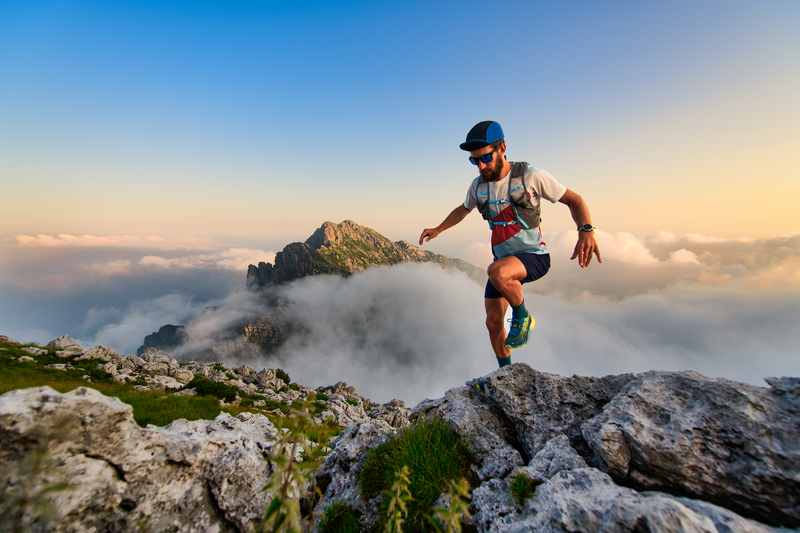
95% of researchers rate our articles as excellent or good
Learn more about the work of our research integrity team to safeguard the quality of each article we publish.
Find out more
REVIEW article
Front. Neural Circuits , 18 October 2019
Volume 13 - 2019 | https://doi.org/10.3389/fncir.2019.00066
This article is part of the Research Topic Coding for Spatial Orientation in Humans and Animals: Behavior, Circuits and Neurons View all 15 articles
Many studies have demonstrated that vestibular sensory input is important for spatial learning and memory. However, it has been unclear what contributions the different parts of the vestibular system – the semi-circular canals and otoliths – make to these processes. The advent of mutant otolith-deficient mice has made it possible to isolate the relative contributions of the otoliths, the utricle and saccule. A number of studies have now indicated that the loss of otolithic function impairs normal spatial memory and also impairs the normal function of head direction cells in the thalamus and place cells in the hippocampus. Epidemiological studies have also provided evidence that spatial memory impairment with aging, may be linked to saccular function. The otoliths may be important in spatial cognition because of their evolutionary age as a sensory detector of orientation and the fact that velocity storage is important to the way that the brain encodes its place in space.
The otolith organs in the vestibular inner ear, which comprise the utricle and saccule, represent the most ancient part of the vestibular system in evolutionary terms. Estimated to have evolved more than 500 million years ago, a primitive otolithic system (“statoliths”) even exists in invertebrates such as jellyfish (see Fritzsch, 1998; Spoor et al., 2002; Jeffery and Spoor, 2004; Schulz-Mirbach et al., 2019, for reviews). In mammals, the vestibular system develops early in embryogenesis and provides the fetus with the ability to detect gravitational vertical and self-motion before birth (Baker, 1998; Ronca, 2003; Jeffery and Spoor, 2004; Ronca et al., 2008; Lim and Brichta, 2016). Unlike the visual system, the vestibular system is almost fully functional at birth (see Lim and Brichta, 2016 for a review) and provides the brain and body with an immediate sense of direction in the spatial environment (Jamon, 2014). While the three semi-circular canals detect angular acceleration of the head in different planes, the otoliths, the utricle and saccule, sense linear acceleration, including linear acceleration by gravity. The maculae of the utricle and saccule are oriented at right angles to one another, and in the usual supine position of the head for humans, the saccule responds to changes in acceleration by gravity as the head is tilted relative to gravitational vertical (see Figure 1). In both the utricle and saccule, the hair cells, which are oriented in different directions, are activated by the inertial force exerted upon them by otoconia (calcium carbonate crystals) which sit above, on an epithelial layer (see Lim and Brichta, 2016 for a review; Figure 1). The specific role of the otoliths is to provide a gravitational frame of reference with which to interpret other sensory signals, including those generated by the semi-circular canals, and to contribute to the perception of linear motion within that framework (Andreescu et al., 2005; Cullen, 2012; Jamon, 2014; Curthoys et al., 2017). This, in turn, is critical for the vestibular contribution to cognitive processes such as spatial memory (see Besnard et al., 2016; Smith, 2017 for reviews), the body representation of the self (see Mast et al., 2014; Lopez et al., 2015 for reviews), and even social cognition (see Deroualle and Lopez, 2014 for a review). Any role that the otoliths have in these processes is especially important since: aging is associated with reduced otolith function (e.g., Agrawal et al., 2012; Zu Eulenburg et al., 2017); impaired vestibular function and specifically otolithic function, has been recently associated with an increased risk of cognitive impairment and dementia (Aranda-Moreno and Jáuregui-Renaud, 2016; Harun et al., 2016; Wei et al., 2017, 2019; Xie et al., 2017; Kamil et al., 2018, in press; see Agrawal et al., 2019, in press, for a review); and otolithic lesions can occur in humans independently of lesions of the semi-circular canals (e.g., Manzari et al., 2014). In addition to other forms of dysfunction that can occur in the vestibular system (e.g., benign paroxysmal positional vertigo, vestibular migraine, Meniere’s disease, vestibular vertigo, vestibular neuritis, vestibular schwannomas, etc.), there is evidence that high intensity noise exposure can damage the otoliths as well as the cochlea (e.g., Stewart et al., 2016; Xu et al., 2016).
Figure 1. Orientation of the sacculus and utriculus in the labyrinth. From Kingma and Van De Berg (2016) with permission.
While evidence has continued to accumulate for a significant role of the vestibular system as a whole, in the development of spatial learning and memory, the importance of the otoliths themselves has remained relatively obscure, due to the difficulty in accessing them surgically in order to lesion or electrically stimulate them selectively and independently of the semi-circular canals, in experimental studies (e.g., Hitier et al., 2016). Hence, while there is a wealth of evidence that global lesions of the peripheral vestibular system impair spatial memory in animals and humans in a variety of tasks (e.g., Zheng et al., 2006, 2009; Baek et al., 2010; see Besnard et al., 2016; Smith, 2017, for reviews), there are relatively few data relating specifically to the otoliths. Studies in extraterrestrial microgravity and in parabolic flight are possible avenues to investigate their significance; however, in each case there are confounding factors (e.g., Zheng et al., 2017). In microgravity the otoliths are no longer stimulated by gravity, but they respond to other forms of linear acceleration (Ronca, 2003). In parabolic flight, subjects experience microgravity but they are also subjected to hypergravity, which may cancel out the effects of the microgravity (Zheng et al., 2017). One development that has provided a new path to investigate the specific contributions of the otoliths, is the production of mutant mouse strains which are devoid of otoconia and therefore, of otolithic function. The aim of this review is to summarize and critically evaluate what is currently known of the importance of the otoliths for spatial memory and its neural substrates.
There are relatively few studies to date, but one model of otolith loss is the B6Ei.GL-Nox3het/J mouse (“tilted Het” mouse), which presents a mutation on chromosome 17 which inhibits the expression of the NADPH oxidase 3 gene (Paffenholz, 2004). Het–/– mice exhibit a complete and specific loss of utricular and saccular otoconia (Bergstrom et al., 1998). Another model is the –/–; B6.Cg-Otop1tlt/j tilted mouse (Jackson Laboratories, Bar Harbor, ME; Jones et al., 2008; Kim et al., 2010), which exhibits a similar deletion of the otoliths (de Caprona et al., 2004; Blankenship et al., 2017; Khan et al., 2019a, b).
The importance of the otoliths for spatial memory has been demonstrated in a number of studies published between 2012 and 2019, which have tested otolith-deficient mice in behavioral tests that assess cognitive performance. Most of these have involved testing spatial memory (e.g., the Y maze test, place recognition test, radial arm maze test, Barnes test, T maze alternation test, homing test, home retrieval test) but others have assessed other aspects of memory such as memory for objects (object recognition memory test).
In order to investigate the effects of selective otolith loss on cognitive and emotional function, Machado et al. (2012) investigated the performance of B6Ei.GL-Nox3het/J mice (“tilted Het” mice) in a series of behavioral tests: the Y maze, place recognition, elevated plus maze tests, as well as the rotarod test as a measure of motor control. The mice were 6–8 months of age. In the object recognition test, only the wild-type controls exhibited an exploration time that was significantly greater than chance; however, the discrimination index was not significantly different between the two groups. In the spontaneous alternation test in the Y maze, again, only the wild-type controls but not the Het mice showed performance significantly greater than chance (Figure 2). In the place recognition test, wild-type mice spent significantly more time in the new arm compared to Het mice (Figure 2). However, the Het mice could execute neither the rotarod test nor the elevated plus maze test. The authors noted that the Het mice were impaired in spatial memory performance (the spontaneous alternation and place recognition tests) but not non-spatial memory performance (object recognition test).
Figure 2. Spontaneous alternation was significantly different from chance level (50%) in the WT group (‡, p < 0.05) (A) while the number of entries remained similar in both het/het and WT groups (B). The place recognition test showed a significant group effect between WT and het/het mice without any specific arm effect or group × arm interaction, but the time spent in the new arm was significantly different in WT and het/het groups (C). Additionally, the discrimination index was different between groups (∗ and ‡, p < 0.05), and not different from zero in the het/het group (D). Reproduced from Machado et al. (2012) with permission.
In order to explore the effects of otolith loss on spatial cognition specifically, Yoder and Kirby (2014) investigated spatial memory in mice lacking otolithic function using the B6.Cg-Otop1tlt/J model, evaluating their performance in both a radial arm maze and a Barnes task in light, compared to heterozygous controls. In this case the status of the mice as –/– or ± was determined using a swim test, since –/– mice are unable to swim at 12 weeks of age. Tilted mice were able to learn to navigate to a visible goal in the radial arm maze; however, they exhibited a significantly lower percentage of correct choices and their improvement in performance over trials was significantly worse than the controls (Figure 3). The authors analyzed the specific types of memory errors that were being made. Tilted mice made more reference memory (RM) errors (the mouse did not enter an arm that was a correct choice) than controls, and also a slower rate of decrease in these errors over trials (Figure 3). Tilted mice also made more working memory (WM) errors than controls (i.e., either they re-entered an arm that was previously baited (WM-C) or an arm that had never been baited (WM-I)). The number of WM-C errors was higher for the tilted mice; however, this did not change across trials. For WM-I errors, tilted mice exhibited a greater number of them and this was maintained across trial blocks (Figure 3). The tilted mice showed a longer latency to complete the radial arm maze task, although the latency decreased across trials and was not significantly different compared to the control group.
Figure 3. Tilted mice were impaired at place learning on the radial arm maze. (A) Percentage of correct arm choices increased more rapidly across trial blocks for control mice than for tilted mice. (B–D) RM, WM-C, and WM-I errors decreased across trial blocks for control mice but not for tilted mice. (E) Latency to complete the task decreased across trial blocks for both groups. (F) Time per arm did not differ between control and tilted mice. Mean ± SEM. Reproduced from Yoder and Kirby (2014) with permission.
In the case of the Barnes maze, the control and tilted mice were similar in terms of RM, with both groups employing a serial strategy during the first 2 days of the experiment. By the final day, the control mice were using either a spatial or serial strategy, whereas the tilted mice were still using a serial strategy. There were no significant differences in latency, error rates or distance traveled. The fact that the tilted mice were impaired in the radial arm maze but not the Barnes maze is very interesting. Impairment in the radial arm maze has been observed previously with complete bilateral vestibular lesions (e.g., Ossenkopp and Hargreaves, 1993; Russell et al., 2003a; Besnard et al., 2012); therefore, these results suggest that similar impairment in this task can be caused by otolith dysfunction alone. Yoder and Kirby (2014) suggested that the impaired performance of the tilted mice was a result of them not being able to discriminate between the arms of the radial arm maze, rather than any general memory deficit. The authors point out that rats with complete bilateral vestibular lesions have been reported to be unimpaired in an open field homing task when distal cues were available (Wallace et al., 2002; see also Stackman and Herbert, 2002), indicating that vestibular information is not necessary for the development of spatial memory. They suggest that in tasks such as these as well as the Barnes maze, animals are only required to navigate to a single goal which is defined by its position in relation to landmarks, whereas in the radial arm maze they are required to learn to navigate between multiple goals.
In a further study of the effects of otolith loss on spatial cognitive function, Yoder et al. (2015) studied the homing ability in the B6.Cg-Otop1tlt/J model, in both darkness and light, compared to heterozygous controls. The mice were classified as –/– or ± based on the same swim test, used by Yoder and Kirby (2014) and were 3–8 months of age at the beginning of testing. They found that in darkness, in the absence of visual information, the tilted mice made more short duration stops while they were on the outward journey to find food, and, once they had found it, their route home was more circuitous than for heterozygous control mice (see Figure 4). When this was quantified, the tilted mice exhibited greater heading error and journey duration than the controls. When they were tested in light, while the tilted mice still exhibited a more circuitous journey than the controls, their heading error and journey duration were similar. Blankenship et al. (2017) have also reported that B6.Cg-Otop1tlt/J mice, at 12 weeks of age, exhibit significantly greater circuitous exploratory movements in darkness, with greater changes in heading direction, compared to controls. The tilted mice also exhibited greater heading changes in light, and the authors concluded that they were using visual cues to compensate for the lack of otolithic information about self-motion.
Figure 4. Kinematic (left) and topographic (right) characteristics are plotted for a representative control (top) and tilted mouse (bottom) on the homing task in darkness. The outward path (gray dashed line) is circuitous for both mice; the homeward segment (black line) is relatively direct for the control mouse, but is more circuitous for the tilted mouse. Reproduced from Yoder et al. (2015) with permission.
As part of a larger study of the effects of otolith loss on sensorimotor development, Le Gall et al. (2019) evaluated tilted Het mice in a home retrieval test on post-natal day 9, in order to test their spatial memory. Their phenotype was confirmed by PCR and they were compared to heterozygous controls. The home retrieval test is based on the natural tendency of blind pups to find their way back to the safety of their nest, using olfactory information. Le Gall et al. (2019) found that the Het mice exhibited a significantly longer latency to reach the nest area, they spent significantly less time in that area and were significantly less successful in reaching it, compared to the controls (Figure 5). Although this task involved an element of spatial memory in the form of spatial olfactory guidance, the Het mice were found to exhibit significant delays in sensorimotor reflex development and ultrasonic communication; maternal care was, however, normal.
Figure 5. The Home Retrieval test performed at PND9. No differences were detected between males and females; therefore, data were collapsed across sex (p-value > 0.05, n = 25 Het–/– versus 36 Het–/+). Two aberrant values were excluded in this test. (A) The Het–/– group needed significantly more time to reach the nest area compared to the Het–/+ control group (“Latency to reach the nest,” Mann–Whitney test, p ≤ 0.01, ∗∗, data ± sem). (B) The time spent in the nest area was significantly lower in the Het–/– group compared to the Het–/+ control group (Mann–Whitney test, p ≤ 0.01, ∗∗, data ± sem). (C) The otolith-deficient Het–/– pups showed a lower percentage of success reaching the nest area compared to Het–/+ mice (Chi2-tests for equality of proportions, p ≤ 0.01, ∗∗, data ± sem). (D) Conversely, the latency before the first movement reaction of pups (Mann–Whitney test, p > 0.05, ns, data ± sem) was similar in both groups (Het–/–, Het–/+). Reproduced from Le Gall et al. (2019) with permission.
In the most recent study of the effects of otolith loss on cognitive and emotional function, Manes et al. (2019) used the mergulhador (mlh) otoconia-deficient mouse model, to investigate the effects of otolith deficiency on open field activity, performance in the novel object recognition test, the T maze alternation test, the elevated plus maze test as well as neurochemical activity in various brain regions. The mlh mutation was confirmed by genetic analysis and the mice were 8 weeks of age at the beginning of testing. Compared to BALB/c mice, the mutant mice exhibited reduced locomotor behavior and rearing, with increased grooming in the open field maze. Motor coordination on the wooden beam test was reduced, and immobility time increased in the tail suspension test. In addition, the mutant mice displayed reduced auricular reflexes and responsiveness to touch. Although the T maze performance was significantly reduced for the mutant mice, there were no significant differences for the novel object recognition test or the elevated plus maze test.
In order to explore the neurochemical basis of the effects of otolith loss, the authors conducted a neurochemical investigation of the cerebellum, anterior part of the cortex, striatum and hippocampus, following decapitation, and analyzed the levels of dopamine (DA), noradrenaline (NA) and their metabolites [4,4-dihydrophenylacetic acid (DOPAC) and homovanillic acid (HVA)], in addition to serotonin and its metabolite, 5-hydroindole, 3-acetic acid (5HIAA). The results were complex (see Table 2 in Manes et al., 2019), but suggested an increase in DA turnover in the hippocampus with a concomitant decreased turnover of DA and reduced NA activity in the frontal cortex. The authors suggested that these neurochemical alterations could be responsible for the impaired response of the mlh mice in the T maze task.
Taken together, although there is still a small number of studies, most of this evidence suggests that the loss of otolith function results in an impairment of spatial memory.
The studies described in the previous section provide evidence that the absence of otolith function from birth in mice, appears to cause spatial memory deficits. Is there also a relationship between otolithic dysfunction and spatial memory in humans and can this develop later in life? Over the last several years, a number of epidemiological studies have been published on the relationship between vestibular function, aging and spatial memory (see Agrawal et al., 2019 for a review). Many of these studies have related spatial memory specifically to cervical vestibular-evoked myogenic potentials (cVEMPs), a vestibulo-spinal reflex that originates in the saccular part of the otoliths (see Curthoys et al., 2017 for a review; see Figure 1). Some studies have also assessed ocular VEMPs (oVEMPs), which are generated by the utricle (see Figure 1).
Harun et al. (2016) studied older subjects with mild cognitive impairment (MCI, n = 15) or Alzheimer’s Disease (AD, n = 32) and measured their cVEMPs and oVEMPs, comparing them with age-, sex-, and education-matched controls (n = 94) from the Baltimore Longitudinal Study of Aging (BLSA). Surprisingly, the authors found that bilaterally absent cVEMPs were associated with a more than 3-fold increased odds of AD (OR 3.42, 95% CI 1.33–8.91). However, there were no significant differences in vestibulo-ocular reflex (VOR) gain between the groups. The same group have reported that patients with MCI (n = 22) or AD (n = 28) and saccular dysfunction, measured using cVEMPs, made significantly more errors on the Money Road Map Test (MRMT) of spatial cognition compared to patients without vestibular impairment. AD patients who were spatially impaired exhibited a significantly greater prevalence of vestibular loss (Wei et al., 2018). Wei et al. (2017) examined driving ability in 21 patients with MCI and 39 with AD and related these data to saccular function. Using multiple regression, they found that patients with MCI or AD, who also had bilateral saccular impairment, had a significant increase in the odds of experiencing driving difficulty (OR 12.1; 95% CI 1.2, 117.7), compared to MCI or AD patients with normal saccular function. The patients’ performance on the MRMT suggested that the driving difficulty might be attributable to spatial memory deficits [see also Anson et al. (2019) and Pineault et al. (2019) for the most recent evidence].
Xie et al. (2017) studied the performance of young (<55 years) and older (≥55 years) subjects in the Triangle Completion Task (TCT), which tests spatial navigation ability, and related it to vestibular function. They found that loss of otolith function, as measured using the cVEMP, as well as loss of function of the semi-circular canals, was related to poor performance in the TCT in older subjects. Kamil et al. (2018) studied 103 subjects from the BLSA, measuring cVEMP function and relating it to MRI analysis of the hippocampus. They found that decreased saccular function, defined as a lower cVEMP amplitude, was significantly related to a lower average hippocampal volume. This result suggests the possibility that age-related impairment of spatial memory, as well as impairment of saccular function, may be related to a decrease in hippocampal volume. In their most recent study, Wei et al. (2019), studied 26 patients with MCI, 51 with AD and 295 matched control subjects and evaluated their cVEMPs, oVEMPs and VOR gain. They found that with increased cognitive impairment, there was a significant decrease in cVEMP and oVEMP responses. The VOR gain was lowest in MCI patients, followed by AD patients and then control subjects. Using logistic regression, they found that abnormal cVEMPs were associated with a 3-fold increased odds of MCI and a 5-fold increased odds of AD, and that abnormal oVEMPs were associated with an almost 4-fold increased odds of MCI and an over 4-fold increased odds of AD. However, VOR gain was not significantly related to the probability of having AD. See Addendum for further references published while this paper was in press.
Vestibular loss has often been associated with depersonalization/derealization (DD) symptoms in humans (see Besnard et al., 2016 for a review). These symptoms include feelings of déjà vu, of time passing slowly, being “spaced out,” difficulty concentrating, thoughts seeming blurred, one’s body feeling strange and not feeling in control of one’s self. In an experimental study, Aranda-Moreno and Jáuregui-Renaud (2016) used unilateral centrifugation in order to stimulate the utricle of 100 healthy subjects, who then completed a depersonalization/derealization (DD) questionnaire. The subjects reported symptoms such as “Body feels strange or different in some way” (56%) and “Time seems to pass very slowly” (55%). The authors concluded that utricular stimulation induces DD symptoms in healthy subjects.
Given the evidence that loss of the otoliths in mice or otolith dysfunction in humans is associated with spatial memory deficits, it was logical to consider what effect the loss of otolithic information might have on the neural pathways subserving spatial memory. An obvious place to look was head direction cells in the thalamus and elsewhere, and place cells in the hippocampus, since they are known to be critical for spatial memory. Although it is clear that vestibular information is transmitted to the thalamus and hippocampus via polysynaptic pathways (see Hitier et al., 2014 for a review), the pathways through which specifically otolithic information might be communicated, are unclear.
Few studies have electrically stimulated the otoliths selectively in rodents, in order to determine where otolithic information might be represented in structures such as the hippocampus. Cuthbert et al. (2000) reported inducing field potentials in CA1 by selective electrical stimulation of the utricle in guinea pig, and furthermore they found that they could still induce field potentials when the stimulus amplitude was too low to evoke the VOR. Hitier et al. (2019) have also reported that they could induce local field potentials in many areas of the hippocampus by selective electrical stimulation of the utricle and saccule in anesthetized rats.
Apart from selective otolithic stimulation, another way of studying the effect of the otoliths on brain regions which are spatially responsive, is to employ the same otolith-deficient mouse models that were described in the behavioral studies. The effects of otolith deficiency on the neural substrates of spatial navigation and memory were first studied by Taube (2011) and Yoder and Taube (2014) (for reviews). Stackman and Taube (1997) had demonstrated that the normal firing properties of head direction (HD) cells in the anterior thalamus of the rat, required vestibular input in general. Yoder and Taube (2009) used Otop1tlt mice to investigate the effects of otolith deficiency on HD neurons in the anterodorsal thalamus. The firing properties of cells in the tilted mice were not as robust as those in wild-type C57BL/6J mice (see Figure 6) and exhibited significant degradation across trials. In similar experiments, Calton and Taube (2005) demonstrated that when rats performed inverted locomotion, 47% of HD cells in the anterodorsal thalamus showed no directional firing selectivity, even though they had demonstrated this on the walls before they were inverted. During the process of inverted locomotion, the action of gravity on the saccule should have been the opposite of what it is normally.
Figure 6. Directional tuning curves of HD cells in C57BL/6J and tilted mice. (A) Robust directional tuning of three classic HD cells recorded simultaneously on different electrodes from the same C57BL/6J mouse. Peak firing rate (PFR), preferred direction (PFD), directional firing range (DFR), and background firing rate (BFR) are unique to each HD cell. (B) Tuning curve of representative HD cell recorded from a tilted mouse. Note the variable background firing rate (outside of the directional firing range) for the tilted HD cell, which contrasts with the relatively uniform background firing rates of C57BL/6J HD cells. Rayleigh’s r is noted for each cell. Reproduced from Yoder and Taube (2009) with permission.
Some studies of HD cells have been conducted in microgravity, where the normal stimulation of the otoliths by gravitational acceleration is absent. Taube et al. (2004) studied anterodorsal thalamic HD cells during the zero gravity phase of parabolic flight. They found that HD cells maintained their directional tuning during both the zero gravity and hypergravity phases of the flight when the rat was positioned on the floor, but that when the rat climbed the wall or moved upside down on the ceiling in zero gravity, they lost their directional firing and exhibited an increase in spontaneous firing rate. There was no significant difference for the peak firing rate and baseline firing rate for 1-G and 0-G on the floor, but significant decreases for the signal-to-noise ratio and information content of the HD firing. The cells exhibited a significantly reduced directionality score in 0-G, on average, by 25.8%, although they still exhibited directional tuning. While on the wall in 0-G, the HD cells that were recorded exhibited little directional preference. Compared to 0-G on the floor, all 7 HD cells that were recorded were found to lose their directional tuning when on the ceiling in 0-G: there were significant decreases in the signal-to-noise ratio, information content and the directionality score, with a significant increase in baseline firing rate. The authors speculated that during 0-G, the rats may have been experiencing visual reorientation illusions (VRIs).
The fact that both HD cell function and spatial memory are degraded by the loss of otolithic information, does not prove that there is a causal connection between the two. The data presented by Taube et al. (2004) would suggest that rats might have difficulty learning and remembering a spatial memory task while upside down. Valerio et al. (2010) investigated whether rats that were inverted on a circular platform suspended from the ceiling, could learn to navigate to an escape hole from a start point. They found that none of the animals trained from 4 start points were able to reach the criterion, even following 29 training sessions; by contrast, all of the rats trained from one start point reached the criterion after a mean of 5.9 training sessions. Further experiments suggested that rats used distal visual landmarks when navigating from 1 or 2 start points, but that their performance was degraded when they needed to navigate to the escape hole from a novel start point. The authors suggested that in the upside down situation, the rats do not learn to reach a certain place, but rather learn a series of separate trajectories to reach the target.
One of the optimal ways of studying the effects of the loss of the otolithic response to gravitational acceleration, without using lesions or a mutant mouse model, is to investigate the behavior of mice in space. Ronca et al. (2019) have recently reported a study of mice aboard the International Space Station (ISS). Following a 4 day transit from Earth to the ISS, they analyzed the behavior of 16- and 32-week old female mice. They found that the young flight mice engaged in more physical activity than controls on Earth, but that 7–10 days following the launch, the younger, but not older mice, exhibited a form of “race-tracking behavior” which was expressed as circling. The significance of this result is unclear. Interestingly, however, circling and hyperactivity are common symptoms of bilateral vestibular loss in rats and mice (see Stiles and Smith, 2015, for a review).
In addition to thalamic HD cells, it is also of interest to determine the effects of the lack of graviception by the otoliths, on place cells in the hippocampus. Place cells in the hippocampus have been demonstrated to rely on input from the vestibular system (e.g., Stackman et al., 2002; Russell et al., 2003b); however, once again, the extent to which the otoliths are specifically implicated has been unclear. Knierim et al. (2000) had recorded hippocampal place cells on the Neurolab Spacelab Mission and reported, in a brief letter, that normal place fields could be obtained by day 9 of the space flight. However, the more comprehensive NASA report did indicate that abnormal place fields were observed earlier in the flight, for example, day 4 (Knierim et al., 2003). The only study to address this question using otolith-deficient mice is by Harvey et al. (2018), who recorded hippocampal place cells in B6.Cg-Otop1tlt/j tilted mice (Jackson Laboratories, Bar Harbor, ME). They investigated place cells across five sessions (standard, cue rotation, standard, dark, standard) and found that those in tilted mice exhibited higher peak firing rates, with lower spatial coherence and smaller fields, compared to controls (see Figure 7); on the other hand, the average firing rates and spatial information content, were similar between the groups. The place fields from tilted mice were also less smooth than for controls and were concentrated near boundaries. Although hippocampal place cells are modulated by theta rhythm and theta has been reported to be degraded following complete bilateral vestibular loss (e.g., Russell et al., 2006), place cell activity was still modulated by theta in tilted mice. The place fields from tilted mice exhibited lower intra-trial stability than controls (Figure 8). It had been suggested in previous studies that otolithic information might be especially important for accurate spatial navigation performance in darkness in homing tasks and radial arm mazes (Yoder and Kirby, 2014; Yoder et al., 2015).
Figure 7. Overview of experimental design, place-cell examples, and basic firing characteristics. (A) Depiction of recording procedure across five recording sessions: Session 1, cue card was positioned in the standard north position; Session 2, cue card was rotated 90° clockwise or counterclockwise from the standard location; Session 3, cue card was returned to the standard location; Session 4, cue card was removed, and overhead lights were extinguished; and Session 5, white cue card was replaced at the standard location, and lights were turned on. (B) Representative place cells from control (cells 1–7) and tilted (cells 8–14) mice over five sessions. Numbers at the top left of each rate map represent peak firing rate (Hz). (C) Plot showing the peak firing rate (spikes/second) for each place cell recorded in tilted and control mice with values from all sessions included. (D) Plot showing the field width (cm) for each place cell recorded in tilted and control mice with values from all sessions included. (E) Plot showing coherence measures for each place cell recorded in tilted and control mice with values from all sessions included. (C–E) Shaded error bars represent SEM. Reproduced from Harvey et al. (2018) with permission.
Figure 8. Intra-session stability. (A) Rate maps depict the stability (r) between the first and second half of the first session using six example cells from Figure 1. Note that tilted cells appear to be less stable than control cells. (B) Stability between groups over all sessions. Note that tilted mice have much lower stability compared to control mice for all sessions. Red, tilted; black: control; shaded lines represent SEM. Reproduced from Harvey et al. (2018) with permission.
One of the most recent studies linking hippocampal and otolithic function in mice is by Kompotis et al. (2019). They observed that rocking mice at 1 Hz increased the amount of time spent in non-rapid eye movement sleep by accelerating the onset of sleep and shortening waking episodes, and that during active wakefulness, the theta EEG (6–10 Hz) moved toward slower frequencies. They used Otop1tlt/tlt tilted mice to test whether the otoliths were involved in this phenomenon and found that they were insensitive to rocking at 1 Hz and did not exhibit the same changes in theta EEG.
Although some would consider that galvanic vestibular stimulation (GVS) might be useful to probe the effects of otolith stimulation on the brain (e.g., Cohen et al., 2012), the evidence that it affects “mainly” the otoliths, is divided, and there is evidence that it also affects the semi-circular canals (see Curthoys and MacDougall, 2012, for a review). Therefore, GVS studies will not be discussed here.
These studies suggest that the otolithic part of the peripheral vestibular system, the utricle and saccule, make some contribution to spatial learning and memory in tests such as the radial arm maze, Y maze and homing tasks, but not the Barnes task or non-spatial memory tests such as the object recognition test or elevated plus maze task. The link between otolithic function and spatial memory is reinforced by human epidemiological studies in which it has been shown that saccular function, as measured using cVEMPs, is a predictor of the odds of having poor spatial memory, AD, and a smaller hippocampal volume. A link between hippocampal volume and vestibular function was first reported by Brandt et al. (2005), in a study in which patients with bilateral vestibular loss were found to have a bilateral atrophy of the hippocampus of approximately 17%. However, it was not clear from this study whether the otoliths were important in this relationship. The neurophysiological studies in otolith-deficient mice indicate that otolithic function is important for the normal function of both thalamic HD cells and hippocampal place cells, although HD cells can maintain directional tuning as long as they are on the floor in microgravity (Taube et al., 2004) and place cells can still develop place fields in microgravity (Knierim et al., 2000, 2003). Taube et al. (2004) suggested that the loss of directional firing of HD cells when they are on the walls or ceiling in 0-G may be a correlate of Type II/III spatial disorientation in humans, in which the subject is consciously aware of their disorientation and tries to correct it (Type II) or is so disoriented that they are incapacitated (Type III), respectively. Clearly, the loss of HD cell tuning is due to more than the loss of a gravitational signal (Taube et al., 2004).
In the case of the otolith-deficient mice, which is the easiest form of otolith manipulation to interpret, an interesting question is whether the sensation of linear acceleration by gravity is more important for spatial memory than the sensation of other kinds of linear acceleration, such as translation in the anterio-caudal or medio-lateral planes? In fact, the “tilt-translation illusion” demonstrates that the otoliths cannot distinguish between tilting of the head with respect to gravitational vertical and any other kind of linear acceleration that would induce the same stimulation of the otoliths (“Einstein’s Equivalence Principle,” Merfeld et al., 1999; Figure 9). Therefore, the brain must use information from the semi-circular canals and other sensory information to disambiguate tilt from translation (Merfeld et al., 1999; Straka et al., 2016). Since every form of sensory stimulation on Earth must be interpreted in terms of the framework of gravitational acceleration, it may be that otolithic stimulation is essential for normal spatial learning and memory. This is consistent with the evolutionary age of the otoliths. Although a number of studies have attempted to neutralize the influence of the vestibular system by fixing the heads of rats or mice and recording from HD, place or grid cells (e.g., Harvey et al., 2009; Ravassard et al., 2013; Aghajan et al., 2015; Acharya et al., 2016), it is debatable whether these studies have done any more than “minimize” vestibular stimulation, because the head will still be subject to linear acceleration by gravity.
Figure 9. During acceleration on Earth, gravity (G) minus linear acceleration (A) yields specific gravito-inertial force (GIF, F). Different combinations of gravity and linear acceleration can yield the same measured GIF. (A) GIF equals gravity minus linear acceleration (F = G – A or F + A = G). (B) GIF equals gravity alone. Since the measurement of GIF relative to the head is identical for these two situations, additional information is required for the nervous system to determine how much of the measured GIF is due to gravity and how much is due to linear acceleration.
Yoder and Taube (2009) speculated that part of the explanation for the broader tuning curves of HD cells in tilted mice in light, might be degraded visual perception of visual cues due to an impaired VOR (“oscillopsia”). This certainly seems possible in light, however, the greater reductions in HD cell directional preference in darkness indicates that oscillopsia is not necessary for their impaired function. The authors suggested that loss of otolithic function may impair velocity storage, which provides a prolonged representation of the angular velocity signal in the brain and may be necessary for accurate spatial navigation. Although the otoliths do not encode angular acceleration themselves, they are known to be important in velocity storage (e.g., Angelaki et al., 1992; Koizuka et al., 1996; Mittelstaedt and Mittelstaedt, 1996; Wearne et al., 1999). Recent studies using Otop1 mice show that although the otoliths do not appear to contribute to the baseline angular velocity of the VOR in mice, they are necessary for its adaptation to changes in gravitational stimulation (Khan et al., 2019a, b). As Shinder and Taube (2014) noted, the fact that the otoliths are known to be important for HD cell firing, suggests that linear acceleration information from the otoliths and rotational acceleration information from the semi-circular canals, must converge by the time they reach the thalamus. Indeed, Curthoys and Markham (1971) demonstrated the convergence of otolith and semi-canal signals at the level of the medial vestibular nucleus.
Taken together, the evidence to date suggests that the otolithic organs of the vestibular system are important for normal spatial learning and memory. Although the mechanisms by which they contribute to these processes are yet to be fully elucidated, the saccule and utricle appear to contribute to the development and maintenance of normal HD and place cell signals. This may be due to the evolutionary age of this part of the vestibular system and the fact that velocity storage has become an important part of the way that the brain encodes its place in space (Yoder and Taube, 2009).
PS conceived the study and wrote the manuscript.
The author declares that the research was conducted in the absence of any commercial or financial relationships that could be construed as a potential conflict of interest.
Acharya, L., Aghajan, Z. M., Vuong, C., Moore, J. J., and Mehta, M. R. (2016). Causal influence of visual cues on hippocampal directional selectivity. Cell 164, 197–207. doi: 10.1016/j.cell.2015.12.015
Aghajan, Z. M., Acharya, L., Moore, J. J., Cushman, J. D., Vuong, C., and Mehta, M. R. (2015). Impaired spatial selectivity and intact phase precession in two-dimensional virtual reality. Nat. Neurosci. 18, 121–128. doi: 10.1038/nn.3884
Agrawal, Y., Smith, P. F., and Rosenberg, P. B. (2019). Vestibular impairment, cognitive decline and Alzheimer’s disease: balancing the evidence. Aging Mental Health 29, 1–4. doi: 10.1080/13607863.2019.1566813
Agrawal, Y., Zuniga, M. G., Davalos-Bichara, M., Schubert, M. C., Walston, J. D., Hughes, J., et al. (2012). Decline in semicircular canal and otolith function with age. Otol. Neurotol. 33, 832–839. doi: 10.1097/MAO.0b013e3182545061
Andreescu, C. E., De Ruiter, M. M., De Zeeuw, C. I., and De Jeu, M. T. (2005). Otolith deprivation induces optokinetic compensation. J. Neurophysiol. 94, 3487–3496. doi: 10.1152/jn.00147.2005
Angelaki, D. E., Perachio, A. A., Mustari, M. J., and Strunk, C. L. (1992). Role of irregular otolith afferents in the steady-state nystagmus during off-vertical axis rotation. J. Neurophysiol. 68, 1895–1900. doi: 10.1152/jn.1992.68.5.1895
Anson, E. R., Ehrenburg, M. R., Wei, E. X., Bakar, D., Simonsick, E., and Agrawal, Y. (2019). Saccular function is associated with both angular and distance errors on the triangle completion test. Clin. Neurophysiol. 130, 2137–2143. doi: 10.1016/j.clinph.2019.08.027 [Epub ahead of print].
Aranda-Moreno, C., and Jáuregui-Renaud, K. (2016). Derealization during utricular stimulation. J. Vestib. Res. 26, 425–431. doi: 10.3233/VES-160597
Baek, J.-H., Zheng, Y., Darlington, C. L., and Smith, P. F. (2010). Evidence that spatial memory deficits in rats following bilateral vestibular loss are probably permanent. Neurobiol. Learn. Mem. 94, 402–413. doi: 10.1016/j.nlm.2010.08.007
Baker, R. (1998). From genes to behavior in the vestibular system. Otolaryngol. Head Neck Surg. 119, 263–275. doi: 10.1016/s0194-5998(98)70061-0
Bergstrom, R. A., You, Y., Erway, L. C., Lyon, M. F., and Schimenti, J. C. (1998). Deletion mapping of the head tilt (het) gene in mice: a vestibular mutation causing specific absence of otoliths. Genetics 150, 815–822.
Besnard, S., Lopez, C., Brandt, T., Denise, P., and Smith, P. F. (eds) (2016). “The vestibular system in cognitive and memory processes in mammals,” in Frontiers in Integrative Neuroscience, (Lausanne: Frontiers Media), 246.
Besnard, S., Machado, M. L., Vignaux, G., Boulouard, M., Coquerel, A., Bouet, V., et al. (2012). Influence of vestibular input on spatial and nonspatial memory and on hippocampal NMDA receptors. Hippocampus. 22, 814–826. doi: 10.1002/hipo.20942
Blankenship, P. A., Cherep, L. A., Donaldson, T. N., Brockman, S. N., Trainer, A. D., Yoder, R. M., et al. (2017). Otolith dysfunction alters exploratory movement in mice. Behav. Brain Res. 325, 1–11. doi: 10.1016/j.bbr.2017.02.031
Brandt, T., Schautzer, F., Hamilton, D. A., Bruning, R., Markowitsch, H., Kalla, R., et al. (2005). Vestibular loss causes hippocampal atrophy and impaired spatial memory in humans. Brain 128, 2732–2741. doi: 10.1093/brain/awh617
Calton, J. L., and Taube, J. S. (2005). Degradation of head direction cell activity during inverted locomotion. J. Neurosci. 25, 2420–2428. doi: 10.1523/jneurosci.3511-04.2005
Cohen, B., Yakushin, S. B., and Holstein, G. R. (2012). What does galvanic vestibular stimulation actually activate? Front. Neurol. 2:90. doi: 10.3389/fneur.2011.00090
Cullen, K. E. (2012). The vestibular system: multimodal integration and encoding of self-motion for motor control. Trends Neurosci. 35, 185–196. doi: 10.1016/j.tins.2011.12.001
Curthoys, I. S., and MacDougall, H. G. (2012). What galvanic vestibular stimulation actually activates. Front. Neurol. 3:117. doi: 10.3389/fneur.2012.00117
Curthoys, I. S., MacDougall, H. G., Vidal, P. P., and de Waele, C. (2017). Sustained and transient vestibular systems: a physiological basis for interpreting vestibular function. Front. Neurol. 8:117. doi: 10.3389/fneur.2017.00117
Curthoys, I. S., and Markham, C. H. (1971). Convergence of labyrinthine influences on units in the vestibular nuclei of the cat. I. Natural stimulation. Brain Res. 35, 469–490. doi: 10.1016/0006-8993(71)90489-6
Cuthbert, P. C., Gilchrist, D. P., Hicks, S. L., MacDougall, H. G., and Curthoys, I. S. (2000). Electrophysiological evidence for vestibular activation of the guinea pig hippocampus. Neuroreport 11, 1443–1447. doi: 10.1097/00001756-200005150-00018
de Caprona, M. D., Beisel, K. W., Nichols, D. H., and Fritzsch, B. (2004). Partial behavioral compensation is revealed in balance tasked mutant mice lacking otoconia. Brain Res. Bull. 64, 289–301. doi: 10.1016/j.brainresbull.2004.08.004
Deroualle, D., and Lopez, C. (2014). Toward a vestibular contribution to social cognition. Front. Integr. Neurosci. 8:16. doi: 10.3389/fnint.2014.00016
Fritzsch, B. (1998). Evolution of the vestibulo-ocular system. Otolaryngol. Head Neck Surg. 119, 182–192. doi: 10.1016/s0194-5998(98)70053-1
Harun, A., Oh, E. S., Bigelow, R. T., Studenski, S., and Agrawal, Y. (2016). Vestibular impairment in dementia. Otol. Neurotol. 37, 1137–1142. doi: 10.1097/mao.0000000000001157
Harvey, C. D., Collman, F., Dombeck, D. A., and Tank, D. W. (2009). Intracellular dynamics of hippocampal place cells during virtual navigation. Nature 461, 941–946. doi: 10.1038/nature08499
Harvey, R. E., Rutan, S. A., Willey, G. R., Siegel, J. J., Clark, B. J., and Yoder, R. M. (2018). Linear self-motion cues support the spatial distribution and stability of hippocampal place cells. Curr. Biol. 28:1803-1810.e5. doi: 10.1016/j.cub.2018.04.034
Hitier, M., Besnard, S., and Smith, P. F. (2014). Vestibular pathways involved in cognition. Front. Integr. Neurosci. 8:59. doi: 10.3389/fnint.2014.00059
Hitier, M., Sato, G., Zhang, Y., Zheng, Y., Besnard, S., Smith, P. F., et al. (2016). Anatomy and surgical approach to rat’s vestibular sensors and nerves. J. Neurosci. Meth. 270, 1–8. doi: 10.1016/j.jneumeth.2016.05.013
Hitier, M., Zhang, Y., Sato, G., Besnard, S., Zheng, Y., and Smith, P. F. (2019). Stratification of Hippocampal Electrophysiological Activation Evoked by Electrical Stimulation of Different Parts of the Rat Peripheral Vestibular System. L Taormina: Spring Hippocampal Research Conference.
Jamon, M. (2014). The development of vestibular system and related functions in mammals: impact of gravity. Front. Integr. Neurosci. 8:11. doi: 10.3389/fnint.2014.00011
Jeffery, N., and Spoor, F. (2004). Prenatal growth and development of the modern human labyrinth. J. Anat. 20, 471–492.
Jones, T. A., Jones, S. M., and Hoffman, L. F. (2008). Resting discharge patterns of macular primary afferents in otoconia-deficient mice. J. Assoc. Res. Otolaryngol. 9, 490–505. doi: 10.1007/s10162-008-0132-0
Kamil, R. J., Jacob, A., Ratnanather, J. T., Resnick, S. M., and Agrawal, Y. (2018). Vestibular function and hippocampal volume in the Baltimore Longitudinal Study of Aging (BLSA). Otol. Neurotol. 39, 765–771. doi: 10.1097/MAO.0000000000001838
Khan, S. I., Della Santina, C. C., and Migliaccio, A. A. (2019a). Angular vestibuloocular reflex responses in Otop1 mice. I. Otolith sensor input is essential for gravity context-specific adaptation. J. Neurophysiol. 121, 2291–2299. doi: 10.1152/jn.00811.2018
Khan, S. I., Della Santina, C. C., and Migliaccio, A. A. (2019b). Angular vestibuloocular reflex responses in Otop1 mice. II. Otolith sensor input improves compensation after unilateral labyrinthectomy. J. Neurophysiol. 121, 2300–2307. doi: 10.1152/jn.00812.2018
Kim, E., Hyrc, K. L., Speck, J., Lundberg, Y. W., Salles, F. T., Kachar, B., et al. (2010). Regulation of cellular calcium in vestibular supporting cells by otopetrin 1. J. Neurophysiol. 104, 3439–3450. doi: 10.1152/jn.00525.2010
Kingma, H., and Van De Berg, R. (2016). “Anatomy, physiology and physics of the peripheral vestibular system,” in Handbook of Clinical Neurology, Vol. 137, Chapter 1, eds J. M. Furman and T. Lempert (New York, NY: Elsevier), 1–16.x.
Knierim, J. J., McNaughton, B. L., and Poe, G. R. (2000). Three-dimensional spatial selectivity of hippocampal neurons during space flight. Nat. Neurosci. 3, 209–210. doi: 10.1038/72910
Knierim, J. J., Poe, G. R., and McNaughton, B. L. (2003). “Ensemble neural coding of place in zero-G,” in The Neurolab Spacelab Mission: Neuroscience Research in Space: Results from the STS-90, Neurolab Spacelab Mission. NASA SP-2003-535, eds J. C. Buckey, and J. L. Homick (Houston, TX: Johnson Space Center), 63–68.
Koizuka, I., Schor, R. H., and Furman, J. M. (1996). Influence of otolith organs, semicircular canals, and neck afferents on post-rotatory nystagmus. J. Vestib. Res. 6, 319–329.
Kompotis, K., Hubbard, J., Emmenegger, Y., Perrault, A., Mühlethaler, M., Schwartz, S., et al. (2019). Rocking promotes sleep in mice through rhythmic stimulation of the vestibular system. Curr. Biol. 29, 392–401. doi: 10.1016/j.cub.2018.12.007
Le Gall, A., Hilber, P., Chesneau, C., Bulla, J., Toulouse, J., Machado, M. L., et al. (2019). The critical role of vestibular graviception during cognitive-motor development. Behav. Brain Res. 372:112040. doi: 10.1016/j.bbr.2019.112040
Lim, R., and Brichta, A. M. (2016). Anatomical and physiological development of the human inner ear. Hear. Res. 338, 9–21. doi: 10.1016/j.heares.2016.02.004
Lopez, C., Falconer, C. J., Deroualle, D., and Mast, F. W. (2015). In the presence of others: self-location, balance control and vestibular processing. Neurophysiol. Clin. 45, 241–254. doi: 10.1016/j.neucli.2015.09.001
Machado, M. L., Kroichvili, N., Freret, T., Philoxène, B., Lelong-Boulouard, V., Denise, P., et al. (2012). Spatial and non-spatial performance in mutant mice devoid of otoliths. Neurosci. Letts. 522, 57–61. doi: 10.1016/j.neulet.2012.06.016
Manes, M., de, S. A., Garcia-Gomes, M., Sandini, T. M., Zaccarelli-Magalhães, J., Florio, J. C., et al. (2019). Behavioural and neurochemical characterization of the mlh mutant mice lacking otoconia. Behav. Brain Res. 359, 958–966. doi: 10.1016/j.bbr.2018.06.012
Manzari, L., MacDougall, H. G., Burgess, A. M., and Curthoys, I. S. (2014). Selective otolith dysfunction objectively verified. J. Vestib. Res. 24, 365–373. doi: 10.3233/VES-140537
Mast, F. W., Preuss, N., Hartmann, M., and Grabherr, L. (2014). Spatial cognition, body representation and affective processes: the role of vestibular information beyond ocular reflexes and control of posture. Front. Integr. Neurosci. 8:44. doi: 10.3389/fnint.2014.00044
Merfeld, D. M., Zupan, L., and Peterka, R. J. (1999). Humans use internal models to estimate gravity and linear acceleration. Nature 398, 615–618. doi: 10.1038/19303
Mittelstaedt, M. L., and Mittelstaedt, H. (1996). The influence of otoliths and somatic graviceptors on angular velocity estimation. J. Vestib. Res. 6, 355–366.
Ossenkopp, K. P., and Hargreaves, E. L. (1993). Spatial learning in an enclosed eight-arm radial maze in rats with sodium arsanilate-induced labyrinthectomies. Behav. Neural Biol. 59, 253–257. doi: 10.1016/0163-1047(93)91034-k
Paffenholz, R. (2004). Vestibular defects in head-tilt mice result from mutations in Nox3, encoding an NADPH oxidase. Genes Dev. 18, 486–491. doi: 10.1101/gad.1172504
Pineault, K., Pearson, D., Wei, E., Kamil, R., Klatt, B., and Agrawal, Y. (2019). Association between saccule and semicircular canal impairments and cognitive performance among vestibular patients. Ear Hear. doi: 10.1097/AUD.0000000000000795 [Epub ahead of print].
Ravassard, P., Kees, A., Willers, B., Ho, D., Aharoni, D. A., Cushman, J., et al. (2013). Multisensory control of hippocampal spatiotemporal selectivity. Science 340, 1342–1346. doi: 10.1126/science.1232655
Ronca, A. E. (2003). Mammalian development in space. Adv. Space Biol. Med. 9, 217–251. doi: 10.1016/s1569-2574(03)09009-9
Ronca, A. E., Fritzsch, B., Bruce, L. L., and Alberts, J. R. (2008). Orbital spaceflight during pregnancy shapes function of mammalian vestibular system. Behav. Neurosci. 122, 224–232. doi: 10.1037/0735-7044.122.1.224
Ronca, A. E., Moyer, E. L., Talyansky, Y., Lowe, M., Padmanabhan, S., Choi, S., et al. (2019). Behavior of mice aboard the international space station. Sci. Rep. 9:4717.
Russell, N., Horii, A., Smith, P. F., Darlington, C. L., and Bilkey, D. (2003a). Effects of bilateral vestibular deafferentation on radial arm maze performance. J. Vest. Res. 13, 9–16.
Russell, N., Horii, A., Smith, P. F., Darlington, C. L., and Bilkey, D. (2003b). The long-term effects of permanent vestibular lesions on hippocampal spatial firing. J. Neurosci. 23, 6490–6498. doi: 10.1523/jneurosci.23-16-06490.2003
Russell, N., Horii, A., Smith, P. F., Darlington, C. L., and Bilkey, D. (2006). Lesions of the vestibular system disrupt hippocampal theta rhythm in the rat. J. Neurophysiol. 96, 4–14. doi: 10.1152/jn.00953.2005
Schulz-Mirbach, T., Ladich, F., Plath, M., and Heß, M. (2019). Enigmatic ear stones: what we know about the functional role and evolution of fish otoliths. Biol. Rev. Camb. Philos. Soc. 94, 457–482. doi: 10.1111/brv.12463
Shinder, M. E., and Taube, J. S. (2014). Resolving the active versus passive conundrum for head direction cells. Neuroscience 270, 123–138. doi: 10.1016/j.neuroscience.2014.03.053
Smith, P. F. (2017). The vestibular system and cognition. Curr. Opin. Neurol. 30, 84–89. doi: 10.1097/wco.0000000000000403
Spoor, F., Bajpai, S., Hussain, S. T., Kumar, K., and Thewissen, J. G. (2002). Vestibular evidence for the evolution of aquatic behaviour in early cetaceans. Nature 417, 163–166. doi: 10.1038/417163a
Stackman, R. W., Clark, A. S., and Taube, J. S. (2002). Hippocampal spatial representations require vestibular input. Hippocampus 12, 291–303. doi: 10.1002/hipo.1112
Stackman, R. W., and Herbert, A. M. (2002). Rats with lesions of the vestibular system require a visual landmark for spatial navigation. Behav. Brain Res. 128, 27–40. doi: 10.1016/s0166-4328(01)00270-4
Stackman, R. W., and Taube, J. S. (1997). Firing properties of head direction cells in the rat anterior thalamic nucleus: dependence on vestibular input. J. Neurosci. 17, 4349–4358. doi: 10.1523/jneurosci.17-11-04349.1997
Stewart, C., Yu, Y., Huang, J., Maklad, A., Tang, X., Allison, J., et al. (2016). Effects of high intensity noise on the vestibular system in rats. Hear. Res. 335, 118–127. doi: 10.1016/j.heares.2016.03.002
Stiles, L., and Smith, P. F. (2015). The vestibular-basal ganglia connection: balancing motor control. Brain Res. 1597, 180–188. doi: 10.1016/j.brainres.2014.11.063
Straka, H., Zwergal, A., and Cullen, K. E. (2016). Vestibular animal models: contributions to understanding physiology and disease. J. Neurol. 263, S10–S23. doi: 10.1007/s00415-015-7909-y
Taube, J. S. (2011). Head direction cell firing properties and behavioural performance in 3-D space. J. Physiol. 589(Pt 4), 835–841. doi: 10.1113/jphysiol.2010.194266
Taube, J. S., Stackman, R. W., Calton, J. L., and Oman, C. M. (2004). Rat head direction cell responses in zero-gravity parabolic flight. J. Neurophysiol. 92, 2887–2997. doi: 10.1152/jn.00887.2003
Valerio, S., Clark, B. J., Chan, J. H., Frost, C. P., Harris, M. J., and Taube, J. S. (2010). Directional learning, but no spatial mapping by rats performing a navigational task in an inverted orientation. Neurobiol. Learn. Mem. 93, 495–505. doi: 10.1016/j.nlm.2010.01.007
Wallace, D. G., Hines, D. J., Pellis, S. M., and Whishaw, I. Q. (2002). Vestibular information is required for dead reckoning in the rat. J. Neurosci. 22, 10009–10017. doi: 10.1523/jneurosci.22-22-10009.2002
Wearne, S., Raphan, T., and Cohen, B. (1999). Effects of tilt of the gravito-inertial acceleration vector on the angular vestibuloocular reflex during centrifugation. J. Neurophysiol. 81, 2175–2190. doi: 10.1152/jn.1999.81.5.2175
Wei, E. X., Oh, E. S., Harun, A., Ehrenburg, M., and Agrawal, Y. (2017). Saccular impairment in Alzheimer’s disease is associated with driving difficulty. Dement. Geriatr. Cogn. Disord. 44, 294–302. doi: 10.1159/000485123
Wei, E. X., Oh, E. S., Harun, A., Ehrenburg, M., and Agrawal, Y. (2018). Vestibular loss predicts poorer spatial cognition in patients with Alzheimer’s disease. J. Alzheimers. Dis. 61, 995–1003. doi: 10.3233/JAD-170751
Wei, E. X., Oh, E. S., Harun, A., Ehrenburg, M., Xue, Q. L., Simonsick, E., et al. (2019). Increased prevalence of vestibular loss in mild cognitive impairment and Alzheimer’s disease. Curr. Alzheimer Res. doi: 10.2174/1567205016666190816114838 [Epub ahead of print].
Xie, Y., Bigelow, R. T., Frankenthaler, S. F., Studenski, S. A., Moffat, S. D., and Agrawal, Y. (2017). Vestibular loss in older adults is associated with impaired spatial navigation: data from the triangle completion task. Front. Neurol. 8:173. doi: 10.3389/fneur.2017.00173
Xu, X. D., Ding, C. R., Yu, J., Han, Z., Gu, J., Gao, N., et al. (2016). The hidden dysfunction of otolithic organs in patients with profound sensorineural hearing loss. Hear. Res. 331, 41–46. doi: 10.1016/j.heares.2015.10.006
Yoder, R. M., Goebel, E. A., Köppen, J. R., Blankenship, P. A., Blackwell, A. A., and Wallace, D. G. (2015). Otolithic information is required for homing in the mouse. Hippocampus 25, 890–899. doi: 10.1002/hipo.22410
Yoder, R. M., and Kirby, S. L. (2014). Otoconia-deficient mice show selective spatial deficits: impaired spatial performance in otoconia-deficient mice. Hippocampus 24, 1169–1177. doi: 10.1002/hipo.22300
Yoder, R. M., and Taube, J. S. (2009). Head direction cell activity in mice: robust directional signal depends on intact otolith organs. J. Neurosci. 29, 1061–1076. doi: 10.1523/JNEUROSCI.1679-08.2009
Yoder, R. M., and Taube, J. S. (2014). The vestibular contribution to the head direction signal and navigation. Front. Integr. Neurosci. 8:32. doi: 10.3389/fnint.2014.00032
Zheng, Y., Darlington, C. L., and Smith, P. F. (2006). Impairment and recovery on a food foraging task following unilateral vestibular deafferentation in rat. Hippocampus 16, 368–378. doi: 10.1002/hipo.20149
Zheng, Y., Gliddon, C. M., Aitken, P., Stiles, L., Machado, M. L., Philoxene, B., et al. (2017). Effects of acute altered gravity during parabolic flight and/or vestibular loss on cell proliferation in the rat dentate gyrus. Neurosci. Lett. 654, 120–124. doi: 10.1016/j.neulet.2017.06.033
Zheng, Y., Goddard, M., Darlington, C. L., and Smith, P. F. (2009). Long-term deficits on a foraging task after bilateral vestibular deafferentation in rats. Hippocampus 19, 480–486. doi: 10.1002/hipo.20533
Keywords: otoliths, vestibular, spatial learning and memory, hippocampus, head direction cells, place cells
Citation: Smith PF (2019) The Growing Evidence for the Importance of the Otoliths in Spatial Memory. Front. Neural Circuits 13:66. doi: 10.3389/fncir.2019.00066
Received: 09 August 2019; Accepted: 30 September 2019;
Published: 18 October 2019.
Edited by:
Mathieu Beraneck, Université Paris Descartes, FranceReviewed by:
Jerome Carriot, McGill University, CanadaCopyright © 2019 Smith. This is an open-access article distributed under the terms of the Creative Commons Attribution License (CC BY). The use, distribution or reproduction in other forums is permitted, provided the original author(s) and the copyright owner(s) are credited and that the original publication in this journal is cited, in accordance with accepted academic practice. No use, distribution or reproduction is permitted which does not comply with these terms.
*Correspondence: Paul F. Smith, cGF1bC5zbWl0aEBvdGFnby5hYy5ueg==; cGF1bC5zbWl0aEBzdG9uZWJvdy5vdGFnby5hYy5ueg==
Disclaimer: All claims expressed in this article are solely those of the authors and do not necessarily represent those of their affiliated organizations, or those of the publisher, the editors and the reviewers. Any product that may be evaluated in this article or claim that may be made by its manufacturer is not guaranteed or endorsed by the publisher.
Research integrity at Frontiers
Learn more about the work of our research integrity team to safeguard the quality of each article we publish.