- 1Department of Pharmacology and Toxicology, College of Pharmacy, University of Arizona, Tucson, AZ, United States
- 2Southern Arizona VA Health Care System, Tucson, AZ, United States
- 3Department of Neurosciences, College of Medicine, University of Arizona, Tucson, AZ, United States
- 4Southwest Environmental Health Science Center, University of Arizona, Tucson, AZ, United States
- 5Center for Innovation in Brain Science, University of Arizona, Tucson, AZ, United States
Introduction: Vascular and mitochondrial dysfunction are well-established consequences of multiple central nervous system (CNS) disorders, including neurodegenerative diseases and traumatic injuries. We previously reported that 5-hydroxytryptamine 1F receptor (5-HT1FR) agonism induces mitochondrial biogenesis (MB) in multiple organ systems, including the CNS.
Methods: Lasmiditan is a selective 5-HT1FR agonist that is FDA-approved for the treatment of migraines. We have recently shown that lasmiditan treatment induces MB, promotes vascular recovery and improves locomotor function in a mouse model of spinal cord injury (SCI). To investigate the mechanism of this effect, primary cerebral microvascular endothelial cells from C57bl/6 mice (mBMEC) were used.
Results: Lasmiditan treatment increased the maximal oxygen consumption rate, mitochondrial proteins and mitochondrial density in mBMEC, indicative of MB induction. Lasmiditan also enhanced endothelial cell migration and tube formation, key components of angiogenesis. Trans-endothelial electrical resistance (TEER) and tight junction protein expression, including claudin-5, were also increased with lasmiditan, suggesting improved barrier function. Finally, lasmiditan treatment decreased phosphorylated VE-Cadherin and induced activation of the Akt-FoxO1 pathway, which decreases FoxO1-mediated inhibition of claudin-5 transcription.
Discussion: These data demonstrate that lasmiditan induces MB and enhances endothelial cell function, likely via the VE-Cadherin-Akt-FoxO1-claudin-5 signaling axis. Given the importance of mitochondrial and vascular dysfunction in neuropathologies, 5-HT1FR agonism may have broad therapeutic potential to address multiple facets of disease progression by promoting MB and vascular recovery.
1 Introduction
Disruption of mitochondrial function is implicated in multiple central nervous system (CNS) pathologies, including both acute traumas and neurodegenerative diseases (Simmons et al., 2020b). Because of this, there is continued interest in therapeutic interventions targeting mitochondrial function and homeostasis. The therapeutic potential of inducing mitochondrial biogenesis (MB), the generation of new functioning mitochondria (Cameron et al., 2016; Gibbs et al., 2018c), in the treatment of CNS pathologies remains a promising, though underexplored strategy (Scholpa and Schnellmann, 2017; Simmons et al., 2020b, 2022). Multiple reports demonstrate the efficacy of MB-related therapies in restoring mitochondrial homeostasis and improving outcomes across preclinical models of both traumatic injuries such as spinal cord injury (SCI), stroke, and traumatic brain injury (TBI), as well as neurodegenerative disorders including Alzheimer’s and Parkinson’s diseases (Gibbs et al., 2018c; Simmons et al., 2020b).
Blood–CNS barriers are comprised of endothelial cells, astrocytes, and pericytes, which work concurrently to form a selectively permeable barrier that both prevents blood-borne pathogens and toxins from entering the CNS and aids in maintaining CNS homeostasis (Daneman and Prat, 2015). Traumatic CNS injuries often result in catastrophic destruction of the blood–brain barrier (BBB) and/or blood–spinal cord barrier (BSCB), leading to enhanced permeability and neuroinflammation that further propagates injury progression (Kumar et al., 2018; Walker et al., 2019). Additionally, evidence of endothelial dysfunction and BBB impairment, including decreased tight junction protein expression, has been identified in patients with Alzheimer’s disease (Yamazaki et al., 2019; Wu et al., 2021), Parkinson’s disease (Gray and Woulfe, 2015), Huntington’s disease (Drouin-Ouellet et al., 2015), and amyotrophic lateral sclerosis (ALS), which is also characterized by decreased BSCB integrity (Henkel et al., 2009; Garbuzova-Davis et al., 2012). Furthermore, blood–CNS barrier breakdown occurs naturally with aging, which, while not associated with negative consequences itself, may become more disadvantageous when exposed to a secondary offense, such as inflammation (Knox et al., 2022).
Restoration of blood–CNS barrier integrity has been associated with improved outcomes in both neurodegenerative disorders and traumatic injuries (Sweeney et al., 2018; van Vliet et al., 2020; Jin et al., 2021; Alarcan et al., 2022; Sousa et al., 2023). Reversing or preventing the loss of tight junctions could aid in this restoration and ultimately ameliorate disease symptoms and/or progression (Li et al., 2021). Angiogenesis has been correlated with enhanced recovery following stroke (Yang and Torbey, 2020), TBI (Chodobski et al., 2011), and SCI (Tsivelekas et al., 2022). Importantly, published studies demonstrate a positive correlation between MB induction and angiogenesis (Arany et al., 2008; Saint-Geniez et al., 2013; Thom et al., 2014). We showed that 5-HT1F receptor (5-HT1FR) agonism induces MB in multiple organ systems, including the CNS (Garrett et al., 2014; Gibbs et al., 2018a; Scholpa et al., 2018; Dupre et al., 2019; Simmons et al., 2020a, 2021), and promotes angiogenesis in glomerular endothelial cells in vitro (Dupre et al., 2019). Treatment with lasmiditan, a highly selective and potent 5-HT1FR agonist FDA-approved for the treatment of migraines, not only induces MB in the spinal cord, but also increases tight junction protein expression, improves BSCB integrity, and enhances locomotor recovery in a mouse model of SCI (Simmons et al., 2021). Despite these promising data, the mechanism of this effect remains unknown. To begin to address this gap in knowledge, we assessed the effects of lasmiditan on MB and endothelial cell function in primary brain microvascular endothelial cells (mBMEC).
2 Methods
2.1 Cell culture and reagents
Primary C57bl/6 mouse cerebral endothelial cells (mBMEC) were purchased from Cell Biologics Inc. (C57-6023; Chicago, IL). mBMEC were cultured on 0.1% gelatin-coated flasks using endothelial cell medium (M1168, Cell Biologics, Inc.) with growth factor supplement additives (Cell Biologics, Inc.) and 5% (v/v) fetal bovine serum (Cell Biologics, Inc.). mBMEC were used at passages 2–5, and at least two different lots were used for each experiment. Mouse cerebellar astrocytes were purchased from ATCC (C8-D1A; Manassas, VA) and cultured in DMEM with 10% fetal bovine serum for trans-endothelial electrical resistance (TEER) experiments. Lasmiditan was purchased from Tocris (Ellisville, MO).
2.2 PCR
DNA was isolated from mBMEC, wild-type C57bl/6NJ spinal cord tissue, and 5-HT1F receptor knockout mouse (B6N(Cg)-Htr1ftm1.1(KOMP)Vlcg/J; Jackson Laboratories) spinal cord tissue using the Qiagen DNeasy Blood and Tissue Kit (Valencia, CA). To determine the presence of the 5-HT1FR gene, DNA was amplified using Promega 2x PCR Master Mix (Promega, Madison, WI) in accordance with the manufacturer’s protocols. Amplified DNA was separated on a 1.5% agarose gel and visualized by ethidium bromide fluorescence. Primers used were sense: 5′-GCCGTGATGATGAGTGTGTC-3′ and antisense: 5′-ACTATCCGACTCGCTTGTCT-′3.
2.3 Analysis of oxygen consumption rate
The oxygen consumption rate (OCR) of mBMECs was measured using the Seahorse Bioscience XF-96 Extracellular Flux Analyzer as previously described (Agilent, Santa Clara, CA) (Beeson et al., 2010). Cells were plated at a density of 1×104 cells/well in 96-well plates. Each plate was treated with either vehicle (DMSO; <0.5%) or lasmiditan (0-100 nM) for 48 h in fetal bovine serum-free media. Basal OCR was measured before injection of carbonyl cyanide 4-(trifluoromethoxy) phenylhydrazone (FCCP; 2 μM; Sigma-Aldrich, St. Louis, MO) to measure uncoupled OCR, a marker of functional MB (Beeson et al., 2010).
2.4 Transmission electron microscopy
mBMECs were plated at a density of 5×104 on D35 cell culture dishes and treated with vehicle or 3 nM lasmiditan for 24 h. mBMECs were then fixed and sectioned for transmission electron microscopy (TEM) as previously described (Dupre et al., 2019; Scholpa et al., 2019). Images were viewed using the FEI Tecnai Spirit microscope (FEI, Hillsboro, OR) operated at 100 kV and captured using an AMT 4 Mpixel camera (Advanced Microscopy Techniques, Woburn, MA) at a magnification of 16,500X. Mitochondrial count and morphology were analyzed using the analyze particles plug-in in ImageJ FIJI. In all cases, four to five images were analyzed blindly per sample.
2.5 Protein isolation and immunoblot analysis
Protein was extracted using RIPA buffer with protease inhibitor cocktail (1:100), 1 mM sodium fluoride, and 1 mM sodium orthovanadate (Sigma-Aldrich, St. Louis, MO) as described previously (Dupre et al., 2019; Scholpa et al., 2019). Protein was quantified using a bicinchoninic acid assay, and up to 10 μg of protein was separated via electrophoresis using 4–15% SDS-PAGE gels, then transferred to nitrocellulose membranes (Bio-Rad, Hercules, CA). Membranes were blocked in 5% milk or BSA in TBST and incubated overnight with primary antibodies with constant agitation at 4°C. Membranes were incubated with the appropriate horseradish peroxidase-conjugated secondary antibody and visualized using chemiluminescence (Thermo Scientific, Waltham, MA) on a GE ImageQuant LAS-4000 (GE Life Sciences, Pittsburg, PA). Optical density was determined using Image Studio Lite software. Primary antibodies used were PGC-1α (1 μg/mL; Abcam ab191838, Cambridge, MA), ATPSB (1 μg/mL; Abcam ab14730), CD31 (0.523 μg/mL; Abcam ab222783), claudin-5 (0.5 μg/mL; Invitrogen 35–2,500, Carlsbad, CA), ZO-1 (0.22 μg/mL; Abcam ab96587), occludin (0.71 μg/mL; Abcam ab216327), VE-cadherin (0.238 μg/mL; Abcam ab205336), p-VE-cadherin (Tyr658; 0.2 μg/mL; Invitrogen 44-1144G), p-Akt (Ser473; 0.091 μg/mL; Cell Signaling 9,271, Danvers, MA), Akt (0.031 μg/mL; Cell Signaling 9,272), p-eNOS (Ser1177; 0.485 μg/mL; Abcam ab215717), eNOS (0.25 μg/mL; Abcam ab76198), Nop-FoxO1 (Ser256; 0.107 μg/mL; Cell Signaling 9,461 s), FoxO1 (0.088 μg/mL, Cell Signaling 2,880 s), and tubulin (0.065 μg/mL, Abcam ab52866).
2.6 Cellular migration assay
Migration was assessed and analyzed as previously described (Liang et al., 2007; Dupre et al., 2019) via MRI wound healing plug-in in ImageJ FIJI. Briefly, mBMEC were plated at a density of 5×104 on D35 cell culture dishes and grown to confluence. Cells were then treated with either vehicle or 3 nM lasmiditan for 24 h in serum-free media. Scratches were applied using a 10-μL pipette tip, and media were replaced with complete media containing lasmiditan or vehicle. Images of five randomly selected areas of the scratch were taken for each treatment at each time point. The percent migration was determined by the following formula: % area of migration 6 h post-scratch = (scratch area 0 h post-scratch − scratch area 6 h post-scratch) / (scratch area 0 h post-scratch) * 100.
2.7 Tube formation assay
mBMEC were plated at a density of 2.2×106 on D100 cell culture dishes pretreated with Gibco attachment factor and grown to approximately 80% confluence in endothelial cell media. Cells were then treated with either vehicle or 3 nM lasmiditan for 24 h. After 24 h, mBMEC were collected via Accutase dissociation and centrifuged at 300 x g for 10 min at 4°C to form a pellet. Cells were resuspended in endothelial cell media containing either vehicle or lasmiditan, counted, and diluted to 1.5×105 cells/mL, and 50 μL of cell suspension was added to an Ibidi (Fitchburg, WI) μ-Slide 15 Well 3D cell culture slide precoated with 10 μL of Corning reduced growth factor phenol red free Matrigel solution (Corning, NY), as per manufacturer’s recommended protocol. mBMEC were allowed to grow in Matrigel-coated wells for 24 h, and wells were imaged with a 10x LWD objective on an EVOS M5000 microscope under phase contrast with a light diffuser insert to help prevent uneven illumination.
Ten images from each lot/passage were analyzed using Ibidi FastTrack AI software (provided by MetaVi Labs).
2.8 TEER
mBMEC were plated at a density of 1.8×105 cells/well on polyester-coated Transwell inserts (CLS3460; Corning) of 0.4 μm pore size in endothelial cell media and grown to confluence. For monoculture experiments, membranes were then placed in 12-well plates containing endothelial cell media and treated with 3 nM lasmiditan or vehicle. For co-culture experiments, 2×105 astrocytes/well were plated and grown 48 h in 12-well plates with DMEM. Inserts containing mBMEC were then added to the astrocyte-containing wells and treated with 3 nM lasmiditan or vehicle in endothelial cell media. TEER was assessed using an EVOM2 Epithelial Voltohmmeter with chopsticks electrode set (World Precision Instruments) after 24 h (day 1) and again after 48 h (day 2). Net resistance (Ω) was determined by subtracting that of media-only blank wells, and TEER was calculated based on the area of the well as Ω·cm2 (Srinivasan et al., 2015).
2.9 Statistical analysis
Primary mBMEC isolated from a single passage represents an individual experiment (n = 1) and n = 5-6/group was used for each experiment. Data were normalized to respective vehicle-treated samples. Differences between two groups were analyzed using a two-tailed paired t-test, while that of three or more groups was analyzed using a one-way ANOVA followed by Tukey’s post-hoc test. In all cases, GraphPad Prism software (La Jolla, CA) was used and a p < 0.05 was considered statistically different between mean values.
3 Results
3.1 5-HT1FR agonism induces mitochondrial biogenesis in mBMEC
Prior to experimentation, expression of the 5-HT1FR was confirmed in mBMEC via PCR (Figure 1A). FCCP-uncoupled oxygen consumption rate (OCR) is a marker of maximal ETC activity and potential MB (Beeson et al., 2010; Wills et al., 2012; Garrett et al., 2014; Cleveland et al., 2020; Simmons et al., 2021). mBMEC were exposed to various concentrations of lasmiditan for 48 h and then assessed for FCCP-OCR. Lasmiditan had a concentration-dependent effect on FCCP-OCR, with a ~ 25% increase with a 3 nM, the lowest effective concentration (Figure 1B). Based on this, 3 nM lasmiditan was used for all experiments. Transmission electron microscopy (TEM) was used to assess mitochondrial content in mBMEC (Figure 1C). Following lasmiditan exposure, mBMEC exhibited increased mitochondrial number and area (Figure 1D) per field compared to vehicle controls, further indicating MB. Immunoblot analysis revealed increased PGC-1α, the master regulator of MB, and ATP synthase β (ATPSB), a subunit of the ETC, with lasmiditan treatment compared to vehicle (Figure 1E).
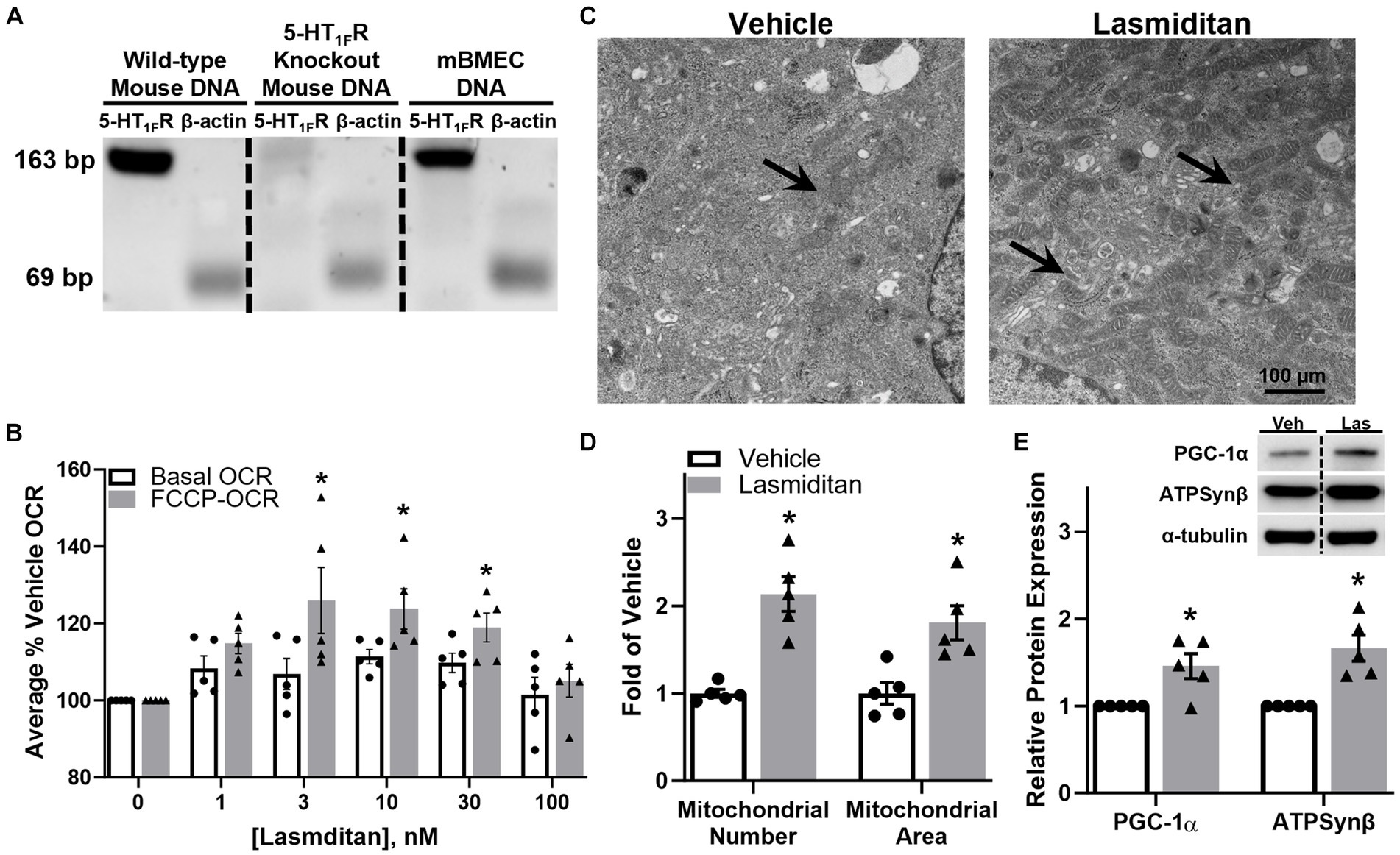
Figure 1. Effect of lasmiditan on mitochondrial biogenesis in mBMEC. The presence of the 5-HT1F receptor was confirmed in mBMEC via PCR (A). mBMEC were treated with either vehicle or lasmiditan (1–300 nM) for 48 h. Uncoupled mitochondrial oxygen consumption rates (FCCP-OCR) were measured using a Seahorse Bioscience XF-96 analyzer (B). Based on these data, mBMEC were treated with vehicle or 3 nM lasmiditan for 72 h and either assessed via TEM ((C); scale bar = 100 μm) to determine mitochondrial number and area (D) or analyzed for MB-related proteins (E). Data represent n = 5 and are expressed as mean ± SEM ((B) *p < 0.05 compared to vehicle using one-way ANOVA followed by Dunnett’s post-hoc test; (D,E) *p < 0.05 compared to vehicle using a paired t-test).
3.2 5-HT1FR agonism stimulates migration in mBMEC
A wound healing model was used to determine whether lasmiditan stimulates migration in mBMEC (Liang et al., 2007) (Figure 2A). Cells were grown to confluence and treated with vehicle or 3 nM lasmiditan for 24 h in serum-free media, after which a scratch was applied (0 h). Lasmiditan treatment decreased the area of damage by ~60% compared to vehicle control by 6 h (Figure 2B), equating to a 2-fold increase in cell migration (Figure 2C). Importantly, no difference in wound area was observed upon injury.
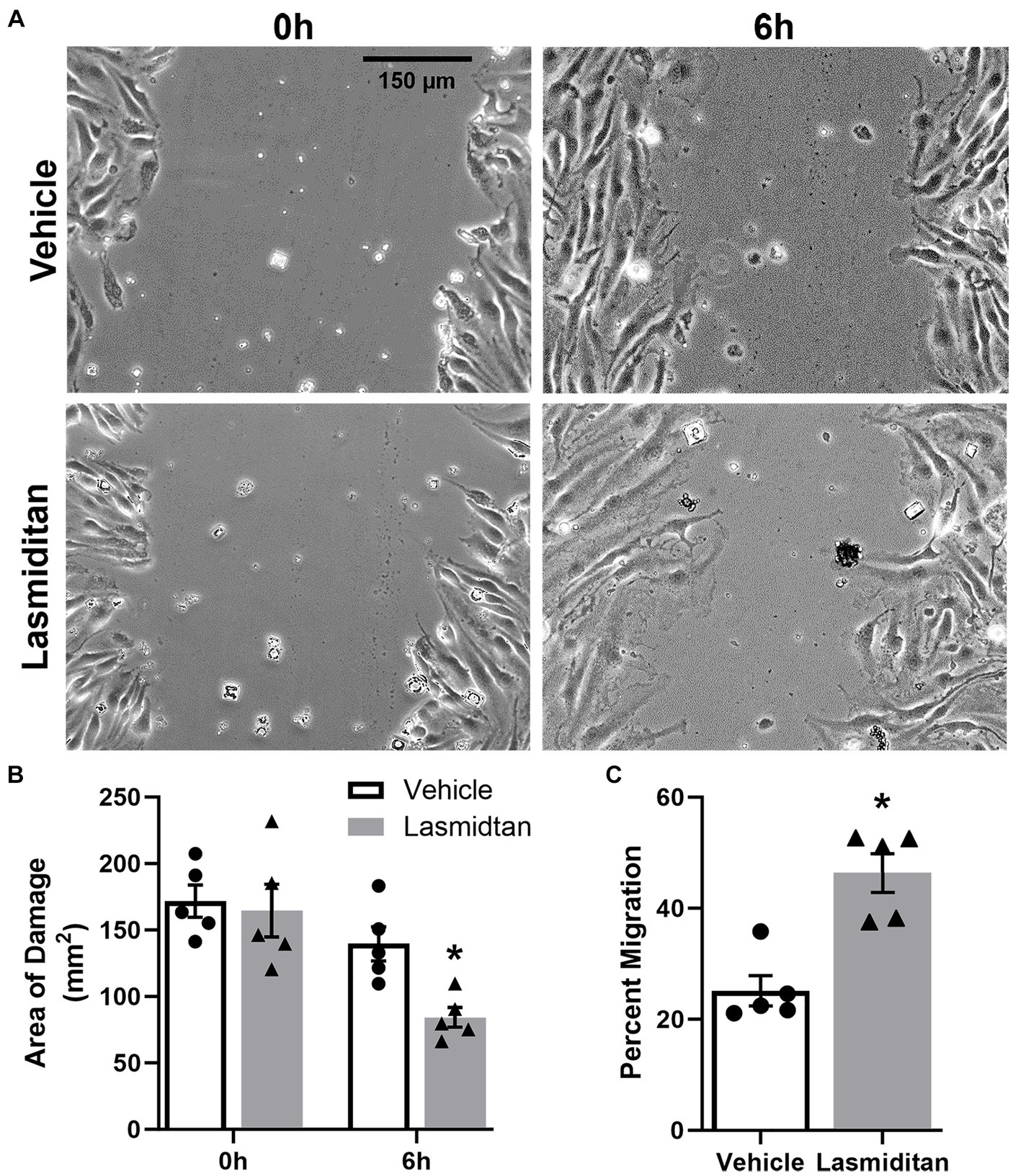
Figure 2. Effect of lasmiditan on cell migration in mBMEC. mBMEC were grown to confluence and then treated with vehicle or 3 nM lasmiditan for 24 h in serum-free media. The cell monolayer was scratched with a 10-μL pipette tip, and serum-free media were replaced with complete media containing lasmiditan or vehicle. mBMEC were imaged at 0 h and 6 h post-injury ((A); scale bar = 150 μm) to determine the area of damage (B) and the percent of migration (C). Data represent n = 5 and are expressed as mean ± SEM (*p < 0.05 compared to vehicle using a paired t-test).
3.3 5-HT1FR agonism stimulates tube formation in mBMEC
A Matrigel-based tube formation assay was used to assess the angiogenic potential of lasmiditan in mBMEC (Arnaoutova and Kleinman, 2010; Almeria et al., 2019; Hunter et al., 2022) (Figure 3A). Following 24 h of treatment, mBMEC were added to Matrigel-coated wells (0 h) and allowed to grow for an additional 24 h in the continued presence of vehicle or 3 nM lasmiditan. Lasmiditan treatment increased loop count 3-fold (Figure 3B), total tube length 1.5-fold (Figure 3C), and branch count 2-fold (Figure 3D) in mBMEC. Figures 3E–G depict the loop count, total tube length, and branch count for all images collected.
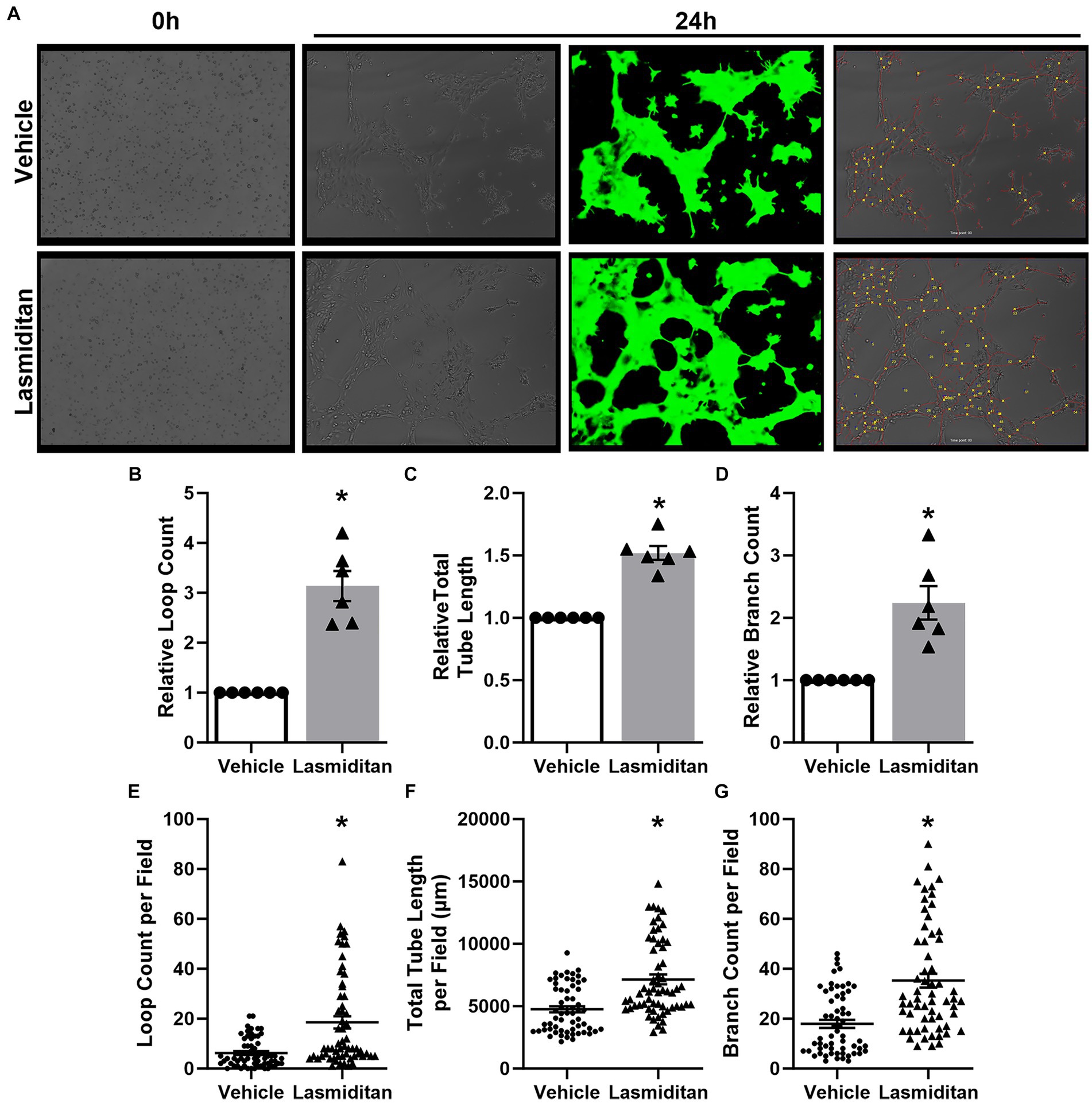
Figure 3. Effect of lasmiditan on tube formation in mBMEC. mBMEC were grown to ~80% confluence and then treated with vehicle or 3 nM lasmiditan for 24 h. Cells were then collected and added to wells of an ibdi μ-Slide 15 Well 3D cell culture slide precoated with 10 μL of Corning reduced growth factor phenol red free Matrigel solution at a density of 7.5×103 cells/well (0 h) where they were allowed to grow for an additional 24 h in the presence of vehicle or lasmiditan (A). Ten images per lot/passage were collected, and Ibidi FastTrack AI software (provided by MetaVi Labs) was used to apply a pseudo-casein tracking mask (green) and to determine loop count (B,E), total tube length (C,F), and branch count (D,G). For (B–D), data are normalized to the respective vehicles and represent n = 6/group. (E–G) Depict raw data collected from each image, representing 60 images per group. Data are expressed as mean ± SEM (*p < 0.05 compared to vehicle using a paired t-test).
3.4 5-HT1FR agonism increases electrical resistance in mBMEC
Trans-endothelial electrical resistance (TEER) was used to examine the effect of lasmiditan on mBMEC barrier function with and without astrocyte co-culture. When grown in monoculture, mBMEC TEER increased nearly 2-fold when treated with lasmiditan compared to vehicle on both day 1 (43 v 24 Ω·cm2) and day 2 (58 v 31 Ω·cm2) (Figure 4A). Similar results were seen in the presence of astrocytes, where lasmiditan treatment increased mBMEC TEER from 33 to 57 Ω·cm2 on day 1 and from 45 to 78 Ω·cm2 on day 2 (Figure 4B). Regardless of the presence or absence of astrocytes or lasmiditan, mBMEC TEER increased from day 1 to day 2. As expected, TEER values were inherently higher with astrocyte co-culture (Abbott, 2002); however, these data suggest that astrocytes have no effect on the lasmiditan-induced increase in mBMEC barrier integrity. Protein was collected from the mBMEC that had undergone TEER assessment in the presence of astrocytes (Figure 4B), and the expression of tight junction proteins was assessed. Claudin-5 and occludin increased 2- and 1.5-fold with lasmiditan treatment, respectively, while no effect was observed with ZO-1 (Figure 4C).
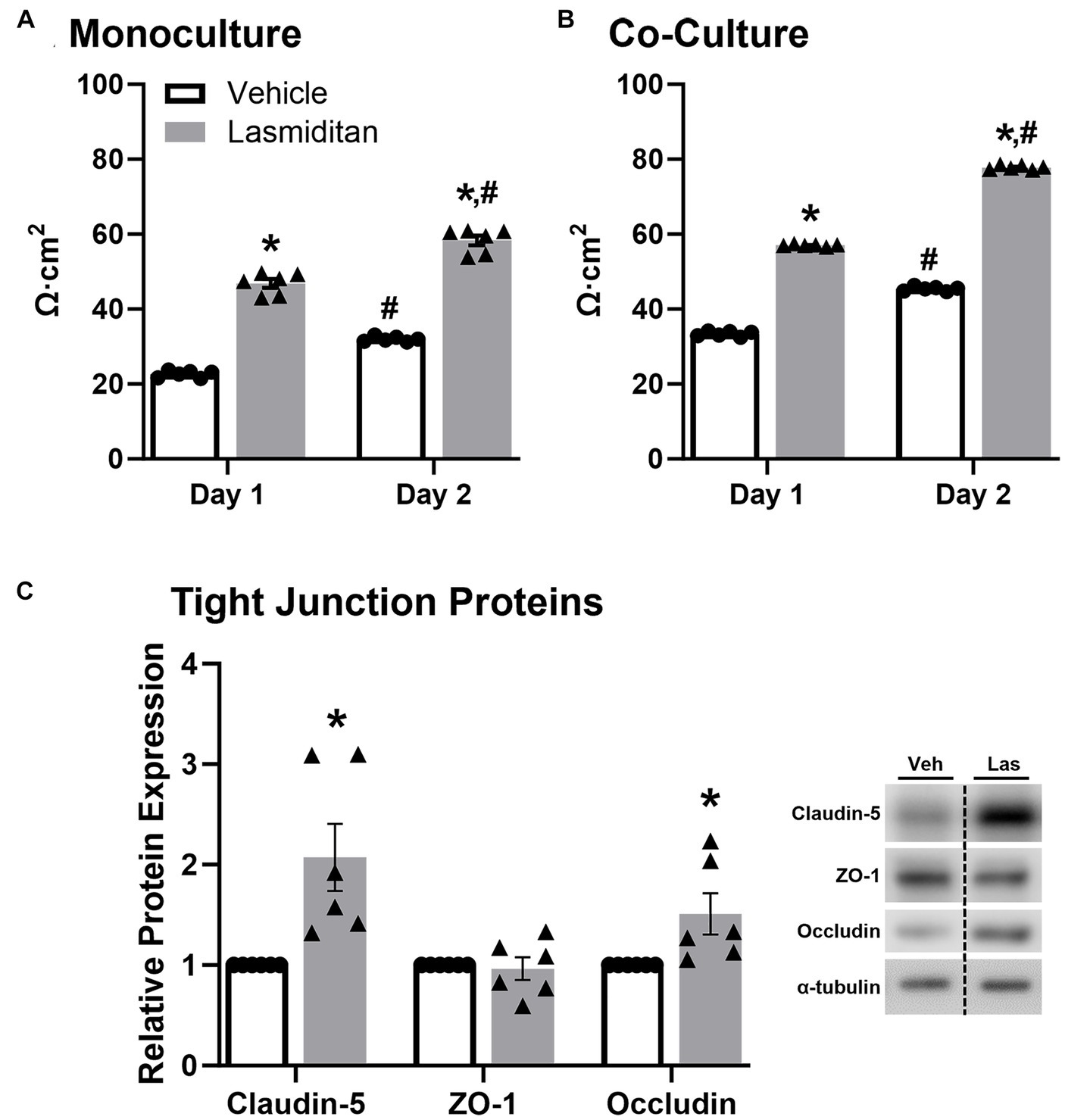
Figure 4. Effect of lasmiditan on trans-endothelial electrical resistance in mBMEC. mBMEC were plated on polyester-coated transwell inserts at a density of 1.8×105 and grown for 48 h. Membranes were placed in 12-well plates containing either endothelial cell media (A) or astrocytes (B) and then treated with vehicle or 3 nM lasmiditan. TEER was measured after 24 h (day 1) and 48 h (day 2). Protein was isolated from the mBMEC from the co-culture TEER condition, and the expression of tight junction proteins was assessed (C). Data represent n = 6 and are expressed as mean ± SEM (*p < 0.05 compared to vehicle, #p < 0.05 compared to day 1 using a paired t-test).
3.5 5-HT1FR agonism decreases VE-cadherin phosphorylation and activates the VE-cadherin–Akt–FoxO1 signaling axis in mBMEC
To begin to discern the pathway of the effect of lasmiditan on endothelial cell function in mBMEC, cells were exposed to lasmiditan or vehicle for 48 h, and protein was isolated for immunoblot analysis. Vascular markers CD31 and VE-cadherin were increased in the lasmiditan-treated compared to vehicle-treated cells (Figure 5A). Additionally, phosphorylation of VE-cadherin at Tyr658, which has been found to disrupt VE-cadherin-mediated junctions (Potter et al., 2005), was decreased with lasmiditan treatment. VE-cadherin can directly contribute to the expression of claudin-5, in that adhesion triggers phosphorylation of Akt and, subsequently, phosphorylation of FoxO1, which prevents its translocation into the nucleus where it can repress claudin-5 transcription (Gavard and Gutkind, 2008). Importantly, lasmiditan-treated mBMEC exhibited increased Akt, eNOS, and FoxO1 phosphorylation compared to vehicle-treated cells (Figure 5B).
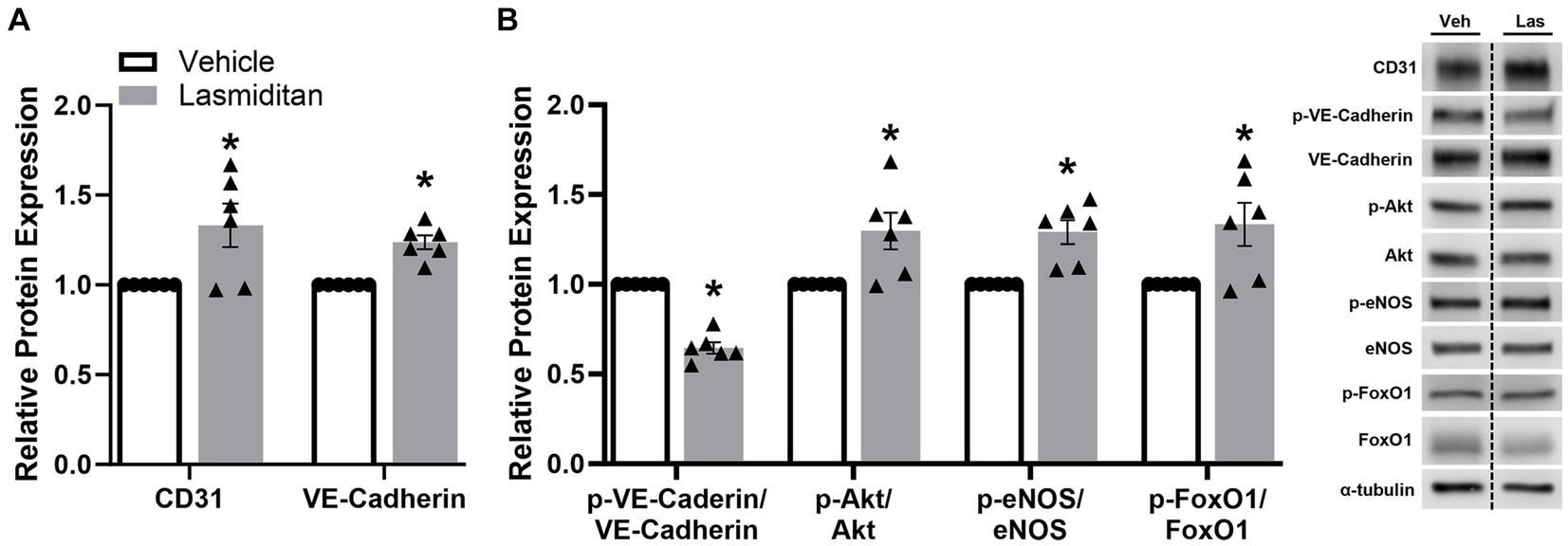
Figure 5. Effect of lasmiditan on the VE-cadherin–Akt–FoxO1 signaling axis in mBMEC. mBMEC were plated on 6-well plates at 3×105 cells/well, grown for 24 h, and then treated with vehicle or 3 nM lasmiditan for 48 h. Cells were collected and the expression of endothelial cell-related proteins (A) and members of the VE-Cadherin-Akt-FoxO1 signaling axis (B) were assessed. Data represent n = 6 and are expressed as mean ± SEM (*p < 0.05 compared to vehicle using a paired t-test).
4 Discussion
Disruption of mitochondrial quality control mechanisms is implicated in the onset and progression of many CNS disorders. In addition to impaired cellular processes and the initiation of cell death mechanisms, mitochondrial dysfunction also impacts vascular integrity, further propagating disease (Vlodavsky et al., 2017). Therefore, targeting the restoration of mitochondrial homeostasis and thereby improving vascular integrity could prove a valuable strategy to promote recovery from neuropathologies. Existing data demonstrate that restoring mitochondrial homeostasis via pharmacological stimulation of MB can promote recovery after CNS injury or neurodegenerative disease (Wang et al., 2015; Li et al., 2016; Golpich et al., 2017; Wang et al., 2019). Our group previously reported that agonism of the 5-HT1FR is a potent inducer of MB peripherally and centrally (Gibbs et al., 2018a; Scholpa et al., 2018; Dupre et al., 2019; Simmons et al., 2020a, 2021; Hurtado et al., 2023a,b). Furthermore, pharmacological induction of MB via 5-HT1FR agonism improved recovery following traumatic SCI and in a mouse model of Parkinson’s disease (Scholpa et al., 2018; Simmons et al., 2020a, 2021). Here, we report treatment with the FDA-approved selective 5-HT1FR agonist lasmiditan induces MB, increases migration, promotes angiogenesis, and enhances vascular integrity in CNS endothelial cells.
While endothelial cells primarily utilize aerobic glycolysis as their source of energy production (Caja and Enríquez, 2017), we observed that lasmiditan stimulated mBMEC oxidative respiration as evidenced by an increase in FCCP-OCR. Similar to observations reported in renal endothelial cells (Dupre et al., 2019), mBMEC treated with lasmiditan exhibited increased maximal mitochondrial respiration, mitochondrial density and area, and PGC-1α, the master regulator of MB. Reports demonstrate that pharmacological activation of MB promotes angiogenesis and restoration of barrier integrity, associated with improved pathological and behavioral outcomes following CNS trauma (Simmons et al., 2020b). Additionally, PGC-1α is documented to stimulate angiogenesis in endothelial cells (Mammoto et al., 2018). In support of this, lasmiditan-treated mBMEC exhibited enhanced tube formation and wound closure following scratch injury, in vitro models established to mimic key components of angiogenesis in vivo (Lamalice et al., 2007; Liang et al., 2007; Arnaoutova and Kleinman, 2010). Blood–CNS barrier integrity and permeability can be evaluated by measuring the electrical resistance of a cell monolayer (Srinivasan et al., 2015). We report increased TEER in mBMEC treated with lasmiditan, with and without co-culture with astrocytes, suggesting enhanced barrier integrity and further supporting increased endothelial cell function with 5-HT1FR agonism.
To maintain vascular integrity, it is critical for new and recovering vessels of blood–CNS barriers to stabilize after angiogenesis. Cell adhesion and tight junction proteins play integral roles in this stability. We observed increased tight junction protein expression as well as increased VE-cadherin, a key cell adhesion molecule responsible for maintaining endothelial barrier function and angiogenesis (Giannotta et al., 2013), in mBMEC treated with lasmiditan. Additionally, lasmiditan treatment decreased phosphorylation of VE-cadherin at Tyr658, which has been shown to decrease adhesion and result in VE-cadherin internalization and, ultimately, junctional breakdown (Schimmel and Gordon, 2018). Therefore, in addition to increased VE-cadherin, the observed decrease in phosphorylation likely results in enhanced VE-cadherin adhesion.
Activation of the PI3K-Akt-eNOS pathway is considered a central factor in angiogenesis and cell migration (Lamalice et al., 2007), while also having implications in MB (Gibbs et al., 2018b,c; Simmons et al., 2020b). VE-cadherin adhesion triggers activation of this pathway, phosphorylation of Akt, and, subsequently, phosphorylation of FoxO1. Phosphorylation prevents the translocation of FoxO1 from the cytoplasm to the nucleus, where it can repress claudin-5 transcription (Gavard and Gutkind, 2008; Farhan et al., 2017). Therefore, reduced phosphorylation of VE-cadherin, and thereby increased adhesion and continued activation of Akt-eNOS-FoxO1, likely contributes to the increased levels of claudin-5 and improved barrier integrity observed in lasmiditan-treated mBMECs. Interestingly, phosphorylation of FoxO1 has also been shown to increase HIF-1 and VEGF expression and promote angiogenesis in some cancer models (Farhan et al., 2017), further implicating its involvement in endothelial cell function.
The results presented here not only corroborate existing data detailing a positive relationship between MB and angiogenesis, but also demonstrate 5-HT1FR agonist-induced enhancement of endothelial cell function, including angiogenesis, migration, and barrier integrity. Furthermore, these data suggest that this effect is likely due in part to increased VE-cadherin adhesion leading to FoxO1 phosphorylation and increased claudin-5 transcription. These findings support lasmiditan and 5-HT1FR agonism as promising potential therapeutic strategies for the treatment of various CNS pathologies such as SCI, TBI, and neurodegenerative diseases, through the promotion of MB, angiogenesis, and vascular recovery.
Data availability statement
The raw data supporting the conclusions of this article will be made available by the authors, without undue reservation.
Ethics statement
Ethical approval was not required for the studies on animals in accordance with the local legislation and institutional requirements because only commercially available established cell lines were used.
Author contributions
NS: Conceptualization, Formal analysis, Investigation, Methodology, Supervision, Writing – original draft, Writing – review & editing, Funding acquisition, Project administration, Visualization. ES: Conceptualization, Investigation, Methodology, Writing – original draft, Writing – review & editing, Formal analysis, Funding acquisition, Project administration, Visualization. AT: Investigation, Methodology, Writing – original draft, Writing – review & editing, Formal analysis, Funding acquisition, Visualization. SC: Investigation, Writing – original draft, Writing – review & editing. RS: Conceptualization, Funding acquisition, Project administration, Resources, Supervision, Writing – original draft, Writing – review & editing.
Funding
The author(s) declare financial support was received for the research, authorship, and/or publication of this article. This study was supported by the Biomedical Laboratory Research and Development Program of the Department of Veterans Affairs: BX: 004868 (RS), BX: 005218 (NS); the National Institute of Neurological Disorders and Stroke F31 NS115413 (ES); and the National Institute of Environment Health Sciences T32-ES007091-40 (AT).
Acknowledgments
We would also like to thank Dr. William A. Day at the University of Arizona, Imaging Cores—Life Sciences North for his technical assistance involving the TEM experiments.
Conflict of interest
The authors declare that the research was conducted in the absence of any commercial or financial relationships that could be construed as a potential conflict of interest.
Publisher’s note
All claims expressed in this article are solely those of the authors and do not necessarily represent those of their affiliated organizations, or those of the publisher, the editors and the reviewers. Any product that may be evaluated in this article, or claim that may be made by its manufacturer, is not guaranteed or endorsed by the publisher.
Author disclaimer
The contents do not represent the views of the U.S. Department of Veterans Affairs or the United States Government.
References
Abbott, N. J. (2002). Astrocyte-endothelial interactions and blood-brain barrier permeability. J. Anat. 200, 629–638. doi: 10.1046/j.1469-7580.2002.00064.x
Alarcan, H., Al Ojaimi, Y., Lanznaster, D., Escoffre, J. M., Corcia, P., Vourc’h, P., et al. (2022). Taking advantages of blood-brain or spinal cord barrier alterations or restoring them to optimize therapy in ALS? J. Pers. Med. 12:1071. doi: 10.3390/jpm12071071
Almeria, C., Weiss, R., Roy, M., Tripisciano, C., Kasper, C., Weber, V., et al. (2019). Hypoxia conditioned mesenchymal stem cell-derived extracellular vesicles induce increased vascular tube formation in vitro. Front. Bioeng. Biotechnol. 7:292. doi: 10.3389/fbioe.2019.00292
Arany, Z., Foo, S. Y., Ma, Y., Ruas, J. L., Bommi-Reddy, A., Girnun, G., et al. (2008). HIF-independent regulation of VEGF and angiogenesis by the transcriptional coactivator PGC-1alpha. Nature 451, 1008–1012. doi: 10.1038/nature06613
Arnaoutova, I., and Kleinman, H. K. (2010). In vitro angiogenesis: endothelial cell tube formation on gelled basement membrane extract. Nat. Protoc. 5, 628–635. doi: 10.1038/nprot.2010.6
Beeson, C. C., Beeson, G. C., and Schnellmann, R. G. (2010). A high-throughput respirometric assay for mitochondrial biogenesis and toxicity. Anal. Biochem. 404, 75–81. doi: 10.1016/j.ab.2010.04.040
Caja, S., and Enríquez, J. A. (2017). Mitochondria in endothelial cells: sensors and integrators of environmental cues. Redox Biol. 12, 821–827. doi: 10.1016/j.redox.2017.04.021
Cameron, R. B., Beeson, C. C., and Schnellmann, R. G. (2016). Development of therapeutics that induce mitochondrial biogenesis for the treatment of acute and chronic degenerative diseases. J. Med. Chem. 59, 10411–10434. doi: 10.1021/acs.jmedchem.6b00669
Chodobski, A., Zink, B. J., and Szmydynger-Chodobska, J. (2011). Blood-brain barrier pathophysiology in traumatic brain injury. Transl. Stroke Res. 2, 492–516. doi: 10.1007/s12975-011-0125-x
Cleveland, K. H., Brosius, F. C. 3rd, and Schnellmann, R. G. (2020). Regulation of mitochondrial dynamics and energetics in the diabetic renal proximal tubule by the β(2)-adrenergic receptor agonist formoterol. Am. J. Physiol. Renal Physiol. 319, F773–f779. doi: 10.1152/ajprenal.00427.2020
Daneman, R., and Prat, A. (2015). The blood-brain barrier. Cold Spring Harb. Perspect. Biol. 7:a020412. doi: 10.1101/cshperspect.a020412
Drouin-Ouellet, J., Sawiak, S. J., Cisbani, G., Lagacé, M., Kuan, W. L., Saint-Pierre, M., et al. (2015). Cerebrovascular and blood-brain barrier impairments in Huntington’s disease: potential implications for its pathophysiology. Ann. Neurol. 78, 160–177. doi: 10.1002/ana.24406
Dupre, T. V., Jenkins, D. P., Muise-Helmericks, R. C., and Schnellmann, R. G. (2019). The 5-hydroxytryptamine receptor 1F stimulates mitochondrial biogenesis and angiogenesis in endothelial cells. Biochem. Pharmacol. 169:113644. doi: 10.1016/j.bcp.2019.113644
Farhan, M., Wang, H., Gaur, U., Little, P. J., Xu, J., and Zheng, W. (2017). FOXO signaling pathways as therapeutic targets in Cancer. Int. J. Biol. Sci. 13, 815–827. doi: 10.7150/ijbs.20052
Garbuzova-Davis, S., Hernandez-Ontiveros, D. G., Rodrigues, M. C., Haller, E., Frisina-Deyo, A., Mirtyl, S., et al. (2012). Impaired blood-brain/spinal cord barrier in ALS patients. Brain Res. 1469, 114–128. doi: 10.1016/j.brainres.2012.05.056
Garrett, S. M., Whitaker, R. M., Beeson, C. C., and Schnellmann, R. G. (2014). Agonism of the 5-hydroxytryptamine 1F receptor promotes mitochondrial biogenesis and recovery from acute kidney injury. J. Pharmacol. Exp. Ther. 350, 257–264. doi: 10.1124/jpet.114.214700
Gavard, J., and Gutkind, J. S. (2008). VE-cadherin and claudin-5: it takes two to tango. Nat. Cell Biol. 10, 883–885. doi: 10.1038/ncb0808-883
Giannotta, M., Trani, M., and Dejana, E. (2013). VE-cadherin and endothelial adherens junctions: active guardians of vascular integrity. Dev. Cell 26, 441–454. doi: 10.1016/j.devcel.2013.08.020
Gibbs, W. S., Collier, J. B., Morris, M., Beeson, C. C., Megyesi, J., and Schnellmann, R. G. (2018a). 5-HT1F receptor regulates mitochondrial homeostasis and its loss potentiates acute kidney injury and impairs renal recovery. Am. J. Physiol. Renal Physiol. 315, F1119–f1128. doi: 10.1152/ajprenal.00077.2018
Gibbs, W. S., Garrett, S. M., Beeson, C. C., and Schnellmann, R. G. (2018b). Identification of dual mechanisms mediating 5-hydroxytryptamine receptor 1F-induced mitochondrial biogenesis. Am. J. Physiol. Renal Physiol. 314, F260–f268. doi: 10.1152/ajprenal.00324.2017
Gibbs, W. S., Scholpa, N. E., Beeson, C. C., and Schnellmann, R. G. (2018c). Pharmacological activation of mitochondrial biogenesis for the treatment of various pathologies. Mitochond. Dysfun. Drug. Environ. Toxican. 2, 569–592. doi: 10.1002/9781119329725.ch39
Golpich, M., Amini, E., Mohamed, Z., Azman Ali, R., Mohamed Ibrahim, N., and Ahmadiani, A. (2017). Mitochondrial dysfunction and biogenesis in neurodegenerative diseases: pathogenesis and treatment. CNS Neurosci. Ther. 23, 5–22. doi: 10.1111/cns.12655
Gray, M. T., and Woulfe, J. M. (2015). Striatal blood-brain barrier permeability in Parkinson’s disease. J. Cereb. Blood Flow Metab. 35, 747–750. doi: 10.1038/jcbfm.2015.32
Henkel, J. S., Beers, D. R., Wen, S., Bowser, R., and Appel, S. H. (2009). Decreased mRNA expression of tight junction proteins in lumbar spinal cords of patients with ALS. Neurology 72, 1614–1616. doi: 10.1212/WNL.0b013e3181a41228
Hunter, E. J., Hamaia, S. W., Kim, P. S. K., Malcor, J.-D. M., and Farndale, R. W. (2022). The effects of inhibition and siRNA knockdown of collagen-binding integrins on human umbilical vein endothelial cell migration and tube formation. Sci. Rep. 12:21601. doi: 10.1038/s41598-022-25937-1
Hurtado, K. A., Janda, J., and Schnellmann, R. G. (2023a). Lasmiditan promotes recovery from acute kidney injury through induction of mitochondrial biogenesis. Am. J. Physiol. Renal Physiol. 324, 56–63. doi: 10.1152/ajprenal.00249.2022
Hurtado, K. A., Janda, J., and Schnellmann, R. G. (2023b). Lasmiditan restores mitochondrial quality control mechanisms and accelerates renal recovery after ischemia-reperfusion injury. Biochem. Pharmacol. 218:115855. doi: 10.1016/j.bcp.2023.115855
Jin, L. Y., Li, J., Wang, K. F., Xia, W. W., Zhu, Z. Q., Wang, C. R., et al. (2021). Blood-spinal cord barrier in spinal cord injury: a review. J. Neurotrauma 38, 1203–1224. doi: 10.1089/neu.2020.7413
Knox, E. G., Aburto, M. R., Clarke, G., Cryan, J. F., and O’Driscoll, C. M. (2022). The blood-brain barrier in aging and neurodegeneration. Mol. Psychiatry 27, 2659–2673. doi: 10.1038/s41380-022-01511-z
Kumar, H., Jo, M. J., Choi, H., Muttigi, M. S., Shon, S., Kim, B. J., et al. (2018). Matrix Metalloproteinase-8 inhibition prevents disruption of blood-spinal cord barrier and attenuates inflammation in rat model of spinal cord injury. Mol. Neurobiol. 55, 2577–2590. doi: 10.1007/s12035-017-0509-3
Lamalice, L., Le Boeuf, F., and Huot, J. (2007). Endothelial cell migration during angiogenesis. Circ. Res. 100, 782–794. doi: 10.1161/01.RES.0000259593.07661.1e
Li, X., Wang, H., Gao, Y., Li, L., Tang, C., Wen, G., et al. (2016). Protective effects of quercetin on mitochondrial biogenesis in experimental traumatic brain injury via the Nrf2 signaling pathway. PLoS One 11:e0164237. doi: 10.1371/journal.pone.0164237
Li, J., Zheng, M., Shimoni, O., Banks, W. A., Bush, A. I., Gamble, J. R., et al. (2021). Development of novel therapeutics targeting the blood-brain barrier: from barrier to carrier. Adv. Sci. 8:e2101090. doi: 10.1002/advs.202101090
Liang, C. C., Park, A. Y., and Guan, J. L. (2007). In vitro scratch assay: a convenient and inexpensive method for analysis of cell migration in vitro. Nat. Protoc. 2, 329–333. doi: 10.1038/nprot.2007.30
Mammoto, A., Muyleart, M., Kadlec, A., Gutterman, D., and Mammoto, T. (2018). YAP1-TEAD1 signaling controls angiogenesis and mitochondrial biogenesis through PGC1α. Microvasc. Res. 119, 73–83. doi: 10.1016/j.mvr.2018.04.003
Potter, M. D., Barbero, S., and Cheresh, D. A. (2005). Tyrosine phosphorylation of VE-cadherin prevents binding of p120-and β-catenin and maintains the cellular mesenchymal state*. J. Biol. Chem. 280, 31906–31912. doi: 10.1074/jbc.M505568200
Saint-Geniez, M., Jiang, A., Abend, S., Liu, L., Sweigard, H., Connor, K. M., et al. (2013). PGC-1alpha regulates normal and pathological angiogenesis in the retina. Am. J. Pathol. 182, 255–265. doi: 10.1016/j.ajpath.2012.09.003
Schimmel, L., and Gordon, E. (2018). The precise molecular signals that control endothelial cell–cell adhesion within the vessel wall. Biochem. Soc. Trans. 46, 1673–1680. doi: 10.1042/bst20180377
Scholpa, N. E., Lynn, M. K., Corum, D., Boger, H. A., and Schnellmann, R. G. (2018). 5-HT1F receptor-mediated mitochondrial biogenesis for the treatment of Parkinson’s disease. Br. J. Pharmacol. 175, 348–358. doi: 10.1111/bph.14076
Scholpa, N. E., and Schnellmann, R. G. (2017). Mitochondrial-based therapeutics for the treatment of spinal cord injury: mitochondrial biogenesis as a potential pharmacological target. J. Pharmacol. Exp. Ther. 363, 303–313. doi: 10.1124/jpet.117.244806
Scholpa, N. E., Simmons, E. C., Tilley, D. G., and Schnellmann, R. G. (2019). beta2-adrenergic receptor-mediated mitochondrial biogenesis improves skeletal muscle recovery following spinal cord injury. Exp. Neurol. 322:113064. doi: 10.1016/j.expneurol.2019.113064
Simmons, E. C., Scholpa, N. E., Cleveland, K. H., and Schnellmann, R. G. (2020a). 5-hydroxytryptamine 1F receptor agonist induces mitochondrial biogenesis and promotes recovery from spinal cord injury. J. Pharmacol. Exp. Ther. 372, 216–223. doi: 10.1124/jpet.119.262410
Simmons, E. C., Scholpa, N. E., Crossman, J. D., and Schnellmann, R. G. (2022). “Mitochondrial biogenesis for the treatment of spinal cord injury” in Diagnosis and treatment of spinal cord injury. eds. V. R. Rajendram and C. R. Martin (Cambridge: Academic Press)
Simmons, E. C., Scholpa, N. E., and Schnellmann, R. G. (2020b). Mitochondrial biogenesis as a therapeutic target for traumatic and neurodegenerative CNS diseases. Exp. Neurol. 329:113309. doi: 10.1016/j.expneurol.2020.113309
Simmons, E. C., Scholpa, N. E., and Schnellmann, R. G. (2021). FDA-approved 5-HT(1F) receptor agonist lasmiditan induces mitochondrial biogenesis and enhances locomotor and blood-spinal cord barrier recovery after spinal cord injury. Exp. Neurol. 341:113720. doi: 10.1016/j.expneurol.2021.113720
Sousa, J. A., Bernardes, C., Bernardo-Castro, S., Lino, M., Albino, I., Ferreira, L., et al. (2023). Reconsidering the role of blood-brain barrier in Alzheimer’s disease: from delivery to target. Front. Aging Neurosci. 15:1102809. doi: 10.3389/fnagi.2023.1102809
Srinivasan, B., Kolli, A. R., Esch, M. B., Abaci, H. E., Shuler, M. L., and Hickman, J. J. (2015). TEER measurement techniques for in vitro barrier model systems. J. Lab. Autom. 20, 107–126. doi: 10.1177/2211068214561025
Sweeney, M. D., Sagare, A. P., and Zlokovic, B. V. (2018). Blood-brain barrier breakdown in Alzheimer disease and other neurodegenerative disorders. Nat. Rev. Neurol. 14, 133–150. doi: 10.1038/nrneurol.2017.188
Thom, R., Rowe, G. C., Jang, C., Safdar, A., and Arany, Z. (2014). Hypoxic induction of vascular endothelial growth factor (VEGF) and angiogenesis in muscle by truncated peroxisome proliferator-activated receptor gamma coactivator (PGC)-1alpha. J. Biol. Chem. 289, 8810–8817. doi: 10.1074/jbc.M114.554394
Tsivelekas, K., Evangelopoulos, D. S., Pallis, D., Benetos, I. S., Papadakis, S. A., Vlamis, J., et al. (2022). Angiogenesis in spinal cord injury: Progress and treatment. Cureus 14:e25475. doi: 10.7759/cureus.25475
van Vliet, E. A., Ndode-Ekane, X. E., Lehto, L. J., Gorter, J. A., Andrade, P., Aronica, E., et al. (2020). Long-lasting blood-brain barrier dysfunction and neuroinflammation after traumatic brain injury. Neurobiol. Dis. 145:105080. doi: 10.1016/j.nbd.2020.105080
Vlodavsky, E., Palzur, E., Shehadeh, M., and Soustiel, J. F. (2017). Post-traumatic cytotoxic edema is directly related to mitochondrial function. J. Cereb. Blood Flow Metab. 37, 166–177. doi: 10.1177/0271678x15621068
Walker, C. L., Wu, X., Liu, N.-K., and Xu, X.-M. (2019). Bisperoxovanadium mediates neuronal protection through inhibition of PTEN and activation of PI3K/AKT-mTOR signaling after traumatic spinal injuries. J. Neurotrauma 36, 2676–2687. doi: 10.1089/neu.2018.6294
Wang, Y., Wang, J., Yang, H., Zhou, J., Feng, X., Wang, H., et al. (2015). Necrostatin-1 mitigates mitochondrial dysfunction post-spinal cord injury. Neuroscience 289, 224–232. doi: 10.1016/j.neuroscience.2014.12.061
Wang, Y., Xu, E., Musich, P. R., and Lin, F. (2019). Mitochondrial dysfunction in neurodegenerative diseases and the potential countermeasure. CNS Neurosci. Ther. 25, 816–824. doi: 10.1111/cns.13116
Wills, L. P., Trager, R. E., Beeson, G. C., Lindsey, C. C., Peterson, Y. K., Beeson, C. C., et al. (2012). The beta2-adrenoceptor agonist formoterol stimulates mitochondrial biogenesis. J. Pharmacol. Exp. Ther. 342, 106–118. doi: 10.1124/jpet.112.191528
Wu, Y. C., Sonninen, T. M., Peltonen, S., Koistinaho, J., and Lehtonen, Š. (2021). Blood-brain barrier and neurodegenerative diseases-modeling with iPSC-derived brain cells. Int. J. Mol. Sci. 22:7710. doi: 10.3390/ijms22147710
Yamazaki, Y., Shinohara, M., Shinohara, M., Yamazaki, A., Murray, M. E., Liesinger, A. M., et al. (2019). Selective loss of cortical endothelial tight junction proteins during Alzheimer’s disease progression. Brain 142, 1077–1092. doi: 10.1093/brain/awz011
Keywords: mitochondrial biogenesis, lasmiditan, 5-HT1F receptor, endothelial cells, blood–brain barrier, blood–spinal cord barrier, vascular recovery
Citation: Scholpa NE, Simmons EC, Thompson AD, Carroll SS and Schnellmann RG (2024) 5-HT1F receptor agonism induces mitochondrial biogenesis and increases cellular function in brain microvascular endothelial cells. Front. Cell. Neurosci. 18:1365158. doi: 10.3389/fncel.2024.1365158
Edited by:
Matthew McMillin, Baylor College of Medicine, United StatesReviewed by:
Samir Patel, University of Kentucky, United StatesYanet Karina Gutierrez-Mercado, University of Guadalajara, Mexico
Copyright © 2024 Scholpa, Simmons, Thompson, Carroll and Schnellmann. This is an open-access article distributed under the terms of the Creative Commons Attribution License (CC BY). The use, distribution or reproduction in other forums is permitted, provided the original author(s) and the copyright owner(s) are credited and that the original publication in this journal is cited, in accordance with accepted academic practice. No use, distribution or reproduction is permitted which does not comply with these terms.
*Correspondence: Rick G. Schnellmann, c2NobmVsbEBhcml6b25hLmVkdQ==
†These authors have contributed equally to this work and share first authorship