- Department of Biomedical Sciences, University of Cagliari, Monserrato, Italy
Conjugated linoleic acid (CLA) isomers exhibit anti-inflammatory properties within the central nervous system (CNS). This study investigated the effects of CLA isomers c9,t11 and t10,c12 on fatty acid (FA) and N-acylethanolamine (NAE) profiles and their association with pro-inflammatory molecule expression in BV-2 microglia cell line, the CNS's resident immune cells responsible for maintaining neuronal activity and immune homeostasis. BV-2 cells were treated with 25 μM of c9,t11-CLA, t10,c12-CLA, or oleic acid (OA) for 24 h, followed by lipopolysaccharide (LPS) stimulation. After treatment, the cell's FA and NAE profiles and pro-inflammatory molecule expression were analyzed. Our results demonstrated that CLA isomers mitigate LPS-induced morphological changes in BV-2 cells and reduce gene expression and protein levels of inflammatory markers. This effect was linked to an upregulation of acyl-CoA oxidase 1, a key enzyme in the anti-inflammatory peroxisomal beta-oxidation pathway that efficiently metabolizes CLA isomers. Notably, t10,c12-CLA significantly suppressed stearoyl-CoA desaturase 1, impacting monounsaturated fatty acid synthesis. The NAEs profile was remarkably altered by CLA isomers, with a significant release of the anti-neuroinflammatory mediator docosahexaenoic acid (DHA)-derived N-acylethanolamine (DHAEA). In conclusion, our findings suggest that the anti-neuroinflammatory effects of CLA isomers are due to their unique influences on FA metabolism and the modulation of bioactive FA-derived NAEs, highlighting a potential strategy for nutritional intervention in conditions characterized by neuroinflammation.
1 Introduction
Microglia represent the resident immune cells in the central nervous system (CNS) that actively survey the microenvironment and ensure normal neuronal activity and immunological integrity (Muzio et al., 2021). These cells protect the brain by phagocytosing bacteria, cellular debris, protein aggregates, and other antigens that may pose a threat to the CNS. Beyond their protective role, microglia are instrumental in neural network remodeling, synaptic pruning, and neurogenesis (Wake et al., 2011). Under pathological conditions, microglia transition to an activated state, adopting an amoeboid form and secreting inflammatory molecules like cytokines, chemokines, eicosanoids, reactive oxygen species, and nitric oxide, targeting pathogens or infected cells (Colonna and Butovsky, 2017). However, chronic or excessive activation can lead to detrimental inflammation, contributing to neurodegenerative diseases' progression by causing neuronal loss. Notably, neuroinflammation is a critical factor in various brain disorders, including ischemic events and neurodegenerative diseases like Alzheimer's, Parkinson's, and multiple sclerosis (Wendimu and Hooks, 2022). In aging, microglia activation is linked to increased sensitivity, potentially accelerating age-related neurodegeneration and disrupting microglia-synapse interactions (Du et al., 2017). Thus, controlling microglial activation might mitigate neuronal impairment in neurological conditions and during aging (Angelova and Brown, 2019).
Dietary fatty acids (FAs) are able to cross the blood-brain barrier (BBB) and are mostly esterified into membrane phospholipids of neurons and glial cells. These FAs influence brain functions directly or through their active metabolites (Bazinet and Layé, 2014). Recent findings suggest FAs can either promote or inhibit microglial inflammatory responses (Leyrolle et al., 2019). Specifically, saturated fatty acids (SFAs) trigger a pro-inflammatory microglial phenotype associated with neurodegeneration (Wang et al., 2012), while n-3 polyunsaturated FAs elicit anti-inflammatory effects, reducing apoptosis in the rodent brain (Jia et al., 2014).
Previous research has shown that conjugated linoleic acid (CLA) isomers, prevalent in meat and dairy, are actively utilized and metabolized in rat brains and cultured astrocytes, influencing the inflammatory response (Fa et al., 2005; Saba et al., 2019). Particularly, a major metabolite from CLA metabolism was identified as CD16:2, derived from its partial peroxisomal β-oxidation, hinting at a specific peroxisomal metabolism pathway for CLA (Fa et al., 2005). Among the isomers, c9,t11-CLA is predominant in dietary sources (Wahle et al., 2004) and has been linked to beneficial effects on neural cells and potential protection against neurodegenerative diseases (Fujita et al., 2021). Specifically, in vitro studies have shown that c9,t11-CLA promotes the proliferation of neural progenitor cells (Wang et al., 2011) and protects from glutamate- or Aβ-induced neuronal cell death (Hunt et al., 2010; Lee et al., 2013).
CLA not only affects FAs metabolism but also impacts the biosynthesis of N-acylethanolamines (NAEs), part of the endocannabinoidome, which regulates neuroprotection and inflammation (Batetta et al., 2009; Di Marzo et al., 2010; Murru et al., 2021a).
The interplay between CLA isomers and microglial activation, particularly through the NF-κB pathway, suggests a neuroprotective role against diet-induced brain inflammation (Salsinha et al., 2023).
The present study aims to assess the anti-inflammatory potential of CLA isomers on microglial cells in vitro, focusing on their impact on FAs metabolism, lipid mediator synthesis, and inflammatory molecule production. The findings could provide new insights into dietary CLA's role in mitigating neuroinflammation and its relevance to neurodegenerative diseases.
2 Materials and methods
2.1 Cell line and culture conditions
BV-2 microglial cells were cultured (2 × 104cells/ml) in complete medium containing low glucose DMEM supplemented with 10% fetal bovine serum (FBS), 1% penicillin/streptomycin (all from Gibco, Thermo Fisher Scientific), and maintained at 37°C with an atmosphere of 5% CO2. After 24 h, the medium was changed, and cells were incubated with 25 μM of c9,t11-CLA (#16413, Sigma Aldrich, Merck, Darmstadt, German) or t10,c12-CLA (#90154, Cayman Chemical, Ann Arbor, MI, USA) or oleic acid (OA) (# O-7008, Sigma Aldrich, Merck, Darmstadt, German) in complete medium. OA was used as control FA due to its similar incorporation with CLA into lipid fractions (Banni et al., 2001). Extensive testing confirmed that it does not induce any inflammatory effect on BV2 cells (Supplementary Figure S1). To establish a cellular model of neuroinflammation, after 48 h of administration of FAs, cells were exposed to lipopolysaccharide (LPS) 0.1 μg/ml (E. coli O26:B6, Sigma Aldrich, Merck, Darmstadt, German) and collected for mRNA, lipids and protein analysis as described in Figure 1.
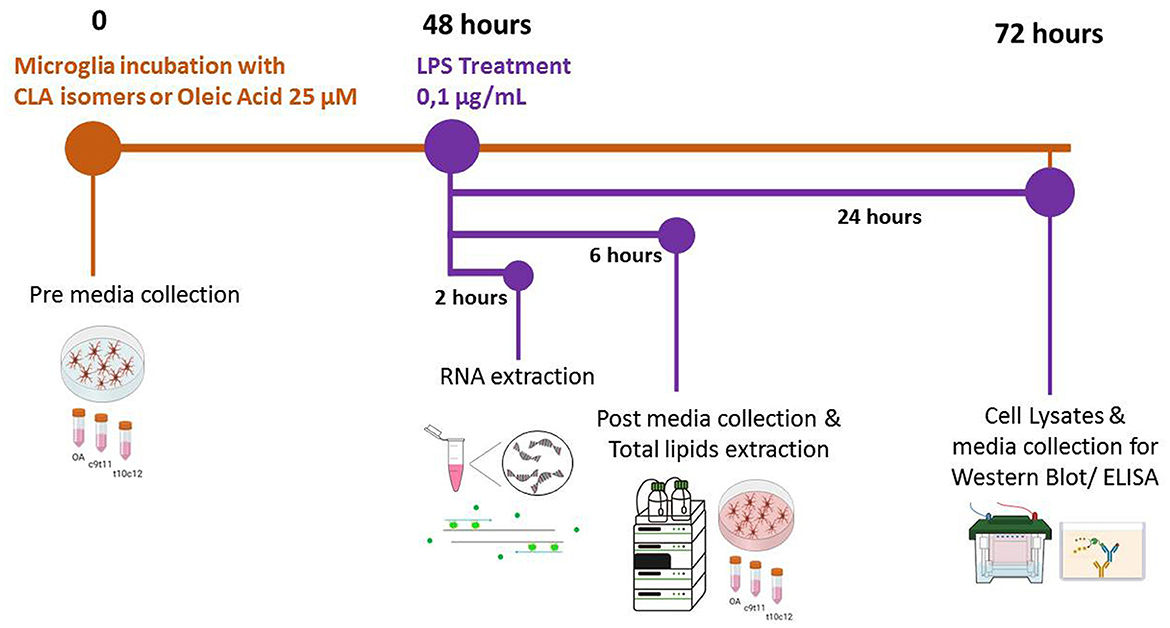
Figure 1. Timeline of the experimental procedures. At the initial time point (0 h), cells were incubated in complete culture media supplemented with 25 μM of either c9,t11-conjugated linoleic acid (CLA), t10,c12-CLA, or oleic acid (OA). Aliquots of this culture medium, henceforth referred to as “pre media”, were collected for subsequent analysis. After a 48-h incubation period, microglia were stimulated with lipopolysaccharide (LPS) for 2, 6, or 24 h, contingent upon the specific analyses planned. Following 6 h of LPS stimulation, additional media samples, designated as “post media”, were collected to enable a comparative analysis of fatty acid (FA) release relative to the “pre media”.
2.2 Real-time PCR
After 2 h of LPS treatment, total RNA was extracted using TRI Reagent (Sigma Aldrich, Merck, Darmstadt, German) according to the manufacturer's instructions. Total RNA was reversely transcribed into cDNA using the High-Capacity cDNA Reverse Transcription Kit (Applied Biosystems, Thermo Fisher Scientific). Real-time PCR was performed using PowerUp SYBR Green Master Mix (Applied Biosystems, Thermo Fisher Scientific) on Rotor-Gene Q (Qiagen) according to the manufacturer's procedure. The 2−ΔΔCT method was used for relative quantitative calculation, and TATA-binding protein (TBP) was used as the internal reference gene for normalization. The following specific primers were used for real-time PCR: Acox1 #qMmuCID0016828 (Bio-Rad, Hercules, California, U.S.A); Ccl5 #Mm.PT.58.43548565 (IDT, Coralville, IA, USA), Il-6 #Mm.PT.58.10005566 (IDT, Coralville, IA, USA); Il-1β #Mm.PT.58.41616450 (IDT, Coralville, IA, USA); Inos #qMmuCI0023087 (Bio-Rad, Hercules, California, U.S.A); Tbp #Mm.PT.39a.22214839 (IDT, Coralville, IA, USA).
2.3 Enzyme-linked immunosorbent assay
To verify cytokines release, 24 h after LPS treatment, the post medium of BV-2 cell culture was collected, centrifugated and tested with the following commercial kits: interleukin (IL)-6 (Invitrogen, Thermo Fisher Scientific, #88-7064-22), CCL5 also known as RANTES (regulated on activation, normal T cell expressed and secreted) (Invitrogen, Thermo Fisher Scientific, #88-56009-22). The assays were performed following the instructions provided by the manufacturers. The absorbance of the samples was measured at 450 nm using a microplate reader (Chameleon, Hidex).
2.4 Western blot
After 24 h of LPS exposure, BV-2 cells were lysed in 2% of sodium dodecyl sulfate (SDS), sonicated and centrifuged at 10,000 × g. Protein concentration was quantified using the Pierce BCA Protein Assay Kit (Sigma Aldrich, Merck, Darmstadt, German). Reducing loading buffer was added and the samples were denatured by boiling for 3 min. Fifteen micrograms of proteins were run on SDS/polyacrylamide gels and transferred onto (PVDF) membranes (Hybond-P, Amersham, Marlborough, MA, USA). Membranes were blocked with 5% of non-fat dry milk for 1 h at room temperature and incubated at 4°C overnight with the primary antibodies anti IL-1β (1:250, ab9722, Abcam, Cambridge, UK) or anti iNOS (1:500; ab178945, Abcam, Cambridge, UK). They were washed and incubated with horseradish-peroxidase-conjugated antirabbit IgG (1:10,000; #111-035-003 Jackson ImmunoResearch, Ely, UK) for 1 h at room temperature. Subsequently, bands were developed with the chemiluminescent substrate Lite a Blot Turbo (Euroclone, Pero, MI, Italy) and visualized with ImageQuant LAS-4000 (GE Healthcare, Little Chalfont, UK). Each experimental group's exposure time was optimized to prevent over-saturation and enable accurate densitometric analysis. Membranes were reprobed with anti GAPDH antibody (1:1,000; Mab 374, Millipore, Merck, Darmstadt, German) to normalize the signal of target proteins. Band intensity was analyzed using Image Studio software (LI-COR, Biosciences, Lincoln, NE, USA).
2.5 Lipid extraction and N-acylethanolamines and FAs analysis
Total lipids were extracted from the pellet containing the BV-2 cell culture after 48 h incubation with CLA isomers or OA and further 6 h of LPS or vehicle treatment, obtained after centrifugation, according to the method of Folch (Folch et al., 1957). Aliquots of the lipid fraction were mildly saponified in order to obtain free FAs for High Performance Liquid Chromatograph (HPLC) using an Agilent 1100 HPLC System (Agilent, Palo Alto, CA, USA) equipped with a diode array detector. A saponified fraction was methylated to obtained FA methyl esters (FAME) to measure SFAs by a Gas-Chromatograph (GC) (Agilent, Model 6890, Palo Alto) equipped with a flame ionization detector (FID) (Murru et al., 2021b).
Deuterated NAEs were added as internal standards to the samples before lipid extraction for quantification by isotope dilution: N-arachidonoylethanolamine [2H]8AEA, N-oleoylethanolamine [2H]2OEA, N-palmitoylethanolamine [2H]4PEA and N-stearoylethanolamine [2H]3SEA were purchased from Cayman Chemicals (MI, USA). NAEs quantification was carried out by an Agilent 1260 Infinity II UHPLC system (Agilent, Palo Alto) equipped with a mass spectrometry (MS) Agilent Technologies QQQ triple quadrupole 6420 with electrospray ionization (ESI) source, using positive mode (ESI+) (Murru et al., 2021b).
2.6 Statistical analysis
Data were analyzed with GraphPad Prism 8.0.1 software (San Diego, CA, USA) using one-way ANOVA for multiple comparisons. The ROUT method and Shapiro-Wilk normality test were employed to identify and remove any outliers and to assess the normal distribution of the data, respectively. Post-hoc analyses were performed with Dunnett's multiple comparison test with significance set at *p ≤ 0.05, **p ≤ 0.01, and ***p ≤ 0.001, ****p ≤ 0.0001. Data are presented as the mean ± SEM of at least three independent experiments.
3 Results
3.1 CLA isomers prevent LPS-induced morphological modification in BV-2 cells
The control cultures treated with OA (Figure 2A) predominantly exhibited a typical quiescent morphology characterized by elongated cell bodies and cytoplasmic extensions, while the cells subjected to LPS exposure (Figure 2B) transitioned to an activated state, adopting a rounded, amoeboid form. Notably, treatment with either isomer of CLA effectively mitigated the LPS-induced activation of BV-2 cells. Among the isomers, c9,t11-CLA (Figure 2C) demonstrated superior efficacy in preventing the activation compared to t10,c12-CLA (Figures 2D, E).
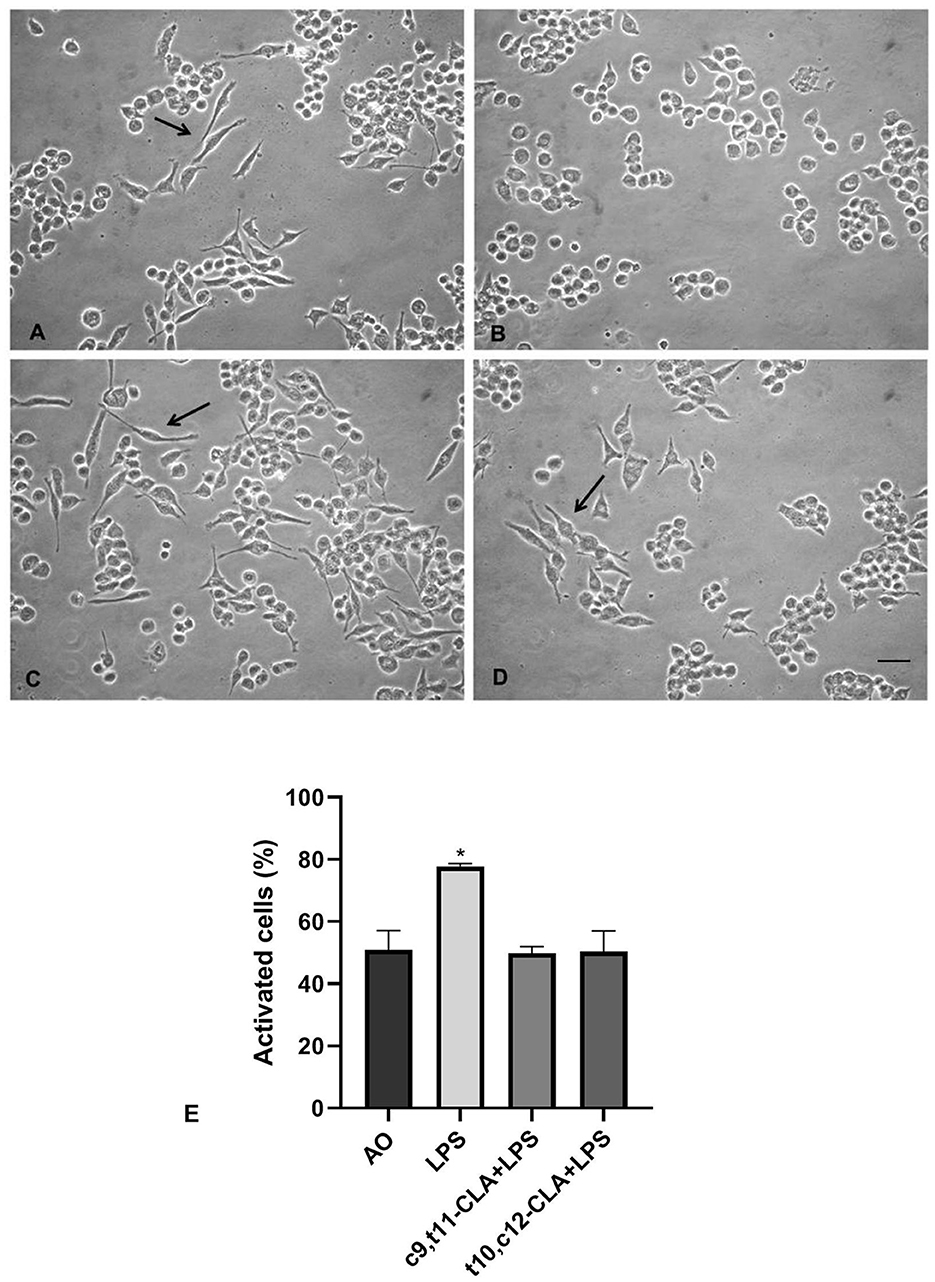
Figure 2. Effects of CLA isomers on LPS-induced morphological changes in BV-2 cells. (A) unstimulated BV-2 cells incubated with oleic acid (OA); (B) LPS-stimulated BV-2 cells incubated with OA; (C) LPS-stimulated BV-2 cells incubated with c9,t11-CLA, or t10,c12-CLA (D). Arrows indicate quiescent morphologies with elongated cellular projections. Bar = 50 μm. (E) Quantification of activated BV-2 cells based on their morphological changes. Data represent means ± SEM of n = 3 experiments. *p < 0.05 respect to OA.
3.2 CLA isomers attenuate gene expression of pro-inflammatory markers
To assess the impact of CLA isomers on the mRNA expression of CCL5, IL-6, and IL-1β, BV-2 microglial cells underwent incubation with c9,t11-CLA or t10,c12-CLA isomers for 48 h, followed by analysis of mRNA levels using real-time PCR. The results, depicted in Figures 3A–C, indicate a notable reduction in the mRNA expression of CCL5, IL-1β, and IL-6 for both CLA isomers. As expected, activation of microglia through LPS exposure markedly increased the mRNA levels of these cytokines. Pre-treatment with c9,t11-CLA significantly mitigated the mRNA expression of CCL5 and IL-6 following LPS challenge but did not alter IL-1β levels. Conversely, t10,c12-CLA was effective in significantly reducing the mRNA expression of all three assessed cytokines, as illustrated in Figures 3D–F.
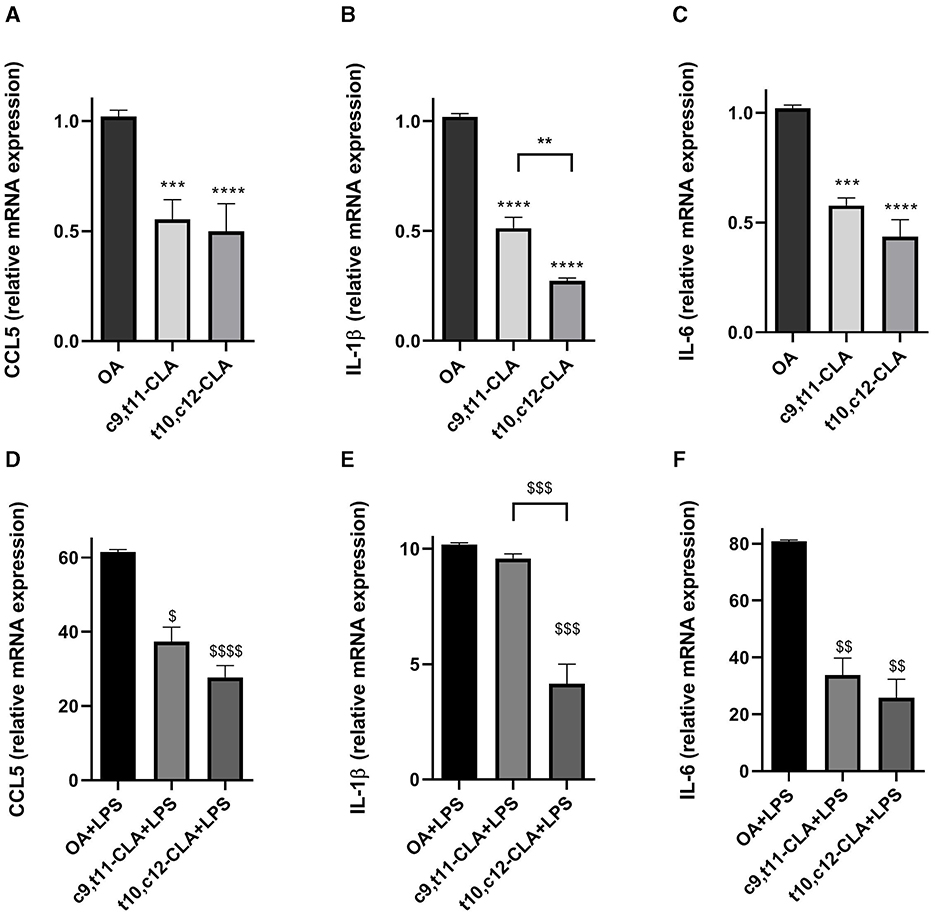
Figure 3. Effects of CLA isomers on the mRNA expression of pro-inflammatory markers CCL5 (A, D), IL-1β (B, E) and IL-6 (C, F) compared to control (oleic acid, OA), in unstimulated (A–C) and LPS-stimulated BV-2 cells (D–F), measured by real-time PCR. The bars represent the fold-change relative to OA. Data represent means ± SEM of n = 3 experiments. **p < 0.01, ***p < 0.001, ****p < 0.0001 respect to OA; $p < 0.05, $$p < 0.01, $$$p < 0.001, $$$$p < 0.0001 respect to OA + LPS.
3.3 CLA isomers attenuate protein levels of pro-inflammatory markers
Cytokine secretion was assessed using ELISA in the medium of BV-2 cells treated with CLA isomers. Both c9,t11-CLA and t10,c12-CLA markedly reduced the secretion of CCL5 and IL-6, irrespectively of whether they were exposed to LPS, with t10,c12-CLA demonstrating greater efficacy (Figures 4A, B, D, E). Since IL-1β was not detectable in the medium by ELISA (data not shown), we explored the effects of CLA isomers on IL-1β expression within BV-2 cell extracts. Western blot analysis identified a band at 31 kDa, indicative of the intracellular pro-IL-1β increased expression following LPS induction. Both c9,t11-CLA and t10,c12-CLA notably decreased the expression of pro-IL-1β in cells, regardless of LPS treatment status (Figures 4C–F).
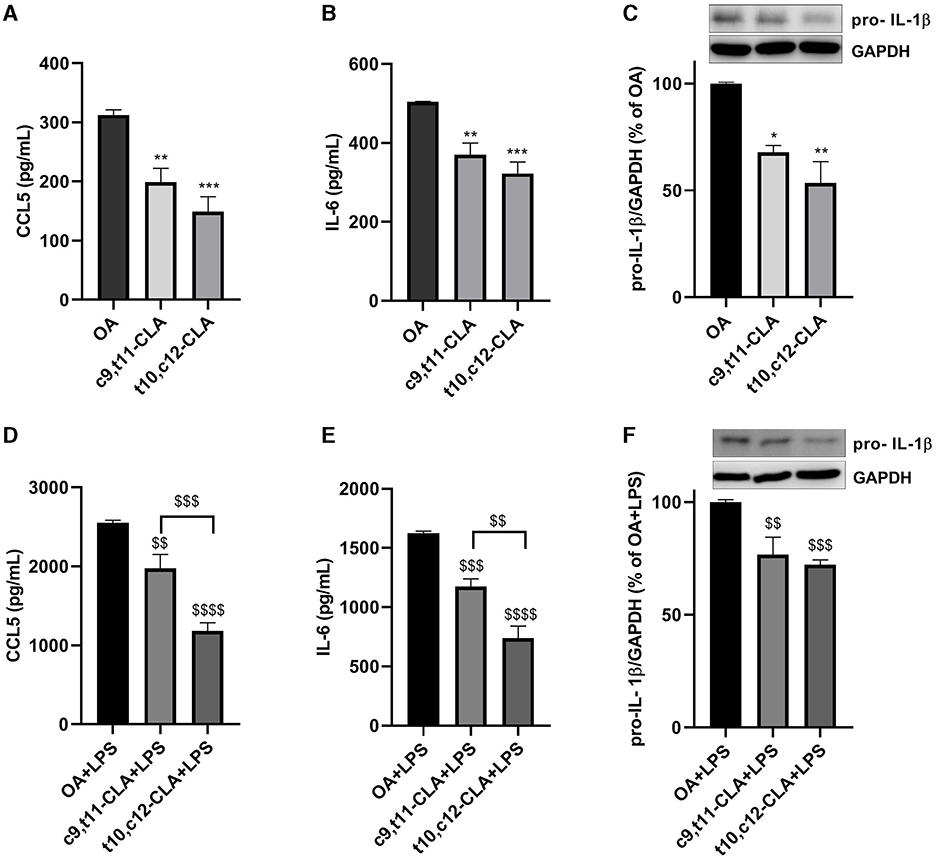
Figure 4. Impact of CLA isomers on the secretion of pro-inflammatory mediators CCL5 (A, D), IL-6 (B, E) and pro-IL-1β (C, F) in comparison to the control (oleic acid, OA), within the culture media of unstimulated (A–C) or LPS-stimulated BV-2 microglial cells (D–F). Data represent means ± SEM of n = 3 experiments. *p < 0.05, **p < 0.01, ***p < 0.001 respect to OA; $$p < 0.01, $$$p < 0.001, $$$$p < 0.0001 respect to OA + LPS.
3.4 CLA isomers reduce gene expression and production of iNOS
The inducible nitric oxide synthase (iNOS) is a critical enzyme whose increased levels are indicative of an active inflammatory process. We evaluated the expression levels of iNOS mRNA and protein in BV-2 cells treated with c9,t11-CLA or t10,c12-CLA, followed by a challenge with LPS, using real-time PCR and western blot analysis, respectively. As anticipated, iNOS mRNA and protein expressions were elevated in BV-2 cells following LPS exposure. However, this upregulation was mitigated when cells were pre-treated with either c9,t11-CLA or t10,c12-CLA, with or without LPS challenge (Figure 5).
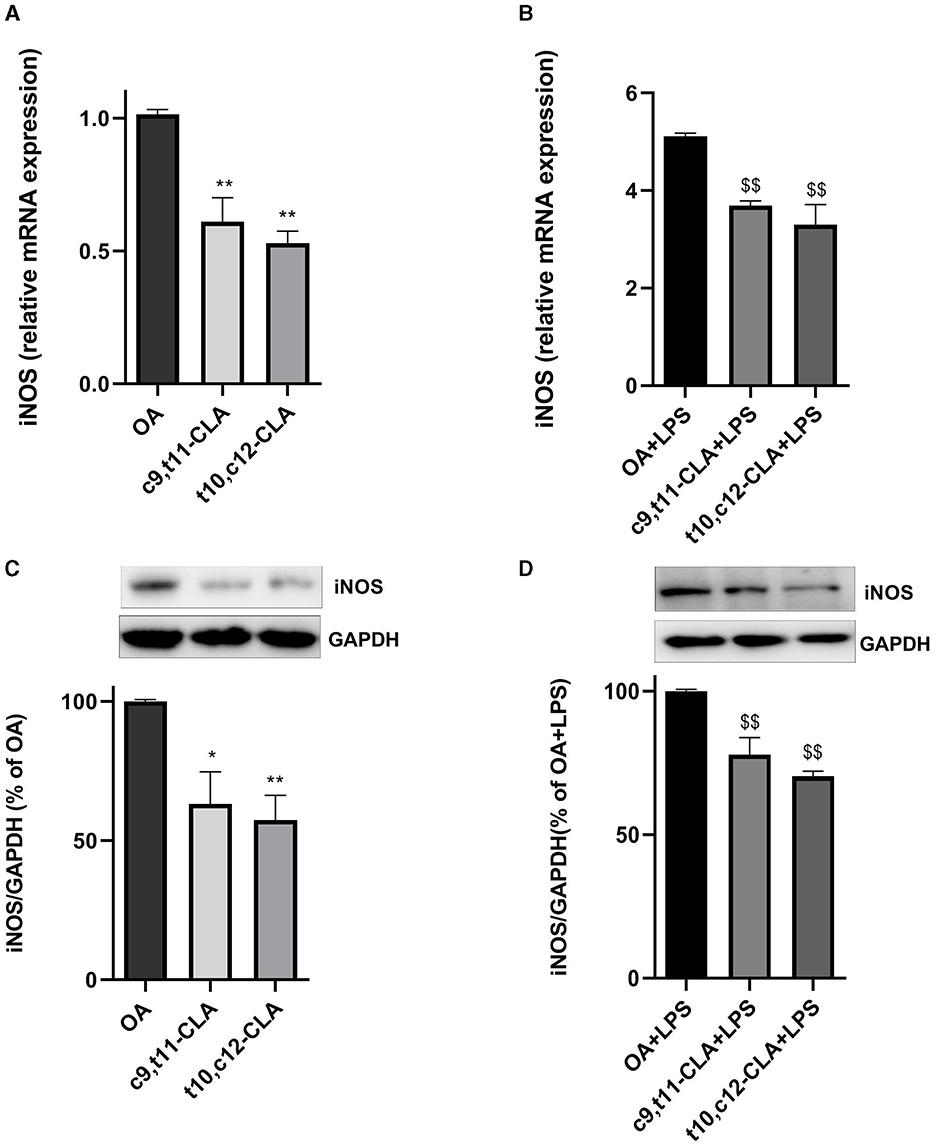
Figure 5. Influence of CLA isomers on iNOS expression in BV-2 cells at both mRNA (A, B) and protein (C, D) levels, compared to control (oleic acid, OA) in unstimulated and LPS-stimulated BV-2 cells. The results are expressed as fold changes in gene expression relative to the OA-treated group (A, B). The lower panels delineate the effects on iNOS protein levels in unstimulated (C) and LPS-stimulated (D) BV-2 cells, assessed by Western blot analysis. Data represent means ± SEM of n = 3 experiments. *p < 0.05, **p < 0.01 respect to OA; $$p < 0.01 respect to OA + LPS.
3.5 CLA isomers effect on β-peroxisomal oxidation
To determine if the anti-inflammatory effects of CLA isomers are linked to alterations in FAs metabolism, we evaluated changes in the profiles of FAs and bioactive metabolites derived from FAs, such as NAEs, which play a crucial role in modulating inflammatory processes. After 48 h of incubation with CLA, both CLA isomers (c9,t11 and t10,c12) were substantially incorporated into BV-2 cells. After LPS exposure, the assimilation of the t10,c12-CLA isomer was significantly higher by 28% compared to c9,t11-CLA (Figure 6A). Moreover, a marked increase in the concentration of the peroxisomal β-oxidation product CD16:2 was observed for t10,c12-CLA, being roughly three times higher than that from c9,t11-CLA. The accumulation pattern of CD16:2 remained unchanged following LPS treatment (Figure 6B).
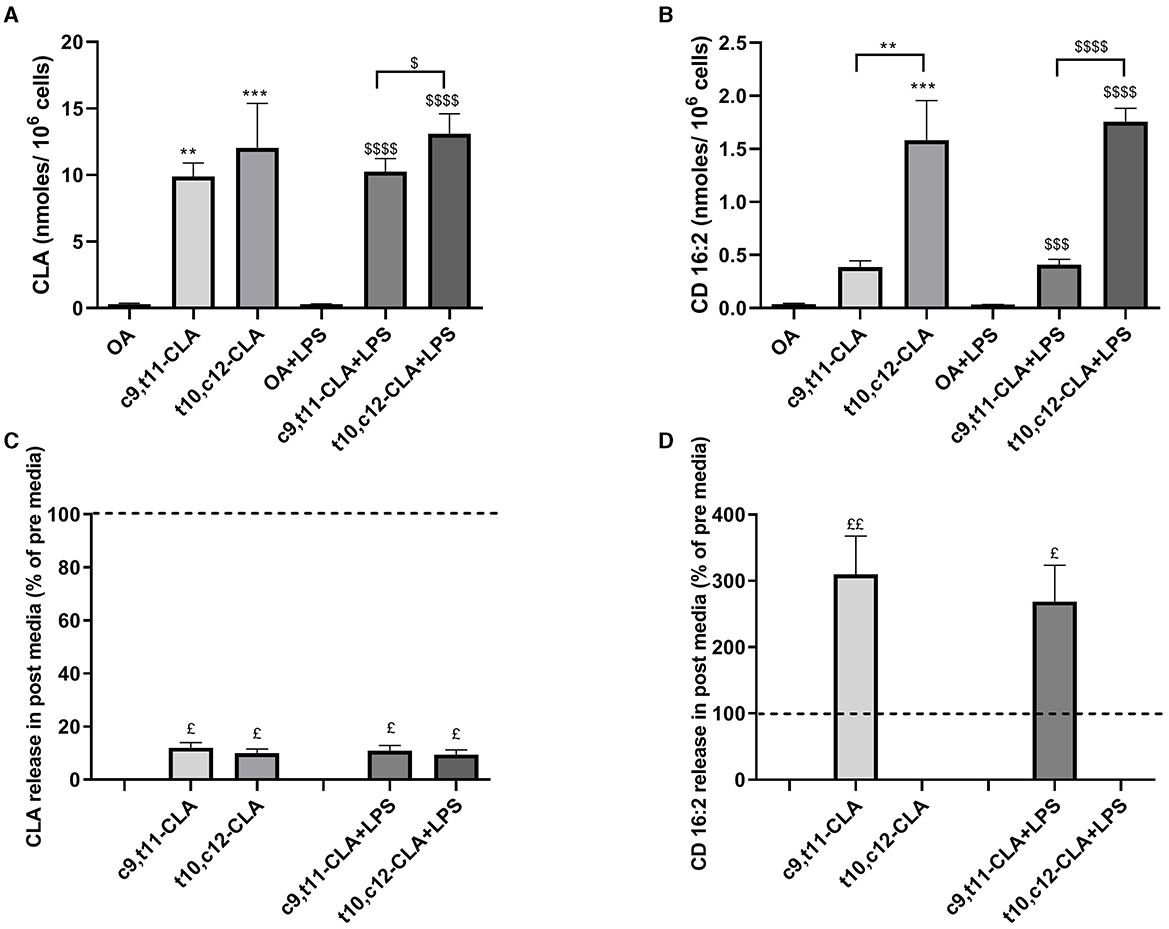
Figure 6. Incorporation of CLA isomers (A) and biosynthesis of their peroxisomal β-oxidation product, CD16:2 (B) in unstimulated and LPS-stimulated BV2 cells with oleic acid (OA) serving as the control. Data are presented as nmoles/106 cells. Panels (C, D) focus on the extracellular release dynamics of CLA and CD16:2, respectively, comparing the concentrations in the media after 48 h of incubation with the isomers, both in the presence and absence of LPS stimulation, relative to initial media concentrations, as indicated by the dashed lines. Data represent means ± SEM of n = 3 experiments. **p < 0.01, ***p < 0.001 respect to OA; $p < 0.05, $$$p < 0.001, $$$$p < 0.0001 respect to OA + LPS; $p < 0.05, $$p < 0.01 respect to pre media.
The extracellular concentrations of CLA isomers and their metabolites were evaluated by examining the culture media before (pre media) and after treatment (post media). The levels of CLA isomers decreased by ~90% in the media, indicating rapid uptake by the microglia (Figure 6C). Intriguingly, only CD16:2 derived from the c9,t11-CLA isomer was detected in the medium, implying that although both isomers undergo β-oxidation in peroxisomes, c9,t11-CLA is less retained within microglial cells. Moreover, the release of CD16:2 into the medium was marginally reduced by LPS treatment (Figure 6D).
The effective β-oxidation of CLA in peroxisomes led us to investigate the gene expression levels of acyl-CoA oxidase 1 (ACOX1), a pivotal enzyme in this metabolic pathway. Under baseline conditions, treatment with c9,t11-CLA and t10,c12-CLA resulted in elevated ACOX1 mRNA levels, showing respective increases of ~35 and 45%. However, exposure to LPS reduced ACOX1 expression by around 45%. Interestingly, the presence of both CLA isomers mitigated the LPS-induced suppression of ACOX1 expression (Figure 7).
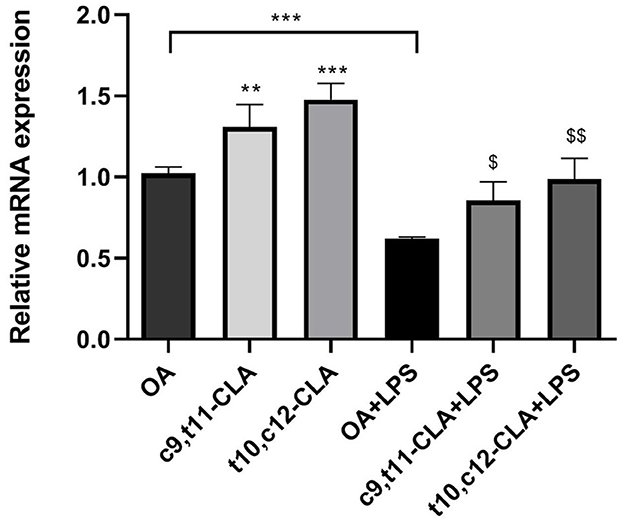
Figure 7. ACOX1 mRNA expression in both unstimulated and LPS-stimulated BV-2 cells, after a 48-h incubation period with CLA isomers. The bar graph illustrates the fold change in ACOX1 mRNA expression for each treatment group, normalized to the expression level observed in cells incubated with oleic acid (OA), which serves as the control. Data represent means ± SEM of n = 3 experiments. **p < 0.01, ***p < 0.001 respect to OA; $p < 0.05, $$p < 0.01 respect to OA + LPS.
3.6 CLA isomers effect on monounsaturated fatty acid metabolism
We conducted analysis of the FAs profiles in BV-2 cells to investigate the potential impact of CLA on cellular lipid metabolism. Treatment with the t10,c12-CLA isomer significantly decreased the levels of OA and palmitoleic acid (POA) when compared to cells incubated with OA or the c9,t11-CLA isomer (Figures 8A, B). The effect of t10,c12-CLA on POA levels was diminished following LPS treatment. Importantly, cells treated with t10,c12-CLA exhibited a reduced POA to palmitic acid (PA) ratio, suggesting a decrease in stearoyl-CoA desaturase-1 (SCD1) activity, which is responsible for the desaturation of SFAs to MUFAs (Figure 8C). No alterations were observed in the concentrations of other FAs.
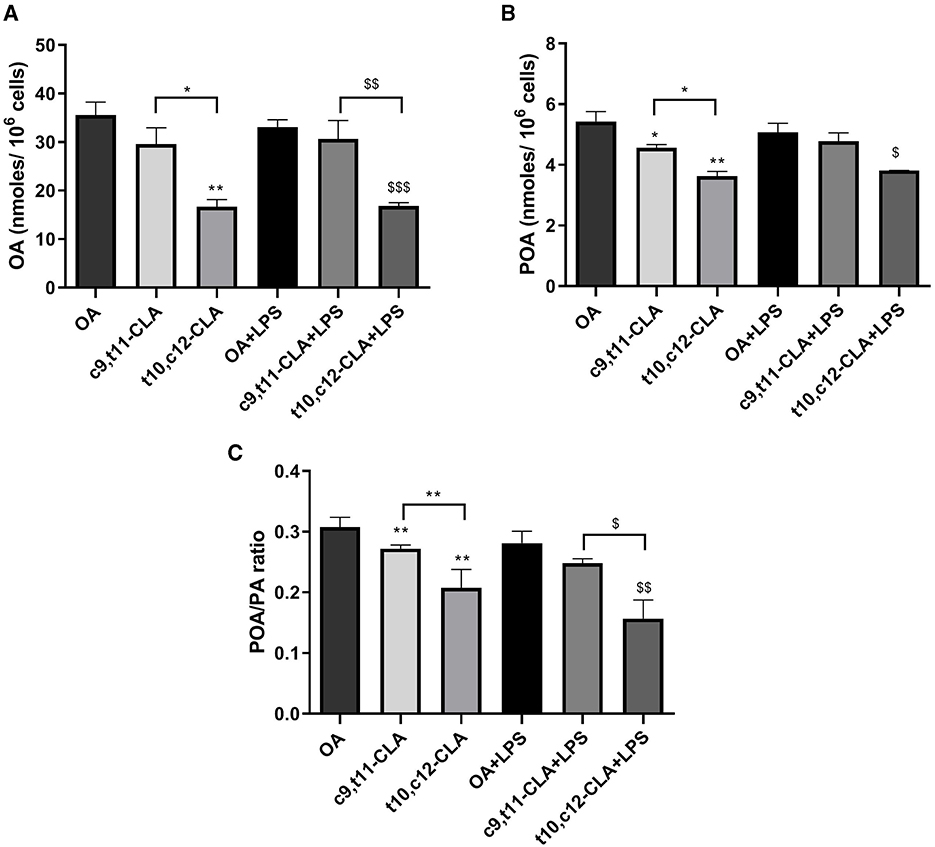
Figure 8. Analysis of main MUFAs oleic acid, OA (A), palmitoleic acid, POA (B) and the ratio of palmitoleic acid to its precursor palmitic acid, PA (C) in BV-2 cells. These measurements were taken after a 48-h incubation period with c9,t11-CLA or t10,c12-CLA. The data compare these levels in both unstimulated and LPS-stimulated cells to the control group treated with OA. Data represent means ± SEM of n = 3 experiments. *p < 0.05, **p < 0.01 respect to OA; $p < 0.05, $$p < 0.01, $$$p < 0.001 respect to OA + LPS.
3.7 Impact of CLA isomers on N-acylethanolamines biosynthesis
Treating BV-2 cells with CLA isomers led to distinct changes in the biosynthesis of lipid mediators, particularly FAs-derived N-acylethanolamines (NAEs). Specifically, the reduction in OA and POA levels in cells treated with the t10,c12-CLA isomer was associated with decreased levels of their corresponding NAEs, palmitoleoylethanolamide (POEA) and oleoylethanolamide (OEA) as shown in Figures 9B, C, respectively. Notably, these changes were not influenced by LPS treatment.
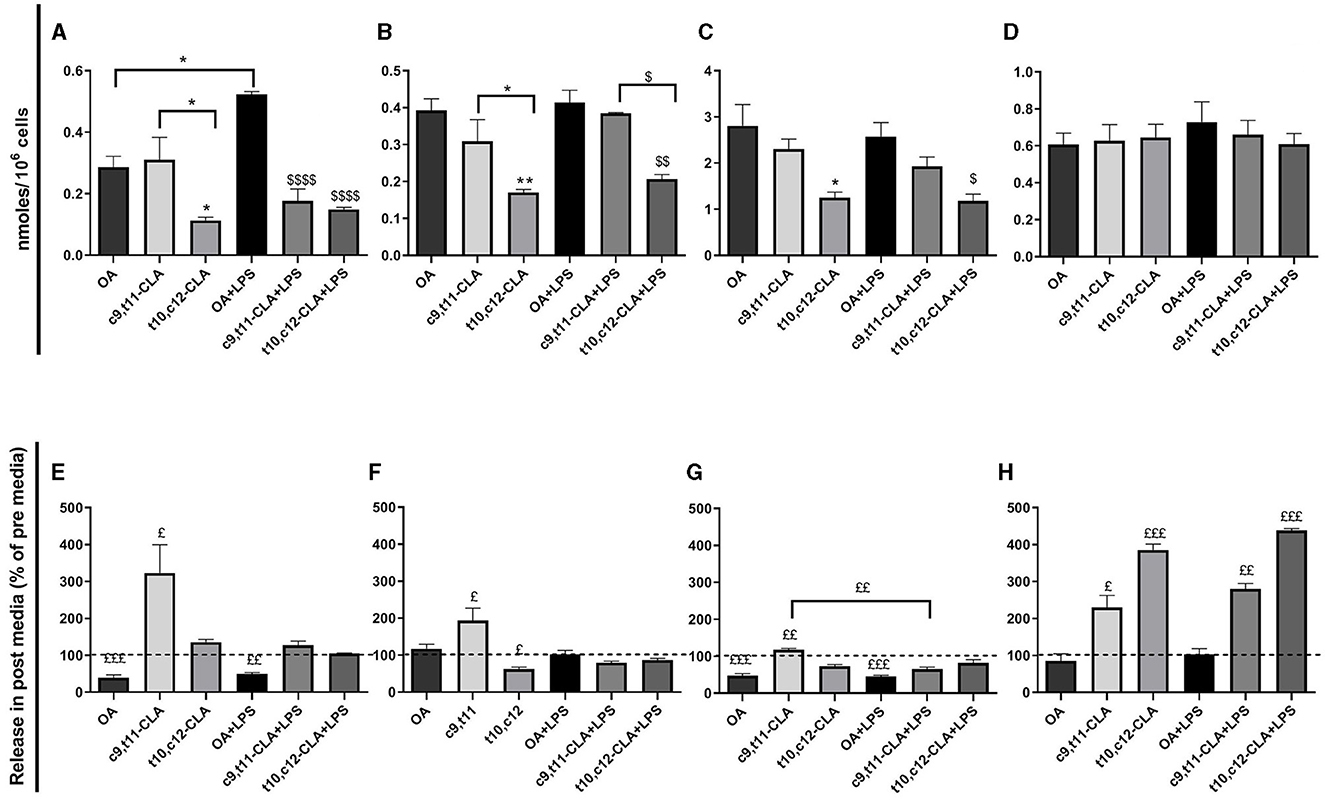
Figure 9. Impact of CLA isomers on the levels of lipid mediators N-acylethanolamines (NAEs) within BV2 cells and in media post-incubation (post media): AEA (A, E), POEA (B, F), OEA (C, G), DHAEA (D, H). The intracellular NAEs levels (A–D) are quantified in nmoles/106 cells and compared across treatments with the two CLA isomers relative to the OA control. In the post media (E–H), NAEs concentrations are presented as a percentage of their release, with the baseline levels in pre media represented by dashed lines. Data represent means ± SEM of n = 3 experiments. *p < 0.05, **p < 0.01 respect to OA; $p < 0.05, $$p < 0.01, $$$$p < 0.001 respect to OA + LPS; $p < 0.05, $$p < 0.01, $$$p < 0.001 respect to pre media.
Additionally, arachidonoylethanolamide (AEA), a NAE derived from arachidonic acid, was reduced in cells treated with t10,c12-CLA. Interestingly, while LPS exposure increased AEA levels in cells treated with OA, this rise was not observed in cells treated with either CLA isomer, as depicted in Figure 9A.
Cells treated with the c9,t11-CLA isomer demonstrated a marked extracellular release of AEA, as illustrated in Figure 6E. Both POEA and OEA were also released into the media from c9,t11-CLA-treated cells, although this release was diminished by LPS exposure (Figures 9F, G). Conversely, docosahexaenoylethanolamide (DHAEA) levels, originating from docosahexaenoic acid (DHA), remained stable within the cells (Figure 9D). However, the extracellular release of DHAEA was significantly more elevated, showing increases of 2.3- and 3.8-fold in media from cells treated with c9,t11-CLA and t10,c12-CLA, respectively. This release was further augmented after LPS treatment, which led to increases of 2.8- and 4.3-fold, correspondingly (Figure 9H).
4 Discussion
Neuroinflammation represents a common feature in most neurodegenerative diseases and is characterized by activation of glial cells, release of inflammatory mediators, such as cytokines and chemokines, and generation of reactive oxygen and nitrogen species (Kempuraj et al., 2016). The main mediators of neuroinflammation are microglia cells, the resident immune cells of the CNS. Microglial activation occurs in response to changes in homeostasis and pathological insults to the brain. Activated microglia rapidly change their morphology and expression of diverse molecules inducing neuronal damage and dysfunction and promoting the progression of neurodegeneration (Colonna and Butovsky, 2017). Hence, inhibiting the persistent activation of microglia and mitigating the release of pro-inflammatory molecules emerges as a potentially effective strategy for both the prevention and treatment of neurodegenerative diseases.
The present study provides compelling evidence for the modulatory impact of CLA isomers on BV-2 microglial cell activation. In fact, in vitro incubation with both c9,t11-CLA and t10,c12-CLA isomers demonstrated a significant reduction of the expression of cytokines and other inflammatory mediators in BV-2 microglial cells, both under physiological conditions and after LPS stimulation. CLA has been reported to exhibit anti-inflammatory and immunomodulatory effects in animal models (Yang et al., 2015) and humans (Haghighatdoost and Nobakht M. Gh, 2018; Rastgoo et al., 2023). Numerous studies have reported the ability of CLA to reduce secretion of pro-inflammatory cytokines in different cell types, such as macrophages (Yu et al., 2002), bronchial epithelial cells (Huang et al., 2016), and peripheral blood mononuclear cells (Kim et al., 2011a). CLA can cross the BBB, reaching the CNS where is incorporated and metabolized (Fa et al., 2005) and exerts various neuroprotective functions, including protection from glutamate excitotoxicity (Hunt et al., 2010), memory improvement (Gama et al., 2015), and prevention of cognitive decline in animal models of neurodegenerative diseases (Monaco et al., 2018; Binyamin et al., 2019). Recent evidence further suggests CLA's potential role in demyelinating disorders by regulating autophagic lysosomal overactivation and cellular dysfunction in microglia (Zhou et al., 2023).
BV-2 cells represent a well-characterized and widely used model system for microglia. In this study, after treatment with LPS to establish a neuroinflammation cell model, most of cells acquired an activated phenotype and increased the expression of CCL5, IL-1β, IL-6, and iNOS. CLA isomers prevented BV-2 activation and reduced the expression of those inflammatory mediators, all involved in neurodegenerative processes. For example, CCL5 is a chemokine involved in several neurodegenerative diseases, neuroinflammation, and excitotoxic neurodegeneration (Cartier et al., 2005; Musante et al., 2008). Moreover, IL-1β may induce synaptic loss and contribute to neuronal injury and cell death (Allan et al., 2005; Sheppard et al., 2019).
In our study, IL-1β was undetectable in the cell culture supernatant of LPS-activated BV-2 cells; however, the intracellular precursor, pro-IL-1β, was identified. This observation aligns with the understanding that IL-1β maturation and secretion are rigorously regulated processes, mediated by cytosolic inflammasome complexes. These complexes activate caspase-1, which is essential for the cleavage of pro-IL-1β into its active form (Chan and Schroder, 2019). Our findings suggest that, in BV-2 cells, LPS stimulation alone does not suffice to trigger the NLRP3 inflammasome activation, indicating that additional stimuli are necessary for this process (Fan et al., 2017).
In the present study, we observed that CLA isomers can suppress the expression of inflammatory molecules in both LPS-activated and unstimulated microglial cells. Notably, BV-2 microglia, when cultured in serum-supplemented media, exhibit minimal activation levels, as demonstrated in our study (shown in Figure 2) and supported by findings from Laurenzi et al. (2001). This minimal activation state mirrors the low-grade inflammation characteristic of the aging brain, which contributes to increased risks of cognitive decline and heightened vulnerability to neurodegenerative diseases (Kempuraj et al., 2016; Matt and Johnson, 2016). Our findings suggest that CLA has the potential to mitigate the risk of neurodegenerative conditions in older adults by downregulating pro-inflammatory mediators associated with low-grade neuroinflammation.
These results are consistent with previous research demonstrating the neuroprotective properties of dietary CLA in a mouse model of age-related neurodegeneration, which suggested that CLA prompts an adaptive response beneficial for brain health (Monaco et al., 2018). Accordingly, we have reported that dietary CLA intake was associated with reduced inflammatory markers in cerebrospinal fluid and enhanced somatosensory evoked potentials in female carriers of X-linked adrenoleukodystrophy (Cappa et al., 2012). Furthermore, a comparative study revealed elevated levels of CLA and DHAEA in elderly individuals residing in a High-Longevity Zone in Sardinia's east-central mountainous region compared to younger individuals and elderly residents of a Low-Longevity Zone (Manca et al., 2021), suggesting a link between these compounds and longevity.
The mechanisms underlying effects of CLA remain largely unexplored and necessitate further investigation. The present study suggests three possible molecular mechanisms of action that may synergistically contribute to the anti-inflammatory effect of CLA.
(1) The increased ACOX1 expression paralleled the β-oxidation of both CLA isomers within peroxisomes in BV-2 microglial cells, yielding CD16:2 metabolite. ACOX1 enzyme catalyzes the first and rate-limiting step of peroxisomal FA β-oxidation and participates in maintaining immune homeostasis in the organism. ACOX1 is widely expressed in the brain (Farioli-Vecchioli et al., 2001) and its deficiency causes a severe neuroinflammatory and neurodegenerative peroxisomal disease, pseudoneonatal adrenoleukodystrophy (Poll-The et al., 1988). LPS induces a decrease in ACOX1 mRNA expression and consequently peroxisomal dysfunction and lipid metabolism alteration (Vamecq et al., 2018). A peroxisomal defect induces microglia cells to acquire a reactive phenotype associated with the upregulation of pro-inflammatory molecules (Tawbeh et al., 2023). The anti-inflammatory role of ACOX1 is mediated by inactivation of oxygenated eicosanoids via peroxisomal β-oxidation which also generates precursors of potent anti-inflammatory mediators, such as resolvins (Di Cara et al., 2019). We observed that CLA not only prevented LPS-induced reduction of the ACOX1 activity in BV-2 cells exposed to LPS, but also induced an increase in ACOX1 expression in unstimulated BV-2 cells. The up-regulation of ACOX1 in mouse livers following dietary CLA intake has been described by Belury et al. (1997) probably mediated via peroxisome proliferator-activated receptor-α (PPAR-α) activation. Our data revealed that the CD16:2 β-oxidized metabolite, derived from c9,t11-CLA was promptly released into the medium. Further studies should be made to establish whether the release of CD16:2 into the medium might possess neuroprotective role as a ligand for PPAR alpha in other neural cells such as oligodendrocytes, astrocytes and neurons. It has been shown that c9,t11-CLA promotes proliferation of rat neural progenitor cells, while the isomer t10,c12-CLA, whose CD16:2 metabolite is not released in the medium in our experimental model, had the opposite effect (Wang et al., 2011).
(2) Importantly, t10,c12-CLA isomer exhibited distinct effect, decreasing MUFAs levels, as indicated by a 66% decrease in the POA/PA ratio by a reduction of SCD1 activity. These findings are consistent with a previous study suggesting that FAs with c12 double bond appear to be a crucial structural feature for inhibiting SCD1 activity, especially when paired with a t10 double, whereas a c11 double bond is less effective (Ntambi and Miyazaki, 2004). SCD1, a key enzyme regulator of lipid metabolism and MUFAs synthesis, appears to exert a dual influence on the microglia phenotype depending on the disease context. A recent study has shown that MUFAs generated by SCD1 can drive microglia and macrophages toward an inflammatory phenotype, influencing inflammatory and repair processes. SCD1 inhibition led to an anti-inflammatory shift in macrophages and microglia, enhancing remyelination (Bogie et al., 2020). We therefore suggest that the potential anti-neuroinflammation role of t10,c12-CLA might be at least in part exerted by SCD1 inhibition.
(3) Both CLA isomers have been demonstrated to significantly alter FA metabolism and the profile of FA-derived lipid mediators, such as NAEs, which play a pivotal role in modulating neuroinflammation (Saba et al., 2019; Murru et al., 2021a), In our current investigation, exposure to both CLA isomers resulted in a reduction of AEA levels in BV-2 microglial cells treated with LPS. Notably, the isomer t10,c12-CLA was also associated with decreased levels of POEA and OEA, potentially due to diminished availability of their MUFA precursors, POA and OA. Conversely, incubation with c9,t11-CLA markedly elevated the release of AEA, POEA, and OEA into the medium, an effect negated by LPS exposure. The suppression of OEA release by LPS could hinder OEA's role in modulating microglial phenotype transition from the pro-inflammatory M1 to the anti-inflammatory M2 state, a process mediated by PPAR alpha (Li et al., 2023).
Interestingly, while CLA isomers did not alter intracellular levels of DHAEA, derived from DHA, in BV-2 cells, they prompted its release into the medium, particularly observed with t10,c12-CLA in both untreated and LPS-stimulated conditions.
DHAEA, also known as synaptamide, may significantly contribute to the anti-neuroinflammatory activity of CLA. In vivo studies have demonstrated DHAEA's capacity to mitigate pro-inflammatory cytokine production, including the suppression of LPS-induced microglial activation and pro-inflammatory cytokine expression (Park et al., 2016). Prior research indicates that DHAEA activates GPR110 receptor, inducing upregulation of cAMP in CNS microglia and peripheral macrophages. cAMP in turn may inhibit NF-κB transcriptional activity, thereby reducing microglial activation and pro-inflammatory cytokine output (Park et al., 2019). Furthermore, DHAEA has been recognized for its biological activity that promotes neurogenesis and synaptogenesis (Kim et al., 2011b; Rashid et al., 2013; Kharebava et al., 2015; Kim and Spector, 2018).
The use of an in vitro model involving a single cell type, such as the BV-2 microglial cell line, inherently presents certain limitations, primarily due to the absence of interactions with other cell types, including neurons and other glial cells. Additionally, the BV-2 cell line exhibits reduced reactivity compared to primary microglial cells when stimulated with LPS. Nevertheless, BV-2 cells retain several key microglial characteristics, including phagocytic activity and the ability to secrete NO and pro-inflammatory cytokines upon stimulation (Henn et al., 2009). These features render BV-2 cells highly suitable for biochemical and molecular studies that require large cell quantities, and they remain the most widely employed cell line for investigating microglial behavior and inflammatory responses in vitro. Given the critical role of microglia in neuroinflammation, a comprehensive understanding of their behavior is essential for elucidating the mechanisms by which CLA isomers influence microglial activity. In this context, in vitro models are indispensable tools for generating foundational knowledge.
In conclusion, our study implies that the anti-inflammatory effects demonstrated by CLA isomers may be attributed to their distinct impact on FA metabolism and modulation of bioactive FA-derived NAEs. This insight offers a promising avenue for therapeutic intervention aimed at preventing disorders where neuroinflammation plays a central role. By addressing neuroinflammation, particularly by mitigating microglial activation and increasing the release of anti-inflammatory mediators, a potential strategy emerges to offer an environment conducive to neuronal function and homeostasis. This underscores the potential of targeting fatty acid metabolism as a viable therapeutic approach to counteract neuroinflammation-associated disorders.
Despite the acknowledged limitations of in vitro systems, our findings provide a basis for future research that includes studies on additional brain cell types and in vivo experiments. These subsequent studies will be crucial for confirming the observed mechanisms in vivo and further exploring the anti-inflammatory properties of CLA.
Data availability statement
The raw data supporting the conclusions of this article will be made available by the authors, without undue reservation.
Ethics statement
Ethical approval was not required for the studies on animals in accordance with the local legislation and institutional requirements because only commercially available established cell lines were used.
Author contributions
CP: Conceptualization, Data curation, Formal analysis, Investigation, Methodology, Writing – original draft, Writing – review & editing. CM: Data curation, Formal analysis, Investigation, Methodology, Writing – review & editing. GC: Data curation, Formal analysis, Investigation, Methodology, Writing – review & editing. FP: Data curation, Formal analysis, Investigation, Methodology, Writing – review & editing. SB: Conceptualization, Funding acquisition, Supervision, Validation, Writing – original draft, Writing – review & editing. VS: Conceptualization, Supervision, Validation, Writing – original draft, Writing – review & editing. EM: Conceptualization, Data curation, Formal analysis, Investigation, Methodology, Writing – original draft, Writing – review & editing.
Funding
The author(s) declare financial support was received for the research, authorship, and/or publication of this article. This work was supported by a grant, with the code POC01_00069 from the Italian Ministry of University and Research (MUR) within the program Proof of Concept.
Acknowledgments
We acknowledge the CeSAR (Centro Servizi Ricerca d'Ateneo) core facility of the University of Cagliari and Dr. Marta Costa for assistance with RNA quantification and quality control.
Conflict of interest
The authors declare that the research was conducted in the absence of any commercial or financial relationships that could be construed as a potential conflict of interest.
Publisher's note
All claims expressed in this article are solely those of the authors and do not necessarily represent those of their affiliated organizations, or those of the publisher, the editors and the reviewers. Any product that may be evaluated in this article, or claim that may be made by its manufacturer, is not guaranteed or endorsed by the publisher.
Supplementary material
The Supplementary Material for this article can be found online at: https://www.frontiersin.org/articles/10.3389/fncel.2024.1442786/full#supplementary-material
References
Allan, S. M., Tyrrell, P. J., and Rothwell, N. J. (2005). Interleukin-1 and neuronal injury. Nat. Rev. Immunol. 5, 629–640. doi: 10.1038/nri1664
Angelova, D. M., and Brown, D. R. (2019). Microglia and the aging brain: are senescent microglia the key to neurodegeneration? J. Neurochem. 151, 676–688. doi: 10.1111/jnc.14860
Banni, S., Carta, G., Angioni, E., Murru, E., Scanu, P., Melis, M. P., et al. (2001). Distribution of conjugated linoleic acid and metabolites in different lipid fractions in the rat liver. J. Lipid Res. 42, 1056–1061. doi: 10.1016/S0022-2275(20)31594-7
Batetta, B., Griinari, M., Carta, G., Murru, E., Ligresti, A., Cordeddu, L., et al. (2009). Endocannabinoids may mediate the ability of (n-3) fatty acids to reduce ectopic fat and inflammatory mediators in obese Zucker Rats. J. Nutr. 139, 1495–1501. doi: 10.3945/jn.109.104844
Bazinet, R. P., and Layé, S. (2014). Polyunsaturated fatty acids and their metabolites in brain function and disease. Nat. Rev. Neurosci. 15, 771–785. doi: 10.1038/nrn3820
Belury, M. A., Moya-Camarena, S. Y., Liu, K.-L., and Vanden Heuvel, J. P. (1997). Dietary conjugated linoleic acid induces peroxisome-specific enzyme accumulation and ornithine decarboxylase activity in mouse liver. J. Nutr. Biochem. 8, 579–584. doi: 10.1016/S0955-2863(97)00093-4
Binyamin, O., Nitzan, K., Frid, K., Ungar, Y., Rosenmann, H., and Gabizon, R. (2019). Brain targeting of 9c,11t-conjugated linoleic acid, a natural calpain inhibitor, preserves memory and reduces Aβ and P25 accumulation in 5XFAD mice. Sci. Rep. 9:18437. doi: 10.1038/s41598-019-54971-9
Bogie, J. F. J., Grajchen, E., Wouters, E., Corrales, A. G., Dierckx, T., Vanherle, S., et al. (2020). Stearoyl-CoA desaturase-1 impairs the reparative properties of macrophages and microglia in the brain. J. Exp. Med. 217:e20191660. doi: 10.1084/jem.20191660
Cappa, M., Bizzarri, C., Petroni, A., Carta, G., Cordeddu, L., Valeriani, M., et al. (2012). A mixture of oleic, erucic and conjugated linoleic acids modulates cerebrospinal fluid inflammatory markers and improve somatosensorial evoked potential in X-linked adrenoleukodystrophy female carriers. J. Inherit. Metab. Dis. 35, 899–907. doi: 10.1007/s10545-011-9432-3
Cartier, L., Hartley, O., Dubois-Dauphin, M., and Krause, K.-H. (2005). Chemokine receptors in the central nervous system: role in brain inflammation and neurodegenerative diseases. Brain Res. Rev. 48, 16–42. doi: 10.1016/j.brainresrev.2004.07.021
Chan, A. H., and Schroder, K. (2019). Inflammasome signaling and regulation of interleukin-1 family cytokines. J. Exp. Med. 217:e20190314. doi: 10.1084/jem.20190314
Colonna, M., and Butovsky, O. (2017). microglia function in the central nervous system during health and neurodegeneration. Annu. Rev. Immunol. 35, 441–468. doi: 10.1146/annurev-immunol-051116-052358
Di Cara, F., Andreoletti, P., Trompier, D., Vejux, A., Bülow, M. H., Sellin, J., et al. (2019). Peroxisomes in immune response and inflammation. Int. J. Mol. Sci. 20:3877. doi: 10.3390/ijms20163877
Di Marzo, V., Griinari, M., Carta, G., Murru, E., Ligresti, A., Cordeddu, L., et al. (2010). Dietary krill oil increases docosahexaenoic acid and reduces 2-arachidonoylglycerol but not N-acylethanolamine levels in the brain of obese Zucker rats. Int. Dairy J. 20, 231–235. doi: 10.1016/j.idairyj.2009.11.015
Du, L., Zhang, Y., Chen, Y., Zhu, J., Yang, Y., and Zhang, H.-L. (2017). Role of microglia in neurological disorders and their potentials as a therapeutic target. Mol. Neurobiol. 54, 7567–7584. doi: 10.1007/s12035-016-0245-0
Fa, M., Diana, A., Carta, G., Cordeddu, L., Melis, M. P., Murru, E., et al. (2005). Incorporation and metabolism of c9,t11 and t10,c12 conjugated linoleic acid (CLA) isomers in rat brain. Biochim. Biophys. Acta 1736, 61–66. doi: 10.1016/j.bbalip.2005.06.010
Fan, Z., Liang, Z., Yang, H., Pan, Y., Zheng, Y., and Wang, X. (2017). Tenuigenin protects dopaminergic neurons from inflammation via suppressing NLRP3 inflammasome activation in microglia. J. Neuroinflamm. 14:256. doi: 10.1186/s12974-017-1036-x
Farioli-Vecchioli, S., Moreno, S., and Cerù, M. P. (2001). Immunocytochemical localization of acyl-CoA oxidase in the rat central nervous system. J. Neurocytol. 30, 21–33. doi: 10.1023/A:1011913223541
Folch, J., Lees, M., and Sloane Stanley, G. H. (1957). A simple method for the isolation and purification of total lipides from animal tissues. J. Biol. Chem. 226, 497–509. doi: 10.1016/S0021-9258(18)64849-5
Fujita, Y., Kano, K., Kishino, S., Nagao, T., Shen, X., Sato, C., et al. (2021). Dietary cis-9, trans-11-conjugated linoleic acid reduces amyloid β-protein accumulation and upregulates anti-inflammatory cytokines in an Alzheimer's disease mouse model. Sci. Rep. 11:9749. doi: 10.1038/s41598-021-88870-9
Gama, M. A. S., Raposo, N. R. B., Mury, F. B., Lopes, F. C. F., Dias-Neto, E., Talib, L. L., et al. (2015). Conjugated linoleic acid-enriched butter improved memory and up-regulated phospholipase A2 encoding-genes in rat brain tissue. J. Neural Transm. 122, 1371–1380. doi: 10.1007/s00702-015-1401-9
Haghighatdoost, F., and Nobakht M. Gh, B. F. (2018). Effect of conjugated linoleic acid on blood inflammatory markers: a systematic review and meta-analysis on randomized controlled trials. Eur. J. Clin. Nutr. 72, 1071–1082. doi: 10.1038/s41430-017-0048-z
Henn, A., Lund, S., Hedtjärn, M., Schrattenholz, A., Pörzgen, P., and Leist, M. (2009). The suitability of BV2 cells as alternative model system for primary microglia cultures or for animal experiments examining brain inflammation. Altern. Anim. Exp. 26, 83–94. doi: 10.14573/altex.2009.2.83
Huang, W.-C., Tu, R.-S., Chen, Y.-L., Tsai, Y.-Y., Lin, C.-F., and Liou, C.-J. (2016). Conjugated linoleic acids suppress inflammatory response and ICAM-1 expression through inhibition of NF-κB and MAPK signaling in human bronchial epithelial cells. Food Funct. 7, 2025–2033. doi: 10.1039/C5FO01037C
Hunt, W. T., Kamboj, A., Anderson, H. D., and Anderson, C. M. (2010). Protection of cortical neurons from excitotoxicity by conjugated linoleic acid. J. Neurochem. 115, 123–130. doi: 10.1111/j.1471-4159.2010.06908.x
Jia, D., Heng, L.-J., Yang, R.-H., and Gao, G.-D. (2014). Fish oil improves learning impairments of diabetic rats by blocking PI3K/AKT/nuclear factor-κB-mediated inflammatory pathways. Neuroscience 258, 228–237. doi: 10.1016/j.neuroscience.2013.11.016
Kempuraj, D., Thangavel, R., Natteru, P., Selvakumar, G., Saeed, D., Zahoor, H., et al. (2016). Neuroinflammation induces neurodegeneration. J. Neurol. Neurosurg. Spine 1:1003.
Kharebava, G., Rashid, M. A., Lee, J.-W., Sarkar, S., Kevala, K., and Kim, H.-Y. (2015). N- docosahexaenoylethanolamine regulates Hedgehog signaling and promotes growth of cortical axons. Biol. Open 4, 1660–1670. doi: 10.1242/bio.013425
Kim, D.-I., Kim, K.-H., Kang, J.-H., Jung, E.-M., Kim, S.-S., Jeung, E.-B., et al. (2011a). Trans-10, cis-12-conjugated linoleic acid modulates NF-κB activation and TNF-α production in porcine peripheral blood mononuclear cells via a PPARγ-dependent pathway. Br. J. Nutr. 105, 1329–1336. doi: 10.1017/S000711451000499X
Kim, H.-Y., and Spector, A. A. (2018). N-Docosahexaenoylethanolamine: a neurotrophic and neuroprotective metabolite of docosahexaenoic acid. Mol. Aspects Med. 64, 34–44. doi: 10.1016/j.mam.2018.03.004
Kim, H.-Y., Spector, A. A., and Xiong, Z.-M. (2011b). A synaptogenic amide N-docosahexaenoylethanolamide promotes hippocampal development. Prostagland. Other Lipid Mediat. 96, 114–120. doi: 10.1016/j.prostaglandins.2011.07.002
Laurenzi, M. A., Arcuri, C., Rossi, R., Marconi, P., and Bocchini, V. (2001). Effects of microenvironment on morphology and function of the microglial cell line BV-2. Neurochem. Res. 26, 1209–1216. doi: 10.1023/A:1013911205494
Lee, E., Eom, J.-E., Kim, H.-L., Baek, K. H., Jun, K.-Y., Kim, H.-J., et al. (2013). Effect of conjugated linoleic acid, μ-calpain inhibitor, on pathogenesis of Alzheimer's disease. Biochim. Biophys. Acta 1831, 709–718. doi: 10.1016/j.bbalip.2012.12.003
Leyrolle, Q., Layé, S., and Nadjar, A. (2019). Direct and indirect effects of lipids on microglia function. Neurosci. Lett. 708:134348. doi: 10.1016/j.neulet.2019.134348
Li, Y., Zhang, Y., Wang, Q., Wu, C., Du, G., and Yang, L. (2023). Oleoylethanolamide protects against acute ischemic stroke by promoting PPARα-mediated microglia/macrophage M2 polarization. Pharmaceuticals 16:621. doi: 10.3390/ph16040621
Manca, C., Carta, G., Murru, E., Abolghasemi, A., Ansar, H., Errigo, A., et al. (2021). Circulating fatty acids and endocannabinoidome-related mediator profiles associated to human longevity. GeroScience 43, 1783–1798. doi: 10.1007/s11357-021-00342-0
Matt, S. M., and Johnson, R. W. (2016). Neuro-immune dysfunction during brain aging: new insights in microglial cell regulation. Curr. Opin. Pharmacol. 26, 96–101. doi: 10.1016/j.coph.2015.10.009
Monaco, A., Ferrandino, I., Boscaino, F., Cocca, E., Cigliano, L., Maurano, F., et al. (2018). Conjugated linoleic acid prevents age-dependent neurodegeneration in a mouse model of neuropsychiatric lupus via the activation of an adaptive response. J. Lipid Res. 59, 48–57. doi: 10.1194/jlr.M079400
Murru, E., Carta, G., Manca, C., Sogos, V., Pistis, M., Melis, M., et al. (2021a). Conjugated linoleic acid and brain metabolism: a possible anti-neuroinflammatory role mediated by PPARα activation. Front. Pharmacol. 11:587140. doi: 10.3389/fphar.2020.587140
Murru, E., Lopes, P. A., Carta, G., Manca, C., Abolghasemi, A., Guil-Guerrero, J. L., et al. (2021b). Different dietary N-3 polyunsaturated fatty acid formulations distinctively modify tissue fatty acid and N-acylethanolamine profiles. Nutrients 13:625. doi: 10.3390/nu13020625
Musante, V., Longordo, F., Neri, E., Pedrazzi, M., Kalfas, F., Severi, P., et al. (2008). RANTES modulates the release of glutamate in human neocortex. J. Neurosci. 28, 12231–12240. doi: 10.1523/JNEUROSCI.3212-08.2008
Muzio, L., Viotti, A., and Martino, G. (2021). Microglia in neuroinflammation and neurodegeneration: from understanding to therapy. Front. Neurosci. 15:742065. doi: 10.3389/fnins.2021.742065
Ntambi, J. M., and Miyazaki, M. (2004). Regulation of stearoyl-CoA desaturases and role in metabolism. Prog. Lipid Res. 43, 91–104. doi: 10.1016/S0163-7827(03)00039-0
Park, T., Chen, H., Kevala, K., Lee, J.-W., and Kim, H.-Y. (2016). N-Docosahexaenoylethanolamine ameliorates LPS-induced neuroinflammation via cAMP/PKA-dependent signaling. J. Neuroinflamm. 13:284. doi: 10.1186/s12974-016-0751-z
Park, T., Chen, H., and Kim, H.-Y. (2019). GPR110 (ADGRF1) mediates anti-inflammatory effects of N-docosahexaenoylethanolamine. J. Neuroinflamm. 16:225. doi: 10.1186/s12974-019-1621-2
Poll-The, B. T., Roels, F., Ogier, H., Scotto, J., Vamecq, J., Schutgens, R. B. H., et al. (1988). A new peroxisomal disorder with enlarged peroxisomes and a specific deficiency of Acyl-CoA oxidase (pseudo–Neonatal adrenoleukodystrophy). Am. J. Hum. Genet. 42, 422–434.
Rashid, M. A., Katakura, M., Kharebava, G., Kevala, K., and Kim, H.-Y. (2013). N-docosahexaenoylethanolamine is a potent neurogenic factor for neural stem cell differentiation. J. Neurochem. 125, 869–884. doi: 10.1111/jnc.12255
Rastgoo, S., Shimi, G., Shiraseb, F., Karbasi, A., Ashtary-Larky, D., Yousefi, M., et al. (2023). The effects of conjugated linoleic acid supplementation on inflammatory cytokines and adipokines in adults: a GRADE-assessed systematic review and dose–response meta-analysis. Front. Immunol. 14:1092077. doi: 10.3389/fimmu.2023.1092077
Saba, F., Sirigu, A., Pillai, R., Caria, P., Cordeddu, L., Carta, G., et al. (2019). Downregulation of inflammatory markers by conjugated linoleic acid isomers in human cultured astrocytes. Nutr. Neurosci. 22, 207–214. doi: 10.1080/1028415X.2017.1367130
Salsinha, A. S., Socodato, R., Rodrigues, A., Vale-Silva, R., Relvas, J. B., Pintado, M., et al. (2023). Potential of omega-3 and conjugated fatty acids to control microglia inflammatory imbalance elicited by obesogenic nutrients. Biochim. Biophys. Acta 1868:159331. doi: 10.1016/j.bbalip.2023.159331
Sheppard, O., Coleman, M. P., and Durrant, C. S. (2019). Lipopolysaccharide-induced neuroinflammation induces presynaptic disruption through a direct action on brain tissue involving microglia-derived interleukin 1 beta. J. Neuroinflamm. 16:106. doi: 10.1186/s12974-019-1490-8
Tawbeh, A., Raas, Q., Tahri-Joutey, M., Keime, C., Kaiser, R., Trompier, D., et al. (2023). Immune response of BV-2 microglial cells is impacted by peroxisomal beta-oxidation. Front. Mol. Neurosci. 16:1299314. doi: 10.3389/fnmol.2023.1299314
Vamecq, J., Andreoletti, P., El Kebbaj, R., Saih, F.-E., Latruffe, N., El Kebbaj, M. H. S., et al. (2018). Peroxisomal acyl-CoA oxidase type 1: anti-inflammatory and anti-aging properties with a special emphasis on studies with LPS and argan oil as a model transposable to aging. Oxid. Med. Cell. Longev. 2018:6986984. doi: 10.1155/2018/6986984
Wahle, K. W. J., Heys, S. D., and Rotondo, D. (2004). Conjugated linoleic acids: are they beneficial or detrimental to health? Prog. Lipid Res. 43, 553–587. doi: 10.1016/j.plipres.2004.08.002
Wake, H., Moorhouse, A. J., and Nabekura, J. (2011). Functions of microglia in the central nervous system – beyond the immune response. Neuron Glia Biol. 7, 47–53. doi: 10.1017/S1740925X12000063
Wang, H., Liu, T., Wang, J., Qi, Y., Ge, D., and Guan, S. (2011). Isomer-specific effects of conjugated linoleic acid on proliferative activity of cultured neural progenitor cells. Mol. Cell. Biochem. 358, 13–20. doi: 10.1007/s11010-011-0914-2
Wang, Z., Liu, D., Wang, F., Liu, S., Zhao, S., Ling, E.-A., et al. (2012). Saturated fatty acids activate microglia via toll-like receptor 4/NF-κB signalling. Br. J. Nutr. 107, 229–241. doi: 10.1017/S0007114511002868
Wendimu, M. Y., and Hooks, S. B. (2022). Microglia phenotypes in aging and neurodegenerative diseases. Cells 11:2091. doi: 10.3390/cells11132091
Yang, B., Chen, H., Stanton, C., Ross, R. P., Zhang, H., Chen, Y. Q., et al. (2015). Review of the roles of conjugated linoleic acid in health and disease. J. Funct. Foods 15, 314–325. doi: 10.1016/j.jff.2015.03.050
Yu, Y., Correll, P. H., and Vanden Heuvel, J. P. (2002). Conjugated linoleic acid decreases production of pro-inflammatory products in macrophages: evidence for a PPAR gamma-dependent mechanism. Biochim. Biophys. Acta 1581, 89–99. doi: 10.1016/S1388-1981(02)00126-9
Keywords: conjugated linoleic acid (CLA), microglia, neuroinflammation, N-acylethanolamines (NAEs), inflammatory mediators
Citation: Porcedda C, Manca C, Carta G, Piras F, Banni S, Sogos V and Murru E (2024) Anti-neuroinflammatory effects of conjugated linoleic acid isomers, c9,t11 and t10,c12, on activated BV-2 microglial cells. Front. Cell. Neurosci. 18:1442786. doi: 10.3389/fncel.2024.1442786
Received: 02 June 2024; Accepted: 09 September 2024;
Published: 27 September 2024.
Edited by:
Renato Socodato, University of Porto, PortugalReviewed by:
Francisco Saez-Orellana, San Sebastian University, ChileShun-Ming Ting, University of Texas Health Science Center at Houston, United States
Copyright © 2024 Porcedda, Manca, Carta, Piras, Banni, Sogos and Murru. This is an open-access article distributed under the terms of the Creative Commons Attribution License (CC BY). The use, distribution or reproduction in other forums is permitted, provided the original author(s) and the copyright owner(s) are credited and that the original publication in this journal is cited, in accordance with accepted academic practice. No use, distribution or reproduction is permitted which does not comply with these terms.
*Correspondence: Valeria Sogos, c29nb3NAdW5pY2EuaXQ=