- 1Department of Brain and Behavioral Sciences, University of Pavia, Pavia, Italy
- 2Digital Neuroscience Center, IRCCS Mondino Foundation, Pavia, Italy
Schizophrenia (SZ) is a complex neuropsychiatric disorder associated with severe cognitive dysfunction. Although research has mainly focused on forebrain abnormalities, emerging results support the involvement of the cerebellum in SZ physiopathology, particularly in Cognitive Impairment Associated with SZ (CIAS). Besides its role in motor learning and control, the cerebellum is implicated in cognition and emotion. Recent research suggests that structural and functional changes in the cerebellum are linked to deficits in various cognitive domains including attention, working memory, and decision-making. Moreover, cerebellar dysfunction is related to altered cerebellar circuit activities and connectivity with brain regions associated with cognitive processing. This review delves into the role of the cerebellum in CIAS. We initially consider the major forebrain alterations in CIAS, addressing impairments in neurotransmitter systems, synaptic plasticity, and connectivity. We then focus on recent findings showing that several mechanisms are also altered in the cerebellum and that cerebellar communication with the forebrain is impaired. This evidence implicates the cerebellum as a key component of circuits underpinning CIAS physiopathology. Further studies addressing cerebellar involvement in SZ and CIAS are warranted and might open new perspectives toward understanding the physiopathology and effective treatment of these disorders.
1 Introduction
Schizophrenia (SZ) is a complex neuropsychiatric syndrome affecting approximately 1% of the population worldwide. Although it is considered a low-prevalence illness, the burden of SZ is substantial and ranks among the top 10 causes of disability globally (Owen et al., 2016; Charlson et al., 2018; Marder and Cannon, 2019). SZ was traditionally classified into several categories, such as paranoid, hebephrenic, undifferentiated, residual, catatonic, and simple. In 2013, a significant change was made with the release of the fifth edition of the Diagnostic and Statistical Manual of Mental Disorders (DSM-5). Following debate, DSM-5 abandoned the traditional subtypes due to several factors and controversies concerning their clinical utility and reliability. The field shifted away from subtyping toward a broader diagnostic framework called “schizophrenia spectrum disorder” due to the need for a more comprehensive dimensional approach to understanding the heterogeneity of SZ. This spectrum reflects the broader conceptual framework outlined in the DSM-5, which recognizes a spectrum of related conditions beyond classical SZ, includes diagnoses such as schizoaffective disorder, schizophreniform disorder, and others, acknowledging the diversity within psychotic disorders. This allows healthcare professionals to diagnose the condition based on the severity of symptoms (American Psychiatric Association, 2013). Symptoms typically appear during the late teenage or early adulthood, mainly among men, while it becomes prevalent in women from age 40 onwards (Charlson et al., 2018; Velligan and Rao, 2023). The onset of SZ during early neurodevelopment establishes it as a neurodevelopmental disorder (Fatemi and Folsom, 2009). The symptoms, course, prognosis, and treatment efficacy vary from patient to patient. It encompasses a range of symptoms, including hallucinations and delusions (positive), lack of emotion, joy, and motivation (negative), and impaired memory, attention, learning, and decision-making (cognitive) (Patel et al., 2014; Queirós et al., 2019). Among these, the “Cognitive Impairment Associated with SZ” (CIAS) is core, accounting for much of the impaired functioning associated with the disorder not responsive to existing therapies (McCutcheon et al., 2023). This may result in higher rates of co-incidence of medical and/or mental illnesses, such as substance abuse, mainly alcohol and cannabis consumption, with prevalence rates up to 41.7% (Hunt et al., 2018), and more likely to get health complications, including cardiovascular disorders (Nielsen et al., 2021), diabetes (Mamakou et al., 2018), immune-related disorders (Pouget et al., 2019), endocrine dysfunctions (Misiak et al., 2021), and respiratory diseases (Suetani et al., 2021). Consequently, SZ patients show higher mortality rates than healthy individuals (Correll et al., 2022). Given the complex nature and wide range of variables involved, the etiology of SZ is multifaceted and requires extensive investigation and an integrated approach. Nonetheless, recent neurobiological research has implicated several factors in the development of SZ (Orsolini et al., 2022), including:
(i) Genetic: SZ is a highly heritable disease, with several genetic alterations implicated in its onset and development, including copy number variants (CNVs), genetic mutations, risk genes, gene polymorphism, and single nucleotide polymorphism, which mainly affect brain functionality during pre-pubertal and pubertal age (DeLisi, 2022; Owen et al., 2023). Family and twin studies show an incidence risk of around 80% (Sullivan et al., 2003; Lichtenstein et al., 2009), while the remaining 20% is attributed to non-heritable factors, including environmental, stochastic, and de novo mutations risk factors. It is worth noting that the 22q11.2 deletion (a type of CNV) showed the highest effective size among SZ patients (Owen et al., 2023). The expression of specific gene alleles of the major histocompatibility complex (MHC) has been related to alterations in white matter microstructure within tracts innervating the frontal lobe (Emily Simmonds et al., 2023), particularly on chromosome 6 and 19, influencing axonal density in tracts connecting to the frontal lobe. This suggests that alterations in axonal packing, driven by MHC risk alleles, might represent a neurobiological mechanism in SZ. Family cohorts, linkage studies, and genome-wide association studies (GWAS) led to identifying multiple loci-related SZ risks (Allen et al., 2008; DeLisi, 2009; Ripke et al., 2020).
(ii) Epigenetic: Epigenetic alterations involve modifications to gene expression influenced by external cues, and are hypothesized to act as a mediator of environmental risk factors (see iii) involved in SZ pathophysiology (Rivollier et al., 2014; Chen et al., 2021). Epigenome-wide association study approaches led to the discovery of genetic loci that undergo differential epigenetic regulation (Perzel Mandell et al., 2021). Neurodevelopmental dysfunction, which is hypothesized as a significant contributor to SZ beyond genetic influences, was initially proposed by Weinberger, who posits that genetic predisposition and environmental insults during gestation alter the neurodevelopmental process and are latent until the maturational changes of adolescent exposure to earlier neurodevelopmental abnormalities (Weinberger, 1987). These findings underscore the complexity of SZ etiology, wherein both genetic and environmental factors interact to shape neurodevelopmental trajectories and contribute to structural and functional alterations in different brain regions. Sustained brain development into young adulthood emphasizes vulnerability until typical SZ onset. The “two-hit” and “multiple hits” hypotheses propose that the chance of developing SZ increases with exposure to several risk factors that alter essential processes during ongoing development (Davis et al., 2016). A comprehensive analysis in a cohort of 381 SZ patients showed a significant contribution of DNA methylation alterations to the phenotypic diversity including cognitive deficits (Kiltschewskij et al., 2023). These factors may lead to structural/functional alterations in different brain regions.
(iii) Environmental: Several environmental factors, including abnormal fetal development and low birth weight, pregnancy-related diabetes, preeclampsia, other birthing complications, maternal malnutrition and vitamin D deficiency during pregnancy, winter births (which are associated with a 10% higher relative risk), social environment, urban residence, childhood trauma or stress are implicated in the development of SZ (Jones, 2013; Löhrs and Hasan, 2019; Crossley et al., 2021; King et al., 2023). Moreover, immune dysfunctions and neuro-inflammatory processes seem to play a role in SZ pathogenesis. Studies evidenced the involvement of neuro-inflammation (Müller, 2018) and autoinflammation in SZ (Delunardo et al., 2016) but microglia activation has not been confirmed by PET studies in humans (Marques et al., 2019). Compared with healthy individuals, SZ patients have an older brain for their chronological age and have the most pronounced acceleration of brain aging based on the model of frontal features (Kaufmann et al., 2019). Thus, early aging negatively affects the brain volume causing an age gap that is considered a potential biomarker of SZ (Man et al., 2021). Moreover, structural and functional abnormalities in some brain regions potentially cause deviations in the brain aging trajectory (Ballester et al., 2023). On the contrary, data from 26 cohorts suggested that advanced structural brain aging among SZ is not associated with specific clinical characteristics (Constantinides et al., 2023).
Several therapeutic strategies exist to reduce the symptoms, including antipsychotics, psychosocial interventions, electroconvulsive therapy, and alternative and complementary therapies (Correll et al., 2023). However, each approach has significant limitations (Khandaker et al., 2015; Stępnicki et al., 2018). Since the existing therapies may only be effective for 50% of patients, the estimated life expectancy of SZ is 15–20 years shorter than that of the general population (Correll et al., 2022), and it mostly addresses positive symptoms rather than negative and cognitive symptoms and causes multiple neurological and metabolic complications (Stępnicki et al., 2018; Robbins, 2019). Therefore, understanding SZ’s mechanisms and alterations is crucial for developing novel, mechanism-based therapies.
Brain imaging techniques have shown abnormalities in different brain regions (Karlsgodt et al., 2010; Zhao et al., 2018; Khalil et al., 2022; Sone et al., 2022). The alterations include reduction in brain volume, particularly in frontal and temporal regions, abnormal connectivity between brain nodes, and altered neurotransmitter activity (Wright et al., 2000; Dean, 2002; Cannon et al., 2003; Karlsgodt et al., 2010; McCutcheon et al., 2020). Decades ago, Andreasen and colleagues, developed a model that implicates the connectivity among brain nodes located in prefrontal regions, thalamic nuclei, and cerebellum, suggesting that disruption in this circuitry produces “cognitive dysmetria” (Andreasen et al., 1998), encompasses difficulty in prioritizing, processing, coordinating, and responding to information. Subsequently, the cerebellum started to attract attention due to its potential role in the SZ physiopathology.
The cerebellum, historically known to control movement and motor coordination, gaining importance also for its involvement in different cognitive, affective, and social functions (D'Angelo and Casali, 2012; Schmahmann, 2019; Jacobi et al., 2021; Supplementary material). Failure of cerebellar functioning determines the so-called cerebellar cognitive affective syndrome (CCAS) (Schmahmann and Sherman, 1998) in addition to ataxia. Furthermore, studies exploring the organization of cerebro-cortical pathways have shown intricate connections between the cerebellum and various regions of the brain involved in high cognitive functions (Palesi et al., 2015, 2017; Schmahmann, 2019; Jacobi et al., 2021). The concept of the universal cerebellar transform suggests that the cerebellum expresses a fundamental computational capability that extends beyond motor processing (D'Angelo and Casali, 2012). This notion is in line with the dysmetria of thought theory, proposing that the cerebellum participates in cognitive operations by fine-tuning the timing and coordination of mental processes (Andreasen and Pierson, 2008; Yeganeh-Doost et al., 2011; Bernard and Mittal, 2015; Cao and Cannon, 2019; Schmahmann, 2019). Growing evidence suggests that cerebellar abnormalities play a central role in the pathophysiology of SZ (Andreasen and Pierson, 2008; Yeganeh-Doost et al., 2011; Bernard and Mittal, 2015; Cao and Cannon, 2019) by influencing cortical processing (Andreasen and Pierson, 2008; Yeganeh-Doost et al., 2011; D'Mello et al., 2015; Mapelli J. et al., 2022).
In the last 50 years, the number of PubMed articles including the terms “schizophrenia” and “cognitive impairment” has increased (Figure 1A), while adding “cerebellum” it remains notably smaller (Figure 1B). This cannot solely be due to the time gap (the cerebellum hypothesis in CIAS was introduced 25 years ago) highlighting the need for further investigating the field. In this review, we first summarize recent literature about brain abnormalities in CIAS and then explore the involvement of cerebellum, emphasizing structural and functional abnormalities, along with alterations in neurotransmitter systems and connectivity.
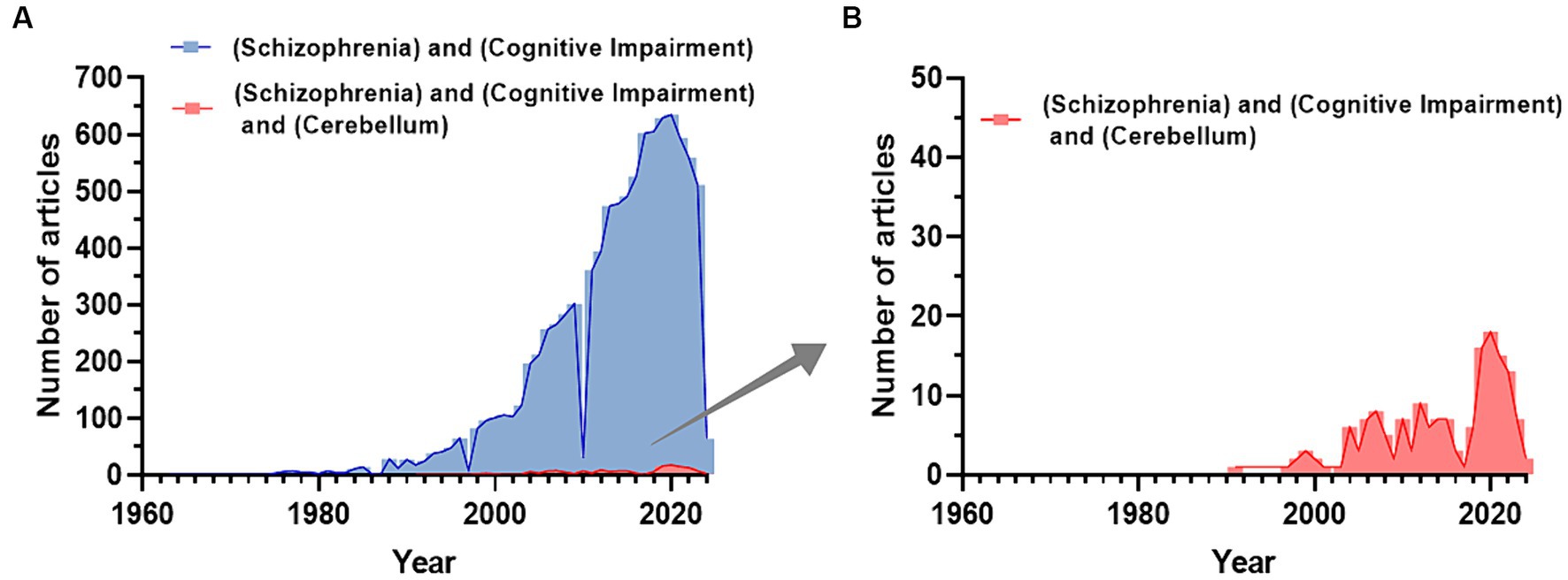
Figure 1. Trend of publications about CIAS on PubMed. (A) Number of publications related to the keywords “Schizophrenia and Cognitive Impairment” extracted from PubMed. (B) The number of publications related to the keywords “Schizophrenia and Cognitive Impairment and Cerebellum” extracted from PubMed. Publications including the cerebellum start to appear with a delay of about 30 years and reach a maximum of about 1/30 of the total publications on SZ and Cognitive impairment (Figures are created with the GraphPad prism 8).
2 Neural dysfunction in cognitive impairment associated with SZ (CIAS): the classical view
Factor analyses of the Measurement and Treatment to Improve Cognition in SZ test battery identified seven cognitive domains: (1) processing speed, (2) attention, (3) working memory, (4) verbal learning and memory, (5) visual learning and memory, (6) reasoning, and (7) social cognition and executive functions (Nuechterlein et al., 2004). Eventually, dimensionality reduction suggests that these seven domains can be reduced to the parent domains of processing speed, attention/working memory, and learning (Burton et al., 2013) bearing relevant cerebellar implications (see below). Among these, processing speed is the most affected domain in SZ. However, it is associated with antipsychotic treatments, and the severity of the impairment does not differ from that observed in verbal and working memory (Knowles et al., 2010; Yeganeh-Doost et al., 2011; D'Angelo and Casali, 2012; Fatouros-Bergman et al., 2014). Considering the time course of CIAS, the overall cognitive impairment is detectable during childhood, while the severity of verbal and nonverbal deficits increases throughout the first two decades of life (Mollon et al., 2018). These deficits can be observed in the first episode and are more severe among the clinical high-risk group. However, cognitive symptoms may manifest before the stabilization of psychotic symptoms during adolescence, and evidence suggests they could even be evident before the onset of psychosis. Notably, the decline of cognitive processes throughout the illness is considered a defining feature of SZ (Hedges et al., 2022). Indeed, most cognitive decline over two decades post-hospitalization often exceeds normal aging. This underscores the importance of focusing on cognition as a therapeutic target during later stages of psychotic illness (Fett et al., 2020). As cognitive shortages are present before the prodromal period and persist throughout the development of the disease, they could be a potent biomarker and target for early detection and prevention. The severity of cognitive impairment is greater in SZ compared to other psychiatric disorders. A meta-analysis suggested more severe cognitive symptoms, particularly in attention and social cognition, among SZ patients compared to bipolar disorder (Li et al., 2020).
Twin and GWAS studies showed a strong negative correlation between liability for SZ and cognitive function (Toulopoulou et al., 2007; Davies et al., 2018; Savage et al., 2018). Additionally, several deficits in the ability to detect sarcasm were observed among mono and heterozygous twin groups as compared to healthy co-twin. However, impairments were also observed in the unaffected homozygous co-twins, indicating that socio-cognitive deficits could be a genetic vulnerability indicator of the illness. The socio-cognitive decline was associated with lower intelligence and higher levels of psychopathology among SZ patients (Lemvigh et al., 2022). Several genes have been correlated with CIAS, due to their implication in modeling and shaping neuronal plasticity, including DISC1, NRG1, AKT1, and DTNBP1, which influence cognitive abilities in SZ (Tripathi et al., 2018). These alterations were detected at both cellular (neuron and glia) and circuit levels (Millan et al., 2012). Nevertheless, the genetic underpinnings of cognitive abilities do not imply a direct link. For instance, when the individual has less access to educational opportunities, the phenotype-associated alleles might negatively correlate with cognitive capacity. Therefore, it is fundamental to consider environmental influences too. Aberrant communication between brain regions and processing in cortical columns are the core pathology of CIAS and are thought to involve alterations in synaptic plasticity and network connectivity. Alterations in gamma band activity were correlated with SZ susceptibility, indicating that shifts in synaptic function and neuronal firing patterns are of pathophysiological relevance rather than consequences of this disorder (Dimitriadis et al., 2021). Alpha and beta band activity was correlated with disrupted temporal connectivity in (para) limbic areas and associated with reduced signal memory and higher variability across time in SZ patients (Alamian et al., 2020). Several neuronal-based alterations seem to contribute to CIAS, including abnormalities in neurotransmitter systems, structural/functional changes (Karlsgodt et al., 2010; Zhao et al., 2018; Sone et al., 2022), impaired synaptic plasticity, and deviations in neural oscillations. Consequently, multiple neurophysiological and neurochemical models were proposed in CIAS (Javitt, 2023).
In this section, we present relevant studies on neuromodulator abnormalities and related hypotheses in CIAS, focusing on cerebral regions following the classical view. The potential role of the cerebellum will be discussed in the subsequent section of this review.
2.1 Abnormal neurotransmitter systems
Several neurotransmitters have been implicated in SZ pathogenesis, but whether their alterations are causative, compensatory, or simply consequential remains unclear. SZ patients have altered levels and activity of neurotransmitter systems, especially dopamine, acetylcholine (Ach), serotonin, glutamate, and GABA (Mandal et al., 2022), Impacting multiple brain circuits through disruption of the Excitatory/Inhibitory (E/I) balance (Liu et al., 2021). Notably, dysfunctions primarily involve the alteration of dopaminergic control. Nevertheless, other neurotransmitter systems appear altered and implicated in CIAS. Here, we illustrate the relevant neurotransmitter hypotheses related to CIAS, particularly those implicated in the forebrain and midbrain regions (Figure 2) and set the basis for SZ physiopathology and pharmacotherapy. Issues related to the cerebellum are considered in the next section.
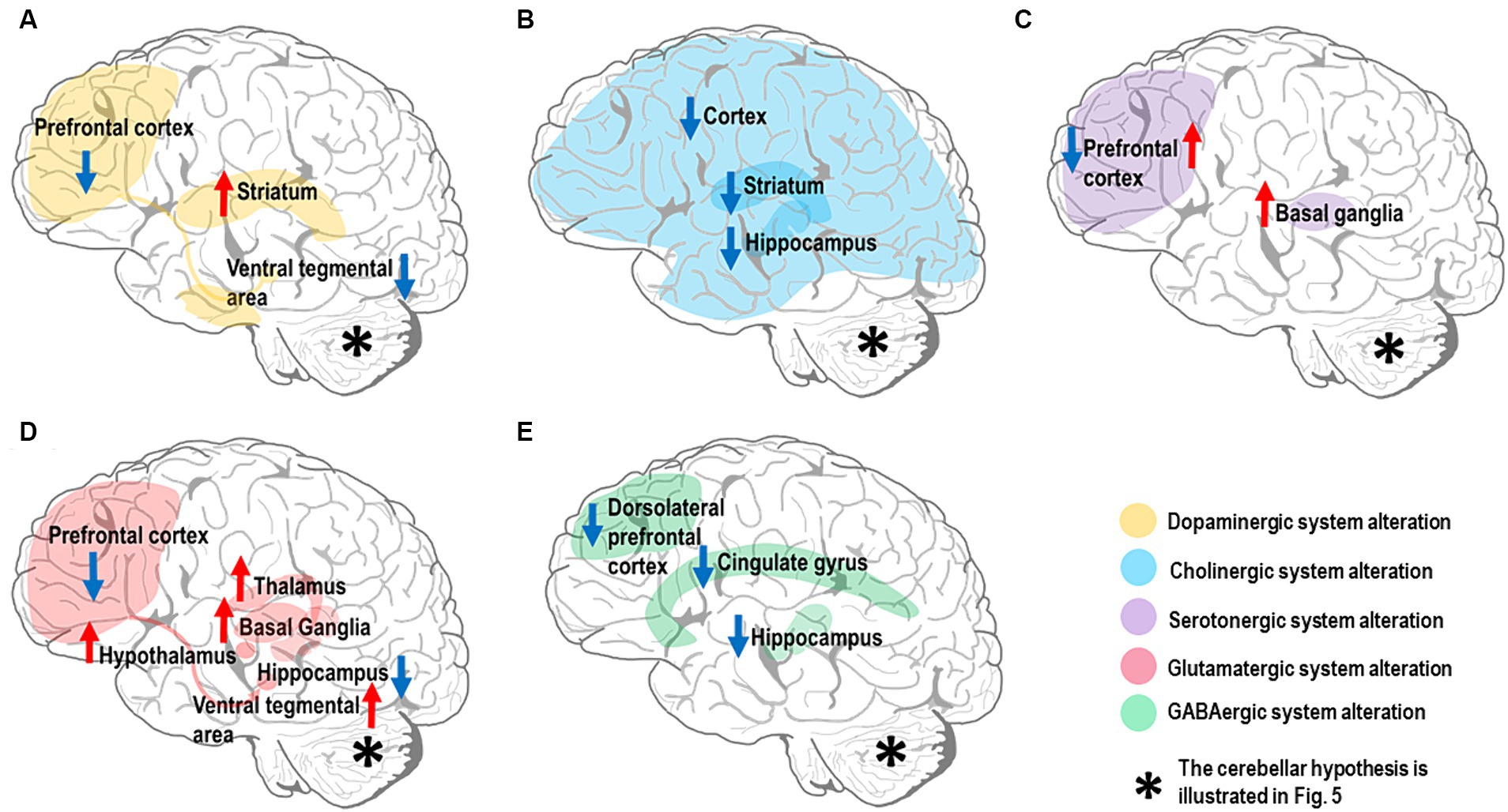
Figure 2. Neurotransmitter alterations in the SZ brain. The most affected brain regions are shown in different colors depending on the neurotransmitter system they belong to. Hyperfunctioning and hypofunctioning regions are identified with red and blue arrows, respectively. Note that the cerebellar neurotransmitter hypothesis is illustrated in Figure 5. (A) Dopamine system: there is evidence for dopamine hypofunction in prefrontal cortex and ventral tegmental area (VTA) (mesocortical pathway). In contrast, there is dopamine hyperfunction in the striatum (mesolimbic pathway). (B) Cholinergic system: there is evidence for alteration of the cholinergic system in multiple brain regions, including downregulation of muscarinic receptors (M1 and M4) in both cerebral cortex and striatum, along with reduction of cholinergic signaling in the hippocampus. (C) Serotonergic system: there is evidence for high serotonin levels and low 5-HT2 receptor density in the prefrontal cortex, while the serotonin level and its metabolites are elevated in basal ganglia. (D) Glutamatergic system: there is evidence for multiple alterations in the brain glutamatergic system; NMDARs in prefrontal cortex and iGlutRs in hippocampus are hypofunctional, while excessive glutamate release is detected in basal ganglia, thalamus, hypothalamus, and VTA. (E) GABAergic system: there is evidence for decreased GABA concentration in the cingulate gyrus, particularly dorsal anterior cingulate cortex, and dorsolateral prefrontal cortical GABA-neurons; a low α5-GABAARs expression level is found in the hippocampus.
2.1.1 Neurotransmitter models
Dopamine plays a central role in SZ pathophysiology (Howes and Kapur, 2009) as shown by rich experimental evidence (Simpson et al., 2010; McCutcheon et al., 2019, 2020; Selten and Ormel, 2023). Current findings underscore dopamine’s central role in CIAS (Slifstein et al., 2015; Simpson and Kellendonk, 2017). The dopamine hypothesis was developed post-hoc to account for the serendipitous discovery of the anti-psychotic effect of some dopaminergic drugs (Carlsson and Lindqvist, 1963; Creese et al., 1996), while genetic support is limited (Edwards et al., 2016). The dopaminergic hypothesis can be summarized as the combination of two main effects: (1) excessive dopamine activity in the mesolimbic pathway (Simpson et al., 2010; Elert, 2014; McCutcheon et al., 2019), specifically in the striatum, disrupts the neurotransmitter balance impairing the functioning of other brain regions involved in cognitive processing; (2) reduced dopamine activity in the mesocortical pathway, connecting the ventral tegmental area (VTA) to the prefrontal cortex (PFC), is linked to both negative symptoms and CIAS (Brisch et al., 2014; Elert, 2014; Slifstein et al., 2015; Simpson and Kellendonk, 2017; Figure 2A). The dual dopamine hypothesis (Elert, 2014; McCutcheon et al., 2020) also opens an issue for the functioning of dopaminergic antipsychotics, whose efficacy is largely linked to their affinity for the D2R distributed both in cortical and subcortical regions (Toda and Abi-Dargham, 2007; Howes et al., 2012; de Bartolomeis et al., 2023). Although the dopamine hypothesis is central to SZ, its dysregulation in the striatum and cerebral cortex is just one aspect of the complex pathophysiology of SZ. The cerebellum, whose dopaminergic system is starting to unveil, is also probably key to CIAS, as explained below.
Another relevant system for SZ is the cholinergic one. Ach plays a vital role in cognitive functions, and pharmacological manipulation targeting the Ach system influences attention, episodic, working, and spatial memories (Newman et al., 2012). Several experimental results converge toward the hypothesis of cholinergic hypofunctioning (Figure 2B) in SZ. Indeed, notable changes in the cholinergic system linked to CIAS, including (1) reduced cholinergic activity, (2) altered receptor functions (especially M1 and M4 mAChRs) (Crook, Tomaskovic-Crook et al., 2000, Carruthers et al., 2015), and (3) disrupted cholinergic-dopaminergic interactions (Foster et al., 2021). Although direct evidence on cerebellar cholinergic alterations in CIAS is scarce, its investigation could open new potential avenues.
Another neurotransmitter implicated in CIAS is serotonin or 5-hydroxytryptamine (5-HT) (Eggers, 2013). Extensive evidence points to serotonergic hypofunctioning, suggesting that targeting the serotonin system might provide a viable treatment for CIAS. Thus, a comprehensive understanding of the mechanisms involved is crucial for designing effective treatments (Figure 2C). The conflicting findings on serotonin alterations, high level (Zhang et al., 2011), low 5-HT2 receptor density, and altered enzyme activity underscore the complexity of its role in CIAS, suggesting potential treatments through serotonin system modulation. However, there are still gaps in understanding broader neural circuits beyond the forebrain and midbrain regions, crucial for addressing cognitive deficits. Thus, considering the serotonergic system in cerebellum (Oostland and van Hooft, 2013) and its influence on other neurotransmitters, consequently, cognitive processes, might further reveal how serotonin dysfunction interacts with CIAS (Section 3.2.3).
The incomplete effectiveness of current antipsychotics suggests that alterations in these systems do not account for most of the negative and cognitive symptoms. Therefore, a glutamatergic model of SZ has been proposed. Elert attributed altered concentrations of dopamine in different brain regions to glutamate dysregulation (Elert, 2014), specifically, glutamate receptors in SZ patients are compromised preventing glutamate from binding to them and dysregulating the function of GABAergic inhibitory interneurons. The lack of inhibition, eventually, causes excessive dopamine in the nucleus accumbens, resulting in positive symptoms of SZ, and reduced dopamine concentration in PFC, leading to negative symptoms (Elert, 2014) (please note that, in Elert’s work, cognitive symptoms are considered together with the negative ones). Extensive evidence, combined with preclinical findings, supports the notion that alterations in the glutamatergic system, including neurotransmitter and transporter high levels in different brain regions (Matute et al., 2005; Merritt et al., 2019, 2021; Adams et al., 2022) and reduced NMDAR function (NMDAR hypofunction) (Gonzalez-Burgos and Lewis, 2012; Moghaddam and Javitt, 2012), play a crucial role in the development of CIAS, thus placing the glutamate system dysfunction at the core of SZ (Swanton, 2020; Figure 2D). Experimental evidence points also to GABAergic system alteration in SZ (Fatemi and Folsom, 2015). Specifically, Glutamic Acid Decarboxylase 67 (GAD67) downregulation (Fatemi et al., 2005; Gonzalez-Burgos and Lewis, 2012; Fujihara, 2023), low GABA level (Nakahara et al., 2022), receptor hypofunction (Marques et al., 2021) and downregulation, and transmission deficits are linked to CIAS (Dienel et al., 2023), emphasizing the potential of targeting the GABAergic system for improving CIAS. For a review of the glutamatergic and GABAergic hypothesis together see Yeganeh-Doost et al. (2011). Given the fundamental role of the glutamatergic and GABAergic systems of cerebellum, these will be considered below for their potential contribution to CIAS (Sections 3.2.4, 3.2.5).
Based on glutamatergic and GABAergic alterations, an altered E/I balance can eventually explain CIAS (Jelen et al., 2019, Liu et al., 2021, Gawande et al., 2023). Restoring the E/I balance and addressing abnormalities in glutamatergic and GABAergic neurotransmission may offer a potential target to improve cognitive function. In particular, the cerebellar E/I balance, potentially involved in various cognitive processes (Sections 1.2, 1.3 in Supplementary material), is a strong candidate to explore CIAS.
2.1.2 Pharmacological implications
Pharmacologically, multiple strategies are exploited to attack the neurotransmitter systems from different sites to ameliorate SZ positive and negative symptoms. The main drugs used are called typical (e.g., haloperidol and chlorpromazine) or atypical (e.g., olanzapine and risperidone), depending on whether they act on the dopaminergic system or also/exclusively on the others. Typical drugs are dopaminergic inhibitors, mostly acting on D2R, ameliorating the positive symptoms but often worsening the negative ones. Given their poor selectivity, these drugs also lead to side effects, including extrapyramidal disturbances, hyperprolactinemia, cognitive decline (Li et al., 2016; Orzelska-Górka et al., 2022), sedation, and cardiovascular issues (Stępnicki et al., 2018). Atypical antipsychotics, instead, address both positive and negative symptoms by affecting various other receptors and have fewer side effects. This is potentially due to their lower D2R affinity or to their preference for mesolimbic over nigrostriatal pathway receptors (Stępnicki et al., 2018; Orzelska-Górka et al., 2022). Consequently, attempts to address cognitive and negative symptoms are growing, rather than exclusively targeting D2R in the dopaminergic system that mainly ameliorates positive symptoms. While atypical drugs might rescue negative symptoms, their primary efficacy remains in targeting positive ones. We argue that recent knowledge on cerebellar physiology will allow for considering new avenues for antipsychotic drug actions and neuromodulation (e.g., transcranial magnetic stimulation, TMS) in CIAS (Section 4.2).
2.2 Abnormal synaptic plasticity
Synaptic plasticity is the ability of synapses, connections between neurons, to endure structural and functional changes in response to stimuli. Short-term changes transiently affect local dynamics of neurotransmitter release and postsynaptic receptor activation, while long-term changes involve biochemical modifications of membrane receptors and ionic channels along with cytoplasmic and nuclear gene regulation, modifying the expression of membrane proteins and the formation/pruning of synapses. Long-term changes occur in the form of either long-term potentiation (LTP) or long-term synaptic depression (LTD). In most cases, these changes involve AMPA and NMDA receptors (Citri and Malenka, 2008), a fact particularly relevant to SZ, in which NMDARs seem to play a central role (Krystal et al., 1994; Verma and Moghaddam, 1996; Catts et al., 2016). In SZ, large-scale gene expression analyses showed minor but significant differences in genes associated with synaptic functioning in post-mortem brain tissue of SZ versus control subjects (Jaffe et al., 2018). Moreover, structural and functional alterations of neuronal circuits have been observed (Wu et al., 2012), such as receptor modifications, dendritic spine adjustments, and postsynaptic density size reduction (Konopaske et al., 2014; McCollum et al., 2015). It was suggested that the synaptic changes and functional dysconnectivity observed in SZ patients are linked to E/I imbalance at the level of cortical microcircuitry, which influences cortical synchrony at the macroscale level (Stephan et al., 2006; Yizhar et al., 2011). Synchronized neural oscillations, in turn, influence cortical network plasticity (Huerta and Lisman, 1993; Singer and Gray, 1995) and are crucial for cognitive functions. Combined alterations in synaptic transmission, long-term synaptic plasticity, and synchronous oscillations seem to underpin CIAS (Uhlhaas and Singer, 2010). Altered presynaptic Ca2+ signaling was proposed to dysregulate LTP and to play a role in CIAS (Nanou and Catterall, 2018; Pereda et al., 2019; Wu et al., 2022).
Several lines of evidence support the role of altered synaptic plasticity in CIAS. LTP and LTD proved to be affected both in clinical and preclinical subjects (Wu et al., 2022) and were significantly associated with the course of the disease (Hasan et al., 2011). TMS and transcranial direct current stimulations (tDCS) revealed impaired LTP-like plasticity due to dysfunctional NMDAR and GABAR (Hasan et al., 2011; Hamilton et al., 2020). In rodents with psychotic symptoms induced by MK-801, LTP following high-frequency stimulation is disrupted (Frankiewicz et al., 1996; Obi-Nagata et al., 2019). This effect may be related to NMDAR hypofunction in GABAergic neurons resulting in E/I imbalance and impaired synaptic plasticity, and results in a range of cognitive deficits, including attention, memory, and learning, while also contributing to hallucinations and delusions (Nakazawa and Sapkota, 2020).
Beyond functional evidence, genetic markers of synaptic plasticity showed alterations in neural cell adhesion molecule-1, Neurotropin-3, and Matrix-metalloproteinase-9 in CIAS (Keshri and Nandeesha, 2023). Large-scale gene expression studies evidenced a reduction in the presynaptic protein synaptophysin in hippocampus, frontal cortex, and cingulate cortex (Osimo et al., 2019). PET imaging revealed that synaptic vesicle glycoprotein 2A, widely expressed in presynaptic terminals and synaptic vesicles, was reduced in different brain regions in SZ, and was associated with either positive or cognitive symptoms (Onwordi et al., 2021; Radhakrishnan et al., 2021). Post-mortem and GWAS findings were confirmed by transcriptomic and proteomic data obtained from patient-derived induced pluripotent stem cells (Santarriaga et al., 2023).
In summary, functional and genetic findings obtained using multiple techniques support alteration in synaptic transmission and plasticity in SZ (Abashkin et al., 2021; Nascimento et al., 2022) opening the question on how and when these changes happen. During neurodevelopment, genetic and environmental factors make synapses vulnerable to stress-triggered glia-mediated elimination, disrupting neuron function and worsening symptoms like psychosis (Howes et al., 2023). This can be especially relevant to SZ, typically emerging during adolescence or adulthood. Since neuronal plasticity is a critical factor implicated in CIAS, it may be targeted to improve synaptic plasticity and cognitive performance in SZ (Mould et al., 2021). Again, the cerebellum presents major plastic mechanisms that might be relevant for CIAS and will be considered below (Section 3.2).
2.3 Abnormal connectivity
Dysconnectivity refers to disruption in communication and coordination between brain regions. The disconnection hypothesis (Friston et al., 2016) posits that a failure of functional integration occurs in the SZ brain. Accordingly, the alterations of structural and functional connectivity within and between brain regions is a core hypothesis of CIAS (Repovs et al., 2011; Frangou, 2014; Canu et al., 2015; Adhikari et al., 2019; Uyy et al., 2020). The analysis of resting state (RS) networks implicated in cognitive control, task set maintenance, attention, and error processing (Fair et al., 2009) suggested that not only the cerebral cortex, primarily the prefrontal and limbic cortex, but also subcortical hubs, including cerebellum, thalamus, and basal ganglia, play an important role in the pathogenesis of SZ (Andreasen et al., 1996; Andreasen and Pierson, 2008; van den Heuvel and Fornito, 2014; Rolls et al., 2020). An fMRI study demonstrated that connectivity alterations are circuit-specific, with prefrontal-limbic hypoconnectivity and primary-sensorimotor hyperconnectivity extending consistently across subcortical nuclei (Avram et al., 2018). However, variations are reported depending on the stage of development of the disease (Kochunov et al., 2017; Anhøj et al., 2018; Sharma et al., 2018; Chen et al., 2019; Hu et al., 2023).
Dysconnectivity could explain distinct symptom dimensions (Avram et al., 2018) and correlated with social cognition, reasoning/problem-solving, and working memory capabilities (Zarghami et al., 2023). Dysconnectivity has been also related to specific neurotransmitter systems. A PET study suggested that aberrant striatal dopamine and cortico-thalamic connectivity are physiologically related within dopamine-modulated cortico-basal ganglia-thalamic circuits in SZ. Moreover, the disconnection between medial PFC and the dorsal hippocampus was related to CIAS in a rodent model of NMDAR hypofunction and was partially rescued by Risperidone (one of the most prescribed atypical antipsychotic drugs primarily targeting D2R and 5-HT2AR receptors and known to improve executive function, attention, learning, and memory) (Delgado-Sallent et al., 2023).
In conclusion, brain dysconnectivity plays a vital role in the pathophysiology of SZ, affecting multiple brain networks and contributing to cognitive impairment. A primary role is apparent for brain circuits involving associative areas, including the prefrontal, temporal, and limbic cortex. Nonetheless, findings across studies show a range of changes, including reduced connectivity in some networks (thalamic-frontal, left frontoparietal, lateral and medial visual, sensorimotor, DMN, and auditory) and increased connectivity in others (right central executive, right ventral attention, subcortical nuclei networks). Moreover, dysconnectivity correlates with distinct symptom dimensions and is associated with specific neurotransmitter systems. The involvement of cerebellum, among the subcortical regions, has also emerged and will be considered in Section 3.3.
2.4 Abnormal neurodevelopment
The neurodevelopmental deficit is a fundamental concept in the pathophysiology of SZ and provides an ontogenetic framework for modifications in neurotransmitters, connectivity, and synaptic plasticity. Weinberger hypothesized that SZ symptoms, despite appearing in early adulthood, stem from environmental and genetic factors causing abnormal prenatal brain development (Weinberger, 1987). The onset of the illness occurs during a vulnerable period in adolescence when neural alterations may be activated (Fatemi and Folsom, 2009). Synaptic formation and maintenance occur during the second and third trimester of pregnancy, then synaptic connectivity develops during childhood. These ontogenetic changes are crucial for learning, memory, and brain functioning. Alterations in synaptic development can lead to SZ and ASD (Hall and Bray, 2022). The onset of SZ in adolescence can be related to the “plasticity switch” secondary to the peripubertal brain maturational changes, caused by modifications in the glutamatergic system. The loss of plasticity could result in social and non-social cognitive deficits (Keshavan and Hogarty, 1999). Synaptic pruning with excessive elimination of synapses and loss of synaptic plasticity alters microconnectivity and can lead to the emergence of symptoms in the predisposed brain. Another possible mechanism is myelination of the heteromodal association cortex that proceeds postnatally. During adolescence (Peters et al., 2012), aberrant myelination and oligodendrocyte number may contribute to connectivity dysfunction in SZ.
The neurodevelopmental hypothesis explains why prodromal symptoms of SZ start during adolescence. Likewise, individuals who will later develop SZ may exhibit non-specific indications of mild brain dysfunction before the onset of the disease, which can be observed as subtle motor abnormalities or cognitive impairments (Cuesta et al., 2018). Cognitive decline continues from the first episode through the chronic stage (Bonner-Jackson et al., 2010; Sheffield et al., 2018). Although cognitive deterioration is common in all stages of SZ, deficiencies in executive functions (e.g., learning, processing speed, organization) are more common in the chronic stage (Stone and Seidman, 2016). In clinical high-risk SZ adolescents, progressive grey matter reduction in the right superior frontal, middle frontal, and medial orbitofrontal cortical regions, as well as a greater rate of expansion of the third ventricle, were observed (Cannon et al., 2015). This was replicated in a subsequent meta-analysis (Ding et al., 2019). In addition, white matter abnormalities, pointing to a neurodevelopmental pathology, were observed (Seitz-Holland et al., 2023).
Genetic alterations are thought to lay at the basis of the aberrant neurodevelopmental processes in SZ and are evaluated using the polygenic risk score and its correlations with clinical and anatomo-functional parameters (Cattarinussi et al., 2022; Fernandez-Cabello et al., 2022).
A factor that could operate during pregnancy is maternal infection with the consequent immune activation that impairs dendritic spine development and synaptic plasticity (Pekala et al., 2021). Reduced synaptic plasticity along with reduced dendritic spines, decreased expression of synaptic genes, and abnormal synaptic neurotransmission have been reported in SZ (Berdenis van Berlekom et al., 2020). Indeed, reduced dendritic spine density (together with reduced parvalbumin interneurons) is a characteristic histopathological feature of SZ. The loss of microconnectivity can cause aberrant myelination, impaired connectivity, and cognitive deficits (Valdés-Tovar et al., 2022; Wu et al., 2022). The interaction between genetic and environmental insults linked to the neurodevelopmental model in SZ has been recently reviewed in detail (Schmitt et al., 2023). Prenatal and perinatal complications, childhood trauma, and maternal immune activation interact with genetic susceptibility to shape neurodevelopmental trajectories and increase the risk of developing SZ (Schmitt et al., 2023).
Relevant to this review, a recent Polygene score analysis evidenced the role of the cerebellum and its connectivity in neurodevelopmental psychiatric disease, suggesting that the genetic patterning for child psychopathology is distinct from that for adults, and implicates fetal cerebellar development (Hughes et al., 2023). A better evaluation of cerebellar neurodevelopmental abnormalities is warranted, given the identification of over 1,000 genes in the cerebellum related to neurodevelopmental disorders (Sepp et al., 2024).
3 The potential role of cerebellum in CIAS
Following the identification of the CCAS (Schmahmann and Sherman, 1998; Schmahmann, 2016; Argyropoulos et al., 2020) and the core hypothesis on cognitive dysmetria (Andreasen et al., 1998; Andreasen and Pierson, 2008), new evidence calls for updating the role of cerebellum in CIAS (see Figure 3 and Sections 1.1, 1.2, 1.3 in Supplementary material for details on cerebellar anatomy and physiology):
1. Cognitive domain: The multiscale analysis of circuit operations supports the cerebellar involvement in the main cognitive domains of CIAS, attention, learning, decision-making (D'Angelo and Casali, 2012), akin to the involvement of cerebellum in cognitive, emotional, and behavioral control (Ciapponi et al., 2023; Supplementary Figure S1).
2. Processing speed: The cerebellum contributes substantially to mechanisms of CIAS, like processing speed, by allowing mental processing to move from controlled to automatic mode (Wong et al., 2021).
3. Connectivity: The cerebellum shows tight bidirectional connectivity with associative areas involved in CIAS, especially the PFC (Palesi et al., 2015, 2017, 2018).
4. Microcircuit level: Cerebellar functioning relies on a delicate regulation of the internal E/I balance, which appears altered in CIAS (D'Angelo and De Zeeuw, 2009; Mapelli et al., 2014; Nieus et al., 2014; Perez-Garcia, 2015; De Schepper et al., 2022).
5. Whole brain level: The cerebellum controls the functioning and rhythms of the cerebral cortex (Popova and Naumenko, 2013; Margarint et al., 2020), which show relevant alterations in CIAS.
6. Neuromodulation systems: The cerebellum is emerging as part of complex regulatory systems that subtend CIAS and are based on dopamine (Ikai et al., 1994; Carta et al., 2019; D'Angelo, 2019; Cutando et al., 2022; Kimura et al., 2023), Ach (Jaarsma et al., 1997; Zhang et al., 2016; Okkels et al., 2023; Zhao et al., 2023), and 5HT (Oostland and van Hooft, 2013; Saitow et al., 2013). Moreover, the cerebellum hosts among the most important NMDA receptor-dependent neurotransmission and plasticity mechanisms in the brain, addressing the glutamatergic hypothesis of CIAS (Hansel et al., 2001; Contestabile, 2002; Bouvier et al., 2016; Mapelli L. et al., 2022).
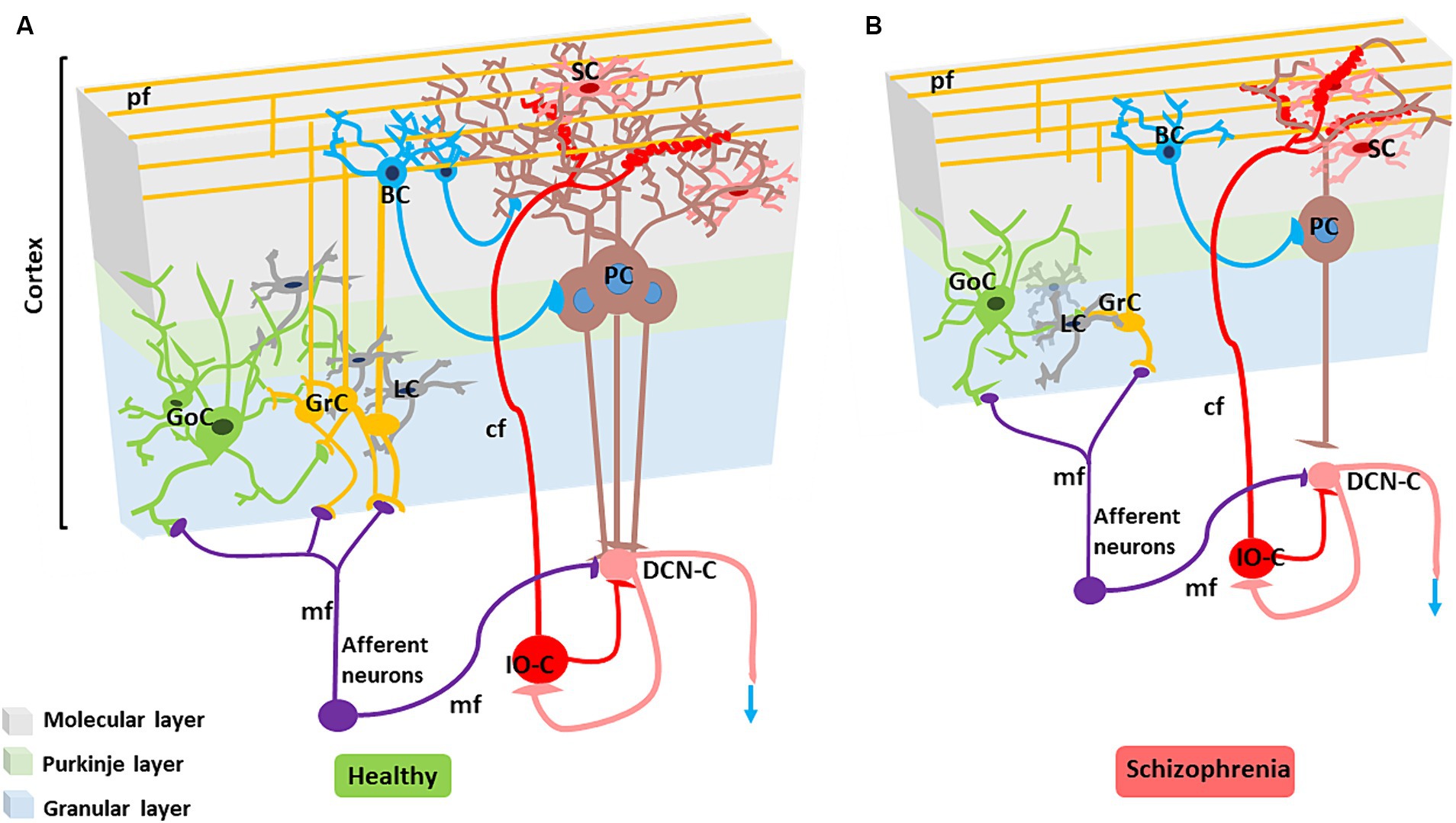
Figure 3. Alterations in the SZ cerebellar microcircuit. (A) Healthy cerebellar microcircuit: The simplified circuit scheme includes cortical (grey area) and subcortical structures. Afferent fibers activate the cerebellar cortex as well as DCN cells (DCN-C) and IO cells (IO-C), then the DCN emits the output and inhibits the IO. The cerebellar cortex is therefore a large side loop controlling DCN activity. The cerebellar cortex contains various types of neurons, primarily granule cells (GrC), Golgi cells (GoC), Purkinje cells (PCs), stellate cells (SC) and basket cells (BC). Other neurons, including Lugaro cells, unipolar brush cells, candelabrum cells, and globular cells are not shown. The two primary inputs are mossy fibers (mf), originating from various brainstem and spinal cord nuclei, and climbing fibers (cf) originating from the IO. Signals conveyed through the mossy fibers diverge, activating the DCN and the granular layer (containing GrC and GoC). The ascending axon of the GrC bifurcates in the molecular layer (containing PC, SC, and BC), forming the parallel fibers (pf). The cerebellar cortical circuit consists in a forward excitatory neuronal chain forming multiple inhibitory loops: mossy fibers excite GrCs, which subsequently activate all the other cortical elements. In the granular layer, inhibition is provided by GoC, and in the molecular layer by SC and BC. Finally, PCs inhibit the DCN. The IO, which is also activated by brainstem and spinal cord projections, controls PC activity through a single powerful synapse. Consequently, the entire system can be seen as a complex mechanism regulating the DCN output. Re-drawn from D'Angelo et al. (2016). (B) Cerebellar microcircuit in schizophrenia condition: The figure illustrates the main alterations reported in the SZ cerebellar microcircuit. The grey matter and white matter thickness are decreased. Microscopic alterations include reduced density of the main neuronal populations (PCs, GrCs, inhibitory interneurons), reduced PC dendritic branching, altered synaptic vesicular transport (not shown), increased connectivity at climbing fiber/PC synapses, disconnection between PC and neuronal populations in the DCN.
3.1 Cerebellar structural and functional abnormalities and CIAS
Cerebellar Crus I- II, VIIB, and, to a lesser extent, VIIIA and VI, are linked to executive functions and cognitive control and deficits in tasks requiring flexibility, inhibition, and goal-directed behavior can emerge from damage to these areas (Schmahmann and Sherman, 1998; Argyropoulos et al., 2020). Posterior cerebellar lesions are associated with deficits in executive functions that resemble those observed in prefrontal lesions, including impairment in planning, verbal fluency, working memory, problem-solving, and multi-task performance and organization (Schmahmann and Sherman, 1998, Argyropoulos et al., 2020), while lesions in vermis and paravermis regions are associated with behavior alteration and mood disturbance. Likewise, cerebellar degeneration can cause impairments of executive function, working memory, and perceptual processing (Kansal et al., 2017). Verbal and phonemic fluency, working memory, cognitive flexibility, immediate and delayed recall, verbal learning, and visuomotor coordination were variably associated with lobule VI, Crus I- II, VII B, and/or IX, whereas immediate, and delayed recall show associations with the anterior lobe.
Cerebellar abnormalities in psychosis are tied not only to a specific diagnosis or illness stage but also to developmental factors and premorbid cognitive disturbances (Moussa-Tooks et al., 2022). Cerebellar dysfunction has been repeatedly correlated with CIAS (Andreasen and Pierson, 2008; Dean and Porrill 2014; Ding et al., 2019, Kim et al., 2021). Neuroimaging studies reported smaller cerebellar volume, altered intra-cerebellar and cerebellar-cerebral RS functional connectivity, and reduced cerebellar activation during cognitive tasks (Zhuo et al., 2018; He et al., 2019; Kim et al., 2021; Lundin et al., 2021; Moussa-Tooks et al., 2022). On a microscopic scale, neuropathological changes include lower Purkinjie Cell (PC) density and reduced distal and terminal dendritic branches (Mavroudis et al., 2017). PCs and granular cells (GrCs) are key in maintaining the E/I balance but, in SZ, deficits in the development of PCs and GrCs (and possibly also in other cell types) could alter the E/I balance required for cerebellar network functioning (Perez-Garcia, 2015; Figure 3).
First-episode SZ patients exhibit reduced cerebellar grey matter and altered functional activation prominently in lobules IV, V, VII, and VIII, and in Crus I-II (Ding et al., 2019; Kaufmann et al., 2019; Li X. et al., 2022; Li Y. et al., 2022). Moreover, mean age and illness duration were negatively associated with the reduction in the left Crus II (Li X. et al., 2022). A correlation study between cerebellar anatomy and functional activation with cognitive scores revealed that anatomical characteristics predicted both cognitive abilities and psychopathology (Moberget et al., 2019) [but see Guo et al. (2018) and Moussa-Tooks et al. (2022)]. A recent preclinical study reported an increase in climbing fiber/Purkinje cell synaptic connectivity following neonatal subchronic administration of Phencyclidine (PCP), a drug of abuse with psychomimetic effects leading to long-term behavioral changes related to SZ in rodents (Veleanu et al., 2022). In a post-mortem study, cerebellar cortex abnormalities correlated with the altered expression of 23 genes involved in cerebellar presynaptic vesicular transport, Golgi function, and GABAergic neurotransmission (Mudge et al., 2008).
In SZ patients, cerebellar cortex volume is significantly decreased, with the most pronounced effects observed in regions functionally connected with frontoparietal cortices. This has been consistently reported as one of the most prominent structural alterations, alongside other changes such as reductions in hippocampus volume and frontotemporal cortical thickness. Positive correlations emerged between cerebellar volume and cerebral cortical thickness in frontotemporal regions, suggesting common underlying disease processes jointly affecting the cerebellum and the cerebrum. Interestingly, cerebellar volume reduction in SZ was highly consistent across the age span 16–66 years and was present already in the youngest patients, which is more in line with neurodevelopmental than neurodegenerative etiology (Moberget et al., 2018, 2019).
Bègue and co-workers used canonical correlation analyses to link cerebellar grey matter volume to cognitive functioning. They proposed two maps: one associated with cognitive flexibility, processing speed and working memory (Crus II and Lobule X) and the other with working memory (Crus I and Lobule VI), both linked also to working memory (Bègue et al., 2023). While cerebellar volume reduction is the most common finding, an increase in right cerebellum and lingual gyrus grey matter volume was also associated with formal thought disorders in SZ patients (Maderthaner et al., 2023).
Finally, sexual dimorphisms have been considered in normative cerebellar developmental trajectories, challenging the belief that males have inherently larger cerebellar volumes, possibly due to differences in hormonal fluctuations and environmental experiences (Sefik et al., 2022). In clinical high-risk SZ females, smaller cerebellar cortex sizes correlated with more severe disorganization symptoms, especially in negative-related domains, while SZ males under 20 showed reduced white matter volume (Sefik et al., 2022) (see Figure 4 for cerebellar structural/functional alterations and Figure 3 for microcircuit abnormalities).
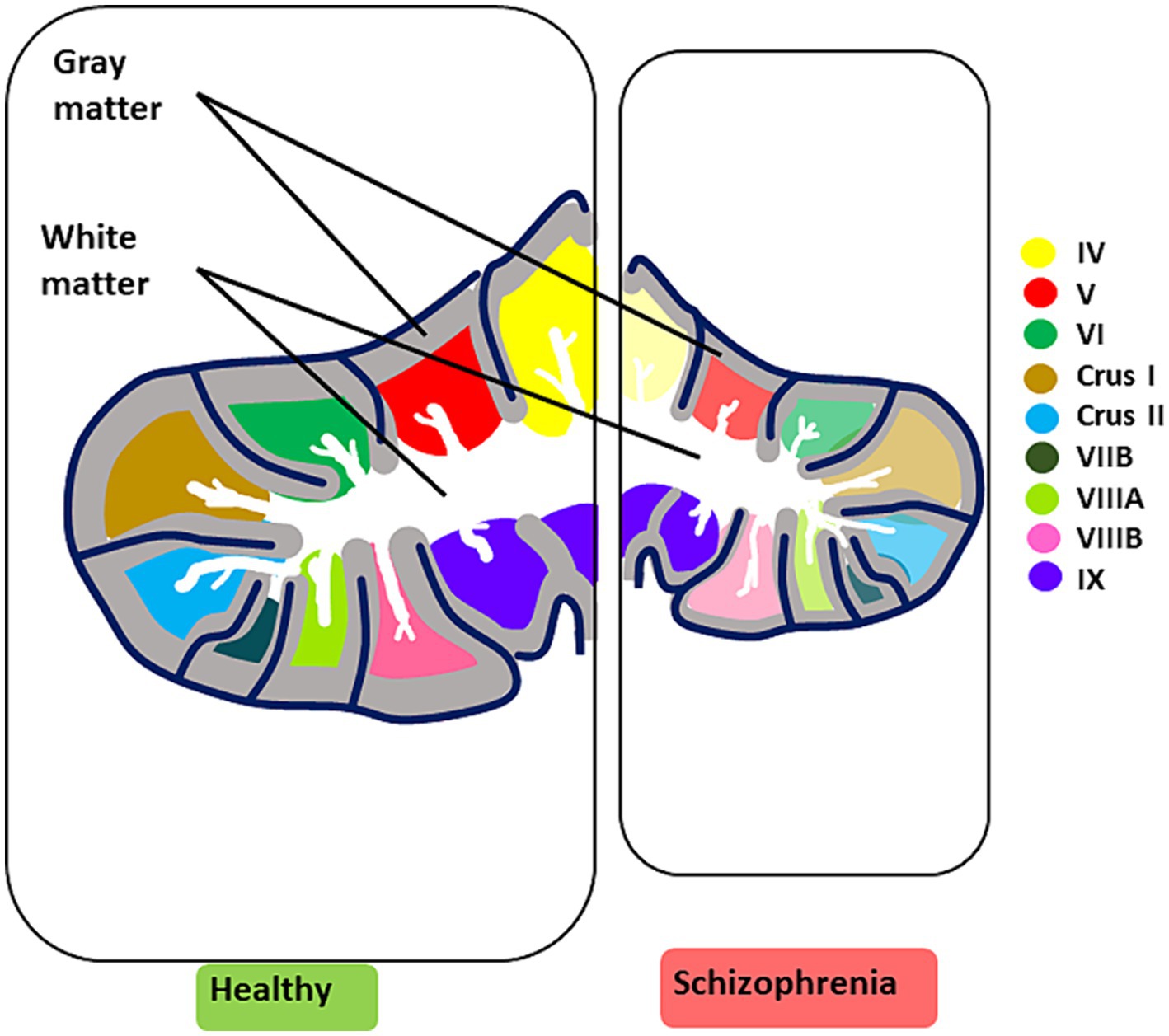
Figure 4. Key structural and functional alterations in the SZ brain. The illustration summarizes the most prominent cerebellar structural and functional alterations observed in CIAS. Structural alterations are characterized by an overall reduction in volume, accompanied by a decrease in grey matter and white matter thickness (for microscopic alterations see Figure 3). Reduced functional activation in CIAS is observed in cerebellar lobules IV, V, VI, Crus I and II, VIIB, VIIIA, and VIIB. Each lobule is color-coded for clarity. In schizophrenia (right panel), the affected lobules are colored in pale shades.
3.2 Cerebellar neurotransmitters alteration in CIAS
Like the forebrain, the cerebellum is regulated through a complex system of neurotransmitters (Ottersen, 1993). Fast excitatory synaptic transmission is mediated by glutamate, and inhibitory synaptic transmission is mostly mediated by GABA (although there are also glycinergic synapses). Glutamate is released from mossy fibers (mfs) onto GrCs (D'Angelo et al., 1990) which, in turn, release glutamate onto PCs. GABA regulates the overall excitability of the cerebellar circuit. GABAergic neurons (Purkinje cells, Golgi cells, stellate cells, and basket cells) provide inhibitory signals that fine-tune and modulate the output from the cerebellum to other parts of the brain (Mapelli et al., 2014; Nieus et al., 2014). In addition to GABA and glutamate, several neuromodulators, such as dopamine, serotonin, noradrenaline, and acetylcholine, play important roles in modulating cerebellar function (Zhang et al., 2016). For example, while the cerebellum is not classically considered an elective dopaminergic region, recent studies showed that it has an important involvement in dopaminergic control and plays a role in dopamine deficit-related neurological and psychiatric disease (Flace et al., 2021). Serotonin is known to modulate GABAergic and glutamatergic signaling in the adult cerebellum, where it can adjust PCs and Lugaro cell firing rate (Dieudonné and Dumoulin, 2000; Fleming and Hull, 2019). Ach can enhance glutamatergic neurotransmission and plasticity in the cerebellar glomeruli (Prestori et al., 2013). Eventually, these neuromodulators can influence cerebellar functioning impacting motor learning and control as well as cognitive processing (Ankri et al., 2015; Mapelli et al., 2015; Gao et al., 2016; Flace et al., 2021). Since dysfunction and imbalance in neurotransmitter systems lead to various neurological disorders including CIAS, addressing the intricate interplay of cerebellar neurotransmitters could provide insight into the underlying causes of these disorders and allow for the development of targeted treatments to restore cognitive decline in SZ. The evidence supporting the impact of cerebellar neurotransmitters in CIAS is presented below and summarized in Figure 5.
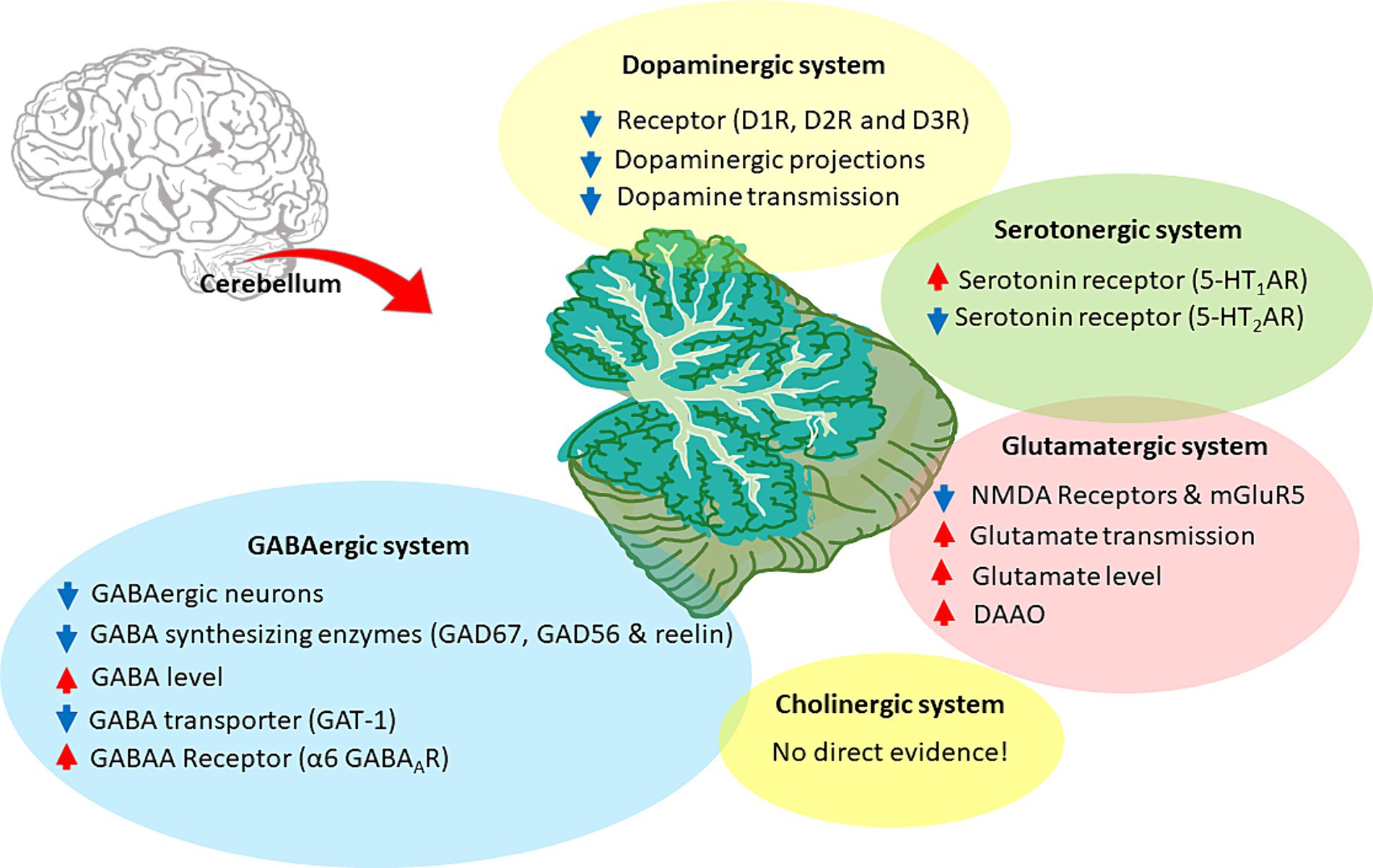
Figure 5. Neurotransmitter alterations in the SZ cerebellum. The figure illustrates the cerebellar neurotransmitter systems and their hypothetical relationship with SZ. Hyperfunctioning and hypofunctioning are identified with red and blue arrows, respectively. Cerebellar dopaminergic system: dysregulation of dopamine receptors (DR), particularly D1R and D2R, would decrease dopaminergic transmission. Alterations also involve aberrant dopaminergic projections and overall downregulation of dopamine signaling. Cerebellar glutamatergic system: the hypothesis focuses on the hypofunction and downregulation of glutamate receptors, particularly NMDAR and mGluR5. It further includes the acceleration of glutamate transmission, release, and related enzymes, suggesting an altered glutamatergic system in the cerebellum. Cerebellar GABAergic system: the hypothesis involves various aspects, such as decreased GABAergic projections, aberrant levels of synthetic enzymes (like GAD67 and GAD56), and elevated GABA concentration. It also highlights specific GABA receptors, particularly α6 GABAAR, along with lower expression of GABA transporters, such as GAT-1. Cerebellar cholinergic system: there is no direct evidence for the involvement of the cerebellar cholinergic system in CIAS. Cerebellar Serotonergic system: some alterations were found in SZ, such as 5-HT1AR upregulation and 5-HT2AR downregulation.
3.2.1 The cerebellar dopaminergic system
As seen above, dopamine in forebrain circuits is crucial for cognitive functioning and is significantly implicated in SZ pathophysiology (Davis et al., 1991; Kesby et al., 2018) (Section 2.1). A fact that has not been sufficiently recognized before is that dopamine is also present at high concentrations in the cerebellum. In rodents, the deep cerebellar nuclei (DCN) show higher dopamine concentrations than hippocampus and cerebellar cortex, and similar to frontal cortex (Versteeg et al., 1976). Several clinical and preclinical studies showed a crucial involvement of cerebellar dopaminergic mechanisms in CIAS (Demirtas-Tatlidede et al., 2010; Rogers et al., 2013; Parker et al., 2014).
Recently, the cerebellar dopaminergic system has been characterized. Receptor subunits D1R-D5R have been reported in various lobules of the cerebellar cortex, mostly in PCs, where they impact synaptic and cellular plasticity (Cutando et al., 2022). Dopaminergic projections to the cerebellar cortex and nuclei originate mainly from VTA (Ikai et al., 1994) and in part from locus coeruleus (Canton-Josh et al., 2022) that also gives rise to the major dopaminergic midbrain and cerebral pathways (Panagopoulos and Matsokis, 1994). Moreover, PCs produce dopamine (Li et al., 2023). Finally, the cerebellum output through the DCN regulates the VTA (Carta et al., 2019) and the substantia nigra (Washburn et al., 2024). Therefore, the cerebellum has a triple relationship with the dopaminergic system: it produces dopamine, it receives dopaminergic innervation, and it regulates the dopaminergic systems of the brain stem and basal ganglia.
PCs synthesize and release dopamine in an activity-dependent manner modifying local microcircuit functioning (Li et al., 2023). Dopamine binds to D1Rs in Bergman glial cells causing membrane depolarization and activating a Ca2+ signaling cascade leading to AMPA receptor GluA1 subunits membrane insertion and glutamate release. This, in turn, enhances interneuron activity reducing PC excitation by parallel fibers and climbing fibers (cfs) and altering the PCs firing frequency and pattern, eventually impacting locomotor and social behavior. These findings indicate that the cerebellar dopaminergic system has a critical pathophysiological role in disorders associated with motor and social dysfunction (Li et al., 2023). Axons coming from the locus coeruleus may regulate cerebellar cortex activity by co-releasing dopamine onto D1R-positive unipolar brush cells. PCs, then directly inhibit the same unipolar brush cells, forming a dopamine-sensitive recurrent circuit (Canton-Josh et al., 2022).
The cerebellum modulates VTA dopamine release via direct projections impacting the expectation/reward mechanism (Carta et al., 2019; Holloway et al., 2019). This pathway extends the role of cerebellum in error detection to the discrepancy between motivation and expectation of reward allowing a cerebellar control on emotional and social behavior (D'Angelo, 2019).
As seen above, hypoactivity in the mesocortical pathway is associated with negative symptoms and CIAS (Toda and Abi-Dargham, 2007; Treadway and Zald, 2011). Interestingly, reduced functionality in cerebellar circuits alters dopaminergic activity in the medial PFC (Rogers et al., 2011), suggesting that a third, cerebellum-related, control system impacts dopaminergic functions in SZ. Indeed, electrical stimulation of the Purkinje layer and DN evokes a long-lasting increase in dopamine release in PFC. Thus, a disconnection between the PCs and neuronal populations of the DN could alter dopaminergic signaling in PFC and impact SZ symptoms (Mittleman et al., 2008) and CIAS. Reduced interaction between the cerebellum and the basal ganglia-dopamine network might be involved in regulating the motivation domain (Yoshida et al., 2022).
Indirect evidence supports this hypothesis. (1) In rat cerebellum, the atypical antipsychotic blonaserin and the anxiolytic buspirone engage extensively in D3R regulation and their action is associated with cognitive impairment (Baba et al., 2015; Di Ciano et al., 2017). (2) A reduced cerebellar expression of SP transcription factors and D2Rs was related to negative symptoms observed in SZ (Pinacho et al., 2013). (3) In genomic DNA isolated from the cerebellum, the atypical antipsychotic agent olanzapine increased methylation of genes related to the dopaminergic system, such as D3R, DOPA decarboxylase, and VMAT2 (SCL18A2/VMAT2) (Melka et al., 2013). (4) Alteration in D2R levels in PCs of male mice during adulthood alters sociability and preference for social novelty without affecting motor functions (Cutando et al., 2022). (5) Aberrant dopamine neurotransmission in SZ influences the cerebellar vermis affecting time processing and directly addressing the cognitive dysmetria hypothesis (Yeganeh-Doost et al., 2011). While more research is warranted, a causal link is beginning to emerge between cerebellar dopamine and CIAS.
3.2.2 The cerebellar cholinergic system
Cholinergic signaling in the cerebral cortex and basal forebrain is strongly related to learning and memory (Lecrux et al., 2017) (Section 2.1) but its role in the cerebellum is less explored. Nevertheless, there is growing evidence indicating that cholinergic projections from the brainstem may influence cerebellar function and play a modulatory role in cognitive processing. Cholinergic projections form the third afferent system of the cerebellum, following cfs and mfs (de Lacalle et al., 1993), and seem to play a modulatory role by biasing neuronal excitability and synaptic responses, eventually influencing the cerebellar output and behavioral responses (Zhang et al., 2016).
Nearly half of cholinergic neurons in the brainstem project to cerebellum (Zhao et al., 2023). The vermis also harbors a substantial population of cholinergic neurons, and a dysfunction in this region may potentially contribute to cognitive deficits (Huang et al., 2007), as observed in Parkinson’s disease patients (Maiti et al., 2020). Both nAChRs and mAChRs are expressed in the cerebellum and are activated by Ach released from cholinergic fibers.
In rodents, mAchRs are present in all cerebellar lobules with differential expression across layers. Expression is higher in the PC layer of lobules I-V, Crus I-II, in the GC layer of lobules VI-VII, and in the molecular layer of all other lobules. Cholinergic fibers emerge from the inferior peduncle and spread across the cerebellar cortex as mfs, glomerular rosettes, and thin varicose fibers (Jaarsma et al., 1997). The cerebellum shows choline acetyltransferase (ChAT) (Jaarsma et al., 1997) and acetylcholine esterase (Okkels et al., 2023) activity, and [18F] FEOBV PET imaging have revealed Ach uptake in the cerebellum in vivo, most markedly in the vermis and flocculonodular lobe (Okkels et al., 2023).
The α7-nACh receptor subunit controls LTD/LTP balance at mf-GrC synapses, which, consequently, facilitates neural adaptation (Prestori et al., 2013). Similarly, applying nicotine during an air-puff stimulation task influences GrCs activity (Xu et al., 2019). Cerebellar nAChRs can also regulate GABA release from interneurons in a subtype-specific manner and affect cognitive functions (Turner et al., 2011).
Alterations of the cerebellar cholinergic system were documented in various mental disorders and associated with cognitive decline. For instance, in a rat model of Japanese encephalitis characterized by marked damage in cognitive functions, transient spatial learning and memory deficits were due to reduced cholinergic activities in various brain regions including the cerebellum (Chauhan et al., 2016). The reduction involved most cholinergic markers, including total muscarinic receptor bindings and M2 receptor, CHRM2 mRNA level, and ChAT expression (Chauhan et al., 2016).
Ach plays a crucial role in the cerebellar interpositus nucleus during execution and coordination of voluntary movements, through activation of muscarinic receptors. Moreover, the cholinergic system is relevant for reward-related behavior (Pickford et al., 2023). Bilateral cerebellar infusion of scopolamine (mAChR antagonist), or Mecamylamine (mAChR antagonist) differentially impaired motor performance (Pickford et al., 2023). Disruption in cholinergic neurotransmission has been associated with executive dysfunction in animals and humans affected by SZ (Section 2.1). While there is currently no direct evidence evaluating the role of cerebellar cholinergic signaling in CIAS, alterations in the cholinergic system might impact CIAS. For instance, individuals with SZ often experience cognitive impairments in working memory, attention, and executive functions, all of which have been associated with cerebellar activity and cerebellar cholinergic signaling. These observations are indirect and further research is required to elucidate the relationship between cerebellar cholinergic dysfunction and CIAS.
3.2.3 The cerebellar serotonergic system
Serotonergic signaling regulates mood, cognition, and various physiological processes, and its alteration is implicated in SZ pathophysiology (Section 2.1). Although serotonin is commonly associated with PFC and limbic system, it is also present in the cerebellum (Oostland and van Hooft, 2013), and serotonergic fibers represent one of the primary input pathways. Cerebellar 5-HT modulates glutamatergic and GABAergic synaptic transmission, regulates signal flow in PCs, facilitates firing, and regulates synaptic transmission and long-term synaptic plasticity in DCN neurons (Saitow et al., 2013). In the cerebellar cortex and DCN, different subtypes of serotonergic receptors (5-HT1B, 5-HT2B, 5-HT2A, 5-HT3, and 5-HT5A) have been identified (Duxon et al., 1997; Oostland and van Hooft, 2013).
The serotonergic system controls cerebellar development and is implicated in neurodevelopmental diseases (Oostland and van Hooft, 2013). Initially, 5-HT regulates dendritic growth and synaptic plasticity. In the first postnatal week, activation of 5-HT₁R expressed by GrCs and PCs stimulates dendritic growth and synapse formation (Oostland et al., 2014). Then, activation of 5-HT₃ Rs in GrCs limits dendritic growth of PCs by modulating pf-PC plasticity and cf competition for PC dendrites. Finally, activation of 5-HT₂R in GrCs and PCs during late postnatal development and in the mature cerebellum stabilizes synaptic activity (Oostland and van Hooft, 2013; Oostland et al., 2014). The Lugaro cells are also specifically targeted by serotonergic inputs that can increase their firing thereby inhibiting Golgi cells (GoC) (Dieudonné and Dumoulin, 2000).
Under physiologic conditions, cerebellar 5-HT1ARs decline during the neonatal stage and disappear by early childhood. In contrast, in SZ, cerebellar 5-HT1ARs persist in adulthood, specifically in the vermis, in relation to abnormal serotonergic innervation (Slater et al., 1998). These results support the notion that SZ has a neurodevelopmental component and that cerebellar 5-HTRs expression goes wrong during ontogenesis. This aspect is intriguing since 5-HT1AR is strongly associated with disturbed mood and emotion (Popova and Naumenko, 2013). Upregulation of cerebellar 5-HT1AR in SZ has been confirmed by in vivo PET imaging (Tauscher et al., 2002) while immunolabeling revealed that cerebellar 5-HT2AR is reduced in SZ subjects (Eastwood et al., 2001).
The reported alterations in 5-HTR expression in the cerebellar cortex and nuclei contribute to the serotonergic hypothesis of SZ, although a direct demonstration is still lacking. Please note that altered cerebellar serotonergic signaling is potentially associated with ASD, where a broad distribution of 5-HT5A mRNA has been revealed in all cerebellar regions (Marazziti, 2002).
3.2.4 The cerebellar glutamatergic system
Alterations of the glutamatergic system in SZ (see Section 2.1) have been reported not just in the basal ganglia, temporal lobe, and thalamus (Merritt et al., 2023) but also in the cerebellum. The cerebellum contains the highest concentration of NMDA receptors in the brain along with a rich variety of receptor subtypes and receptor-dependent mechanisms. A recent preclinical study suggested the crucial role of cerebellar glutamatergic neurotransmission during brain development in motor and social behavior (van der Heijden et al., 2023).
Cerebellar NMDARs are crucial for neuronal survival (Contestabile, 2002) and circuit development and functioning (Rabacchi et al., 1992; Bidoret et al., 2009). Repeated ketamine administration causes neurodegeneration in the cerebellum and memory loss in rats (Onaolapo et al., 2019). PCP administration during the neonatal stage impacts development of the olivocerebellar circuit. In the PCP model, the mRNA levels of two GoC selective NMDAR subunits, NR2B and NR2D, decreased (Bullock et al., 2009). In humans, in the first episode of psychosis, individuals displayed elevated levels of glutamate both in the associative striatum and cerebellum (de la Fuente-Sandoval et al., 2013). All NMDAR subunits are expressed in the cerebellum, with significant expression of GluN2C and GluN2D (Thompson et al., 2000), and might be altered in SZ (Schmitt et al., 2010).
Alterations in cerebellar NMDAR expression and activity were implicated in cerebellar circuit dysconnectivity and strongly correlated with CIAS (Yeganeh-Doost et al., 2011). The expression of the NR2C subunit in mature mf-GrC synapses (Mullasseril et al., 2010) is regulated by NRG1 (Ozaki et al., 1997), which is a vulnerability gene for CIAS (Douet et al., 2014). In addition, D-serine deregulation is significantly implicated in CIAS (Ma et al., 2019). D-serine, a co-agonist of NMDAR on the glycine binding site (D'Angelo et al., 1990), is oxidized by D-amino acid oxidase (DAO/DAAO), which can regulate the NMDAR function via D-serine breakdown. DAAOs are expressed mainly in cerebellum with little expression in the frontal cortex (Benzel et al., 2008; Jagannath et al., 2017).
In SZ subjects, a reduced expression of the monomeric form of mGluR5 was specifically revealed in the lateral cerebellum and associated with mood disorders in SZ (Fatemi et al., 2013; Matosin et al., 2014; Fatemi and Folsom, 2015).
Moreover, histological alterations were observed in cerebellar slices in a ketamine-induced SZ model in mice; the changes were seen mostly in neurodegenerating cerebellar areas, particularly in PCs showing apoptosis with pyknotic nuclei, irregular dark cytoplasm, and wide interstitial spaces around the cells. This effect was reversed in groups treated with Carpolobia lutea G. Don extract and clozapine (Omeiza et al., 2023). Notably, the recovery of tissue damage was associated with mitigation of positive, negative, and cognitive symptoms.
3.2.5 The cerebellar GABAergic system
GABA is the main inhibitory neurotransmitter in the cerebellum and the entire brain (Section 2.1). In the cerebellum, GABA helps regulate and balance neural activity, contributing to motor control, coordination, and cognitive functions. It was hypothesized that, in the cerebellum, the effectiveness of the GABAergic inhibitory system might be reduced in SZ to counterbalance NMDAR hypofunction (Yeganeh-Doost et al., 2011) contributing to cognitive impairment (Piras et al., 2019). The cellular density of GABAergic Purkinje inhibitory neurons in cerebellum is decreased in psychotic patients (Maloku et al., 2010).
Early findings from clinical and preclinical trials showed alterations in cerebellar GABA signaling in SZ patients. The mRNA and protein levels of GABA synthesizing enzymes GAD67 and reelin, which are expressed in GABAergic interneurons, were downregulated (Guidotti et al., 2000; Fatemi et al., 2005; Bullock et al., 2008). Like other neuromodulators, the alterations were not limited to receptor function and expression but also involved GABA levels. A higher concentration of cerebellar GABA was detected in SZ patients, and this was associated with lower phonemic fluency and a reduced number of switches between subcategories compared to healthy subjects (Piras et al., 2019). GAD56 and the presynaptic GABA transporter GAT-1 were also reduced along with PC density (Bullock et al., 2009; Maloku et al., 2010).
GABAAR containing α6 subunits (α6GABAARs) are located at cerebellar GoCs-GrCs synapses and extra-synaptic sites, where they regulate the precision of inputs required for cerebellar timing (Mapelli et al., 2014; Mapelli J. et al., 2022). These receptors have an impact on motor activity and are involved in cognitive processing and adequate responses to external stimuli in the cerebellum, eventually implicated in CIAS (Lee et al., 2022). The α6GABAARs were upregulated in post-mortem cerebellar tissues and in a rat model induced by PCP (Bullock et al., 2009).
Dysfunction in cerebellar GABAergic interneurons leads to reduced synchronization across brain regions, affecting cortical information processing (Yeganeh-Doost et al., 2011). The specific impairment of subsets of cerebellar GABA-expressing interneurons in SZ (Piras et al., 2019; Lee et al., 2022) could disrupt the coordination between cerebellum and cortex contributing to neuropsychological deficits (Piras et al., 2019).
3.3 Cerebellar dysconnectivity and CIAS
The cerebellar cortical circuit is illustrated in Figure 3A. PCs integrate signals from the IO-cf and the mf-GrC-pf pathways, and project to DCN neurons which, in turn, projects back to various brain regions (D'Angelo and Casali, 2012). This way, the cerebellar cortex forms intricate connections with the cerebral cortex, basal ganglia and VTA that are core to CIAS and SZ. The disruption in cerebellar network communication, tied to deficiencies in dopamine, glutamate, and GABA transmission, has been indeed proposed to explain reduced connectivity in SZ patients (Giersch et al., 2016, Katz Shroitman et al., 2023; Figure 6).
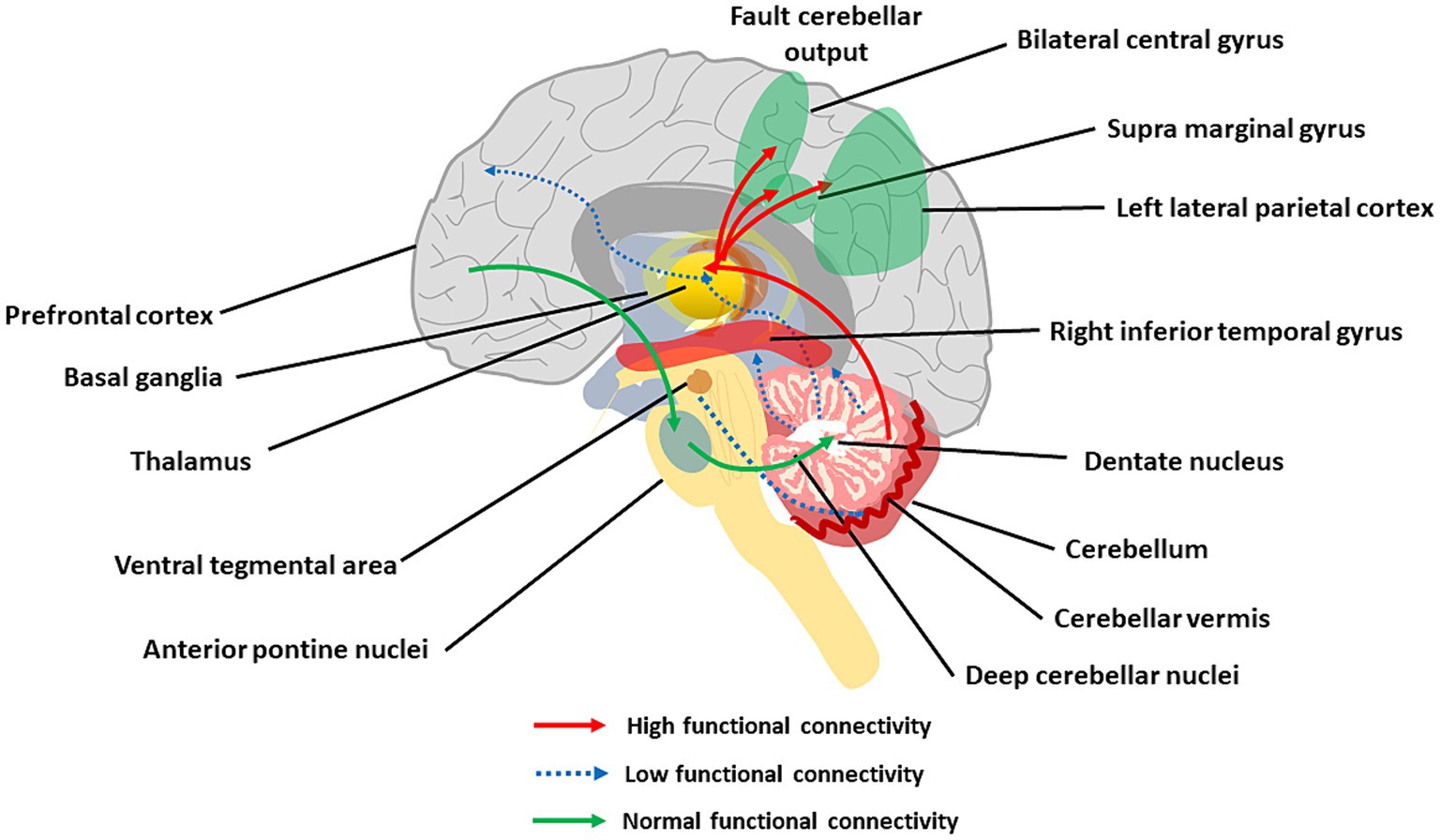
Figure 6. The main aspects of cerebellar dysconnectivity in SZ. In the scheme, different cerebellar projections show either increased (red arrows), decreased (blue arrow), or normal (green arrows) functional connectivity. Note the reduced functional connectivity with the prefrontal cortex and inferior temporal gyrus, the reduced functional connectivity with VTA, and the increased functional connectivity with the parietal cortex (see text for details).
3.3.1 CTCC loops and cognitive dysmetria
The cerebellum forms extensive connections with the forebrain via DCN and thalamus generating cerebello-thalamo-cortical circuits (CTCCs) that are thought to underly cognitive and affective functions (Schmahmann, 2016; Ribeiro and Sherrard, 2023). Functionally, the cerebellar and cerebral systems work in concert to refine the timing of neural operations (Castellazzi et al., 2014, 2018; Palesi et al., 2015, 2017, 2018; Bohne et al., 2019; Fujita et al., 2020; Pisano et al., 2021; Li Y. et al., 2022) (see Supplementary material). Through the thalamus, the cerebellum is implicated in coordinating the coherence of oscillations between cerebral cortical structures (Popa et al., 2014; Gambosi et al., 2023; Heck et al., 2023). The cerebellum, as part of the CTCC, performs an error-detection duty and works as a modulator of cognitive information acquired from the cortex (Bang et al., 2018). SZ was early hypothesized to arise from a disrupted CTCC communication impairing the error detection function of the cerebellum (Andreasen et al., 1998; Andreasen and Pierson, 2008; D'Angelo and Casali, 2012), and a wealth of studies have recently focused on abnormal CTCC connectivity as a core pathology of SZ as well as other psychiatric disorders (Okugawa et al., 2004; Koch et al., 2010; Bang et al., 2018; Hanaie et al., 2018; Wang et al., 2018; Ding et al., 2019; Kim et al., 2021; Park et al., 2021). Lesions of the posterior cerebellum have been related to cognitive dysmetria and CIAS (Yeruva et al., 2021). A seminal work revealed the functional connectivity of DN with whole-brain and its association with cognitive impairments and other psychotic symptoms in patients with drug-naïve and first-episode SZ (Xie et al., 2021). The increased connectivity of DN with the bilateral postcentral gyrus and decreased connectivity of DN with the right inferior temporal gyrus and regional cerebellum (e.g., Vermis IV, V, and Crus I) were correlated with CIAS. The other hub of the CTCC is the thalamus, and altered functional connectivity between cerebellar hemispheres, mediodorsal nucleus, and lateral geniculate nucleus of the thalamus was reported in SZ (Collin et al., 2011; Liu et al., 2011; Chen et al., 2013; Anticevic et al., 2014; Barch, 2014).
Both in first-episode and chronic SZ patients, altered cerebellar functional connectivity in RS fMRI was observed with broad cerebral regions, including association networks, the sensorimotor network, the limbic network, basal ganglia network, and the DMN (Liu et al., 2011; Chen et al., 2017; Guo et al., 2018; Zhuo et al., 2018; Xie et al., 2021; Feng et al., 2022). Critical connector hubs were identified using voxel-based analysis in the cerebellum, midbrain, thalamus, insula, and calcarine sulcus, with connectivity to multiple RS networks affected in SZ (Yamamoto et al., 2022). These findings were supported by cognitive task-dependent fMRI, in which SZ patients showed significantly increased connectivity between the cerebellum and left lateral parietal cortex compared to healthy participants (King et al., 2023). Reduced blood flow in the CTCC during cognitive tasks in SZ was related to deficits in cerebellar inhibition of the DCN (Daskalakis et al., 2005). The strength of functional connectivity between the cerebellum and lateral parietal regions, such as the postcentral gyrus and supramarginal gyrus, was associated with negative symptoms, including socio-cognitive dysfunctions and cognitive decline in SZ (Guo et al., 2018; Brady et al., 2019; Park et al., 2021; Choi et al., 2023).
3.3.2 Cerebellar connectivity with basal ganglia
The cerebellum sends monosynaptic glutamatergic projections to dopaminergic and non-dopaminergic neurons of substantia nigra pars compacta (Washburn et al., 2024). Moreover, the cerebellum and striatum communicate with the thalamus and cortex via monosynaptic and polysynaptic connections, producing cortico-striatal-thalamic-cerebellar (CSTC) loops. Associative CSTC subdivisions showed consistent brain-wide bi-directional changes in SZ, hyperconnectivity with sensory cortices, and hypoconnectivity with association cortex. Such alterations were strongly related to cognitive impairment (Ji et al., 2019). A study of resting-state networks in SZ patients showed increased functional connectivity in the DMN associated with decreased connectivity in the cerebellar network (Rong et al., 2023). Connectivity of the cerebellum with basal ganglia and regions involved in visual, sensorimotor processing and reward was also altered (Yoshida et al., 2022).
3.3.3 Cerebellar connectivity with VTA
The cerebellum is connected to and transmits direct stimulatory signals to the VTA, a brain region responsible for the elaboration of rewarding experiences. Optogenetic activation of the cerebellum-VTA connections led to a sense of reward. In the three-chambers social task, these connections become more active when the animal engages with the social chamber during exploration. These data define a major, previously unappreciated role of the cerebellum in controlling the reward circuitry and social behavior, indicating that the cerebellum may mediate SZ symptoms through abnormal connections with the midbrain dopamine brain regions, such as VTA (Carta et al., 2019). Interestingly, recent RS fMRI imaging findings from first-episode SZ patients showed decreased static and dynamic functional connectivity of VTA and substantia nigra pars-compacta to cerebellar vermis (lobules VII and IX), thalamus, striatum, prefrontal lobe, and cingulate gyrus (Xue et al., 2023).
3.3.4 Cerebellar neurodevelopment and neuroinflammation
The cerebellum fetal development endures during childhood and influences the postnatal maturation of multiple cortical regions (Wang et al., 2014). Alterations in this process might, in turn, impact on SZ. Indeed, a combined volume reduction in cerebellum (lobules I–V, VIII) and sensorimotor cortex are associated with increased SZ externalizing symptoms (Miquel et al., 2019). Moreover, reduced grey matter volumes in cerebellum and functionally coupled cortical regions are associated with psychiatric symptoms in mid-childhood (Hughes et al., 2023). Interestingly, altered functional connectivity between cerebellum and medial PFC in SZ patients was linked to high childhood trauma scores (Dauvermann et al., 2021). Moreover, increased connectivity between left lateral parietal cortex and cerebellum was correlated with low-grade systemic inflammation and high plasma IL-6 level, higher childhood neglect, and increased DMN connectivity (King et al., 2023). Therefore, the main genetic and epigenetic factors of SZ may also act on the cerebellum driving a cascade of effects impacting the pathogenesis of the disease.
4 Summary, conclusions, and perspectives
4.1 Summary and key findings
Since the original proposal for the cerebellar involvement in SZ 25 years ago (Andreasen et al., 1998; Andreasen and Pierson, 2008), a large body of evidence has accumulated showing that the schizophrenic brain exhibits various abnormalities in most brain regions controlling cognitive processing (Karlsgodt et al., 2010; Zhao et al., 2018; Sone et al., 2022; Javitt, 2023). On one hand, the cerebral cortex and forebrain regions have revealed alterations in micro- and macro-structure, development, neurotransmission, plasticity, and connectivity. On the other, the hypothesis of the involvement of the cerebellum in SZ is gaining credit. The cerebellum is involved in multiple aspects of cognitive processing (Schmahmann, 2016; D'Angelo, 2018; D'Angelo, 2019; Schmahmann, 2019; Jacobi et al., 2021; Ciapponi et al., 2023; Nguyen et al., 2023) and is connected functionally and anatomically to brain regions that are core domains of CIAS, such as PFC, basal ganglia, and VTA. Cerebellar alterations can either be primary (genetic and epi-genetic) or secondary (compensatory) in origin and emerge on different scales (Figures 3–6):
1. Reduced cerebellar volume, more accentuated in specific areas, reflecting decreased grey matter and white matter thickness.
2. Microcircuit and cellular alterations, including reduced cell density (GrC, PC, and inhibitory interneuron), reduced PC dendritic branching, altered synaptic vesicular transport, and increased connectivity at climbing fiber/PC synapses.
3. Reduced connectivity with other brain structures, including PFC, basal ganglia, and VTA.
4. Reduced functional activation of specific areas during cognitive tasks.
5. Dopaminergic hypofunction, serotonergic unbalance, glutamatergic and GABAergic dysfunction.
A potential explanation of this broad set of alterations is that any changes in brain circuits bring about both direct effects and compensatory responses in various system components, which then reverberate across scales. Altered bidirectional connectivity in psychosis may stem from neurodevelopmental disruptions or compensatory mechanisms, influenced by neurotransmitter systems abnormalities. Dopaminergic dysregulation may disrupt cerebellar E/I balance and dopaminergic projections to cortical regions, while upregulated serotonin receptors promote synaptic pruning and plasticity, possibly leading to hyperconnectivity. Glutamate and GABA elevation in the cerebellum might influence hyperconnectivity by modulating excitatory and inhibitory neurotransmission thus inducing plasticity and connectivity. As a result, these micro-scale modulations of neuronal activity shift network dynamics in response to ongoing demands. These alterations, occurring during neurodevelopment and persisting into adulthood, may disrupt normal connectivity patterns, contributing to psychosis manifestation and progression. This cascade of effects puts cerebellar alterations at the core of the extended brain dysfunction characterizing CIAS.
4.2 Cerebellar therapeutic targeting
The cerebellum may provide a promising target for innovative SZ treatments (Parker et al., 2014; Cao and Cannon, 2019; Hua et al., 2022). The specific expression of certain synaptic receptor subtypes (e.g., D1R, and NR2C/DAAO, mGluR5, GABAa6,) in the cerebellar circuit might be exploited.
Cerebellar D1R is a promising therapeutic target for CIAS going beyond the more common D2R antagonists (Goldman-Rakic et al., 2004). D1R agonists were found to enhance blood oxygenation level-dependent (BOLD) signals in the cerebellum in addition to striatum, thalamus, and PFC, while D1R antagonists did the opposite (Kimura et al., 2023). This observation suggests revisiting the Weinberger’s view that D1Rs are principally located in PFC where they are hypo-activated causing negative symptoms (Weinberger, 1987; Slifstein et al., 2015; Rao et al., 2018; McCutcheon et al., 2020), by integrating cerebellar D1R hypofunction as a potential cause of CIAS.
Cerebellar NMDA receptors may be targeted using DAAO antagonists that exploit D-serine sensitivity (Kölker, 2018). Luvadaxistat, a potent DAAO inhibitor, is being developed for the treatment of CIAS and was recently tested with some success in SZ patients (O'Donnell et al., 2023).
Cerebellar α6GABAARs may be targeted by selective positive allosteric modulators, which proved to alleviate positive, negative, and cognitive impairment in SZ in preclinical studies and rescued PPI by attenuating GrG activity (Chiou et al., 2018; Lee et al., 2022; Sieghart et al., 2022).
Moreover, invasive and non-invasive neuromodulation methods have been proposed to specifically target the cerebellum (Heath et al., 1980, 1981; Aparício et al., 2016; Laidi et al., 2020; Hua et al., 2022; Pilloni et al., 2022). TMS and tDCS can modulate PCs and then regulate DCN activity (Koch, 2010) and neural plasticity (D'Angelo et al., 2016). In preclinical studies, low-intensity rTMS caused PC dendrite and spine changes (Morellini et al., 2015) and tDCS regulated the PC output (Grimaldi et al., 2014; Pope and Miall, 2014; van Dun et al., 2016). Interestingly, the effectiveness of these stimulations extended beyond the local circuit to extracerebellar networks causing, for example, changes in dopamine release (Fonteneau et al., 2018). Improvements in SZ cognitive symptoms were detected following cerebellar stimulation in different clinical trials (Escelsior and Belvederi Murri, 2019). For example, rTMS on posterior cerebellum could boost functional connectivity of the cerebellar-prefrontal circuitry ameliorating clinical symptoms (Brady et al., 2019; Singh et al., 2019; Basavaraju et al., 2021; Chauhan et al., 2021). It has been proposed that rTMS corrects alterations in error processing, which depend on information transfer and integration in the cerebellar-cortical circuitry (Hengyi Cao and Cannon, 2019). Interestingly, optogenetic stimulation of thalamic synaptic terminals of lateral cerebellar projection neurons in a rodent model of SZ-related frontal dysfunction rescued timing performance as well as medial frontal activity (Parker et al., 2017), suggesting that pathway-specific targeting is needed to improve the specificity of physical interventions on the cerebellum.
4.3 Open issues
There is still a large gap in our understanding of cerebellar involvement in SZ, especially concerning the initial alterations and their subsequent development, propagation, and compensation.
First, although abnormalities in the cerebellar neurotransmitter system have been documented, the intricate interconnections among these systems await elucidation. Open issues concern potential alterations in E/I balance and synaptic plasticity (Mapelli L. et al., 2022), whose exploration would require physiological investigations in animal models of SZ (Section 2 in Supplementary material). Of special interest is understanding how cerebellar hypo-dopaminergic function might influence the glutamatergic and GABAergic systems and how this, in turn, modulates cerebellar E/I balance and determines the PC output. This is also true for cerebellar serotonin shortages observed in CIAS, and a full revisitation is needed for the cholinergic system (Zhang et al., 2016).
Secondly, several questions regarding how cerebellar circuits operate in the context of CIAS-related circuits remain open. The main one is whether the universal cerebellar transform (Ito, 2008; D'Angelo and Casali, 2012) is altered and how, in turn, this impacts cognitive performance in SZ. Related to this is the differentiation of activity and neuromodulation among specific cerebellar regions (Ciapponi et al., 2023). This is particularly pertinent to the posterior lobules, which hold a pivotal role in cognitive processing.
Thirdly, it is not clear how shortages in cerebellar connectivity with other brain regions, including cerebral cortex, basal ganglia, and VTA, impact SZ. In turn, cerebellar dysconnectivity is related to neurodevelopment changes. Dysconnectivity may be, again, either a primary or a secondary event in SZ pathogenesis and bring about plastic changes that modify brain functions at system level.
4.4 Perspectives
Cognitive and negative symptoms are principal contributors to disability in SZ, but they are yet poorly treated by current therapies. The cerebellar involvement in CIAS (Section 4.1) is disclosing a promising target for therapeutic interventions (see Section 4.2). Thus, addressing gaps in knowledge is necessary to achieve a more comprehensive understanding of CIAS and its underlying mechanisms (see Section 4.3). In addition to MRI and electrophysiological recordings in humans, the precise analysis of neuronal activity and synaptic transmission and plasticity in animal models is needed to explain SZ-related alterations in connectivity, E/I balance, and synaptic plasticity, as well as in the GABAergic, glutamatergic, dopaminergic, serotonergic, and cholinergic systems of the cerebellum. Computational models can then be used to further understand the complex and heterogeneous nature of this disorder (Amunts et al., 2022; D'Angelo and Jirsa, 2022; Monteverdi et al., 2022, 2023) paving the way for precise and personalized therapeutic approaches, especially in treating cognitive shortages.
Author contributions
PF: Conceptualization, Supervision, Visualization, Writing – original draft, Writing – review & editing. DP: Writing – original draft, Writing – review & editing. FP: Writing – original draft, Writing – review & editing. ED’A: Conceptualization, Funding acquisition, Supervision, Visualization, Writing – original draft, Writing – review & editing.
Funding
The author(s) declare that financial support was received for the research, authorship, and/or publication of this article. This work was supported by #NEXTGENERATIONEU (NGEU) and funded by the Ministry of University and Research (MUR); National Recovery and Resilience Plan (NRRP) project; MNESYS (PE0000006) – A Multiscale integrated approach to the study of the nervous system in health and disease (DN. 1553 11.10.2022); Digital Europe Grant TEF-Health #101100700; HORIZON-HLTH-2023-TOOL-05-03 Integrated, multi-scale computational models of patient patho-physiology (‘virtual twins’) for personalized disease management #101137289 — VIRTUAL BRAIN TWIN — HORIZON-HLTH-2023-TOOL-05.
Acknowledgments
We would like to thank Neil Harrison for his valuable feedback on this paper.
Conflict of interest
The authors declare that the research was conducted in the absence of any commercial or financial relationships that could be construed as a potential conflict of interest.
The author(s) declared that they were an editorial board member of Frontiers, at the time of submission. This had no impact on the peer review process and the final decision.
Publisher’s note
All claims expressed in this article are solely those of the authors and do not necessarily represent those of their affiliated organizations, or those of the publisher, the editors and the reviewers. Any product that may be evaluated in this article, or claim that may be made by its manufacturer, is not guaranteed or endorsed by the publisher.
Supplementary material
The Supplementary material for this article can be found online at: https://www.frontiersin.org/articles/10.3389/fncel.2024.1386583/full#supplementary-material
References
Abashkin, D. A., Kurishev, A. O., Karpov, D. S., and Golimbet, V. E. (2021). Cellular models in schizophrenia research. Int. J. Mol. Sci. 22:518. doi: 10.3390/ijms22168518
Adams, R. A., Pinotsis, D., Tsirlis, K., Unruh, L., Mahajan, A., Horas, A. M., et al. (2022). Computational modeling of electroencephalography and functional magnetic resonance imaging paradigms indicates a consistent loss of pyramidal cell synaptic gain in schizophrenia. Biol. Psychiatry 91, 202–215. doi: 10.1016/j.biopsych.2021.07.024
Adhikari, B. M., Hong, L. E., Sampath, H., Chiappelli, J., Jahanshad, N., Thompson, P. M., et al. (2019). Functional network connectivity impairments and core cognitive deficits in schizophrenia. Hum. Brain Mapp. 40, 4593–4605. doi: 10.1002/hbm.24723
Alamian, G., Pascarella, A., Lajnef, T., Knight, L., Walters, J., Singh, K. D., et al. (2020). Patient, interrupted: MEG oscillation dynamics reveal temporal dysconnectivity in schizophrenia. Neuroimage Clin. 28:102485. doi: 10.1016/j.nicl.2020.102485
Allen, N. C., Bagade, S., McQueen, M. B., Ioannidis, J. P., Kavvoura, F. K., Khoury, M. J., et al. (2008). Systematic meta-analyses and field synopsis of genetic association studies in schizophrenia: the SzGene database. Nat. Genet. 40, 827–834. doi: 10.1038/ng.171
American Psychiatric Association (2013). Diagnostic and statistical manual of mental disorders. 5th Edn. Washington, DC: American Psychiatric Publishing, Inc.
Amunts, K., DeFelipe, J., Pennartz, C., Destexhe, A., Migliore, M., Ryvlin, P., et al. (2022). Linking brain structure, activity, and cognitive function through computation. Eneuro 9:ENEURO.0316-0321.2022. doi: 10.1523/ENEURO.0316-21.2022
Andreasen, N. C., O'Leary, D. S., Cizadlo, T., Arndt, S., Rezai, K., Ponto, L. L., et al. (1996). Schizophrenia and cognitive dysmetria: a positron-emission tomography study of dysfunctional prefrontal-thalamic-cerebellar circuitry. Proc. Natl. Acad. Sci. USA 93, 9985–9990. doi: 10.1073/pnas.93.18.9985
Andreasen, N. C., Paradiso, S., and O'Leary, D. S. (1998). "Cognitive dysmetria" as an integrative theory of schizophrenia: a dysfunction in cortical-subcortical-cerebellar circuitry? Schizophr. Bull. 24, 203–218. doi: 10.1093/oxfordjournals.schbul.a033321
Andreasen, N. C., and Pierson, R. (2008). The role of the cerebellum in schizophrenia. Biol. Psychiatry 64, 81–88. doi: 10.1016/j.biopsych.2008.01.003
Anhøj, S., Ødegaard Nielsen, M., Jensen, M. H., Ford, K., Fagerlund, B., Williamson, P., et al. (2018). Alterations of intrinsic connectivity networks in antipsychotic-Naïve first-episode schizophrenia. Schizophr. Bull. 44, 1332–1340. doi: 10.1093/schbul/sbx171
Ankri, L., Husson, Z., Pietrajtis, K., Proville, R., Léna, C., Yarom, Y., et al. (2015). A novel inhibitory nucleo-cortical circuit controls cerebellar Golgi cell activity. eLife 4:6262. doi: 10.7554/eLife.06262
Anticevic, A., Yang, G., Savic, A., Murray, J. D., Cole, M. W., Repovs, G., et al. (2014). Mediodorsal and visual thalamic connectivity differ in schizophrenia and bipolar disorder with and without psychosis history. Schizophr. Bull. 40, 1227–1243. doi: 10.1093/schbul/sbu100
Aparício, L. V. M., Guarienti, F., Razza, L. B., Carvalho, A. F., Fregni, F., and Brunoni, A. R. (2016). A systematic review on the acceptability and tolerability of transcranial direct current stimulation treatment in neuropsychiatry trials. Brain Stimul. 9, 671–681. doi: 10.1016/j.brs.2016.05.004
Argyropoulos, G. P. D., van Dun, K., Adamaszek, M., Leggio, M., Manto, M., Masciullo, M., et al. (2020). The cerebellar cognitive affective/Schmahmann syndrome: a task force paper. Cerebellum 19, 102–125. doi: 10.1007/s12311-019-01068-8
Avram, M., Brandl, F., Bäuml, J., and Sorg, C. (2018). Cortico-thalamic hypo- and hyperconnectivity extend consistently to basal ganglia in schizophrenia. Neuropsychopharmacology 43, 2239–2248. doi: 10.1038/s41386-018-0059-z
Baba, S., Enomoto, T., Horisawa, T., Hashimoto, T., and Ono, M. (2015). Blonanserin extensively occupies rat dopamine D3 receptors at antipsychotic dose range. J. Pharmacol. Sci. 127, 326–331. doi: 10.1016/j.jphs.2015.01.007
Ballester, P. L., Suh, J. S., Ho, N. C. W., Liang, L., Hassel, S., Strother, S. C., et al. (2023). Gray matter volume drives the brain age gap in schizophrenia: a SHAP study. Schizophrenia 9:3. doi: 10.1038/s41537-022-00330-z
Bang, M., Park, H. J., Pae, C., Park, K., Lee, E., Lee, S. K., et al. (2018). Aberrant cerebro-cerebellar functional connectivity and minimal self-disturbance in individuals at ultra-high risk for psychosis and with first-episode schizophrenia. Schizophr. Res. 202, 138–140. doi: 10.1016/j.schres.2018.06.031
Barch, D. M. (2014). Cerebellar-thalamic connectivity in schizophrenia. Schizophr. Bull. 40, 1200–1203. doi: 10.1093/schbul/sbu076
Basavaraju, R., Ithal, D., Thanki, M. V., Ramalingaiah, A. H., Thirthalli, J., Reddy, R. P., et al. (2021). Intermittent theta burst stimulation of cerebellar vermis enhances fronto-cerebellar resting state functional connectivity in schizophrenia with predominant negative symptoms: a randomized controlled trial. Schizophr. Res. 238, 108–120. doi: 10.1016/j.schres.2021.10.005
Bègue, I., Elandaloussi, Y., Delavari, F., Cao, H., Moussa-Tooks, A., Roser, M., et al. (2023). The cerebellum and cognitive function: anatomical evidence from a Transdiagnostic sample. Cerebellum :2023.2002.2022.23286149. doi: 10.1007/s12311-023-01645-y
Benzel, I., Kew, J. N., Viknaraja, R., Kelly, F., de Belleroche, J., Hirsch, S., et al. (2008). Investigation of G72 (DAOA) expression in the human brain. BMC Psychiatry 8:94. doi: 10.1186/1471-244X-8-94
Berdenis van Berlekom, A., Muflihah, C. H., Snijders, G., MacGillavry, H. D., Middeldorp, J., Hol, E. M., et al. (2020). Synapse pathology in schizophrenia: a Meta-analysis of postsynaptic elements in postmortem brain studies. Schizophr. Bull. 46, 374–386. doi: 10.1093/schbul/sbz060
Bernard, J. A., and Mittal, V. A. (2015). Dysfunctional activation of the cerebellum in schizophrenia: a functional neuroimaging Meta-analysis. Clin. Psychol. Sci. 3, 545–566. doi: 10.1177/2167702614542463
Bidoret, C., Ayon, A., Barbour, B., and Casado, M. (2009). Presynaptic NR2A-containing NMDA receptors implement a high-pass filter synaptic plasticity rule. Proc. Natl. Acad. Sci. USA 106, 14126–14131. doi: 10.1073/pnas.0904284106
Bohne, P., Schwarz, M. K., Herlitze, S., and Mark, M. D. (2019). A new projection from the Deep cerebellar nuclei to the Hippocampus via the ventrolateral and Laterodorsal thalamus in mice. Front. Neural Circuits 13:51. doi: 10.3389/fncir.2019.00051
Bonner-Jackson, A., Grossman, L. S., Harrow, M., and Rosen, C. (2010). Neurocognition in schizophrenia: a 20-year multi–follow-up of the course of processing speed and stored knowledge. Compr. Psychiatry 51, 471–479. doi: 10.1016/j.comppsych.2010.02.005
Bouvier, G., Higgins, D., Spolidoro, M., Carrel, D., Mathieu, B., Léna, C., et al. (2016). Burst-dependent bidirectional plasticity in the cerebellum is driven by presynaptic NMDA receptors. Cell Rep. 15, 104–116. doi: 10.1016/j.celrep.2016.03.004
Brady, R. O. Jr., Gonsalvez, I., Lee, I., Öngür, D., Seidman, L. J., Schmahmann, J. D., et al. (2019). Cerebellar-prefrontal network connectivity and negative symptoms in schizophrenia. Am. J. Psychiatry 176, 512–520. doi: 10.1176/appi.ajp.2018.18040429
Brisch, R., Saniotis, A., Wolf, R., Bielau, H., Bernstein, H.-G., Steiner, J., et al. (2014). The role of dopamine in schizophrenia from a neurobiological and evolutionary perspective: old fashioned, but still in vogue. Front. Psych. 5:47. doi: 10.3389/fpsyt.2014.00047
Bullock, W. M., Bolognani, F., Botta, P., Valenzuela, C. F., and Perrone-Bizzozero, N. I. (2009). Schizophrenia-like GABAergic gene expression deficits in cerebellar Golgi cells from rats chronically exposed to low-dose phencyclidine. Neurochem. Int. 55, 775–782. doi: 10.1016/j.neuint.2009.07.010
Bullock, W. M., Cardon, K., Bustillo, J., Roberts, R. C., and Perrone-Bizzozero, N. I. (2008). Altered expression of genes involved in GABAergic transmission and neuromodulation of granule cell activity in the cerebellum of schizophrenia patients. Am. J. Psychiatry 165, 1594–1603. doi: 10.1176/appi.ajp.2008.07121845
Burton, C. Z., Vella, L., Harvey, P. D., Patterson, T. L., Heaton, R. K., and Twamley, E. W. (2013). Factor structure of the MATRICS consensus cognitive battery (MCCB) in schizophrenia. Schizophr. Res. 146, 244–248. doi: 10.1016/j.schres.2013.02.026
Cannon, T. D., Chung, Y., He, G., Sun, D., Jacobson, A., van Erp, T. G., et al. (2015). Progressive reduction in cortical thickness as psychosis develops: a multisite longitudinal neuroimaging study of youth at elevated clinical risk. Biol. Psychiatry 77, 147–157. doi: 10.1016/j.biopsych.2014.05.023
Cannon, T. D., van Erp, T. G., Bearden, C. E., Loewy, R., Thompson, P., Toga, A. W., et al. (2003). Early and late neurodevelopmental influences in the prodrome to schizophrenia: contributions of genes, environment, and their interactions. Schizophr. Bull. 29, 653–669. doi: 10.1093/oxfordjournals.schbul.a007037
Canton-Josh, J. E., Qin, J., Salvo, J., and Kozorovitskiy, Y. (2022). Dopaminergic regulation of vestibulo-cerebellar circuits through unipolar brush cells. eLife 11:e76912. doi: 10.7554/eLife.76912
Canu, E., Agosta, F., and Filippi, M. (2015). A selective review of structural connectivity abnormalities of schizophrenic patients at different stages of the disease. Schizophr. Res. 161, 19–28. doi: 10.1016/j.schres.2014.05.020
Cao, H., and Cannon, T. D. (2019). Cerebellar dysfunction and schizophrenia: from "cognitive Dysmetria" to a potential therapeutic target. Am. J. Psychiatry 176, 498–500. doi: 10.1176/appi.ajp.2019.19050480
Carlsson, A., and Lindqvist, M. (1963). Effect of chlorpromazine or haloperidol on formation of 3METHOXYTYRAMINE and NORMETANEPHRINE in mouse BRAIN. Acta Pharmacol. Toxicol. 20, 140–144. doi: 10.1111/j.1600-0773.1963.tb01730.x
Carruthers, S. P., Gurvich, C. T., and Rossell, S. L. (2015). The muscarinic system, cognition and schizophrenia. Neurosci. Biobehav. Rev. 55, 393–402. doi: 10.1016/j.neubiorev.2015.05.011
Carta, I., Chen, C. H., Schott, A. L., Dorizan, S., and Khodakhah, K. (2019). Cerebellar modulation of the reward circuitry and social behavior. Science 363:eaav0581. doi: 10.1126/science.aav0581
Castellazzi, G., Bruno, S. D., Toosy, A. T., Casiraghi, L., Palesi, F., Savini, G., et al. (2018). Prominent changes in Cerebro-cerebellar functional connectivity during continuous cognitive processing. Front. Cell. Neurosci. 12:331. doi: 10.3389/fncel.2018.00331
Castellazzi, G., Palesi, F., Casali, S., Vitali, P., Sinforiani, E., Wheeler-Kingshott, C. A., et al. (2014). A comprehensive assessment of resting state networks: bidirectional modification of functional integrity in cerebro-cerebellar networks in dementia. Front. Neurosci. 8:223,
Cattarinussi, G., Delvecchio, G., Sambataro, F., and Brambilla, P. (2022). The effect of polygenic risk scores for major depressive disorder, bipolar disorder and schizophrenia on morphological brain measures: a systematic review of the evidence. J. Affect. Disord. 310, 213–222. doi: 10.1016/j.jad.2022.05.007
Catts, V. S., Lai, Y. L., Weickert, C. S., Weickert, T. W., and Catts, S. V. (2016). A quantitative review of the postmortem evidence for decreased cortical N-methyl-d-aspartate receptor expression levels in schizophrenia: how can we link molecular abnormalities to mismatch negativity deficits? Biol. Psychol. 116, 57–67. doi: 10.1016/j.biopsycho.2015.10.013
Charlson, F. J., Ferrari, A. J., Santomauro, D. F., Diminic, S., Stockings, E., Scott, J. G., et al. (2018). Global epidemiology and burden of schizophrenia: findings from the global burden of disease study 2016. Schizophr. Bull. 44, 1195–1203. doi: 10.1093/schbul/sby058
Chauhan, P., Garg, S., Tikka, S. K., and Khattri, S. (2021). Efficacy of intensive cerebellar intermittent Theta burst stimulation (iCiTBS) in treatment-resistant schizophrenia: a randomized placebo-controlled study. Cerebellum 20, 116–123. doi: 10.1007/s12311-020-01193-9
Chauhan, P. S., Misra, U. K., Kalita, J., Chandravanshi, L. P., and Khanna, V. K. (2016). Memory and learning seems to be related to cholinergic dysfunction in the JE rat model. Physiol. Behav. 156, 148–155. doi: 10.1016/j.physbeh.2016.01.006
Chen, X., Jiang, Y., Chen, L., He, H., Dong, L., Hou, C., et al. (2017). Altered Hippocampo-Cerebello-cortical circuit in schizophrenia by a spatiotemporal consistency and causal connectivity analysis. Front. Neurosci. 11:25. doi: 10.3389/fnins.2017.00025
Chen, Q., Li, D., Jin, W., Shi, Y., Li, Z., Ma, P., et al. (2021). Research Progress on the correlation between epigenetics and schizophrenia. Front. Neurosci. 15:688727. doi: 10.3389/fnins.2021.688727
Chen, Y. L., Tu, P. C., Lee, Y. C., Chen, Y. S., Li, C. T., and Su, T. P. (2013). Resting-state fMRI mapping of cerebellar functional dysconnections involving multiple large-scale networks in patients with schizophrenia. Schizophr. Res. 149, 26–34. doi: 10.1016/j.schres.2013.05.029
Chen, P., Ye, E., Jin, X., Zhu, Y., and Wang, L. (2019). Association between Thalamocortical functional connectivity abnormalities and cognitive deficits in schizophrenia. Sci. Rep. 9:2952. doi: 10.1038/s41598-019-39367-z
Chiou, L. C., Lee, H. J., Ernst, M., Huang, W. J., Chou, J. F., Chen, H. L., et al. (2018). Cerebellar α(6) -subunit-containing GABA(a) receptors: a novel therapeutic target for disrupted prepulse inhibition in neuropsychiatric disorders. Br. J. Pharmacol. 175, 2414–2427. doi: 10.1111/bph.14198
Choi, S. Y., Ha, M., Choi, S., Moon, S.-Y., Park, S., Kim, M., et al. (2023). Altered intrinsic cerebellar-cerebral functional connectivity is related to negative symptoms in patients with first-episode psychosis. Schizophr. Res. 252, 56–63. doi: 10.1016/j.schres.2022.12.041
Ciapponi, C., Li, Y., Osorio Becerra, D. A., Rodarie, D., Casellato, C., Mapelli, L., et al. (2023). Variations on the theme: focus on cerebellum and emotional processing. Front. Syst. Neurosci. 17:5752. doi: 10.3389/fnsys.2023.1185752
Citri, A., and Malenka, R. C. (2008). Synaptic plasticity: multiple forms, functions, and mechanisms. Neuropsychopharmacology 33, 18–41. doi: 10.1038/sj.npp.1301559
Collin, G., Hulshoff Pol, H. E., Haijma, S. V., Cahn, W., Kahn, R. S., and van den Heuvel, M. P. (2011). Impaired cerebellar functional connectivity in schizophrenia patients and their healthy siblings. Front. Psych. 2:73. doi: 10.3389/fpsyt.2011.00073
Constantinides, C., Han, L. K. M., Alloza, C., Antonucci, L. A., Arango, C., Ayesa-Arriola, R., et al. (2023). Brain ageing in schizophrenia: evidence from 26 international cohorts via the ENIGMA schizophrenia consortium. Mol. Psychiatry 28, 1201–1209. doi: 10.1038/s41380-022-01897-w
Contestabile, A. (2002). Cerebellar granule cells as a model to study mechanisms of neuronal apoptosis or survival in vivo and in vitro. Cerebellum 1, 41–55. doi: 10.1080/147342202753203087
Correll, C. U., Solmi, M., Cortese, S., Fava, M., Højlund, M., Kraemer, H. C., et al. (2023). The future of psychopharmacology: a critical appraisal of ongoing phase 2/3 trials, and of some current trends aiming to de-risk trial programmes of novel agents. World Psychiatry 22, 48–74. doi: 10.1002/wps.21056
Correll, C. U., Solmi, M., Croatto, G., Schneider, L. K., Rohani-Montez, S. C., Fairley, L., et al. (2022). Mortality in people with schizophrenia: a systematic review and meta-analysis of relative risk and aggravating or attenuating factors. World Psychiatry 21, 248–271. doi: 10.1002/wps.20994
Creese, I., Burt, D. R., and Snyder, S. H. (1996). Dopamine receptor binding predicts clinical and pharmacological potencies of antischizophrenic drugs. J. Neuropsychiatry Clin. Neurosci. 8, 223–226
Crook, J. M., Tomaskovic-Crook, E., Copolov, D. L., and Dean, B. (2000). Decreased muscarinic receptor binding in subjects with schizophrenia: a study of the human hippocampal formation. Biol. Psychiatry 48, 381–388. doi: 10.1016/S0006-3223(00)00918-5
Crossley, N. A., Zugman, A., Reyes-Madrigal, F., Czepielewski, L. S., Castro, M. N., Diaz-Zuluaga, A. M., et al. (2021). Structural brain abnormalities in schizophrenia in adverse environments: examining the effect of poverty and violence in six Latin American cities. Br. J. Psychiatry 218, 112–118. doi: 10.1192/bjp.2020.143
Cuesta, M. J., Moreno-Izco, L., Ribeiro, M., López-Ilundain, J. M., Lecumberri, P., Cabada, T., et al. (2018). Motor abnormalities and cognitive impairment in first-episode psychosis patients, their unaffected siblings and healthy controls. Schizophr. Res. 200, 50–55. doi: 10.1016/j.schres.2017.10.035
Cutando, L., Puighermanal, E., Castell, L., Tarot, P., Belle, M., Bertaso, F., et al. (2022). Cerebellar dopamine D2 receptors regulate social behaviors. Nat. Neurosci. 25, 900–911. doi: 10.1038/s41593-022-01092-8
D'Angelo, E. (2018). “Chapter 6 – physiology of the cerebellum” in Handbook of clinical neurology. eds. M. Manto and T. A. G. M. Huisman, vol. 154 (Amsterdam: Elsevier), 85–108.
D'Angelo, E., and Casali, S. (2012). Seeking a unified framework for cerebellar function and dysfunction: from circuit operations to cognition. Front. Neural Circuits 6:116. doi: 10.3389/fncir.2012.00116
D'Angelo, E., and De Zeeuw, C. I. (2009). Timing and plasticity in the cerebellum: focus on the granular layer. Trends Neurosci. 32, 30–40. doi: 10.1016/j.tins.2008.09.007
D'Angelo, E., and Jirsa, V. (2022). The quest for multiscale brain modeling. Trends Neurosci. 45, 777–790. doi: 10.1016/j.tins.2022.06.007
D'Angelo, E., Mapelli, L., Casellato, C., Garrido, J. A., Luque, N., Monaco, J., et al. (2016). Distributed circuit plasticity: new clues for the cerebellar mechanisms of learning. Cerebellum 15, 139–151. doi: 10.1007/s12311-015-0711-7
D'Angelo, E., Rossi, P., and Garthwaite, J. (1990). Dual-component NMDA receptor currents at a single central synapse. Nature 346, 467–470. doi: 10.1038/346467a0
Daskalakis, Z. J., Christensen, B. K., Fitzgerald, P. B., Fountain, S. I., and Chen, R. (2005). Reduced cerebellar inhibition in schizophrenia: a preliminary study. Am. J. Psychiatry 162, 1203–1205. doi: 10.1176/appi.ajp.162.6.1203
Dauvermann, M. R., Mothersill, D., Rokita, K. I., King, S., Holleran, L., Kane, R., et al. (2021). Changes in default-mode network associated with childhood trauma in schizophrenia. Schizophr. Bull. 47, 1482–1494. doi: 10.1093/schbul/sbab025
Davies, G., Lam, M., Harris, S. E., Trampush, J. W., Luciano, M., Hill, W. D., et al. (2018). Study of 300,486 individuals identifies 148 independent genetic loci influencing general cognitive function. Nat. Commun. 9:2098. doi: 10.1038/s41467-018-04362-x
Davis, J., Eyre, H., Jacka, F. N., Dodd, S., Dean, O., McEwen, S., et al. (2016). A review of vulnerability and risks for schizophrenia: beyond the two hit hypothesis. Neurosci. Biobehav. Rev. 65, 185–194. doi: 10.1016/j.neubiorev.2016.03.017
Davis, K. L., Kahn, R. S., Ko, G., and Davidson, M. (1991). Dopamine in schizophrenia: a review and reconceptualization. Am. J. Psychiatry 148, 1474–1486
de Bartolomeis, A., Ciccarelli, M., De Simone, G., Mazza, B., Barone, A., and Vellucci, L. (2023). Canonical and non-canonical antipsychotics’ dopamine-related mechanisms of present and next generation molecules: a systematic review on translational highlights for treatment response and treatment-resistant schizophrenia. Int. J. Mol. Sci. 24:5945. doi: 10.3390/ijms24065945
de la Fuente-Sandoval, C., León-Ortiz, P., Azcárraga, M., Stephano, S., Favila, R., Díaz-Galvis, L., et al. (2013). Glutamate levels in the associative striatum before and after 4 weeks of antipsychotic treatment in first-episode psychosis: a longitudinal proton magnetic resonance spectroscopy study. JAMA Psychiatry 70, 1057–1066. doi: 10.1001/jamapsychiatry.2013.289
de Lacalle, S., Hersh, L. B., and Saper, C. B. (1993). Cholinergic innervation of the human cerebellum. J. Comp. Neurol. 328, 364–376. doi: 10.1002/cne.903280304
De Schepper, R., Geminiani, A., Masoli, S., Rizza, M. F., Antonietti, A., Casellato, C., et al. (2022). Model simulations unveil the structure-function-dynamics relationship of the cerebellar cortical microcircuit. Commun Biol. 5:1240. doi: 10.1038/s42003-022-04213-y
Dean, B. (2002). Understanding the pathology of schizophrenia: recent advances from the study of the molecular architecture of postmortem CNS tissue. Postgrad. Med. J. 78, 142–148. doi: 10.1136/pmj.78.917.142
Dean, P., and Porrill, J. (2014). Decorrelation learning in the cerebellum: computational analysis and experimental questions. Prog. Brain. Res. 210, 157–92. doi: 10.1016/B978-0-444-63356-9.00007-8
Delgado-Sallent, C., Gener, T., Nebot, P., López-Cabezón, C., and Puig, M. V. (2023). Neural substrates of cognitive impairment in a NMDAR hypofunction mouse model of schizophrenia and partial rescue by risperidone. Front. Cell. Neurosci. 17:1152248. doi: 10.3389/fncel.2023.1152248
DeLisi, L. E. (2009). “An overview and current perspective on family studies of schizophrenia” in Handbook of neurochemistry and molecular neurobiology: Schizophrenia. eds. A. Lajtha, D. Javitt, and J. Kantrowitz (Boston, MA: Springer US), 493–504.
DeLisi, L. E. (2022). Redefining schizophrenia through genetics: a commentary on 50 years searching for biological causes. Schizophr. Res. 242, 22–24. doi: 10.1016/j.schres.2021.11.017
Delunardo, F., Soldati, D., Bellisario, V., Berry, A., Camerini, S., Crescenzi, M., et al. (2016). Anti-GAPDH autoantibodies as a pathogenic determinant and potential biomarker of neuropsychiatric diseases. Arthritis Rheumatol. 68, 2708–2716. doi: 10.1002/art.39750
Demirtas-Tatlidede, A., Freitas, C., Cromer, J. R., Safar, L., Ongur, D., Stone, W. S., et al. (2010). Safety and proof of principle study of cerebellar vermal theta burst stimulation in refractory schizophrenia. Schizophr. Res. 124, 91–100. doi: 10.1016/j.schres.2010.08.015
Di Ciano, P., Cormick, P. M., Stefan, C., Wong, E., Kim, A., Remington, G., et al. (2017). The effects of buspirone on occupancy of dopamine receptors and the rat gambling task. Psychopharmacology 234, 3309–3320. doi: 10.1007/s00213-017-4715-5
Dienel, S. J., Fish, K. N., and Lewis, D. A. (2023). The nature of prefrontal cortical GABA neuron alterations in schizophrenia: markedly lower somatostatin and Parvalbumin gene expression without missing neurons. Am. J. Psychiatry 180, 495–507. doi: 10.1176/appi.ajp.20220676
Dieudonné, S., and Dumoulin, A. (2000). Serotonin-driven long-range inhibitory connections in the cerebellar cortex. J. Neurosci. 20, 1837–1848. doi: 10.1523/JNEUROSCI.20-05-01837.2000
Dimitriadis, S. I., Perry, G., Foley, S. F., Tansey, K. E., Jones, D. K., Holmans, P., et al. (2021). Genetic risk for schizophrenia is associated with altered visually-induced gamma band activity: evidence from a population sample stratified polygenic risk. Transl. Psychiatry 11:592. doi: 10.1038/s41398-021-01678-z
Ding, Y., Ou, Y., Pan, P., Shan, X., Chen, J., Liu, F., et al. (2019). Cerebellar structural and functional abnormalities in first-episode and drug-naive patients with schizophrenia: a meta-analysis. Psychiatry Res. Neuroimaging 283, 24–33. doi: 10.1016/j.pscychresns.2018.11.009
D'Mello, A. M., Crocetti, D., Mostofsky, S. H., and Stoodley, C. J. (2015). Cerebellar gray matter and lobular volumes correlate with core autism symptoms. Neuroimage Clin. 7, 631–639. doi: 10.1016/j.nicl.2015.02.007
Douet, V., Chang, L., Pritchett, A., Lee, K., Keating, B., Bartsch, H., et al. (2014). Schizophrenia-risk variant rs6994992 in the neuregulin-1 gene on brain developmental trajectories in typically developing children. Transl. Psychiatry 4:e392. doi: 10.1038/tp.2014.41
Duxon, M. S., Flanigan, T. P., Reavley, A. C., Baxter, G. S., Blackburn, T. P., and Fone, K. C. (1997). Evidence for expression of the 5-hydroxytryptamine-2B receptor protein in the rat central nervous system. Neuroscience 76, 323–329. doi: 10.1016/S0306-4522(96)00480-0
Eastwood, S. L., Burnet, P. W., Gittins, R., Baker, K., and Harrison, P. J. (2001). Expression of serotonin 5-HT(2A) receptors in the human cerebellum and alterations in schizophrenia. Synapse 42, 104–114. doi: 10.1002/syn.1106
Edwards, A. C., Bacanu, S. A., Bigdeli, T. B., Moscati, A., and Kendler, K. S. (2016). Evaluating the dopamine hypothesis of schizophrenia in a large-scale genome-wide association study. Schizophr. Res. 176, 136–140. doi: 10.1016/j.schres.2016.06.016
Eggers, A. E. (2013). A serotonin hypothesis of schizophrenia. Med. Hypotheses 80, 791–794. doi: 10.1016/j.mehy.2013.03.013
Elert, E. (2014). Aetiology: searching for schizophrenia's roots. Nature 508, S2–S3. doi: 10.1038/508S2a
Emily Simmonds, A. P., Anney, R., et al. (2023). Common risk alleles for schizophrenia within the major histocompatibility complex predict white matter microstructure. Transl. Psychiatry 14:194. doi: 10.1038/s41398-024-02910-2
Escelsior, A., and Belvederi Murri, M. (2019). Modulation of cerebellar activity in schizophrenia: is it the time for clinical trials? Schizophr. Bull. 45, 947–949. doi: 10.1093/schbul/sbz017
Fair, D. A., Cohen, A. L., Power, J. D., Dosenbach, N. U., Church, J. A., Miezin, F. M., et al. (2009). Functional brain networks develop from a "local to distributed" organization. PLoS Comput. Biol. 5:e1000381. doi: 10.1371/journal.pcbi.1000381
Fatemi, S. H., and Folsom, T. D. (2009). The neurodevelopmental hypothesis of schizophrenia, revisited. Schizophr. Bull. 35, 528–548. doi: 10.1093/schbul/sbn187
Fatemi, S. H., and Folsom, T. D. (2015). GABA receptor subunit distribution and FMRP-mGluR5 signaling abnormalities in the cerebellum of subjects with schizophrenia, mood disorders, and autism. Schizophr. Res. 167, 42–56. doi: 10.1016/j.schres.2014.10.010
Fatemi, S. H., Folsom, T. D., Rooney, R. J., and Thuras, P. D. (2013). mRNA and protein expression for novel GABAA receptors θ and ρ2 are altered in schizophrenia and mood disorders; relevance to FMRP-mGluR5 signaling pathway. Transl. Psychiatry 3:e271. doi: 10.1038/tp.2013.46
Fatemi, S. H., Stary, J. M., Earle, J. A., Araghi-Niknam, M., and Eagan, E. (2005). GABAergic dysfunction in schizophrenia and mood disorders as reflected by decreased levels of glutamic acid decarboxylase 65 and 67 kDa and Reelin proteins in cerebellum. Schizophr. Res. 72, 109–122. doi: 10.1016/j.schres.2004.02.017
Fatouros-Bergman, H., Cervenka, S., Flyckt, L., Edman, G., and Farde, L. (2014). Meta-analysis of cognitive performance in drug-naïve patients with schizophrenia. Schizophr. Res. 158, 156–162. doi: 10.1016/j.schres.2014.06.034
Feng, S., Zheng, S., Zou, H., Dong, L., Zhu, H., Liu, S., et al. (2022). Altered functional connectivity of cerebellar networks in first-episode schizophrenia. Front. Cell. Neurosci. 16:1024192. doi: 10.3389/fncel.2022.1024192
Fernandez-Cabello, S., Alnæs, D., van der Meer, D., Dahl, A., Holm, M., Kjelkenes, R., et al. (2022). Associations between brain imaging and polygenic scores of mental health and educational attainment in children aged 9-11. NeuroImage 263:119611. doi: 10.1016/j.neuroimage.2022.119611
Fett, A. J., Velthorst, E., Reichenberg, A., Ruggero, C. J., Callahan, J. L., Fochtmann, L. J., et al. (2020). Long-term changes in cognitive functioning in individuals with psychotic disorders: findings from the Suffolk County mental health project. JAMA Psychiatry 77, 387–396. doi: 10.1001/jamapsychiatry.2019.3993
Flace, P., Livrea, P., Basile, G. A., Galletta, D., Bizzoca, A., Gennarini, G., et al. (2021). The cerebellar dopaminergic system. Front. Syst. Neurosci. 15:650614. doi: 10.3389/fnsys.2021.650614
Fleming, E., and Hull, C. (2019). Serotonin regulates dynamics of cerebellar granule cell activity by modulating tonic inhibition. J. Neurophysiol. 121, 105–114. doi: 10.1152/jn.00492.2018
Fonteneau, C., Redoute, J., Haesebaert, F., Le Bars, D., Costes, N., Suaud-Chagny, M. F., et al. (2018). Frontal transcranial direct current stimulation induces dopamine release in the ventral striatum in human. Cereb. Cortex 28, 2636–2646. doi: 10.1093/cercor/bhy093
Foster, D. J., Bryant, Z. K., and Conn, P. J. (2021). Targeting muscarinic receptors to treat schizophrenia. Behav. Brain Res. 405:113201. doi: 10.1016/j.bbr.2021.113201
Frangou, S. (2014). A systems neuroscience perspective of schizophrenia and bipolar disorder. Schizophr. Bull. 40, 523–531. doi: 10.1093/schbul/sbu017
Frankiewicz, T., Potier, B., Bashir, Z. I., Collingridge, G. L., and Parsons, C. G. (1996). Effects of memantine and MK-801 on NMDA-induced currents in cultured neurones and on synaptic transmission and LTP in area CA1 of rat hippocampal slices. Br. J. Pharmacol. 117, 689–697. doi: 10.1111/j.1476-5381.1996.tb15245.x
Friston, K., Brown, H. R., Siemerkus, J., and Stephan, K. E. (2016). The dysconnection hypothesis. Schizophr. Res. 176, 83–94. doi: 10.1016/j.schres.2016.07.014
Fujihara, K. (2023). Beyond the γ-aminobutyric acid hypothesis of schizophrenia. Front. Cell. Neurosci. 17:1161608. doi: 10.3389/fncel.2023.1161608
Fujita, H., Kodama, T., and du Lac, S. (2020). Modular output circuits of the fastigial nucleus for diverse motor and nonmotor functions of the cerebellar vermis. eLife 9:58613. doi: 10.7554/eLife.58613
Gambosi, B., Sheiban, F. J., Biasizzo, M., Antonietti, A., D’Angelo, E., Mazzoni, A., et al. (2023). Dopamine-dependent cerebellar dysfunction enhances beta oscillations and disrupts motor learning in a multiarea model. bioRxiv :2023.2007.2018.549459. doi: 10.1101/2023.07.18.549459
Gao, Z., Proietti-Onori, M., Lin, Z., Ten Brinke, M. M., Boele, H. J., Potters, J. W., et al. (2016). Excitatory cerebellar Nucleocortical circuit provides internal amplification during associative conditioning. Neuron 89, 645–657. doi: 10.1016/j.neuron.2016.01.008
Gawande, D. Y., Narasimhan, K. K. S., Shelkar, G. P., Pavuluri, R., Stessman, H. A. F., and Dravid, S. M. (2023). GluN2D subunit in parvalbumin interneurons regulates prefrontal cortex feed-forward inhibitory circuit and molecular networks relevant to schizophrenia. Biol. Psychiatry 94, 297–309. doi: 10.1016/j.biopsych.2023.03.020
Giersch, A., Lalanne, L., and Isope, P. (2016). Implicit timing as the missing link between neurobiological and self disorders in schizophrenia? Front. Hum. Neurosci. 10:303. doi: 10.3389/fnhum.2016.00303
Goldman-Rakic, P. S., Castner, S. A., Svensson, T. H., Siever, L. J., and Williams, G. V. (2004). Targeting the dopamine D1 receptor in schizophrenia: insights for cognitive dysfunction. Psychopharmacology 174, 3–16. doi: 10.1007/s00213-004-1793-y
Gonzalez-Burgos, G., and Lewis, D. A. (2012). NMDA receptor hypofunction, parvalbumin-positive neurons, and cortical gamma oscillations in schizophrenia. Schizophr. Bull. 38, 950–957. doi: 10.1093/schbul/sbs010
Grimaldi, G., Argyropoulos, G. P., Boehringer, A., Celnik, P., Edwards, M. J., Ferrucci, R., et al. (2014). Non-invasive cerebellar stimulation—a consensus paper. Cerebellum 13, 121–138. doi: 10.1007/s12311-013-0514-7
Guidotti, A., Pesold, C., and Costa, E. (2000). New neurochemical markers for psychosis: a working hypothesis of their operation. Neurochem. Res. 25, 1207–1218. doi: 10.1023/A:1007635927069
Guo, W., Zhang, F., Liu, F., Chen, J., Wu, R., Chen, D. Q., et al. (2018). Cerebellar abnormalities in first-episode, drug-naive schizophrenia at rest. Psychiatry Res. Neuroimaging 276, 73–79. doi: 10.1016/j.pscychresns.2018.03.010
Hall, J., and Bray, N. J. (2022). Schizophrenia genomics: convergence on synaptic development, adult synaptic plasticity, or both? Biol. Psychiatry 91, 709–717. doi: 10.1016/j.biopsych.2021.10.018
Hamilton, H. K., Roach, B. J., Cavus, I., Teyler, T. J., Clapp, W. C., Ford, J. M., et al. (2020). Impaired potentiation of Theta oscillations during a visual cortical plasticity paradigm in individuals with schizophrenia. Front. Psych. 11:590567. doi: 10.3389/fpsyt.2020.590567
Hanaie, R., Mohri, I., Kagitani-Shimono, K., Tachibana, M., Matsuzaki, J., Hirata, I., et al. (2018). Aberrant cerebellar–cerebral functional connectivity in children and adolescents with autism Spectrum disorder. Front. Hum. Neurosci. 12:454. doi: 10.3389/fnhum.2018.00454
Hansel, C., Linden, D. J., and D'Angelo, E. (2001). Beyond parallel fiber LTD: the diversity of synaptic and non-synaptic plasticity in the cerebellum. Nat. Neurosci. 4, 467–475. doi: 10.1038/87419
Hasan, A., Nitsche, M. A., Rein, B., Schneider-Axmann, T., Guse, B., Gruber, O., et al. (2011). Dysfunctional long-term potentiation-like plasticity in schizophrenia revealed by transcranial direct current stimulation. Behav. Brain Res. 224, 15–22. doi: 10.1016/j.bbr.2011.05.017
He, H., Luo, C., Luo, Y., Duan, M., Yi, Q., Biswal, B. B., et al. (2019). Reduction in gray matter of cerebellum in schizophrenia and its influence on static and dynamic connectivity. Hum. Brain Mapp. 40, 517–528. doi: 10.1002/hbm.24391
Heath, R. G., Llewellyn, R. C., and Rouchell, A. M. (1980). The cerebellar pacemaker for intractable behavioral disorders and epilepsy: follow-up report. Biol. Psychiatry 15, 243–256
Heath, R. G., Rouchell, A. M., Llewellyn, R. C., and Walker, C. F. (1981). Cerebellar pacemaker patients: an update. Biol. Psychiatry 16, 953–962
Heck, D. H., Fox, M. B., Correia Chapman, B., McAfee, S. S., and Liu, Y. (2023). Cerebellar control of thalamocortical circuits for cognitive function: a review of pathways and a proposed mechanism. Front. Syst. Neurosci. 17:1126508. doi: 10.3389/fnsys.2023.1126508
Hedges, E. P., See, C., Si, S., McGuire, P., Dickson, H., and Kempton, M. J. (2022). Meta-analysis of longitudinal neurocognitive performance in people at clinical high-risk for psychosis. Psychol. Med. 52, 2009–2016. doi: 10.1017/S0033291722001830
Holloway, Z. R., Paige, N. B., Comstock, J. F., Nolen, H. G., Sable, H. J., and Lester, D. B. (2019). Cerebellar modulation of mesolimbic dopamine transmission is functionally asymmetrical. Cerebellum 18, 922–931. doi: 10.1007/s12311-019-01074-w
Howes, O. D., Cummings, C., Chapman, G. E., and Shatalina, E. (2023). Neuroimaging in schizophrenia: an overview of findings and their implications for synaptic changes. Neuropsychopharmacology 48, 151–167. doi: 10.1038/s41386-022-01426-x
Howes, O. D., Kambeitz, J., Kim, E., Stahl, D., Slifstein, M., Abi-Dargham, A., et al. (2012). The nature of dopamine dysfunction in schizophrenia and what this means for treatment. Arch. Gen. Psychiatry 69, 776–786. doi: 10.1001/archgenpsychiatry.2012.169
Howes, O. D., and Kapur, S. (2009). The dopamine hypothesis of schizophrenia: version III—The final common pathway. Schizophr. Bull. 35, 549–562. doi: 10.1093/schbul/sbp006
Hu, X., Wang, S., Zhou, H., Li, N., Zhong, C., Luo, W., et al. (2023). Altered functional connectivity strength in distinct brain networks of children with early-onset schizophrenia. J. Magn. Reson. Imaging 58, 1617–1623. doi: 10.1002/jmri.28682
Hua, J. P. Y., Abram, S. V., and Ford, J. M. (2022). Cerebellar stimulation in schizophrenia: a systematic review of the evidence and an overview of the methods. Front. Psych. 13:1069488. doi: 10.3389/fpsyt.2022.1069488
Huang, C., Mattis, P., Tang, C., Perrine, K., Carbon, M., and Eidelberg, D. (2007). Metabolic brain networks associated with cognitive function in Parkinson's disease. NeuroImage 34, 714–723. doi: 10.1016/j.neuroimage.2006.09.003
Huerta, P. T., and Lisman, J. E. (1993). Heightened synaptic plasticity of hippocampal CA1 neurons during a cholinergically induced rhythmic state. Nature 364, 723–725. doi: 10.1038/364723a0
Hughes, D. E., Kunitoki, K., Elyounssi, S., Luo, M., Bazer, O. M., Hopkinson, C. E., et al. (2023). Genetic patterning for child psychopathology is distinct from that for adults and implicates fetal cerebellar development. Nat. Neurosci. 26, 959–969. doi: 10.1038/s41593-023-01321-8
Hunt, G. E., Large, M. M., Cleary, M., Lai, H. M. X., and Saunders, J. B. (2018). Prevalence of comorbid substance use in schizophrenia spectrum disorders in community and clinical settings, 1990-2017: systematic review and meta-analysis. Drug Alcohol Depend. 191, 234–258. doi: 10.1016/j.drugalcdep.2018.07.011
Ikai, Y., Takada, M., and Mizuno, N. (1994). Single neurons in the ventral tegmental area that project to both the cerebral and cerebellar cortical areas by way of axon collaterals. Neuroscience 61, 925–934. doi: 10.1016/0306-4522(94)90413-8
Ito, M. (2008). Control of mental activities by internal models in the cerebellum. Nat. Rev. Neurosci. 9, 304–313. doi: 10.1038/nrn2332
Jaarsma, D., Ruigrok, T. J. H., Caffé, R., Cozzari, C., Levey, A. I., Mugnaini, E., et al. (1997). “Chapter 5 cholinergic innervation and receptors in the cerebellum” in Progress in brain research. eds. D. C. I. Zeeuw, P. Strata, and J. Voogd, vol. 114 (Amsterdam: Elsevier), 67–96.
Jacobi, H., Faber, J., Timmann, D., and Klockgether, T. (2021). Update cerebellum and cognition. J. Neurol. 268, 3921–3925. doi: 10.1007/s00415-021-10486-w
Jaffe, A. E., Straub, R. E., Shin, J. H., Tao, R., Gao, Y., Collado-Torres, L., et al. (2018). Developmental and genetic regulation of the human cortex transcriptome illuminate schizophrenia pathogenesis. Nat. Neurosci. 21, 1117–1125. doi: 10.1038/s41593-018-0197-y
Jagannath, V., Marinova, Z., Monoranu, C. M., Walitza, S., and Grünblatt, E. (2017). Expression of D-amino acid oxidase (DAO/DAAO) and D-amino acid oxidase activator (DAOA/G72) during development and aging in the human post-mortem brain. Front. Neuroanat. 11:31. doi: 10.3389/fnana.2017.00031
Javitt, D. C. (2023). Cognitive impairment associated with schizophrenia: from pathophysiology to treatment. Annu. Rev. Pharmacol. Toxicol. 63, 119–141. doi: 10.1146/annurev-pharmtox-051921-093250
Jelen, L. A., King, S., Horne, C. M., Lythgoe, D. J., Young, A. H., and Stone, J. M. (2019). Functional magnetic resonance spectroscopy in patients with schizophrenia and bipolar affective disorder: glutamate dynamics in the anterior cingulate cortex during a working memory task. Eur. Neuropsychopharmacol. 29, 222–234. doi: 10.1016/j.euroneuro.2018.12.005
Ji, J. L., Diehl, C., Schleifer, C., Tamminga, C. A., Keshavan, M. S., Sweeney, J. A., et al. (2019). Schizophrenia exhibits bi-directional brain-wide alterations in Cortico-Striato-cerebellar circuits. Cereb. Cortex 29, 4463–4487. doi: 10.1093/cercor/bhy306
Jones, P. B. (2013). Adult mental health disorders and their age at onset. Br. J. Psychiatry Suppl. 54, s5–s10. doi: 10.1192/bjp.bp.112.119164
Kansal, K., Yang, Z., Fishman, A. M., Sair, H. I., Ying, S. H., Jedynak, B. M., et al. (2017). Structural cerebellar correlates of cognitive and motor dysfunctions in cerebellar degeneration. Brain 140, 707–720. doi: 10.1093/brain/aww327
Karlsgodt, K. H., Sun, D., and Cannon, T. D. (2010). Structural and functional brain abnormalities in schizophrenia. Curr. Dir. Psychol. Sci. 19, 226–231. doi: 10.1177/0963721410377601
Katz Shroitman, N., Yitzhaky, A., Ben Shachar, D., Gurwitz, D., and Hertzberg, L. (2023). Meta-analysis of brain samples of individuals with schizophrenia detects down-regulation of multiple ATP synthase encoding genes in both females and males. J. Psychiatr. Res. 158, 350–359. doi: 10.1016/j.jpsychires.2023.01.005
Kaufmann, T., van der Meer, D., Doan, N. T., Schwarz, E., Lund, M. J., Agartz, I., et al. (2019). Common brain disorders are associated with heritable patterns of apparent aging of the brain. Nat. Neurosci. 22, 1617–1623. doi: 10.1038/s41593-019-0471-7
Kesby, J. P., Eyles, D. W., McGrath, J. J., and Scott, J. G. (2018). Dopamine, psychosis and schizophrenia: the widening gap between basic and clinical neuroscience. Transl. Psychiatry 8:30. doi: 10.1038/s41398-017-0071-9
Keshavan, M. S., and Hogarty, G. E. (1999). Brain maturational processes and delayed onset in schizophrenia. Dev. Psychopathol. 11, 525–543. doi: 10.1017/S0954579499002199
Keshri, N., and Nandeesha, H. (2023). Dysregulation of synaptic plasticity markers in schizophrenia. Indian J. Clin. Biochem. 38, 4–12. doi: 10.1007/s12291-022-01068-2
Khalil, M., Hollander, P., Raucher-Chéné, D., Lepage, M., and Lavigne, K. M. (2022). Structural brain correlates of cognitive function in schizophrenia: a meta-analysis. Neurosci. Biobehav. Rev. 132, 37–49. doi: 10.1016/j.neubiorev.2021.11.034
Khandaker, G. M., Cousins, L., Deakin, J., Lennox, B. R., Yolken, R., and Jones, P. B. (2015). Inflammation and immunity in schizophrenia: implications for pathophysiology and treatment. Lancet Psychiatry 2, 258–270. doi: 10.1016/S2215-0366(14)00122-9
Kiltschewskij, D. J., Reay, W. R., Geaghan, M. P., Atkins, J. R., Xavier, A., Zhang, X., et al. (2023). Alteration of DNA methylation and epigenetic scores associated with features of schizophrenia and common variant genetic risk. Biol. Psychiatry 95, 647–661. doi: 10.1016/j.biopsych.2023.07.010
Kim, S. E., Jung, S., Sung, G., Bang, M., and Lee, S.-H. (2021). Impaired cerebro-cerebellar white matter connectivity and its associations with cognitive function in patients with schizophrenia. NPJ Schizophr. 7:38. doi: 10.1038/s41537-021-00169-w
Kimura, Y., Nakazawa, S., Nishigori, K., Mori, Y., Ichihara, J., and Yoshioka, Y. (2023). Ultra-high-field pharmacological functional MRI of dopamine D1 receptor-related interventions in anesthetized rats. Pharmacol. Res. Perspect. 11:e01055. doi: 10.1002/prp2.1055
King, S., Mothersill, D., Holleran, L., Patlola, S. R., Burke, T., McManus, R., et al. (2023). Early life stress, low-grade systemic inflammation and weaker suppression of the default mode network (DMN) during face processing in schizophrenia. Transl. Psychiatry 13:213. doi: 10.1038/s41398-023-02512-4
Knowles, E. E., David, A. S., and Reichenberg, A. (2010). Processing speed deficits in schizophrenia: reexamining the evidence. Am. J. Psychiatry 167, 828–835. doi: 10.1176/appi.ajp.2010.09070937
Koch, G. (2010). Repetitive transcranial magnetic stimulation: a tool for human cerebellar plasticity. Funct. Neurol. 25, 159–163
Koch, K., Wagner, G., Dahnke, R., Schachtzabel, C., Schultz, C., Roebel, M., et al. (2010). Disrupted white matter integrity of corticopontine-cerebellar circuitry in schizophrenia. Eur. Arch. Psychiatry Clin. Neurosci. 260, 419–426. doi: 10.1007/s00406-009-0087-0
Kochunov, P., Coyle, T. R., Rowland, L. M., Jahanshad, N., Thompson, P. M., Kelly, S., et al. (2017). Association of White Matter with Core Cognitive Deficits in patients with schizophrenia. JAMA Psychiatry 74, 958–966. doi: 10.1001/jamapsychiatry.2017.2228
Kölker, S. (2018). Metabolism of amino acid neurotransmitters: the synaptic disorder underlying inherited metabolic diseases. J. Inherit. Metab. Dis. 41, 1055–1063. doi: 10.1007/s10545-018-0201-4
Konopaske, G. T., Lange, N., Coyle, J. T., and Benes, F. M. (2014). Prefrontal cortical dendritic spine pathology in schizophrenia and bipolar disorder. JAMA Psychiatry 71, 1323–1331. doi: 10.1001/jamapsychiatry.2014.1582
Krystal, J. H., Karper, L. P., Seibyl, J. P., Freeman, G. K., Delaney, R., Bremner, J. D., et al. (1994). Subanesthetic effects of the noncompetitive NMDA antagonist, ketamine, in humans. Psychotomimetic, perceptual, cognitive, and neuroendocrine responses. Arch. Gen. Psychiatry 51, 199–214. doi: 10.1001/archpsyc.1994.03950030035004
Laidi, C., Levenes, C., Suarez-Perez, A., Février, C., Durand, F., Bouaziz, N., et al. (2020). Cognitive impact of cerebellar non-invasive stimulation in a patient with schizophrenia. Front. Psych. 11:174. doi: 10.3389/fpsyt.2020.00174
Lecrux, C., Sandoe, C. H., Neupane, S., Kropf, P., Toussay, X., Tong, X. K., et al. (2017). Impact of altered cholinergic tones on the neurovascular coupling response to whisker stimulation. J. Neurosci. 37, 1518–1531. doi: 10.1523/JNEUROSCI.1784-16.2016
Lee, M. T., Mouri, A., Kubota, H., Lee, H. J., Chang, M. H., Wu, C. Y., et al. (2022). Targeting α6GABA(a) receptors as a novel therapy for schizophrenia: a proof-of-concept preclinical study using various animal models. Biomed. Pharmacother. 150:113022. doi: 10.1016/j.biopha.2022.113022
Lemvigh, C. K., Glenthøj, B. Y., and Fagerlund, B. (2022). A nation-wide twin study of social cognition in schizophrenia spectrum disorders. Schizophrenia (Heidelb) 8:12. doi: 10.1038/s41537-022-00223-1
Li, X., Liu, N., Yang, C., Zhang, W., and Lui, S. (2022). Cerebellar gray matter volume changes in patients with schizophrenia: a voxel-based meta-analysis. Front. Psych. 13:1083480. doi: 10.3389/fpsyt.2022.1083480
Li, C., Saliba, N. B., Martin, H., Losurdo, N. A., Kolahdouzan, K., Siddiqui, R., et al. (2023). Purkinje cell dopaminergic inputs to astrocytes regulate cerebellar-dependent behavior. Nat. Commun. 14:1613. doi: 10.1038/s41467-023-37319-w
Li, P., Snyder, G. L., and Vanover, K. E. (2016). Dopamine targeting drugs for the treatment of schizophrenia: past, present and future. Curr. Top. Med. Chem. 16, 3385–3403. doi: 10.2174/1568026616666160608084834
Li, Y., Yang, L., Li, L., Xie, Y., and Fang, P. (2022). The resting-state cerebro-cerebellar function connectivity and associations with verbal working memory performance. Behav. Brain Res. 417:113586. doi: 10.1016/j.bbr.2021.113586
Li, W., Zhou, F. C., Zhang, L., Ng, C. H., Ungvari, G. S., Li, J., et al. (2020). Comparison of cognitive dysfunction between schizophrenia and bipolar disorder patients: a meta-analysis of comparative studies. J. Affect. Disord. 274, 652–661. doi: 10.1016/j.jad.2020.04.051
Lichtenstein, P., Yip, B. H., Björk, C., Pawitan, Y., Cannon, T. D., Sullivan, P. F., et al. (2009). Common genetic determinants of schizophrenia and bipolar disorder in Swedish families: a population-based study. Lancet 373, 234–239. doi: 10.1016/S0140-6736(09)60072-6
Liu, H., Fan, G., Xu, K., and Wang, F. (2011). Changes in cerebellar functional connectivity and anatomical connectivity in schizophrenia: a combined resting-state functional MRI and diffusion tensor imaging study. J. Magn. Reson. Imaging 34, 1430–1438. doi: 10.1002/jmri.22784
Liu, Y., Ouyang, P., Zheng, Y., Mi, L., Zhao, J., Ning, Y., et al. (2021). A selective review of the excitatory-inhibitory imbalance in schizophrenia: underlying biology, genetics, microcircuits, and symptoms. Front. Cell Dev. Biol. 9:664535. doi: 10.3389/fcell.2021.664535
Löhrs, L., and Hasan, A. (2019). Risk factors for the development of schizophrenia. Fortschr. Neurol. Psychiatr. 87, 133–143. doi: 10.1055/a-0836-7839
Lundin, N. B., Kim, D.-J., Tullar, R. L., Moussa-Tooks, A. B., Kent, J. S., Newman, S. D., et al. (2021). Cerebellar activation deficits in schizophrenia during an Eyeblink conditioning task. Schizophrenia Bulletin Open 2:40. doi: 10.1093/schizbullopen/sgab040
Ma, T., Wu, Y., Chen, B., Zhang, W., Jin, L., Shen, C., et al. (2019). D-serine contributes to seizure development via ERK signaling. Front. Neurosci. 13:254. doi: 10.3389/fnins.2019.00254
Maderthaner, L., Pavlidou, A., Lefebvre, S., Nadesalingam, N., Chapellier, V., von Känel, S., et al. (2023). Neural correlates of formal thought disorder dimensions in psychosis. Schizophr. Bull. 49, S104–s114. doi: 10.1093/schbul/sbac120
Maiti, B., Koller, J. M., Snyder, A. Z., Tanenbaum, A. B., Norris, S. A., Campbell, M. C., et al. (2020). Cognitive correlates of cerebellar resting-state functional connectivity in Parkinson disease. Neurology 94, e384–e396. doi: 10.1212/WNL.0000000000008754
Maloku, E., Covelo, I. R., Hanbauer, I., Guidotti, A., Kadriu, B., Hu, Q., et al. (2010). Lower number of cerebellar Purkinje neurons in psychosis is associated with reduced reelin expression. Proc. Natl. Acad. Sci. USA 107, 4407–4411. doi: 10.1073/pnas.0914483107
Mamakou, V., Thanopoulou, A., Gonidakis, F., Tentolouris, N., and Kontaxakis, V. (2018). Schizophrenia and type 2 diabetes mellitus. Psychiatriki 29, 64–73. doi: 10.22365/jpsych.2018.291.64
Man, W., Ding, H., Chai, C., An, X., Liu, F., Qin, W., et al. (2021). Brain age gap as a potential biomarker for schizophrenia: a multi-site structural MRI study. Annu. Int. Conf. IEEE Eng. Med. Biol. Soc. 2021, 4060–4063. doi: 10.1109/EMBC46164.2021.9631085
Mandal, P. K., Gaur, S., Roy, R. G., Samkaria, A., Ingole, R., and Goel, A. (2022). Schizophrenia, bipolar and major depressive disorders: overview of clinical features, neurotransmitter alterations, pharmacological interventions, and impact of oxidative stress in the disease process. ACS Chem. Neurosci. 13, 2784–2802. doi: 10.1021/acschemneuro.2c00420
Mapelli, J., Boiani, G. M., D'Angelo, E., Bigiani, A., and Gandolfi, D. (2022). Long-term synaptic plasticity Tunes the gain of information channels through the cerebellum granular layer. Biomedicines 10:3185. doi: 10.3390/biomedicines10123185
Mapelli, L., Pagani, M., Garrido, J. A., and D'Angelo, E. (2015). Integrated plasticity at inhibitory and excitatory synapses in the cerebellar circuit. Front. Cell. Neurosci. 9:169. doi: 10.3389/fncel.2015.00169
Mapelli, L., Soda, T., D’Angelo, E., and Prestori, F. (2022). The cerebellar involvement in autism Spectrum disorders: from the social brain to mouse models. Int. J. Mol. Sci. 23:3894. doi: 10.3390/ijms23073894
Mapelli, L., Solinas, S., and D'Angelo, E. (2014). Integration and regulation of glomerular inhibition in the cerebellar granular layer circuit. Front. Cell. Neurosci. 8:55. doi: 10.3389/fncel.2014.00055
Marazziti, D. (2002). A further support to the hypothesis of a link between serotonin, autism, and the cerebellum. Biol. Psychiatry 52:143; author reply 143. doi: 10.1016/S0006-3223(02)01406-3
Marder, S. R., and Cannon, T. D. (2019). Schizophrenia. N. Engl. J. Med. 381, 1753–1761. doi: 10.1056/NEJMra1808803
Margarint, E. L. G., Georgescu, I. A., Zahiu, C. D. M., Tirlea, S. A., Şteopoaie, A. R., Zǎgrean, L., et al. (2020). Reduced Interhemispheric Coherence in Cerebellar Kainic Acid-Induced Lateralized Dystonia. Front Neurol. 11:580540. doi: 10.3389/fneur.2020.580540
Marques, T. R., Ashok, A. H., Angelescu, I., Borgan, F., Myers, J., Lingford-Hughes, A., et al. (2021). GABA-A receptor differences in schizophrenia: a positron emission tomography study using [11C]Ro154513. Mol. Psychiatry 26, 2616–2625. doi: 10.1038/s41380-020-0711-y
Marques, T. R., Ashok, A. H., Pillinger, T., Veronese, M., Turkheimer, F. E., Dazzan, P., et al. (2019). Neuroinflammation in schizophrenia: meta-analysis of in vivo microglial imaging studies. Psychol. Med. 49, 2186–2196. doi: 10.1017/S0033291718003057
Matosin, N., Fernandez-Enright, F., Frank, E., Deng, C., Wong, J., Huang, X. F., et al. (2014). Metabotropic glutamate receptor mGluR2/3 and mGluR5 binding in the anterior cingulate cortex in psychotic and nonpsychotic depression, bipolar disorder and schizophrenia: implications for novel mGluR-based therapeutics. J. Psychiatry Neurosci. 39, 407–416. doi: 10.1503/jpn.130242
Matute, C., Melone, M., Vallejo-Illarramendi, A., and Conti, F. (2005). Increased expression of the astrocytic glutamate transporter GLT-1 in the prefrontal cortex of schizophrenics. Glia 49, 451–455. doi: 10.1002/glia.20119
Mavroudis, I. A., Petrides, F., Manani, M., Chatzinikolaou, F., Ciobică, A. S., Pădurariu, M., et al. (2017). Purkinje cells pathology in schizophrenia. A morphometric approach. Romanian J. Morphol. Embryol. 58, 419–424
McCollum, L. A., Walker, C. K., Roche, J. K., and Roberts, R. C. (2015). Elevated excitatory input to the nucleus Accumbens in schizophrenia: a postmortem ultrastructural study. Schizophr. Bull. 41, 1123–1132. doi: 10.1093/schbul/sbv030
McCutcheon, R. A., Abi-Dargham, A., and Howes, O. D. (2019). Schizophrenia, dopamine and the striatum: from biology to symptoms. Trends Neurosci. 42, 205–220. doi: 10.1016/j.tins.2018.12.004
McCutcheon, R. A., Keefe, R. S. E., and McGuire, P. K. (2023). Cognitive impairment in schizophrenia: aetiology, pathophysiology, and treatment. Mol. Psychiatry 28, 1902–1918. doi: 10.1038/s41380-023-01949-9
McCutcheon, R. A., Krystal, J. H., and Howes, O. D. (2020). Dopamine and glutamate in schizophrenia: biology, symptoms and treatment. World Psychiatry 19, 15–33. doi: 10.1002/wps.20693
McCutcheon, R. A., Reis Marques, T., and Howes, O. D. (2020). Schizophrenia—An overview. JAMA Psychiatry 77, 201–210. doi: 10.1001/jamapsychiatry.2019.3360
Melka, M. G., Castellani, C. A., Laufer, B. I., Rajakumar, R. N., O'Reilly, R., and Singh, S. M. (2013). Olanzapine induced DNA methylation changes support the dopamine hypothesis of psychosis. J Mol Psychiatry 1:19. doi: 10.1186/2049-9256-1-19
Merritt, K., McCutcheon, R. A., Aleman, A., Ashley, S., Beck, K., Block, W., et al. (2023). Variability and magnitude of brain glutamate levels in schizophrenia: a meta and mega-analysis. Mol Psychiatry 28, 2039–2048. doi: 10.1038/s41380-023-01991-7
Merritt, K., McGuire, P. K., Egerton, A., Aleman, A., Block, W., Bloemen, O. J. N., et al. (2021). Association of age, antipsychotic medication, and symptom severity in schizophrenia with proton magnetic resonance spectroscopy brain glutamate level: a mega-analysis of individual participant-level data. JAMA Psychiatry 78, 667–681. doi: 10.1001/jamapsychiatry.2021.0380
Merritt, K., Perez-Iglesias, R., Sendt, K. V., Goozee, R., Jauhar, S., Pepper, F., et al. (2019). Remission from antipsychotic treatment in first episode psychosis related to longitudinal changes in brain glutamate. NPJ Schizophr. 5:12. doi: 10.1038/s41537-019-0080-1
Millan, M. J., Agid, Y., Brüne, M., Bullmore, E. T., Carter, C. S., Clayton, N. S., et al. (2012). Cognitive dysfunction in psychiatric disorders: characteristics, causes and the quest for improved therapy. Nat. Rev. Drug Discov. 11, 141–168. doi: 10.1038/nrd3628
Miquel, M., Nicola, S. M., Gil-Miravet, I., Guarque-Chabrera, J., and Sanchez-Hernandez, A. (2019). A working hypothesis for the role of the cerebellum in impulsivity and compulsivity. Front. Behav. Neurosci. 13:99. doi: 10.3389/fnbeh.2019.00099
Misiak, B., Stańczykiewicz, B., Wiśniewski, M., Bartoli, F., Carra, G., Cavaleri, D., et al. (2021). Thyroid hormones in persons with schizophrenia: a systematic review and meta-analysis. Prog. Neuro-Psychopharmacol. Biol. Psychiatry 111:110402. doi: 10.1016/j.pnpbp.2021.110402
Mittleman, G., Goldowitz, D., Heck, D. H., and Blaha, C. D. (2008). Cerebellar modulation of frontal cortex dopamine efflux in mice: relevance to autism and schizophrenia. Synapse 62, 544–550. doi: 10.1002/syn.20525
Moberget, T., Alnæs, D., Kaufmann, T., Doan, N. T., Córdova-Palomera, A., Norbom, L. B., et al. (2019). Cerebellar Gray matter volume is associated with cognitive function and psychopathology in adolescence. Biol. Psychiatry 86, 65–75. doi: 10.1016/j.biopsych.2019.01.019
Moberget, T., Doan, N. T., Alnæs, D., Kaufmann, T., Córdova-Palomera, A., Lagerberg, T. V., et al. (2018). Cerebellar volume and cerebellocerebral structural covariance in schizophrenia: a multisite mega-analysis of 983 patients and 1349 healthy controls. Mol. Psychiatry 23, 1512–1520. doi: 10.1038/mp.2017.106
Moghaddam, B., and Javitt, D. (2012). From revolution to evolution: The glutamate hypothesis of schizophrenia and its implication for treatment. Neuropsychopharmacology 37, 4–15. doi: 10.1038/npp.2011.181
Mollon, J., David, A. S., Zammit, S., Lewis, G., and Reichenberg, A. (2018). Course of cognitive development from infancy to early adulthood in the psychosis Spectrum. JAMA Psychiatry 75, 270–279. doi: 10.1001/jamapsychiatry.2017.4327
Monteverdi, A., Palesi, F., Costa, A., Vitali, P., Pichiecchio, A., Cotta Ramusino, M., et al. (2022). Subject-specific features of excitation/inhibition profiles in neurodegenerative diseases. Front. Aging Neurosci. 14:868342. doi: 10.3389/fnagi.2022.868342
Monteverdi, A., Palesi, F., Schirner, M., Argentino, F., Merante, M., Redolfi, A., et al. (2023). Virtual brain simulations reveal network-specific parameters in neurodegenerative dementias. Front. Aging Neurosci. 15:2023.2003.2010.532087. doi: 10.3389/fnagi.2023.1204134
Morellini, N., Grehl, S., Tang, A., Rodger, J., Mariani, J., Lohof, A. M., et al. (2015). What does low-intensity rTMS do to the cerebellum? Cerebellum 14, 23–26. doi: 10.1007/s12311-014-0617-9
Mould, A. W., Hall, N. A., Milosevic, I., and Tunbridge, E. M. (2021). Targeting synaptic plasticity in schizophrenia: insights from genomic studies. Trends Mol. Med. 27, 1022–1032. doi: 10.1016/j.molmed.2021.07.014
Moussa-Tooks, A. B., Rogers, B. P., Huang, A. S., Sheffield, J. M., Heckers, S., and Woodward, N. D. (2022). Cerebellar structure and cognitive ability in psychosis. Biol. Psychiatry 92, 385–395. doi: 10.1016/j.biopsych.2022.03.013
Mudge, J., Miller, N. A., Khrebtukova, I., Lindquist, I. E., May, G. D., Huntley, J. J., et al. (2008). Genomic convergence analysis of schizophrenia: mRNA sequencing reveals altered synaptic vesicular transport in post-mortem cerebellum. PLoS One 3:e3625. doi: 10.1371/journal.pone.0003625
Mullasseril, P., Hansen, K. B., Vance, K. M., Ogden, K. K., Yuan, H., Kurtkaya, N. L., et al. (2010). A subunit-selective potentiator of NR2C- and NR2D-containing NMDA receptors. Nat. Commun. 1:90. doi: 10.1038/ncomms1085
Müller, N. (2018). Inflammation in schizophrenia: Pathogenetic aspects and therapeutic considerations. Schizophr. Bull. 44, 973–982. doi: 10.1093/schbul/sby024
Nakahara, T., Tsugawa, S., Noda, Y., Ueno, F., Honda, S., Kinjo, M., et al. (2022). Glutamatergic and GABAergic metabolite levels in schizophrenia-spectrum disorders: a meta-analysis of (1)H-magnetic resonance spectroscopy studies. Mol. Psychiatry 27, 744–757. doi: 10.1038/s41380-021-01297-6
Nakazawa, K., and Sapkota, K. (2020). The origin of NMDA receptor hypofunction in schizophrenia. Pharmacol. Ther. 205:107426. doi: 10.1016/j.pharmthera.2019.107426
Nanou, E., and Catterall, W. A. (2018). Calcium channels, synaptic plasticity, and neuropsychiatric disease. Neuron 98, 466–481. doi: 10.1016/j.neuron.2018.03.017
Nascimento, J. M., Saia-Cereda, V. M., Zuccoli, G. S., Reis-de-Oliveira, G., Carregari, V. C., Smith, B. J., et al. (2022). Proteomic signatures of schizophrenia-sourced iPSC-derived neural cells and brain organoids are similar to patients' postmortem brains. Cell Biosci. 12:189. doi: 10.1186/s13578-022-00928-x
Newman, E., Gupta, K., Climer, J., Monaghan, C., and Hasselmo, M. (2012). Cholinergic modulation of cognitive processing: insights drawn from computational models. Front. Behav. Neurosci. 6:24. doi: 10.3389/fnbeh.2012.00024
Nguyen, T. M., Thomas, L. A., Rhoades, J. L., Ricchi, I., Yuan, X. C., Sheridan, A., et al. (2023). Structured cerebellar connectivity supports resilient pattern separation. Nature 613, 543–549. doi: 10.1038/s41586-022-05471-w
Nielsen, R. E., Banner, J., and Jensen, S. E. (2021). Cardiovascular disease in patients with severe mental illness. Nat. Rev. Cardiol. 18, 136–145. doi: 10.1038/s41569-020-00463-7
Nieus, T. R., Mapelli, L., and D'Angelo, E. (2014). Regulation of output spike patterns by phasic inhibition in cerebellar granule cells. Front. Cell. Neurosci. 8:246. doi: 10.3389/fncel.2014.00246
Nuechterlein, K. H., Barch, D. M., Gold, J. M., Goldberg, T. E., Green, M. F., and Heaton, R. K. (2004). Identification of separable cognitive factors in schizophrenia. Schizophr. Res. 72, 29–39. doi: 10.1016/j.schres.2004.09.007
Obi-Nagata, K., Temma, Y., and Hayashi-Takagi, A. (2019). Synaptic functions and their disruption in schizophrenia: from clinical evidence to synaptic optogenetics in an animal model. Proc. Jpn. Acad. Ser. B Phys. Biol. Sci. 95, 179–197. doi: 10.2183/pjab.95.014
O'Donnell, P., Dong, C., Murthy, V., Asgharnejad, M., Du, X., Summerfelt, A., et al. (2023). The D-amino acid oxidase inhibitor luvadaxistat improves mismatch negativity in patients with schizophrenia in a randomized trial. Neuropsychopharmacology 48, 1052–1059. doi: 10.1038/s41386-023-01560-0
Okkels, N., Horsager, J., Labrador-Espinosa, M. A., Hansen, F. O., Andersen, K. B., Just, M. K., et al. (2023). Distribution of cholinergic nerve terminals in the aged human brain measured with [18F]FEOBV PET and its correlation with histological data. NeuroImage 269:119908. doi: 10.1016/j.neuroimage.2023.119908
Okugawa, G., Nobuhara, K., Minami, T., Tamagaki, C., Takase, K., Sugimoto, T., et al. (2004). Subtle disruption of the middle cerebellar peduncles in patients with schizophrenia. Neuropsychobiology 50, 119–123. doi: 10.1159/000079101
Omeiza, N. A., Bakre, A., Ben-Azu, B., Sowunmi, A. A., Abdulrahim, H. A., Chimezie, J., et al. (2023). Mechanisms underpinning Carpolobia lutea G. Don ethanol extract's neurorestorative and antipsychotic-like activities in an NMDA receptor antagonist model of schizophrenia. J. Ethnopharmacol. 301:115767. doi: 10.1016/j.jep.2022.115767
Onaolapo, A. Y., Ayeni, O. J., Ogundeji, M. O., Ajao, A., Onaolapo, O. J., and Owolabi, A. R. (2019). Subchronic ketamine alters behaviour, metabolic indices and brain morphology in adolescent rats: involvement of oxidative stress, glutamate toxicity and caspase-3-mediated apoptosis. J. Chem. Neuroanat. 96, 22–33. doi: 10.1016/j.jchemneu.2018.12.002
Onwordi, E. C., Whitehurst, T., Mansur, A., Statton, B., Berry, A., Quinlan, M., et al. (2021). The relationship between synaptic density marker SV2A, glutamate and N-acetyl aspartate levels in healthy volunteers and schizophrenia: a multimodal PET and magnetic resonance spectroscopy brain imaging study. Transl. Psychiatry 11:393. doi: 10.1038/s41398-021-01515-3
Oostland, M., Buijink, M. R., Teunisse, G. M., von Oerthel, L., Smidt, M. P., and van Hooft, J. A. (2014). Distinct temporal expression of 5-HT(1A) and 5-HT(2A) receptors on cerebellar granule cells in mice. Cerebellum 13, 491–500. doi: 10.1007/s12311-014-0565-4
Oostland, M., and van Hooft, J. A. (2013). The role of serotonin in cerebellar development. Neuroscience 248, 201–212. doi: 10.1016/j.neuroscience.2013.05.029
Orsolini, L., Pompili, S., and Volpe, U. (2022). Schizophrenia: a narrative review of Etiopathogenetic, diagnostic and treatment aspects. J. Clin. Med. 11:40. doi: 10.3390/jcm11175040
Orzelska-Górka, J., Mikulska, J., Wiszniewska, A., and Biała, G. (2022). New atypical antipsychotics in the treatment of schizophrenia and depression. Int. J. Mol. Sci. 23:624. doi: 10.3390/ijms231810624
Osimo, E. F., Beck, K., Reis Marques, T., and Howes, O. D. (2019). Synaptic loss in schizophrenia: a meta-analysis and systematic review of synaptic protein and mRNA measures. Mol. Psychiatry 24, 549–561. doi: 10.1038/s41380-018-0041-5
Owen, M. J., Legge, S. E., Rees, E., Walters, J. T. R., and O'Donovan, M. C. (2023). Genomic findings in schizophrenia and their implications. Mol. Psychiatry 28, 3638–3647. doi: 10.1038/s41380-023-02293-8
Owen, M. J., Sawa, A., and Mortensen, P. B. (2016). Schizophrenia. Lancet 388, 86–97. doi: 10.1016/S0140-6736(15)01121-6
Ozaki, M., Sasner, M., Yano, R., Lu, H. S., and Buonanno, A. (1997). Neuregulin-β induces expression of an NMDA-receptor subunit. Nature 390, 691–694. doi: 10.1038/37795
Palesi, F., De Rinaldis, A., Castellazzi, G., Calamante, F., Muhlert, N., Chard, D., et al. (2017). Contralateral cortico-ponto-cerebellar pathways reconstruction in humans in vivo: implications for reciprocal cerebro-cerebellar structural connectivity in motor and non-motor areas. Sci. Rep. 7:12841. doi: 10.1038/s41598-017-13079-8
Palesi, F., De Rinaldis, A., Vitali, P., Castellazzi, G., Casiraghi, L., Germani, G., et al. (2018). Specific patterns of white matter alterations help distinguishing Alzheimer's and vascular dementia. Front. Neurosci. 12:274. doi: 10.3389/fnins.2018.00274
Palesi, F., Tournier, J. D., Calamante, F., Muhlert, N., Castellazzi, G., Chard, D., et al. (2015). Contralateral cerebello-thalamo-cortical pathways with prominent involvement of associative areas in humans in vivo. Brain Struct. Funct. 220, 3369–3384. doi: 10.1007/s00429-014-0861-2
Panagopoulos, N. T., and Matsokis, N. A. (1994). Pharmacologic characterization of [3H]dopamine and [3H]spiperone binding in mouse cerebellum. Gen. Pharmacol. 25, 131–137. doi: 10.1016/0306-3623(94)90022-1
Park, S. H., Kim, T., Ha, M., Moon, S.-Y., Lho, S. K., Kim, M., et al. (2021). Intrinsic cerebellar functional connectivity of social cognition and theory of mind in first-episode psychosis patients. NPJ Schizophr. 7:59. doi: 10.1038/s41537-021-00193-w
Parker, K. L., Kim, Y. C., Kelley, R. M., Nessler, A. J., Chen, K. H., Muller-Ewald, V. A., et al. (2017). Delta-frequency stimulation of cerebellar projections can compensate for schizophrenia-related medial frontal dysfunction. Mol. Psychiatry 22, 647–655. doi: 10.1038/mp.2017.50
Parker, K. L., Narayanan, N. S., and Andreasen, N. C. (2014). The therapeutic potential of the cerebellum in schizophrenia. Front. Syst. Neurosci. 8:163. doi: 10.3389/fnsys.2014.00163
Patel, K. R., Cherian, J., Gohil, K., and Atkinson, D. (2014). Schizophrenia: overview and treatment options. P t. 39, 638–645
Pekala, M., Doliwa, M., and Kalita, K. (2021). Impact of maternal immune activation on dendritic spine development. Dev. Neurobiol. 81, 524–545. doi: 10.1002/dneu.22804
Pereda, D., Al-Osta, I., Okorocha, A. E., Easton, A., and Hartell, N. A. (2019). Changes in presynaptic calcium signalling accompany age-related deficits in hippocampal LTP and cognitive impairment. Aging Cell 18:e13008. doi: 10.1111/acel.13008
Perez-Garcia, C. G. (2015). ErbB4 in laminated brain structures: a neurodevelopmental approach to schizophrenia. Front. Cell. Neurosci. 9:472. doi: 10.3389/fncel.2015.00472
Perzel Mandell, K. A., Eagles, N. J., Wilton, R., Price, A. J., Semick, S. A., Collado-Torres, L., et al. (2021). Genome-wide sequencing-based identification of methylation quantitative trait loci and their role in schizophrenia risk. Nat. Commun. 12:5251. doi: 10.1038/s41467-021-25517-3
Peters, B. D., Szeszko, P. R., Radua, J., Ikuta, T., Gruner, P., DeRosse, P., et al. (2012). White matter development in adolescence: diffusion tensor imaging and meta-analytic results. Schizophr. Bull. 38, 1308–1317. doi: 10.1093/schbul/sbs054
Pickford, J., Iosif, C. I., Bashir, Z. I., and Apps, R. (2023). Inhibiting cholinergic signalling in the cerebellar interpositus nucleus impairs motor behaviour. Eur. J. Neurosci. 1–17. doi: 10.1111/ejn.16066
Pilloni, G., Vogel-Eyny, A., Lustberg, M., Best, P., Malik, M., Walton-Masters, L., et al. (2022). Tolerability and feasibility of at-home remotely supervised transcranial direct current stimulation (RS-tDCS): single-center evidence from 6,779 sessions. Brain Stimul. 15, 707–716. doi: 10.1016/j.brs.2022.04.014
Pinacho, R., Villalmanzo, N., Roca, M., Iniesta, R., Monje, A., Haro, J. M., et al. (2013). Analysis of Sp transcription factors in the postmortem brain of chronic schizophrenia: a pilot study of relationship to negative symptoms. J. Psychiatr. Res. 47, 926–934. doi: 10.1016/j.jpsychires.2013.03.004
Piras, F., Piras, F., Banaj, N., Ciullo, V., Vecchio, D., Edden, R. A. E., et al. (2019). Cerebellar GABAergic correlates of cognition-mediated verbal fluency in physiology and schizophrenia. Acta Psychiatr. Scand. 139, 582–594. doi: 10.1111/acps.13027
Pisano, T. J., Dhanerawala, Z. M., Kislin, M., Bakshinskaya, D., Engel, E. A., Hansen, E. J., et al. (2021). Homologous organization of cerebellar pathways to sensory, motor, and associative forebrain. Cell Rep. 36:109721. doi: 10.1016/j.celrep.2021.109721
Popa, L. S., Hewitt, A. L., and Ebner, T. J. (2014). The cerebellum for jocks and nerds alike. Front. Syst. Neurosci. 8:113. doi: 10.3389/fnsys.2014.00113
Pope, P. A., and Miall, R. C. (2014). Restoring cognitive functions using non-invasive brain stimulation techniques in patients with cerebellar disorders. Front. Psych. 5:33. doi: 10.3389/fpsyt.2014.00033
Popova, N. K., and Naumenko, V. S. (2013). 5-HT1A receptor as a key player in the brain 5-HT system. Rev. Neurosci. 24, 191–204. doi: 10.1515/revneuro-2012-0082
Pouget, J. G., Han, B., Wu, Y., Mignot, E., Ollila, H. M., Barker, J., et al. (2019). Cross-disorder analysis of schizophrenia and 19 immune-mediated diseases identifies shared genetic risk. Hum. Mol. Genet. 28, 3498–3513. doi: 10.1093/hmg/ddz145
Prestori, F., Bonardi, C., Mapelli, L., Lombardo, P., Goselink, R., De Stefano, M. E., et al. (2013). Gating of long-term potentiation by nicotinic acetylcholine receptors at the cerebellum input stage. PLoS One 8:e64828. doi: 10.1371/journal.pone.0064828
Queirós, T. P., Coelho, F. S., Linhares, L. A., and Correia, D. T. (2019). Schizophrenia: what non-psychiatrist physicians need to know. Acta Medica Port. 32, 70–77. doi: 10.20344/amp.10768
Rabacchi, S., Bailly, Y., Delhaye-Bouchaud, N., and Mariani, J. (1992). Involvement of the N-methyl D-aspartate (NMDA) receptor in synapse elimination during cerebellar development. Science 256, 1823–1825. doi: 10.1126/science.1352066
Radhakrishnan, R., Skosnik, P. D., Ranganathan, M., Naganawa, M., Toyonaga, T., Finnema, S., et al. (2021). In vivo evidence of lower synaptic vesicle density in schizophrenia. Mol. Psychiatry 26, 7690–7698. doi: 10.1038/s41380-021-01184-0
Rao, N., Northoff, G., Tagore, A., Rusjan, P., Kenk, M., Wilson, A., et al. (2018). Impaired prefrontal cortical dopamine release in schizophrenia during a cognitive task: a [11C]FLB 457 positron emission tomography study. Schizophr. Bull. 45, 670–679. doi: 10.1093/schbul/sby076
Repovs, G., Csernansky, J. G., and Barch, D. M. (2011). Brain network connectivity in individuals with schizophrenia and their siblings. Biol. Psychiatry 69, 967–973. doi: 10.1016/j.biopsych.2010.11.009
Ribeiro, S., and Sherrard, R. M. (2023). Cerebellum and neurodevelopmental disorders: RORα is a unifying force. Front. Cell. Neurosci. 17:1108339. doi: 10.3389/fncel.2023.1108339
Ripke, S., Walters, J. T., and O’Donovan, M. C. (2020). Mapping genomic loci prioritises genes and implicates synaptic biology in schizophrenia. medRxiv :2020.2009.2012.20192922. doi: 10.1038/s41586-022-04434-5
Rivollier, F., Lotersztajn, L., Chaumette, B., Krebs, M. O., and Kebir, O. (2014). Epigenetics of schizophrenia: a review. Encéphale 40, 380–386. doi: 10.1016/j.encep.2014.06.005
Robbins, T. W. (2019). Pharmacological treatment of cognitive deficits in nondementing mental health disorders. Dialogues Clin. Neurosci. 21, 301–308. doi: 10.31887/DCNS.2019.21.3/trobbins
Rogers, T. D., Dickson, P. E., Heck, D. H., Goldowitz, D., Mittleman, G., and Blaha, C. D. (2011). Connecting the dots of the cerebro-cerebellar role in cognitive function: neuronal pathways for cerebellar modulation of dopamine release in the prefrontal cortex. Synapse 65, 1204–1212. doi: 10.1002/syn.20960
Rogers, T. D., Dickson, P. E., McKimm, E., Heck, D. H., Goldowitz, D., Blaha, C. D., et al. (2013). Reorganization of circuits underlying cerebellar modulation of prefrontal cortical dopamine in mouse models of autism spectrum disorder. Cerebellum 12, 547–556. doi: 10.1007/s12311-013-0462-2
Rolls, E. T., Cheng, W., Gilson, M., Gong, W., Deco, G., Lo, C. Z., et al. (2020). Beyond the disconnectivity hypothesis of schizophrenia. Cereb. Cortex 30, 1213–1233. doi: 10.1093/cercor/bhz161
Rong, B., Huang, H., Gao, G., Sun, L., Zhou, Y., Xiao, L., et al. (2023). Widespread intra- and inter-network Dysconnectivity among Large-scale resting state networks in schizophrenia. J. Clin. Med. 12:3176. doi: 10.3390/jcm12093176
Saitow, F., Hirono, M., and Suzuki, H. (2013). “Serotonin and synaptic transmission in the cerebellum” in Handbook of the cerebellum and cerebellar disorders. eds. M. Manto, J. D. Schmahmann, F. Rossi, D. L. Gruol, and N. Koibuchi (Dordrecht: Springer Netherlands), 915–926.
Santarriaga, S., Gerlovin, K., Layadi, Y., and Karmacharya, R. (2023). Human stem cell-based models to study synaptic dysfunction and cognition in schizophrenia: A narrative review. Schizophr Res. doi: 10.1016/j.schres.2023.02.029
Savage, J. E., Jansen, P. R., Stringer, S., Watanabe, K., Bryois, J., de Leeuw, C. A., et al. (2018). Genome-wide association meta-analysis in 269,867 individuals identifies new genetic and functional links to intelligence. Nat. Genet. 50, 912–919. doi: 10.1038/s41588-018-0152-6
Schmahmann, J. D. (2016). “The Cerebrocerebellar system” in Essentials of cerebellum and cerebellar disorders: A primer for graduate students. eds. D. L. Gruol, N. Koibuchi, and M. Manto, et al. (Cham: Springer International Publishing), 101–115.
Schmahmann, J. D. (2019). The cerebellum and cognition. Neurosci. Lett. 688, 62–75. doi: 10.1016/j.neulet.2018.07.005
Schmahmann, J. D., and Sherman, J. C. (1998). The cerebellar cognitive affective syndrome. Brain 121, 561–579. doi: 10.1093/brain/121.4.561
Schmitt, A., Falkai, P., and Papiol, S. (2023). Neurodevelopmental disturbances in schizophrenia: evidence from genetic and environmental factors. J. Neural Transm. 130, 195–205. doi: 10.1007/s00702-022-02567-5
Schmitt, A., Koschel, J., Zink, M., Bauer, M., Sommer, C., Frank, J., et al. (2010). Gene expression of NMDA receptor subunits in the cerebellum of elderly patients with schizophrenia. Eur. Arch. Psychiatry Clin. Neurosci. 260, 101–111. doi: 10.1007/s00406-009-0017-1
Sefik, E., Boamah, M., Addington, J., Bearden, C. E., Cadenhead, K. S., Cornblatt, B. A., et al. (2022). Sex- and age-specific deviations in cerebellar structure and their link with symptom dimensions and clinical outcome in individuals at clinical high risk for psychosis. Schizophr. Bull. 49, 350–363. doi: 10.1093/schbul/sbac169
Seitz-Holland, J., Nägele, F. L., Kubicki, M., Pasternak, O., Cho, K. I. K., Hough, M., et al. (2023). Shared and distinct white matter abnormalities in adolescent-onset schizophrenia and adolescent-onset psychotic bipolar disorder. Psychol. Med. 53, 4707–4719. doi: 10.1017/S003329172200160X
Selten, J. P., and Ormel, J. (2023). Low status, humiliation, dopamine and risk of schizophrenia. Psychol. Med. 53, 1–5. doi: 10.1017/S0033291722003816
Sepp, M., Leiss, K., Murat, F., Okonechnikov, K., Joshi, P., Leushkin, E., et al. (2024). Cellular development and evolution of the mammalian cerebellum. Nature 625, 788–796. doi: 10.1038/s41586-023-06884-x
Sharma, A., Kumar, A., Singh, S., Bhatia, T., Beniwal, R. P., Khushu, S., et al. (2018). Altered resting state functional connectivity in early course schizophrenia. Psychiatry Res. Neuroimaging 271, 17–23. doi: 10.1016/j.pscychresns.2017.11.013
Sheffield, J. M., Karcher, N. R., and Barch, D. M. (2018). Cognitive deficits in psychotic disorders: a lifespan perspective. Neuropsychol. Rev. 28, 509–533. doi: 10.1007/s11065-018-9388-2
Sieghart, W., Chiou, L.-C., Ernst, M., Fabjan, J., Savić, M. M., and Lee, M. T. (2022). α6-containing GABAa receptors: functional roles and therapeutic potentials. Pharmacol. Rev. 74, 238–270. doi: 10.1124/pharmrev.121.000293
Simpson, E. H., and Kellendonk, C. (2017). Insights about striatal circuit function and schizophrenia from a mouse model of dopamine D(2) receptor upregulation. Biol. Psychiatry 81, 21–30. doi: 10.1016/j.biopsych.2016.07.004
Simpson, E. H., Kellendonk, C., and Kandel, E. (2010). A possible role for the striatum in the pathogenesis of the cognitive symptoms of schizophrenia. Neuron 65, 585–596. doi: 10.1016/j.neuron.2010.02.014
Singer, W., and Gray, C. M. (1995). Visual feature integration and the temporal correlation hypothesis. Annu. Rev. Neurosci. 18, 555–586. doi: 10.1146/annurev.ne.18.030195.003011
Singh, A., Trapp, N. T., De Corte, B., Cao, S., Kingyon, J., Boes, A. D., et al. (2019). Cerebellar Theta frequency transcranial pulsed stimulation increases frontal Theta oscillations in patients with schizophrenia. Cerebellum 18, 489–499. doi: 10.1007/s12311-019-01013-9
Slater, P., Doyle, C. A., and Deakin, J. F. (1998). Abnormal persistence of cerebellar serotonin-1A receptors in schizophrenia suggests failure to regress in neonates. J. Neural Transm. (Vienna) 105, 305–315. doi: 10.1007/s007020050060
Slifstein, M., van de Giessen, E., Van Snellenberg, J., Thompson, J. L., Narendran, R., Gil, R., et al. (2015). Deficits in prefrontal cortical and Extrastriatal dopamine release in schizophrenia: a positron emission tomographic functional magnetic resonance imaging study. JAMA Psychiatry 72, 316–324. doi: 10.1001/jamapsychiatry.2014.2414
Sone, M., Koshiyama, D., Zhu, Y., Maikusa, N., Okada, N., Abe, O., et al. (2022). Structural brain abnormalities in schizophrenia patients with a history and presence of auditory verbal hallucination. Transl. Psychiatry 12:511. doi: 10.1038/s41398-022-02282-5
Stephan, K. E., Baldeweg, T., and Friston, K. J. (2006). Synaptic plasticity and Dysconnection in schizophrenia. Biol. Psychiatry 59, 929–939. doi: 10.1016/j.biopsych.2005.10.005
Stępnicki, P., Kondej, M., and Kaczor, A. A. (2018). Current concepts and treatments of schizophrenia. Molecules 23:2087. doi: 10.3390/molecules23082087
Stone, W. S., and Seidman, L. J. (2016). Neuropsychological and structural neuroimaging Endophenotypes in schizophrenia. Developmental. Psychopathology, 1–35. doi: 10.1002/9781119125556.devpsy224
Suetani, S., Honarparvar, F., Siskind, D., Hindley, G., Veronese, N., Vancampfort, D., et al. (2021). Increased rates of respiratory disease in schizophrenia: a systematic review and meta-analysis including 619,214 individuals with schizophrenia and 52,159,551 controls. Schizophr. Res. 237, 131–140. doi: 10.1016/j.schres.2021.08.022
Sullivan, P. F., Kendler, K. S., and Neale, M. C. (2003). Schizophrenia as a complex trait: evidence from a meta-analysis of twin studies. Arch. Gen. Psychiatry 60, 1187–1192. doi: 10.1001/archpsyc.60.12.1187
Swanton, T. (2020). The dopamine, glutamate, and GABA hypotheses of schizophrenia: glutamate may be the key. ANU Undergraduate Res. J. 10, 88–96,
Tauscher, J., Kapur, S., Verhoeff, N. P., Hussey, D. F., Daskalakis, Z. J., Tauscher-Wisniewski, S., et al. (2002). Brain serotonin 5-HT(1A) receptor binding in schizophrenia measured by positron emission tomography and [11C]WAY-100635. Arch. Gen. Psychiatry 59, 514–520. doi: 10.1001/archpsyc.59.6.514
Thompson, C. L., Drewery, D. L., Atkins, H. D., Stephenson, F. A., and Chazot, P. L. (2000). Immunohistochemical localization of N-methyl-d-aspartate receptor NR1, NR2A, NR2B and NR2C/D subunits in the adult mammalian cerebellum. Neurosci. Lett. 283, 85–88. doi: 10.1016/S0304-3940(00)00930-7
Toda, M., and Abi-Dargham, A. (2007). Dopamine hypothesis of schizophrenia: making sense of it all. Curr. Psychiatry Rep. 9, 329–336. doi: 10.1007/s11920-007-0041-7
Toulopoulou, T., Picchioni, M., Rijsdijk, F., Hua-Hall, M., Ettinger, U., Sham, P., et al. (2007). Substantial genetic overlap between neurocognition and schizophrenia: genetic modeling in twin samples. Arch. Gen. Psychiatry 64, 1348–1355. doi: 10.1001/archpsyc.64.12.1348
Treadway, M. T., and Zald, D. H. (2011). Reconsidering anhedonia in depression: lessons from translational neuroscience. Neurosci. Biobehav. Rev. 35, 537–555. doi: 10.1016/j.neubiorev.2010.06.006
Tripathi, A., Kar, S. K., and Shukla, R. (2018). Cognitive deficits in schizophrenia: understanding the biological correlates and remediation strategies. Clin. Psychopharmacol. Neurosci. 16, 7–17. doi: 10.9758/cpn.2018.16.1.7
Turner, J. R., Ortinski, P. I., Sherrard, R. M., and Kellar, K. J. (2011). Cerebellar nicotinic cholinergic receptors are intrinsic to the cerebellum: implications for diverse functional roles. Cerebellum 10, 748–757. doi: 10.1007/s12311-011-0285-y
Uhlhaas, P. J., and Singer, W. (2010). Abnormal neural oscillations and synchrony in schizophrenia. Nat. Rev. Neurosci. 11, 100–113. doi: 10.1038/nrn2774
Uyy, E., Suica, V. I., Boteanu, R. M., Safciuc, F., Cerveanu-Hogas, A., Ivan, L., et al. (2020). Diabetic nephropathy associates with deregulation of enzymes involved in kidney Sulphur metabolism. J. Cell. Mol. Med. 24, 12131–12140. doi: 10.1111/jcmm.15855
Valdés-Tovar, M., Rodríguez-Ramírez, A. M., Rodríguez-Cárdenas, L., Sotelo-Ramírez, C. E., Camarena, B., Sanabrais-Jiménez, M. A., et al. (2022). Insights into myelin dysfunction in schizophrenia and bipolar disorder. World J. Psychiatry 12, 264–285. doi: 10.5498/wjp.v12.i2.264
van den Heuvel, M. P., and Fornito, A. (2014). Brain networks in schizophrenia. Neuropsychol. Rev. 24, 32–48. doi: 10.1007/s11065-014-9248-7
van der Heijden, M. E., Rey Hipolito, A. G., Kim, L. H., Kizek, D. J., Perez, R. M., Lin, T., et al. (2023). Glutamatergic cerebellar neurons differentially contribute to the acquisition of motor and social behaviors. Nat. Commun. 14:2771. doi: 10.1038/s41467-023-38475-9
van Dun, K., Bodranghien, F. C., Mariën, P., and Manto, M. U. (2016). tDCS of the cerebellum: where Do we stand in 2016? Technical issues and critical review of the literature. Front. Hum. Neurosci. 10:199. doi: 10.3389/fnhum.2016.00199
Veleanu, M., Urrieta-Chávez, B., Sigoillot, S. M., Paul, M. A., Usardi, A., Iyer, K., et al. (2022). Modified climbing fiber/Purkinje cell synaptic connectivity in the cerebellum of the neonatal phencyclidine model of schizophrenia. Proc. Natl. Acad. Sci. USA 119:e2122544119. doi: 10.1073/pnas.2122544119
Velligan, D. I., and Rao, S. (2023). The epidemiology and global burden of schizophrenia. J. Clin. Psychiatry 84:5. doi: 10.4088/JCP.MS21078COM5
Verma, A., and Moghaddam, B. (1996). NMDA receptor antagonists impair prefrontal cortex function as assessed via spatial delayed alternation performance in rats: modulation by dopamine. J. Neurosci. 16, 373–379. doi: 10.1523/JNEUROSCI.16-01-00373.1996
Versteeg, D. H. G., Van der Gugten, J., De Jong, W., and Palkovits, M. S. (1976). Regional concentrations of noradrenaline and dopamine in rat brain. Brain Res. 113, 563–574. doi: 10.1016/0006-8993(76)90057-3
Wang, S. S., Kloth, A. D., and Badura, A. (2014). The cerebellum, sensitive periods, and autism. Neuron 83, 518–532. doi: 10.1016/j.neuron.2014.07.016
Wang, Y., Zhong, S., Chen, G., Liu, T., Zhao, L., Sun, Y., et al. (2018). Altered cerebellar functional connectivity in remitted bipolar disorder: a resting-state functional magnetic resonance imaging study. Australian New Zealand J. Psychiatry 52, 962–971. doi: 10.1177/0004867417745996
Washburn, S., Oñate, M., Yoshida, J., Vera, J., Bhuvanasundaram, R., Khatami, L., et al. (2024). The cerebellum directly modulates the substantia nigra dopaminergic activity. Nat. Neurosci. 27, 497–513. doi: 10.1038/s41593-023-01560-9
Weinberger, D. R. (1987). Implications of normal brain development for the pathogenesis of schizophrenia. Arch. Gen. Psychiatry 44, 660–669. doi: 10.1001/archpsyc.1987.01800190080012
Wong, C. H. Y., Liu, J., Lee, T. M. C., Tao, J., Wong, A. W. K., Chau, B. K. H., et al. (2021). Fronto-cerebellar connectivity mediating cognitive processing speed. NeuroImage 226:117556. doi: 10.1016/j.neuroimage.2020.117556
Wright, I. C., Rabe-Hesketh, S., Woodruff, P. W., David, A. S., Murray, R. M., and Bullmore, E. T. (2000). Meta-analysis of regional brain volumes in schizophrenia. Am. J. Psychiatry 157, 16–25. doi: 10.1176/ajp.157.1.16
Wu, X., Fu, Y., Knott, G., Lu, J., Cristo, G. D., and Huang, Z. J. (2012). GABA signaling promotes synapse elimination and axon pruning in developing cortical inhibitory interneurons. J. Neurosci. 32, 331–343. doi: 10.1523/JNEUROSCI.3189-11.2012
Wu, X. L., Yan, Q. J., and Zhu, F. (2022). Abnormal synaptic plasticity and impaired cognition in schizophrenia. World J. Psychiatry 12, 541–557. doi: 10.5498/wjp.v12.i4.541
Xie, Y. J., Xi, Y. B., Cui, L.-B., Guan, M. Z., Li, C., Wang, Z. H., et al. (2021). Functional connectivity of cerebellar dentate nucleus and cognitive impairments in patients with drug-naive and first-episode schizophrenia. Psychiatry Res. 300:113937. doi: 10.1016/j.psychres.2021.113937
Xu, Y.-H., Zhang, B.-B., Su, W.-H., Wu, M.-C., Bing, Y.-H., Cui, S.-B., et al. (2019). Nicotine modulates the facial stimulation-evoked responses in cerebellar granule cell layer in vivo in mice. Eur. J. Pharmacol. 843, 126–133. doi: 10.1016/j.ejphar.2018.11.022
Xue, K. K., Chen, J. L., Wei, Y. R., Chen, Y., Han, S. S., Wang, C. H., et al. (2023). Abnormal changes of static and dynamic functional connectivity of dopaminergic midbrain in patients with first-episode schizophrenia and their correlations with clinical symptoms. Zhonghua Yi Xue Za Zhi 103, 1623–1630. doi: 10.3760/cma.j.cn112137-20221118-02428
Yamamoto, M., Bagarinao, E., Shimamoto, M., Iidaka, T., and Ozaki, N. (2022). Involvement of cerebellar and subcortical connector hubs in schizophrenia. NeuroImage 35:103140. doi: 10.1016/j.nicl.2022.103140
Yeganeh-Doost, P., Gruber, O., Falkai, P., and Schmitt, A. (2011). The role of the cerebellum in schizophrenia: from cognition to molecular pathways. Clinics 66 Suppl 1, 71–77. doi: 10.1590/S1807-59322011001300009
Yeruva, R. R., Shang, Y., Schoenbachler, B., Nuss, S., and El-Mallakh, R. S. (2021). Anatomical association between schizophrenia and cerebellum. Innov. Clin. Neurosci. 18, 47–49
Yizhar, O., Fenno, L. E., Prigge, M., Schneider, F., Davidson, T. J., O'Shea, D. J., et al. (2011). Neocortical excitation/inhibition balance in information processing and social dysfunction. Nature 477, 171–178. doi: 10.1038/nature10360
Yoshida, J., Oñate, M., Khatami, L., Vera, J., Nadim, F., and Khodakhah, K. (2022). Cerebellar contributions to the basal ganglia influence motor coordination, reward processing, and movement vigor. J. Neurosci. 42, 8406–8415. doi: 10.1523/JNEUROSCI.1535-22.2022
Zarghami, T. S., Zeidman, P., Razi, A., Bahrami, F., and Hossein-Zadeh, G. A. (2023). Dysconnection and cognition in schizophrenia: a spectral dynamic causal modeling study. Hum. Brain Mapp. 44, 2873–2896. doi: 10.1002/hbm.26251
Zhang, C., Li, Z., Shao, Y., Xie, B., Du, Y., Fang, Y., et al. (2011). Association study of tryptophan hydroxylase-2 gene in schizophrenia and its clinical features in Chinese Han population. J. Mol. Neurosci. 43, 406–411. doi: 10.1007/s12031-010-9458-2
Zhang, C., Zhou, P., and Yuan, T. (2016). The cholinergic system in the cerebellum: from structure to function. Rev. Neurosci. 27, 769–776. doi: 10.1515/revneuro-2016-0008
Zhao, P., Jiang, T., Wang, H., Jia, X., Li, A., Gong, H., et al. (2023). Upper brainstem cholinergic neurons project to ascending and descending circuits. BMC Biol. 21:135. doi: 10.1186/s12915-023-01625-y
Zhao, C., Zhu, J., Liu, X., Pu, C., Lai, Y., Chen, L., et al. (2018). Structural and functional brain abnormalities in schizophrenia: a cross-sectional study at different stages of the disease. Prog. Neuro-Psychopharmacol. Biol. Psychiatry 83, 27–32. doi: 10.1016/j.pnpbp.2017.12.017
Keywords: cerebellum, schizophrenia, cognitive impairment, cerebellar neurotransmitters, cerebellar connectivity
Citation: Faris P, Pischedda D, Palesi F and D’Angelo E (2024) New clues for the role of cerebellum in schizophrenia and the associated cognitive impairment. Front. Cell. Neurosci. 18:1386583. doi: 10.3389/fncel.2024.1386583
Edited by:
Fu-Chin Liu, National Yang Ming Chiao Tung University, TaiwanReviewed by:
Yuriko Iwakura, Niigata University, JapanHengyi Cao, Feinstein Institute for Medical Research, United States
Copyright © 2024 Faris, Pischedda, Palesi and D’Angelo. This is an open-access article distributed under the terms of the Creative Commons Attribution License (CC BY). The use, distribution or reproduction in other forums is permitted, provided the original author(s) and the copyright owner(s) are credited and that the original publication in this journal is cited, in accordance with accepted academic practice. No use, distribution or reproduction is permitted which does not comply with these terms.
*Correspondence: Egidio D’Angelo, ZWdpZGlvdWdvLmRhbmdlbG9AdW5pcHYuaXQ=; Pawan Faris, ZmFyaXMucGF3YW5AdW5pcHYuaXQ=