- 1Faculty of Medicine, Institute of Anatomy and Cell Biology, University of Freiburg, Freiburg, Germany
- 2Faculty of Biology, University of Freiburg, Freiburg, Germany
- 3Center for Basics in NeuroModulation (NeuroModulBasics), Faculty of Medicine, University of Freiburg, Freiburg, Germany
Wound healing of the central nervous system (CNS) is characterized by the classical phases of ‘hemostasis’, ‘inflammation’, ‘proliferation’, and ‘remodeling’. Uncontrolled wound healing results in pathological scar formation hindering tissue remodeling and functional recovery in the CNS. Initial blood protein extravasation and activation of the coagulation cascade secure hemostasis in CNS diseases featuring openings in the blood–brain barrier. However, the relevance of blood-derived coagulation factors was overlooked for some time in CNS wound healing and scarring. Recent advancements in animal models and human tissue analysis implicate the blood-derived coagulation factor fibrinogen as a molecular link between vascular permeability and scar formation. In this perspective, we summarize the current understanding of how fibrinogen orchestrates scar formation and highlight fibrinogen-induced signaling pathways in diverse neural and non-neural cells that may contribute to scarring in CNS disease. We particularly highlight a role of fibrinogen in the formation of the lesion border between the healthy neural tissue and the fibrotic scar. Finally, we suggest novel therapeutic strategies via manipulating the fibrinogen–scar-forming cell interaction to improve functional outcomes.
Introduction
Tissue or organ fibrosis is the pathological scarring that occurs when the wound healing pathway is dysregulated (i.e., the appropriate healing response does not stop and becomes chronic). Pathological fibrosis is a common cause of organ failure and a leading cause of death in developed countries (Henderson et al., 2020).
Scar formation in the central nervous system (CNS) is often associated with chronic non-resolving pathology that hinders CNS repair (Silver and Miller, 2004; Cregg et al., 2014; Sofroniew, 2018; Bradbury and Burnside, 2019; Dorrier et al., 2022). The fibrotic scar is conserved across CNS injury and disease models in rodents [e.g., spinal cord injury, optic nerve injury, multiple sclerosis, and stroke (Dias et al., 2021)], suggesting that fibrosis is a common pathology in many CNS disorders. Regardless of the primary insult, fibrotic scar formation in the CNS is driven by stromal cells depositing an excess of the extracellular matrix (ECM) proteins [e.g., collagen I (Col I)] (Dias and Goritz, 2018; Dias et al., 2021; Dorrier et al., 2021, 2022; Fehlberg and Lee, 2022; Holl and Goritz, 2023). The fibrotic scar replaces lost parenchymal cells and is coordinated with the generation of the lesion border reactive astrocytes (Wanner et al., 2013; Anderson et al., 2014). However, why fibrotic scar formation is often not resolved over time and replaced by CNS parenchymal tissue is not understood. A deeper understanding of the underlying mechanisms of CNS scar formation and the cross-communication of the scar-forming cells with reactive astrocytes at the lesion border might lead to new therapeutic strategies to resolve the chronic non-resolving scar and to improve CNS repair.
Recently, our lab advanced understanding of how fibrinogen coordinates the formation of the lesion border by reactive astrocytes separating the fibrotic scar lesion center from the intact brain parenchyma (Conforti et al., 2022). Here, we will summarize our most recent findings on how the blood-derived coagulation factor fibrinogen orchestrates the formation of the astrocyte-fibroblast interface, potentially resulting in formation of a new glia limitans. This process may underlie the failure of repopulating the fibrotic scar with parenchymal cells and resolving the fibrotic lesion in the CNS over time.
A short overview on scar formation in the CNS
The CNS lesion comprises two components. The area of focal tissue damage forms a non-neural fibrotic scar (lesion core), composed of stromal-derived fibroblasts and inflammatory cells. The surrounding penumbra consists of microglial cells and reactive astrocytes (Cregg et al., 2014). Reactive astrocytes are vital for the acute containment of inflammation and prevention of the expansion of inflammatory processes (Bush et al., 1999; Faulkner et al., 2004; Sofroniew, 2005), and these cells ultimately contribute to the chronic failure of axon regeneration (Cregg et al., 2014). Reactive astrogliosis is characterized by gradual changes in astrocytic morphology, molecular expression profile and cell proliferation with respect to distance from the lesion site. In particular, lesion border–forming astrocytes are derived from local, proliferating astrocytes (Wanner et al., 2013) and are uniquely positioned precisely at the interface to the non-neural tissue lesion core and change their physiologically defined individual domains in CNS disease (Wanner et al., 2013; Anderson et al., 2014). Neural stem/precursor cell–derived astrogenesis from the subventricular zone (SVZ) stem cell niche contributes to the reactive astrocytes in the penumbra; however, their exact morphology, localization and function at the lesion site are still not understood (Pous et al., 2020).
The central non-neural “fibrotic” lesion core is composed of stromal cells, which are restricted to vascular and meningeal niches, and CNS-associated macrophages, including perivascular macrophages and peripherally derived macrophages, which together produce an excess of ECM components, such as collagens. Different cell types are proposed to contribute to fibrotic scar formation in the CNS (e.g., type-A pericytes, and fibroblasts) (Göritz et al., 2011; Fernández-Klett et al., 2013; Soderblom et al., 2013; Kelly et al., 2016; Dias and Goritz, 2018; Yahn et al., 2019; Dias et al., 2021; Dorrier et al., 2021, 2022; Fehlberg and Lee, 2022).
Fibrinogen instructing scar formation
The relevance of the blood circulatory system and the coagulation cascade was not considered for a long time in neuroscience, at least with regard to their roles in initiating scar formation. The wound-healing process in the CNS, such as after a stroke, typically begins with the immediate activation of the coagulation cascade to achieve hemostasis. The soluble blood-derived coagulation factor fibrinogen extravasates into the CNS parenchyma upon blood–brain barrier (BBB) disruption, is cleaved by thrombin and, upon conversion to insoluble fibrin, serves as a key matrix of blood clots to enable hemostasis (Adams et al., 2004). Beyond its role in fibrin clot formation, fibrinogen acts as a perivascular matrix to directly interact with all cellular components of the neurovascular unit to influence inflammatory, neurodegenerative and repair processes in the injured CNS (Adams et al., 2007; Schachtrup et al., 2007, 2010, 2011; Petersen et al., 2017; Pous et al., 2020).
Fibrinogen triggers lesion border–forming astrocyte properties
In the CNS, fibrinogen acts as a multi-faceted signaling molecule by interacting with integrin and non-integrin receptors and by functioning as a carrier of growth factors (Adams et al., 2004; Martino et al., 2013; Petersen et al., 2018). We found that fibrinogen triggers astrocyte reactivity by promoting the availability of active TGF-β (Schachtrup et al., 2010) and provokes astrocyte differentiation from neural stem/precursor cells via BMP receptor I (BMP RI) signaling (Pous et al., 2020).
Recently, we reported that fibrinogen deposition guides the reactivity and morphology of astrocytes forming the lesion border (Conforti et al., 2022). Pharmacologic depletion of fibrinogen reduced the deposition of major inhibitory ECM components, such as chondroitin-sulfate proteoglycans, and resulted in a reduced astrocyte activation status. The astrocytes of fibrinogen-depleted mice revealed morphological changes (e.g., absence of flat, elongated scar border-forming astrocytes) and could penetrate the fibrotic lesion core. So far, mechanisms of how reactive astrocytes at the lesion border segregate spatially from the fibrotic lesion core are only sparsely known, but some recent results point toward the environment-dependent plasticity of reactive astrogliosis. Reactive astrocytes segregate from the lesion core via cell contact–mediated bidirectional signaling between astrocytes and fibroblasts (Ephrins and Eph receptors) and via injury-induced Plexin-B2 or type I collagen enriched in the lesion core (Bundesen et al., 2003; Hara et al., 2017; Zhou et al., 2020). Interestingly, we have discovered that fibrinogen after ischemic stroke remains deposited at the lesion border, the interface of fibrotic cells and reactive, border-forming astrocytes, potentially preventing the parenchymal cell penetration into the fibrotic scar. Fibrinogen depletion reduced injury-induced Plexin-B2 expression in the lesion core, suggesting that fibrinogen orchestrates the environment-dependent plasticity of astrocytes and fibrotic cells at the lesion border. We propose that the initial hemostasis and massive fibrinogen deposition in the CNS parenchyma is a major driver and organizer of scar formation and that prolonged fibrinogen deposition at the lesion border establishes the segregation between parenchymal astrocytes and fibrotic cells in the CNS.
Glia limitans and lesion border – similarities and differences
The glia limitans or the glial limiting membrane is a thin barrier of astrocyte foot processes associated with the parenchymal basal lamina surrounding the brain and spinal cord. It is the outermost layer of neural tissue, and among its responsibilities is the prevention of the over-migration of neurons and neuroglia, the supporting cells of the nervous system, into the meninges.
The development of the long astrocyte cellular processes that are integral to the glia limitans structure has been linked to the presence of meningeal cells in the pia mater (Struckhoff, 1995). Astrocytes are bushy star-shaped glial cells in the CNS that, like neurons and oligodendrocytes, arise from a radial glial cell population of ectodermal, neuroepithelial origin surrounding the ventricular zone in the early developing brain (Rowitch and Kriegstein, 2010). In proximity to the pia mater, astrocytes send out processes to form the glia limitans superficialis (Figure 1) (Struckhoff, 1995). A thin barrier of astrocyte endfeet processes is associated with the parenchymal basal lamina via the dystrophin-associated glycoprotein complex, connecting the astrocyte basal membrane to the astrocyte cytoskeleton (Jiang et al., 2002). Fluid and low-molecular-weight tracers pass freely through the glia limitans from the CSF (Iliff et al., 2012), but trafficking of immune cells, such as T cells, is limited (Howell et al., 2015).
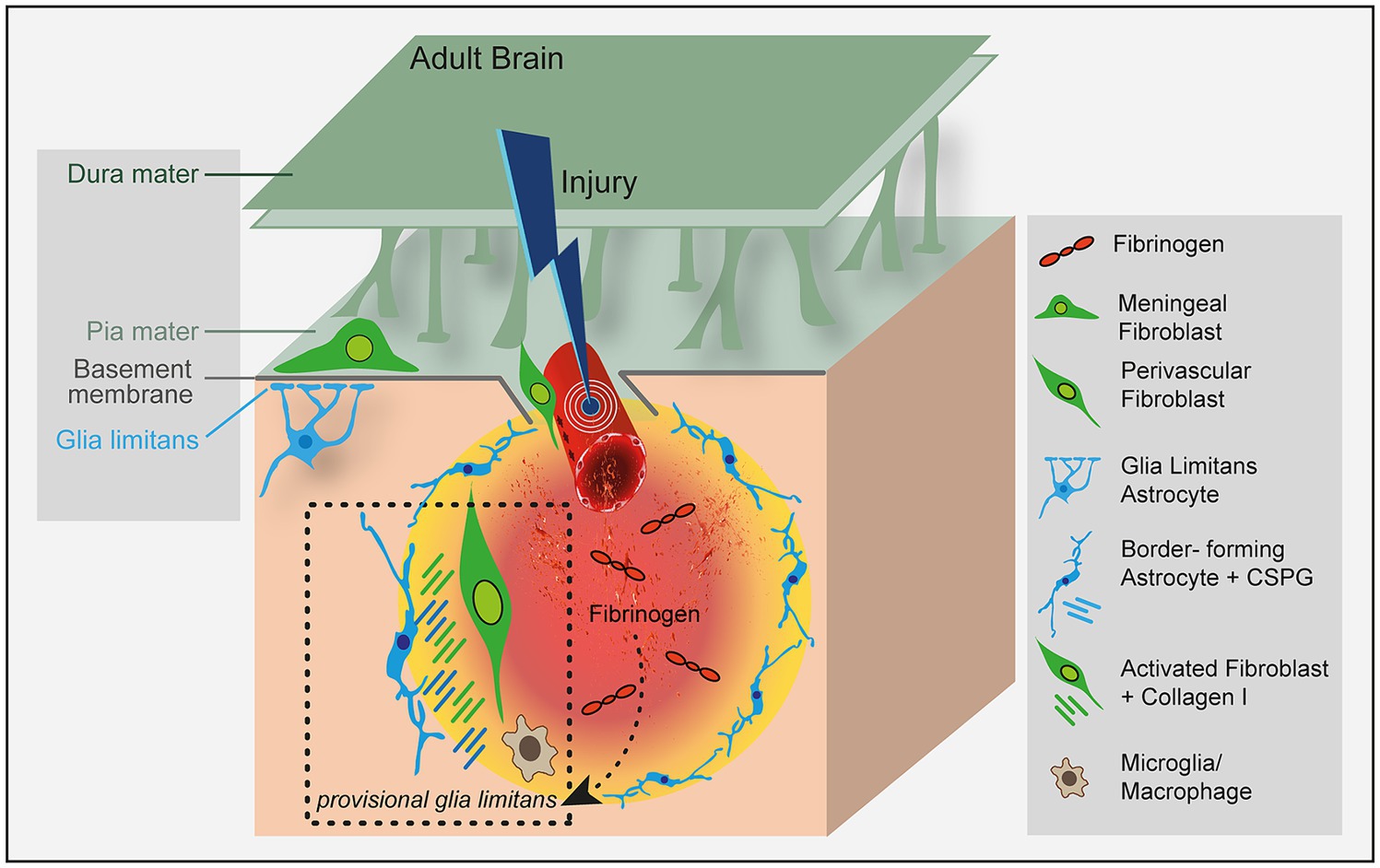
Figure 1. Fibrinogen regulates lesion border formation at the astrocyte-fibroblast interface. Fibrinogen triggers astrocyte reactivity by promoting the availability of active TGF-β (Schachtrup et al., 2010) and altering their morphology and functionality (Conforti et al., 2022) to form the perilesional border. The effects of fibrinogen on mesenchymal cells that contribute to fibrotic scarring have not been studied so far. Fibrinogen might connect the blood circulatory system with CNS scar formation by orchestrating the formation of a provisional glia limitans in CNS disease with BBB openings.
Meningeal fibroblasts in the midbrain, hindbrain and spinal cord originate from the somatic and cephalic mesoderm, whereas those in the forebrain originate from the neural crest (Couly et al., 1992; Jiang et al., 2002; Yoshida et al., 2008). Intensive signaling events between meningeal fibroblasts and the brain regulate cortical development and the formation of the glia limitans superficialis (e.g., pia mater fibroblasts begin to secrete leukemia inhibitory factor, retinoic acid, and BMP4 to initiate astrogliogenesis at E14, forming the glia limitans at the boundaries of the meninges) (Kawamura et al., 2017; Katada et al., 2021). Additionally, astrocyte endfeet form the glia limitans perivascularis, which line the CNS side of the blood brain barrier (BBB) to further separate the CNS from the peripheral circulation; this provides an additional physical barrier to keep peripheral immune cells out of the CNS.
Interestingly, perivascular fibroblasts (PVF) located on the outside of the pericyte or vascular smooth muscle cell (vSMC) layer in the perivascular space of large-diameter arterioles and venules, but not capillaries, oppose the astrocyte endfeet (Figure 1). With the advent of single-cell RNA sequencing (scRNA-seq) technologies, it has become increasingly recognized that PVF show enriched expression for ECM structural components and its modifiers or receptors, suggesting a function in the astrocytic basement membrane formation (Yang et al., 2022). However, whether PVF and astrocytes communicate to form the glia limitans perivascularis is so far unknown. Intensive research has focused on the robust response of astrocytes and fibrotic cells in CNS disease, summarized in excellent recent review articles (Dorrier et al., 2022; Fehlberg and Lee, 2022). However, how astrocytes and fibroblasts communicate and which molecules affect their interaction to form a lesion border in CNS disease are largely unknown.
Lesions in the brain trigger formation of the fibrotic scar in parallel and in coordination with reactive astrogliosis. The resulting interface between the CNS parenchymal tissue and the fibrotic lesion has similarities in appearance and function to the glia limitans formed by perimeningeal astrocytes that interface with stromal cells of the meninges around the entire CNS (Sofroniew, 2020; Wahane and Sofroniew, 2022). A physicochemical barrier composed of chondroitin sulfate proteoglycans, collagen I and dense extracellular matrix behaves as a basement membrane located at the interface between the reactive astrocyte-formed lesion border and the fibrotic scar (Figure 1). After a brain lesion, the breach of the BBB immediately initiates the coagulation cascade and a drastic change in the ECM by deposition of the provisional fibrin matrix (Schachtrup et al., 2007). The provisional fibrin matrix triggers reactive responses of neural parenchymal cells, most notably reactive astrogliosis (Schachtrup et al., 2010; Pous et al., 2020; Conforti et al., 2022) and microgliosis (Adams et al., 2007; Ryu et al., 2018). However, the contribution of fibrinogen to the complex picture of the forming barrier, its underlying molecular mechanisms of the genesis, and its implications for brain repair are only marginally understood.
Fibrinogen – bridging neural and mesenchymal tissue
As we strive to understand the biological complexity of scar tissue formation and tissue fibrosis in the CNS, we clearly cannot study glia, non-neural stromal cells, and the ECM in isolation. A first key change in the molecular composition of the extracellular microenvironment in CNS disease is the abundant extravasation of the plasma protein fibrinogen into the CNS parenchyma through a leaky BBB. Given its pleiotropic functions, fibrinogen may be an apical signal that orchestrates the molecular and cellular composition of the CNS after vascular damage affecting neural and mesenchymal cells to coordinate scar formation.
Human fibrinogen is made up of three pairs of polypeptide chains, namely Aα, Bβ and γ (Lord, 2011). Each chain contains Arg-Gly-Asp (RGD) sequences that serve as attachment sites for multiple integrins and receptors (Petersen et al., 2018). Besides, the αC domains can bind latent TGF-β (Schachtrup et al., 2010) and the dimeric sequence (β15-66)2, which is exposed after fibrinogen conversion into fibrin, binds heparin and growth factors from the PDGF/VEGF, FGF, TGF-β and neurotrophin families (Martino et al., 2013).
Fibrinogen induces growth factor receptor pathway activation in parenchymal astrocytes and SVZ-derived newborn astrocytes to regulate scar formation and astrogliogenesis, respectively (Figure 2; Schachtrup et al., 2010; Conforti et al., 2022). Our studies revealed that fibrinogen triggers parenchymal astrocyte activation in a TGF-β type I receptor-dependent manner and that fibrinogen induces SVZ-derived Thbs4 + NSPC differentiation into newborn reactive astrocytes as well as the formation of astrocyte-like cells from NG2 + oligodendrocyte progenitor cells in a BMP RI–dependent manner. However, depletion of fibrinogen did not affect the proliferation and formation of new (Ki67+/EdU+) local, juxtavascular astrocytes at the lesion site, suggesting that in the lesion area fibrinogen deposition does not affect the overall astrocyte cell number, but its activation status. The fibrinogen-induced changes in morphology and functionality of the different astrocyte subtypes that contribute to repair processes still need to be determined. Furthermore, how fibrinogen exerts biological effects and signaling on mesenchymal cells, including meningeal and perivascular fibroblasts, pericytes and vSMCs to contribute to CNS fibrotic scarring, has not yet been studied (Figure 2).
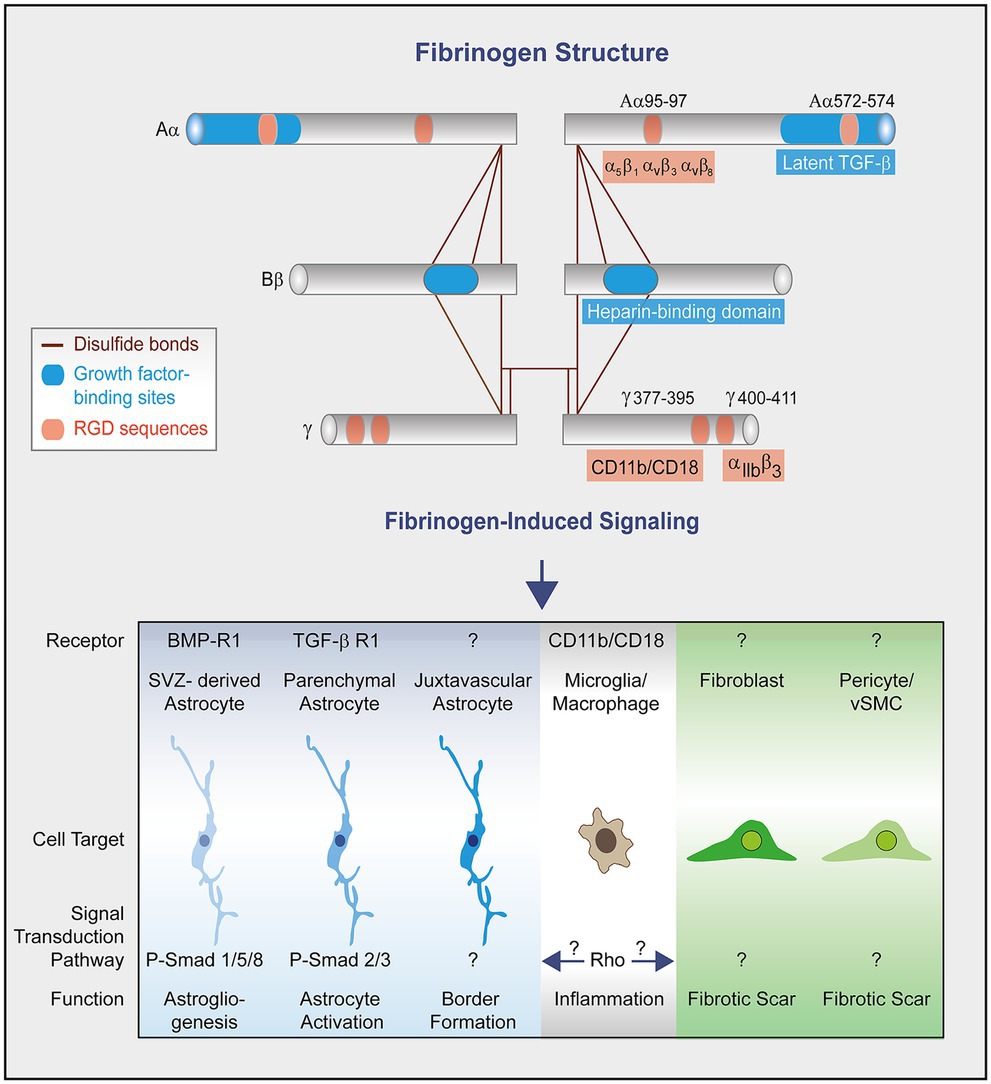
Figure 2. Fibrinogen structure and signaling in scar formation. Fibrinogen is a dimer composed of three distinct polypeptidic chains (Aα, Bβ, and γ) bound by disulfide bonds (Lord, 2011). Each chain contains both RGD sequences and binding sites for growth factors. Fibrinogen chain Aα binds, among other, latent transforming growth factor-β (TGFβ) (Schachtrup et al., 2010) and interacts with the integrin receptors α5β1, αvβ3 and αvβ8 through its Arg-Gly-Asp (RGD) motifs (Smith et al., 1990; Suehiro et al., 2000; Chernousov and Carey, 2003). The dimeric chain Bβ sequence (β15-66)2 in fibrin (heparin binding domain) binds heparin and growth factors from the PDGF/VEGF, FGF, and TGF-β families (Martino et al., 2013). Fibrinogen chain γ binds the integrin receptors CD11b/CD18 (Ugarova et al., 2003) and αIIbβ3 (Weisel, 2005). Fibrinogen activates growth factor receptor pathways in SVZ-derived astrocytes and parenchymal astrocytes to regulate astrogliogenesis and astrocyte activation, respectively (Schachtrup et al., 2010; Pous et al., 2020). The fibrinogen-induced signal pathway to regulate the morphology and functionality of juxtavascular astrocytes to form the lesion border (Conforti et al., 2022) is unknown so far. The conversion of fibrinogen into fibrin exposes cryptic epitopes, such as the γ377–395 epitope, which is the binding site for the CD11b/CD18 integrin receptor and activates microglia/macrophages (Adams et al., 2007). Fibrinogen-induced microglia/macrophage activation might in turn trigger activation of scar forming cells. The effects of fibrinogen on mesenchymal cells (e.g., fibroblasts, pericytes and vascular smooth muscle cells) leading to fibrotic scar formation remain unknown.
Microglia, monocyte-derived macrophages, and immune cell infiltrates are also intimately involved in the scarring process (Bellver-Landete et al., 2019; Li et al., 2020; Zhou et al., 2020; Dorrier et al., 2021). Conversion of fibrinogen into fibrin exposes cryptic epitopes, such as the γ377–395 epitope, which is the binding site for the CD11b/CD18 integrin receptor, inducing microglia and macrophage activation upon receptor binding (Figure 2; Adams et al., 2007). Therefore, fibrin-induced microglia and macrophage activation might affect cell activation and function of other scar forming cells, such as astrocytes, pericytes, and vSMCs, as well as meningeal and perivascular fibroblasts.
Thus, blood-derived fibrinogen with its unique function to form a provisional matrix might instruct neural and mesenchymal tissue to orchestrate scar formation and to connect tissues of different origin at the lesion border potentially forming a new glia limitans.
Conclusion
The molecular triggers for development of the lesion border by reactive astrocytes and the fibrotic scar are poorly described in CNS diseases. However, fibrinogen, which is immediately and massively deposited at the lesion site and which, due its unique molecular structure to interact with the different neural and non-neural cell types, may orchestrate wound healing in the CNS. Cutting-edge techniques to determine the fibrinogen-induced transcriptome and secretome of scar-forming cells over time will provide fundamental information on fibrinogen-driven cell–cell communication in the lesion area and will improve understand of how wound healing in the CNS is organized. Furthermore, genetic or pharmacologic approaches to deplete fibrinogen might be potent tools for understanding how fibrinogen affects the different wound-healing phases hemostasis, inflammation, proliferation, and remodeling in the CNS. The manipulation of fibrinogen-induced molecular cascades on specific cell types driving pathological scarring might create an environment that is more beneficial for promoting repair processes. This was recently shown for a newly generated monoclonal antibody that selectively inhibits fibrin-induced inflammation and oxidative stress without interfering with clotting (Ryu et al., 2018). We hope to foster research into the nature and development of neural-mesenchymal interfaces, with the aim of designing strategies that resolve fibrotic scarring and improve the functional outcome in CNS disease.
Data availability statement
The original contributions presented in the study are included in the article/supplementary material, further inquiries can be directed to the corresponding authors.
Ethics statement
The animal study was approved by Federal Ministry for Nature, Environment and Consumers Protection of the state of Baden-Württemberg. The study was conducted in accordance with the local legislation and institutional requirements.
Author contributions
PC: Conceptualization, Writing – original draft. JM: Conceptualization, Writing – original draft. CS: Conceptualization, Writing – original draft.
Funding
The author(s) declare that financial support was received for the research, authorship, and/or publication of this article. This work was supported by the Heinrich Kircher-Stiftung fellowship to PC, the ZNS – Hannelore Kohl Stiftung fellowship to JS, and the German Research Foundation Grant SCHA 1442/8-1 and SCHA 1442/8-3 to CS.
Acknowledgments
We are grateful to A. Schober for graphics and Gary Howard for language editing.
Conflict of interest
The authors declare that the research was conducted in the absence of any commercial or financial relationships that could be construed as a potential conflict of interest.
Publisher’s note
All claims expressed in this article are solely those of the authors and do not necessarily represent those of their affiliated organizations, or those of the publisher, the editors and the reviewers. Any product that may be evaluated in this article, or claim that may be made by its manufacturer, is not guaranteed or endorsed by the publisher.
References
Adams, R. A., Bauer, J., Flick, M. J., Sikorski, S. L., Nuriel, T., Lassmann, H., et al. (2007). The fibrin-derived gamma377-395 peptide inhibits microglia activation and suppresses relapsing paralysis in central nervous system autoimmune disease. J. Exp. Med. 204, 571–582. doi: 10.1084/jem.20061931
Adams, R. A., Passino, M., Sachs, B. D., Nuriel, T., and Akassoglou, K. (2004). Fibrin mechanisms and functions in nervous system pathology. Mol. Interv. 4, 163–176. doi: 10.1124/mi.4.3.6
Anderson, M. A., Ao, Y., and Sofroniew, M. V. (2014). Heterogeneity of reactive astrocytes. Neurosci. Lett. 565, 23–29. doi: 10.1016/j.neulet.2013.12.030
Bellver-Landete, V., Bretheau, F., Mailhot, B., Vallières, N., Lessard, M., Janelle, M. E., et al. (2019). Microglia are an essential component of the neuroprotective scar that forms after spinal cord injury. Nat. Commun. 10:518. doi: 10.1038/s41467-019-08446-0
Bradbury, E. J., and Burnside, E. R. (2019). Moving beyond the glial scar for spinal cord repair. Nat. Commun. 10:3879. doi: 10.1038/s41467-019-11707-7
Bundesen, L. Q., Scheel, T. A., Bregman, B. S., and Kromer, L. F. (2003). Ephrin-B2 and EphB2 regulation of astrocyte-meningeal fibroblast interactions in response to spinal cord lesions in adult rats. J. Neurosci. 23, 7789–7800. doi: 10.1523/JNEUROSCI.23-21-07789.2003
Bush, T. G., Puvanachandra, N., Horner, C. H., Polito, A., Ostenfeld, T., Svendsen, C. N., et al. (1999). Leukocyte infiltration, neuronal degeneration, and neurite outgrowth after ablation of scar-forming, reactive astrocytes in adult transgenic mice. Neuron 23, 297–308. doi: 10.1016/S0896-6273(00)80781-3
Chernousov, M. A., and Carey, D. J. (2003). alphaVbeta8 integrin is a Schwann cell receptor for fibrin. Exp. Cell Res. 291, 514–524. doi: 10.1016/S0014-4827(03)00409-9
Conforti, P., Mezey, S., Nath, S., Chu, Y. H., Malik, S. C., Martínez Santamaría, J. C., et al. (2022). Fibrinogen regulates lesion border-forming reactive astrocyte properties after vascular damage. Glia 70, 1251–1266. doi: 10.1002/glia.24166
Couly, G. F., Coltey, P. M., and le Douarin, N. M. (1992). The developmental fate of the cephalic mesoderm in quail-chick chimeras. Development 114, 1–15. doi: 10.1242/dev.114.1.1
Cregg, J. M., DePaul, M. A., Filous, A. R., Lang, B. T., Tran, A., and Silver, J. (2014). Functional regeneration beyond the glial scar. Exp. Neurol. 253, 197–207. doi: 10.1016/j.expneurol.2013.12.024
Dias, D. O., and Goritz, C. (2018). Fibrotic scarring following lesions to the central nervous system. Matrix Biol. 68-69, 561–570. doi: 10.1016/j.matbio.2018.02.009
Dias, D. O., Kalkitsas, J., Kelahmetoglu, Y., Estrada, C. P., Tatarishvili, J., Holl, D., et al. (2021). Pericyte-derived fibrotic scarring is conserved across diverse central nervous system lesions. Nat. Commun. 12:5501. doi: 10.1038/s41467-021-25585-5
Dorrier, C. E., Aran, D., Haenelt, E. A., Sheehy, R. N., Hoi, K. K., Pintarić, L., et al. (2021). CNS fibroblasts form a fibrotic scar in response to immune cell infiltration. Nat. Neurosci. 24, 234–244. doi: 10.1038/s41593-020-00770-9
Dorrier, C. E., Jones, H. E., Pintarić, L., Siegenthaler, J. A., and Daneman, R. (2022). Emerging roles for CNS fibroblasts in health, injury and disease. Nat. Rev. Neurosci. 23, 23–34. doi: 10.1038/s41583-021-00525-w
Faulkner, J. R., Herrmann, J. E., Woo, M. J., Tansey, K. E., Doan, N. B., and Sofroniew, M. V. (2004). Reactive astrocytes protect tissue and preserve function after spinal cord injury. J. Neurosci. 24, 2143–2155. doi: 10.1523/JNEUROSCI.3547-03.2004
Fehlberg, C. R., and Lee, J. K. (2022). Fibrosis in the central nervous system: from the meninges to the vasculature. Cell Tissue Res. 387, 351–360. doi: 10.1007/s00441-021-03491-y
Fernández-Klett, F., Potas, J. R., Hilpert, D., Blazej, K., Radke, J., Huck, J., et al. (2013). Early loss of pericytes and perivascular stromal cell-induced scar formation after stroke. J. Cereb. Blood Flow Metab. 33, 428–439. doi: 10.1038/jcbfm.2012.187
Göritz, C., Dias, D. O., Tomilin, N., Barbacid, M., Shupliakov, O., and Frisén, J. (2011). A pericyte origin of spinal cord scar tissue. Science 333, 238–242. doi: 10.1126/science.1203165
Hara, M., Kobayakawa, K., Ohkawa, Y., Kumamaru, H., Yokota, K., Saito, T., et al. (2017). Interaction of reactive astrocytes with type I collagen induces astrocytic scar formation through the integrin-N-cadherin pathway after spinal cord injury. Nat. Med. 23, 818–828. doi: 10.1038/nm.4354
Henderson, N. C., Rieder, F., and Wynn, T. A. (2020). Fibrosis: from mechanisms to medicines. Nature 587, 555–566. doi: 10.1038/s41586-020-2938-9
Holl, D., and Goritz, C. (2023). Decoding fibrosis in the human central nervous system. Am. J. Physiol. Cell Physiol. 325, C1415–C1420. doi: 10.1152/ajpcell.00243.2023
Howell, O. W., Schulz-Trieglaff, E. K., Carassiti, D., Gentleman, S. M., Nicholas, R., Roncaroli, F., et al. (2015). Extensive grey matter pathology in the cerebellum in multiple sclerosis is linked to inflammation in the subarachnoid space. Neuropathol. Appl. Neurobiol. 41, 798–813. doi: 10.1111/nan.12199
Iliff, J. J., Wang, M., Liao, Y., Plogg, B. A., Peng, W., Gundersen, G. A., et al. (2012). A paravascular pathway facilitates CSF flow through the brain parenchyma and the clearance of interstitial solutes, including amyloid beta. Sci. Transl. Med. 4:147ra111. doi: 10.1126/scitranslmed.3003748
Jiang, X., Iseki, S., Maxson, R. E., Sucov, H. M., and Morriss-Kay, G. M. (2002). Tissue origins and interactions in the mammalian skull vault. Dev. Biol. 241, 106–116. doi: 10.1006/dbio.2001.0487
Katada, S., Takouda, J., Nakagawa, T., Honda, M., Igarashi, K., Imamura, T., et al. (2021). Neural stem/precursor cells dynamically change their epigenetic landscape to differentially respond to BMP signaling for fate switching during brain development. Genes Dev. 35, 1431–1444. doi: 10.1101/gad.348797.121
Kawamura, Y., Katada, S., Noguchi, H., Yamamoto, H., Sanosaka, T., Iihara, K., et al. (2017). Synergistic induction of astrocytic differentiation by factors secreted from meninges in the mouse developing brain. FEBS Lett. 591, 3709–3720. doi: 10.1002/1873-3468.12881
Kelly, K. K., MacPherson, A. M., Grewal, H., Strnad, F., Jones, J. W., Yu, J., et al. (2016). Col1a1+ perivascular cells in the brain are a source of retinoic acid following stroke. BMC Neurosci. 17:49. doi: 10.1186/s12868-016-0284-5
Li, Y., He, X., Kawaguchi, R., Zhang, Y., Wang, Q., Monavarfeshani, A., et al. (2020). Microglia-organized scar-free spinal cord repair in neonatal mice. Nature 587, 613–618. doi: 10.1038/s41586-020-2795-6
Lord, S. T. (2011). Molecular mechanisms affecting fibrin structure and stability. Arterioscler. Thromb. Vasc. Biol. 31, 494–499. doi: 10.1161/ATVBAHA.110.213389
Martino, M. M., Briquez, P. S., Ranga, A., Lutolf, M. P., and Hubbell, J. A. (2013). Heparin-binding domain of fibrin(ogen) binds growth factors and promotes tissue repair when incorporated within a synthetic matrix. Proc. Natl. Acad. Sci. USA 110, 4563–4568. doi: 10.1073/pnas.1221602110
Montagne, A., Nikolakopoulou, A. M., Zhao, Z., Sagare, A. P., Si, G., Lazic, D., et al. (2018). Pericyte degeneration causes white matter dysfunction in the mouse central nervous system. Nat. Med. 24, 326–337. doi: 10.1038/nm.4482
Petersen, M. A., Ryu, J. K., and Akassoglou, K. (2018). Fibrinogen in neurological diseases: mechanisms, imaging and therapeutics. Nat. Rev. Neurosci. 19, 283–301. doi: 10.1038/nrn.2018.13
Petersen, M. A., Ryu, J. K., Chang, K. J., Etxeberria, A., Bardehle, S., Mendiola, A. S., et al. (2017). Fibrinogen activates BMP signaling in oligodendrocyte progenitor cells and inhibits Remyelination after vascular damage. Neuron 96, 1003–1012.e7. doi: 10.1016/j.neuron.2017.10.008
Pous, L., Deshpande, S. S., Nath, S., Mezey, S., Malik, S. C., Schildge, S., et al. (2020). Fibrinogen induces neural stem cell differentiation into astrocytes in the subventricular zone via BMP signaling. Nat. Commun. 11:630. doi: 10.1038/s41467-020-14466-y
Rowitch, D. H., and Kriegstein, A. R. (2010). Developmental genetics of vertebrate glial-cell specification. Nature 468, 214–222. doi: 10.1038/nature09611
Ryu, J. K., Rafalski, V. A., Meyer-Franke, A., Adams, R. A., Poda, S. B., Rios Coronado, P. E., et al. (2018). Fibrin-targeting immunotherapy protects against neuroinflammation and neurodegeneration. Nat. Immunol. 19, 1212–1223. doi: 10.1038/s41590-018-0232-x
Schachtrup, C., Le Moan, N., Passino, M. A., and Akassoglou, K. (2011). Hepatic stellate cells and astrocytes: stars of scar formation and tissue repair. Cell Cycle 10, 1764–1771. doi: 10.4161/cc.10.11.15828
Schachtrup, C., Lu, P., Jones, L. L., Lee, J. K., Lu, J., Sachs, B. D., et al. (2007). Fibrinogen inhibits neurite outgrowth via beta 3 integrin-mediated phosphorylation of the EGF receptor. Proc. Natl. Acad. Sci. USA 104, 11814–11819. doi: 10.1073/pnas.0704045104
Schachtrup, C., Ryu, J. K., Helmrick, M. J., Vagena, E., Galanakis, D. K., Degen, J. L., et al. (2010). Fibrinogen triggers astrocyte scar formation by promoting the availability of active TGF-beta after vascular damage. J. Neurosci. 30, 5843–5854. doi: 10.1523/JNEUROSCI.0137-10.2010
Silver, J., and Miller, J. H. (2004). Regeneration beyond the glial scar. Nat. Rev. Neurosci. 5, 146–156. doi: 10.1038/nrn1326
Smith, J. W., Ruggeri, Z. M., Kunicki, T. J., and Cheresh, D. A. (1990). Interaction of integrins alpha v beta 3 and glycoprotein IIb-IIIa with fibrinogen. Differential peptide recognition accounts for distinct binding sites. J. Biol. Chem. 265, 12267–12271. doi: 10.1016/S0021-9258(19)38340-1
Soderblom, C., Luo, X., Blumenthal, E., Bray, E., Lyapichev, K., Ramos, J., et al. (2013). Perivascular fibroblasts form the fibrotic scar after contusive spinal cord injury. J. Neurosci. 33, 13882–13887. doi: 10.1523/JNEUROSCI.2524-13.2013
Sofroniew, M. V. (2005). Reactive astrocytes in neural repair and protection. Neuroscientist 11, 400–407. doi: 10.1177/1073858405278321
Sofroniew, M. V. (2018). Dissecting spinal cord regeneration. Nature 557, 343–350. doi: 10.1038/s41586-018-0068-4
Sofroniew, M. V. (2020). Astrocyte reactivity: subtypes, states, and functions in CNS innate immunity. Trends Immunol. 41, 758–770. doi: 10.1016/j.it.2020.07.004
Struckhoff, G. (1995). Cocultures of meningeal and astrocytic cells--a model for the formation of the glial-limiting membrane. Int. J. Dev. Neurosci. 13, 595–606. doi: 10.1016/0736-5748(95)00040-N
Suehiro, K., Mizuguchi, J., Nishiyama, K., Iwanaga, S., Farrell, D. H., and Ohtaki, S. (2000). Fibrinogen binds to integrin alpha(5)beta(1) via the carboxyl-terminal RGD site of the Aalpha-chain. J. Biochem. 128, 705–710. doi: 10.1093/oxfordjournals.jbchem.a022804
Ugarova, T. P., Lishko, V. K., Podolnikova, N. P., Okumura, N., Merkulov, S. M., Yakubenko, V. P., et al. (2003). Sequence gamma 377-395(P2), but not gamma 190-202(P1), is the binding site for the alpha MI-domain of integrin alpha M beta 2 in the gamma C-domain of fibrinogen. Biochemistry 42, 9365–9373. doi: 10.1021/bi034057k
Wahane, S., and Sofroniew, M. V. (2022). Loss-of-function manipulations to identify roles of diverse glia and stromal cells during CNS scar formation. Cell Tissue Res. 387, 337–350. doi: 10.1007/s00441-021-03487-8
Wanner, I. B., Anderson, M. A., Song, B., Levine, J., Fernandez, A., Gray-Thompson, Z., et al. (2013). Glial scar borders are formed by newly proliferated, elongated astrocytes that interact to corral inflammatory and fibrotic cells via STAT3-dependent mechanisms after spinal cord injury. J. Neurosci. 33, 12870–12886. doi: 10.1523/JNEUROSCI.2121-13.2013
Weisel, J. W. (2005). Fibrinogen and fibrin. Adv. Protein Chem. 70, 247–299. doi: 10.1016/S0065-3233(05)70008-5
Yahn, S. L., Li, J., Goo, I., Gao, H., Brambilla, R., and Lee, J. K. (2019). Fibrotic scar after experimental autoimmune encephalomyelitis inhibits oligodendrocyte differentiation. Neurobiol. Dis. 134:104674. doi: 10.1016/j.nbd.2019.104674
Yang, A. C., Vest, R. T., Kern, F., Lee, D. P., Agam, M., Maat, C. A., et al. (2022). A human brain vascular atlas reveals diverse mediators of Alzheimer's risk. Nature 603, 885–892. doi: 10.1038/s41586-021-04369-3
Yoshida, T., Vivatbutsiri, P., Morriss-Kay, G., Saga, Y., and Iseki, S. (2008). Cell lineage in mammalian craniofacial mesenchyme. Mech. Dev. 125, 797–808. doi: 10.1016/j.mod.2008.06.007
Keywords: CNS disease, blood–brain barrier opening, fibrinogen, astrocyte activation, lesion border, fibrosis, perivascular fibroblast
Citation: Conforti P, Martínez Santamaría JC and Schachtrup C (2024) Fibrinogen: connecting the blood circulatory system with CNS scar formation. Front. Cell. Neurosci. 18:1402479. doi: 10.3389/fncel.2024.1402479
Edited by:
Dirk M. Hermann, University of Duisburg-Essen, GermanyReviewed by:
Shigetaka Yoshida, Asahikawa Medical University, JapanRichard Milner, San Diego Biomedical Research Institute, United States
Copyright © 2024 Conforti, Martínez Santamaría and Schachtrup. This is an open-access article distributed under the terms of the Creative Commons Attribution License (CC BY). The use, distribution or reproduction in other forums is permitted, provided the original author(s) and the copyright owner(s) are credited and that the original publication in this journal is cited, in accordance with accepted academic practice. No use, distribution or reproduction is permitted which does not comply with these terms.
*Correspondence: Christian Schachtrup, Y2hyaXN0aWFuLnNjaGFjaHRydXBAYW5hdC51bmktZnJlaWJ1cmcuZGU=
†ORCID: Christian Schachtrup, https://orcid.org/0000-0001-9851-6299