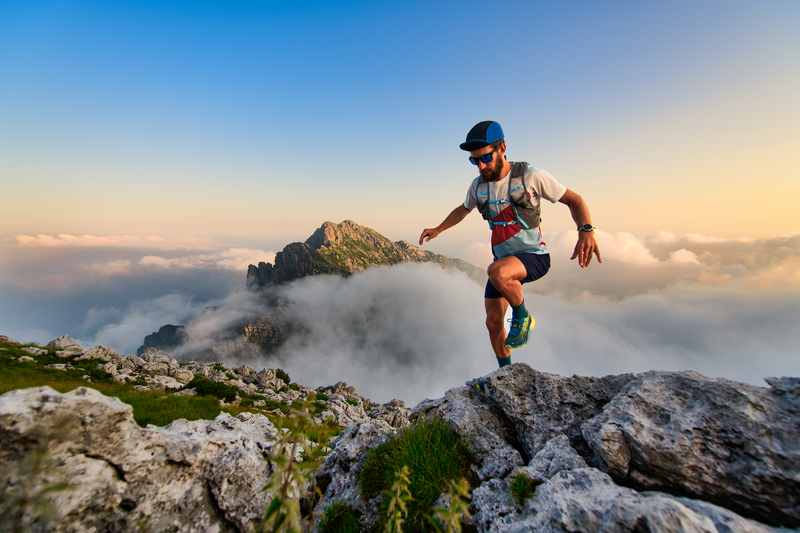
95% of researchers rate our articles as excellent or good
Learn more about the work of our research integrity team to safeguard the quality of each article we publish.
Find out more
REVIEW article
Front. Neural Circuits , 21 October 2016
Volume 10 - 2016 | https://doi.org/10.3389/fncir.2016.00083
This article is part of the Research Topic Patterning and functional development of brainstem and cerebellar circuits View all 8 articles
Glial cells, previously thought to have generally supporting roles in the central nervous system, are emerging as essential contributors to multiple aspects of neuronal circuit function and development. This review focuses on the contributions of glial cells to the development of auditory pathways in the brainstem. These pathways display specialized synapses and an unusually high degree of precision in circuitry that enables sound source localization. The development of these pathways thus requires highly coordinated molecular and cellular mechanisms. Several classes of glial cells, including astrocytes, oligodendrocytes and microglia, have now been explored in these circuits in both avian and mammalian brainstems. Distinct populations of astrocytes are found over the course of auditory brainstem maturation. Early appearing astrocytes are associated with spatial compartments in the avian auditory brainstem. Factors from late appearing astrocytes promote synaptogenesis and dendritic maturation, and astrocytes remain integral parts of specialized auditory synapses. Oligodendrocytes play a unique role in both birds and mammals in highly regulated myelination essential for proper timing to decipher interaural cues. Microglia arise early in brainstem development and may contribute to maturation of auditory pathways. Together these studies demonstrate the importance of non-neuronal cells in the assembly of specialized auditory brainstem circuits.
Our ability to localize sound sources relies in large part on specialized circuitry in the auditory brainstem. Unlike other sensory modalities, such as the visual and somatosensory systems, in which spatial information is encoded in the sensory epithelium, the cochlea is unique in that it instead contains an orderly representation of frequency selectivity. This frequency map is conveyed through spiral ganglion cells to the brainstem, and interaural time and intensity differences are computed through specialized circuitry in the superior olivary complex. The development of these specialized neural pathways requires coordination of multiple molecular and cellular mechanisms. Several classes of axon guidance molecules have been identified that contribute to the formation of synaptic connections with precisely selected targets. Emerging evidence has shown that several classes of glial cells, including astrocytes, oligodendrocytes and microglia, play important roles in nervous system development (Stevens, 2008; Allen, 2013; Clarke and Barres, 2013; Edmonson et al., 2016). Here we review recent studies that have begun to demonstrate the contributions of these non-neuronal cells to multiple aspects of auditory brainstem circuit assembly.
In vertebrates, sound stimuli are transduced in the ear, where auditory hair cells in the cochlea are spatially ordered according to their preferred stimulus frequency. Hair cells synapse onto peripheral processes of cochlear ganglion cells, whose central processes enter the brainstem through the eighth cranial nerve (nVIII) and contact the cochlear nuclei, preserving tonotopy. Birds and mammals both use interaural time differences (ITDs) and interaural level differences (ILDs) to varying degrees to infer the locations of sound sources in space.
The chick pathway, shown in Figure 1, demonstrates the specialized spatial arrangement of auditory brainstem connectivity that allows for computation of ITDs. The key circuit element is the projection from nucleus magnocellularis (NM; Figure 1A), which receives ipsilateral nVIII input, to nucleus laminaris (NL), which contains a sheet of bitufted neurons with dorsal and ventral dendrites (Smith and Rubel, 1979; Jhaveri and Morest, 1982). NM neurons project through a bifurcated axon to NL on both sides of the brainstem. Ipsilateral NM axon branches synapse onto the dorsal NL dendrites, whereas contralateral NM axon branches synapse onto ventral NL dendrites (Parks and Rubel, 1975; Hackett et al., 1982; Young and Rubel, 1983). The contralateral NM axon branches introduce delay lines so that conduction time is longer to reach more lateral NL neurons than the more medial neurons (Overholt et al., 1992). Highly coincident input from the two NMs is needed for NL neuronal firing. The arrangement of the NM-NL pathway results in a correlation between the sound source and the location of NL neurons receiving coincident input (Figure 1B). Sounds presented close to one ear will result in coincident input and activation of lateral NL neurons contralateral to that ear, while sounds presented directly ahead will activate medial NL neurons on both sides of the brain (Carr and Konishi, 1990; Hyson, 2005).
Figure 1. Auditory brainstem pathways used in sound localization in the chick. (A) Auditory nerve axons innervate ipsilateral nucleus magnocellularis (NM) neurons, which in turn innervate nucleus laminaris (NL) bilaterally. Ipsilateral and contralateral NM axon branches make contact with dorsal and ventral NL dendrites, respectively. Ventral NM axon branches display delay lines and dorsal axon branches course around NM before making their contacts. Inhibitory input is largely provided by the superior olivary nucleus (SON), shown on the left side. (B) Delay lines in NM axons and coincidence detection in NL neurons are key factors in determination of interaural time difference (ITD) determination. Coincidence detection in NL neurons varies along the mediolateral axis depending on the location of the sound source relative to the animal. NL neurons with coincident input and the corresponding locations are shown here in matching colors.
Several additional organizing features enhance the function of the NM-NL pathway in ITD computation (Ohmori, 2014). Coincidence detection in NL neurons is improved with inhibitory input, which arises mainly from axons of the superior olivary nucleus (SON) in the ventral region of the auditory brainstem (Lachica et al., 1994; Yang et al., 1999; Burger et al., 2011). The spatial arrangement of best ILDs is orthogonal to the frequency axis, in which high frequencies are represented in rostromedial NL and low frequencies are represented in caudolateral NL. While NL dendrites generally show symmetry for the dorsal and ventral sides, a steep gradient of dendritic arbor size emerges late in embryonic development (Smith and Rubel, 1979; Smith, 1981). Mature NL neurons have fewer and longer dendrites in the low frequency region and numerous short dendrites in the high frequency region. This specialization is thought to optimize coincidence detection for each frequency (Agmon-Snir et al., 1998).
Glial cells are found throughout the auditory brainstem during the formation and maturation of the NM-NL pathway and are well positioned to contribute to most of the spatially ordered features of the auditory circuitry described above. Astrocytes are seen in the brainstem when NM and NL are still part of a common cell group known as the auditory anlage, at about embryonic day (E) 8. Glial fibers express the intermediate filament vimentin, a marker for radial glia. At E10, when NM-NL connections form, vimentin expression is limited to the dorsal region of NL (Korn and Cramer, 2008), coinciding with the ipsilateral NM input. This intriguing pattern suggests a potential role for glial fibers in appropriate segregation of ipsilateral and contralateral NM axon branches to dorsal and ventral regions of NL.
Further suggestion of a role for glia in NL axon guidance is seen in the glial cell bodies around NL. NM and NL separate from each other in the auditory anlage at about E10, and NL forms a monolayer surrounded by neuropil composed of dendrites, axonal terminal processes and glial processes that is largely devoid of cell bodies. This neuropil region is surrounded by a dense margin of small glial cells (Rubel et al., 1976) that remain outside the neuropil until later embryonic ages (Figure 2A). These glial cells were shown to express the axon guidance molecule ephrin-B2 (Person et al., 2004). Moreover, the expression of ephrin-B2 displays a concentration gradient corresponding with the tonotopic axis of NL, alongside a similar ephrin-B2 expression gradient in the adjacent neurons of NL. The role of NL glial cells in axon guidance has not been critically tested. However, when Eph family proteins were experimentally inhibited during development, we found that mistargeting of NM axons was accompanied by disruption of NL morphogenesis, suggesting that the structural integrity of NL and its glial margin are linked to axon growth (Cramer et al., 2004; Allen-Sharpley and Cramer, 2012). This link is further supported by experiments in which the crossing NM axon branches were severed (Figure 2B), resulting in deafferentation of the ventral NL dendrites (Rubel et al., 1981). The resulting rapid atrophy of these dendrites was accompanied by a substantial invasion of glial cells into the ventral cell-free neuropil region. NL glia thus define a boundary around the nuclei, and the integrity of this boundary is dependent on innervation from NM.
Figure 2. The glial margin surrounding NL is disrupted by deafferentation. (A) Normal Nissl stained coronal section illustrating NM and the monolayer of cell bodies in NL (arrow). A relatively cell body-free neuropil zone is bordered by densely packed, small glial cells. (B) Eight days after a tract cut at the midline, a massive movement of glial cells into the denervated ventral region was observed. Adapted from Rubel et al. (1981). Scale bar, 50 μm, applies to both panels.
The topographic gradient of ephrin-B2 in glia surrounding NL suggests a potential role for these cells not only in axon targeting, but perhaps also in other features that are graded along the frequency axis. We explored one such feature, the gradient of NL dendritic arbor morphology that emerges in late embryonic development. We reasoned that the relatively late appearance of glial fibrillary acidic protein (GFAP)-positive astrocytes coincides with this process, and we tested the effect of GFAP-positive astrocyte conditioned medium (ACM) on dendritic maturation in an organotypic auditory brainstem slice culture that permitted repeated imaging of individual labeled dendrites (Korn et al., 2011). We prepared slices from E13 embryos, when few GFAP-positive astrocytes are seen, and exposed them to ACM from astrocytes purified from more mature brainstems. We found that in slices cultured with ACM, dendrites in NL exhibited a loss of primary dendrites, with a greater effect in caudolateral (low frequency) regions, whereas control cultures did not show a significant change over the same period. The results suggest that this aspect of dendritic maturation is regulated by secreted signals from astrocytes. The graded nature of the effect, obtained with bath application of ACM, indicates that NL cells vary in their responsiveness to astrocytic factors. Orthogonal to this axis, the dorsoventrally oriented glial fibers seen in mature NL have been postulated to contribute to the bidirectional orientation of NL dendrites (Kalman et al., 1998). Glial influences may thus contribute to several aspects of dendrite arbor morphology.
In addition to these studies of dendritic regulation, we used our organotypic preparation to study the effects of ACM on synaptogenesis (Korn et al., 2012). We found that inhibitory synapses begin forming in NL later than excitatory synapses, and they become more abundant at ages when GFAP-positive astrocytes are present. Inhibitory inputs from SON were quantified with counts of puncta immunolabeled for the vesicular GABA transporter (VGAT). We found that ACM resulted in a significantly enhanced rate of inhibitory synaptogenesis compared to control cultures. Notably, the treated cultures added inhibitory synapses at a time course similar to that seen in vivo, suggesting that astrocyte secreted factors may mediate progression of inhibitory synapse formation. The identity of these factors remains to be determined.
Conduction timing in the NM-NL pathway is a critical factor in ITD detection. It is especially complicated by the fact that coincidence is needed from two distinct inputs, one from the nearby ipsilateral NM and the other from the distant contralateral NM. Early studies showed that the ipsilateral NM axon branch loops around NM before arriving in NL (Young and Rubel, 1986), thereby increasing the conduction time to make it more similar to the contralateral branch. However, several additional factors are needed to adjust this timing. Recently, precise measurements of axon length incorporating 3-dimensional reconstructions showed that the contralateral NM axon branches are roughly twice the length of the ipsilateral branch in the chick (Seidl et al., 2010; Seidl, 2014). Remarkably, the two NM axon branches differed in diameter and in the spacing between nodes of Ranvier, resulting in differences in conduction velocity to optimize temporal integration (Seidl et al., 2014). The regulation of axon thickness and internodal distance demonstrates a critical role for myelinating oligodendrocytes in the establishment of the functional pathway.
While the mechanisms that regulate spacing between nodes of Ranvier in this pathway are not known, some of the factors that guide myelination during development are beginning to be understood. Myelination is regulated by activity-dependent communication between neurons and oligodendrocytes, both during initial development and in adult life (Barres and Raff, 1993; Lin and Bergles, 2004; Fields, 2015; Hines et al., 2015). Myelination of NM axons in the chick embryo (Korn and Cramer, 2008) and particularly in the barn owl (Cheng and Carr, 2007) occurs relatively late in development, after the onset of neuronal activity. The link between neuronal activity and myelination may result directly from the non-synaptic release of neurotransmitters that bind to receptors on oligodendrocytes. Activity may also act by increasing expression of molecules that have necessary roles in myelination, such as brain derived neurotrophic factor (BDNF) and the cell adhesion molecule L1CAM (Barbin et al., 2004; Wong et al., 2013; Purger et al., 2015). The remarkable and important contribution by the studies on the chick and mammalian studies (see below) on the brainstem ITD pathways is that the ipsilateral and contralateral processes of the same axon are independently regulated. These findings indicate that the molecular cues must act locally on individual branches rather than through the cell bodies of the glial cells.
As in birds, the mammalian auditory brainstem displays precise innervation and specialized synaptic structures that facilitate sound source localization. Glial cells contribute to the maturation and function of these pathways.
Action potential timing is also important in mammalian sound localization pathways, shown in Figure 3. In the mammalian ITD pathway (Figure 3A), spherical bushy cells (SBCs) in the anterior ventral cochlear nucleus (VCN) send axons that branch and make excitatory contacts with neurons in the medial superior olive (MSO) on both sides of the brain. A recent study in gerbils demonstrated that, like NM axons, contralateral SBC axon branches projecting to MSO have thicker axon diameters and longer internode distances (Seidl and Rubel, 2016). The difference in axon thickness was first observed at postnatal day (P) 20. In contrast, the difference in internode distance was already evident at P10, which is prior to hearing onset (Woolf and Ryan, 1984). If activity-dependent regulation is important for this aspect of myelination, it may rely on spontaneous neuronal activity, which is generated early in development (Tritsch et al., 2010; Wang and Bergles, 2015). It is also important to consider later maturation because the relative timing between the two ears will be altered by changes in head size. This aspect has not been studied to date and it will likely involve a change in the distribution of nodes that depends on activity.
Figure 3. Neuroanatomy of mammalian auditory brainstem pathways used in sound localization. (A) In the ITD pathway, neurons in the ventral cochlear nucleus (VCN) project axons that branch and provide excitatory input to the medial superior olive (MSO) on both sides of the brain, with ipsilateral and contralateral inputs to lateral and medial MSO dendrites, respectively. Globular bushy cells (GBCs) in VCN synapse with neurons in the medial nucleus of the trapezoid body (MNTB) on the contralateral side, where they terminate in the calyx of Held, shown on the left side. MNTB provides one source of inhibitory input to MSO neurons. (B) In the interaural level difference (ILD) pathway, lateral superior olive (LSO) neurons receive excitatory input from spherical bushy cells (SBCs) in ipsilateral VCN and inhibitory input from ipsilateral MNTB. Because sounds are louder near the sound source, the LSO on the side of the sound source receives more excitation and less inhibition, while the opposite holds in the LSO more distant from the sound source.
In the ILD pathway (Figure 3B), SBCs project to the lateral superior olive (LSO) on the ipsilateral side. A major component of both pathways includes inhibition from the ipsilateral medial nucleus of the trapezoid body (MNTB), a sign reversing relay nucleus that receives excitatory input from globular bushy cells (GBCs) in contralateral VCN (Kuwabara and Zook, 1991; Grothe and Sanes, 1993, 1994; Pecka et al., 2008). The long axon of the GBC neuron terminates in MNTB in a large specialized synaptic ending known as the calyx of Held (Held, 1893; Kuwabara and Zook, 1991; Kuwabara et al., 1991). LSO neurons receive excitatory input from the ipsilateral side, and inhibitory input originating from the contralateral side, and the balance of excitation and inhibition is used to determine ILDs (Tollin, 2003). Although the disynaptic inhibitory input from MNTB is longer and subject to synaptic delay, it arrives at the same time as the monosynaptic excitatory inputs, and even early in MSO (Roberts et al., 2013, 2014). A recent study (Ford et al., 2015) demonstrated that GBCs have thicker axon diameters and increased internodal distances compared to SBCs, accounting for this disparity. Additionally, they found systematic differences in these properties that varied with GBC projections along the mediolateral (high frequency to low frequency) axis of MNTB and showed that these variations provide optimal conduction times for these tonotopic locations. Thus in both ITD and ILD pathways, axon thickness and myelination are adjusted to generate precise timing.
Because of the wide range of glial functions involved in neuronal development, we characterized glial cell populations at key stages of auditory brainstem development. Oligodendrocytes and their precursors, identified with expression of oligodendrocyte transcription factor 2 (OLIG2), are seen in VCN and MNTB during the first postnatal week in mice (Dinh et al., 2014; Kolson et al., 2016). In rats, myelin basic protein is expressed in MNTB beginning at about P9 (Saliu et al., 2014), consistent with previous reports that myelination begins at about that age (Leão et al., 2005). In gerbils, myelin associated proteins are expressed in LSO during the first postnatal week, after which they display oriented patterns consistent with a role in guiding dendritic orientation of LSO neurons (Hafidi et al., 1996).
Along with oligodendrocytes, subpopulations of astrocytes are found in auditory nuclei during the early postnatal period in mice. As early as P0 we identified astrocytes in VCN and MNTB that were immunolabeled for aldehyde dehydrogenase 1 family member L1 (ALDH1L1), a specific marker that broadly labels astrocytes (Cahoy et al., 2008; Dinh et al., 2014; Kolson et al., 2016) and these cells increased in number during brainstem maturation. In contrast, we did not observe expression of the calcium binding protein, S100ß, or the intermediate filament, GFAP, two other astrocyte markers, in either nucleus at P0. S100ß-positive astrocytes were abundant in both VCN and MNTB by P6 (Dinh et al., 2014). In rats, S100ß-positive astrocytes were found in MNTB during the first postnatal week, where expression of this protein appears to be temporally correlated with the appearance of a group of non-neuronal cells that proliferate in the nucleus at this age (Saliu et al., 2014). As we found in chick embryos, GFAP expression was seen at later ages, with expression in VCN and MNTB first evident at about P14–23 in mice (Dinh et al., 2014). The GFAP expression revealed astrocytic fibers that coursed throughout the nucleus. In contrast, ALDH1L1 immunolabel at these ages appeared to coalesce densely around MNTB principal neurons. Vital labeling of astrocytes using sulforhodamine showed that they are present in LSO as early as P3, arranged in dense clusters of cells that express both GABA and glycine transporters and sequester inhibitory neurotransmitters (Stephan and Friauf, 2014). Together these studies reveal multiple populations of astrocytes throughout development. Their varied appearance and unique expression patterns suggest a range of functions in these developing nuclei.
A distinct population of glial cells, the microglia, also populate the auditory brainstem nuclei at early ages (Dinh et al., 2014). These cells, visualized with an antibody that recognizes the ionized calcium binding adaptor molecule 1 (IBA1), are found in small numbers in VCN at P0 and in MNTB at P6. Microglia in VCN and MNTB increase in number and in the abundance of ramified processes until they peak at about P14 and subsequently taper off in number. Both astrocytes and microglia are thus present at the time of hearing onset and both cell types are found in close apposition to the developing calyx of Held.
Glial cells form close associations with the calyx of Held. In mature animals, the calyx has a fenestrated morphology, with astrocytic processes labeled in openings in the calyx (Ford et al., 2009). Along with S100ß, ALDH1L1 and GFAP, astrocytes associated with the mature calyx also express glutamate transporters that result in sequestration of glutamate and release of glutamine, which would improve temporal precision at the calyx. (Ford et al., 2009; Uwechue et al., 2012; Dinh et al., 2014). Both astrocytes and microglia are seen in close apposition with the developing calyx of Held (Dinh et al., 2014), and ultrastructural studies have shown that long glial processes cover MNTB neurons at early postnatal ages, in some cases interposed between nascent calyxes and the postsynaptic neuronal surface (Holcomb et al., 2013). Similar associations with astrocytic processes are seen in the large synaptic endings of auditory nerve inputs to VCN known as the endbulbs of Held. These astrocytic processes are remarkably dynamic. Studies in the chick cochlear nucleus showed that the astrocytic process grow and retract dramatically within hours after changes in eighth nerve activity (Canady and Rubel, 1992; Rubel and MacDonald, 1992). An ultrastructural analysis further showed that when activity is dramatically reduced glial process rapidly intercalate between the axonal contacts and the postsynaptic neurons (Canady et al., 1994).
While GBC projections to MNTB are usually limited to the contralateral side, early unilateral removal of the cochlea leads to loss of VCN neurons on the lesioned side (Trune, 1982; Hashisaki and Rubel, 1989; Mostafapour et al., 2000) and subsequent branching of intact VCN axons that leads to induction of calyceal terminations in their ipsilateral MNTB (Kitzes et al., 1995; Russell and Moore, 1995; Hsieh et al., 2007). Both astrocytes and microglia show a similar proximity to the developing induced calyces (Dinh et al., 2014). The rapid appearance of the ipsilateral calyx and its association with glial cells precedes a measurable glial response to the injury. Glial cells in MNTB thus appear to have a distinct role in synaptic development, and this role can be activated for ipsilateral inputs when the normally occurring contralateral inputs are lost.
While specialized auditory pathways engage glial cells for their mature function, glial cells also contribute significantly to the assembly of these pathways. Recent studies have shown roles for glial cells in synaptogenesis and dendrite maturation. Additionally, developmental regulation of myelination and internode distance are critical factors in establishing conduction times required for temporal processing in sound localization pathways of birds and mammals. While much remains to be discovered on the mechanisms of neuron-glial communication in these developmental processes, progress has been made determining the effects of this communication at numerous points of contact. Glial cells are integral components of functioning auditory brainstem pathways. These recent studies suggest that subpopulations of glia contribute to brainstem development and to their integration into these circuit elements.
KSC drafted and edited the manuscript and prepared figures. EWR contributed to revision of the manuscript and preparation of figures.
The authors declare that the research was conducted in the absence of any commercial or financial relationships that could be construed as a potential conflict of interest.
The authors are grateful to Forrest Weghorst for comments on the manuscript. The research reviewed here from KSC’s laboratory was funded by NIH R01DC010796 and from EWR’s laboratory by DC04661, DC03829 and DC11343.
Agmon-Snir, H., Carr, C. E., and Rinzel, J. (1998). The role of dendrites in auditory coincidence detection. Nature 393, 268–272. doi: 10.1038/30505
Allen, N. J. (2013). Role of glia in developmental synapse formation. Curr. Opin. Neurobiol. 23, 1027–1033. doi: 10.1016/j.conb.2013.06.004
Allen-Sharpley, M. R., and Cramer, K. S. (2012). Coordinated Eph-ephrin signaling guides migration and axon targeting in the avian auditory system. Neural Dev. 7:29. doi: 10.1186/1749-8104-7-29
Barbin, G., Aigrot, M. S., Charles, P., Foucher, A., Grumet, M., Schachner, M., et al. (2004). Axonal cell-adhesion molecule L1 in CNS myelination. Neuron Glia Biol. 1, 65–72. doi: 10.1017/s1740925X04000092
Barres, B. A., and Raff, M. C. (1993). Proliferation of oligodendrocyte precursor cells depends on electrical activity in axons. Nature 361, 258–260. doi: 10.1038/361258a0
Burger, R. M., Fukui, I., Ohmori, H., and Rubel, E. W. (2011). Inhibition in the balance: binaurally coupled inhibitory feedback in sound localization circuitry. J. Neurophysiol. 106, 4–14. doi: 10.1152/jn.00205.2011
Cahoy, J. D., Emery, B., Kaushal, A., Foo, L. C., Zamanian, J. L., Christopherson, K. S., et al. (2008). A transcriptome database for astrocytes, neurons and oligodendrocytes: a new resource for understanding brain development and function. J. Neurosci. 28, 264–278. doi: 10.1523/JNEUROSCI.4178-07.2008
Canady, K. S., Hyson, R. L., and Rubel, E. W. (1994). The astrocytic response to afferent activity blockade in chick nucleus magnocellularis is independent of synaptic activation, age and neuronal survival. J. Neurosci. 14, 5973–5985.
Canady, K. S., and Rubel, E. W. (1992). Rapid and reversible astrocytic reaction to afferent activity blockade in chick cochlear nucleus. J. Neurosci. 12, 1001–1009.
Carr, C. E., and Konishi, M. (1990). A circuit for detection of interaural time differences in the brain stem of the barn owl. J. Neurosci. 10, 3227–3246.
Cheng, S. M., and Carr, C. E. (2007). Functional delay of myelination of auditory delay lines in the nucleus laminaris of the barn owl. Dev. Neurobiol. 67, 1957–1974. doi: 10.1002/dneu.20541
Clarke, L. E., and Barres, B. A. (2013). Emerging roles of astrocytes in neural circuit development. Nat. Rev. Neurosci. 14, 311–321. doi: 10.1038/nrn3484
Cramer, K. S., Bermingham-McDonogh, O., Krull, C. E., and Rubel, E. W. (2004). EphA4 signaling promotes axon segregation in the developing auditory system. Dev. Biol. 269, 26–35. doi: 10.1016/j.ydbio.2004.01.002
Dinh, M. L., Koppel, S. J., Korn, M. J., and Cramer, K. S. (2014). Distribution of glial cells in the auditory brainstem: normal development and effects of unilateral lesion. Neuroscience 278, 237–252. doi: 10.1016/j.neuroscience.2014.08.016
Edmonson, C. A., Ziats, M. N., and Rennert, O. M. (2016). A non-inflammatory role for microglia in autism spectrum disorders. Front. Neurol. 7:9. doi: 10.3389/fneur.2016.00009
Fields, R. D. (2015). A new mechanism of nervous system plasticity: activity-dependent myelination. Nat. Rev. Neurosci. 16, 756–767. doi: 10.1038/nrn4023
Ford, M. C., Alexandrova, O., Cossell, L., Stange-Marten, A., Sinclair, J., Kopp-Scheinpflug, C., et al. (2015). Tuning of Ranvier node and internode properties in myelinated axons to adjust action potential timing. Nat. Commun. 6:8073. doi: 10.1038/ncomms9073
Ford, M. C., Grothe, B., and Klug, A. (2009). Fenestration of the calyx of Held occurs sequentially along the tonotopic axis, is influenced by afferent activity and facilitates glutamate clearance. J. Comp. Neurol. 514, 92–106. doi: 10.1002/cne.21998
Grothe, B., and Sanes, D. H. (1993). Bilateral inhibition by glycinergic afferents in the medial superior olive. J. Neurophysiol. 69, 1192–1196.
Grothe, B., and Sanes, D. H. (1994). Synaptic inhibition influences the temporal coding properties of medial superior olivary neurons: an in vitro study. J. Neurosci. 14, 1701–1709.
Hackett, J. T., Jackson, H., and Rubel, E. W. (1982). Synaptic excitation of the second and third order auditory neurons in the avian brain stem. Neuroscience 7, 1455–1469. doi: 10.1016/0306-4522(82)90257-3
Hafidi, A., Katz, J. A., and Sanes, D. H. (1996). Differential expression of MAG, MBP and L1 in the developing lateral superior olive. Brain Res. 736, 35–43. doi: 10.1016/s0006-8993(96)00653-1
Hashisaki, G. T., and Rubel, E. W. (1989). Effects of unilateral cochlea removal on anteroventral cochlear nucleus neurons in developing gerbils. J. Comp. Neurol. 283, 5–73. doi: 10.1002/cne.902830402
Hines, J. H., Ravanelli, A. M., Schwindt, R., Scott, E. K., and Appel, B. (2015). Neuronal activity biases axon selection for myelination in vivo. Nat. Neurosci. 18, 683–689. doi: 10.1038/nn.3992
Holcomb, P. S., Hoffpauir, B. K., Hoyson, M. C., Jackson, D. R., Deerinck, T. J., Marrs, G. S., et al. (2013). Synaptic inputs compete during rapid formation of the calyx of Held: a new model system for neural development. J. Neurosci. 33, 12954–12969. doi: 10.1523/JNEUROSCI.1087-13.2013
Hsieh, C. Y., Hong, C. T., and Cramer, K. S. (2007). Deletion of EphA4 enhances deafferentation-induced ipsilateral sprouting in auditory brainstem projections. J. Comp. Neurol. 504, 508–518. doi: 10.1002/cne.21465
Hyson, R. L. (2005). The analysis of interaural time differences in the chick brain stem. Physiol. Behav. 86, 297–305. doi: 10.1016/j.physbeh.2005.08.003
Jhaveri, S., and Morest, D. K. (1982). Neuronal architecture in nucleus magnocellularis of the chicken auditory system with observations on nucleus laminaris: a light and electron microscope study. Neuroscience 7, 809–836. doi: 10.1016/0306-4522(82)90045-8
Kalman, M., Szekely, A. D., and Csillag, A. (1998). Distribution of glial fibrillary acidic protein and vimentin-immunopositive elements in the developing chicken brain from hatch to adulthood. Anat. Embryol. (Berl) 198, 213–235. doi: 10.1007/s004290050179
Kitzes, L. M., Kageyama, G. H., Semple, M. N., and Kil, J. (1995). Development of ectopic projections from the ventral cochlear nucleus to the superior olivary complex induced by neonatal ablation of the contralateral cochlea. J. Comp. Neurol. 353, 341–363. doi: 10.1002/cne.903530303
Kolson, D. R., Wan, J., Wu, J., Dehoff, M., Brandebura, A. N., Qian, J., et al. (2016). Temporal patterns of gene expression during calyx of held development. Dev. Neurobiol. 76, 166–189. doi: 10.1002/dneu.22306
Korn, M. J., and Cramer, K. S. (2008). Distribution of glial-associated proteins in the developing chick auditory brainstem. Dev. Neurobiol. 68, 1093–1106. doi: 10.1002/dneu.20645
Korn, M. J., Koppel, S. J., and Cramer, K. S. (2011). Astrocyte-secreted factors modulate a gradient of primary dendritic arbors in nucleus laminaris of the avian auditory brainstem. PLoS One 6:e27383. doi: 10.1371/journal.pone.0027383
Korn, M. J., Koppel, S. J., Li, L. H., Mehta, D., Mehta, S. B., Seidl, A. H., et al. (2012). Astrocyte-secreted factors modulate the developmental distribution of inhibitory synapses in nucleus laminaris of the avian auditory brainstem. J. Comp. Neurol. 520, 1262–1277. doi: 10.1002/cne.22786
Kuwabara, N., DiCaprio, R. A., and Zook, J. M. (1991). Afferents to the medial nucleus of the trapezoid body and their collateral projections. J. Comp. Neurol. 314, 684–706. doi: 10.1002/cne.903140405
Kuwabara, N., and Zook, J. M. (1991). Classification of the principal cells of the medial nucleus of the trapezoid body. J. Comp. Neurol. 314, 707–720. doi: 10.1002/cne.903140406
Lachica, E. A., Rubsamen, R., and Rubel, E. W. (1994). GABAergic terminals in nucleus magnocellularis and laminaris originate from the superior olivary nucleus. J. Comp. Neurol. 348, 403–418. doi: 10.1002/cne.903480307
Leão, R. M., Kushmerick, C., Pinaud, R., Renden, R., Li, G. L., Taschenberger, H., et al. (2005). Presynaptic Na+ channels: locus, development and recovery from inactivation at a high-fidelity synapse. J. Neurosci. 25, 3724–3738. doi: 10.1523/JNEUROSCI.3983-04.2005
Lin, S. C., and Bergles, D. E. (2004). Synaptic signaling between neurons and glia. Glia 47, 290–298. doi: 10.1002/glia.20060
Mostafapour, S. P., Cochran, S. L., Del Puerto, N. M., and Rubel, E. W. (2000). Patterns of cell death in mouse anteroventral cochlear nucleus neurons after unilateral cochlea removal. J. Comp. Neurol. 426, 561–571. doi: 10.1002/1096-9861(20001030)426:4<561::AID-CNE5>3.0.CO;2-G
Ohmori, H. (2014). Neuronal specializations for the processing of interaural difference cues in the chick. Front. Neural Circuits 8:47. doi: 10.3389/fncir.2014.00047
Overholt, E. M., Rubel, E. W., and Hyson, R. L. (1992). A circuit for coding interaural time differences in the chick brainstem. J. Neurosci. 12, 1698–1708.
Parks, T. N., and Rubel, E. W. (1975). Organization and development of brain stem auditory nuclei of the chicken: organization of projections from n. magnocellularis to n. laminaris. J. Comp. Neurol. 164, 435–448. doi: 10.1002/cne.901640404
Pecka, M., Brand, A., Behrend, O., and Grothe, B. (2008). Interaural time difference processing in the mammalian medial superior olive: the role of glycinergic inhibition. J. Neurosci. 28, 6914–6925. doi: 10.1523/JNEUROSCI.1660-08.2008
Person, A. L., Cerretti, D. P., Pasquale, E. B., Rubel, E. W., and Cramer, K. S. (2004). Tonotopic gradients of Eph family proteins in the chick nucleus laminaris during synaptogenesis. J. Neurobiol. 60, 28–39. doi: 10.1002/neu.10330
Purger, D., Gibson, E. M., and Monje, M. (2015). Myelin plasticity in the central nervous system. Neuropharmacology doi: 10.1016/j.neuropharm.2015.08.001 [Epub ahead of print].
Roberts, M. T., Seeman, S. C., and Golding, N. L. (2013). A mechanistic understanding of the role of feedforward inhibition in the mammalian sound localization circuitry. Neuron 78, 923–935. doi: 10.1016/j.neuron.2013.04.022
Roberts, M. T., Seeman, S. C., and Golding, N. L. (2014). The relative contributions of MNTB and LNTB neurons to inhibition in the medial superior olive assessed through single and paired recordings. Front. Neural Circuits 8:49. doi: 10.3389/fncir.2014.00049
Rubel, E. W., and MacDonald, G. H. (1992). Rapid growth of astrocytic processes in N. magnocellularis following cochlea removal. J. Comp. Neurol. 318, 415–425. doi: 10.1002/cne.903180406
Rubel, E. W., Smith, D. J., and Miller, L. C. (1976). Organization and development of brain stem auditory nuclei of the chicken: ontogeny of n. magnocellularis and n. laminaris. J. Comp. Neurol. 166, 469–489. doi: 10.1002/cne.901660408
Rubel, E. W., Smith, Z. D., and Steward, O. (1981). Sprouting in the avian brainstem auditory pathway: dependence on dendritic integrity. J. Comp. Neurol. 202, 397–414. doi: 10.1002/cne.902020309
Russell, F. A., and Moore, D. R. (1995). Afferent reorganisation within the superior olivary complex of the gerbil: development and induction by neonatal, unilateral cochlear removal. J. Comp. Neurol. 352, 607–625. doi: 10.1002/cne.903520409
Saliu, A., Adise, S., Xian, S., Kudelska, K., and Rodríguez-Contreras, A. (2014). Natural and lesion-induced decrease in cell proliferation in the medial nucleus of the trapezoid body during hearing development. J. Comp. Neurol. 522, 971–985. doi: 10.1002/cne.23473
Seidl, A. H. (2014). Regulation of conduction time along axons. Neuroscience 276, 126–134. doi: 10.1016/j.neuroscience.2013.06.047
Seidl, A. H., and Rubel, E. W. (2016). Systematic and differential myelination of axon collaterals in the mammalian auditory brainstem. Glia 64, 487–494. doi: 10.1002/glia.22941
Seidl, A. H., Rubel, E. W., and Barría, A. (2014). Differential conduction velocity regulation in ipsilateral and contralateral collaterals innervating brainstem coincidence detector neurons. J. Neurosci. 34, 4914–4919. doi: 10.1523/JNEUROSCI.5460-13.2014
Seidl, A. H., Rubel, E. W., and Harris, D. M. (2010). Mechanisms for adjusting interaural time differences to achieve binaural coincidence detection. J. Neurosci. 30, 70–80. doi: 10.1523/JNEUROSCI.3464-09.2010
Smith, Z. D. (1981). Organization and development of brain stem auditory nuclei of the chicken: dendritic development in N. laminaris. J. Comp. Neurol. 203, 309–333. doi: 10.1002/cne.902030302
Smith, D. J., and Rubel, E. W. (1979). Organization and development of brain stem auditory nuclei of the chicken: dendritic gradients in nucleus laminaris. J. Comp. Neurol. 186, 213–239. doi: 10.1002/cne.901860207
Stephan, J., and Friauf, E. (2014). Functional analysis of the inhibitory neurotransmitter transporters GlyT1, GAT-1 and GAT-3 in astrocytes of the lateral superior olive. Glia 62, 1992–2003. doi: 10.1002/glia.22720
Stevens, B. (2008). Neuron-astrocyte signaling in the development and plasticity of neural circuits. Neurosignals 16, 278–288. doi: 10.1159/000123038
Tollin, D. J. (2003). The lateral superior olive: a functional role in sound source localization. Neuroscientist 9, 127–143. doi: 10.1177/1073858403252228
Tritsch, N. X., Rodriguez-Contreras, A., Crins, T. T., Wang, H. C., Borst, J. G., and Bergles, D. E. (2010). Calcium action potentials in hair cells pattern auditory neuron activity before hearing onset. Nat. Neurosci. 13, 1050–1052. doi: 10.1038/nn.2604
Trune, D. R. (1982). Influence of neonatal cochlear removal on the development of mouse cochlear nucleus: I. Number, size and density of its neurons. J. Comp. Neurol. 209, 409–424. doi: 10.1002/cne.902090410
Uwechue, N. M., Marx, M. C., Chevy, Q., and Billups, B. (2012). Activation of glutamate transport evokes rapid glutamine release from perisynaptic astrocytes. J. Physiol. 590, 2317–2331. doi: 10.1113/jphysiol.2011.226605
Wang, H. C., and Bergles, D. E. (2015). Spontaneous activity in the developing auditory system. Cell Tissue Res. 361, 65–75. doi: 10.1007/s00441-014-2007-5
Wong, A. W., Xiao, J., Kemper, D., Kilpatrick, T. J., and Murray, S. S. (2013). Oligodendroglial expression of TrkB independently regulates myelination and progenitor cell proliferation. J. Neurosci. 33, 4947–4957. doi: 10.1523/jneurosci.3990-12.2013
Woolf, N. K., and Ryan, A. F. (1984). The development of auditory function in the cochlea of the mongolian gerbil. Hear. Res. 13, 277–283. doi: 10.1016/0378-5955(84)90081-9
Yang, L., Monsivais, P., and Rubel, E. W. (1999). The superior olivary nucleus and its influence on nucleus laminaris: a source of inhibitory feedback for coincidence detection in the avian auditory brainstem. J. Neurosci. 19, 2313–2325.
Young, S. R., and Rubel, E. W. (1983). Frequency-specific projections of individual neurons in chick brainstem auditory nuclei. J. Neurosci. 3, 1373–1378.
Keywords: nucleus laminaris, nucleus magnocellularis, medial nucleus of the trapezoid body, astrocyte, oligodendrocyte, delay line, microglia, calyx of Held
Citation: Cramer KS and Rubel EW (2016) Glial Cell Contributions to Auditory Brainstem Development. Front. Neural Circuits 10:83. doi: 10.3389/fncir.2016.00083
Received: 03 August 2016; Accepted: 04 October 2016;
Published: 21 October 2016.
Edited by:
Joel C. Glover, University of Oslo, NorwayReviewed by:
Karl Kandler, University of Pittsburgh, USACopyright © 2016 Cramer and Rubel. This is an open-access article distributed under the terms of the Creative Commons Attribution License (CC BY). The use, distribution and reproduction in other forums is permitted, provided the original author(s) or licensor are credited and that the original publication in this journal is cited, in accordance with accepted academic practice. No use, distribution or reproduction is permitted which does not comply with these terms.
*Correspondence: Karina S. Cramer, Y3JhbWVya0B1Y2kuZWR1
Disclaimer: All claims expressed in this article are solely those of the authors and do not necessarily represent those of their affiliated organizations, or those of the publisher, the editors and the reviewers. Any product that may be evaluated in this article or claim that may be made by its manufacturer is not guaranteed or endorsed by the publisher.
Research integrity at Frontiers
Learn more about the work of our research integrity team to safeguard the quality of each article we publish.