- 1Department of Neurosurgery, Wuhan Asia General Hospital, Wuhan, Hubei, China
- 2Department of Neurosurgery, Zhongnan Hospital of Wuhan University, Wuhan, Hubei, China
- 3Department of Infectious Diseases, Zhongnan Hospital of Wuhan University, Wuhan, Hubei, China
- 4Department of Respiratory and Critical Care Medicine, Laifeng County People’s Hospital, Enshi, Hubei, China
Cerebral aneurysm (CA) is a significant health concern that results from pathological dilations of blood vessels in the brain and can lead to severe and potentially life-threatening conditions. While the pathogenesis of CA is complex, emerging studies suggest that endothelial progenitor cells (EPCs) play a crucial role. In this paper, we conducted a comprehensive literature review to investigate the potential role of EPCs in the pathogenesis and treatment of CA. Current research indicates that a decreased count and dysfunction of EPCs disrupt the balance between endothelial dysfunction and repair, thus increasing the risk of CA formation. Reversing these EPCs abnormalities may reduce the progression of vascular degeneration after aneurysm induction, indicating EPCs as a promising target for developing new therapeutic strategies to facilitate CA repair. This has motivated researchers to develop novel treatment options, including drug applications, endovascular-combined and tissue engineering therapies. Although preclinical studies have shown promising results, there is still a considerable way to go before clinical translation and eventual benefits for patients. Nonetheless, these findings offer hope for improving the treatment and management of this condition.
Introduction
Cerebral aneurysms (CA), also known as intracranial aneurysms, are abnormal focal dilations of the wall of cerebral arteries, typically occurring at branching points within the circle of Willis (Vlak et al., 2011; Brown and Broderick, 2014; Macdonald and Schweizer, 2017). These aneurysms are often saccular in shape, hence the term “berry aneurysms” (Brown and Broderick, 2014). CA occur in about 1–2% of the population, with prevalence estimates from imaging studies suggesting a frequency of 0.5–3% in the general population (Brown and Broderick, 2014; Tawk et al., 2021). They are more commonly found in women than men, with a ratio of 3:1, and are predominantly detected in the anterior locations of the circle of Willis (Sedat et al., 2005; Brown and Broderick, 2014; Abecassis et al., 2017). CA can lead to numerous clinical manifestations such as subarachnoid hemorrhage (SAH), compression of adjacent cerebral structures, causing symptoms like cranial nerve palsies, hemiparesis, field defects, seizures, brainstem compression, and transient ischemic attacks or cerebral infarction due to distal embolization (Brown and Broderick, 2014).
About 50% of CA are discovered following a SAH, which has a global incidence of approximately 6.1 per 100,000 person-years, with a prevalence of 8.09 million cases (Etminan et al., 2019; Tsao et al., 2023). The burden of SAH includes a high rate of mortality and long-term disability. About 13% of patients will die in the hospital of aneurysmal SAH, and up to 26% will die before reaching the hospital (Wahood et al., 2022). And of those who survive, many experience significant long-term disabilities., such as brain damage, hydrocephalus, and cognitive impairment (Wan et al., 2016; Toth and Cerejo, 2018). The economic impact is substantial, with inpatient hospital charges for patients with aneurysmal SAH reported to be $373,353.94 in the United States (Mualem et al., 2022).
CA is not a single disease entity: some can result from a genetic vulnerability, others from infection or inflammation. The pathophysiology of cerebral aneurysms involves complex interactions between genetic predisposition, hemodynamic stress, and vascular wall abnormalities. Factors such as hypertension, smoking, and genetic mutations contribute to the weakening of the arterial wall, leading to aneurysm formation and potential rupture (Brisman et al., 2006; Etminan and Rinkel, 2017; Frösen et al., 2019; Toader et al., 2023). And the functional deficiencies of cells involved in vascular wall remodeling, such as endothelial progenitor cells (EPCs) and smooth muscle cells (SMCs), may be crucial actors in the pathophysiology of CA (Lee et al., 2015; Xu et al., 2019).
The relationship between EPCs and CA is complex and multifaceted. EPCs are cells that originate from the bone marrow and migrate to areas of blood vessel damage, where they differentiate into functional endothelial cells (ECs) and contribute to the repair and maintenance of blood vessels integrity (Asahara et al., 1997). In patients with CA, the levels and function of circulating EPCs are altered, suggesting that these cells play a crucial role in the development and progression of these conditions (Liang et al., 2014). EPCs represent the essential initiating factor of re-endothelialization process that responded for aneurysm complete occlusion after endovascular treatment (Li et al., 2013, 2021). Targeted approaches that promote the mobilization of EPCs and increase their circulating count have demonstrated promising therapeutic effects in preclinical studies of CA (Liu et al., 2016a,b). Therefore, a deeper understanding of the relationship between EPCs and CA at the pathological level may provide valuable insights into the development and progression of these conditions and inform the development of new, more effective treatments. It is not optimistic that there is not a lot of research about EPCs and CA at present, which also suggests that a summary is urgently needed in this field to provide reference for future research direction.
In this narrative review, we aim to provide a comprehensive understanding of the involvement of EPCs in the pathogenesis of CA and to evaluate the potential of EPCs as a target for the prevention and treatment of intracranial aneurysms.
The role of EPCs in endothelial function and integrity
The vascular endothelium acts as the first line of defense in protecting the vascular wall from direct stress caused by chemical, inflammatory, and mechanical factors (Aoki et al., 2011). Fortunately, our body has a natural mechanism for repairing damaged or dysfunctional endothelium, which involves the activation of various signaling pathways and the recruitment of repair cells (Evans et al., 2021). EPCs have been proven to play a crucial role in maintaining vascular homeostasis and function by promoting neovascularization and re-endothelialization of injured vessels (Sobhan et al., 2012). EPCs were first identified by Asahara et al. (1997) in isolated mononuclear cells from human peripheral blood (Asahara et al., 1997). These bone marrow (BM)–derived cells with high proliferative ability were found to have the potential to differentiate into ECs (Laurenzana et al., 2015) and are involved in both physiological processes triggered by endothelial dysfunction, such as wound healing and the ovarian cycle, as well as pathological events such as hypertension, stroke, and cancer (Schlager et al., 2011; de Cavanagh et al., 2014). EPCs are believed to originate from mesoderm cells during embryonic development, just like hematopoietic stem cells (De Val and Black, 2009). In a healthy state, EPCs reside in the BM microenvironment with low oxygen levels and high levels of stromal cell-derived factor 1 alpha (SDF-1α), which is essential for their survival (Balaji et al., 2013). However, during times of inflammation, injury, or hypoxia, EPCs are “mobilized” and leave the BM, entering the bloodstream due to triggers such as chemokines, matrix metalloproteinase (MMP) 9, vascular endothelial growth factor (VEGF), and nitric oxide (NO) (Velazquez, 2007; Tilling et al., 2009). Once they reach the target tissue, they are activated and guided by tissue-specific chemokine signaling. After adhering to the blood vessel and tissue repair sites through integrins, EPCs migrate through the endodermis to perform their function in neovascularization and re-endothelialization by transforming into ECs and remodeling the extracellular matrix components (Qin et al., 2006; Hynes, 2009). EPCs play a crucial role in maintaining the integrity of vascular endothelium by producing pro-angiogenic factors that boost the proliferation, survival, and function of mature ECs and other nearby progenitor cells, such as smooth muscle progenitor cells and SMCs (Laurenzana et al., 2015).
The formation of aneurysms is widely believed to result from an imbalance between the factors that damage the vascular endothelium and the mechanisms responsible for repairing this damage (Soldozy et al., 2019; Xu et al., 2019). Thus, exploring the different expressions of EPCs between CA patients and healthy people may open the window to gain insight into the mechanisms of CA pathogenesis.
Current research on EPCs count and function in CA
In order to summarize the current knowledge on the relationship between EPCs and CA, A comprehensive literature search in PubMed, Embase and Ovid was performed. Search terms included all possible combinations of “Cerebral aneurysm,” “Intracranial aneurysm” and “Endothelial progenitor cell.” Only studies published in English or Chinese up to 1 April 2024, were considered. References in identified articles were also manually screened. This review was reported in accordance with the SANRA guidelines (Baethge et al., 2019). Several clinical and preclinical studies focused on EPCs characteristics in the CA condition have been found. Details of these studies can be found in Table 1.
Human research
Direct evidence from human CA clinical samples confirmed abnormal count and dysfunction of EPCs in the CA condition. For example, in Wei H. et al. (2011) described a decreased number and impaired migratory capacity of EPCs from peripheral blood in both unruptured (21 patients) and ruptured (35 patients) CA patients compared with healthy control subjects. Consistently, data from Liang et al. (2014) indicated the EPCs count was also significantly decreased in 24 unruptured CA patients, accompanied by decreases in proliferative, migratory and adhesive capacities, compared with EPCs from the control group. These findings seem to be contrary to our conventional understanding that a larger number of EPCs should be sent into circulation after aneurysm formation to repair damaged vascular intima. This discrepancy may be due to the fact that the same factors that cause harm to the vascular intima–such as trauma or blood flow shear stress–also hinder the mobilization or activation of EPCs (Dzau et al., 2005; Zampetaki et al., 2008). Wei H. J. et al. (2011) measured numbers of circulating EPCs in CA patients before and after surgical coil embolization, and they found a biphasic change pattern of circulating EPCs, which first decreased upon admission (before surgery) and then increased after surgery as compared to healthy subjects. They proposed that the mechanism of reducing circulating EPCs after CA induction may be related to consumption and stress induced by injury, similar to a phenomenon in the early hours of traumatic brain injury (Liu et al., 2007).
Translational (animal and in vitro) research
In translational research, animal models and in vitro studies have also explored EPCs in the context of CA. When researchers created animal models of CA by surgically ligating intracranial arteries to cause hemodynamic damage to the intima, decreased EPCs levels was detected only in rats that underwent surgery, rather than non-surgical control rats (Xu et al., 2011). These findings suggest that the same factors that cause endothelial damage—such as hemodynamic stress—also impede the mobilization or activation of EPCs. Further supporting this, in vitro studies have shown that the levels and functionality of EPCs are altered in CA patients, which may be due to increased EPCs senescence (Leone et al., 2009). For example, Wei H. et al. (2011) found that EPCs isolated from CA patients showed higher levels of senescence-associated β-galactosidase activity, a marker for cell senescence, than those from normal subjects. This increased senescence activity was significantly negatively correlated with the number of circulating EPCs, indicating that EPCs senescence might play a role in the reduced EPCs count observed in CA conditions (Figure 1 and Table 1).
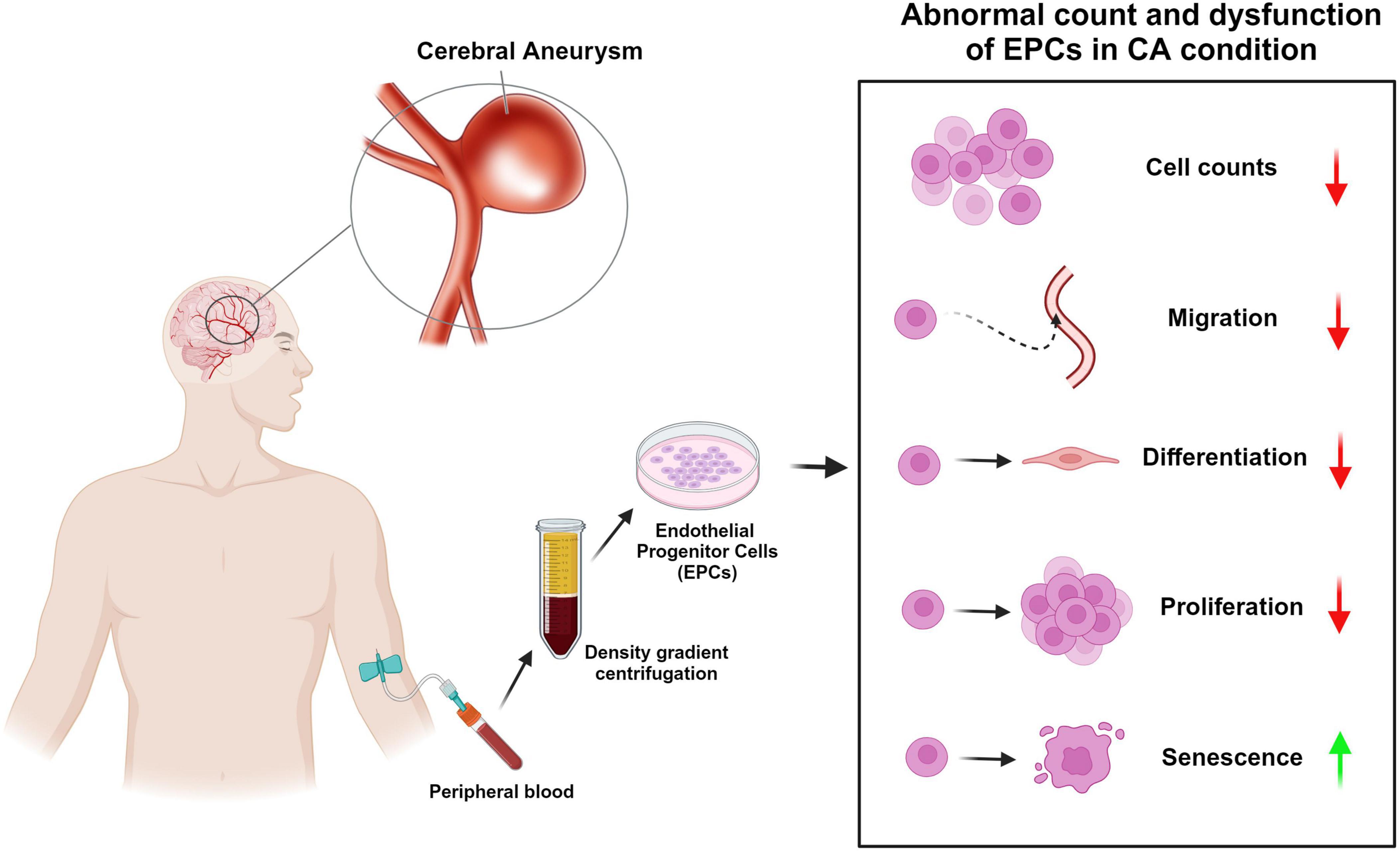
Figure 1. Abnormal count and dysfunction of endothelial progenitor cells (EPCs) in cerebral aneurysm (CA) condition. This diagram shows the rough process of obtaining EPCs from peripheral blood of CA patients and summarizes the abnormal cell counts and dysfunction of EPCs in CA condition reported in previous studies. The red arrow indicates “decreases” and the green arrow indicates “increase.”
Pre-clinical EPCs-based treatment research for CA
Above findings suggest that the formation of CA is related to both a decrease in the number of circulating EPCs and a decrease in their biological abilities. These findings naturally raise the question of whether reversing the abnormalities of circulating EPCs in CA patients could repair the intima or stabilize aneurysms.
To test this hypothesis, Fang et al. (2012) performed an elastase-induced approach (Wang et al., 2009) to simulate the destruction of the vascular intima during aneurysm formation to induce carotid saccular aneurysms in rabbit models. Then, the autologous fluorescent labeled EPCs were injected into the animals in either the in situ carotid artery (ISCT group) or marginal ear veins (IVT group). Three weeks after undergoing elastase treatment, the EPCs-transfusion group animals were found to have thicker aneurysmal vessel walls and more ECs and a less-disrupted internal elastic lamina compared to controls. Fluorescein–labeled EPCs were found in both the thrombosis and neointima of the aneurysm wall in 5/5 animals in the ISCT group and in 3/5 animals in the IVT group. These results suggested that EPCs are required in the repair response to vascular injury after aneurysm formation. However, labeled EPCs in this study were not found at the intimal surfaces of the aneurysms, and immunohistochemical staining showed that there was no ECs growth in the neointima. Since EPCs differentiation depends on the microenvironment provided, the authors proposed that the inflammatory microenvironment of injured vessels may have suppressed EPCs from differentiating into the epithelium in this rabbit model. Besides, this study didn’t show whether the transfusion of EPCs could reduce the size of the aneurysm or the risk of aneurysm rupture. Previous studies demonstrated that the degeneration of the vessel wall and subsequent inflammation invasion followed by vascular remodeling and aneurysm enlargement are associated with CA prone to rupture (Kataoka et al., 1999; Aoki et al., 2008). In this regard, Li et al. (2014) conducted a study to see if the supplementation of EPCs could reverse vascular degeneration by regulating the inflammation cascade and apoptosis activity. Endothelial colony-forming cells (ECFCs), a unique population of EPCs that possess high proliferation, self-renewal and in vivo vasculogenic potentials (Ingram et al., 2004), were adopted in this study. Briefly, CA was generated in rats via arterial surgical ligation, and ECFCs were then intravenously injected. At 2 months after aneurysm induction, the aneurysm vessel was harvested for pathological, immunological and molecular biological tests. Interestingly, ECFCs transfusion significantly reduced the degeneration of the internal elastic lamina (IEL), media thinning and the CA size. ECFCs effectively inhibited the MMP-driven wall destruction by downregulating MMP-2 and MMP-9 expression and upregulating the tissue inhibitor of metalloproteinases-1 (TIMP-1). Furthermore, inflammation factors like vascular cell adhesion molecule-1 (VCAM-1) and NF-κB and macrophage infiltration in the aneurysm wall were also suppressed by ECFCs transfusion.
Besides the direct transplantation of EPCs, various factors stimulating the mobilization and recruitment of EPCs to the site of an aneurysm were applied to test the potential role of EPCs in CA repair. Erythropoietin (EPO) is believed to promote stem cell mobilization, differentiation and maturation toward cells of endothelial lineage (Burger et al., 2006). Xu et al. (2011) treated CA rats EPO and found that EPO treatment significantly increased the numbers of circulating EPCs, reduced aneurysm volume, decreased IEL score, and thickened medial layer at 1 month and 3 months after surgery as compared to rats without EPO treatment. Similarly, SDF-1α is a vital inflammation cytokine, and is also related to mobilizing progenitor cells and stem cells into injured tissues (Ceradini et al., 2004; Wang et al., 2008). Li et al. (2018) found the in vivo administration of SDF-α to rabbits with saccular aneurysms promoted endothelial-lineage cell mobilization into the peripheral blood and reendothelialization of the aneurysm wall. Hoh et al. (2014) also found that SDF-1 promotes endothelial cell and macrophage migration complied with robust angiogenesis in the walls of aneurysms. However, they accidentally found that mice given an anti-SDF-1 blocking antibody developed significantly fewer intracranial aneurysms. This infers that SDF-1 can promote endothelial repair by inducing endothelial cell migration and capillary tube formation, while it can also facilitate aneurysm formation and rupture by promoting angiogenesis and inflammatory cell infiltration of the aneurysm wall. This finding suggests that the role of EPCs in the pathogenesis of aneurysms is complex and multifaceted.
Taken together, the above findings demonstrated that the transfusion or stimulation of EPCs was effective in reducing the progression of vascular degeneration after aneurysm induction in animal models, which implies EPCs are a promising target for the development of new therapeutic strategies to facilitate CA repair (Figure 2 and Table 1). It is worth noting that these studies are currently preclinical animal studies, and clinical studies involving humans are still lacking.
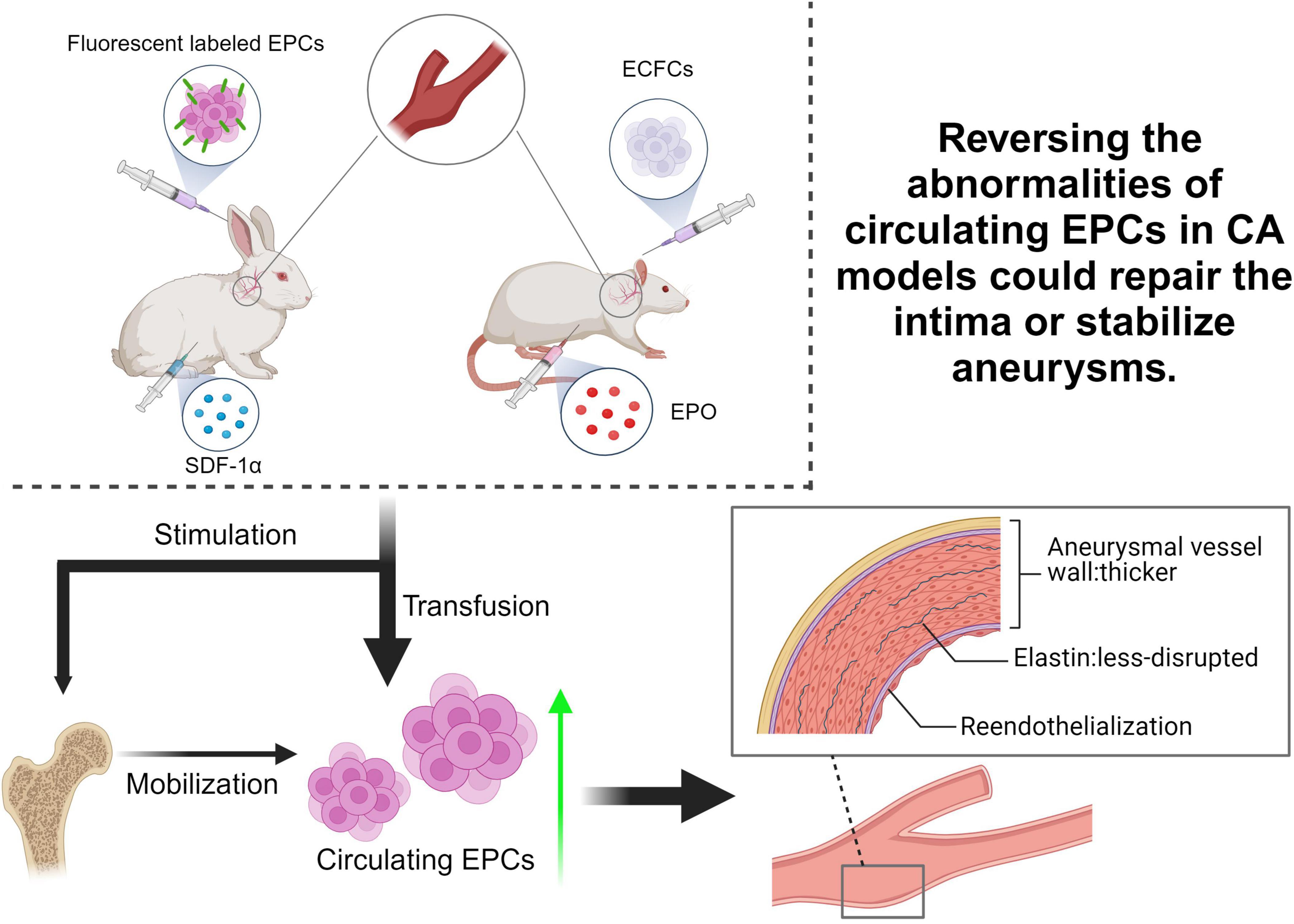
Figure 2. Summary of pre-clinical EPC-based treatment researches for CA. Current studies primarily used rabbit or rat as animal models to simulate CA, aiming to verify the hypothesis that reversing the abnormalities of circulating EPCs in CA patients could repair the intima or stabilize aneurysms. By transfusing EPCs or factors stimulating EPCs mobilization, such as Stromal Cell-Derived Factor 1 alpha (SDF-1α) and Erythropoietin (EPO), into CA animal models to increase circulating EPCs, the progression of vascular degeneration after aneurysm induction was effectively reduced. ECFCs, endothelial colony-forming cells.
The effect of current and emerging treatments for CA on EPCs
The involvement of EPCs in the formation and repair of aneurysm has inspired researchers to consider new therapeutic approaches for treating CA based on EPCs. This involves using agents to regulate the quantity/function of EPCs in the patient’s circulation to enhance the process of repairing the vascular intima and stabilize the aneurysm, or even treat it.
Drugs stimulating EPCs mobilization
One common approach explored in published studies is the use of clinical drugs to stimulate the mobilization and recruitment of EPCs to the site of an aneurysm. For example, pre-clinical animal experiments demonstrated that statins such as rosuvastatin and atorvastatin can promote the mobilization of EPCs, leading to enhanced aneurysm neck endothelialization and reduced degeneration of aneurysms (Wei et al., 2019; Xu et al., 2021). Similarly, aspirin, a well-established antiplatelet and nonsteroidal anti-inflammatory drug, was also proven to significantly reduce the degeneration of aneurysm walls in a rat model by augmenting EPCs mobilization and inhibiting macrophage-mediated chronic vascular inflammation (Li et al., 2015). It is worth noting that both statins and aspirin, in addition to increasing the number of EPCs, also have the effect of suppressing inflammation in the aneurysm wall. The expression of many inflammatory factors such as iNOS, MMP-2, MMP-9, VEGF, NF-κB and macrophage chemoattractant protein 1 (MCP-1) is inhibited by these drugs. Previous studies have demonstrated that after sustained hemodynamic shear stress causes endothelial dysfunction at the bifurcation, inflammation is activated and extensive invasion of macrophages, T lymphocytes, and B lymphocytes occurs in aneurysm tissue, leading to increased damage to the vessel wall (Chyatte et al., 1999; Deanfield et al., 2007). Therefore, these drugs with the dual effect of activating EPCs and anti-inflammation may be more effective in stabilizing aneurysm walls and preventing aneurysm rupture than factors with the sole effect of EPCs elevating.
Endovascular surgery combined with EPCs agonists
In addition to drug applications, novel tissue engineering therapies of “endovascular surgery combined with EPCs agonists” also show promising prospects in CA treatment (Ravindran et al., 2019). After coil embolization, the neointima and endothelium are formed in the neck of CAs to seal the aneurysm cavity in order to prevent hemodynamic stress from the parent artery toward the aneurysm wall (Sugiu et al., 1995; Berenstein et al., 2009). Animal research has revealed that recanalization of previously closed aneurysms occurs only when there is insufficient endothelialization covering the ostium (Reul et al., 1997; Macdonald et al., 1998; Kallmes et al., 1999; Li et al., 2013). This has also been supported by autopsy and clinical studies conducted on humans, which have linked recanalization to inadequate endothelialization of the aneurysm ostium (Molyneux et al., 1995; Mizoi et al., 1996). Therefore, the use of EPCs activators to enhance endothelialization over the aneurysm lumen / neck after endovascular therapy seems reasonable, and this approach has indeed shown promising clinical application in animal studies. For example, the combination of the stimulation of EPCs by agonists such as miR-31a-5p (Yu et al., 2019), EPO (Liu et al., 2016b) and rosuvastatin (Liu et al., 2016a) before or after with endovascular surgery have shown promising results in the treatment of aneurysms in rats model. By inducing the process of endothelialization in the neck of an aneurysm after coil embolization, these approaches can reduce the risk of aneurysm rupture and provide a safer and more effective treatment option for patients.
As mentioned before, SDF-1α is a vital chemoattractant to stem cells and potentially facilitates reendothelialization. Gao et al. (2014) used the SDF-1α-coated coils in CA endovascular occlusion and found better organized fibrous tissue bridging the orifice of aneurysms on days 14 and 28 after coil implantation in SDF-1α-coated coils + EPCs transplantation rats than in the unmodified coils rats’ group. They suggested that SDF-1α-coated coils with EPCs or mesenchymal stem cells transplantation may be beneficial in the aneurysm healing and endothelialization at the orifice of the embolized aneurysm (Gao et al., 2016).
Flow diverter (FD) implants and EPC-based tissue engineering approaches
Besides coils, high rates of complete aneurysm occlusion have been reported using FD implants that lead to parent vessel reconstruction and intrasaccular aneurysm thrombosis. According to Marosfoi et al. (2017), circulating CD34+ EPCs contribute to endothelialization after FD implantation in rabbit model and that EPCs are present throughout the healing process up to 60 days. Preliminary evidence demonstrated the temporal and spatial dependence of endothelialization on FD design. Special FD designs can incorporate these analyses during development to accelerate the in-situ tissue response to the scaffold. What’s more, the joint use of recombinant human SDF-1α administration accelerates endothelial-like cells expansion at the aneurysm neck in elastase-induced saccular aneurysm rabbits after FD treatment (Li et al., 2017). In addition, a novel tissue engineering approach using an endothelial progenitor cell-seeded biopolymer to treat intracranial saccular aneurysm has been proposed (Aronson et al., 2012). In this study, EPCs cultures were trypsinized and combined with the fibrin polymer. The fibrin + EPCs polymer was then injected into the rabbits’ aneurysm via the microcatheter. In aneurysms treated with endovascular fibrin + EPCs, a confluent monolayer of ECs with underlying neointima was demonstrated across the neck at 16 weeks post-treatment, which was not observed with aneurysms treated using the other methods such as surgical clipping, coil and fibrin polymer treatment alone (Aronson et al., 2012).
Briefly, the role of EPCs in the formation and repair of cerebral aneurysms has gained increasing attention in recent years. Various therapeutic approaches have been explored to utilize EPCs for the treatment of CA, including drug applications with / without endovascular surgery, and novel device and tissue engineering therapies. These novel therapeutic approaches have provided new insights into the treatment of CAs and offer promising possibilities for patients in the future (Figure 3 and Table 1). It is important to note that these findings are based on preclinical animal studies, and there is still a lack of clinical studies involving humans.
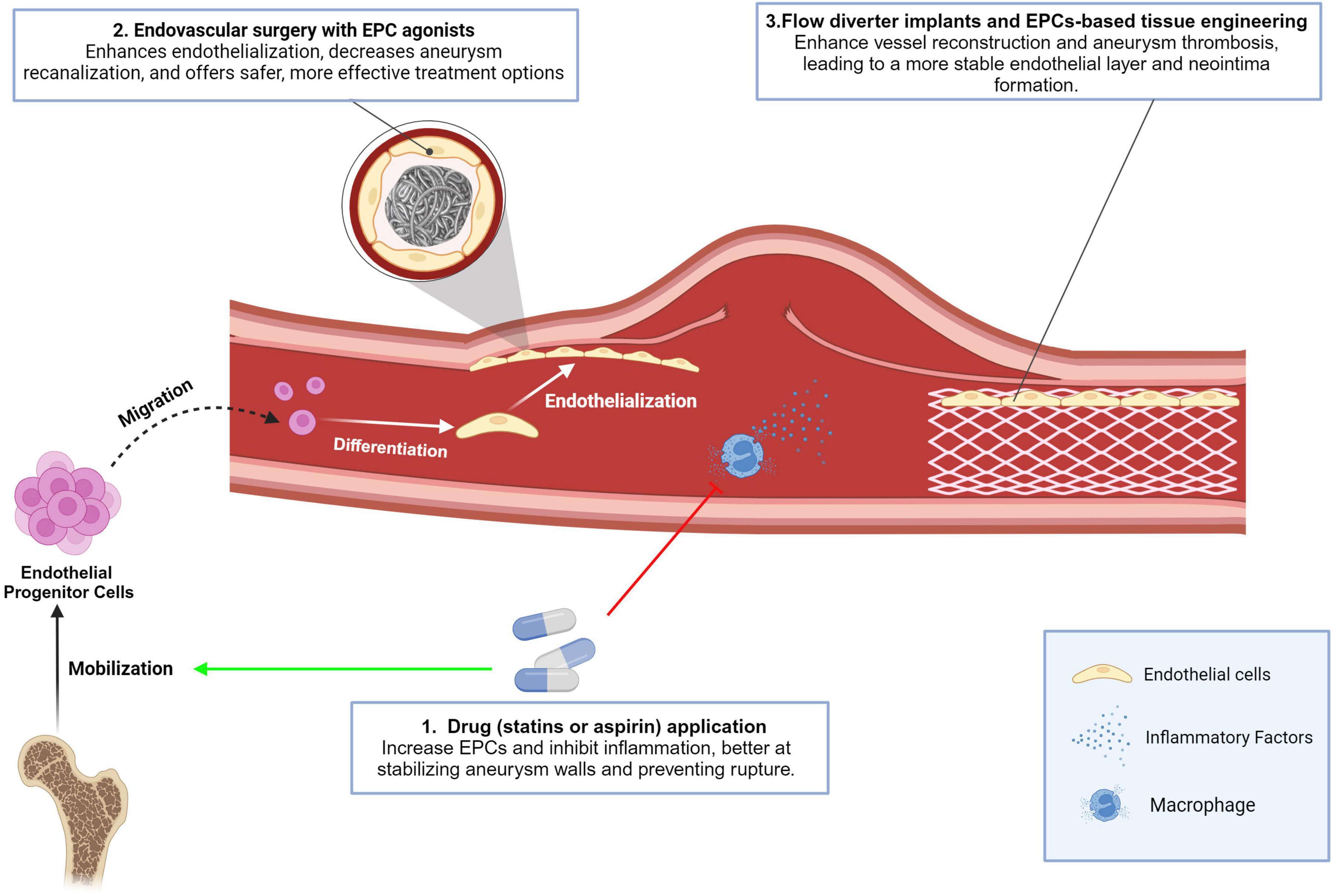
Figure 3. Conclusion in the effect of current and emerging treatments for CA on EPCs. Drugs stimulating EPCs mobilization: Statins (rosuvastatin, atorvastatin) and aspirin mobilize EPCs to aneurysm sites, enhancing endothelialization of aneurysm necks, reducing degeneration, and suppressing inflammation by inhibiting factors like iNOS, MMP-2, MMP-9, VEGF, NF-κB, MCP-1, and VCAM-1. These drugs also offer anti-inflammatory benefits, aiding aneurysm stabilization and rupture prevention; Endovascular surgery with EPCs agonists: EPCs activators enhance endothelialization over aneurysm areas after surgery. Combining EPCs stimulation with agonists (e.g., miR-31a-5p, EPO, rosuvastatin) improves treatment outcomes by reducing recanalization and enhancing safety and effectiveness; Flow diverter implants and EPCs-based tissue engineering: Flow diverter implants facilitate vessel reconstruction and aneurysm thrombosis, with EPCs supporting endothelialization. EPC-based tissue engineering uses biopolymer- combined EPCs injected into aneurysms, resulting in a stable endothelial layer and neointima formation.
Future directions
The existing problems will guide the future direction. First of all, there is a scarcity of relevant studies, particularly those investigating the expression characteristics of EPCs in clinical samples of patients with CA, and the sample sizes in each study are relatively small. As a result, the relevant findings may be subject to considerable deviation. For example, the studies failed to clarify the exact role of EPCs subtypes in the development of CA. As we know, EPCs can be categorized as “early EPCs” or “late EPCs” based on their morphology and function. Early EPCs are characterized by their ability to form clusters or colonies in vitro, while late EPCs are more similar to mature ECs in phenotype and exhibit tubular formation and proliferative capabilities (Hur et al., 2004; Shantsila et al., 2007). These differences are identified through different culture methods and various cell surface markers, such as CD34, CD45, CD133, and VEGFR-2 (Yu et al., 2020). In the reviewed studies, researchers have not made a clear distinction between the two EPC subtypes, and have mainly used CD34+/CD133+ with or without VEGFR2+ to define “circulating EPCs.” Although these studies have produced consistent results regarding the decreased number and function of EPCs in CA patients, it is important to distinguish between the expression characteristics of EPC subtypes in CA, as different subtypes may exhibit opposite characteristics in the same disease. For instance, in Moyamoya disease, another cerebrovascular disease with progressive vascular stenosis associated with intracranial abnormal angiogenesis, a decrease in the number and reduced proliferation ability of early EPCs was observed, but late EPCs presented a completely opposite characteristic (Jung et al., 2008; Rafat et al., 2009). In fact, different subtypes of EPCs also show different effects on CA repair in the CA. for example, ECFCs, a “late EPCs,” is considered to have strong proliferation ability and shows significantly better arterial wall repair ability than unscreened circulating EPCs (Fang et al., 2012; Li et al., 2014). Future studies should use targeted cell markers to describe the expression characteristics of different EPCs subtypes in CA patients and explore the different contributions of early / late EPCs to the pathogenesis of CA.
Second, the studies included in this paper lack comprehensive investigation into the mechanisms through which EPCs affect CA formation or repair. Although some studies have identified related factors such as VEGF, MMP, and iNOS, the specific mechanisms by which these factors regulate EPCs have not been explored. Given the complexity of organisms, the emergence of EPCs may merely represent one of many reactions that can occur when drugs or implants are introduced. For example, statins lower blood lipids, aspirin inhibits inflammation, and coils can cause thrombosis. Thus, reduced vascular degeneration following aneurysm induction cannot be solely attributed to EPCs, and these factors likely participate in vascular repair through alternative mechanisms. Similarly, the conditions, dosages, side effects, and long-term effects of drugs and new tissue engineering materials have yet to be assessed through long-term, rigorous experiments. We anticipate the participation of more medical centers in future research on this topic, as well as additional exploration of the molecular mechanisms underlying EPCs regulation of CA formation, the standardization of drug/device usage, and an examination of side effects.
Summary
Mechanistic link between EPCs and CA
The involvement of EPCs in the pathogenesis of CA is primarily linked to their role in vascular repair and endothelial regeneration. EPCs are crucial for maintaining vascular integrity through neovascularization and re-endothelialization. Their dysfunction or reduced presence can exacerbate endothelial damage, which is a key precursor to aneurysm formation (Mandelbaum et al., 2013). This imbalance between endothelial damage and repair mechanisms, exacerbated by factors such as high wall shear stress and chronic inflammation, contributes to aneurysm pathogenesis (Soldozy et al., 2019; Xu et al., 2019). High shear stress leads to endothelial dysfunction, promoting inflammation and matrix degradation, processes that EPCs normally counteract through their reparative functions (Tutino et al., 2014; Zhou et al., 2014; Frösen et al., 2019; Koseki et al., 2020). Referring to the “Current research on EPCs count and function in CA” section we mentioned above, there is evidence that EPCs play an important role in both the occurrence and progression of CA due to their role in endothelial repair and their interaction with inflammation and stromal remodeling processes.
Potential as a treatment strategy
Recent pre-clinical and clinical research has explored the potential of EPCs as a therapeutic target for CA. Various strategies have been employed to enhance EPCs function or mobilization, such as drug treatments and novel surgical techniques. Drugs like statins and aspirin have been shown to mobilize EPCs and simultaneously reduce inflammation within aneurysm walls, thus stabilizing aneurysms and reducing the risk of rupture (Li et al., 2015; Wei et al., 2019; Xu et al., 2021). Endovascular surgeries combined with EPCs agonists, such as EPO and SDF-1α, have demonstrated promise in enhancing endothelialization and reducing aneurysm recanalization (Liu et al., 2016a,b; Yu et al., 2019). Additionally, EPC-based tissue engineering approaches, including the use of EPC-seeded biopolymers and flow diverter implants, have shown improved outcomes in pre-clinical models, suggesting a significant therapeutic potential (Aronson et al., 2012; Li et al., 2017; Marosfoi et al., 2017).
Integration with existing models of CA pathogenesis
The evidence linking EPCs to CA fits well into existing models of aneurysm formation, which emphasize the balance between endothelial damage and repair. EPCs play a pivotal role in counteracting endothelial dysfunction and promoting vascular repair, which aligns with the understanding that aneurysm formation involves a disruption of this balance. Current models of CA pathogenesis focus on the interplay between mechanical stress, inflammation, and endothelial dysfunction. EPCs, by influencing these processes, provide a crucial link between endothelial damage and the potential for therapeutic intervention. This mechanistic insight supports the integration of EPCs-targeted therapies within these models, highlighting their role in not only understanding CA development but also in developing effective treatments (Frosen et al., 2006; Aoki et al., 2011).
Strengths and limitations
This review provides a comprehensive examination of the role of EPCs in CA pathogenesis and treatment, synthesizing evidence from clinical, preclinical, and translational research; it summarized various therapeutic approaches, including drug-based and tissue engineering strategies, showing promising results in preclinical models; and it discusses the potential of EPCs as a target for novel treatments, offering new insights into CA management.
However, the review also has limitations: it relies on a restricted literature search from specific databases, potentially missing relevant studies; Due to the limited number of relevant studies, most of which focus on preclinical animal research with limited clinical validation, the conclusions and inferences drawn from this review may not fully reflect the human condition; Many studies included have small sample sizes, which may introduce bias and limit generalizability.
Conclusion
In conclusion, this paper has provided a detailed overview of the current understanding of the role of EPCs in the pathogenesis and treatment of CAs. Clinical and preclinical studies consistently show a decrease in both the number and function of EPCs in CA patients, which impairs the repair response to vascular injury and may contribute to aneurysm formation and progression. Preclinical studies using EPCs-based therapies, such as EPCs transfusion and drugs that stimulate EPCs mobilization, have demonstrated potential benefits in enhancing aneurysm neck endothelialization, reducing aneurysm wall degeneration, and stabilizing aneurysms. Specifically, drugs like statins and aspirin, as well as tissue engineering approaches combining EPCs with endovascular surgery, have shown promise in animal models. However, these findings are primarily from preclinical studies, and there is a lack of clinical trials to validate these approaches in human patients. Future research should focus on distinguishing the roles of different EPCs subtypes in CA, understanding the molecular mechanisms underlying EPCs-mediated repair, and conducting rigorous clinical trials to assess the safety and efficacy of EPC-based therapies in CA treatment. Despite these challenges, the promising results from preclinical studies suggest that targeting EPCs could be a viable strategy for improving the management and treatment of CA.
Author contributions
JY: Conceptualization, Data curation, Formal analysis, Funding acquisition, Investigation, Methodology, Project administration, Resources, Software, Supervision, Validation, Visualization, Writing – original draft, Writing – review & editing. QD: Writing – original draft, Writing – review & editing. XL: Writing – review & editing, Conceptualization, Visualization, Software. WW: Writing – review & editing, Conceptualization, Visualization, Software. YF: Writing – original draft, Writing – review & editing. JZ: Writing – original draft, Writing – review & editing. JC: Conceptualization, Data curation, Formal analysis, Funding acquisition, Investigation, Methodology, Project administration, Resources, Software, Supervision, Validation, Visualization, Writing – original draft, Writing – review & editing.
Funding
The authors declare that financial support was received for the research, authorship, and/or publication of this article. This work was supported by the Hubei Provincial Natural Science Foundation of China (2024AFB308), The Outstanding Postdoctoral Research Fund of Zhongnan Hospital, Wuhan University (ZNYB2021002), and The Science and Technology Innovation Cultivation Fund of Zhongnan Hospital, Wuhan University (CXPY2022027).
Acknowledgments
The authors thank all participants in the study.
Conflict of interest
The authors declare that the research was conducted in the absence of any commercial or financial relationships that could be construed as a potential conflict of interest.
Publisher’s note
All claims expressed in this article are solely those of the authors and do not necessarily represent those of their affiliated organizations, or those of the publisher, the editors and the reviewers. Any product that may be evaluated in this article, or claim that may be made by its manufacturer, is not guaranteed or endorsed by the publisher.
References
Abecassis, I. J., Morton, R. P., McGrath, L., Hanson, J., Xue, A. H., Kelly, C. M., et al. (2017). Respiratory and swallowing outcomes based on aneurysm location in 360 patients with subarachnoid hemorrhage. World Neurosurg. 105, 108–114. doi: 10.1016/j.wneu.2017.05.110
Aoki, T., Kataoka, H., Ishibashi, R., Nozaki, K., and Hashimoto, N. (2008). Nifedipine inhibits the progression of an experimentally induced cerebral aneurysm in rats with associated down-regulation of NF-kappa B transcriptional activity. Cur. Neurovasc. Res. 5, 37–45. doi: 10.2174/156720208783565663
Aoki, T., Nishimura, M., Matsuoka, T., Yamamoto, K., Furuyashiki, T., Kataoka, H., et al. (2011). PGE(2) -EP(2) signalling in endothelium is activated by haemodynamic stress and induces cerebral aneurysm through an amplifying loop via NF-κB. Br. J. Pharmacol. 163, 1237–1249. doi: 10.1111/j.1476-5381.2011.01358.x
Aronson, J. P., Mitha, A. P., Hoh, B. L., Auluck, P. K., Pomerantseva, I., Vacanti, J. P., et al. (2012). A novel tissue engineering approach using an endothelial progenitor cell-seeded biopolymer to treat intracranial saccular aneurysms. J. Neurosurg. 117, 546–554. doi: 10.3171/2012.5.JNS091308
Asahara, T., Murohara, T., Sullivan, A., Silver, M., van der Zee, R., Li, T., et al. (1997). Isolation of putative progenitor endothelial cells for angiogenesis. Science 275, 964–967. doi: 10.1126/science.275.5302.964
Baethge, C., Goldbeck-Wood, S., and Mertens, S. (2019). SANRA-a scale for the quality assessment of narrative review articles. Res. Integr. Peer Rev. 4:5. doi: 10.1186/s41073-019-0064-8
Balaji, S., King, A., Crombleholme, T. M., and Keswani, S. G. (2013). The role of endothelial progenitor cells in postnatal vasculogenesis: Implications for therapeutic neovascularization and wound healing. Adv. Wound Care 2, 283–295. doi: 10.1089/wound.2012.0398
Berenstein, A., Song, J. K., Tsumoto, T., Namba, K., and Niimi, Y. (2009). Treatment of experimental aneurysms with an embolic-containing device and liquid embolic agent: Feasibility and angiographic and histological results. Neurosurgery 64, 367–373; discussion373. doi: 10.1227/01.NEU.0000335790.91413.64
Brisman, J. L., Song, J. K., and Newell, D. W. (2006). Cerebral aneurysms. N. Engl. J. Med. 355, 928–939. doi: 10.1056/NEJMra052760
Brown, R. D. Jr., and Broderick, J. P. (2014). Unruptured intracranial aneurysms: Epidemiology, natural history, management options, and familial screening. Lancet Neurol. 13, 393–404. doi: 10.1016/S1474-4422(14)70015-8
Burger, D., Lei, M., Geoghegan-Morphet, N., Lu, X., Xenocostas, A., and Feng, Q. (2006). Erythropoietin protects cardiomyocytes from apoptosis via up-regulation of endothelial nitric oxide synthase. Cardiovasc. Res. 72, 51–59. doi: 10.1016/j.cardiores.2006.06.026
Ceradini, D. J., Kulkarni, A. R., Callaghan, M. J., Tepper, O. M., Bastidas, N., Kleinman, M. E., et al. (2004). Progenitor cell trafficking is regulated by hypoxic gradients through HIF-1 induction of SDF-1. Nat. Med. 10, 858–864.
Chyatte, D., Bruno, G., Desai, S., and Todor, D. R. (1999). Inflammation and intracranial aneurysms. Neurosurgery 45, 1137–1146; discussion1146–1137. doi: 10.1097/00006123-199911000-00024
de Cavanagh, E. M., Gonzalez, S. A., Inserra, F., Forcada, P., Castellaro, C., Chiabaut-Svane, J., et al. (2014). Sympathetic predominance is associated with impaired endothelial progenitor cells and tunneling nanotubes in controlled-hypertensive patients. Am. J. Physiol. Heart Circ. Physiol. 307, H207–H215. doi: 10.1152/ajpheart.00955.2013
De Val, S., and Black, B. L. (2009). Transcriptional control of endothelial cell development. Dev. Cell 16, 180–195. doi: 10.1016/j.devcel.2009.01.014
Deanfield, J. E., Halcox, J. P., and Rabelink, T. J. (2007). Endothelial function and dysfunction: Testing and clinical relevance. Circulation 115, 1285–1295. doi: 10.1161/CIRCULATIONAHA.106.652859
Dzau, V. J., Gnecchi, M., Pachori, A. S., Morello, F., and Melo, L. G. (2005). Therapeutic potential of endothelial progenitor cells in cardiovascular diseases. Hypertension 46, 7–18. doi: 10.1161/01.HYP.0000168923.92885.f7
Etminan, N., and Rinkel, G. J. (2017). Unruptured intracranial aneurysms: Development, rupture and preventive management. Nat. Rev. Neurol. 13:126. doi: 10.1038/nrneurol.2017.14
Etminan, N., Chang, H. S., Hackenberg, K., de Rooij, N. K., Vergouwen, M. D. I., Rinkel, G. J. E., et al. (2019). Worldwide incidence of aneurysmal subarachnoid hemorrhage according to region, time period, blood pressure, and smoking prevalence in the population: A systematic review and meta-analysis. JAMA Neurol. 76, 588–597. doi: 10.1001/jamaneurol.2019.0006
Evans, C. E., Iruela-Arispe, M. L., and Zhao, Y.-Y. (2021). Mechanisms of endothelial regeneration and vascular repair and their application to regenerative medicine. Am. J. Pathol. 191, 52–65. doi: 10.1016/j.ajpath.2020.10.001
Fang, X., Zhao, R., Wang, K., Li, Z., Yang, P., Huang, Q., et al. (2012). Bone marrow-derived endothelial progenitor cells are involved in aneurysm repair in rabbits. J. Clin. Neurosci. 19, 1283–1286. doi: 10.1016/j.jocn.2011.09.038
Frösen, J., Cebral, J., Robertson, A. M., and Aoki, T. (2019). Flow-induced, inflammation-mediated arterial wall remodeling in the formation and progression of intracranial aneurysms. Neurosurg. Focus 47:E21. doi: 10.3171/2019.5.FOCUS19234
Frosen, J., Piippo, A., Paetau, A., Kangasniemi, M., Niemela, M., Hernesniemi, J., et al. (2006). Growth factor receptor expression and remodeling of saccular cerebral artery aneurysm walls: Implications for biological therapy preventing rupture. Neurosurgery 58, 534–541; discussion 534–541. doi: 10.1227/01.NEU.0000197332.55054.C8
Gao, Y., Lu, Z., Chen, C., Cui, X., Liu, Y., Zheng, T., et al. (2016). Mesenchymal stem cells and endothelial progenitor cells accelerate intra-aneurysmal tissue organization after treatment with SDF-1alpha-coated coils. Neurol. Res. 38, 333–341. doi: 10.1080/01616412.2016.1164433
Gao, Y., Wang, Q., Cui, X., Liu, Y., Zheng, T., Chen, C., et al. (2014). Controlled release of stromal cell-derived factor-1α from silk fibroin-coated coils accelerates intra-aneurysmal organization and occlusion of neck remnant by recruiting endothelial progenitor cells. Int. J. Clin. Exp. Pathol. 7, 8366–8380.
Hoh, B. L., Hosaka, K., Downes, D. P., Nowicki, K. W., Wilmer, E. N., Velat, G. J., et al. (2014). Stromal cell-derived factor-1 promoted angiogenesis and inflammatory cell infiltration in aneurysm walls. J. Neurosurg. 120, 73–86. doi: 10.3171/2013.9.JNS122074
Hur, J., Yoon, C.-H., Kim, H.-S., Choi, J.-H., Kang, H.-J., Hwang, K.-K., et al. (2004). Characterization of two types of endothelial progenitor cells and their different contributions to neovasculogenesis. Arteriosclerosis, Thrombosis, and Vascular Biology 24, 288–293.
Hynes, R. O. (2009). The extracellular matrix: Not just pretty fibrils. Science 326, 1216–1219. doi: 10.1126/science.1176009
Ingram, D. A., Mead, L. E., Tanaka, H., Meade, V., Fenoglio, A., Mortell, K., et al. (2004). Identification of a novel hierarchy of endothelial progenitor cells using human peripheral and umbilical cord blood. Blood 104, 2752–2760. doi: 10.1182/blood-2004-04-1396
Jung, K.-H., Chu, K., Lee, S.-T., Park, H.-K., Kim, D.-H., Kim, J.-H., et al. (2008). Circulating endothelial progenitor cells as a pathogenetic marker of moyamoya disease. J. Cereb. Blood Flow Metab. 28, 1795–1803. doi: 10.1038/jcbfm.2008.67
Kallmes, D. F., Helm, G. A., Hudson, S. B., Altes, T. A., Do, H. M., Mandell, J. W., et al. (1999). Histologic evaluation of platinum coil embolization in an aneurysm model in rabbits. Radiology 213, 217–222. doi: 10.1148/radiology.213.1.r99oc16217
Kataoka, K., Taneda, M., Asai, T., Kinoshita, A., Ito, M., and Kuroda, R. (1999). Structural fragility and inflammatory response of ruptured cerebral aneurysms. A comparative study between ruptured and unruptured cerebral aneurysms. Stroke 30, 1396–1401. doi: 10.1161/01.str.30.7.1396
Koseki, H., Miyata, H., Shimo, S., Ohno, N., Mifune, K., Shimano, K., et al. (2020). Two diverse hemodynamic forces, a mechanical stretch and a high wall shear stress, determine intracranial aneurysm formation. Transl. Stroke Res. 11, 80–92. doi: 10.1007/s12975-019-0690-y
Laurenzana, A., Fibbi, G., Margheri, F., Biagioni, A., Luciani, C., Del Rosso, M., et al. (2015). Endothelial progenitor cells in sprouting angiogenesis: Proteases pave the way. Curr. Mol. Med. 15, 606–620. doi: 10.2174/1566524015666150831131214
Lee, S., Kim, I. K., Ahn, J. S., Woo, D. C., Kim, S. T., Song, S., et al. (2015). Deficiency of endothelium-specific transcription factor Sox17 induces intracranial aneurysm. Circulation 131, 995–1005. doi: 10.1161/CIRCULATIONAHA.114.012568
Leone, A. M., Valgimigli, M., Giannico, M. B., Zaccone, V., Perfetti, M., D’Amario, D., et al. (2009). From bone marrow to the arterial wall: The ongoing tale of endothelial progenitor cells. Eur. Heart J. 30, 890–899. doi: 10.1093/eurheartj/ehp078
Li, J., Ma, Y., Miao, X. H., Guo, J. D., and Li, D. W. (2021). Neovascularization and tissue regeneration by endothelial progenitor cells in ischemic stroke. Neurol. Sci. 42, 3585–3593. doi: 10.1007/s10072-021-05428-3
Li, S., Tian, Y., Huang, X., Zhang, Y., Wang, D., Wei, H., et al. (2014). Intravenous transfusion of endothelial colony-forming cells attenuates vascular degeneration after cerebral aneurysm induction. Brain Res. 1593, 65–75. doi: 10.1016/j.brainres.2014.09.077
Li, S., Wang, D., Tian, Y., Wei, H., Zhou, Z., Liu, L., et al. (2015). Aspirin inhibits degenerative changes of aneurysmal wall in a rat model. Neurochem. Res. 40, 1537–1545. doi: 10.1007/s11064-015-1603-4
Li, Z. F., Fang, X. G., Yang, P. F., Huang, Q. H., Zhao, W. Y., Liang, C., et al. (2013). Endothelial progenitor cells contribute to neointima formation in rabbit elastase-induced aneurysm after flow diverter treatment. CNS Neurosci.Ther. 19, 352–357. doi: 10.1111/cns.12086
Li, Z., Zhao, R., Fang, X., Huang, Q., and Liu, J. (2017). Recombinant human SDF-1α administration accelerates aneurysm neck reendothelialization in rabbit saccular aneurysm after flow diverter treatment. Acta Biochim. Biophys. Sin. 49, 246–253. doi: 10.1093/abbs/gmx001
Li, Z.-F., Fang, X.-G., Zhao, R., Yang, P.-F., Huang, Q.-H., and Liu, J.-M. (2018). Stromal cell-derived factor 1α facilitates aneurysm remodeling in elastase-induced rabbit saccular aneurysm. Cytokine 102, 123–130. doi: 10.1016/j.cyto.2017.07.020
Liang, C., Feng, H., Deng, B. Q., Li, Z. F., Huang, Q. H., Zhao, W., et al. (2014). Decreased levels and function of circulating endothelial progenitor cells in unruptured intracranial saccular aneurysm patients. Neurol. Sci. 35, 23–28. doi: 10.1007/s10072-013-1459-9
Liu, L., Liu, H., Jiao, J., Liu, H., Bergeron, A., Dong, J. F., et al. (2007). Changes in circulating human endothelial progenitor cells after brain injury. J. Neurotrauma 24, 936–943. doi: 10.1089/neu.2006.0250
Liu, P., An, Q., Chen, X., Huang, J., Yang, G. Y., and Zhu, W. (2016a). Rosuvastatin for enhancement of aneurysm neck endothelialization after coil embolization: Promotion of endothelial progenitor cells in a rodent model. J. Neurosurg. 124, 1265–1274. doi: 10.3171/2015.3.JNS142841
Liu, P., Zhou, Y., An, Q., Song, Y., Chen, X., Yang, G. Y., et al. (2016b). Erythropoietin stimulates endothelial progenitor cells to induce endothelialization in an aneurysm neck after coil embolization by modulating vascular endothelial growth factor. Stem Cells Transl. Med. 5, 1182–1189. doi: 10.5966/sctm.2015-0264
Macdonald, R. L., and Schweizer, T. A. (2017). Spontaneous subarachnoid haemorrhage. Lancet 389, 655–666. doi: 10.1016/S0140-6736(16)30668-7
Macdonald, R. L., Mojtahedi, S., Johns, L., and Kowalczuk, A. (1998). Randomized comparison of Guglielmi detachable coils and cellulose acetate polymer for treatment of aneurysms in dogs. Stroke 29, 478–485; discussion 485–476. doi: 10.1161/01.str.29.2.478
Mandelbaum, M., Kolega, J., Dolan, J. M., Siddiqui, A. H., and Meng, H. (2013). A critical role for proinflammatory behavior of smooth muscle cells in hemodynamic initiation of intracranial aneurysm. PLoS One 8:e74357. doi: 10.1371/journal.pone.0074357
Marosfoi, M., Langan, E. T., Strittmatter, L., van der Marel, K., Vedantham, S., Arends, J., et al. (2017). In situ tissue engineering: Endothelial growth patterns as a function of flow diverter design. J. Neurointerv. Surg. 9, 994–998. doi: 10.1136/neurintsurg-2016-012669
Mizoi, K., Yoshimoto, T., Takahashi, A., and Nagamine, Y. (1996). A pitfall in the surgery of a recurrent aneurysm after coil embolization and its histological observation: Technical case report. Neurosurgery 39, 165–168; discussion 168–169. doi: 10.1097/00006123-199607000-00035
Molyneux, A. J., Ellison, D. W., Morris, J., and Byrne, J. V. (1995). Histological findings in giant aneurysms treated with Guglielmi detachable coils. Report of two cases with autopsy correlation. J. Neurosurg. 83, 129–132. doi: 10.3171/jns.1995.83.1.0129
Mualem, W., Durrani, S., Ghaith, A. K., Bhandarkar, A. R., Wahood, W., Tjoumakaris, S., et al. (2022). Factors associated with increased inpatient charges following aneurysmal subarachnoid hemorrhage with vasospasm: A nationwide analysis. Clin. Neurol. Neurosurg. 218:107259. doi: 10.1016/j.clineuro.2022.107259
Qin, G., Ii, M., Silver, M., Wecker, A., Bord, E., Ma, H., et al. (2006). Functional disruption of alpha4 integrin mobilizes bone marrow-derived endothelial progenitors and augments ischemic neovascularization. J. Exp. Med. 203, 153–163. doi: 10.1084/jem.20050459
Rafat, N., Beck, G., Pena-Tapia, P. G., Schmiedek, P., and Vajkoczy, P. (2009). Increased levels of circulating endothelial progenitor cells in patients with Moyamoya disease. Stroke 40, 432–438. doi: 10.1161/STROKEAHA.108.529420
Ravindran, K., Salem, M. M., Alturki, A. Y., Thomas, A. J., Ogilvy, C. S., and Moore, J. M. (2019). Endothelialization following flow diversion for intracranial aneurysms: A systematic review. AJNR Am. J. Neuroradiol. 40, 295–301. doi: 10.3174/ajnr.A5955
Reul, J., Weis, J., Spetzger, U., Konert, T., Fricke, C., and Thron, A. (1997). Long-term angiographic and histopathologic findings in experimental aneurysms of the carotid bifurcation embolized with platinum and tungsten coils. AJNR Am. J. Neuroradiol. 18, 35–42.
Schlager, O., Giurgea, A., Schuhfried, O., Seidinger, D., Hammer, A., Groger, M., et al. (2011). Exercise training increases endothelial progenitor cells and decreases asymmetric dimethylarginine in peripheral arterial disease: A randomized controlled trial. Atherosclerosis 217, 240–248. doi: 10.1016/j.atherosclerosis.2011.03.018
Sedat, J., Dib, M., Rasendrarijao, D., Fontaine, D., Lonjon, M., and Paquis, P. (2005). Ruptured intracranial aneurysms in the elderly: Epidemiology, diagnosis, and management. Neurocrit. Care 2, 119–123. doi: 10.1385/NCC:2:2:119
Shantsila, E., Watson, T., and Lip, G. Y. H. (2007). Endothelial progenitor cells in cardiovascular disorders. J. Am. Coll. Cardiol. 49, 741–752.
Sobhan, P. K., Seervi, M., Joseph, J., Varghese, S., Pillai, P. R., Sivaraman, D. M., et al. (2012). Immortalized functional endothelial progenitor cell lines from umbilical cord blood for vascular tissue engineering. Tissue Eng. Part C Methods 18, 890–902. doi: 10.1089/ten.TEC.2011.0482
Soldozy, S., Norat, P., Elsarrag, M., Chatrath, A., Costello, J. S., Sokolowski, J. D., et al. (2019). The biophysical role of hemodynamics in the pathogenesis of cerebral aneurysm formation and rupture. Neurosurg. Focus 47:E11. doi: 10.3171/2019.4.FOCUS19232
Sugiu, K., Kinugasa, K., Mandai, S., Tokunaga, K., and Ohmoto, T. (1995). Direct thrombosis of experimental aneurysms with cellulose acetate polymer (CAP): Technical aspects, angiographic follow up, and histological study. J. Neurosurg. 83, 531–538. doi: 10.3171/jns.1995.83.3.0531
Tawk, R. G., Hasan, T. F., D’Souza, C. E., Peel, J. B., and Freeman, W. D. (2021). Diagnosis and treatment of unruptured intracranial aneurysms and aneurysmal subarachnoid hemorrhage. Mayo Clin. Proc. 96, 1970–2000. doi: 10.1016/j.mayocp.2021.01.005
Tilling, L., Chowienczyk, P., and Clapp, B. (2009). Progenitors in motion: Mechanisms of mobilization of endothelial progenitor cells. Br. J. Clin. Pharmacol. 68, 484–492. doi: 10.1111/j.1365-2125.2009.03486.x
Toader, C., Eva, L., Bratu, B. G., Covache-Busuioc, R. A., Costin, H. P., Dumitrascu, D. I., et al. (2023). Intracranial aneurysms and genetics: An extensive overview of genomic variations, underlying molecular dynamics, inflammatory indicators, and forward-looking insights. Brain Sci. 13:1454. doi: 10.3390/brainsci13101454
Toth, G., and Cerejo, R. (2018). Intracranial aneurysms: Review of current science and management. Vasc. Med. 23, 276–288. doi: 10.1177/1358863X18754693
Tsao, C. W., Aday, A. W., Almarzooq, Z. I., Anderson, C. A. M., Arora, P., Avery, C. L., et al. (2023). Heart disease and stroke statistics-2023 update: A report from the american heart association. Circulation 147, e93–e621. doi: 10.1161/CIR.0000000000001123
Tutino, V. M., Mandelbaum, M., Choi, H., Pope, L. C., Siddiqui, A., Kolega, J., et al. (2014). Aneurysmal remodeling in the circle of Willis after carotid occlusion in an experimental model. J Cereb Blood Flow Metab 34, 415–424. doi: 10.1038/jcbfm.2013.209
Velazquez, O. C. (2007). Angiogenesis and vasculogenesis: Inducing the growth of new blood vessels and wound healing by stimulation of bone marrow-derived progenitor cell mobilization and homing. J. Vasc. Surg. 45, A39–A47. doi: 10.1016/j.jvs.2007.02.068
Vlak, M. H., Algra, A., Brandenburg, R., and Rinkel, G. J. (2011). Prevalence of unruptured intracranial aneurysms, with emphasis on sex, age, comorbidity, country, and time period: A systematic review and meta-analysis. Lancet Neurol. 10, 626–636. doi: 10.1016/S1474-4422(11)70109-0
Wahood, W., Rizvi, A. A., Alexander, A. Y., Yolcu, Y. U., Lanzino, G., Brinjikji, W., et al. (2022). Trends in admissions and outcomes for treatment of aneurysmal subarachnoid hemorrhage in the United States. Neurocrit. Care 37, 209–218. doi: 10.1007/s12028-022-01476-5
Wan, A., Jaja, B. N., Schweizer, T. A., and Macdonald, R. L. (2016). Clinical characteristics and outcome of aneurysmal subarachnoid hemorrhage with intracerebral hematoma. J. Neurosurg. 125, 1344–1351. doi: 10.3171/2015.10.JNS151036
Wang, K., Huang, Q., Hong, B., Xu, Y., Zhao, W., Chen, J., et al. (2009). Neck injury is critical to elastase-induced aneurysm model. AJNR Am. J. Neuroradiol. 30, 1685–1687. doi: 10.3174/ajnr.A1542
Wang, Y., Deng, Y., and Zhou, G. Q. (2008). SDF-1alpha/CXCR4-mediated migration of systemically transplanted bone marrow stromal cells towards ischemic brain lesion in a rat model. Brain Res. 1195, 104–112. doi: 10.1016/j.brainres.2007.11.068
Wei, H., Mao, Q., Liu, L., Xu, Y., Chen, J., Jiang, R., et al. (2011). Changes and function of circulating endothelial progenitor cells in patients with cerebral aneurysm. J. Neurosci. Res. 89, 1822–1828. doi: 10.1002/jnr.22696
Wei, H. J., Wang, D., Chen, J. L., Xu, Y., Lei, P., Jiang, R. C., et al. (2011). Mobilization of circulating endothelial progenitor cells after endovascular therapy for ruptured cerebral aneurysms. Neurosci. Lett. 498, 114–118. doi: 10.1016/j.neulet.2011.04.061
Wei, H., Yang, M., Yu, K., Dong, W., Liang, W., Wang, Z., et al. (2019). Atorvastatin protects against cerebral aneurysmal degenerative pathology by promoting endothelial progenitor cells (EPC) mobilization and attenuating vascular deterioration in a rat model. Med. Sci. Monit. 25, 928–936. doi: 10.12659/MSM.915005
Xu, Y., Tian, Y., Wei, H. J., Chen, J., Dong, J. F., Zacharek, A., et al. (2011). Erythropoietin increases circulating endothelial progenitor cells and reduces the formation and progression of cerebral aneurysm in rats. Neuroscience 181, 292–299. doi: 10.1016/j.neuroscience.2011.02.051
Xu, Y., Zhang, B., Chen, Y., Wang, X., Li, Y., Wu, J., et al. (2021). Simvastatin increases circulating endothelial progenitor cells and inhibits the formation of intracranial aneurysms in rats with diet-induced hyperhomocysteinemia. Neurosci. Lett. 760:136072. doi: 10.1016/j.neulet.2021.136072
Xu, Z., Rui, Y. N., Hagan, J. P., and Kim, D. H. (2019). Intracranial aneurysms: Pathology, genetics, and molecular mechanisms. Neuromol. Med. 21, 325–343. doi: 10.1007/s12017-019-08537-7
Yu, G., Liu, P., Shi, Y., Li, S., Liu, Y., Fan, Z., et al. (2019). Stimulation of endothelial progenitor cells by microRNA-31a-5p to induce endothelialization in an aneurysm neck after coil embolization by modulating the Axin1-mediated beta-catenin/vascular endothelial growth factor pathway. J. Neurosurg. 133, 918–926. doi: 10.3171/2019.5.JNS182901
Yu, J., Du, Q., Hu, M., Zhang, J., and Chen, J. (2020). Endothelial progenitor cells in Moyamoya disease: Current situation and controversial issues. Cell Transplant. 29:963689720913259. doi: 10.1177/0963689720913259
Zampetaki, A., Kirton, J. P., and Xu, Q. (2008). Vascular repair by endothelial progenitor cells. Cardiovasc. Res. 78, 413–421. doi: 10.1093/cvr/cvn081
Keywords: endothelial progenitor cells, cerebral aneurysm, endothelial dysfunction, endothelialization, endovascular therapy
Citation: Yu J, Du Q, Li X, Wei W, Fan Y, Zhang J and Chen J (2024) Potential role of endothelial progenitor cells in the pathogenesis and treatment of cerebral aneurysm. Front. Cell. Neurosci. 18:1456775. doi: 10.3389/fncel.2024.1456775
Received: 29 June 2024; Accepted: 30 July 2024;
Published: 12 August 2024.
Edited by:
Julie Ahn, George Washington University, United StatesReviewed by:
Ravi Philip Rajkumar, Jawaharlal Institute of Postgraduate Medical Education and Research (JIPMER), IndiaKeith David Rochfort, Dublin City University, Ireland
Copyright © 2024 Yu, Du, Li, Wei, Fan, Zhang and Chen. This is an open-access article distributed under the terms of the Creative Commons Attribution License (CC BY). The use, distribution or reproduction in other forums is permitted, provided the original author(s) and the copyright owner(s) are credited and that the original publication in this journal is cited, in accordance with accepted academic practice. No use, distribution or reproduction is permitted which does not comply with these terms.
*Correspondence: Jincao Chen, Y2hlbmpjMjAyMEAxMjYuY29t; Jianjian Zhang, emo1NzQ3MEAxMjYuY29t
†These authors have contributed equally to this work and share first authorship