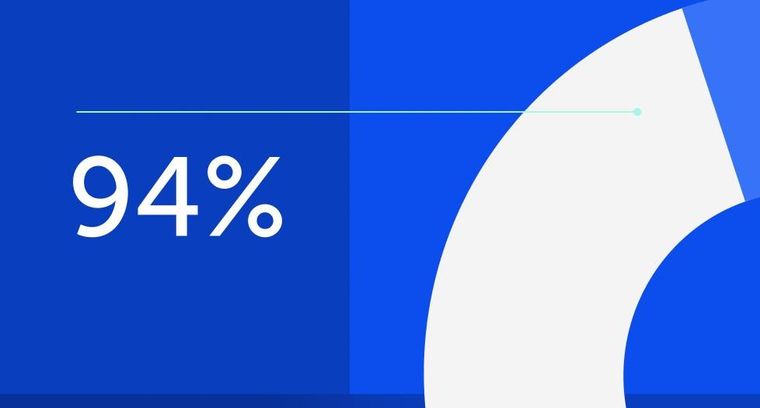
94% of researchers rate our articles as excellent or good
Learn more about the work of our research integrity team to safeguard the quality of each article we publish.
Find out more
ORIGINAL RESEARCH article
Front. Cell. Neurosci., 03 April 2024
Sec. Cellular Neurophysiology
Volume 18 - 2024 | https://doi.org/10.3389/fncel.2024.1374555
Introduction: Repetitive transcranial magnetic stimulation (rTMS) is a widely used therapeutic tool in neurology and psychiatry, but its cellular and molecular mechanisms are not fully understood. Standardizing stimulus parameters, specifically electric field strength, is crucial in experimental and clinical settings. It enables meaningful comparisons across studies and facilitates the translation of findings into clinical practice. However, the impact of biophysical properties inherent to the stimulated neurons and networks on the outcome of rTMS protocols remains not well understood. Consequently, achieving standardization of biological effects across different brain regions and subjects poses a significant challenge.
Methods: This study compared the effects of 10 Hz repetitive magnetic stimulation (rMS) in entorhino-hippocampal tissue cultures from mice and rats, providing insights into the impact of the same stimulation protocol on similar neuronal networks under standardized conditions.
Results: We observed the previously described plastic changes in excitatory and inhibitory synaptic strength of CA1 pyramidal neurons in both mouse and rat tissue cultures, but a higher stimulation intensity was required for the induction of rMS-induced synaptic plasticity in rat tissue cultures. Through systematic comparison of neuronal structural and functional properties and computational modeling, we found that morphological parameters of CA1 pyramidal neurons alone are insufficient to explain the observed differences between the groups. Although morphologies of mouse and rat CA1 neurons showed no significant differences, simulations confirmed that axon morphologies significantly influence individual cell activation thresholds. Notably, differences in intrinsic cellular properties were sufficient to account for the 10% higher intensity required for the induction of synaptic plasticity in the rat tissue cultures.
Conclusion: These findings demonstrate the critical importance of axon morphology and intrinsic cellular properties in predicting the plasticity effects of rTMS, carrying valuable implications for the development of computer models aimed at predicting and standardizing the biological effects of rTMS.
Repetitive transcranial magnetic stimulation (rTMS) is a non-invasive technique that modulates cortical excitability beyond the stimulation period (Chen et al., 1997; Huang et al., 2005; Suppa et al., 2016). Despite its increasing use for treating neuropsychiatric disorders such as major depression (Cocchi et al., 2018; Garnaat et al., 2018; Rehn et al., 2018; Voigt et al., 2019; Somaa et al., 2022), the cellular and molecular mechanisms of rTMS in human cortical networks remain not well-understood (Müller-Dahlhaus and Vlachos, 2013; Cirillo et al., 2017). Animal models, both in vivo and in vitro, have provided important insights into mechanisms by which rTMS modifies neuronal circuit excitability and plasticity (Vlachos et al., 2012; Tokay et al., 2014; Lenz et al., 2016; Hong et al., 2020; Romero et al., 2022; Eichler et al., 2023). It has been shown for example that rTMS affects the functional and structural properties of excitatory and inhibitory synapses (Tokay et al., 2009; Vlachos et al., 2012; Lenz et al., 2016), and that it facilitates the reorganisation of abnormal cortical circuits (Tang et al., 2021; Moretti et al., 2022). High frequency rTMS enhances plasticity in the primary motor cortex and mitigates cognitive deficits of aged mice (Ma et al., 2019; Cambiaghi et al., 2021). Conversely, low-frequency rTMS triggers plasticity in the dentate gyrus of the hippocampus with potential antidepressant-like effects (Cambiaghi et al., 2020). Recently, experimental evidence for an involvement of microglia, the brains resident immune cells in rTMS-induced synaptic plasticity was provided (Eichler et al., 2023).
Although rTMS has shown robust neurobiological effects in animal models, its efficacy in humans varies significantly (Goldsworthy et al., 2014; López-Alonso et al., 2014; Vallence et al., 2015; Guerra et al., 2020) due to challenges in dose standardization, among others (Peterchev et al., 2012; Turi et al., 2021). Considerable effort has been made to standardize the electric field strength across brain regions and subjects to improve reproducibility and better understand the effects of single pulse and rTMS across brain regions (Opitz et al., 2011; Thielscher et al., 2011; Saturnino et al., 2019). Meanwhile, it is becoming increasingly clear that computational models that predict the strength and orientation of TMS-induced electric field must be extended to biological effects, i.e., the electric fields must be coupled to biophysically realistic models (Aberra et al., 2018; Shirinpour et al., 2021). Indeed, these computational approaches provided important insight into the role of neuronal morphologies, specifically axons and myelination, which seem to play a critical role for single pulse TMS (Aberra et al., 2020). Yet, the majority of these models rely on partial reconstructions from acute brain slices or “artificial axons,” highlighting the challenge of achieving complete neuronal morphologies. Despite some efforts to model rTMS-induced changes in intracellular calcium levels as a proxy for predicting plasticity outcomes (Shirinpour et al., 2021), the current understanding of the dose–response relationship governing rTMS-induced synaptic plasticity remains limited. As a consequence, it is currently also not possible to compute and standardize synaptic plasticity induction across brain regions and subjects.
This study employed a cross-species analysis to elucidate the effects of 10 Hz repetitive magnetic stimulation (rMS) on CA1 pyramidal neurons in mouse and rat entorhino-hippocampal slice cultures (c.f., Vlachos et al., 2012; Lenz et al., 2016; Eichler et al., 2023), underscored by the well-documented connectivity and plasticity of the CA1 region. This approach allowed for an exploration of highly standardized rMS conditions on synaptic plasticity induction in two similar yet distinct neuronal networks. We found that CA1 pyramidal neurons in rat slice cultures required a 10% stronger intensity (measured by maximum stimulator output, MSO) than those in mice for the induction of plasticity. Leveraging multiscale single-cell modeling with axon morphology reconstructions our findings indicate that neuronal morphology alone does not predict rTMS-plasticity thresholds. However, axon morphologies significantly affect individual cell activation thresholds across species. Differences in intrinsic cellular properties were sufficient to account for the 10% higher intensity required for the induction of synaptic plasticity in the rat tissue cultures. Thus, intrinsic cellular properties are crucial in determining the effects of rTMS on plasticity. These findings highlight the significance of considering morphology and intrinsic properties for computerized predictions of activation thresholds and standardization of rTMS-induced synaptic plasticity.
Mice and rats were maintained in a 12 h light/dark cycle with food and water ad libitum. Every effort to minimize the distress and pain of animals was made. All experimental procedures were performed according to the German animal welfare legislation, approved by the appropriate animal welfare committee and the animal welfare officer of the University of Freiburg.
Mice of the strain C57BL/6J and rats of the strain Wistar (Crl:WI) of both sexes were used in this study.
Organotypic tissue cultures were prepared from mice and rats of both sexes at postnatal day 3–5 and allowed to mature for 3 weeks in a humidified atmosphere prior to experimental assessment. Cultures were stimulated in a standard 35 mm petri dish with rMS (10 Hz, 900 pulses); sham-stimulated cultures underwent the same handling but without stimulation. Whole cell voltage-clamp and current-clamp recordings were conducted 2–4 h after stimulation. High density microelectrode array (HD-MEA) recordings and current-clamp recordings were also performed on non-stimulated slice cultures to determine species-specific differences. CA1 pyramidal neurons from non-stimulated tissue cultures were post hoc stained and reconstructed using Neurolucida 360 (ver. 2019.1.3; MBF Bioscience). These reconstructions were utilized for multiscale single-cell modeling.
300 μm thick organotypic tissue cultures containing the hippocampus and the entorhinal cortex were prepared at postnatal day 3–5 from mice and rats of either sex as described previously (Vlachos et al., 2012; Galanis et al., 2021). The tissue cultures were maintained in an incubator at 35°C with 5% CO2 for at least 18 days before any experimental assessment. Tissue culture medium was changed 3 times per week and consisted of 50% (v/v) MEM, 25% (v/v) basal medium eagle (BME), 25% (v/v) heat-inactivated normal horse serum, 25 mm HEPES, 0.15% (w/v) NaHCO3, 0.65% (w/v) glucose, 0.1 mg/mL streptomycin, 100 U/mL penicillin, and 2 mm Glutamax (pH 7.3 with HCl or NaOH).
Tissue cultures were transferred in a standard 35 mm petri dish filled with standard extracellular solution (129 mM NaCl, 4 mM KCl, 1 mM MgCl2, 2 mM CaCl2, 4.2 mM glucose, 10 mM HEPES, 0.1 mg/mL streptomycin, 100 U/mL penicillin, pH 7.4, preheated to 35°C; 365 mOsm with sucrose). A 70 mm figure-of-eight coil (D70 Air Film Coil, Magstim) connected to a Magstim Super Rapid2 Plus1 (Magstim) was placed 1 mm above the lid of the petri dish and the cultures were stimulated with a protocol consisting of 900 pulses at 10 Hz. Tissue cultures were orientated in a way that the induced electric field within the tissue was approximately parallel to the dendritic tree of CA1 pyramidal neurons. Species- and time-matched cultures were not stimulated, but otherwise identically treated served as the controls.
Whole-cell voltage-clamp recordings of CA1 pyramidal cells were conducted as previously described (Vlachos et al., 2012; Lenz et al., 2016; Galanis et al., 2021). Recordings were conducted at 35°C. The bath solution contained 126 mM NaCl, 2.5 mM KCl, 26 mM NaHCO3, 1.25 mM NaH2PO4, 2 mM CaCl2, 2 mM MgCl2, and 10 mM glucose and was saturated with 95% O2/5% CO2. Miniature α-amino-3-hydroxy-5-methyl-4-isoxazolepropionic acid receptor-mediated excitatory postsynaptic currents (mEPSCs) were recorded in the presence of 10 μM D-APV and 0.5 μM TTX in the bath solution while the patch pipettes contained 126 mM K-gluconate, 4 mM KCl, 4 mM ATP-Mg, 0.3 mM GTP-Na2, 10 mM PO-creatine, 10 mM HEPES, and 0.1% (w/v) biocytin (pH 7.25 with KOH, 290 mOsm with sucrose). Miniature inhibitory postsynaptic currents (mIPSCs) were recorded in the presence of 0.5 μM TTX, 10 μM D-APV, and 10 μM CNQX in the bath solution while the patch pipettes contained 125 mM CsCl, 5 mM NaCl, 2 mM MgCl2, 2 mM Mg-ATP, 0.5 mM Na2-GTP, 0.1 mM EGTA and 10 mM HEPES (pH = 7.33 with CsOH; 275 mOsm with sucrose). Neurons were recorded at a holding potential of −70 mV. Series resistance was monitored in 2–4 min intervals and recordings were discarded if the series resistance reached ≥30 MΩ and the leak current changed significantly.
Whole-cell current-clamp recordings of CA1 pyramidal cells were conducted at 35°C. The bath solution contained 126 mM NaCl, 2.5 mM KCl, 26 mM NaHCO3, 1.25 mM NaH2PO4, 2 mM CaCl2, 2 mM MgCl2, 10 mM glucose, 10 μM D-APV, 10 μM CNQX, and 10 μM bicuculline methiodide and was saturated with 95% O2/5% CO2. Patch pipettes contained 126 mM K-gluconate, 4 mM KCl, 4 mM ATP-Mg, 0.3 mM GTP-Na2, 10 mM PO-creatine, 10 mM HEPES, and 0.1% (w/v) biocytin (pH 7.25 with KOH, 290 mOsm with sucrose). Neurons were hyperpolarized with −100 pA and then depolarized up to +400 pA with 1-s-long 10 pA current injection steps. Recordings were discarded of the series resistance reached ≥15 MΩ.
HD-MEA recordings of mouse and rat tissue cultures were conducted at 35°C. The bath solution was similar to the one used for voltage-clamp recordings without the addition of any drugs. Cultures were placed on an Accura HD-MEA chip (3Brain, Switzerland) and acclimatized for 2 min before recording. Each tissue culture was recorded for 10 min with a BioCAM DupleX (3Brain, Switzerland).
CA1 pyramidal neurons were patched with pipettes containing 126 mM K-gluconate, 4 mM KCl, 4 mM ATP-Mg, 0.3 mM GTP-Na2, 10 mM PO-creatine, 10 mM HEPES, and 1% (w/v) biocytin (pH 7.25 with KOH, 290 mOsm with sucrose). The neurons were kept in the whole-cell configuration for at least 10 min during which they were depolarized with 100 ms current injections of 200 pA at 5 Hz. Tissue cultures were fixed in a solution of 4% (w/v) PFA and 4% (w/v) sucrose in 0.01 M PBS for 1 h and further processed and images as previously described (Galanis et al., 2021).
CA1 pyramidal cells were reconstructed using Neurolucida 360 (ver. 2019.1.3; MBF Bioscience) as described previously (Shirinpour et al., 2021). Somata were reconstructed in 2D using the contour method in order to avoid overestimation of soma volume due to the somatic whole cell recording method used to fill the cells and the high signal intensity of these compartments.
Finite element method was used to create a three-dimensional mesh model consisting of two compartments, representing the bath solution and tissue cultures. The physical dimensions of the mesh model were based on the physical parameters of the in vitro settings, with a coil-to-Petri dish distance of 1 mm and the coil positioned above the culture. Electrical conductivities of 1.654 S/m and 0.275 S/m were assigned to the bath solution and culture, respectively. The rate of change of the coil current was set to 1.4 A/ms at 1% MSO and scaled up to higher stimulation intensities. Simulations of macroscopic electric fields were performed using SimNIBS (3.2.6) and MATLAB (2023a). A validated 70 mm MagStim figure-of-eight coil was utilized in all simulations (Thielscher and Kammer, 2004). The 99th percentile of the E-field, which represents the robust maximum value, was extracted from the volume compartment of the tissue culture.
Reconstructions were imported into the NeMo-TMS pipeline and endowed with a Jarsky model (Jarsky et al., 2005). When axons are “swapped,” the original axon is removed from the cell at the point of intersection with the soma or dendrite, and replaced with the axon of another cell that has been severed at the same point. Each cell is oriented with the apical dendrite pointing in the positive y direction, and axon orientations relative to this are preserved in the swapping process. For single-cell simulations, TMS is simulated as a uniform electric field of varying intensity, with the threshold defined as the smallest TMS amplitude that elicits a somatic action potential.
Analyses were performed with the person analyzing the data blind to the experimental condition. For this project, we used one or two tissue cultures from each animal. Electrophysiological data were analyzed using pClamp 11.2 software suite (Molecular Devices), the Easy Electrophysiology 2.5.0.2 (Easy Electrophysiology Ltd.) and BrainWave (3Brain) software. Statistical comparisons were made using Mann–Whitney test (to compare two groups) two-way ANOVA and Kruskal-Wallis test as indicated in the figure captions and text (GraphPad Prism 7). p values of <0.05 were considered a significant difference. All values represent mean ± SEM.
Confocal image stacks were exported as 2D projections and stored as TIFF files. Figures were prepared using Photoshop graphics software (Adobe). Image brightness and contrast were adjusted.
A 10 Hz stimulation protocol consisting of 900 pulses at 50% MSO was used to assess the effects of rMS on synaptic plasticity in brain tissue cultures prepared from mice of either sex (Figures 1A–C). Individual CA1 pyramidal neurons were patched and AMPA receptor-mediated mEPSCs were recorded 2–4 h after stimulation. In line with our previous work (c.f., Vlachos et al., 2012; Lenz et al., 2015, 2020; Eichler et al., 2023) a significant increase in mean mEPSC amplitude was observed as compared to age−/time-matched control cultures that were treated in the exact same way except for 10 Hz rMS (control; Figures 1D,E).
Figure 1. 10 Hz repetitive magnetic stimulation (rMS) induces synaptic plasticity in mouse CA1 pyramidal neurons. (A) Schematic illustration of the experimental setting. Organotypic tissue cultures are stimulated in a standard 35 mm petri dish filled with extracellular solution using a 70 mm figure-of-eight coil (900 pulses, 10 Hz, at 50% maximum stimulator output). (B) Overview of an organotypic tissue culture. Visualization of cytoarchitecture with DAPI. DG, Dentate gyrus; EC, entorhinal cortex; CA1 and CA3, Cornu Ammonis areas 1 and 3. Scale bar, 500 μm. (C) Patched CA1 pyramidal neurons filled with biocytin and identified post hoc with streptavidin-A488. Scale bar, 50 μm. (D,E) Sample traces and group data of AMPA receptor-mediated miniature excitatory postsynaptic currents (mEPSCs) recorded from mouse CA1 pyramidal neurons in sham-(control) and rMS-stimulated cultures 2–4 h after stimulation (control, n = 31 cells; rMS, n = 28 cells; Mann–Whitney test). (F,G) Sample traces and group data of GABA receptor-mediated miniature inhibitory postsynaptic currents (mIPSCs) recorded from mouse CA1 pyramidal neurons in sham-(control) and rMS-stimulated cultures 2–4 h after stimulation (control, n = 14 cells; rMS, n = 14 cells; Mann–Whitney test). Individual data points are indicated in this and the following figures by gray dots. Data are mean ± SEM. NS, not significant. *p < 0.05. **p < 0.01.
In a different set of cultures, we assessed 10 Hz rMS-induced changes in GABA receptor mediated mIPSCs onto CA1 pyramidal neurons using the experimental approach described above. A reduction in mean mIPSC amplitude was observed in these experiments as reported in our previous study (Figures 1F,G; c.f., Lenz et al., 2016). These results confirm the robust effects of 10 Hz rMS on mEPSC and mIPSC amplitudes of CA1 pyramidal neurons in mouse entorhino-hippocampal tissue cultures, which are consistent with a potentiation of excitatory synapses and a depression of inhibitory synapses.
The same 10 Hz protocol (10 Hz, 900 pulses, 50% MSO) was applied to tissue cultures prepared from rat brains (Figure 2), aiming to assess the rMS effects across two similar yet distinct neuronal networks. Age-matched rat entorhino-hippocampal cultures displayed a larger cross-section than mouse tissue cultures (Figure 2A), without any apparent morphological differences in CA1 pyramidal neurons (Figure 2B). Recordings of AMPA receptor-mediated mEPSCs from CA1 pyramidal neurons showed no statistically significant differences between control and 10 Hz rMS-stimulated preparations (Figures 2C,D). Inhibitory synaptic strength was also unaffected, as no significant differences in mean mIPSC amplitude and frequency were detected 2–4 h after stimulation (Figures 2E,F).
Figure 2. 10 Hz repetitive magnetic stimulation (rMS) at 50% maximum stimulator output fails to induce synaptic plasticity in rat CA1 pyramidal neurons. (A) Overview images of a mouse and rat organotypic tissue culture. DG, Dentate gyrus; EC, entorhinal cortex; CA1 and CA3, Cornu Ammonis areas 1 and 3. Scale bar, 1500 μm. (B) Patched rat CA1 pyramidal neuron filled with biocytin and identified post hoc with streptavidin-A488. Scale bar, 50 μm. (C,D) Sample traces and group data of AMPA receptor-mediated miniature excitatory postsynaptic currents (mEPSCs) recorded from rat CA1 pyramidal neurons in sham-(control) and rMS-stimulated cultures 2–4 h after stimulation (control, n = 38 cells; rMS, n = 71 cells; Mann–Whitney test). (E,F) Sample traces and group data of GABA receptor-mediated miniature inhibitory postsynaptic currents (mIPSCs) recorded from rat CA1 pyramidal neurons in sham-(control) and rMS-stimulated cultures 2–4 h after stimulation (control, n = 12 cells; rMS, n = 9 cells; Mann–Whitney test). Data are mean ± SEM. NS, Not significant.
The electric field (E-field) strength induced in the mouse and rat slice cultures was described using computational modeling (Saturnino et al., 2019). Three-dimensional mesh models were created with two compartments (i.e., bath solution and slice cultures) using the finite element method (Figure 3A). The physical dimensions of the mesh models were adapted from data obtained in mouse and rat brain issue cultures (Figure 3B). Macroscopic modeling of the E-field revealed that stimulation at 50% MSO induces a stronger electric field in the mouse (20.4 V/m) when compared to the rat tissue culture (19.3 V/m). Based on the modeling we determined that 53% MSO stimulation of rat tissue cultures would result in an E-field that is comparable to what we estimated in the mouse tissue cultures stimulated with 50% MSO (Figure 3C). Accordingly, another set of rat tissue cultures was stimulated with 53% MSO (10 Hz, 900 pulses) and AMPA receptor-mediated mEPSCs were recorded from CA1 pyramidal neurons 2–4 h after stimulation. No significant differences in mean mEPSC amplitude and frequency were observed in these experiments (Figure 3D). We conclude that simulation-based standardization of electric fields may not suffice to achieve comparable biological effects in mouse and rat CA1 pyramidal neurons, i.e., in neurons embedded in networks with comparable architectures and properties.
Figure 3. Modeling of electric fields in mouse and rat tissue cultures. (A) Visualization of the macroscopic electric field simulations from magnetic stimulation in vitro. (B) Three-dimensional mesh models of mouse and rat tissue cultures and the electric fields generated by a single magnetic pulse, respectively. (C) Comparison of the maximum electric field generated at distinct stimulation intensities in mouse and rat tissue cultures. The electric field generated in mouse slice cultures at 50% maximum stimulator output is attained with 53% maximum stimulator output in rat tissue cultures. (D) Group data of AMPA receptor-mediated mEPSCs recorded 2–4 h after stimulation from rat CA1 pyramidal neurons in sham-(control) and rMS-stimulated cultures; stimulation at 53% maximum stimulator output (control, n = 12 cells; rMS, n = 12 cells; Mann–Whitney test). Data are mean ± SEM. NS, not significant.
To test for differences in spontaneous network activity between mouse and rat entorhino-hippocampal slice cultures basal firing rates and field potential rates were recorded in a different set of 3-week-old mouse and rat tissue cultures using HD-MEA recordings (Figures 4A,B). No significant differences between mouse and rat tissue cultures were observed in firing and field potential (FP) rates in these experiments (Figures 4C–F). We conclude that baseline network activity is not responsible for the inability of rMS to induce plasticity in rat CA1 pyramidal neurons.
Figure 4. No significant differences in baseline network activity in mouse and rat tissue cultures. (A,B) Overview images of mouse and rat tissue culture on high-density microelectrode array chips. DG, dentate gyrus; EC, entorhinal cortex; CA1 and CA3, Cornu Ammonis areas 1 and 3. (C) Raster plots of spikes during a 10 min recording period in mouse and rat tissue cultures. (D–F) Group data of mean firing rate and mean field potential rate from mouse and rat tissue cultures (mouse, n = 4 cultures; rat, n = 5 cultures; Mann–Whitney test). Data are mean ± SEM. NS, not significant.
To investigate whether differences in CA1 pyramidal neuron size and complexity could explain the variation in rMS outcome, we reconstructed biocytin-filled and streptavidin-A488 stained CA1 pyramidal neurons from both rat and mouse hippocampal tissue cultures and analyzed their dendrites and axons (Figure 5). This was motivated by the observation that the brain sizes of mice and rats, as well as their tissue cultures, differ.
Figure 5. No significant morphological differences of CA1 pyramidal neurons in mouse and rat tissue cultures. (A) Examples of patched and biocytin-filled rat CA1 pyramidal neurons identified post hoc with streptavidin-A488, Scale bar, 100 μm. (B) Examples of three-dimensional neuronal reconstructions of mouse and rat CA1 pyramidal neurons. (C–H) Group data of mouse and rat apical and basal dendrites (mouse, n = 11 cells; rat, n = 11 cells; statistical comparisons for panels (C,D,G,H) were performed with Mann–Whitney test; statistical comparisons for panels (E,F) were performed with 2-way ANOVA). (I) Rat CA1 pyramidal neuron patched and filled with biocytin, identified post hoc with streptavidin-A488, and used for comprehensive neuronal reconstruction, encompassing dendritic and axonal neuronal structures. Scale bar, 50 μm. (J–L) Group data of mouse and rat axons [mouse, n = 6 cells; rat, n = 6 cells; statistical comparisons for panels (J,L) were performed with Mann–Whitney test; statistical comparisons for panel (K) were performed with 2-way ANOVA].
No significant differences were observed between the two groups in apical and basal dendritic length (Figures 5C,D). Sholl and diameter/volume analyses (Figures 5E–G) did not show any statistical significance between CA1 dendrites and their complexity of rat and mouse CA1 pyramidal neurons in entorhino-hippocampal tissue cultures. Similarly, no significant differences were observed when CA1 axons were reconstructed and compared in mouse and rat tissue cultures (Figures 5I–L). We conclude, that structural properties of CA1 pyramidal neurons are not statistically different and cannot explain why the rat tissue cultures do not respond to 10 Hz rMS even when the E-field is closely matched based on E-field simulations.
We assessed the impact of rMS on CA1 pyramidal neurons through a multiscale computational model that connects the physical input parameters of rMS to dendritic and axonal morphologies (Figure 6). This approach was necessary because our morphological analysis might not have encompassed distinctions pertinent to the neuronal activation induced by rMS.
Figure 6. Multiscale single-cell modeling of electromagnetic stimulation. (A) Changes in membrane voltage, to electromagnetic stimulation were modeled in realistic dendritic and axonal morphologies from reconstructed mouse and rat CA1 pyramidal neurons. (B) Group data of realistic dendritic morphologies with a standardized artificial axon (mouse, n = 6 cells; rat, n = 6 cells; Mann–Whitney test). (C) Group data of simulations with realistic dendritic and axonal morphologies (mouse, n = 6 cells; rat, n = 6 cells; Mann–Whitney test). (D) Group data for mouse and rat CA1 pyramidal neurons, categorizing those with axons exhibiting lowest (left) and highest (right) rMS depolarization thresholds (mouse, n = 6 cells; rat, n = 6 cells; Kruskal-Wallis test). Data are mean ± SEM. NS, not significant. *p < 0.01.
When examining the dendritic architecture of CA1 neurons in mice and rats, and employing a standardized artificial axon across all cells (c.f., Aberra et al., 2018; Shirinpour et al., 2021; Eichler et al., 2023), our simulations revealed no significant difference in the depolarization threshold elicited by rMS (Figures 6A,B). Subsequently, we investigated whether axonal morphologies might underlie the observed variability in our experimental outcomes. An additional series of simulations was conducted, this time integrating the authentic axonal morphologies of these neurons. Again, no significant differences in the depolarization thresholds were observed between the two groups (Figure 6C).
A noteworthy insight emerged from these simulations, confirming previous observations (e.g., Aberra et al., 2018, 2020): the axon’s influence is pivotal in establishing the rMS-induced depolarization threshold (Table 1). We followed up on this observation, by establishing connections between the axons responsible for the lowest and highest rMS depolarization thresholds across all mouse and rat cells. Indeed, an almost 2-fold difference in the depolarization thresholds was observed in these simulations across all reconstructed neurons (Figure 6D). Yet, despite these simulation results, the dissimilarity in rMS-triggered plasticity between mouse and rat tissue cultures remained unresolved, eluding a complete explanation based solely on the interactions of dendritic and axonal morphologies.
Next, active and passive membrane properties were recorded from CA1 pyramidal neurons and analyzed. Indeed, this set of experiments identified significant differences in the passive and active properties between mouse and rat CA1 pyramidal neurons (Figure 7).
Figure 7. Rat CA1 pyramidal neurons exhibit lower excitability in comparison to mice. (A) Sample traces from input–output recordings of CA1 pyramidal neurons of mouse and rat tissue cultures. (B,C) Group data of resting membrane potentials and input resistances from mouse and rat CA1 pyramidal neurons (mouse, n = 44 cells; rat, n = 56 cells; Mann–Whitney test). (D,E) Group data of action potential (AP) amplitude and threshold from mouse and rat CA1 pyramidal neurons (mouse, n = 44 cells; rat, n = 56 cells; Mann–Whitney test). (F) Current/frequency curve of CA1 pyramidal neurons of mouse and rat tissue cultures (mouse, n = 52 cells; rat, n = 63 cells; 2-way ANOVA). Data are mean ± SEM. NS, not significant. **p < 0.01. ***p < 0.001.
While no significant differences in membrane capacitances were noted between the two species (mouse: 90.7 ± 5.863 pF and rat: 101.7 ± 6.204 pF; Mann–Whitney test; p = 0.37; data not shown), the input resistance of mouse CA1 pyramidal neurons was significantly higher as compared to rat CA1 pyramidal neurons (mouse: 156.8 ± 11.65 MOhm and rat: 67.25 ± 4.909 MOhm; Mann–Whitney test; p < 0.001; U = 279), while the cells of both mice and rats were resting at comparable membrane potentials (Figures 7A–C). Consistently, the current–voltage (I/V) curves demonstrated that depolarizing mouse CA1 pyramidal neurons required less current compared to those in rat slice cultures.
Looking at the active membrane properties (Figures 7D–F) a similar trend was observed with the most striking differences being in the action potential induction threshold (mouse: −31.81 ± 0.877 mV; rat: −28.47 ± 0.744 mV; Mann–Whitney test; p = 0.0021; U = 794) and the first spike latency (mouse: 419.8 ± 56.03 ms; rat: 715 ± 77.36 ms; Mann–Whitney test; p = 0.0074; U = 15; data not shown). Figure 7F, shows that current injections produced stronger responses in mouse CA1 pyramidal neurons than in rat neurons, i.e., higher action potential frequencies at a lower current injection. These results indicated that mouse CA1 pyramidal neurons are more excitable than rat neurons, suggesting that higher stimulation intensities may be needed to induce rMS-induced plasticity in rat tissue cultures.
Subsequently, we tested whether a 10 Hz stimulation protocol applied at a higher intensity would induce plasticity in rat CA1 pyramidal neurons. Indeed, when rat tissue cultures were stimulated with 10 Hz rMS at 60% MSO a robust increase in the mean mEPSC amplitude was detected (Figure 8A), similar to what we observe in the mouse cultures stimulated at 50% MSO (cf., Figures 1E). In addition, a significant reduction in mean mIPSC amplitude was evident 2–4 h after rMS stimulation at 60% MSO in a different set of rat tissue cultures (Figure 8B; c.f., Figure 1G). These results demonstrate that rat CA1 pyramidal neurons do express rMS-induced plasticity, but require a higher stimulation intensity for rMS-induced potentiation of excitatory synapses and depression of inhibition to occur.
Figure 8. 10 Hz repetitive magnetic stimulation (rMS) at 60% MSO induces synaptic plasticity in rat CA1 pyramidal neurons. (A) Group data of AMPA receptor-mediated miniature excitatory postsynaptic currents (mEPSCs) recorded from rat CA1 pyramidal neurons from sham-(control) and rMS-stimulated cultures (control, n = 34 cells; rMS, n = 16 cells; Mann–Whitney test). (B) Sample traces and group data of miniature inhibitory postsynaptic currents (mIPSCs) recorded from rat CA1 pyramidal neurons from sham- (control) and rMS- stimulated cultures (control, n = 14 cells; rMS, n = 17 cells; Mann–Whitney test. One data point outside of axis limits in mIPSC amplitude and frequency respectively). Data are mean ± SEM. NS, not significant. *p < 0.05. ***p < 0.001.
In this study, we explored the factors influencing the threshold for 10 Hz rTMS-induced synaptic plasticity. Using mouse and rat entorhino-hippocampal slice cultures, we investigated neuronal structure, excitability, and network activity. In mouse CA1 pyramidal neurons, we confirmed the well-known potentiation of excitatory synapses and depression of inhibitory synapses, highlighting robust rTMS-induced synaptic plasticity under controlled conditions. However, despite similar neuronal morphology and network activity in rat CA1 pyramidal neurons, standardizing electric fields through prospective modeling did not produce the same biological effect. Adjusting the stimulation protocol to account for rat neurons’ lower excitability led to comparable synaptic changes. These results emphasize that electric field standardization alone cannot predict rTMS effects, necessitating realistic compartmental models of cellular properties in different brain regions for accurate predictions.
Over the past decade, the utilization of rTMS has experienced a significant surge in both research and clinical domains (Dayan et al., 2013; Paulus et al., 2013; Suppa et al., 2016; Blumberger et al., 2018; Lefaucheur et al., 2020; Lorentzen et al., 2022). Consequently, extensive efforts have been dedicated to identify the crucial parameters that influence the effects of rTMS on brain tissue (Deng et al., 2013; Lefaucheur et al., 2020; Zmeykina et al., 2020; Turi et al., 2021). Among these parameters, the induced electric field has been identified as a critical factor directly influencing the effects of rTMS on cortical tissue (Liu et al., 2018). While advancements in computational tools have enabled the calculation of rTMS-induced electric field (Thielscher et al., 2015), these models have primarily relied on mesoscopic structural parameters of the targeted stimulation area, i.e., head and brain geometries. In recent years, there has been a growing adoption of multiscale modeling approaches to investigate the impact of TMS on individual neurons (Kamitani et al., 2001; Aberra et al., 2018, 2020; Shirinpour et al., 2021). Notably, these neuronal models are being integrated into mesoscopic brain models, enabling exploration of the effects of cortical folding and the precise positioning of neurons, such as distinguishing between the gyral crown and gyral groove, in individual subjects (Salvador et al., 2011; Seo and Jun, 2019; Aberra et al., 2020; Turi et al., 2022). While these models represent a significant advancement toward standardization and precision medicine in the field, it is increasingly evident that solely modeling electric fields and their interactions with individual neuronal morphologies (derived from animal models) may not be sufficient to predict and standardize the biological effects of rTMS across various brain regions and individuals (Turi et al., 2022). The findings from this cross-species study present experimental evidence, underscoring the insufficiency of meticulous experimental standardization and electric field modeling in guaranteeing robust biological effects of rTMS. Notably, computational modeling showed weaker induced electric fields in rat tissue cultures despite their size difference compared to mouse tissue cultures. Even when efforts were made to match electric fields, the plasticity effects in rat cultures could not be reproduced.
In this context, it is crucial to highlight that our experiments revealed no statistically significant morphological differences between the cultured CA1 pyramidal neurons of mice and rats. The comprehensive analysis of both apical and basal dendrites demonstrated comparable total dendritic length, complexity, and overall volume in both rat and mouse pyramidal neurons of organotypic tissue cultures. These results align with previously published data that compared mouse and rat hippocampal CA1 neurons in acute slice preparations (Routh et al., 2009). However, it is worth noting that the total volume of these cells, apart from the observed morphological features, was found to be higher in rat slices. Though differences between acute brain slices and tissue cultures could contribute to the observed discrepancy, and reliable volume-reconstructions of patched somata were not feasible in our study, it is crucial to highlight the key advantage of tissue cultures. Using 3-week-old tissue cultures enabled us to investigate neurons within brain tissue that had not undergone acute slicing immediately before experimental assessment. This allowed us to study undamaged pyramidal neurons and enabled us to generate detailed morphological reconstructions, encompassing both dendrites and axons. Specifically, complete reconstructions of axons are of utmost importance for precise evaluation of rTMS outcomes, considering their substantial interaction with the electric field (Siebner et al., 2022). Previous studies, including our own work, often relied on artificial or simplified axon morphologies (Aberra et al., 2018, 2020; Shirinpour et al., 2021; Eichler et al., 2023). Importantly, our investigation revealed no significant differences in axons of cultured CA1 neurons between mice and rats. This finding suggests that the observed inability of rat CA1 neurons to exhibit synaptic plasticity cannot be trivially attributed to differences in axon morphology.
Nevertheless, our simulations identified axons that are twice as effective at depolarizing neurons, irrespective of soma and dendrite shapes. This emphasizes the need for a systematic assessment of various axonal morphologies in rTMS-induced synaptic plasticity, also considering factors like myelination and the role of oligodendrocytes. We propose the possibility of “super-responder cells” within complex cortical networks–cells highly responsive to rTMS at specific stimulation intensities. This notion finds support in the observation that not all neurons of the present and our previous studies (c.f., Vlachos et al., 2012; Lenz et al., 2016, 2020; Eichler et al., 2023) displayed elevated mEPSC amplitudes or decreased mIPSC within the 2–4 h following stimulation.
The results of the present study suggest that understanding the differing effects of rTMS on mouse and rat CA1 pyramidal neurons requires considering their intrinsic cellular properties. Consistent with prior research on rat and mouse slices (Routh et al., 2009), our study shows that rat CA1 pyramidal neurons have a higher action potential threshold compared to mice, making them less excitable. Notably, we found that rat CA1 neurons have lower input resistance than mouse neurons, further highlighting reduced excitability in rat neurons. However, it is worth noting that a study by Routh and colleagues in 2009 reported similar input resistance between the two species (Routh et al., 2009) potentially due to differences in acute slices prepared from adult animals and organotypic tissue cultures.
Do morphological and biophysical properties alone predict rTMS outcomes adequately? Additional factors, like neuromodulators such as dopamine, serotonin, and noradrenaline, influence cortical excitability, impacting how neurons respond to rTMS and altering plasticity threshold, magnitude, and direction (Greenberg et al., 2000; Nitsche et al., 2006; Martorana et al., 2009; Nitsche et al., 2010; le Grand et al., 2011; Kuo et al., 2017). Furthermore, neuromodulators can impact the capacity of neurons to express plasticity without affecting excitability and other baseline functional and structural properties, a phenomenon known as metaplasticity (Abraham and Bear, 1996; Seol et al., 2007). It is important to also note that non-neuronal cells can significantly influence the capacity of neurons to express synaptic plasticity (Stellwagen et al., 2005; Henneberger et al., 2010; Allen, 2014; Andoh and Koyama, 2021; Sancho et al., 2021; Kleidonas et al., 2023). Our prior work has provided evidence that cytokines derived from microglia play a crucial role in facilitating rTMS-induced plasticity (Eichler et al., 2023). Finally, the impact of network activity on the outcome of rTMS must be considered. These factors collectively underscore the multifaceted nature of the processes involved in influencing and modulating the outcomes of rTMS-induced plasticity. Organotypic slice cultures serve as valuable tools for investigating these and other aspects of rTMS-induced plasticity, highlighting the necessity for rigorously validated computer models that link the induced electric fields with biophysically realistic neurons and networks. These models hold the potential to predict the biological outcomes of rTMS, offering valuable insights into its effects and guiding the adaptation of stimulation protocols to achieve consistent desired effects across different brain regions and individuals.
The raw data supporting the conclusions of this article will be made available by the authors, without undue reservation.
The animal study was approved by Animal welfare officer of the University of Freiburg. The study was conducted in accordance with the local legislation and institutional requirements.
CG: Conceptualization, Data curation, Formal analysis, Investigation, Methodology, Supervision, Visualization, Writing – original draft, Writing – review & editing. LN: Formal analysis, Investigation, Writing – original draft. NH: Data curation, Formal analysis, Investigation, Software, Writing – original draft. ZT: Data curation, Formal analysis, Investigation, Software, Writing – original draft. PJ: Data curation, Methodology, Software, Supervision, Writing – review & editing. AV: Conceptualization, Data curation, Funding acquisition, Project administration, Supervision, Writing – original draft, Writing – review & editing.
The author(s) declare financial support was received for the research, authorship, and/or publication of this article. The work was supported by National Institutes of Health, USA (NIH; 1R01NS109498) and by the Federal Ministry of Education and Research, Germany (BMBF, 01GQ2205A).
We thank Susanna Glaser and Emina Deumic for skillful assistance in tissue culturing.
The authors declare that the research was conducted in the absence of any commercial or financial relationships that could be construed as a potential conflict of interest.
The author(s) declared that they were an editorial board member of Frontiers, at the time of submission. This had no impact on the peer review process and the final decision.
All claims expressed in this article are solely those of the authors and do not necessarily represent those of their affiliated organizations, or those of the publisher, the editors and the reviewers. Any product that may be evaluated in this article, or claim that may be made by its manufacturer, is not guaranteed or endorsed by the publisher.
Aberra, A. S., Peterchev, A. V., and Grill, W. M. (2018). Biophysically realistic neuron models for simulation of cortical stimulation. J. Neural Eng. 15:066023. doi: 10.1088/1741-2552/aadbb1
Aberra, A. S., Wang, B., Grill, W. M., and Peterchev, A. V. (2020). Simulation of transcranial magnetic stimulation in head model with morphologically-realistic cortical neurons. Brain Stimul. 13, 175–189. doi: 10.1016/j.brs.2019.10.002
Abraham, W. C., and Bear, M. F. (1996). Metaplasticity: the plasticity of synaptic plasticity. Trends Neurosci. 19, 126–130. doi: 10.1016/s0166-2236(96)80018-x
Allen, N. J. (2014). Astrocyte regulation of synaptic behavior. Annu. Rev. Cell Dev. Biol. 30, 439–463. doi: 10.1146/annurev-cellbio-100913-013053
Andoh, M., and Koyama, R. (2021). Microglia regulate synaptic development and plasticity. Dev. Neurobiol. 81, 568–590. doi: 10.1002/dneu.22814
Blumberger, D. M., Vila-Rodriguez, F., Thorpe, K. E., Feffer, K., Noda, Y., Giacobbe, P., et al. (2018). Effectiveness of theta burst versus high-frequency repetitive transcranial magnetic stimulation in patients with depression (THREE-D): a randomised non-inferiority trial. Lancet 391, 1683–1692. doi: 10.1016/S0140-6736(18)30295-2
Cambiaghi, M., Cherchi, L., Masin, L., Infortuna, C., Briski, N., Caviasco, C., et al. (2021). High-frequency repetitive transcranial magnetic stimulation enhances layer II/III morphological dendritic plasticity in mouse primary motor cortex. Behav. Brain Res. 410:113352. doi: 10.1016/j.bbr.2021.113352
Cambiaghi, M., Crupi, R., Bautista, E. L., Elsamadisi, A., Malik, W., Pozdniakova, H., et al. (2020). The effects of 1-Hz rTMS on emotional behavior and dendritic complexity of mature and newly generated dentate gyrus neurons in male mice. Int. J. Environ. Res. Public Health 17:4074. doi: 10.3390/ijerph17114074
Chen, R., Classen, J., Gerloff, C., Celnik, P., Wassermann, E. M., Hallett, M., et al. (1997). Depression of motor cortex excitability by low-frequency transcranial magnetic stimulation. Neurology 48, 1398–1403. doi: 10.1212/WNL.48.5.1398
Cirillo, G., Di Pino, G., Capone, F., Ranieri, F., Florio, L., Todisco, V., et al. (2017). Neurobiological after-effects of non-invasive brain stimulation. Brain Stimul. 10, 1–18. doi: 10.1016/j.brs.2016.11.009
Cocchi, L., Zalesky, A., Nott, Z., Whybird, G., Fitzgerald, P. B., and Breakspear, M. (2018). Transcranial magnetic stimulation in obsessive-compulsive disorder: a focus on network mechanisms and state dependence. Neuroimage Clin. 19, 661–674. doi: 10.1016/j.nicl.2018.05.029
Dayan, E., Censor, N., Buch, E. R., Sandrini, M., and Cohen, L. G. (2013). Noninvasive brain stimulation: from physiology to network dynamics and back. Nat. Neurosci. 16, 838–844. doi: 10.1038/nn.3422
Deng, Z.-D., Lisanby, S. H., and Peterchev, A. V. (2013). Electric field depth-focality tradeoff in transcranial magnetic stimulation: simulation comparison of 50 coil designs. Brain Stimul. 6, 1–13. doi: 10.1016/j.brs.2012.02.005
Eichler, A., Kleidonas, D., Turi, Z., Fliegauf, M., Kirsch, M., Pfeifer, D., et al. (2023). Microglial cytokines mediate plasticity induced by 10 Hz repetitive magnetic stimulation. J. Neurosci. 43, 3042–3060. doi: 10.1523/JNEUROSCI.2226-22.2023
Galanis, C., Fellenz, M., Becker, D., Bold, C., Lichtenthaler, S. F., Müller, U. C., et al. (2021). Amyloid-Beta mediates homeostatic synaptic plasticity. J. Neurosci. 41, 5157–5172. doi: 10.1523/JNEUROSCI.1820-20.2021
Garnaat, S. L., Yuan, S., Wang, H., Philip, N. S., and Carpenter, L. L. (2018). Updates on transcranial magnetic stimulation therapy for major depressive disorder. Psychiatr. Clin. North Am. 41, 419–431. doi: 10.1016/j.psc.2018.04.006
Goldsworthy, M. R., Müller-Dahlhaus, F., Ridding, M. C., and Ziemann, U. (2014). Inter-subject variability of LTD-like plasticity in human motor cortex: a matter of preceding motor activation. Brain Stimul. 7, 864–870. doi: 10.1016/j.brs.2014.08.004
Greenberg, B. D., Ziemann, U., Corá-Locatelli, G., Harmon, A., Murphy, D. L., Keel, J. C., et al. (2000). Altered cortical excitability in obsessive–compulsive disorder. Neurology 54:142. doi: 10.1212/WNL.54.1.142
Guerra, A., López-Alonso, V., Cheeran, B., and Suppa, A. (2020). Variability in non-invasive brain stimulation studies: reasons and results. Neurosci. Lett. 719:133330. doi: 10.1016/j.neulet.2017.12.058
Henneberger, C., Papouin, T., Oliet, S. H. R., and Rusakov, D. A. (2010). Long-term potentiation depends on release of D-serine from astrocytes. Nature 463, 232–236. doi: 10.1038/nature08673
Hong, Y., Liu, Q., Peng, M., Bai, M., Li, J., Sun, R., et al. (2020). High-frequency repetitive transcranial magnetic stimulation improves functional recovery by inhibiting neurotoxic polarization of astrocytes in ischemic rats. J. Neuroinflammation 17:150. doi: 10.1186/s12974-020-01747-y
Huang, Y.-Z., Edwards, M. J., Rounis, E., Bhatia, K. P., and Rothwell, J. C. (2005). Theta burst stimulation of the human motor cortex. Neuron 45, 201–206. doi: 10.1016/j.neuron.2004.12.033
Jarsky, T., Roxin, A., Kath, W. L., and Spruston, N. (2005). Conditional dendritic spike propagation following distal synaptic activation of hippocampal CA1 pyramidal neurons. Nat. Neurosci. 8, 1667–1676. doi: 10.1038/nn1599
Kamitani, Y., Bhalodia, V. M., Kubota, Y., and Shimojo, S. (2001). A model of magnetic stimulation of neocortical neurons. Neurocomputing 38–40, 697–703. doi: 10.1016/S0925-2312(01)00447-7
Kleidonas, D., Kirsch, M., Andrieux, G., Pfeifer, D., Boerries, M., and Vlachos, A. (2023). Microglia modulate TNFα-mediated synaptic plasticity. Glia 71, 2117–2136. doi: 10.1002/glia.24383
Kuo, H.-I., Paulus, W., Batsikadze, G., Jamil, A., Kuo, M.-F., and Nitsche, M. A. (2017). Acute and chronic noradrenergic effects on cortical excitability in healthy humans. Int. J. Neuropsychopharmacol. 20, 634–643. doi: 10.1093/ijnp/pyx026
le Grand, S. M., Supornsilpchai, W., Saengjaroentham, C., and Srikiatkhachorn, A. (2011). Serotonin depletion leads to cortical Hyperexcitability and trigeminal nociceptive facilitation via the nitric oxide pathway. Headache 51, 1152–1160. doi: 10.1111/j.1526-4610.2011.01931.x
Lefaucheur, J.-P., Aleman, A., Baeken, C., Benninger, D. H., Brunelin, J., Di Lazzaro, V., et al. (2020). Evidence-based guidelines on the therapeutic use of repetitive transcranial magnetic stimulation (rTMS): an update (2014–2018). Clin. Neurophysiol. 131, 474–528. doi: 10.1016/j.clinph.2019.11.002
Lenz, M., Eichler, A., Kruse, P., Strehl, A., Rodriguez-Rozada, S., Goren, I., et al. (2020). Interleukin 10 restores lipopolysaccharide-induced alterations in synaptic plasticity probed by repetitive magnetic stimulation. Front. Immunol. 11:614509. doi: 10.3389/fimmu.2020.614509
Lenz, M., Galanis, C., Müller-Dahlhaus, F., Opitz, A., Wierenga, C. J., Szabó, G., et al. (2016). Repetitive magnetic stimulation induces plasticity of inhibitory synapses. Nat. Commun. 7:10020. doi: 10.1038/ncomms10020
Lenz, M., Platschek, S., Priesemann, V., Becker, D., Willems, L. M., Ziemann, U., et al. (2015). Repetitive magnetic stimulation induces plasticity of excitatory postsynapses on proximal dendrites of cultured mouse CA1 pyramidal neurons. Brain Struct. Funct. 220, 3323–3337. doi: 10.1007/s00429-014-0859-9
Liu, A., Vöröslakos, M., Kronberg, G., Henin, S., Krause, M. R., Huang, Y., et al. (2018). Immediate neurophysiological effects of transcranial electrical stimulation. Nat. Commun. 9:5092. doi: 10.1038/s41467-018-07233-7
López-Alonso, V., Cheeran, B., Río-Rodríguez, D., and Fernández-Del-Olmo, M. (2014). Inter-individual variability in response to non-invasive brain stimulation paradigms. Brain Stimul. 7, 372–380. doi: 10.1016/j.brs.2014.02.004
Lorentzen, R., Nguyen, T. D., McGirr, A., Hieronymus, F., and Østergaard, S. D. (2022). The efficacy of transcranial magnetic stimulation (TMS) for negative symptoms in schizophrenia: a systematic review and meta-analysis. Schizophrenia (Heidelb) 8, 35–12. doi: 10.1038/s41537-022-00248-6
Ma, Q., Geng, Y., Wang, H.-L., Han, B., Wang, Y.-Y., Li, X.-L., et al. (2019). High frequency repetitive transcranial magnetic stimulation alleviates cognitive impairment and modulates hippocampal synaptic structural plasticity in aged mice. Front. Aging Neurosci. 11:235. doi: 10.3389/fnagi.2019.00235
Martorana, A., Mori, F., Esposito, Z., Kusayanagi, H., Monteleone, F., Codecà, C., et al. (2009). Dopamine modulates cholinergic cortical excitability in Alzheimer’s disease patients. Neuropsychopharmacology 34, 2323–2328. doi: 10.1038/npp.2009.60
Moretti, J., Terstege, D. J., Poh, E. Z., Epp, J. R., and Rodger, J. (2022). Low intensity repetitive transcranial magnetic stimulation modulates brain-wide functional connectivity to promote anti-correlated c-Fos expression. Sci. Rep. 12:20571. doi: 10.1038/s41598-022-24934-8
Müller-Dahlhaus, F., and Vlachos, A. (2013). Unraveling the cellular and molecular mechanisms of repetitive magnetic stimulation. Front. Mol. Neurosci. 6:50. doi: 10.3389/fnmol.2013.00050
Nitsche, M. A., Lampe, C., Antal, A., Liebetanz, D., Lang, N., Tergau, F., et al. (2006). Dopaminergic modulation of long-lasting direct current-induced cortical excitability changes in the human motor cortex. Eur. J. Neurosci. 23, 1651–1657. doi: 10.1111/j.1460-9568.2006.04676.x
Nitsche, M. A., Monte-Silva, K., Kuo, M.-F., and Paulus, W. (2010). Dopaminergic impact on cortical excitability in humans. Rev. Neurosci. 21, 289–298. doi: 10.1515/REVNEURO.2010.21.4.289
Opitz, A., Windhoff, M., Heidemann, R. M., Turner, R., and Thielscher, A. (2011). How the brain tissue shapes the electric field induced by transcranial magnetic stimulation. NeuroImage 58, 849–859. doi: 10.1016/j.neuroimage.2011.06.069
Paulus, W., Peterchev, A. V., and Ridding, M. (2013). Transcranial electric and magnetic stimulation: technique and paradigms. Handb. Clin. Neurol. 116, 329–342. doi: 10.1016/B978-0-444-53497-2.00027-9
Peterchev, A. V., Wagner, T. A., Miranda, P. C., Nitsche, M. A., Paulus, W., Lisanby, S. H., et al. (2012). Fundamentals of transcranial electric and magnetic stimulation dose: definition, selection, and reporting practices. Brain Stimul. 5, 435–453. doi: 10.1016/j.brs.2011.10.001
Rehn, S., Eslick, G. D., and Brakoulias, V. (2018). A meta-analysis of the effectiveness of different cortical targets used in repetitive transcranial magnetic stimulation (rTMS) for the treatment of obsessive-compulsive disorder (OCD). Psychiatry Q. 89, 645–665. doi: 10.1007/s11126-018-9566-7
Romero, M. C., Merken, L., Janssen, P., and Davare, M. (2022). Neural effects of continuous theta-burst stimulation in macaque parietal neurons. eLife 11:e65536. doi: 10.7554/eLife.65536
Routh, B. N., Johnston, D., Harris, K., and Chitwood, R. A. (2009). Anatomical and electrophysiological comparison of CA1 pyramidal neurons of the rat and mouse. J. Neurophysiol. 102, 2288–2302. doi: 10.1152/jn.00082.2009
Salvador, R., Silva, S., Basser, P. J., and Miranda, P. C. (2011). Determining which mechanisms lead to activation in the motor cortex: a modeling study of transcranial magnetic stimulation using realistic stimulus waveforms and sulcal geometry. Clin. Neurophysiol. 122, 748–758. doi: 10.1016/j.clinph.2010.09.022
Sancho, L., Contreras, M., and Allen, N. J. (2021). Glia as sculptors of synaptic plasticity. Neurosci. Res. 167, 17–29. doi: 10.1016/j.neures.2020.11.005
Saturnino, G. B., Puonti, O., Nielsen, J. D., Antonenko, D., Madsen, K. H., and Thielscher, A. (2019). “SimNIBS 2.1: a comprehensive pipeline for individualized electric field modelling for transcranial brain stimulation,” In Brain and human body modeling: computational human modeling at EMBC 2018, eds. S. Makarov, M. Horner, and G. Noetscher (Cham (CH): Springer). Available at: http://www.ncbi.nlm.nih.gov/books/NBK549569/ (Accessed June 13, 2023).
Seo, H., and Jun, S. C. (2019). Relation between the electric field and activation of cortical neurons in transcranial electrical stimulation. Brain Stimul. 12, 275–289. doi: 10.1016/j.brs.2018.11.004
Seol, G. H., Ziburkus, J., Huang, S., Song, L., Kim, I. T., Takamiya, K., et al. (2007). Neuromodulators control the polarity of spike-timing-dependent synaptic plasticity. Neuron 55, 919–929. doi: 10.1016/j.neuron.2007.08.013
Shirinpour, S., Hananeia, N., Rosado, J., Tran, H., Galanis, C., Vlachos, A., et al. (2021). Multi-scale modeling toolbox for single neuron and subcellular activity under transcranial magnetic stimulation. Brain Stimul. 14, 1470–1482. doi: 10.1016/j.brs.2021.09.004
Siebner, H. R., Funke, K., Aberra, A. S., Antal, A., Bestmann, S., Chen, R., et al. (2022). Transcranial magnetic stimulation of the brain: what is stimulated? – a consensus and critical position paper. Clin. Neurophysiol. 140, 59–97. doi: 10.1016/j.clinph.2022.04.022
Somaa, F. A., de Graaf, T. A., and Sack, A. T. (2022). Transcranial magnetic stimulation in the treatment of neurological diseases. Front. Neurol. 13:793253. doi: 10.3389/fneur.2022.793253
Stellwagen, D., Beattie, E. C., Seo, J. Y., and Malenka, R. C. (2005). Differential regulation of AMPA receptor and GABA receptor trafficking by tumor necrosis factor-alpha. J. Neurosci. 25, 3219–3228. doi: 10.1523/JNEUROSCI.4486-04.2005
Suppa, A., Huang, Y.-Z., Funke, K., Ridding, M. C., Cheeran, B., Di Lazzaro, V., et al. (2016). Ten years of Theta burst stimulation in humans: established knowledge, unknowns and prospects. Brain Stimul. 9, 323–335. doi: 10.1016/j.brs.2016.01.006
Tang, A. D., Bennett, W., Bindoff, A. D., Bolland, S., Collins, J., Langley, R. C., et al. (2021). Subthreshold repetitive transcranial magnetic stimulation drives structural synaptic plasticity in the young and aged motor cortex. Brain Stimul. 14, 1498–1507. doi: 10.1016/j.brs.2021.10.001
Thielscher, A., Antunes, A., and Saturnino, G. B. (2015). Field modeling for transcranial magnetic stimulation: a useful tool to understand the physiological effects of TMS?, In 2015 37th Annual International Conference of the IEEE Engineering in Medicine and Biology Society (EMBC), 222–225. Available at: https://ieeexplore.ieee.org/document/7318340
Thielscher, A., and Kammer, T. (2004). Electric field properties of two commercial figure-8 coils in TMS: calculation of focality and efficiency. Clin. Neurophysiol. 115, 1697–1708. doi: 10.1016/j.clinph.2004.02.019
Thielscher, A., Opitz, A., and Windhoff, M. (2011). Impact of the gyral geometry on the electric field induced by transcranial magnetic stimulation. NeuroImage 54, 234–243. doi: 10.1016/j.neuroimage.2010.07.061
Tokay, T., Holl, N., Kirschstein, T., Zschorlich, V., and Köhling, R. (2009). High-frequency magnetic stimulation induces long-term potentiation in rat hippocampal slices. Neurosci. Lett. 461, 150–154. doi: 10.1016/j.neulet.2009.06.032
Tokay, T., Kirschstein, T., Rohde, M., Zschorlich, V., and Köhling, R. (2014). NMDA receptor-dependent metaplasticity by high-frequency magnetic stimulation. Neural Plast. 2014:684238, 1–8. doi: 10.1155/2014/684238
Turi, Z., Hananeia, N., Shirinpour, S., Opitz, A., Jedlicka, P., and Vlachos, A. (2022). Dosing transcranial magnetic stimulation of the primary motor and dorsolateral prefrontal cortices with multi-scale modeling. Front. Neurosci. 16:929814. doi: 10.3389/fnins.2022.929814
Turi, Z., Lenz, M., Paulus, W., Mittner, M., and Vlachos, A. (2021). Selecting stimulation intensity in repetitive transcranial magnetic stimulation studies: a systematic review between 1991 and 2020. Eur. J. Neurosci. 53, 3404–3415. doi: 10.1111/ejn.15195
Vallence, A.-M., Goldsworthy, M. R., Hodyl, N. A., Semmler, J. G., Pitcher, J. B., and Ridding, M. C. (2015). Inter- and intra-subject variability of motor cortex plasticity following continuous theta-burst stimulation. Neuroscience 304, 266–278. doi: 10.1016/j.neuroscience.2015.07.043
Vlachos, A., Müller-Dahlhaus, F., Rosskopp, J., Lenz, M., Ziemann, U., and Deller, T. (2012). Repetitive magnetic stimulation induces functional and structural plasticity of excitatory postsynapses in mouse organotypic hippocampal slice cultures. J. Neurosci. 32, 17514–17523. doi: 10.1523/JNEUROSCI.0409-12.2012
Voigt, J., Carpenter, L., and Leuchter, A. (2019). A systematic literature review of the clinical efficacy of repetitive transcranial magnetic stimulation (rTMS) in non-treatment resistant patients with major depressive disorder. BMC Psychiatry 19:13. doi: 10.1186/s12888-018-1989-z
Keywords: synaptic plasticity, morphology, axons, inhibition, excitation, whole-cell patch-clamp recordings, organotypic tissue cultures
Citation: Galanis C, Neuhaus L, Hananeia N, Turi Z, Jedlicka P and Vlachos A (2024) Axon morphology and intrinsic cellular properties determine repetitive transcranial magnetic stimulation threshold for plasticity. Front. Cell. Neurosci. 18:1374555. doi: 10.3389/fncel.2024.1374555
Received: 22 January 2024; Accepted: 13 March 2024;
Published: 03 April 2024.
Edited by:
Corette J. Wierenga, Radboud University, NetherlandsReviewed by:
Marco Cambiaghi, University of Verona, ItalyCopyright © 2024 Galanis, Neuhaus, Hananeia, Turi, Jedlicka and Vlachos. This is an open-access article distributed under the terms of the Creative Commons Attribution License (CC BY). The use, distribution or reproduction in other forums is permitted, provided the original author(s) and the copyright owner(s) are credited and that the original publication in this journal is cited, in accordance with accepted academic practice. No use, distribution or reproduction is permitted which does not comply with these terms.
*Correspondence: Andreas Vlachos, dmxhY2hvc0BhbmF0LnVuaS1mcmVpYnVyZy5kZQ==
Disclaimer: All claims expressed in this article are solely those of the authors and do not necessarily represent those of their affiliated organizations, or those of the publisher, the editors and the reviewers. Any product that may be evaluated in this article or claim that may be made by its manufacturer is not guaranteed or endorsed by the publisher.
Research integrity at Frontiers
Learn more about the work of our research integrity team to safeguard the quality of each article we publish.