- 1Department of Neurology, Medical Center ˗ University of Freiburg, Freiburg, Germany
- 2Faculty of Medicine, University of Freiburg, Freiburg, Germany
- 3Faculty of Biology, University of Freiburg, Freiburg, Germany
Microglia, the resident immune cells of the central nervous system, play a crucial role in regulating adult neurogenesis and contribute significantly to the pathogenesis of Alzheimer’s disease (AD). Under physiological conditions, microglia support and modulate neurogenesis through the secretion of neurotrophic factors, phagocytosis of apoptotic cells, and synaptic pruning, thereby promoting the proliferation, differentiation, and survival of neural progenitor cells (NPCs). However, in AD, microglial function becomes dysregulated, leading to chronic neuroinflammation and impaired neurogenesis. This review explores the intricate interplay between microglia and adult neurogenesis in health and AD, synthesizing recent findings to provide a comprehensive overview of the current understanding of microglia-mediated regulation of adult neurogenesis. Furthermore, it highlights the potential of microglia-targeted therapies to modulate neurogenesis and offers insights into potential avenues for developing novel therapeutic interventions.
1 Microglia and adult neurogenesis
Microglia are the resident immune cells of the central nervous system (CNS), originating from primitive myeloid progenitors of the yolk sac, that migrate into the CNS during early embryonic development (Ginhoux et al., 2010; Kierdorf et al., 2013). Within the brain, these immature macrophages proliferate and differentiate into microglia, maintaining themselves exclusively through self-renewal under normal physiological conditions (Ginhoux et al., 2013). Traditionally viewed as the brain’s innate immune cells, microglia monitor the brain microenvironment and adopt a reactive inflammatory phenotype under harmful stimuli or pathological conditions (Ransohoff and Perry, 2009; Prinz and Priller, 2014; Meyer-Luehmann and Prinz, 2015), our understanding of microglia has expanded greatly in the last decade.
It is now firmly established that new neurons continue to be generated post-developmentally in specific neurogenic brain regions, namely the subventricular zone (SVZ) of the lateral ventricles and subgranular zone (SGZ) of the dentate gyrus in the hippocampus throughout adulthood in the mammalian brain, a process called adult neurogenesis (Altman and Das, 1965; Altman, 1969; Gage, 2000). The process of generating new neurons consists of several phases including proliferation, migration, differentiation, and survival of neurons. Neurogenesis originates from neural stem cells (NSCs) that give rise to neural progenitor cells (NPCs), which generate neuroblasts that differentiate into functional neurons and integrate into existing neural circuits (for review see Ming and Song, 2011). In the SGZ of the hippocampus, immature neurons migrate into the granule cell layer and differentiate into dentate granule cells, where they are functionally integrated into the hippocampal circuitry (van Praag et al., 2002), while cells in the SVZ migrate through the rostral migratory stream to the olfactory bulb (Gage, 2000).
Microglia are an essential component of the neurogenic niche, as they appear densely populated in the SVZ (Mosher et al., 2012), and exhibit a different heterogeneity, as microglia activation differs in neurogenic regions compared to non-neurogenic brain regions (Goings et al., 2006). Emerging evidence shows a fundamental role of microglia in various stages of adult neurogenesis under physiological conditions, including neuronal proliferation, differentiation, migration, survival and integration of newborn neurons into the pre-existing neuronal network (Aarum et al., 2003; Monje et al., 2003; Butovsky et al., 2006; Ziv et al., 2006; Choi et al., 2008; Sierra et al., 2010; Bachstetter et al., 2011; Vukovic et al., 2012; Reshef et al., 2014). Despite recognizing microglia’s influential role in neurogenesis, the field is only beginning to elucidate the intricate cellular and molecular interplay that facilitate this regulation.
Depletion of microglia in the adult mouse brain impaired the survival and migration of newly generated neuroblasts in the adult hippocampus and SVZ (Ribeiro Xavier et al., 2015; Kreisel et al., 2019). In contradiction, Kyle et al. (2019) found no involvement of microglia in adult SVZ neurogenesis. However, microglial ablation was further shown to reduce dendritic spine elimination, spine density and disrupt normal functional development of adult-born neurons in the olfactory bulb (Reshef et al., 2017).
The pruning of newly generated cells during their initial period of survival is a key mechanism by which microglia modulate hippocampal neurogenesis. Markedly, only small fractions of generated cells survive and join the hippocampal circuitry as mature neurons (Kempermann et al., 2003). Sierra et al. (2010) demonstrated that ramified microglia have an important function in effectively engulfing and clearing apoptotic cells through phagocytosis during the first days of their life in an immunologically silent process (i.e., without inflammation). Additionally, microglia have been reported to prune weak synaptic neuronal connections on mature neurons by phagocytosis, refining neural circuits and supporting functional integration of adult-born neurons (Stevens et al., 2007; Sierra et al., 2010; Tremblay et al., 2011; Schafer et al., 2012).
Through the secretion of various soluble factors, including cytokines, trophic factors, and growth factors, microglia support and modulate neurogenesis (Elkabes et al., 1996; Butovsky et al., 2006; Shigemoto-Mogami et al., 2014). Several studies have proposed that microglia-derived factors influence neurogenesis through various mechanisms, including: (1) determining the neuronal phenotype through instructive signaling, (2) promoting neural progenitor proliferation via secreted neurotrophic factors, and (3) regulating the survival and circuit integration of newborn neurons through the production of specific factors (Gemma and Bachstetter, 2013). Several in vitro studies show that microglia can guide precursor cell differentiation toward a neuronal phenotype, and support NPC migration and neuronal survival (Aarum et al., 2003; Morgan et al., 2004; Walton et al., 2006), through secreted factors like IGF-1 and BDNF. These factors aid NSC proliferation, NPC differentiation, migration, and newborn cell survival (O’Kusky et al., 2000; Aberg et al., 2003; Morgan et al., 2004; Scharfman et al., 2005; Choi et al., 2008; Li et al., 2008; Yuan et al., 2015). Co-culturing NPCs with microglia stimulated by IL-4, IL-10 or TGF-β increases new cell survival, proliferation, and/or neuronal differentiation (Battista et al., 2006; Butovsky et al., 2006; Kiyota et al., 2012; Matsui and Mori, 2018). Furthermore, pharmacological suppression of microglial activation inhibited neurogenesis and oligodendrogenesis by decreasing pro-inflammatory mediators like IL-1β, TNF-α, and IFN-γ (Shigemoto-Mogami et al., 2014) in a concentration-dependent manner (Butovsky et al., 2006; Bernardino et al., 2008).
Physical exercise and environmental enrichment (EE) can stimulate hippocampal neurogenesis (Kempermann et al., 1997; van Praag et al., 1999; Brown et al., 2003), potentially by modulating microglial activation and promoting a pro-neurogenic phenotype (Vukovic et al., 2012). This effect may involve increased expression of neurotrophic factors like BDNF and IGF-1 by microglia in the dentate gyrus (Kohman et al., 2012; Littlefield et al., 2015). However, some studies have reported conflicting results regarding the correlation between microglia and exercise-induced neurogenesis (Olah et al., 2009; Gebara et al., 2013).
The microglia–neuron crosstalk mediated by the CX3CL1-CX3CR1 signaling pathway has been critically implicated in regulating adult neurogenesis (reviewed in Al-Onaizi et al., 2020). Disruption of this pathway by genetic deletion or pharmacological antagonism of CX3CR1 in young adult rodents suppressed hippocampal neurogenesis, impaired synaptic integration, changed neuronal morphology and functionality (Bolós et al., 2018) and impaired cognitive functions and long-term potentiation (LTP) induction, potentially through the involvement of IL-1β (Bachstetter et al., 2011; Rogers et al., 2011; Vukovic et al., 2012; Reshef et al., 2014). Further, the aforementioned exercise-induced NPC proliferation changes in microglia are dependent on CX3CL1–CX3CR1 signaling (Vukovic et al., 2012).
2 Microglia dysregulation in Alzheimer’s disease
Alzheimer’s disease (AD) is a complex neurodegenerative disorder characterized by progressive cognitive decline. The neuropathological hallmarks of AD are extracellular amyloid-β (Aβ) plaques and intraneuronal neurofibrillary tangles (NFTs) composed of hyperphosphorylated tau protein (pTau), culminating in synaptic dysfunction, neuronal loss and brain atrophy (Duyckaerts et al., 2009; Selkoe, 2011). Aβ plaques generally appear early in disease before clinical symptoms occur, prompting the widely articulated “amyloid cascade hypothesis” (Hardy and Higgins, 1992). The imbalance between Aβ production and clearance, resulting in increased extracellular Aβ plaque deposition, is believed to be the principal pathogenic mechanism (Selkoe and Hardy, 2016).
Recent research has implicated microglia as key cellular players modulating neuroinflammation, neurodegeneration, and cognitive deficits in AD (Muzio et al., 2021). Loss of microglial homeostatic functions may significantly impact disease progression. Genome-wide association studies (GWAS) identified most AD risk genes being highly or exclusively expressed in microglia, suggesting microglial dysfunction contributes to AD development (Lambert et al., 2013; Jones et al., 2015; Villegas-Llerena et al., 2016; Wightman et al., 2021). These include genes implicated in immune functions like APOE, TREM2, PLD3, CD33, and others involved in the innate immune response, phagocytosis, and lipid metabolism (Efthymiou and Goate, 2017; Hansen et al., 2017; Verheijen and Sleegers, 2018).
During homeostasis, ramified microglia dynamically extend and retract processes to monitor the brain parenchyma (Nimmerjahn et al., 2005). Upon insults, microglia become activated, undergoing complex morphological and functional transformations to maintain homeostasis. Single-cell transcriptomic analyses revealed remarkable microglial heterogeneity in AD, challenging the traditional dichotomous classification of M1 (pro-inflammatory) and M2 (anti-inflammatory) phenotypes (Keren-Shaul et al., 2017; Friedman et al., 2018; Gerrits et al., 2021). Notably, a distinct microglial subpopulation termed “disease-associated microglia” (DAM) has been identified in both mouse models and human AD brains, exhibiting a unique transcriptional signature (Keren-Shaul et al., 2017; Mathys et al., 2017). Additionally, “dark microglia” or “microglial neurodegenerative” (MGnD) phenotypes were described (Bisht et al., 2016; Krasemann et al., 2017) that associate with Aβ plaques and show decreased expressions of homeostatic microglia marker genes.
This microglial association with dense-core Aβ plaques, but not diffuse plaques, has been observed in both AD patients and transgenic mouse models (Perlmutter et al., 1990; Frautschy et al., 1998; Stalder et al., 1999; Meyer-Luehmann et al., 2008). Microglia possess a range of pattern recognition receptors (PRRs; Khoury et al., 1996; Venegas and Heneka, 2017) that detect Aβ, thereby influencing the microglial phenotype and triggering an inflammatory response (Salminen et al., 2009). Microglia activation induces morphological alterations and functional remodeling, transitioning from a ramified to an amoeboid, activated state (Kettenmann et al., 2011). In response to lesions or infections, reactive microglia migrate to the affected areas and undergo mitotic proliferation (Kettenmann et al., 2011; Heneka et al., 2015). Additionally, microglia can initiate receptor-mediated uptake and lysosomal degradation of Aβ, as well as produce various Aβ-degrading enzymes (Heneka, 2017). Aβ stimulates a pathway dependent on nuclear factor-kappa B (NF-B), which subsequently activates the production of inflammatory mediators and proinflammatory cytokine secretion, including IL-1β, TNF-α, and IL-6, as well as ROS and NO (Combs et al., 2001; Walker et al., 2001; Ho et al., 2005). Post-mortem brain tissues from patients suffering from AD show increased production of pro-inflammatory cytokines, particularly in the vicinity of amyloid plaques (Griffin et al., 1995).
One of the primary microglial functions in AD is Aβ clearance through phagocytosis (Hickman et al., 2008). The microglial surface receptor TREM2 is critical for this process, as it binds to Aβ-lipoprotein complexes (Wang et al., 2015) and promotes the conversion of microglia to the DAM phenotype, responsible for Aβ phagocytosis (Keren-Shaul et al., 2017). Loss of TREM2 impairs Aβ phagocytosis, leading to increased amyloid seeding in AD mouse models (Wang et al., 2015; Parhizkar et al., 2019). This clearance capacity appears impaired in later stages of AD, potentially due to age-related alterations or the overwhelming accumulation of Aβ (El Khoury et al., 2007; Hickman et al., 2008). Notably, microglia also form a protective barrier around Aβ deposits, compacting amyloid fibrils into a tightly packed core and potentially limiting neurotoxicity. This “corralling” function appears to be more effective for small, early-stage plaques and is compromised in the absence of TREM2 (Condello et al., 2015; Yuan et al., 2016). Furthermore, TREM2 has been implicated in controlling the physiological process of microglia-mediated synapse elimination during synaptic pruning (Filipello et al., 2018; Tagliatti et al., 2024). It enhances microglial phagocytic efficiency in clearing damaged synapses around Aβ plaques (Fracassi et al., 2023), by activating signal transduction pathways that promote microglial chemotaxis, phagocytosis, and survival (Konishi and Kiyama, 2018), offering protection against Aβ toxicity and limiting spreading of damage in AD models (Jiang et al., 2018; Lee et al., 2018).
The neuron–microglia signaling unit involving fractalkine (CX3CL1) and its receptor CX3CR1 regulates microglial inflammation in neurodegenerative diseases (Cardona et al., 2006; Bhaskar et al., 2010). CX3CR1 deficiency reduced Aβ load but worsened neuronal and behavioral deficits in a plaque-independent manner in AD mice (Bhaskar et al., 2010; Lee et al., 2010). Furthermore, depletion with CSF1R inhibitor reduced Aβ plaque compaction and increased diffuse plaques and dystrophic neurites in AD mouse models (Spangenberg et al., 2016; Casali et al., 2020). Interestingly, microglia may also contribute to Aβ propagation of amyloid pathology by facilitating the spreading of Aβ in an CX3CR1 dependent manner (d’Errico et al., 2022). These findings highlight the complex role of microglia and fractalkine signaling in the pathogenesis of AD.
Microglia exhibit a double-edged role in AD pathogenesis. Initially, they exert neuroprotective functions by clearing Aβ protein aggregates, producing neurotrophic factors, and forming physical barriers around plaques. These functions reduce neurotoxicity, limit plaque growth, while simultaneously delaying disease progression and onset of AD symptoms. As AD progresses, sustained microglial activation induced by Aβ leads to a detrimental pro-inflammatory state. Growing evidence indicates that chronic neuroinflammation, mediated by activated microglia secreting neurotoxic cytokines and inflammatory mediators, causes a decline in microglial homeostatic functions (Sarlus and Heneka, 2017), synaptic toxicity and consequently synapse loss and neuronal injury (Hong et al., 2016). Microglia are critically involved in parenchymal and vascular plaque formation, growth, and compaction, as well as influencing neuronal gene expression and mitigating neuritic dystrophy. Their ability to internalize and deposit aggregated Aβ is vital for the initial plaque development and progression, highlighting their complex involvement in AD (Spangenberg et al., 2019). Furthermore, microglia can promote Aβ pathology by stimulating neurons to overproduce Aβ, leading to a self-perpetuating cycle of neuroinflammation and neurodegeneration. Moreover, chronic stimulation of microglia by pathological Aβ deposits can cause these initially protective microglia to transform into a dysfunctional phenotype, thereby further aggravating AD progression.
Importantly, age is a critical factor influencing microglial function in AD. Senescence in microglia is characterized by changes in density, activation state, morphology, lipofuscin accumulation, phenotype, cytokine expression, and phagocytic capacity (Perry et al., 1993; Hart et al., 2012; Li et al., 2023; Stillman et al., 2023), contributing to persistent inflammation and Aβ pathology (Michelucci et al., 2009).
Taken together, microglia exhibit remarkable diversity and adaptability. Unraveling microglia’s multifaceted roles, both in health and across AD progression, is crucial for understanding the disease’s underlying mechanisms.
3 Interplay between microglia, adult neurogenesis and Alzheimer’s disease
Our comprehension of the role of adult neurogenesis in learning and memory among healthy individuals is still evolving, and we have yet to fully understand how impaired neurogenesis contributes to cognitive decline in aging and AD. The dentate gyrus of the hippocampus, a brain region critical for pattern separation, emotional memory, cognitive flexibility, learning and memory formation, exhibits adult neurogenesis (Lazarov and Hollands, 2016).
In AD, the hippocampus is among the earliest and most severely affected brain regions (Selkoe, 2011), with progressive memory impairment, associated with hippocampal degeneration (Thompson et al., 2004). Recent evidence suggests that impaired adult neurogenesis in the SVZ and SGZ, caused by intracellular accumulation of Aβ oligomers, may constitute an early event in AD pathogenesis, actively contributing to disease progression (Scopa et al., 2020). However, whether Aβ directly impairs neurogenesis and the hippocampal circuitry involved in memory formation, or whether altered neurogenesis is a byproduct of AD pathology that contributes insignificantly to the AD phenotype and cognitive dysfunction, remains debated (Hollands et al., 2016; Li Puma et al., 2021). Considerable discrepancies exist regarding whether neurogenesis is enhanced or repressed in AD (Mu and Gage, 2011; Sierra et al., 2011), likely stemming from varying approaches, markers, tissue preparation, and disease progression effects on neurogenesis, or AD’s impact on neuronal maturation stages. A valid hypothesis is that the early overstimulation of neurogenesis as a compensatory mechanism by Aβ causes a depletion of the stem cell pool and a decline in neurogenesis. Over time, as senile plaques form, the balance may shift toward neurotoxic, fibrillar Aβ, which further exacerbates the neurogenesis rate (Kent et al., 2020). More recent studies have confirmed impaired adult hippocampal neurogenesis (AHN) in AD and cognitively impaired patients (Moreno-Jiménez et al., 2019; Tobin et al., 2019), as detected by single-nucleus RNA sequencing (snRNA-seq; Zhou et al., 2022). The number of NPCs, neuroblasts and adult-born neurons progressively declined with advancing Braak stages in AD patients, preceding tangle and plaque formation (Moreno-Jiménez et al., 2019).
Similar to human studies, contradictory conclusions from varying mouse models have hindered a clear understanding of neurogenesis in AD. Furthermore, the stage at which neurogenesis is impaired varies, with certain models showing defects exclusively during the maturation stage (Hollands et al., 2017). Comprehensive summaries of the results can be found elsewhere (Babcock et al., 2021; Choi and Tanzi, 2023). Briefly, increased neurogenesis was exhibited in APPsw transgenic (Jin et al., 2004) and APP23 mice (Ermini et al., 2008), while presenilin-1 (PS-1) overexpression models and APP/PS-1 double transgenic mice exhibited varying neurogenesis patterns, with both increases and decreases depending on age and disease progression (Chevallier et al., 2005; Sotthibundhu et al., 2009; Demars et al., 2010; Biscaro et al., 2012; Zeng et al., 2016). However, mounting evidence from studies including 3xTg (Rodríguez et al., 2008; Hamilton et al., 2010), 5xFAD (Moon et al., 2014; Ziegler-Waldkirch et al., 2018b), and several APP overexpression models, supports alterations in AHN. In vivo and in vitro studies showed Aβ contribute to AHN impairment in AD (for review Culig et al., 2022; Farioli-Vecchioli et al., 2022).
Severe impairment of AHN and/or maturation of newborn neurons in early AD stages, occurring before memory impairment, has been observed. Interestingly, stimulating neurogenesis rescues cognitive deficits (Choi et al., 2018; Ziegler-Waldkirch et al., 2018b), suggesting impaired adult neurogenesis might contribute to AD-associated cognitive dysfunction.
Ablation of adult neurogenesis in AD transgenic mice substantially reduced the number of adult-born neurons and induced cognitive deficits by compromising hippocampal functions (Hollands et al., 2017; Choi et al., 2018; Zhang et al., 2021). Conversely, stimulating neurogenesis at the dentate gyrus through genetic, pharmacological, EE, or physical exercise rescues cognitive deficits in transgenic 5 × FAD and 3xTg mice, reduces amyloid burden (Valero et al., 2011; Sun et al., 2018; Ziegler-Waldkirch et al., 2018b; Kim et al., 2019), and simultaneously increases BDNF levels (Choi et al., 2018). Finally, EE increases progenitor proliferation, survival, differentiation, and dendritic arborization (Mirochnic et al., 2009; Valero et al., 2011).
Given microglia’s crucial role in regulating adult neurogenesis and their involvement in the inflammatory response associated with neurodegenerative diseases, a pertinent question arises: How do microglia modulate adult neurogenesis in the context of AD? Microglia are activated in the AD brain, causing inflammation and altering their effects on neurogenesis. During chronic stress, aging, and neurodegenerative diseases, microglia exhibit a pro-inflammatory phenotype, which can compromise the neurogenic cascade by releasing neurotoxic pro-inflammatory cytokines like IL-1β, IL-6, IL-17, and TNFα (Vallières et al., 2002; Zou and Crews, 2012; Wu et al., 2013; Liu et al., 2014). Evidence links microglia to facilitating AD pathology, as IGF1 expression, implicated in regulating neurogenesis, increases in APP/PS1 mice along with increased microglial activation and reduced SGZ neurogenesis (Myhre et al., 2019). Furthermore, reducing TGFβ in another AD mouse model accelerated neurodegeneration and AD-like pathology (Tesseur et al., 2006). Administration of minocycline, a microglia activation inhibitor, improved hippocampal-dependent learning and increased dentate granule cell survival in APP/PS1 mice, concomitantly decreasing inflammatory cytokines and microglial cells. Hence, the activated and inflammatory microglial phenotype causes neurogenetic and cognitive decay. However, Aβ levels or Aβ-related morphological deficits remained unaffected (Biscaro et al., 2012). Repopulation of microglia after CSF1R inhibitor cessation reverses AD-associated cognitive deficits, dysregulated neurotrophic signaling pathway and hippocampal neurogenesis by restoring BDNF expression in microglia in 5xFAD mice (Wang et al., 2023).
In parallel, physical exercise attenuates the age-dependent aberrant microglia activation in an AD model (Nichol et al., 2008) and reduced hyper-activated microglia in aged mice, increasing their pro-neurogenic phenotype by upregulating IGF1 (Kohman et al., 2012). Environmental enrichment reduced Aβ plaque load by activating phagocytic microglia in 5xFAD mice, reviving adult hippocampal neurogenesis and rescuing cognitive deficits (Ziegler-Waldkirch et al., 2018a,b). Additionally, EE reversed Aβ seeding-induced olfactory deficits in the olfactory bulb of 5xFAD mice (Ziegler-Waldkirch et al., 2022).
Notably, deficits in EE-dependent AHN observed in an AD model mouse expressing PS1 were completely restored upon microglia depletion via CSF1R antagonist (Ortega-Martinez et al., 2019). Impairment of CX3CR1 in AD disrupts hippocampal neurogenesis and learning due to increased IL-1β (Parkitny and Maletic-Savatic, 2021). In this regard, CX3CR1 depletion in AD models has been shown to mitigate AD-associated pathology by enhancing microglial phagocytosis (Lee et al., 2010; Liu et al., 2010). Amyloid pathology accelerates microglial senescence, progressively impairing their phagocytic capacity as degeneration increases (Flanary et al., 2007; Miller and Streit, 2007). Consequently, as microglial phagocytosis maintains the neurogenic niche, diminished phagocytic capacity of senescent microglia likely dysregulates the niche environment, suppressing AHN due to impaired clearance of Aβ and other factors disrupting niche homeostasis.
Of note, during physiological aging, both rodents and humans experience a significant decline in adult neurogenesis within the neurogenic niches (Kuhn et al., 1996; Kase et al., 2020). In addition, microglia undergo transcriptional and morphological changes with age, adopting a pro-inflammatory phenotype that is associated with impairments in neurogenesis and synaptic plasticity (Walton et al., 2006; Kohman et al., 2012; Vukovic et al., 2012; Gebara et al., 2013; Solano Fonseca et al., 2016). Studies have shown that microglia in aged mice, when depleted, promote the proliferation and maturation of NSC, indicating a potential anti-neurogenic role of microglia in the aging brain (Vukovic et al., 2012; Elmore et al., 2018). Thus, age-related alterations in microglial signatures may have additive derogatory effects on neurogenesis in the aged AD brain, exacerbating the decline in brain function over time.
In summary, activated microglia in AD impair multiple processes of neurogenesis (Figure 1). Further studies are needed to elucidate how microglial control of neurogenesis is influenced by AD pathological hallmarks.
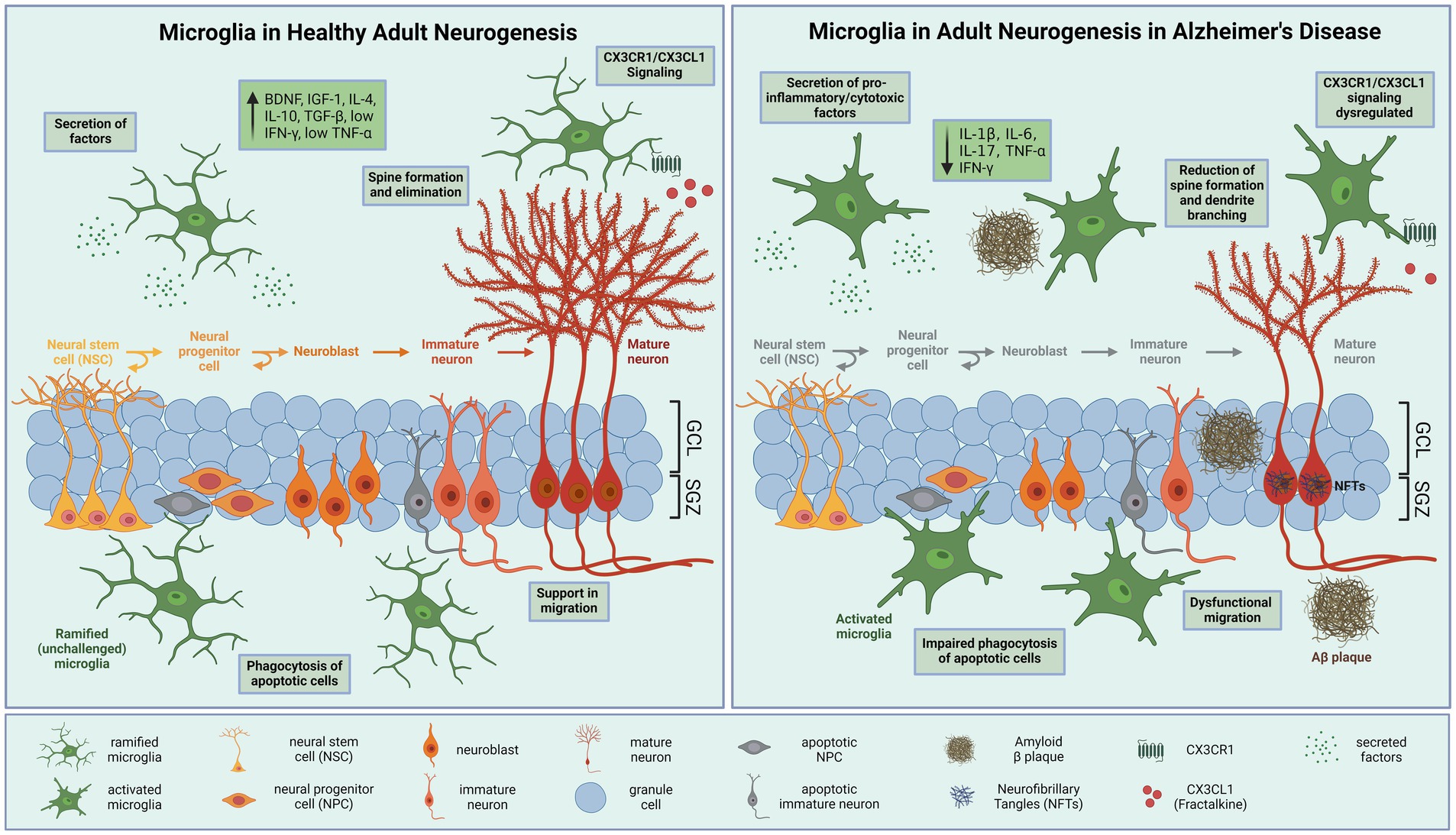
Figure 1. Microglial modulation of hippocampal neurogenesis in health and disease. (A) During physiological conditions, neural stem cells (NSCs) in the hippocampal subgranular zone (SGZ) produce neural progenitor cells (NPCs), which differentiate into neuroblasts and migrate into the granule cell layer (GCL), where the cells mature and integrate into the hippocampal neural circuitry. Ramified microglia effectively eliminate excess apoptotic newborn cells by phagocytosis. Secretion of neurotrophic factors effects proliferation, differentiation, and survival of neurons. In addition, microglia prune synapses and induce spine formation to support adult neurogenesis. Furthermore, microglia communicate with neurons through CXCR1/CX3CL1 signaling, which contribute to the ability of microglia to maintain a ramified phenotype. (B) In Alzheimer’s disease neurogenesis is reduced. Proliferation and differentiation of neurons are inhibited due to amyloid-β (Aβ) aggregation and neurofibrillary tangles (NFTs). Activated microglia adapt a pro-inflammatory phenotype releasing neurotoxic cytokines that impair neurogenesis and synaptic integrity, such as interleukin (IL)-1β, IL-6, IL-17 and tumor necrosis factor (TNF)-α. These cytokines have profound detrimental effects on adult neurogenesis by reducing proliferation, differentiation, survival, and integration of newborn neurons. Microglia display an impaired phagocytic activity and CX3CR1-CX3CL1 signaling. BDNF, brain-derived neurotrophic factor; IGF-1, insulin-like growth factor 1; IL-(1β,4,6,10,17), Interleukin-(1β,4,6,10,17); TGFβ, transforming growth factor β; IFN-γ, interferon-γ; TNF-α, tumor necrosis factor α. Created with BioRender.com.
4 Therapeutic targeting of microglia in Alzheimer’s disease
Malfunctioning of adult neurogenesis is considered a contributing factor to neurodegenerative diseases like AD, potentially leading to cognitive decline. Restoring or stimulating neurogenesis by increasing NSC proliferation in AD patients or individuals at high risk could provide a potential approach to prevent, delay, or counteract disease progression in the early stages of disease, particularly in terms of learning and memory impairments. Furthermore, interventions that improve or stimulate endogenous neurogenesis have been shown to decrease AD hallmarks, like Aβ accumulation and pTau. This indicates a potential bidirectional relationship: while Aβ and pTau impact neurogenesis, the molecular pathways governing neurogenesis may also influence Aβ clearance and tau phosphorylation (Lazarov et al., 2005; Biscaro et al., 2009; Choi et al., 2018; Ziegler-Waldkirch et al., 2018b; Kim et al., 2019). However, this strategy presents several crucial considerations. Highly promoted neurogenesis can deplete NSCs and cause early cessation of neurogenesis (Encinas et al., 2011; Sierra et al., 2015). Instead of boosting neuron production and proliferation in an already aged and Alzheimer’s-diseased brain, it may prove more beneficial to devise strategies aimed at preserving existing neurons and NPCs in presymptomatic individuals before age-related neuronal loss occurs. Additionally, a major challenge is the poor long-term survival of new neurons, likely due to the pathological and inflammatory environment. Therefore, exploring how microglia in different activation states regulate adult neurogenesis is highly warranted. Modulating microglial function and phenotype represents a promising approach to enhance adult neurogenesis and potentially alleviate cognitive deficits in AD. Restoring the homeostatic, neuroprotective microglial phenotype could normalize defective neurogenesis. Specific microglial subpopulations in the SVZ have been shown to promote adult neurogenesis, suggesting that targeting these populations could be therapeutically beneficial in AD (Ribeiro Xavier et al., 2015). Anti-inflammatory approaches skewing microglia toward an alternatively-activated, pro-neurogenic phenotype could enhance endogenous neurogenesis and potentially delay neurodegeneration (Varnum and Ikezu, 2012; Shohayeb et al., 2018; Muzio et al., 2021). For example, stimulating fractalkine CX3CL1/CX3CR1 signaling reduces neuroinflammation and may protect against age or disease-related neurogenic decline (Bachstetter et al., 2011). Furthermore, enhancing the neuroprotective, phagocytic phenotype of microglia could help clear pathological protein aggregates and reduce neuroinflammation. Clinical trials are ongoing to test microglia-targeted therapies like the CSF1R kinase inhibitors in AD (Martin-Estebane and Gomez-Nicola, 2020). Inhibiting CSF1R modulates microglial activation and has shown promising outcomes in animal models (Dagher et al., 2015; Spangenberg et al., 2016, 2019), particularly in regard to neurogenesis (Elmore et al., 2018). Genetic engineering of microglia is an emerging strategy being explored, including altering gene expression to reprogram microglia into a neuroprotective state for targeted delivery of therapeutics (Luo and Sugimura, 2024). However, technical limitations have prevented clinical translation so far. In summary, while still in early stages, modulating microglial phenotype and function through various approaches like small molecules, genetic engineering, or immunomodulation represents a promising avenue for developing novel neurorestorative therapies targeting neuroinflammation and neurogenesis deficits in neurodegenerative diseases like AD.
Author contributions
IF: Writing – original draft. MM-L: Conceptualization, Writing – review & editing.
Funding
The author(s) declare that financial support was received for the research, authorship, and/or publication of this article. We acknowledge support by the Open Access Publication Fund of the University of Freiburg.
Acknowledgments
We would like to thank Katrin Kierdorf for assistance with the graphical illustration.
Conflict of interest
The authors declare that the research was conducted in the absence of any commercial or financial relationships that could be construed as a potential conflict of interest.
Publisher’s note
All claims expressed in this article are solely those of the authors and do not necessarily represent those of their affiliated organizations, or those of the publisher, the editors and the reviewers. Any product that may be evaluated in this article, or claim that may be made by its manufacturer, is not guaranteed or endorsed by the publisher.
References
Aarum, J., Sandberg, K., Haeberlein, S. L. B., and Persson, M. A. A. (2003). Migration and differentiation of neural precursor cells can be directed by microglia. Proc. Natl. Acad. Sci. USA 100, 15983–15988. doi: 10.1073/pnas.2237050100
Aberg, M. A. I., Aberg, N. D., Palmer, T. D., Alborn, A.-M., Carlsson-Skwirut, C., Bang, P., et al. (2003). IGF-I has a direct proliferative effect in adult hippocampal progenitor cells. Mol. Cell. Neurosci. 24, 23–40. doi: 10.1016/s1044-7431(03)00082-4
Al-Onaizi, M., Al-Khalifah, A., Qasem, D., and ElAli, A. (2020). Role of microglia in modulating adult neurogenesis in health and neurodegeneration. Int. J. Mol. Sci. 21:6875. doi: 10.3390/ijms21186875
Altman, J. (1969). Autoradiographic and histological studies of postnatal neurogenesis. IV. Cell proliferation and migration in the anterior forebrain, with special reference to persisting neurogenesis in the olfactory bulb. J. Comp. Neurol. 137, 433–457. doi: 10.1002/cne.901370404
Altman, J., and Das, G. D. (1965). Autoradiographic and histological evidence of postnatal hippocampal neurogenesis in rats. J. Comp. Neurol. 124, 319–335. doi: 10.1002/cne.901240303
Babcock, K. R., Page, J. S., Fallon, J. R., and Webb, A. E. (2021). Adult hippocampal neurogenesis in aging and Alzheimer’s disease. Stem Cell Reports 16, 681–693. doi: 10.1016/j.stemcr.2021.01.019
Bachstetter, A. D., Morganti, J. M., Jernberg, J., Schlunk, A., Mitchell, S. H., Brewster, K. W., et al. (2011). Fractalkine and CX 3 CR1 regulate hippocampal neurogenesis in adult and aged rats. Neurobiol. Aging 32, 2030–2044. doi: 10.1016/j.neurobiolaging.2009.11.022
Battista, D., Ferrari, C. C., Gage, F. H., and Pitossi, F. J. (2006). Neurogenic niche modulation by activated microglia: transforming growth factor beta increases neurogenesis in the adult dentate gyrus. Eur. J. Neurosci. 23, 83–93. doi: 10.1111/j.1460-9568.2005.04539.x
Bernardino, L., Agasse, F., Silva, B., Ferreira, R., Grade, S., and Malva, J. O. (2008). Tumor necrosis factor-alpha modulates survival, proliferation, and neuronal differentiation in neonatal subventricular zone cell cultures. Stem Cells 26, 2361–2371. doi: 10.1634/stemcells.2007-0914
Bhaskar, K., Konerth, M., Kokiko-Cochran, O. N., Cardona, A., Ransohoff, R. M., and Lamb, B. T. (2010). Regulation of tau pathology by the microglial Fractalkine receptor. Neuron 68, 19–31. doi: 10.1016/j.neuron.2010.08.023
Biscaro, B., Lindvall, O., Hock, C., Ekdahl, C. T., and Nitsch, R. M. (2009). Aβ immunotherapy protects morphology and survival of adult-born neurons in doubly transgenic APP/PS1 mice. J. Neurosci. 29, 14108–14119. doi: 10.1523/JNEUROSCI.2055-09.2009
Biscaro, B., Lindvall, O., Tesco, G., Ekdahl, C. T., and Nitsch, R. M. (2012). Inhibition of microglial activation protects hippocampal neurogenesis and improves cognitive deficits in a transgenic mouse model for Alzheimer’s disease. Neurodegener. Dis. 9, 187–198. doi: 10.1159/000330363
Bisht, K., Sharma, K. P., Lecours, C., Gabriela Sánchez, M., El Hajj, H., Milior, G., et al. (2016). Dark microglia: a new phenotype predominantly associated with pathological states. Glia 64, 826–839. doi: 10.1002/glia.22966
Bolós, M., Perea, J. R., Terreros-Roncal, J., Pallas-Bazarra, N., Jurado-Arjona, J., Ávila, J., et al. (2018). Absence of microglial CX3CR1 impairs the synaptic integration of adult-born hippocampal granule neurons. Brain Behav. Immun. 68, 76–89. doi: 10.1016/j.bbi.2017.10.002
Brown, J., Cooper-Kuhn, C. M., Kempermann, G., Van Praag, H., Winkler, J., Gage, F. H., et al. (2003). Enriched environment and physical activity stimulate hippocampal but not olfactory bulb neurogenesis. Eur. J. Neurosci. 17, 2042–2046. doi: 10.1046/j.1460-9568.2003.02647.x
Butovsky, O., Ziv, Y., Schwartz, A., Landa, G., Talpalar, A. E., Pluchino, S., et al. (2006). Microglia activated by IL-4 or IFN-gamma differentially induce neurogenesis and oligodendrogenesis from adult stem/progenitor cells. Mol. Cell. Neurosci. 31, 149–160. doi: 10.1016/j.mcn.2005.10.006
Cardona, A. E., Pioro, E. P., Sasse, M. E., Kostenko, V., Cardona, S. M., Dijkstra, I. M., et al. (2006). Control of microglial neurotoxicity by the fractalkine receptor. Nat. Neurosci. 9, 917–924. doi: 10.1038/nn1715
Casali, B. T., MacPherson, K. P., Reed-Geaghan, E. G., and Landreth, G. E. (2020). Microglia depletion rapidly and reversibly alters amyloid pathology by modification of plaque compaction and morphologies. Neurobiol. Dis. 142:104956. doi: 10.1016/j.nbd.2020.104956
Chevallier, N. L., Soriano, S., Kang, D. E., Masliah, E., Hu, G., and Koo, E. H. (2005). Perturbed neurogenesis in the adult Hippocampus associated with Presenilin-1 A246E mutation. Am. J. Pathol. 167, 151–159. doi: 10.1016/S0002-9440(10)62962-8
Choi, S. H., Bylykbashi, E., Chatila, Z. K., Lee, S. W., Pulli, B., Clemenson, G. D., et al. (2018). Combined adult neurogenesis and BDNF mimic exercise effects on cognition in an Alzheimer’s mouse model. Science 361:eaan8821. doi: 10.1126/science.aan8821
Choi, Y.-S., Cho, H.-Y., Hoyt, K. R., Naegele, J. R., and Obrietan, K. (2008). IGF-1 receptor-mediated ERK/MAPK signaling couples status epilepticus to progenitor cell proliferation in the subgranular layer of the dentate gyrus. Glia 56, 791–800. doi: 10.1002/glia.20653
Choi, S. H., and Tanzi, R. E. (2023). Adult neurogenesis in Alzheimer’s disease. Hippocampus 33, 307–321. doi: 10.1002/hipo.23504
Combs, C. K., Karlo, J. C., Kao, S.-C., and Landreth, G. E. (2001). β-Amyloid stimulation of microglia and monocytes results in TNFα-dependent expression of inducible nitric oxide synthase and neuronal apoptosis. J. Neurosci. 21, 1179–1188. doi: 10.1523/JNEUROSCI.21-04-01179.2001
Condello, C., Yuan, P., Schain, A., and Grutzendler, J. (2015). Microglia constitute a barrier that prevents neurotoxic protofibrillar Aβ42 hotspots around plaques. Nat. Commun. 6:6176. doi: 10.1038/ncomms7176
Culig, L., Chu, X., and Bohr, V. A. (2022). Neurogenesis in aging and age-related neurodegenerative diseases. Ageing Res. Rev. 78:101636. doi: 10.1016/j.arr.2022.101636
D’Errico, P., Ziegler-Waldkirch, S., Aires, V., Hoffmann, P., Mezö, C., Erny, D., et al. (2022). Microglia contribute to the propagation of Aβ into unaffected brain tissue. Nat. Neurosci. 25, 20–25. doi: 10.1038/s41593-021-00951-0
Dagher, N. N., Najafi, A. R., Kayala, K. M. N., Elmore, M. R. P., White, T. E., Medeiros, R., et al. (2015). Colony-stimulating factor 1 receptor inhibition prevents microglial plaque association and improves cognition in 3xTg-AD mice. J. Neuroinflammation 12:139. doi: 10.1186/s12974-015-0366-9
Demars, M., Hu, Y.-S., Gadadhar, A., and Lazarov, O. (2010). Impaired neurogenesis is an early event in the etiology of familial Alzheimer’s disease in transgenic mice. J. Neurosci. Res. 88, 2103–2117. doi: 10.1002/jnr.22387
Duyckaerts, C., Delatour, B., and Potier, M.-C. (2009). Classification and basic pathology of Alzheimer disease. Acta Neuropathol. 118, 5–36. doi: 10.1007/s00401-009-0532-1
Efthymiou, A. G., and Goate, A. M. (2017). Late onset Alzheimer’s disease genetics implicates microglial pathways in disease risk. Mol. Neurodegener. 12:43. doi: 10.1186/s13024-017-0184-x
El Khoury, J., Toft, M., Hickman, S. E., Means, T. K., Terada, K., Geula, C., et al. (2007). Ccr2 deficiency impairs microglial accumulation and accelerates progression of Alzheimer-like disease. Nat. Med. 13, 432–438. doi: 10.1038/nm1555
Elkabes, S., DiCicco-Bloom, E. M., and Black, I. B. (1996). Brain microglia/macrophages express neurotrophins that selectively regulate microglial proliferation and function. J. Neurosci. 16, 2508–2521. doi: 10.1523/JNEUROSCI.16-08-02508.1996
Elmore, M. R. P., Hohsfield, L. A., Kramár, E. A., Soreq, L., Lee, R. J., Pham, S. T., et al. (2018). Replacement of microglia in the aged brain reverses cognitive, synaptic, and neuronal deficits in mice. Aging Cell 17:e12832. doi: 10.1111/acel.12832
Encinas, J. M., Michurina, T. V., Peunova, N., Park, J.-H., Tordo, J., Peterson, D. A., et al. (2011). Division-coupled astrocytic differentiation and age-related depletion of neural stem cells in the adult Hippocampus. Cell Stem Cell 8, 566–579. doi: 10.1016/j.stem.2011.03.010
Ermini, F. V., Grathwohl, S., Radde, R., Yamaguchi, M., Staufenbiel, M., Palmer, T. D., et al. (2008). Neurogenesis and alterations of neural stem cells in mouse models of cerebral amyloidosis. Am. J. Pathol. 172, 1520–1528. doi: 10.2353/ajpath.2008.060520
Farioli-Vecchioli, S., Ricci, V., and Middei, S. (2022, 2022). Adult hippocampal neurogenesis in Alzheimer’s disease: an overview of human and animal studies with implications for therapeutic perspectives aimed at memory recovery. Neural Plast. 1, 9959044–9959018. doi: 10.1155/2022/9959044
Filipello, F., Morini, R., Corradini, I., Zerbi, V., Canzi, A., Michalski, B., et al. (2018). The microglial innate immune receptor TREM2 is required for synapse elimination and Normal brain connectivity. Immunity 48, 979–991.e8. doi: 10.1016/j.immuni.2018.04.016
Flanary, B. E., Sammons, N. W., Nguyen, C., Walker, D., and Streit, W. J. (2007). Evidence that aging and amyloid promote microglial cell senescence. Rejuvenation Res. 10, 61–74. doi: 10.1089/rej.2006.9096
Fracassi, A., Marcatti, M., Tumurbaatar, B., Woltjer, R., Moreno, S., and Taglialatela, G. (2023). TREM2-induced activation of microglia contributes to synaptic integrity in cognitively intact aged individuals with Alzheimer’s neuropathology. Brain Pathol. 33:e13108. doi: 10.1111/bpa.13108
Frautschy, S. A., Yang, F., Irrizarry, M., Hyman, B., Saido, T. C., Hsiao, K., et al. (1998). Microglial response to amyloid plaques in APPsw transgenic mice. Am. J. Pathol. 152, 307–317
Friedman, B. A., Srinivasan, K., Ayalon, G., Meilandt, W. J., Lin, H., Huntley, M. A., et al. (2018). Diverse brain myeloid expression profiles reveal distinct microglial activation states and aspects of Alzheimer’s disease not evident in mouse models. Cell Rep. 22, 832–847. doi: 10.1016/j.celrep.2017.12.066
Gage, F. H. (2000). Mammalian neural stem cells. Science 287, 1433–1438. doi: 10.1126/science.287.5457.1433
Gebara, E., Sultan, S., Kocher-Braissant, J., and Toni, N. (2013). Adult hippocampal neurogenesis inversely correlates with microglia in conditions of voluntary running and aging. Front. Neurosci. 7:145. doi: 10.3389/fnins.2013.00145
Gemma, C., and Bachstetter, A. D. (2013). The role of microglia in adult hippocampal neurogenesis. Front. Cell. Neurosci. 7:229. doi: 10.3389/fncel.2013.00229
Gerrits, E., Brouwer, N., Kooistra, S. M., Woodbury, M. E., Vermeiren, Y., Lambourne, M., et al. (2021). Distinct amyloid-β and tau-associated microglia profiles in Alzheimer’s disease. Acta Neuropathol. 141, 681–696. doi: 10.1007/s00401-021-02263-w
Ginhoux, F., Greter, M., Leboeuf, M., Nandi, S., See, P., Gokhan, S., et al. (2010). Fate mapping analysis reveals that adult microglia derive from primitive macrophages. Science 330, 841–845. doi: 10.1126/science.1194637
Ginhoux, F., Lim, S., Hoeffel, G., Low, D., and Huber, T. (2013). Origin and differentiation of microglia. Front. Cell. Neurosci. 7:45. doi: 10.3389/fncel.2013.00045
Goings, G. E., Kozlowski, D. A., and Szele, F. G. (2006). Differential activation of microglia in neurogenic versus non-neurogenic regions of the forebrain. Glia 54, 329–342. doi: 10.1002/glia.20381
Griffin, W. S. T., Sheng, J. G., Roberts, G. W., and Mrak, R. E. (1995). Interleukin-1 expression in different plaque types in Alzheimer’s disease: significance in plaque evolution. J. Neuropathol. Exp. Neurol. 54, 276–281.
Hamilton, L. K., Aumont, A., Julien, C., Vadnais, A., Calon, F., and Fernandes, K. J. L. (2010). Widespread deficits in adult neurogenesis precede plaque and tangle formation in the 3xTg mouse model of Alzheimer’s disease. Eur. J. Neurosci. 32, 905–920. doi: 10.1111/j.1460-9568.2010.07379.x
Hansen, D. V., Hanson, J. E., and Sheng, M. (2017). Microglia in Alzheimer’s disease. J. Cell Biol. 217, 459–472. doi: 10.1083/jcb.201709069
Hardy, J. A., and Higgins, G. A. (1992). Alzheimer’s disease: the amyloid cascade hypothesis. Science 256, 184–185. doi: 10.1126/science.1566067
Hart, A. D., Wyttenbach, A., Hugh Perry, V., and Teeling, J. L. (2012). Age related changes in microglial phenotype vary between CNS regions: Grey versus white matter differences. Brain Behav. Immun. 26, 754–765. doi: 10.1016/j.bbi.2011.11.006
Heneka, M. T. (2017). Inflammasome activation and innate immunity in Alzheimer’s disease. Brain Pathol. 27, 220–222. doi: 10.1111/bpa.12483
Heneka, M. T., Carson, M. J., Khoury, J. E., Landreth, G. E., Brosseron, F., Feinstein, D. L., et al. (2015). Neuroinflammation in Alzheimer’s disease. Lancet Neurol. 14, 388–405. doi: 10.1016/S1474-4422(15)70016-5
Hickman, S. E., Allison, E. K., and Khoury, J. E. (2008). Microglial dysfunction and defective β-amyloid clearance pathways in aging Alzheimer’s disease mice. J. Neurosci. 28, 8354–8360. doi: 10.1523/JNEUROSCI.0616-08.2008
Ho, G. J., Drego, R., Hakimian, E., and Masliah, E. (2005). Mechanisms of cell signaling and inflammation in Alzheimer’s disease. Current Drug Targets - Inflammation & Allergy 4, 247–256. doi: 10.2174/1568010053586237
Hollands, C., Bartolotti, N., and Lazarov, O. (2016). Alzheimer’s disease and hippocampal adult neurogenesis; exploring shared mechanisms. Front. Neurosci. 10:178. doi: 10.3389/fnins.2016.00178
Hollands, C., Tobin, M. K., Hsu, M., Musaraca, K., Yu, T.-S., Mishra, R., et al. (2017). Depletion of adult neurogenesis exacerbates cognitive deficits in Alzheimer’s disease by compromising hippocampal inhibition. Mol. Neurodegener. 12:64. doi: 10.1186/s13024-017-0207-7
Hong, S., Beja-Glasser, V. F., Nfonoyim, B. M., Frouin, A., Li, S., Ramakrishnan, S., et al. (2016). Complement and microglia mediate early synapse loss in Alzheimer mouse models. Science 352, 712–716. doi: 10.1126/science.aad8373
Jiang, T., Zhang, Y.-D., Gao, Q., Ou, Z., Gong, P.-Y., Shi, J.-Q., et al. (2018). TREM2 ameliorates neuronal tau pathology through suppression of microglial inflammatory response. Inflammation 41, 811–823. doi: 10.1007/s10753-018-0735-5
Jin, K., Galvan, V., Xie, L., Mao, X. O., Gorostiza, O. F., Bredesen, D. E., et al. (2004). Enhanced neurogenesis in Alzheimer’s disease transgenic (PDGF-APPSw,Ind) mice. Proc. Natl. Acad. Sci. 101, 13363–13367. doi: 10.1073/pnas.0403678101
Jones, L., Lambert, J.-C., Wang, L.-S., Choi, S.-H., and Harold, D.Consortium (IGAP), et al. (2015). Convergent genetic and expression data implicate immunity in Alzheimer’s disease. Alzheimers Dement. 11, 658–671. doi: 10.1016/j.jalz.2014.05.1757
Kase, Y., Shimazaki, T., and Okano, H. (2020). Current understanding of adult neurogenesis in the mammalian brain: how does adult neurogenesis decrease with age? Inflam. Regen. 40:10. doi: 10.1186/s41232-020-00122-x
Kempermann, G., Gast, D., Kronenberg, G., Yamaguchi, M., and Gage, F. H. (2003). Early determination and long-term persistence of adult-generated new neurons in the hippocampus of mice. Development 130, 391–399. doi: 10.1242/dev.00203
Kempermann, G., Kuhn, H. G., and Gage, F. H. (1997). More hippocampal neurons in adult mice living in an enriched environment. Nature 386, 493–495. doi: 10.1038/386493a0
Kent, S. A., Spires-Jones, T. L., and Durrant, C. S. (2020). The physiological roles of tau and Aβ: implications for Alzheimer’s disease pathology and therapeutics. Acta Neuropathol. 140, 417–447. doi: 10.1007/s00401-020-02196-w
Keren-Shaul, H., Spinrad, A., Weiner, A., Matcovitch-Natan, O., Dvir-Szternfeld, R., Ulland, T. K., et al. (2017). A unique microglia type associated with restricting development of Alzheimer’s disease. Cell 169, 1276–1290.e17. doi: 10.1016/j.cell.2017.05.018
Kettenmann, H., Hanisch, U.-K., Noda, M., and Verkhratsky, A. (2011). Physiology of microglia. Physiol. Rev. 91, 461–553. doi: 10.1152/physrev.00011.2010
Khoury, J. E., Hickman, S. E., Thomas, C. A., Cao, L., Silverstein, S. C., and Loike, J. D. (1996). Scavenger receptor-mediated adhesion of microglia to β-amyloid fibrils. Nature 382, 716–719. doi: 10.1038/382716a0
Kierdorf, K., Erny, D., Goldmann, T., Sander, V., Schulz, C., Perdiguero, E. G., et al. (2013). Microglia emerge from erythromyeloid precursors via Pu.1-and Irf8-dependent pathways. Nat. Neurosci. 16, 273–280. doi: 10.1038/nn.3318
Kim, D., Cho, J., and Kang, H. (2019). Protective effect of exercise training against the progression of Alzheimer’s disease in 3xTg-AD mice. Behav. Brain Res. 374:112105. doi: 10.1016/j.bbr.2019.112105
Kiyota, T., Ingraham, K. L., Swan, R. J., Jacobsen, M. T., Andrews, S. J., and Ikezu, T. (2012). AAV serotype 2/1-mediated gene delivery of anti-inflammatory interleukin-10 enhances neurogenesis and cognitive function in APP+PS1 mice. Gene Ther. 19, 724–733. doi: 10.1038/gt.2011.126
Kohman, R. A., DeYoung, E. K., Bhattacharya, T. K., Peterson, L. N., and Rhodes, J. S. (2012). Wheel running attenuates microglia proliferation and increases expression of a proneurogenic phenotype in the hippocampus of aged mice. Brain Behav. Immun. 26, 803–810. doi: 10.1016/j.bbi.2011.10.006
Konishi, H., and Kiyama, H. (2018). Microglial TREM2/DAP12 signaling: a double-edged sword in neural diseases. Front. Cell. Neurosci. 12:206. doi: 10.3389/fncel.2018.00206
Krasemann, S., Madore, C., Cialic, R., Baufeld, C., Calcagno, N., El Fatimy, R., et al. (2017). The TREM2-APOE pathway drives the transcriptional phenotype of dysfunctional microglia in neurodegenerative diseases. Immunity 47, 566–581.e9. doi: 10.1016/j.immuni.2017.08.008
Kreisel, T., Wolf, B., Keshet, E., and Licht, T. (2019). Unique role for dentate gyrus microglia in neuroblast survival and in VEGF-induced activation. Glia 67, 594–618. doi: 10.1002/glia.23505
Kuhn, H. G., Dickinson-Anson, H., and Gage, F. H. (1996). Neurogenesis in the dentate gyrus of the adult rat: age-related decrease of neuronal progenitor proliferation. J. Neurosci. 16, 2027–2033. doi: 10.1523/JNEUROSCI.16-06-02027.1996
Kyle, J., Wu, M., Gourzi, S., and Tsirka, S. E. (2019). Proliferation and differentiation in the adult subventricular zone are not affected by CSF1R inhibition. Front. Cell. Neurosci. 13:97. doi: 10.3389/fncel.2019.00097
Lambert, J.-C., Ibrahim-Verbaas, C. A., Harold, D., Naj, A. C., Sims, R., Bellenguez, C., et al. (2013). Meta-analysis of 74,046 individuals identifies 11 new susceptibility loci for Alzheimer’s disease. Nat. Genet. 45, 1452–1458. doi: 10.1038/ng.2802
Lazarov, O., and Hollands, C. (2016). Hippocampal neurogenesis: learning to remember. Prog. Neurobiol. 138-140, 1–18. doi: 10.1016/j.pneurobio.2015.12.006
Lazarov, O., Robinson, J., Tang, Y.-P., Hairston, I. S., Korade-Mirnics, Z., Lee, V. M.-Y., et al. (2005). Environmental enrichment reduces Aβ levels and amyloid deposition in transgenic mice. Cell 120, 701–713. doi: 10.1016/j.cell.2005.01.015
Lee, C. Y. D., Daggett, A., Gu, X., Jiang, L.-L., Langfelder, P., Li, X., et al. (2018). Elevated TREM2 gene dosage reprograms microglia responsivity and ameliorates pathological phenotypes in Alzheimer’s disease models. Neuron 97, 1032–1048.e5. doi: 10.1016/j.neuron.2018.02.002
Lee, S., Varvel, N. H., Konerth, M. E., Xu, G., Cardona, A. E., Ransohoff, R. M., et al. (2010). CX3CR1 deficiency alters microglial activation and reduces Beta-amyloid deposition in two Alzheimer’s disease mouse models. Am. J. Pathol. 177, 2549–2562. doi: 10.2353/ajpath.2010.100265
Li, X., Li, Y., Jin, Y., Zhang, Y., Wu, J., Xu, Z., et al. (2023). Transcriptional and epigenetic decoding of the microglial aging process. Nat Aging 3, 1288–1311. doi: 10.1038/s43587-023-00479-x
Li, Y., Luikart, B. W., Birnbaum, S., Chen, J., Kwon, C.-H., Kernie, S. G., et al. (2008). TrkB regulates hippocampal neurogenesis and governs sensitivity to antidepressive treatment. Neuron 59, 399–412. doi: 10.1016/j.neuron.2008.06.023
Li Puma, D. D., Piacentini, R., and Grassi, C. (2021). Does impairment of adult neurogenesis contribute to pathophysiology of Alzheimer’s disease? A still open question. Front. Mol. Neurosci. 13:578211. doi: 10.3389/fnmol.2020.578211
Littlefield, A. M., Setti, S. E., Priester, C., and Kohman, R. A. (2015). Voluntary exercise attenuates LPS-induced reductions in neurogenesis and increases microglia expression of a proneurogenic phenotype in aged mice. J. Neuroinflammation 12:138. doi: 10.1186/s12974-015-0362-0
Liu, Z., Condello, C., Schain, A., Harb, R., and Grutzendler, J. (2010). CX3CR1 in microglia regulates brain amyloid deposition through selective Protofibrillar amyloid-β phagocytosis. J. Neurosci. 30, 17091–17101. doi: 10.1523/JNEUROSCI.4403-10.2010
Liu, Q., Xin, W., He, P., Turner, D., Yin, J., Gan, Y., et al. (2014). Interleukin-17 inhibits adult hippocampal neurogenesis. Sci. Rep. 4:7554. doi: 10.1038/srep07554
Luo, E. Y., and Sugimura, R. R. (2024). Taming microglia: the promise of engineered microglia in treating neurological diseases. J. Neuroinflammation 21:19. doi: 10.1186/s12974-024-03015-9
Martin-Estebane, M., and Gomez-Nicola, D. (2020). Targeting microglial population dynamics in Alzheimer’s disease: are we ready for a potential impact on immune function? Front. Cell. Neurosci. 14:149. doi: 10.3389/fncel.2020.00149
Mathys, H., Adaikkan, C., Gao, F., Young, J. Z., Manet, E., Hemberg, M., et al. (2017). Temporal tracking of microglia activation in neurodegeneration at single-cell resolution. Cell Rep. 21, 366–380. doi: 10.1016/j.celrep.2017.09.039
Matsui, T. K., and Mori, E. (2018). Microglia support neural stem cell maintenance and growth. Biochem. Biophys. Res. Commun. 503, 1880–1884. doi: 10.1016/j.bbrc.2018.07.130
Meyer-Luehmann, M., and Prinz, M. (2015). Myeloid cells in Alzheimer’s disease: culprits, victims or innocent bystanders? Trends Neurosci. 38, 659–668. doi: 10.1016/j.tins.2015.08.011
Meyer-Luehmann, M., Spires-Jones, T. L., Prada, C., Garcia-Alloza, M., de Calignon, A., Rozkalne, A., et al. (2008). Rapid appearance and local toxicity of amyloid-beta plaques in a mouse model of Alzheimer’s disease. Nature 451, 720–724. doi: 10.1038/nature06616
Michelucci, A., Heurtaux, T., Grandbarbe, L., Morga, E., and Heuschling, P. (2009). Characterization of the microglial phenotype under specific pro-inflammatory and anti-inflammatory conditions: effects of oligomeric and fibrillar amyloid-β. J. Neuroimmunol. 210, 3–12. doi: 10.1016/j.jneuroim.2009.02.003
Miller, K. R., and Streit, W. J. (2007). The effects of aging, injury and disease on microglial function: a case for cellular senescence. Neuron Glia Biol. 3, 245–253. doi: 10.1017/S1740925X08000136
Ming, G.-L., and Song, H. (2011). Adult neurogenesis in the mammalian brain: significant answers and significant questions. Neuron 70, 687–702. doi: 10.1016/j.neuron.2011.05.001
Mirochnic, S., Wolf, S., Staufenbiel, M., and Kempermann, G. (2009). Age effects on the regulation of adult hippocampal neurogenesis by physical activity and environmental enrichment in the APP23 mouse model of Alzheimer disease. Hippocampus 19, 1008–1018. doi: 10.1002/hipo.20560
Monje, M. L., Toda, H., and Palmer, T. D. (2003). Inflammatory blockade restores adult hippocampal neurogenesis. Science 302, 1760–1765. doi: 10.1126/science.1088417
Moon, M., Cha, M.-Y., and Mook-Jung, I. (2014). Impaired hippocampal neurogenesis and its enhancement with ghrelin in 5XFAD mice. J. Alzheimers Dis. 41, 233–241. doi: 10.3233/JAD-132417
Moreno-Jiménez, E. P., Flor-García, M., Terreros-Roncal, J., Rábano, A., Cafini, F., Pallas-Bazarra, N., et al. (2019). Adult hippocampal neurogenesis is abundant in neurologically healthy subjects and drops sharply in patients with Alzheimer’s disease. Nat. Med. 25, 554–560. doi: 10.1038/s41591-019-0375-9
Morgan, S. C., Taylor, D. L., and Pocock, J. M. (2004). Microglia release activators of neuronal proliferation mediated by activation of mitogen-activated protein kinase, phosphatidylinositol-3-kinase/Akt and delta-notch signalling cascades. J. Neurochem. 90, 89–101. doi: 10.1111/j.1471-4159.2004.02461.x
Mosher, K. I., Andres, R. H., Fukuhara, T., Bieri, G., Hasegawa-Moriyama, M., He, Y., et al. (2012). Neural progenitor cells regulate microglia functions and activity. Nat. Neurosci. 15, 1485–1487. doi: 10.1038/nn.3233
Mu, Y., and Gage, F. H. (2011). Adult hippocampal neurogenesis and its role in Alzheimer’s disease. Mol. Neurodegener. 6:85. doi: 10.1186/1750-1326-6-85
Muzio, L., Viotti, A., and Martino, G. (2021). Microglia in Neuroinflammation and neurodegeneration: from understanding to therapy. Front. Neurosci. 15:742065. doi: 10.3389/fnins.2021.742065
Myhre, C. L., Thygesen, C., Villadsen, B., Vollerup, J., Ilkjær, L., Krohn, K. T., et al. (2019). Microglia express insulin-like growth Factor-1 in the Hippocampus of aged APPswe/PS1ΔE9 transgenic mice. Front. Cell. Neurosci. 13:308. doi: 10.3389/fncel.2019.00308
Nichol, K. E., Poon, W. W., Parachikova, A. I., Cribbs, D. H., Glabe, C. G., and Cotman, C. W. (2008). Exercise alters the immune profile in Tg2576 Alzheimer mice toward a response coincident with improved cognitive performance and decreased amyloid. J. Neuroinflammation 5:13. doi: 10.1186/1742-2094-5-13
Nimmerjahn, A., Kirchhoff, F., and Helmchen, F. (2005). Resting microglial cells are highly dynamic Surveillants of brain parenchyma in vivo. Science 308, 1314–1318. doi: 10.1126/science.1110647
O’Kusky, J. R., Ye, P., and D’Ercole, A. J. (2000). Insulin-like growth factor-I promotes neurogenesis and synaptogenesis in the hippocampal dentate gyrus during postnatal development. J. Neurosci. 20, 8435–8442. doi: 10.1523/JNEUROSCI.20-22-08435.2000
Olah, M., Ping, G., De Haas, A. H., Brouwer, N., Meerlo, P., Van Der Zee, E. A., et al. (2009). Enhanced hippocampal neurogenesis in the absence of microglia T cell interaction and microglia activation in the murine running wheel model. Glia 57, 1046–1061. doi: 10.1002/glia.20828
Ortega-Martinez, S., Palla, N., Zhang, X., Lipman, E., and Sisodia, S. S. (2019). Deficits in enrichment-dependent neurogenesis and enhanced anxiety behaviors mediated by expression of Alzheimer’s disease-linked Ps1 variants are rescued by microglial depletion. J. Neurosci. 39, 6766–6780. doi: 10.1523/JNEUROSCI.0884-19.2019
Parhizkar, S., Arzberger, T., Brendel, M., Kleinberger, G., Deussing, M., Focke, C., et al. (2019). Loss of TREM2 function increases amyloid seeding but reduces plaque-associated ApoE. Nat. Neurosci. 22, 191–204. doi: 10.1038/s41593-018-0296-9
Parkitny, L., and Maletic-Savatic, M. (2021). Glial PAMPering and DAMPening of adult hippocampal neurogenesis. Brain Sci. 11:1299. doi: 10.3390/brainsci11101299
Perlmutter, L. S., Barron, E., and Chui, H. C. (1990). Morphologic association between microglia and senile plaque amyloid in Alzheimer’s disease. Neurosci. Lett. 119, 32–36. doi: 10.1016/0304-3940(90)90748-X
Perry, V. H., Matyszak, M. K., and Fearn, S. (1993). Altered antigen expression of microglia in the aged rodent CNS. Glia 7, 60–67. doi: 10.1002/glia.440070111
Prinz, M., and Priller, J. (2014). Microglia and brain macrophages in the molecular age: from origin to neuropsychiatric disease. Nat. Rev. Neurosci. 15, 300–312. doi: 10.1038/nrn3722
Ransohoff, R. M., and Perry, V. H. (2009). Microglial physiology: unique stimuli, specialized responses. Annu. Rev. Immunol. 27, 119–145. doi: 10.1146/annurev.immunol.021908.132528
Reshef, R., Kreisel, T., Beroukhim Kay, D., and Yirmiya, R. (2014). Microglia and their CX3CR1 signaling are involved in hippocampal-but not olfactory bulb-related memory and neurogenesis. Brain Behav. Immun. 41, 239–250. doi: 10.1016/j.bbi.2014.04.009
Reshef, R., Kudryavitskaya, E., Shani-Narkiss, H., Isaacson, B., Rimmerman, N., Mizrahi, A., et al. (2017). The role of microglia and their CX3CR1 signaling in adult neurogenesis in the olfactory bulb. eLife 6:e30809. doi: 10.7554/eLife.30809
Ribeiro Xavier, A. L., Kress, B. T., Goldman, S. A., Lacerda de Menezes, J. R., and Nedergaard, M. (2015). A distinct population of microglia supports adult neurogenesis in the subventricular zone. J. Neurosci. 35, 11848–11861. doi: 10.1523/JNEUROSCI.1217-15.2015
Rodríguez, J. J., Jones, V. C., Tabuchi, M., Allan, S. M., Knight, E. M., LaFerla, F. M., et al. (2008). Impaired adult neurogenesis in the dentate gyrus of a triple transgenic mouse model of Alzheimer’s disease. PLoS One 3:e2935. doi: 10.1371/journal.pone.0002935
Rogers, J. T., Morganti, J. M., Bachstetter, A. D., Hudson, C. E., Peters, M. M., Grimmig, B. A., et al. (2011). CX3CR1 deficiency leads to impairment of hippocampal cognitive function and synaptic plasticity. J. Neurosci. 31, 16241–16250. doi: 10.1523/JNEUROSCI.3667-11.2011
Salminen, A., Ojala, J., Kauppinen, A., Kaarniranta, K., and Suuronen, T. (2009). Inflammation in Alzheimer’s disease: amyloid-β oligomers trigger innate immunity defence via pattern recognition receptors. Prog. Neurobiol. 87, 181–194. doi: 10.1016/j.pneurobio.2009.01.001
Sarlus, H., and Heneka, M. T. (2017). Microglia in Alzheimer’s disease. J. Clin. Invest. 127, 3240–3249. doi: 10.1172/JCI90606
Schafer, D. P., Lehrman, E. K., Kautzman, A. G., Koyama, R., Mardinly, A. R., Yamasaki, R., et al. (2012). Microglia sculpt postnatal neural circuits in an activity and complement-dependent manner. Neuron 74, 691–705. doi: 10.1016/j.neuron.2012.03.026
Scharfman, H., Goodman, J., Macleod, A., Phani, S., Antonelli, C., and Croll, S. (2005). Increased neurogenesis and the ectopic granule cells after intrahippocampal BDNF infusion in adult rats. Exp. Neurol. 192, 348–356. doi: 10.1016/j.expneurol.2004.11.016
Scopa, C., Marrocco, F., Latina, V., Ruggeri, F., Corvaglia, V., La Regina, F., et al. (2020). Impaired adult neurogenesis is an early event in Alzheimer’s disease neurodegeneration, mediated by intracellular Aβ oligomers. Cell Death Differ. 27, 934–948. doi: 10.1038/s41418-019-0409-3
Selkoe, D. J. (2011). Alzheimer’s Disease. Cold Spring Harb. Perspect. Biol. 3:a004457. doi: 10.1101/cshperspect.a004457
Selkoe, D. J., and Hardy, J. (2016). The amyloid hypothesis of Alzheimer’s disease at 25 years. EMBO Mol. Med. 8, 595–608. doi: 10.15252/emmm.201606210
Shigemoto-Mogami, Y., Hoshikawa, K., Goldman, J. E., Sekino, Y., and Sato, K. (2014). Microglia enhance neurogenesis and oligodendrogenesis in the early postnatal subventricular zone. J. Neurosci. 34, 2231–2243. doi: 10.1523/JNEUROSCI.1619-13.2014
Shohayeb, B., Diab, M., Ahmed, M., and Ng, D. C. H. (2018). Factors that influence adult neurogenesis as potential therapy. Transl Neurodegener 7:4. doi: 10.1186/s40035-018-0109-9
Sierra, A., Encinas, J. M., Deudero, J. J. P., Chancey, J. H., Enikolopov, G., Overstreet-Wadiche, L. S., et al. (2010). Microglia shape adult hippocampal neurogenesis through apoptosis-coupled phagocytosis. Cell Stem Cell 7, 483–495. doi: 10.1016/j.stem.2010.08.014
Sierra, A., Encinas, J. M., and Maletic-Savatic, M. (2011). Adult human neurogenesis: from microscopy to magnetic resonance imaging. Front. Neurosci. 5:47. doi: 10.3389/fnins.2011.00047
Sierra, A., Martín-Suárez, S., Valcárcel-Martín, R., Pascual-Brazo, J., Aelvoet, S.-A., Abiega, O., et al. (2015). Neuronal hyperactivity accelerates depletion of neural stem cells and impairs hippocampal neurogenesis. Cell Stem Cell 16, 488–503. doi: 10.1016/j.stem.2015.04.003
Solano Fonseca, R., Mahesula, S., Apple, D. M., Raghunathan, R., Dugan, A., Cardona, A., et al. (2016). Neurogenic niche microglia undergo positional remodeling and progressive activation contributing to age-associated reductions in neurogenesis. Stem Cells Dev. 25, 542–555. doi: 10.1089/scd.2015.0319
Sotthibundhu, A., Li, Q.-X., Thangnipon, W., and Coulson, E. J. (2009). Aβ1–42 stimulates adult SVZ neurogenesis through the p75 neurotrophin receptor. Neurobiol. Aging 30, 1975–1985. doi: 10.1016/j.neurobiolaging.2008.02.004
Spangenberg, E. E., Lee, R. J., Najafi, A. R., Rice, R. A., Elmore, M. R. P., Blurton-Jones, M., et al. (2016). Eliminating microglia in Alzheimer’s mice prevents neuronal loss without modulating amyloid-β pathology. Brain 139, 1265–1281. doi: 10.1093/brain/aww016
Spangenberg, E., Severson, P. L., Hohsfield, L. A., Crapser, J., Zhang, J., Burton, E. A., et al. (2019). Sustained microglial depletion with CSF1R inhibitor impairs parenchymal plaque development in an Alzheimer’s disease model. Nat. Commun. 10:3758. doi: 10.1038/s41467-019-11674-z
Stalder, M., Phinney, A., Probst, A., Sommer, B., Staufenbiel, M., and Jucker, M. (1999). Association of Microglia with amyloid plaques in brains of APP23 transgenic mice. Am. J. Pathol. 154, 1673–1684. doi: 10.1016/S0002-9440(10)65423-5
Stevens, B., Allen, N. J., Vazquez, L. E., Howell, G. R., Christopherson, K. S., Nouri, N., et al. (2007). The classical complement cascade mediates CNS synapse elimination. Cell 131, 1164–1178. doi: 10.1016/j.cell.2007.10.036
Stillman, J. M., Lopes, F. M., Lin, J.-P., Hu, K., Reich, D. S., and Schafer, D. P. (2023). Lipofuscin-like autofluorescence within microglia and its impact on studying microglial engulfment. Nat. Commun. 14:7060. doi: 10.1038/s41467-023-42809-y
Sun, L., Qi, J., and Gao, R. (2018). Physical exercise reserved amyloid-beta induced brain dysfunctions by regulating hippocampal neurogenesis and inflammatory response via MAPK signaling. Brain Res. 1697, 1–9. doi: 10.1016/j.brainres.2018.04.040
Tagliatti, E., Desiato, G., Mancinelli, S., Bizzotto, M., Gagliani, M. C., Faggiani, E., et al. (2024). Trem2 expression in microglia is required to maintain normal neuronal bioenergetics during development. Immunity 57, 86–105.e9. doi: 10.1016/j.immuni.2023.12.002
Tesseur, I., Zou, K., Esposito, L., Bard, F., Berber, E., Can, J. V., et al. (2006). Deficiency in neuronal TGF-β signaling promotes neurodegeneration and Alzheimer’s pathology. J. Clin. Invest. 116, 3060–3069. doi: 10.1172/JCI27341
Thompson, P. M., Hayashi, K. M., de Zubicaray, G. I., Febke, A. L., Rose, S. E., Semple, J., et al. (2004). Mapping hippocampal and ventricular change in Alzheimer disease. NeuroImage 22, 1754–1766. doi: 10.1016/j.neuroimage.2004.03.040
Tobin, M. K., Musaraca, K., Disouky, A., Shetti, A., Bheri, A., Honer, W. G., et al. (2019). Human hippocampal neurogenesis persists in aged adults and Alzheimer’s disease patients. Cell Stem Cell 24, 974–982.e3. doi: 10.1016/j.stem.2019.05.003
Tremblay, M.-È., Stevens, B., Sierra, A., Wake, H., Bessis, A., and Nimmerjahn, A. (2011). The role of microglia in the healthy brain. J. Neurosci. 31, 16064–16069. doi: 10.1523/JNEUROSCI.4158-11.2011
Valero, J., España, J., Parra-Damas, A., Martín, E., Rodríguez-Álvarez, J., and Saura, C. A. (2011). Short-term environmental enrichment rescues adult neurogenesis and memory deficits in APPSw,Ind transgenic mice. PLoS One 6:e16832. doi: 10.1371/journal.pone.0016832
Vallières, L., Campbell, I. L., Gage, F. H., and Sawchenko, P. E. (2002). Reduced hippocampal neurogenesis in adult transgenic mice with chronic astrocytic production of Interleukin-6. J. Neurosci. 22, 486–492. doi: 10.1523/JNEUROSCI.22-02-00486.2002
van Praag, H., Christie, B. R., Sejnowski, T. J., and Gage, F. H. (1999). Running enhances neurogenesis, learning, and long-term potentiation in mice. Proc. Natl. Acad. Sci. USA 96, 13427–13431. doi: 10.1073/pnas.96.23.13427
van Praag, H., Schinder, A. F., Christie, B. R., Toni, N., Palmer, T. D., and Gage, F. H. (2002). Functional neurogenesis in the adult hippocampus. Nature 415, 1030–1034. doi: 10.1038/4151030a
Varnum, M. M., and Ikezu, T. (2012). The classification of microglial activation phenotypes on neurodegeneration and regeneration in Alzheimer’s disease brain. Arch. Immunol. Ther. Exp. 60, 251–266. doi: 10.1007/s00005-012-0181-2
Venegas, C., and Heneka, M. T. (2017). Danger-associated molecular patterns in Alzheimer’s disease. J. Leukoc. Biol. 101, 87–98. doi: 10.1189/jlb.3MR0416-204R
Verheijen, J., and Sleegers, K. (2018). Understanding Alzheimer disease at the Interface between genetics and transcriptomics. Trends Genet. 34, 434–447. doi: 10.1016/j.tig.2018.02.007
Villegas-Llerena, C., Phillips, A., Garcia-Reitboeck, P., Hardy, J., and Pocock, J. M. (2016). Microglial genes regulating neuroinflammation in the progression of Alzheimer’s disease. Curr. Opin. Neurobiol. 36, 74–81. doi: 10.1016/j.conb.2015.10.004
Vukovic, J., Colditz, M. J., Blackmore, D. G., Ruitenberg, M. J., and Bartlett, P. F. (2012). Microglia modulate hippocampal neural precursor activity in response to exercise and aging. J. Neurosci. 32, 6435–6443. doi: 10.1523/JNEUROSCI.5925-11.2012
Walker, D. G., Lue, L.-F., and Beach, T. G. (2001). Gene expression profiling of amyloid beta peptide-stimulated human post-mortem brain microglia. Neurobiol. Aging 22, 957–966. doi: 10.1016/S0197-4580(01)00306-2
Walton, N. M., Sutter, B. M., Laywell, E. D., Levkoff, L. H., Kearns, S. M., Marshall, G. P., et al. (2006). Microglia instruct subventricular zone neurogenesis. Glia 54, 815–825. doi: 10.1002/glia.20419
Wang, Y., Cella, M., Mallinson, K., Ulrich, J. D., Young, K. L., Robinette, M. L., et al. (2015). TREM2 lipid sensing sustains the microglial response in an Alzheimer’s disease model. Cell 160, 1061–1071. doi: 10.1016/j.cell.2015.01.049
Wang, W., Li, Y., Ma, F., Sheng, X., Chen, K., Zhuo, R., et al. (2023). Microglial repopulation reverses cognitive and synaptic deficits in an Alzheimer’s disease model by restoring BDNF signaling. Brain Behav. Immun. 113, 275–288. doi: 10.1016/j.bbi.2023.07.011
Wightman, D. P., Jansen, I. E., Savage, J. E., Shadrin, A. A., Bahrami, S., Holland, D., et al. (2021). A genome-wide association study with 1,126,563 individuals identifies new risk loci for Alzheimer’s disease. Nat. Genet. 53, 1276–1282. doi: 10.1038/s41588-021-00921-z
Wu, M. D., Montgomery, S. L., Rivera-Escalera, F., Olschowka, J. A., and O’Banion, M. K. (2013). Sustained IL-1β expression impairs adult hippocampal neurogenesis independent of IL-1 signaling in nestin+ neural precursor cells. Brain Behav. Immun. 32, 9–18. doi: 10.1016/j.bbi.2013.03.003
Yuan, H., Chen, R., Wu, L., Chen, Q., Hu, A., Zhang, T., et al. (2015). The regulatory mechanism of neurogenesis by IGF-1 in adult mice. Mol. Neurobiol. 51, 512–522. doi: 10.1007/s12035-014-8717-6
Yuan, P., Condello, C., Keene, C. D., Wang, Y., Bird, T. D., Paul, S. M., et al. (2016). TREM2 Haplodeficiency in mice and humans impairs the microglia barrier function leading to decreased amyloid compaction and severe axonal dystrophy. Neuron 90, 724–739. doi: 10.1016/j.neuron.2016.05.003
Zeng, Q., Zheng, M., Zhang, T., and He, G. (2016). Hippocampal neurogenesis in the APP/PS1/nestin-GFP triple transgenic mouse model of Alzheimer’s disease. Neuroscience 314, 64–74. doi: 10.1016/j.neuroscience.2015.11.054
Zhang, X., Mei, Y., He, Y., Wang, D., Wang, J., Wei, X., et al. (2021). Ablating adult neural stem cells improves synaptic and cognitive functions in Alzheimer models. Stem Cell Reports 16, 89–105. doi: 10.1016/j.stemcr.2020.12.003
Zhou, Y., Su, Y., Li, S., Kennedy, B. C., Zhang, D. Y., Bond, A. M., et al. (2022). Molecular landscapes of human hippocampal immature neurons across lifespan. Nature 607, 527–533. doi: 10.1038/s41586-022-04912-w
Ziegler-Waldkirch, S., Friesen, M., Loreth, D., Sauer, J.-F., Kemna, S., Hilse, A., et al. (2022). Seed-induced Aβ deposition alters neuronal function and impairs olfaction in a mouse model of Alzheimer’s disease. Mol. Psychiatry 27, 4274–4284. doi: 10.1038/s41380-022-01686-5
Ziegler-Waldkirch, S., Marksteiner, K., Stoll, J., d’Errico, P., Friesen, M., Eiler, D., et al. (2018a). Environmental enrichment reverses Aβ pathology during pregnancy in a mouse model of Alzheimer’s disease. Acta Neuropathol. Commun. 6:44. doi: 10.1186/s40478-018-0549-6
Ziegler-Waldkirch, S., Sauer, J., Erny, D., Savanthrapadian, S., Loreth, D., et al. (2018b). Seed-induced Aβ deposition is modulated by microglia under environmental enrichment in a mouse model of Alzheimer’s disease. EMBO J. 37, 167–182. doi: 10.15252/embj.201797021
Ziv, Y., Ron, N., Butovsky, O., Landa, G., Sudai, E., Greenberg, N., et al. (2006). Immune cells contribute to the maintenance of neurogenesis and spatial learning abilities in adulthood. Nat. Neurosci. 9, 268–275. doi: 10.1038/nn1629
Keywords: Alzheimer’s disease, amyloid plaques, microglia, adult neurogenesis, neurodegeneration
Citation: Früholz I and Meyer-Luehmann M (2024) The intricate interplay between microglia and adult neurogenesis in Alzheimer’s disease. Front. Cell. Neurosci. 18:1456253. doi: 10.3389/fncel.2024.1456253
Edited by:
Vassilis Stratoulias, University of Helsinki, FinlandReviewed by:
Andrew Octavian Sasmita, University College Cork, IrelandCopyright © 2024 Früholz and Meyer-Luehmann. This is an open-access article distributed under the terms of the Creative Commons Attribution License (CC BY). The use, distribution or reproduction in other forums is permitted, provided the original author(s) and the copyright owner(s) are credited and that the original publication in this journal is cited, in accordance with accepted academic practice. No use, distribution or reproduction is permitted which does not comply with these terms.
*Correspondence: Melanie Meyer-Luehmann, bWVsYW5pZS5tZXllci1sdWVobWFubkB1bmlrbGluaWstZnJlaWJ1cmcuZGU=