- Department of Neurosurgery and Neurology, Clinical Neuroscience Research Center, Tulane Brain Institute, Tulane University School of Medicine, New Orleans, LA, United States
The immune system has emerged as a key regulator of central nervous system (CNS) function in health and in disease. Importantly, improved understanding of immune contributions to mood disorders has provided novel opportunities for the treatment of debilitating stress-related mental health conditions such as major depressive disorder (MDD). Yet, the impact to, and involvement of, B lymphocytes in the response to stress is not well-understood, leaving a fundamental gap in our knowledge underlying the immune theory of depression. Several emerging clinical and preclinical findings highlight pronounced consequences for B cells in stress and MDD and may indicate key roles for B cells in modulating mood. This review will describe the clinical and foundational observations implicating B cell-psychological stress interactions, discuss potential mechanisms by which B cells may impact brain function in the context of stress and mood disorders, describe research tools that support the investigation of their neurobiological impacts, and highlight remaining research questions. The goal here is for this discussion to illuminate both the scope and limitations of our current understanding regarding the role of B cells, stress, mood, and depression.
1 Introduction
As appreciation for the complexity of neuroimmune interactions continues to grow, there is increasing evidence that B lymphocytes may play important roles in central nervous system (CNS) structure and function across the lifespan (Dwyer et al., 2022). Indeed, pathogenic as well as protective effects of B cells have been reported in the context of neurological and spinal cord injuries (Engler-Chiurazzi et al., 2020; Plantone et al., 2022; Maheshwari et al., 2023; Malone et al., 2023), autoimmune diseases (Jain and Yong, 2022), and brain aging, neurodegeneration, and dementia (Plantone et al., 2022), to name just a few examples. Neuroinflammation is a shared feature of many of the neurological diseases in which B cells are implicated (Bersano et al., 2023). And yet, while systemic and neuroinflammation is being increasingly linked to the response to psychosocial stress and the manifestation of chronic stress-related conditions (Maes, 2011; Wohleb et al., 2016; Herkenham and Kigar, 2017; Dantzer, 2018), the role of the B cell in this context is not well understood.
This discussion will focus on B cells in the context of psychosocial stress and the impacts these cells may have on stress disorders, including major depression (MDD). The sections below will reiterate the clinical significance posed by stress-related disorders, review B cell functional roles within the immune system, describe clinical and foundational observations implicating B cell-psychological stress interactions, discuss potential mechanisms by which B cells may impact brain function in the context of stress and mood disorders, describe research tools that support the investigation of their neurobiological impacts, and highlight remaining research questions. The goal here is that this discussion will illuminate both the scope and limitations of our current understanding regarding the role of B cells, stress, mood, and depression.
2 Brief background of B cell development and activation
The process underlying B cell development, activation, maturation, and effector functions is complex and has been extensively reviewed elsewhere (Meinl et al., 2006; Jain and Yong, 2022). In brief here, the majority of B cell development begins in the bone marrow. There, hematopoietic stem cells differentiate into pro- and then pre-B cells, proceeding through several key developmental stages of random recombination of the B cell receptor heavy and light chains to generate lymphocytes with responsivity to a huge diversity of immunogens. Self-reactivity, determined by at least three checkpoints (immature, transitional, and activated naïve B cell stages) must be lacking for survival and migration to secondary immune organs as naïve B cells. Naïve B1 B cells have limited B cell receptor diversity and are predominantly found in the peritoneal and pleural cavities. B2 follicular naïve B cells comprise the majority of B cells in lymphoid organs, namely the spleen. Following encounters with their antigen, a subset of activated B cells migrate to germinal centers (GCs) to undergo somatic hypermutation and class switch recombination to support affinity maturation. From there, maturing B cells become memory B cells or antibody secreting cells. A separate subset of antigen activated B cells will mature into short-lived plasmablasts outside of lymphoid structures while others become long-lived plasma cells (PC). Of note, following encounter with an antigen, activation can take place in T cell-dependent or -independent cascades depending on the B cell subset. Once activated, the functions of various B cell subsets can be classified into several domains: (1) producers of antibodies that, once created and bound to antigen, promote complement signaling as well as antigen neutralization, antigen opsonization (coating with antibodies), and ultimately destruction by other immune cells, (2) antigen presenters, and (3) cytokine and trophic factor secretors, (4) immune response regulators, and (5) memory cells that enable a more rapid and specific threat response in future encounters with that specific antigen (Figure 1). However, it is noteworthy that there is significant overlap and redundancy among the subset populations with regards to functional roles in response to immunogenic stimuli.
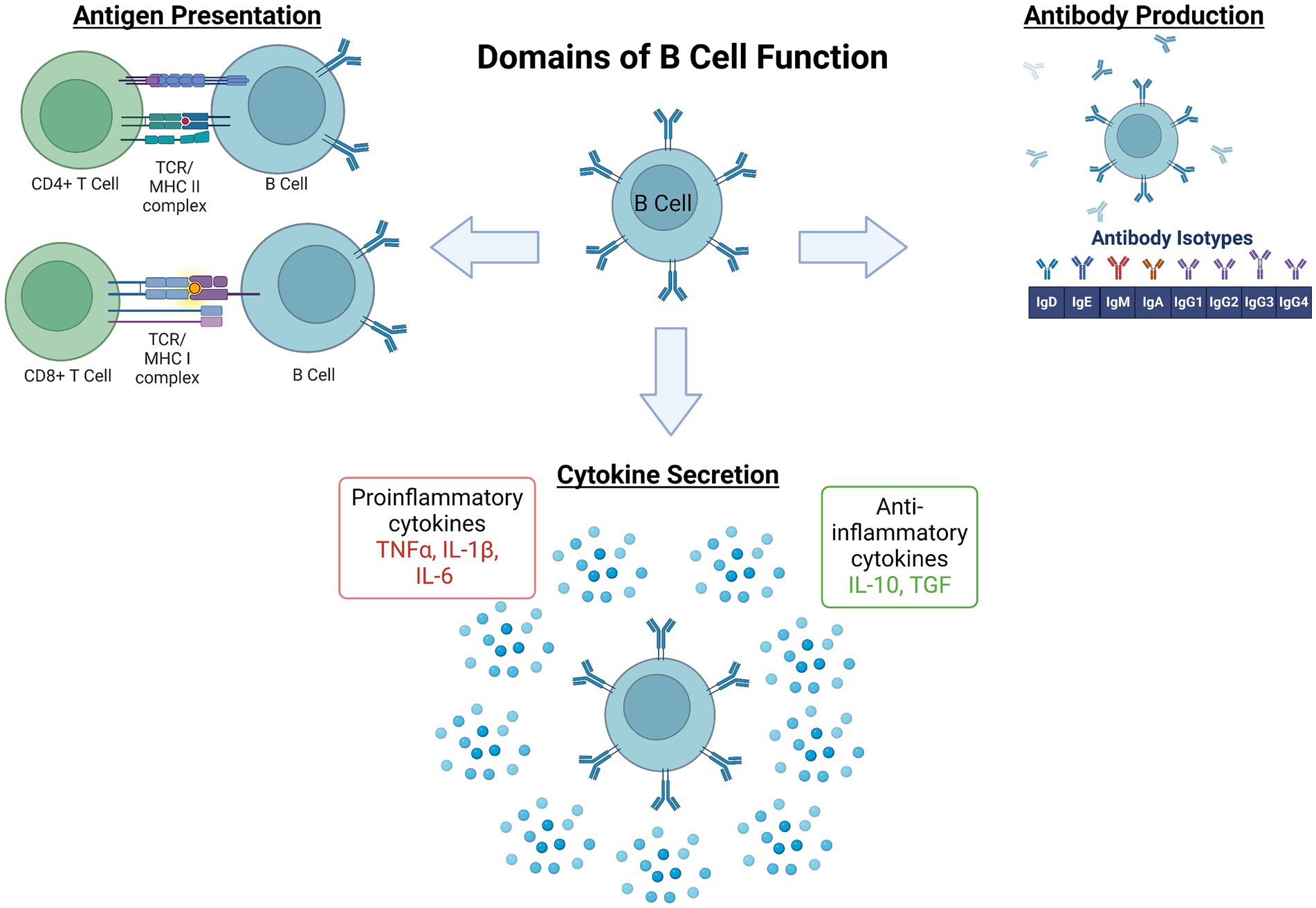
Figure 1. Domains of B lymphocyte function in the immune system. The process governing development, activation, and maturation of B lymphocytes is multifaceted and complex. Once activated by their cognate pathogenic signal, B cell subset functions within the broader immune response include (1) antigen presentation via major histocompatibility complexes (MHC) to effector immune cells, namely CD4+ or CD8+ T cells, (2) antibody production, and (3) pro- or anti-inflammatory cytokine secretion. These functions coordinate and potentially amplify, restrain, or resolve the broader immune response (Engler-Chiurazzi et al., 2020), and also serve as the foundation for immune memory to a given immunogen (Plantone et al., 2022). IL, Interleukin; TCR, T Cell Receptor; TGF, Transforming Growth Factor; TNF, Tumor Necrosis Factor. Figure created with BioRender.com.
3 Clinical significance of stress-related disorders
Mood disorders, including MDD, are a significant global health issue. Lifetime prevalence of mood disorders has been reported to be nearly 10% (Steel et al., 2014; World Health Organization, 2017) and it was recently estimated that more than 320 million people currently are affected by MDD worldwide (Ferrari et al., 2013). Problematically, the burden of mood disorders appears to be increasing. Indeed, between 2005 and 2018 in the United States alone, the number of adults with MDD increased from 15.5 to 17.5 million patients; the annual costs associated with this condition (including direct, suicide-related, and workplace costs) are estimated at $326 billion (Greenberg et al., 2021), a nearly 38% increase from previous cost estimates (Greenberg et al., 2015). While treatment interventions including antidepressant drugs are available, there is significant heterogeneity with regards to latency to symptom amelioration and in their response efficacy profiles (Trivedi et al., 2006; Al-Harbi, 2012). These factors have coalesced to make MDD a leading cause of disability worldwide (Friedrich, 2017) and a significant source of human suffering. There is, therefore, an urgent need to improve understanding of its pathogenic mechanisms and develop novel, more effective treatment interventions.
4 Evidence supporting a role for the B cell in stress and depression
Although once considered “immune privileged,” a compelling body of literature indicates that the CNS and the peripheral immune systems engage in bidirectional communication, profoundly influencing one another during homeostasis and in pathological/diseased neurological states (Pavlov et al., 2018), including those associated with chronic stress and MDD. Indeed, it is now accepted that inflammatory cascades mediated by brain resident microglia as well as peripheral innate inflammatory and adaptive T cell-mediated arms of the immune system significantly contribute to MDD, at least in some patient subsets (Maes, 2011; Wohleb et al., 2016; Herkenham and Kigar, 2017; Dantzer, 2018). Is there evidence also implicating B cells?
4.1 Observations among clinical populations
Interest in interrogating immune underpinnings of mood began in the late-1980s/early 1990s, when initial pioneering studies revealed distinct patterns of peripheral immune cell profiles among patients with MDD and mentally healthy controls (Maes, 2011; Wohleb et al., 2016). Indeed, Maes et al. (1990) noted that MDD patients displayed elevated levels of cluster of differentiation (CD)-4+ T cells, higher circulating soluble interleukin (IL)-2 receptor levels, and higher percentages of cells expressing CD25 (IL-2 receptor). As well, elevated levels of circulating cytokines, principally tumor necrosis factor (TNF)-α, IL-1β and IL-6, have been consistently reported among some subsets of depressed populations (Dowlati et al., 2010; Köhler-Forsberg et al., 2017). Depression-associated profiles of some immune cell populations and proinflammatory cytokines also normalized among patients responsive to pharmacological therapeutic intervention (Roumestan et al., 2007; Arteaga-Henríquez et al., 2019). Antidepressant treatment with co-administration of anti-inflammatory agents, such as non-steroidal anti-inflammatory drugs, statins, or cytokine inhibitors, improved depressive symptoms and MDD remission rates (Köhler‐Forsberg et al., 2019). However, high levels of inflammatory biomarkers are also often associated with poor responsiveness to serotonin (5-HT) targeting interventions (Arteaga-Henríquez et al., 2019), possibly accounting for the large heterogeneity in antidepressant efficacy for symptom remission in this population (Trivedi et al., 2006; Al-Harbi, 2012; Akil et al., 2018). Finally, observations that autoimmune patients prescribed inflammatory interventions developed rapid and profound depressed mood provided strong empirical support for immune-MDD associations (Pryce and Fontana, 2017).
At least initially, available evidence suggested that B lymphocytes were not consistently impacted by MDD. For instance, studies have reported both an increase or a decrease in B lymphocyte counts among students experiencing exam stress (Maes et al., 1999; McGregor et al., 2008). Some studies found no difference in B cell counts between MDD patients and controls (Darko et al., 1988) while others reported increased total or subset B cell counts (Maes et al., 1992) and still others showed reduced numbers (Pavón et al., 2006). Later, Ahmetspahic et al. (2018) identified that while numbers of total B cells were not altered among MDD patients relative to healthy controls, there were reduced counts of IgD+CD27− naïve, CD1d+CD5+ regulatory-like, and CD24+CD38hi B cell populations. However, these early studies were limited by a number of factors, including a highly heterogenous patient population, small sample sizes, and a focus on circulating immune cell profiles identified with lineage markers and immune cell phenotyping tools available at the time. Interestingly, a recent meta-analysis revealed elevated B cell counts in MDD patients (Foley et al., 2023). These findings are summarized in Table 1.
4.2 Preclinical associations between B lymphocytes and the response to stress: focus on glucocorticoid signaling through the hypothalamic–pituitary–adrenal axis
4.2.1 Shifts in B cell number and functional profiles with pharmacological and behavioral stress exposure
The response to psychological stress is generally considered to be mediated by engagement of the hormones involved in the hypothalamic–pituitary–adrenal axis (HPA; namely glucocorticoids), the sympathetic nervous system via catecholamines (discussed below), and more recently cytokines and immune cells. While glucocorticoids were historically considered immunosuppressive, collective evidence now indicates that stress-immune interactions, including those pertaining to B cells, are dynamic and complex (Kovacs, 2014). Indeed, like T cells, B cells of virtually all developmental stages express the cellular machinery [namely glucocorticoid (GR) and mineralocorticoid (MR) receptors] to respond to stress signals (Gruver-Yates et al., 2014) and studies have evaluated the effects of stress exposure on the number and function of B cells in key immune- and stress-related tissues. For instance, findings from several in vitro studies indicate that B cells, and particularly immature B cells, are susceptible to apoptotic consequences with exposure to high levels of corticosteroids (Garvy and Fraker, 1991; Lill-Elghanian et al., 2002; Igarashi et al., 2005). Further, in long-term cultured B lymphoblastoids collected from MDD patients or healthy controls, basal GR expression was elevated in depressed patients but showed a larger expression reduction when exposed hydrocortisone stimulation (Henning et al., 2005). In vivo, circulating B lymphocyte counts are reduced with administration of exogenous corticosterone (Dhabhar et al., 2012; Gruver-Yates et al., 2014) and tablet implant-based elevations in corticosterone levels in young adult male mice resulted in profound loss of bone marrow B cell subsets (Garvy and Fraker, 1991).
Behaviorally-induced glucocorticoid signaling has been shown to have a profound impact on B cells. For example, in adult male Sprague–Dawley rats, circulating B cell numbers initially increased in the minutes following a single acute restraint stress exposure; numbers then decreased over 2 h to below baseline levels, which the authors considered indicative of lymphocyte trafficking into tissues (Dhabhar et al., 2012). Stefanski and Engler (1998) noted reduced circulating B cell numbers with exposure to 2 h of social defeat stress in Long Evans rats, suggesting similar lymphocyte consequences in other stress conditions. B cell changes have also been reported in the context of chronic stress paradigms in a tissue-dependent manner. Although splenic and bone marrow B cell counts remained steady, 14 days of restraint for 2, 3, or 5 h/day significantly reduced B cell numbers in blood and thymus (Domínguez-Gerpe and Rey-Méndez, 2001). Leveraging a nine-week chronic variable stress paradigm, Gurfein and colleagues noted that stress exposure increased the frequency of immature and marginal zone, but decreased the frequency of follicular, B cells; interestingly total B cell counts were not altered (Gurfein et al., 2014, 2017). Notably, many of these effects are altered by environmental enrichment, an intervention known to attenuate the negative effects of stress paradigms (Fox et al., 2006). Corticotrophin releasing hormone (CRH)-transgenic mice, a well-established genetic model of stress in which persistent activation of the HPA axis is associated with increased corticosterone production and avoidance behaviors seen in chronically stressed organisms (Stenzel-Poore et al., 1992, 1994), display fewer total splenic, circulating, and bone marrow B cell counts (Murray et al., 2001). More specifically, B cell profiles tended to be skewed, with transgenic animals having higher proportions of immature cells within the tissues assayed. Finally, functional capacity of B cells may be affected by stress as chronic restraint also impaired GC responses including immunoglobulin (Ig)G1 antibody production, an effect which was prevented by GR blockade (Sun et al., 2019).
Appreciation for the importance of brain-spleen interactions in CNS health and disease is growing (Wei et al., 2022). Splenic changes associated with stress/inflammatory exposure include altered gross morphology, shifts in profiles of distinct immune cells, and changes in cytokine secretion patterns (Wei et al., 2022). For instance, repeated social defeat stress increases spleen weights, promotes hematopoietic stem cell progenitor release from bone marrow and recruitment to spleen, and induces splenomegaly with significant increases in CD11b+, natural killer, and granulocyte cell populations observed among stress-susceptible mice (McKim et al., 2018; Zhang et al., 2021). Similarly, systemic inflammation, like that induced by the bacterial infection mimic and well-established model of sickness behavior, lipopolysaccharide (LPS) (Dantzer, 2018), can profoundly alter splenic morphology and levels of circulating proinflammatory cytokines (Zhang et al., 2020). Stress exposure prior to LPS administration primes inflammatory responses in systemic and central macrophages (Johnson et al., 2002; Frank et al., 2007). It is noteworthy that stress and inflammation influences on splenic immune populations can vary by cell type and even cell subtype (Gurfein et al., 2014, 2017). Moreover, LPS is associated with inducing sickness and depressive-like behaviors and these behaviors appear to be mediated by activation of indoleamine 2,3-dioxygenase and other components of the kynurenine pathway (Bluthé et al., 1992; O'Connor et al., 2009; Remus and Dantzer, 2016).
Yet, while brain-spleen innervation patterns have been known for some time, and include some notable stress-related regions (e.g., locus coeruleus, central amygdala, and hypothalamic nuclei) (Cano et al., 2001), until recently very few studies have investigated the convergence of stress exposure, splenic B cells and the brain. In an elegant series of experiments, Zhang et al. (2020) found that CRH expressing neurons in the central amygdala and paraventricular nucleus (PVN) of the hypothalamus signal though the splenic nerve to impact B cell maturation. Activation of these neurons when mice were exposed to a chronic regimen of daily brief elevated platform stress increased numbers of splenic PCs, a finding that was later replicated by Lynall et al. (2021) using a chronic social defeat stress paradigm. PVN CRH-expressing neurons were also found to control acute restraint stress-induced shifts in peripheral lymphocyte pools (Poller et al., 2022). Indeed, stress exposure was associated with transiently reduced circulating splenic, and lymph node, B cells that homed to bone marrow. Excitingly, these findings demonstrate top-down brain control of peripheral adaptive immunity in response to certain types of psychosocial stress and lay mechanistic foundations for the continued exploration of these associations.
In addition to glucocorticoids, mineralocorticoids impact the stress response and MDD (de Kloet et al., 2016). Although aldosterone (a key MR ligand) has been reported to increase B cell activation and recruitment, the consequences of MR expression and binding on B cell function, especially in the context of stress, has been largely unexplored (Bene et al., 2014; Ferreira et al., 2021). Emerging data suggests that activation of MRs may play critical roles in modulating stress response resolution and promoting resilience (ter Heegde et al., 2015). Thus, future interrogation of the interplay between B cell and MR signaling will be key to discerning the full scope of stress-B cell interactions.
4.2.2 Genetic modification of immune function alters stress responsivity
Further support for a role for lymphocytes in the response to stress comes from findings leveraging mouse models genetically modified to capture specific immune phenotypes. Studies in lymphocyte-deficient mice (nude, scid, or Rag −/− mice) have noted deficits in adaptability to stress; reconstitution of various lymphocyte populations in these mice generally implicated the absence of T cells in mediating these deficits (Cohen et al., 2006; Beurel et al., 2013; Rattazzi et al., 2013; Clark et al., 2014; Brachman et al., 2015). Importantly, (primarily T) lymphocytes from stress-exposed mice can modify the behavioral response to stress when adoptively transferred into lymphocyte deficient subjects (Brachman et al., 2015; Scheinert et al., 2016).
However, some evidence may support an association between the absence in B cells and pro-stress susceptible phenotype. Along with CD38, CD157, also known as bone marrow stromal cell antigen-1 (aka bone marrow stromal cell antigen-1), plays an important role in nicotinamide adenine dinucleotide metabolism and cell signaling cascades (Lopatina et al., 2020). CD157 is expressed on a number of cell types, including central and peripheral immune cells but also many nestin-positive neural cells (Higashida et al., 2017). Interestingly, CD157−/− mice displayed pronounced maladaptive responses to forced swim stress, leading authors to conclude that CD157 may play an important role in anxiety and social avoidance (Lopatina et al., 2014). Notably, B cell development in these mice is impaired as is the B cell-driven antibody response to infection (Itoh et al., 1998). This suggests that B cell deficiency may be at least partially involved with maladaptive stress responses and social interactions.
4.3 Evidence for B cell presence in CNS sites in stress and depression
The presence of peripheral immune cells in healthy brain parenchyma is rare (Korin et al., 2017) though robust immune cell recruitment to CNS can take place in times of neuroinflammatory challenge (Londoño and Mora, 2018), such as stress and MDD (Medina-Rodriguez et al., 2023). The neurobiology of stress and MDD is complex, and potentially injurious changes to brain structure and function have been reported when the stress response becomes dysregulated. Indeed, while these cascades have been extensively discussed elsewhere (McEwen, 2017; Godoy et al., 2018; McEwen and Karatsoreos, 2020), some stress-related consequences include reductions in brain volume (Koolschijn et al., 2009), region-specific changes in neuronal excitability leading to hypertrophy or atrophy (Chaudhury et al., 2015), tract-specific white matter disruptions and demyelination (Breit et al., 2023), blood–brain barrier (BBB) disruption and increased permeability (Medina-Rodriguez et al., 2023), and impaired hippocampal neurogenesis (Schoenfeld and Cameron, 2015). These highlight just a few examples of the numerous CNS impacts reported in both acute and chronic stress conditions among human and preclinical populations. A key question to address here is Do B cells accumulate in brain or brain-adjacent tissues during stress or depression, and if so, what role do they play? Do they promote chronic stress cascades, support resilience to the negative consequences of stress, or both?
Much of our understanding of B cell accumulation in CNS comes from neurological aging, injury or disease models such as stroke, or autoimmunity. Indeed, in multiple sclerosis (MS), gut origin IgA+ PCs that mediate mucosal immunity represent at least some of the B cell subsets that are recruited to CNS border sites and accumulate at lesions (Fitzpatrick et al., 2020; Pröbstel et al., 2020). Immunosenescence is associated with a profound shift in the B cell compartment and the emergence of innate-like age-associated B cells that accumulate in meninges (Brioschi et al., 2021). During stroke, B cells traffic to brain and accumulate both in the infarcted tissue and also at sites distal to stroke damage (Ortega et al., 2020). The functional significance of this accumulation is still being interrogated given that several reports note neuroprotective and neurorestorative actions of B cells (Ren et al., 2011; Ortega et al., 2020) while others suggest that autoreactive B cells may promote long-term poststroke cognitive deficits (Doyle et al., 2015).
There is limited but emerging support for brain-B cell interactions in the context of stress and MDD. For instance, in a few small cohort studies evaluating post-mortem brain tissues of schizophrenic, mood disorder, MDD, or bipolar disorder patients, a subset of subjects from each diagnosis condition displayed elevated brain lymphocyte profiles (Bogerts et al., 2017; Schlaaff et al., 2020). More specifically, in mood disorders, T and B cells were elevated relative to non-psychiatric patient controls. Interestingly, B cells appeared to accumulate in hippocampus/parahippocampal region, pre/peri/postcentral gyrus area, central white matter, and entorhinal and inferior temporal regions. Finally, B cells accumulated in hippocampi of mice exposed to a prolonged (but not acute) foot shock-induced learned helplessness or chronic restraint relative to non-stress exposed mice (Beurel et al., 2018). Surprisingly, chronic restraint stress did not result in similar T cell elevations, suggesting that stressor type may influence immune cell type recruitment patterns.
In addition to blood or organ-derived B cells, recent data identifying local CNS/CNS-adjacent originating B cells could suggest an additional mechanism by which B cells respond to neuroinflammatory states. Indeed, Brioschi et al. (2021) identified that B cells represent approximately 30% of CD45+ cells in the meninges. In addition, they identified a population of CNS antigen educated B cells that are derived from a bone marrow niche in the skull and reach meninges through vascular channels from the calvaria. Schafflick et al. further identified the dura as a site of progenitor B cell residence (Schafflick et al., 2021). Interestingly, while chronic learned helplessness increased hippocampal B cell numbers, splenic total B cell counts were not affected (Beurel et al., 2018). This observation, while considering that meningeal B cells were reduced in mice exposed to chronic social defeat (Lynall et al., 2021), may implicate meningeal or other local CNS-adjacent lymphocyte pools as the source of these parenchymal infiltrating cells.
In addition to trafficking to secondary lymphoid organs, leukocytes including B cells influence the local immune response by migrating to inflamed non-lymphoid tissues and forming ectopic or tertiary lymphoid structures (ELS) (Londoño and Mora, 2018; Harrer et al., 2022). ELSs in the CNS were first discovered in meninges close to inflammatory lesions in the context of MS (Negron et al., 2020), where the trafficking of B cells via key chemokine ligand-receptor interactions (discussed below) was shown to potentiate disease progression (Magliozzi et al., 2007). Additional studies have since identified B cell aggregating ELS in neuropsychiatric lupus (Stock et al., 2019), stroke (Doyle et al., 2015), and acute or chronic spinal cord damage (Cohen et al., 2021), to name a few examples. Importantly, research regarding development of local CNS ELS is in its infancy. Whether such structures form in response to the neuroinflammatory state produced by stress and MDD is not yet known though one study in a mouse model of lupus, a model in which depressive-like phenotypes have been well-established (Gao et al., 2009), found evidence for ELS in choroid plexus (Stock et al., 2019).
5 Mechanisms by which B cells migrate to, access, and remain at sites of inflammation
To address the role of B cells in the stressed brain, it is important to determine the extent to which B cell recruitment, trafficking, and survival signals are engaged during the experience of stressful life events, and to discern whether these signals become dysregulated in chronic stress.
5.1 B cell recruitment and trafficking signals
Central to an effective immune response to challenge is the tightly regulated movement of immune cells within lymphoid organs and to sites of inflammation. This process is governed by chemokines (Stein and Nombela-Arrieta, 2005; Chen et al., 2018). Several chemokines are implicated in the recruitment of B cells to key organs and sites, including C-X-C motif ligand (CXCL)12 (and cognate receptors CXCR4/7), CXCL13 (CXCR5), CC chemokine ligand (CCL)19 (CCR7), and CCL21 (CCR7) (Irani, 2016; Harrer et al., 2022; Jain and Yong, 2022). These chemokines can be homeostatic (e.g., CXCL13, CCL21), regulating cellular trafficking during immune surveillance or inflammation-induced (e.g., CXCL9, CXCL10). They can support development (e.g., CXCL12), and can modulate migration through primary (CXCL12) and secondary lymphoid organs (e.g., CXCL13, CCL19, CCL21). Some chemokines (CXCL12, CCL19, CCL21) are considered to have key brain homing functions. Importantly, some chemokines regulate the movement of several immune cell subtypes, including T cells (e.g., CCL19, CCL21, CXCL10).
B cell trafficking chemokines play diverse roles in the CNS. CXCL12, CCL19, and CCL21 are constitutively expressed in CNS at low levels namely by neurons, glial cells, and vascular endothelial cells (Li and Ransohoff, 2008; Lalor and Segal, 2010; Milenkovic et al., 2019) while these in addition to CXCL13 may be inducible in neural cell types in a disease state-dependent fashion (Krumbholz et al., 2007; Irani, 2016). Indeed, spinal cord neurons produce CXCL13 in a preclinical ligation model of neuropathic pain (Jiang et al., 2016) but not in viral encephalomyelitis where microglia show upregulated Cxcl13 gene expression (Esen et al., 2014). Various CNS cell populations are responsive to chemokine signals but regional distribution of chemokine receptors is varied and suggests distinct functional roles for each ligand-receptor system (Turbic et al., 2011). For example, CXCR4, and CXCR7 to a lesser extent, is expressed on a wide variety of CNS cell types (such as astrocytes, microglia, and neurons and neural progenitor cells) in the hippocampus and subventricular zone. Thus, it is perhaps unsurprising that the CXCL12-CXCR4/7 system facilitates proliferation and migration of neural progenitor cells during development and adulthood (Schönemeier et al., 2008; Sánchez-Alcañiz et al., 2011). CXCL12 can also act directly on cells as it has been shown to induce GABAergic activity in diverse neuronal populations (Guyon, 2014). CXCR5 expression is not well represented regionally in CNS tissues though other findings suggest that this receptor is expressed by most CNS cell types (Turbic et al., 2011; Stuart et al., 2015). CXCL13 expression was upregulated in neurospheres exposed to inflammatory cytokine challenge (Turbic et al., 2011) and other cellular sources of CXCL13 produced during inflammatory conditions include microglia (Irani, 2016; Londoño and Mora, 2018) and astrocytes (Szpakowski et al., 2022). Several investigations regarding CXCL13 and the brain center on its contributions to autoimmunity, with converging findings suggests that CXCL13-mediated recruitment of B cells is associated with disease severity, progression, and poor prognosis (Londoño and Mora, 2018). CXCL13 neutralization in systemic lupus prone mice improved object recognition cognitive function and attenuated forced swim depressive-like behavior (Huang et al., 2021). However, in stroke, CXCL13 levels support trafficking of B cells to brain where they may mediate neuroprotective effects (Monson et al., 2014; Ortega et al., 2020). Increased CXCL13 and CXCR5 in anterior cingulate cortex facilitated conditioned place aversion in a rodent model of neuropathic pain, suggesting that these cascades, and possibly B cells themselves may benefit aversive learning and memory under physical stress conditions on the water maze (Wu et al., 2019). However, B cell deficiency did not impact memory performance (Wolf et al., 2009). CXCR5−/− knockout mice displayed enhanced adult dentate gyrus neurogenesis and possible anxiolytic effects as indicated by increased movement in general and in the inner zone of the open field (Stuart et al., 2015). CCL21 may also impact adult neuronal differentiation given the high expression of CCR7 in the subventricular zone and on astrocytes, microglia, and neurons (Turbic et al., 2011; Noor and Wilson, 2012). This position adjacent to the CSF-filled lateral ventricle may also poise this chemokine-receptor system to engage in T and B immune cell recruitment. Indeed, CCL21 is expressed on the surface of choroid plexus epithelium and on endothelial venules only during inflammation and may mediate T or other CXCR7 expressing cell transit through brain-CSF and BBB routes (Alt et al., 2002; Kivisäkk et al., 2004).
Levels of chemokines have been investigated in the context of stress and MDD (Milenkovic et al., 2019). The majority of the published clinical studies reveal consistent circulating or CSF expression elevations in CCL2 and CXCL8 chemokines in depressed patients (Eyre et al., 2016). Of note, in vitro evidence suggests that these chemokines along with CCL20 may also support B cell trafficking and possible entry into CNS (Alter et al., 2003; Jain and Yong, 2022). Some clinical studies noted higher CXCL12 in depressed men and women in comparison with controls (Ogłodek et al., 2014; Romero-Sanchiz et al., 2020). Similarly, one study in rats exposed to prenatal stress (dam received gestational bright light exposure) revealed that CXCL12 was upregulated in the hippocampus and prefrontal cortex (Ślusarczyk et al., 2015) and microglial CXCR4 expression was reduced with prenatal stress (Ślusarczyk et al., 2015). Stress exposure may also modulate expression of recruitment signal receptors on B cells. Poller et al. (2022) demonstrated that bone marrow homing restraint stress-exposed B and T cells unregulated expression of CXCR4. Further, Lynall et al. (2021) noted that meningeal B cells from chronic social defeat exposed mice showed trends toward increased expression of CXCL13 genes. Other generalized immune cell recruitment signals may support B cell trafficking to CNS. Indeed, pathogen challenge increased brain chemokine expression and facilitated B cell recruitment in a CXCL9- and − 10-dependent manner (Lokensgard et al., 2016); these chemokines have been found to be elevated in patients with high levels of anxiety and MDD (Leighton et al., 2018). Finally, a thorough investigation of B cell recruitment signaling in CSF is still outstanding but may warrant further direct interrogation given that under normal conditions, levels of chemokines in CSF are low but increase manifold with inflammatory challenge (Pilz et al., 2020; DiSano et al., 2021).
5.2 Mechanisms modulating B cell access to brain
The mechanisms regulating immune cell migration across tissues, namely the BBB, are complex and has been extensively discussed elsewhere (Marchetti and Engelhardt, 2020; Jain and Yong, 2022). In brief here, several primary factors appear to regulate B cell movement across the BBB. First, activated leukocyte cell adhesion molecule (ALCAM) expressed on the surface of the activated proinflammatory B cells as well as on endothelial cells supports B cell diapedesis across the BBB (Michel et al., 2019). Leukocyte function-associated antigen-1 (LFA-1) on the surface of B cells binds its ligand intracellular adhesion molecule-1 (ICAM1). Very late antigen-4 (VLA4) expressed on activated B cells has been well studied in autoimmune diseases. Further, in autoimmune models, B cells appear to be recruited to CNS in an antigen-independent manner (Tesfagiorgis et al., 2017) and do not take up residence in sites of inflammation. L-selectin (aka CD62L) and vascular cell adhesion molecule-1 do not appear to play a robust role in B cell movement across membranes (Alter et al., 2003). While mechanisms regulating immune cell trafficking across BBB were extensively reviewed, whether B cells engaged these mechanisms in times of immune surveillance was not discussed (Marchetti and Engelhardt, 2020).
Studies on the effects of stress exposure, and stress-associated hormonal signaling, on leukocyte adhesion mechanisms have yielded interesting observations (Ince et al., 2018). Following 12 days of chronic unpredictable stress, RNAseq data in prefrontal cortex revealed increased gene expression of Alcam in non-neuronal cell clusters as well as several types of inhibitory and excitatory neuronal populations (Kwon et al., 2022). Fifteen minutes of forced public speaking resulted in elevated LFA-1 on mixed lymphocytes, no impact to ICAM1 density, and a decrease in L-selectin on T cells (Goebel and Mills, 2000). Similarly, VLA-4, CD44, and LFA-1 expression in T cells was altered by a single 16–18 h restraint stress exposure (Tarcic et al., 1995). With the exception of (Dhabhar et al., 2012), in which acute restraint stress reduced circulating B cell numbers and induced a biphasic decrease in L-selectin expression on B cells in the 2 h following stress cessation, the impact of stress exposure on adhesion mechanisms specifically in B cells were not measured in these studies. Interestingly, administration of norepinephrine (NE, aka noradrenaline), the receptors for which are expressed on B cells, decreased the number of L-selectin (CD62L) positive B cells but increased circulating B cells negative for this cell adhesion marker without impacting overall B cell L-selectin expression.
It is noteworthy that the BBB is not the only barrier impacted by stress. Several studies have found that intestinal barriers are leaky among patients with psychiatric disease (Wasiak and Gawlik-Kotelnicka, 2023). Immune cells are critically involved in the extent of intestinal barrier integrity. Indeed, B cells support intestinal homeostasis through production of IgA and IgM antibodies, immune regulatory functions, and cytokine secretion; whether they play a protective, pathogenic, or mixed role in intestinal inflammatory conditions is the subject of intense investigation (Zogorean and Wirtz, 2023). Gut microbiota composition is closely related to intestinal permeability status, and shifts in microbiome taxa composition have been reported in MDD (Cheung et al., 2019). As appreciation for gut-brain-immune interactions in the context of stress and MDD continues to increase, novel interventions such as psychobiotics or fecal microbiome transfers may prove beneficial (Trzeciak and Herbet, 2021).
5.3 B cell survival and retention in CNS or CNS-adjacent sites
If cells are recruited to sites of inflammation, such as the stressed brain, another question remains: is there sufficient support for B cells to survive and remain there to enact their cellular functions?
5.3.1 BAFF and APRIL
Two principal B cell survival signals are well described in the immunology literature (Vincent et al., 2013). B cell activating factor (BAFF; aka B lymphocyte stimulator, TNFα APOL-related leukocyte expressed ligand, and CD257), is a cytokine belonging to the TNFα ligand family. It is expressed by a variety of immune cell types and acts to activate B cells as well as to induce their proliferation and differentiation. Receptors for BAFF include BAFF-R, BCMA, and TACI, in order of ligand affinity. BAFF-R is expressed on immature B cells, while TACI is principally expressed on innate like B1 cells. Inadequate BAFF fails to activate B cells to mature and go on to produce antibody or enact other effector functions; excessive BAFF levels limit B cell apoptosis and may cause overproduction of antibodies, potentially leading to autoimmunity.
A proliferation-inducing ligand (APRIL, aka TNFSF13) is another B cell survival signal (Vincent et al., 2013). APRIL acts as a co-stimulator for B and T cell proliferation and acts via TACI and BCMA receptors. APRIL also promotes IL-10 production and regulatory functions of B cells (Hua et al., 2016). Within CNS, APRIL is expressed by astrocytes in postmortem MS patient brains (Thangarajh et al., 2007) and facilitated axon growth of hippocampal pyramidal and midbrain and striatal dopaminergic neurons during embryonic development (Osório et al., 2014; McWilliams et al., 2017). Expression of BAFF/APRIL receptors in the brain is unclear (Marella et al., 2022) though a recently identified BAFF receptor Noggo-66 was found to be expressed on neurons and possibly glial cells, and may be a negative regulator of cell function (Zhang et al., 2009).
BAFF and APRIL may play a role in stress. Indeed, increased anxiety-like behaviors (open field, elevated plus maze, novelty suppressed feeding), evidence of neuroinflammation (reactive astrocytes, activated microglia), impaired hippocampal neurogenesis, and disrupted long-term potentiation were found in BAFF overexpressing transgenic mice, a common preclinical model of autoimmunity (Crupi et al., 2010). In humans, one small cohort study reported reduced circulating BAFF in MDD patients; levels were elevated with antidepressant intervention (Schmidt et al., 2019). However, in a larger study of 3,221 subjects, plasma levels of BAFF were not significantly different between healthy control subjects and patients with schizophrenia or MDD, though BAFF levels were elevated among bipolar patients (Engh et al., 2022). Plasma levels of APRIL were lower in psychosis patients compared to healthy controls and significantly correlated with higher psychotic symptom load, though other studies have not found APRIL levels to differ (Crupi et al., 2010; Schmidt et al., 2019).
While CNS levels of BAFF and APRIL have not been well studied in the stress exposed brain, Lynall et al. (2021) noted that meningeal B cells from chronic social defeat exposed mice were lower in number but showed trends toward increased expression of BAFF genes. As the stressed brain represents a state of neuroinflammation (Liu et al., 2017) and collective evidence suggests that the inflamed CNS provides a pro-survival microenvironment for leukocytes including B cells (Meinl et al., 2006), perspectives gleaned from other neuroinflammatory conditions can inform our understanding of B cell interactions with stress and MDD. For example, following experimental stroke, BAFF expression is elevated in microglia (Li et al., 2017) and CD11bhigh B cells recruited to brain after injury are known to regulate microglia activation, increasing their phagocytic capacity (Korf et al., 2022). BAFF ligand-receptor interactions have been found to support neuronal survival and impart neuroprotection in an animal model of amyotrophic lateral sclerosis, an effect that may be independent of B cells (Tada et al., 2013). Astrocytes also express BAFF and promote B cell activation in response to viral infection (Lokensgard et al., 2016) or in MS (Krumbholz et al., 2005). Interestingly, in animals exposed to experimental autoimmune encephalomyelitis (animal model of MS) and in MS patients, disease attenuating anti-CD20 treatment increased BAFF levels in serum, CSF, and leptomeninges (Wang et al., 2024). Further, the beneficial effects for gray matter and microgliosis were reversed when anti-BAFF treatment was given in conjunction with B cell depletion, suggesting that BAFF plays a neuroprotective role in MS (Wang et al., 2024). Whether other CNS cell types produce BAFF and support B cell survival, especially in the context of psychosocial stress or MDD, is not known and represents an important question to be answered.
5.3.2 Growth factors
Neurotrophins, such as brain derived neurotrophic factor (BDNF), neurotrophic factor 3 (NT3), and nerve growth factor (NGF), play important roles in development, survival, and function of a wide variety of CNS and immune cell types. Of relevance here, evidence suggests that B cells express neurotrophin receptors p75TR, TrkA, TrkB, and TrkC in a subtype-specific pattern (Hillis et al., 2016). Neurotrophins have complementary and sometimes redundant impacts to B cells. BDNF supports bone marrow B cell maturation as well as mature B cell survival (D'Onofrio et al., 2000), while NGF, and NT3 to a lesser extent, facilitate proliferation, survival, differentiation, antibody production, and CD40 T cell co-stimulatory expression (Hillis et al., 2016). Importantly, B lymphocytes can also secrete neurotrophins and this may have neuroprotective effects against inflammatory insults (Kerschensteiner et al., 1999).
A large body of evidence has implicated growth factors in mood disorders and/or the response to antidepressants (Zhang et al., 2015; Ceci et al., 2021). For instance, BDNF and TrkB levels are reduced in hippocampi of depressed patients (Zhang et al., 2015) and BDNF knock out mice display increased depressive-like behaviors and also have fewer B cells (Schuhmann et al., 2005). NGF, a potent survival factor for memory B cells (Hillis et al., 2016), is increased in inflamed tissues and has been shown to be elevated in blood in response to acute stress (Ceci et al., 2021). However, chronic stress and depressed mood conditions are often (though not always) associated with downregulation of NGF. Like other growth factors, NT3 is highly expressed in brain regions implicated in MDD, such as hippocampus, and is often elevated in MDD brains (Zhang et al., 2015). Known to regulate BDNF expression, one study reported reduced NT3 mRNA expression in peripheral white blood cells of MDD patients (Otsuki et al., 2008). While these associations are interesting, the paucity of work directly addressing the convergence of stress/depression, growth factors, and B cells leaves several remaining questions as to the extent to which these three factors interact to influence mood. Moreover, still more work is needed to address how B cells engage with other growth factors, including insulin-like growth factor, platelet derived growth factor, and glial derived growth factor, in these contexts.
5.3.3 Interactions with T cells
Interactions with CD4+ T helper cells support survival and differentiation of B cells as well as GC formation, B cell isotype switching and somatic hypermutation, and formation of long-lived antibody-secreting PC (Aloulou and Fazilleau, 2019). The classic mechanism by which these interactions take place is via CD40 binding with CD40 ligand (CD40L) expressed by CD4 T and B cells (among other cell types) under inflammatory conditions. Importantly, a number of studies have identified important roles for CD4 T cells in CNS, namely in modulating neurological function in the healthy, stressed, injured, or diseased brain (Fletcher et al., 2010; Filiano et al., 2017; Herkenham and Kigar, 2017; Rayasam et al., 2018). In fact, one of the most consistently reported immune compartment alterations in depressed patient populations is an elevated circulating CD4+/CD8+ ratio (Maes, 2011; Herkenham and Kigar, 2017; Khedri et al., 2020). Further, preclinical studies in lymphocyte-deficient mice (nude, scid or Rag −/− mice) have noted deficits in adaptability to stress; reconstitution of various lymphocyte populations in these mice generally implicates the absence of T cells in mediating these deficits (Cohen et al., 2006; Beurel et al., 2013; Rattazzi et al., 2013; Clark et al., 2014; Brachman et al., 2015). A variety of T cell subsets have been found in brain parenchyma following acute and chronic stress, including CD4+ T cells (Beurel et al., 2013; Peng et al., 2022; Medina-Rodriguez et al., 2023). T cells also robustly respond to glutamatergic signaling (Ganor and Levite, 2014), a neurotransmitter system that is emerging as a key contributor to MDD and a principal target for novel, fast acting antidepressants (Wang et al., 2021).
There is evidence that co-stimulatory pathways involved with T-B cell interactions are impacted in stress and depression. Basal platelet CD40 expression is higher in patients with MDD (Neubauer et al., 2013). As well, circulating soluble CD40L levels were elevated in first episode unmedicated MDD patients and these levels were reduced when antidepressant interventions were administered (Leo et al., 2006; Myung et al., 2016), though another study reported reduced CD40L serum levels in MDD patients (Zhang et al., 2023). CD40 activation induces sickness behavior and depressive-like phenotypes in mice, as revealed by reduced saccharine preference and impaired fear learning (Cathomas et al., 2015). Thus, while T cells in the stressed brain may exert their own effector functions, their presence may serve to facilitate stress-responsive actions of B cells, or vice versa- B cells may facilitate the CNS effector functions of T cells.
One potential though controversial action of B cells is that they can regulate the development and activation of subsets of T cells via OX40-OX40 ligand or inducible T cell co-stimulator (ICOS)-ICOS ligand interactions (Petersone et al., 2018). While mice exposed to a single 12 h session of restraint did not show differences in splenic OX40 protein expression (Ahmad et al., 2015), hippocampal ICOS mRNA expression was elevated in mice exposed to footshock induced learned helplessness (Beurel et al., 2018). B cells may be a potential source of both OX40 or ICOS ligands (Petersone et al., 2018), yet there is little known as to how either ligands are impacted by stress or depression. Of note, though ICOS ligand is the exclusive binding partner for ICOS, it can be produced by most antigen presenting cells (Wikenheiser and Stumhofer, 2016) and some barrier cell types. Whether B cells are a significant source of ICOS ligand production in response to stress is currently unknown.
5.4 B cell-neurotransmitter interactions
5.4.1 Serotonin
B cells express receptors for a wide variety of neurotransmitters and this may have important implications in stress and depression. The serotonergic (5HT) system has been historically implicated in chronic stress disorders and functioned as targets of antidepressant action, though that association is not without controversy (Jauhar et al., 2023). B cells express 5HT receptors 1A, 2A, 3 and 7 along with the 5HT transporter (SERT) (Herr et al., 2017). 5HT stimulation of B cells appears to induce proliferation, likely in a 5HT-1A receptor dependent mechanism (Iken et al., 1995) that requires inflammatory gene transcription (Abdouh et al., 2001). Some evidence in B cell and lymphoma lines suggests that SERT expression increases in activated B cells enabling them to take up 5HT (Meredith et al., 2005) and transport it, potentially long distances. However, B cell-derived lymphoma cells failed to proliferate with 5HT stimulation and showed increased apoptosis with 5HT uptake (Serafeim et al., 2002; Kolan et al., 2019). Interestingly, antidepressant intervention restores some B cell population numbers in MDD (Hernandez et al., 2010; Ahmetspahic et al., 2018). Lacking expression of tryptophan hydroxylase 1, B cells do not appear to be able to synthesize 5HT like T cells (Herr et al., 2017). MDD patients consistently show reduced expression of SERT on peripheral lymphocytes that can be ameliorated at least in part with administration of one of several classes of antidepressant intervention (Urbina et al., 1999; Peña et al., 2005). As 5HT can also be synthesized in both the brain and the periphery via distinct mechanisms, this should be considered when trying to discern the convergence of 5HT, B cells, and stress (Herr et al., 2017).
5.4.2 Norepinephrine/noradrenaline
In addition to regulating the immune response via stress signaling through the HPA axis, the brain leverages the sympathetic nervous system innervation of primary and secondary immune organs principally through release of NE (Kin and Sanders, 2006; Herkenham and Kigar, 2017; Chan et al., 2023). Antigen-mediated immune activation resulted in increased NE release and turnover in lymphoid organs, an effect that was partially blocked when NE signaling from brain to periphery was inhibited (Kohm et al., 2000). Immune cells located in these organs express NE receptors, specifically β2 adrenergic receptors (Kerage et al., 2019); moreover, this receptor is expressed much more highly on B cells than T cells (Kin and Sanders, 2006). Lymphocytes express the NE transporter (Mata et al., 2005), and dopamine (DA)-β hydroxylase, a key enzyme governing the conversion of DA to NE, is also found in B cells (Papa et al., 2017). This suggests that B cells can take up and also synthesize NE de novo.
In the context of public speaking stress, which elevated circulating NE levels, there was a trend toward elevated peripheral B cell counts but also a tendency toward less IgM produced in response to mitogen stimulation in vitro when compared with B cells collected pre-stress (Matthews et al., 1995). Retrograde viral tracing revealed that several stress/reward-associated brain regions, such as hypothalamus, hippocampus, amygdala, ventral tegmental area, and locus coeruleus, innervate bone marrow, a site of NE release (Dénes et al., 2005). Similar regions were reflected in brain-spleen innervation patterns (Cano et al., 2001), further supporting an association between NE, B cell immunity, and stress. In MDD patients, while NE levels were not altered, lymphocyte NE transporter expression was reduced (Mata et al., 2005). It may be that stress effects on at least some immune cells are mediated by both corticosterone and catecholaminergic stimulation as noted that adrenalectomy or catecholamine receptor antagonism prevent at least some stress-associated circulating leukocyte shifts (Engler et al., 2004). Interestingly, epinephrine (adrenaline), but not NE administration, reduced total B lymphocytes in circulation, suggesting these cells were trafficked into tissues (Dhabhar et al., 2012).
5.4.3 Dopamine
DA, a key mediator of reward circuits known to be dysregulated in MDD (Russo and Nestler, 2013), is now appreciated to have potent immunomodulatory actions (Vidal and Pacheco, 2020). Relative to other immune cell types, B cells displayed the most robust expression of DA receptors (DRDs); DRDs 2–5 were expressed on B cells, with DRD3 expressed at the highest levels (McKenna et al., 2002). Stimulation of unique DRDs can exert distinct and sometimes competing effects within the immune system. For example, B cell DRD1 stimulation by CD4+ T follicular cells, found to be potent secretors of DA, reorganizes expression of co-stimulator machinery, facilitates GC synapses, and promotes B cell maturation (Papa et al., 2017). In a small cohort study of patients with rheumatoid arthritis, B cell DRD expression was lower than that of healthy controls, B cell DRD2 stimulation is negatively correlated with disease activity (Wei et al., 2015), and DRD2 and − 3 expression levels were increased among patients treated for 3 months with an anti-rheumatic disease modifying drug (Wieber et al., 2022). However, in female rheumatoid arthritis patients, B cells were shown to express DRD1, the frequency of DRD1 was correlated with severity of disease, and stimulation of these cells increased expression of proinflammatory factors, suggesting sex-specific effects with regard to DA-B cell interactions in this disease. Further, the consequences of DAergic stimulation of B cells may be disease context-specific, as MOG-induced mouse models of MS promoted CNS infiltration of DRD3 expressing B cells with regulatory/immunosuppressive and antigen presenting phenotypes (Prado et al., 2021). Expression of the DA transporter is found on lymphocytes and mice in which it is lacking show exaggerated memory B cell responses (Gopinath et al., 2023). Further, DA transporter expression on resting lymphocytes was reduced in schizophrenic and bipolar psychosis patients (Marazziti et al., 2010). Chronic stress can induce hyperexcitability in certain DAergic neuronal populations, namely in stress- and mood-related brain areas in which DA expression is high (including hippocampus, paraventricular nucleus, and striatum) (Russo and Nestler, 2013). Whether these stress-related consequences in DAergic signaling impact the number and function of B cells that are present in or trafficked to these regions and what that means for stress susceptibility or resolution is worthy of further exploration. However, evidence suggests this potential as designer receptors activated only by designer drug-induced activation of the ventral tegmental area increased IgM+ B cells and potentiated IgM antibody secretion with E. coli immune challenge relative to mice who did not receive neuronal activation (Ben-Shaanan et al., 2016).
5.4.4 Glutamate and GABA
Dysfunction in the glutamatergic system, representing the major excitatory neurotransmitter of the CNS, has emerged as a key component of MDD development and potential novel target for fast-acting depressive symptom amelioration (Sarawagi et al., 2021). Indeed postmortem assessments of MDD patient brains revealed reduced size of the glutamatergic neuronal layer VI (Cotter et al., 2002) as well as reduced expression of glutamatergic receptor machinery in prefrontal cortex (Feyissa et al., 2009). Similarly, in MDD, size and density of various GABAergic interneurons are reduced in this and other regions implicated including the anterior cingulate and amygdala (Rajkowska et al., 2007; Tripp et al., 2011; Guilloux et al., 2012). Imaging studies of a variety of modalities have confirmed disruption in these neurotransmitter systems (Sarawagi et al., 2021) and recent meta-analyses revealed decreased levels of glutamate+glutamine in the anterior cingulate and medial frontal cortex as well as decreased GABA in occipital and prefrontal cortex of MDD patients (Luykx et al., 2012; Romeo et al., 2018; Moriguchi et al., 2019).
Emerging evidence suggests that B cells can be impacted by glutamatergic receptor ligands. Indeed, human B cells expressed GRIK2, −3, −4, and − 5 kainate receptor genes and activation of these receptors resulted in increased proliferation and IgE release (Sturgill et al., 2011). B cells may also express metabotropic glutamate receptors and ligand binding may mediate apoptotic cascades (Ma et al., 2015). Finally, B cells may express functional N-methyl-D-aspartate receptors (NMDA; GluN2A and B) (Torres et al., 2021). The functional significance of which is not currently well studied though one recent report noted that non-competitive antagonists impaired B cell migration, proliferation and Ig production but increased numbers of IL-10 secreting B cells (Simma et al., 2014). Further interrogation of these complex interactions will be important considering the recent regulatory approvals for glutamatergic modulation in patients with refractory MDD (Sarawagi et al., 2021).
B cells may also interact with the GABA system, the key inhibitory neurotransmitter of the CNS (Sarawagi et al., 2021). Indeed, B cells can take up glutamine and process it to produce glutamate or GABA; CD8+ T cell or macrophage proinflammatory antitumor responses were dampened as a result (Zhang et al., 2021). Importantly, GABA deficiency has long been considered an important component of disordered mood (Sarawagi et al., 2021). Whether B cells in the CNS of behavioral stress-exposed or depressed organisms also secrete GABA and exert anti-inflammatory effects or neuromodulatory effects that regulate immune cascades present in stress/MDD-implicated brain regions is yet to be determined. For both glutamate and GABA, an informed understanding of B cell impacts will need to include the observation of B cell production of autoantibodies against these receptors must also be considered (Sun et al., 2020) (see below).
5.5 Potential CNS-directed effector functions of B cells
The next question to be answered is what might B cells be doing in the stressed CNS? The answer may be condition specific as B cells appear to be pathogenic drivers in autoimmunity and Alzheimer’s-like dementia whereas they may be neurorestorative in acute brain or spinal cord injury (Engler-Chiurazzi et al., 2020; Plantone et al., 2022; Maheshwari et al., 2023; Malone et al., 2023).
5.5.1 B cell antibody production
A key effector function of B cells is their capacity to produce antibodies, including IgA, IgD, IgE, IgG, and IgM. Antibody production ability of B cells can have important functional consequences for CNS. For instance, B cells, and their production of IgM, may play a critical role in myelin development, a tightly regulated process dependent on local microenvironment signaling (Nishiyama et al., 2021). Tanabe and Yamashita (2018) identified that B cells, specifically B1a B cells, are recruited to choroid plexus and meningeal spaces via CXCL13 and supported oligodendrocyte proliferation via IgM secretion. Importantly, antibody titers may be altered by stress and in stress-related disease (Kronfol and House, 1989; Joyce et al., 1992; Song et al., 1994; Gold et al., 2012). Relative to mentally healthy control subjects, Gold et al. (2012) noted that depressed populations displayed reductions in serum IgA, but not IgM or IgG levels. However, Joyce et al. (1992) reported increased IgA in MDD patients. Exam and occupational stress was associated with elevated IgA, IgG, and IgM levels (Maes et al., 1999; Matos-Gomes et al., 2010). Methodological differences between sample populations and measurement approaches may account for some of the discrepancy between these studies, though recent meta-analyses confirmed that acute stress elevated Ig levels while chronically stressed humans showed age-related increased IgA or decreased IgM (Khan et al., 2021; Castro-Quintas et al., 2023). Mainly implicated in response to allergens, serum IgE levels are elevated in MDD patients (El-Ansary, 2022) and hydrocortisone increased IgE levels in IL-4 stimulated human lymphocytes (Wu et al., 1991). Though one study noted elevated IgD levels among MDD patients (El-Ansary, 2022), very little is known about how psychosocial stress or MDD impact IgD, likely due to its very low level of basal expression (Vladutiu, 2000). In mice, elevated platform stress-induced B cell profile shifts corresponded to an increase in IgG antibody titers in blood in the weeks following the stress exposure (Zhang et al., 2020). The functional consequences of these stress-induced shifts in Ig secretion for immunity are conflicting and may be Ig subtype specific. Shifted B cell profiles among CRH overexpressing transgenic mice were associated with less robust IgM and IgG antibody responses to a thymus-dependent, bacterial-like antigen immunization, yet there were no differences in survival when mice were exposed to gram-positive S. pneumoniae bacterial immune challenge (Murray et al., 2001). This suggests that stress-induced changes in B cell responsivity may not negatively impact overall immune response to at least some pathogenic challenges. Yet, speech making stress exposure increased the magnitude of allergen skin prick test wheal responses among allergic rhinitis (Kiecolt-Glaser et al., 2009).
Beyond classic immunological roles for immunoglobulins, clinical observations have noted that B cell-derived autoantibodies against CNS targets, namely glutamatergic but also GABAergic receptors, are implicated in several neurological disorders (Sun et al., 2020). For instance, NMDA receptor antibody encephalitis is associated with modest CNS perivascular and meningeal CD20+ B cells while CD138+ plasma cells have been observed in the CNS (Tüzün et al., 2009; Martinez-Hernandez et al., 2011), an effect supported by the observation of elevated CSF levels of CXCL13 observed in these patients (Leypoldt et al., 2015). Several reports note that patients with anti-NMDA encephalitis report poor psychosocial function (Blum et al., 2020; Nguyen et al., 2020) and humans (migrants) or mice with chronic life stress experience display serum NMDAR1-antibody (IgA) (Pan et al., 2021). As well, neuronal surface autoantibody expression has been implicated in a number of neuropsychiatric conditions, MDD included (Zong et al., 2017). Though the process governing immune tolerant B cell development is complex (Sun et al., 2020), two key sources of self-reactive autoantibodies have been identified: antibody-secreting plasmablasts from GCs in secondary lymphoid organs and bone marrow resident long-lived PCs (Hansen, 2022). This is important given the recent findings noting increased splenic PCs with acute and chronic stress in rodents (Zhang et al., 2020; Lynall et al., 2021), linking the possibility for B cells to play a significant role in stress exposure-induced CNS reactive autoantibody generation mechanisms. As to where these cells are located, the BBB disruption reported in stress and MDD provides a promising mechanism by which Igs (autoreactive or not), expressed at very low levels under normal conditions (Zong et al., 2017), are able to access the CNS. Intrathecal synthesis by PCs is another possibility that has yet to be fully discerned. It is worth noting that understanding in this field is still being developed and remains controversial (Vahabi et al., 2021), thus added clarity is likely to emerge with additional investigations in the coming years.
5.5.2 B cell interactions with T cells
In addition to supporting activation and survival (above), B-T cell interactions take place within the context of antigen presentation via major histocompatibility complexes (MHC) (Chen and Jensen, 2008). Indeed, after internalization via pinocytosis or B cell receptor-mediated endocytosis, B cells display antigenic peptide via MHCs to the T cell receptor of their antigen-cognate T cell. This interaction serves discrete functions, dependent on MHC class. MHCI molecules are ubiquitously expressed on all nucleated cell types, including B cells (Rock et al., 2016) as well as neurons and glial cells in brain regions underlying mood and cognition (Elmer and McAllister, 2012). MHCI displays critical non-immune roles in cortical development, neurite outgrowth, synaptic regulation, and learning and memory (Shatz, 2009; Elmer and McAllister, 2012; Nelson et al., 2013). Further, MHCI expression on neurons is altered by cytokines, an important observation given that stress exposure induces proinflammatory shifts in cytokine profiles. When activated B cells present antigen via MHCI, CD8+ cytotoxic T cells are activated (Wieczorek et al., 2017) or in some cases tolerized (Bennett et al., 1998; Castiglioni et al., 2005). Like other professional antigen presenting cells, B cells constitutively express modest levels of MHCII surface molecules, though expression is substantially increased following cell activation or cytokine stimulation (Rock et al., 2016). Given the strong evidence for critical roles for T cells in MDD, namely the elevated ratio of CD4+/CD8+ T cells in MDD patients (Maes et al., 1990) and that T cell deficiency in mice increases maladaptive stress response behaviors which are ameliorated by T cell adoptive transfer (Cohen et al., 2006), antigen presentation and subsequent co-stimulation of T and B cells could have critical impacts on the stress response. Several genome wide association studies have revealed variants in expression of MHC-related genes among MDD patient populations (Woo et al., 2018; Wray et al., 2018; Li et al., 2019). Maes et al. (1992) also identified increased expression of MHC-II on the surface of T cells collected from blood of depressed patients. MHC expression has also been found altered in rodent models of stress. The percent of peritoneal macrophages displaying MHCII surface molecules was reduced in adult male mice exposed to a single session of 12–18 h of restraint stress (Zwilling et al., 1990). This effect appears to be mediated by glucocorticoid signaling as in vitro, MHCII expression in B cells exposed to corticosterone is reduced (Nugent et al., 2011). Whether similar changes are occurring on B cells in response to behavioral stress is not known.
5.5.3 B cell cytokine production
B cells represent a heterogenous cell population with the capacity to secrete a variety of cytokines in response to immune stimulation and these secretion patterns can have fundamentally distinct consequences for immunity and for CNS (Hoffman et al., 2016; Jain and Yong, 2022). As elegantly depicted in 6, B cell-derived cytokines can promote macrophage activation (granulocyte-macrophage colony stimulating factor), support PC differentiation and survival (IL-6), regulate T cell differentiation, proliferation and inflammatory function (IL-6; transforming growth factor-β, and IL-35), facilitate formation of follicle-like structures (lymphotoxin-α) and modulate microglial cell activation (TNFα; interferon-γ). Indeed, proinflammatory B cells regulated microglia and macrophage proinflammatory cytokine expression in MS (Touil et al., 2018). In contrast, B regulatory cells have been recently been recognized to down-modulate inflammation, predominantly by restraining the inflammatory immune response via interleukin (IL)-10 secretion (Hoffman et al., 2016; Jain and Yong, 2022). This cytokine plays a protective role in various brain inflammatory conditions (Lobo-Silva et al., 2016), systemic levels are altered in MDD, and converging data support its critical role in mediating stress susceptibility (Mesquita et al., 2008; Gazal et al., 2015; Laumet et al., 2018). Importantly, in MS IL10-expressing B cells upregulated quiescence associated molecules (Touil et al., 2018). Such cell-to-cell interactions may exert important functional consequences considering the complex roles played by microglia in regulated CNS function under normal and MDD conditions (Wang et al., 2022).
6 Research tools
The pioneering insights gleaned from B cell interactions with CNS in other neurological conditions coupled with the availability of numerous B cell-modulating immunology research tools (Lee et al., 2021; Utset et al., 2021) poises the neuroscience field to rigorously interrogate mechanisms by which these cells may impact the response to stress. For instance, recent advances in spectral flow cytometry allow for more robust and comprehensive phenotyping of immune and resident cell panels in distinct tissues (Nolan, 2022). Administration of fluorescently labeled immune cells permits tracking of immune cell migration with a high degree of spatial specificity. Adoptive transfer techniques can be used to reconstitute transgenic animals lacking in distinct leukocyte populations (e.g., B cells absent in μMT) with distinct immune cell populations (e.g., B regulatory cells) or with cells which lack certain functional capabilities (e.g., IL-6 vs. IL-10 secreting capacity). Further monoclonal antibody (mAb)-mediated strategies to deplete distinct B cell populations, cytokines, or survival signals in vivo can differentiate functional impacts during adulthood from those during some critical developmental window. Some of the many available approaches including anti-CD19 or -CD20 mAbs (e.g., Inebilizumab; Rituximab; Ocrelizumab) are in trials or approved for use in autoimmune SLE or MS (Zhang et al., 2023). Moreover, various BAFF B cell survival signal inhibitors (e.g., Belimumab) are being developed or are currently deployed in clinical populations. Treatments developed for other therapeutic applications that are directed against other central [e.g., microglial depletion with colony stimulating factor 1 receptor inhibitors (PLX3397) (Basilico et al., 2022)] or systemic [e.g., anti-CD3 muromonab (Kuhn and Weiner, 2016)] immune cell populations can be used for discerning the extent to which interactions with other cells types is an important mechanism by which B cells influence the response to stress. MAbs directed against distinct cytokines are also available. A thorough understanding of the strengths and weaknesses of each approach is necessary to properly interpret results using these various research tools. For example, μMT mice exhibit reduced T cell numbers and functionality (Homann et al., 1998; Bergmann et al., 2001). Anti-CD20 B cell depletion (Rituximab) spares T cells (Uchida et al., 2004), innate-like T cell–independent B1 cells (Hamaguchi et al., 2005) and plasma cells (Hauser et al., 2008) while BAFF receptor targeting with TACI-IgG (Atacicept) robustly depletes the plasma cell compartment (Kappos et al., 2014). As appreciation for the role these cells play in orchestrating immune and neurological function during homeostasis and stress grows, this understanding should better inform our current and future interventions to support mental health and wellbeing.
7 Conclusion
Taken together, evidence above supports complex B cell-stress-brain interactions (Figure 2). Psychosocial stress exerts robust effects on B cells; these effects can vary on a number of factors including the nature of the stressor, the tissue, and the B cell subtype being assayed. Brain control of peripheral immune cell compartments suggest complex regulatory cascades are engaged to control this response. Perturbation of B cell systems may be associated with alterations to hedonic state that could render an organism susceptible to a depressive-like phenotype. Multifaceted signaling and trafficking systems are engaged with stress exposure, some of which may result in B cell migration through blood or CSF to CNS target sites such as meningeal ELS, circumventricular organs, or even parenchyma itself. Finally, given their ability to engage with key neurotransmitter systems, B cells may impact the stressed brain in functionally meaningful ways that will continue to be revealed as research in this area progresses in the coming years.
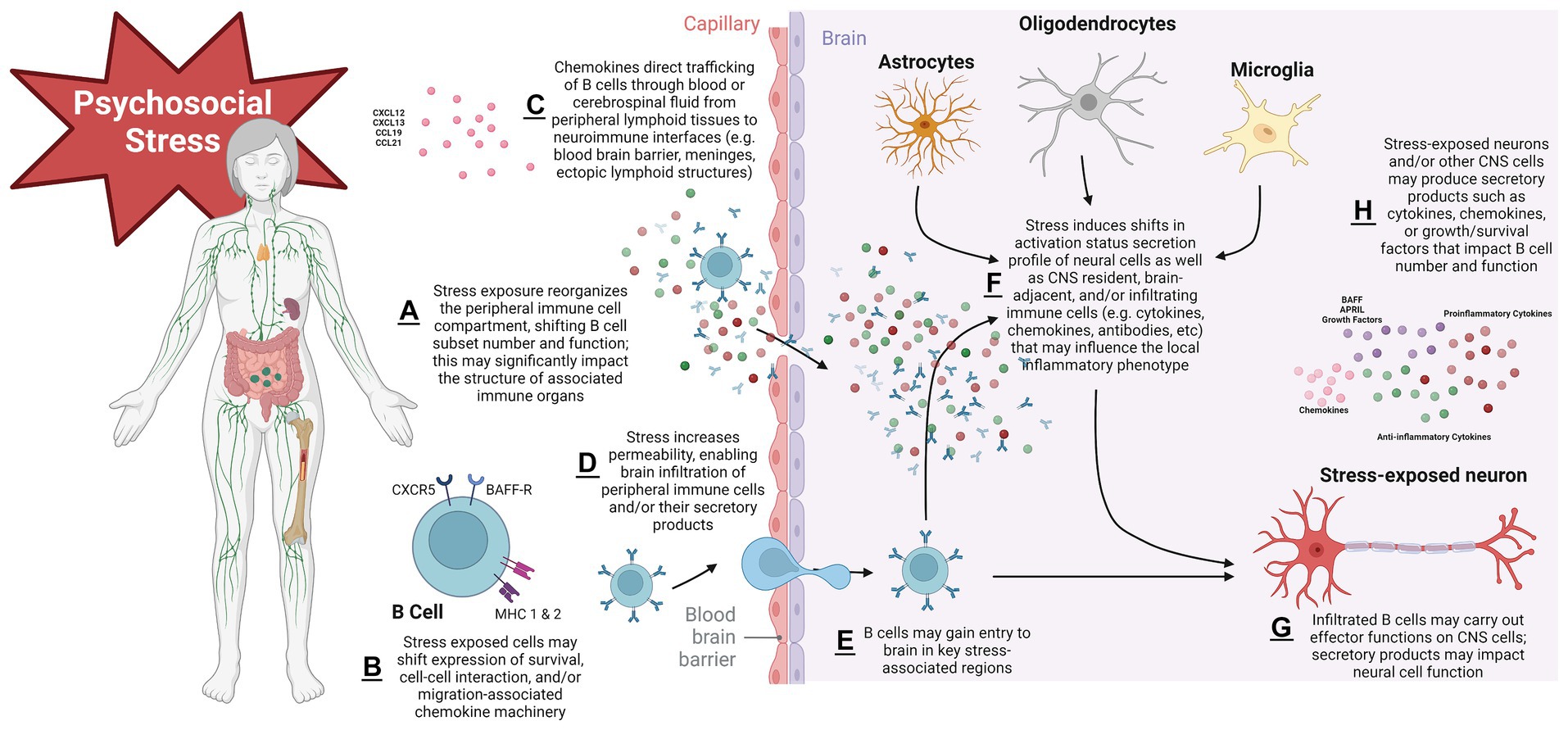
Figure 2. Proposed schema of mechanisms governing B lymphocyte impacts to the psychosocial stressed brain. Stress exposure initiates shifts in secondary lymphoid organs that reorganize the peripheral B cell compartment and alter number and function in a subtype-specific manner. This can have important morphological consequences for immune organs. Stress modifies expression of cellular surface machinery components that impact B cell development/survival, cell–cell interaction capacity, and migration. B cell trafficking signals govern the movement of cells to sites of inflammation in target organs, in this case the CNS. Stress exposure may render CNS protective barriers leaky, permitting infiltration of B cell secretory products (such as pro- and anti-inflammatory cytokines or antibodies) or cells themselves into brain parenchyma, where activation states and functional phenotypes of local resident CNS and/or immune cells have been altered. Cell survival signals may modulate the lifespan with which infiltrated cells have to carry out their effector functions on the stressed CNS. With repeated stress exposure, this cascade becomes dysregulated, leading to chronic stress disorders such as MDD. Figure created with BioRender.com.
Author contributions
EE-C: Conceptualization, Funding acquisition, Writing – original draft, Writing – review & editing.
Funding
The author(s) declare that financial support was received for the research, authorship, and/or publication of this article. This work was supported by funds awarded to EE-C including NIH K01 MH117343, NIH P20 GM109036, the Tulane Brain Institute Research Fund Award, A Studio in the Woods, and start-up funds provided by the Tulane University.
Conflict of interest
The author declares that the research was conducted in the absence of any commercial or financial relationships that could be construed as a potential conflict of interest.
The reviewer, RS, declared a past co-authorship with the author EE-C to the handling Editor.
The author(s) declared that they were an editorial board member of Frontiers, at the time of submission. This had no impact on the peer review process and the final decision.
Publisher’s note
All claims expressed in this article are solely those of the authors and do not necessarily represent those of their affiliated organizations, or those of the publisher, the editors and the reviewers. Any product that may be evaluated in this article, or claim that may be made by its manufacturer, is not guaranteed or endorsed by the publisher.
References
Abdouh, M., Storring, J. M., Riad, M., Paquette, Y., Albert, P. R., Drobetsky, E., et al. (2001). Transcriptional mechanisms for induction of 5-HT1A receptor mRNA and protein in activated B and T lymphocytes. J. Biol. Chem. 276, 4382–4388. doi: 10.1074/jbc.M004559200
Ahmad, S. F., Zoheir, K. M., Ansari, M. A., Korashy, H. M., Bakheet, S. A., Ashour, A. E., et al. (2015). Stimulation of the histamine 4 receptor with 4-methylhistamine modulates the effects of chronic stress on the Th1/Th2 cytokine balance. Immunobiology 220, 341–349. doi: 10.1016/j.imbio.2014.10.014
Ahmetspahic, D., Schwarte, K., Ambrée, O., Bürger, C., Falcone, V., Seiler, K., et al. (2018). Altered B cell homeostasis in patients with major depressive disorder and normalization of CD5 surface expression on regulatory B cells in treatment responders. J. Neuroimmune Pharmacol. 13, 90–99. doi: 10.1007/s11481-017-9763-4
Akil, H., Gordon, J., Hen, R., Javitch, J., Mayberg, H., McEwen, B., et al. (2018). Treatment resistant depression: a multi-scale, systems biology approach. Neurosci. Biobehav. Rev. 84, 272–288. doi: 10.1016/j.neubiorev.2017.08.019
Al-Harbi, K. S. (2012). Treatment-resistant depression: therapeutic trends, challenges, and future directions. Patient Prefer. Adherence 6, 369–388. doi: 10.2147/PPA.S29716
Aloulou, M., and Fazilleau, N. (2019). Regulation of B cell responses by distinct populations of CD4 T cells. Biom. J. 42, 243–251. doi: 10.1016/j.bj.2019.06.002
Alt, C., Laschinger, M., and Engelhardt, B. (2002). Functional expression of the lymphoid chemokines CCL19 (ELC) and CCL 21 (SLC) at the blood-brain barrier suggests their involvement in G-protein-dependent lymphocyte recruitment into the central nervous system during experimental autoimmune encephalomyelitis. Eur. J. Immunol. 32, 2133–2144. doi: 10.1002/1521-4141(200208)32:8<2133::AID-IMMU2133>3.0.CO;2-W
Alter, A., Duddy, M., Hebert, S., Biernacki, K., Prat, A., Antel, J. P., et al. (2003). Determinants of human B cell migration across brain endothelial cells. J. Immunol. 170, 4497–4505. doi: 10.4049/jimmunol.170.9.4497
Arteaga-Henríquez, G., Simon, M. S., Burger, B., Weidinger, E., Wijkhuijs, A., Arolt, V., et al. (2019). Low-grade inflammation as a predictor of antidepressant and anti-inflammatory therapy response in MDD patients: a systematic review of the literature in combination with an analysis of experimental data collected in the EU-MOODINFLAME consortium. Front. Psych. 10:458. doi: 10.3389/fpsyt.2019.00458
Basilico, B., Ferrucci, L., Khan, A., Di Angelantonio, S., Ragozzino, D., and Reverte, I. (2022). What microglia depletion approaches tell us about the role of microglia on synaptic function and behavior. Front. Cell. Neurosci. 16:1022431. doi: 10.3389/fncel.2022.1022431
Bene, N. C., Alcaide, P., Wortis, H. H., and Jaffe, I. Z. (2014). Mineralocorticoid receptors in immune cells: emerging role in cardiovascular disease. Steroids 91, 38–45. doi: 10.1016/j.steroids.2014.04.005
Bennett, S. R., Carbone, F. R., Toy, T., Miller, J. F., and Heath, W. R. (1998). B cells directly tolerize CD8(+) T cells. J. Exp. Med. 188, 1977–1983. doi: 10.1084/jem.188.11.1977
Ben-Shaanan, T. L., Azulay-Debby, H., Dubovik, T., Starosvetsky, E., Korin, B., Schiller, M., et al. (2016). Activation of the reward system boosts innate and adaptive immunity. Nat. Med. 22, 940–944. doi: 10.1038/nm.4133
Bergmann, C. C., Ramakrishna, C., Kornacki, M., and Stohlman, S. A. (2001). Impaired T cell immunity in B cell-deficient mice following viral central nervous system infection. J. Immunol. 167, 1575–1583. doi: 10.4049/jimmunol.167.3.1575
Bersano, A., Engele, J., and Schäfer, M. K. E. (2023). Neuroinflammation and brain disease. BMC Neurol. 23:227. doi: 10.1186/s12883-023-03252-0
Beurel, E., Harrington, L. E., and Jope, R. S. (2013). Inflammatory T helper 17 cells promote depression-like behavior in mice. Biol. Psychiatry 73, 622–630. doi: 10.1016/j.biopsych.2012.09.021
Beurel, E., Lowell, J. A., and Jope, R. S. (2018). Distinct characteristics of hippocampal pathogenic T(H)17 cells in a mouse model of depression. Brain Behav. Immun. 73, 180–191. doi: 10.1016/j.bbi.2018.04.012
Blum, R. A., Tomlinson, A. R., Jetté, N., Kwon, C. S., Easton, A., and Yeshokumar, A. K. (2020). Assessment of long-term psychosocial outcomes in anti-NMDA receptor encephalitis. Epilepsy Behav. 108:107088. doi: 10.1016/j.yebeh.2020.107088
Bluthé, R. M., Dantzer, R., and Kelley, K. W. (1992). Effects of interleukin-1 receptor antagonist on the behavioral effects of lipopolysaccharide in rat. Brain Res. 573, 318–320. doi: 10.1016/0006-8993(92)90779-9
Bogerts, B., Winopal, D., Schwarz, S., Schlaaff, K., Dobrowolny, H., Mawrin, C., et al. (2017). Evidence of neuroinflammation in subgroups of schizophrenia and mood disorder patients: a semiquantitative postmortem study of CD3 and CD20 immunoreactive lymphocytes in several brain regions. Neurol. Psychiatry Brain Res. 23, 2–9. doi: 10.1016/j.npbr.2016.11.001
Brachman, R. A., Lehmann, M. L., Maric, D., and Herkenham, M. (2015). Lymphocytes from chronically stressed mice confer antidepressant-like effects to naive mice. J. Neurosci. 35, 1530–1538. doi: 10.1523/JNEUROSCI.2278-14.2015
Breit, S., Mazza, E., Poletti, S., and Benedetti, F. (2023). White matter integrity and pro-inflammatory cytokines as predictors of antidepressant response in MDD. J. Psychiatr. Res. 159, 22–32. doi: 10.1016/j.jpsychires.2022.12.009
Brioschi, S., Wang, W. L., Peng, V., Wang, M., Shchukina, I., Greenberg, Z. J., et al. (2021). Heterogeneity of meningeal B cells reveals a lymphopoietic niche at the CNS borders. Science 373:eabf9277. doi: 10.1126/science.abf9277
Cano, G., Sved, A. F., Rinaman, L., Rabin, B. S., and Card, J. P. (2001). Characterization of the central nervous system innervation of the rat spleen using viral transneuronal tracing. J. Comp. Neurol. 439, 1–18. doi: 10.1002/cne.1331
Castiglioni, P., Gerloni, M., Cortez-Gonzalez, X., and Zanetti, M. (2005). CD8 T cell priming by B lymphocytes is CD4 help dependent. Eur. J. Immunol. 35, 1360–1370. doi: 10.1002/eji.200425530
Castro-Quintas, Á., Palma-Gudiel, H., San Martín-González, N., Caso, J. R., Leza, J. C., and Fañanás, L. (2023). Salivary secretory immunoglobulin A as a potential biomarker of psychosocial stress response during the first stages of life: a systematic review. Front. Neuroendocrinol. 71:101083. doi: 10.1016/j.yfrne.2023.101083
Cathomas, F., Fuertig, R., Sigrist, H., Newman, G. N., Hoop, V., Bizzozzero, M., et al. (2015). CD40-TNF activation in mice induces extended sickness behavior syndrome co-incident with but not dependent on activation of the kynurenine pathway. Brain Behav. Immun. 50, 125–140. doi: 10.1016/j.bbi.2015.06.184
Ceci, F. M., Ferraguti, G., Petrella, C., Greco, A., Tirassa, P., Iannitelli, A., et al. (2021). Nerve growth factor, stress and diseases. Curr. Med. Chem. 28, 2943–2959. doi: 10.2174/0929867327999200818111654
Chan, K. L., Poller, W. C., Swirski, F. K., and Russo, S. J. (2023). Central regulation of stress-evoked peripheral immune responses. Nat. Rev. Neurosci. 24, 591–604. doi: 10.1038/s41583-023-00729-2
Chaudhury, D., Liu, H., and Han, M. H. (2015). Neuronal correlates of depression. Cell. Mol. Life Sci. 72, 4825–4848. doi: 10.1007/s00018-015-2044-6
Chen, K., Bao, Z., Tang, P., Gong, W., Yoshimura, T., and Wang, J. M. (2018). Chemokines in homeostasis and diseases. Cell. Mol. Immunol. 15, 324–334. doi: 10.1038/cmi.2017.134
Chen, X., and Jensen, P. E. (2008). The role of B lymphocytes as antigen-presenting cells. Arch. Immunol. Ther. Exp. 56, 77–83. doi: 10.1007/s00005-008-0014-5
Cheung, S. G., Goldenthal, A. R., Uhlemann, A. C., Mann, J. J., Miller, J. M., and Sublette, M. E. (2019). Systematic review of gut microbiota and major depression. Front. Psych. 10:34. doi: 10.3389/fpsyt.2019.00034
Clark, S. M., Sand, J., Francis, T. C., Nagaraju, A., Michael, K. C., Keegan, A. D., et al. (2014). Immune status influences fear and anxiety responses in mice after acute stress exposure. Brain Behav. Immun. 38, 192–201. doi: 10.1016/j.bbi.2014.02.001
Cohen, M., Giladi, A., Raposo, C., Zada, M., Li, B., Ruckh, J., et al. (2021). Meningeal lymphoid structures are activated under acute and chronic spinal cord pathologies. Life Sci Alliance 4:e202000907. doi: 10.26508/lsa.202000907
Cohen, H., Ziv, Y., Cardon, M., Kaplan, Z., Matar, M. A., Gidron, Y., et al. (2006). Maladaptation to mental stress mitigated by the adaptive immune system via depletion of naturally occurring regulatory CD4+CD25+ cells. J. Neurobiol. 66, 552–563. doi: 10.1002/neu.20249
Cotter, D., Mackay, D., Chana, G., Beasley, C., Landau, S., and Everall, I. P. (2002). Reduced neuronal size and glial cell density in area 9 of the dorsolateral prefrontal cortex in subjects with major depressive disorder. Cereb. Cortex 12, 386–394. doi: 10.1093/cercor/12.4.386
Crupi, R., Cambiaghi, M., Spatz, L., Hen, R., Thorn, M., Friedman, E., et al. (2010). Reduced adult neurogenesis and altered emotional behaviors in autoimmune-prone B-cell activating factor transgenic mice. Biol. Psychiatry 67, 558–566. doi: 10.1016/j.biopsych.2009.12.008
Dantzer, R. (2018). Neuroimmune interactions: from the brain to the immune system and vice versa. Physiol. Rev. 98, 477–504. doi: 10.1152/physrev.00039.2016
Darko, D. F., Lucas, A. H., Gillin, J. C., Risch, S. C., Golshan, S., Hamburger, R. N., et al. (1988). Cellular immunity and the hypothalamic-pituitary axis in major affective disorder: a preliminary study. Psychiatry Res. 25, 1–9. doi: 10.1016/0165-1781(88)90152-7
de Kloet, E. R., Otte, C., Kumsta, R., Kok, L., Hillegers, M. H., Hasselmann, H., et al. (2016). Stress and depression: a crucial role of the mineralocorticoid receptor. J. Neuroendocrinol. 28. doi: 10.1111/jne.12379
Dénes, A., Boldogkoi, Z., Uhereczky, G., Hornyák, A., Rusvai, M., Palkovits, M., et al. (2005). Central autonomic control of the bone marrow: multisynaptic tract tracing by recombinant pseudorabies virus. Neuroscience 134, 947–963. doi: 10.1016/j.neuroscience.2005.03.060
Dhabhar, F. S., Malarkey, W. B., Neri, E., and McEwen, B. S. (2012). Stress-induced redistribution of immune cells--from barracks to boulevards to battlefields: a tale of three hormones--Curt Richter award winner. Psychoneuroendocrinology 37, 1345–1368. doi: 10.1016/j.psyneuen.2012.05.008
DiSano, K. D., Gilli, F., and Pachner, A. R. (2021). Are CSF CXCL13 concentrations solely dependent on intrathecal production? A commentary on “chemokine CXCL13 in serum, CSF, and blood–CSF barrier function”. Fluids Barr. CNS 18:9. doi: 10.1186/s12987-021-00244-5
Domínguez-Gerpe, L., and Rey-Méndez, M. (2001). Alterations induced by chronic stress in lymphocyte subsets of blood and primary and secondary immune organs of mice. BMC Immunol. 2:7. doi: 10.1186/1471-2172-2-7
D'Onofrio, M., de Grazia, U., Morrone, S., Cuomo, L., Spinsanti, P., Frati, L., et al. (2000). Expression of neurotrophin receptors in normal and malignant B lymphocytes. Eur. Cytokine Netw. 11, 283–291.
Dowlati, Y., Herrmann, N., Swardfager, W., Liu, H., Sham, L., Reim, E. K., et al. (2010). A meta-analysis of cytokines in major depression. Biol. Psychiatry 67, 446–457. doi: 10.1016/j.biopsych.2009.09.033
Doyle, K. P., Quach, L. N., Solé, M., Axtell, R. C., Nguyen, T. V., Soler-Llavina, G. J., et al. (2015). B-lymphocyte-mediated delayed cognitive impairment following stroke. J. Neurosci. 35, 2133–2145. doi: 10.1523/jneurosci.4098-14.2015
Dwyer, L. J., Stowe, A. M., Doyle, K., Popovich, P., Engler-Chiurazzi, E., LeGuern, C., et al. (2022). The 2022 FASEB virtual catalyst Conference on B cells in injury and regeneration, March 30, 2022. FASEB J. 36, 8:e22459. doi: 10.1096/fj.202201027
El-Ansary, M. A. E. (2022). Hossam: immunoglobulins level in patients suffering from major depressive disorder (MDD) in comparison with normal healthy individuals: a prospective study. Egyptian. J. Med. Microbiol. 31, 45–48. doi: 10.21608/ejmm.2022.228622
Elmer, B. M., and McAllister, A. K. (2012). Major histocompatibility complex class I proteins in brain development and plasticity. Trends Neurosci. 35, 660–670. doi: 10.1016/j.tins.2012.08.001
Engh, J. A., Ueland, T., Agartz, I., Andreou, D., Aukrust, P., Boye, B., et al. (2022). Plasma levels of the cytokines B cell-activating factor (BAFF) and A proliferation-inducing ligand (APRIL) in schizophrenia, bipolar, and major depressive disorder: a cross sectional, multisite study. Schizophr. Bull. 48, 37–46. doi: 10.1093/schbul/sbab106
Engler, H., Dawils, L., Hoves, S., Kurth, S., Stevenson, J. R., Schauenstein, K., et al. (2004). Effects of social stress on blood leukocyte distribution: the role of alpha- and beta-adrenergic mechanisms. J. Neuroimmunol. 156, 153–162. doi: 10.1016/j.jneuroim.2004.08.005
Engler-Chiurazzi, E. B., Monaghan, K. L., Wan, E. C. K., and Ren, X. (2020). Role of B cells and the aging brain in stroke recovery and treatment. Geroscience 42, 1199–1216. doi: 10.1007/s11357-020-00242-9
Esen, N., Rainey-Barger, E. K., Huber, A. K., Blakely, P. K., and Irani, D. N. (2014). Type-I interferons suppress microglial production of the lymphoid chemokine, CXCL13. Glia 62, 1452–1462. doi: 10.1002/glia.22692
Eyre, H. A., Air, T., Pradhan, A., Johnston, J., Lavretsky, H., Stuart, M. J., et al. (2016). A meta-analysis of chemokines in major depression. Prog. Neuro-Psychopharmacol. Biol. Psychiatry 68, 1–8. doi: 10.1016/j.pnpbp.2016.02.006
Ferrari, A. J., Charlson, F. J., Norman, R. E., Flaxman, A. D., Patten, S. B., Vos, T., et al. (2013). The epidemiological modelling of major depressive disorder: application for the global burden of disease study 2010. PLoS One 8:e69637. doi: 10.1371/journal.pone.0069637
Ferreira, N. S., Tostes, R. C., Paradis, P., and Schiffrin, E. L. (2021). Aldosterone, inflammation, immune system, and hypertension. Am. J. Hypertens. 34, 15–27. doi: 10.1093/ajh/hpaa137
Feyissa, A. M., Chandran, A., Stockmeier, C. A., and Karolewicz, B. (2009). Reduced levels of NR2A and NR2B subunits of NMDA receptor and PSD-95 in the prefrontal cortex in major depression. Prog. Neuro-Psychopharmacol. Biol. Psychiatry 33, 70–75. doi: 10.1016/j.pnpbp.2008.10.005
Filiano, A. J., Gadani, S. P., and Kipnis, J. (2017). How and why do T cells and their derived cytokines affect the injured and healthy brain? Nat. Rev. Neurosci. 18:375. doi: 10.1038/nrn.2017.39
Fitzpatrick, Z., Frazer, G., Ferro, A., Clare, S., Bouladoux, N., Ferdinand, J., et al. (2020). Gut-educated IgA plasma cells defend the meningeal venous sinuses. Nature 587, 472–476. doi: 10.1038/s41586-020-2886-4
Fletcher, J. M., Lalor, S. J., Sweeney, C. M., Tubridy, N., and Mills, K. H. (2010). T cells in multiple sclerosis and experimental autoimmune encephalomyelitis. Clin. Exp. Immunol. 162, 1–11. doi: 10.1111/j.1365-2249.2010.04143.x
Foley, É. M., Parkinson, J. T., Mitchell, R. E., Turner, L., and Khandaker, G. M. (2023). Peripheral blood cellular immunophenotype in depression: a systematic review and meta-analysis. Mol. Psychiatry 28, 1004–1019. doi: 10.1038/s41380-022-01919-7
Fox, C., Merali, Z., and Harrison, C. (2006). Therapeutic and protective effect of environmental enrichment against psychogenic and neurogenic stress. Behav. Brain Res. 175, 1–8. doi: 10.1016/j.bbr.2006.08.016
Frank, M. G., Baratta, M. V., Sprunger, D. B., Watkins, L. R., and Maier, S. F. (2007). Microglia serve as a neuroimmune substrate for stress-induced potentiation of CNS pro-inflammatory cytokine responses. Brain Behav. Immun. 21, 47–59. doi: 10.1016/j.bbi.2006.03.005
Friedrich, M. J. (2017). Depression is the leading cause of disability around the world. JAMA 317:1517. doi: 10.1001/jama.2017.3826
Ganor, Y., and Levite, M. (2014). The neurotransmitter glutamate and human T cells: glutamate receptors and glutamate-induced direct and potent effects on normal human T cells, cancerous human leukemia and lymphoma T cells, and autoimmune human T cells. J. Neural Transm. 121, 983–1006. doi: 10.1007/s00702-014-1167-5
Gao, H. X., Campbell, S. R., Cui, M. H., Zong, P., Hee-Hwang, J., Gulinello, M., et al. (2009). Depression is an early disease manifestation in lupus-prone MRL/lpr mice. J. Neuroimmunol. 207, 45–56. doi: 10.1016/j.jneuroim.2008.11.009
Garvy, B. A., and Fraker, P. J. (1991). Suppression of the antigenic response of murine bone marrow B cells by physiological concentrations of glucocorticoids. Immunology 74, 519–523.
Gazal, M., Jansen, K., Souza, L. D., Oses, J. P., Magalhães, P. V., Pinheiro, R., et al. (2015). Association of interleukin-10 levels with age of onset and duration of illness in patients with major depressive disorder. Braz J Psychiatry 37, 296–302. doi: 10.1590/1516-4446-2014-1452
Godoy, L. D., Rossignoli, M. T., Delfino-Pereira, P., Garcia-Cairasco, N., and de Lima Umeoka, E. H. (2018). A comprehensive overview on stress neurobiology: basic concepts and clinical implications. Front. Behav. Neurosci. 12:127. doi: 10.3389/fnbeh.2018.00127
Goebel, M. U., and Mills, P. J. (2000). Acute psychological stress and exercise and changes in peripheral leukocyte adhesion molecule expression and density. Psychosom. Med. 62, 664–670. doi: 10.1097/00006842-200009000-00010
Gold, P. W., Pavlatou, M. G., Carlson, P. J., Luckenbaugh, D. A., Costello, R., Bonne, O., et al. (2012). Unmedicated, remitted patients with major depression have decreased serum immunoglobulin A. Neurosci. Lett. 520, 1–5. doi: 10.1016/j.neulet.2012.04.072
Gopinath, A., Mackie, P. M., Phan, L. T., Mirabel, R., Smith, A. R., Miller, E., et al. (2023). Who knew? Dopamine transporter activity is critical in innate and adaptive immune responses. Cells 12:269. doi: 10.3390/cells12020269
Greenberg, P. E., Fournier, A. A., Sisitsky, T., Pike, C. T., and Kessler, R. C. (2015). The economic burden of adults with major depressive disorder in the United States (2005 and 2010). J. Clin. Psychiatry 76, 155–162. doi: 10.4088/JCP.14m09298
Greenberg, P. E., Fournier, A. A., Sisitsky, T., Simes, M., Berman, R., Koenigsberg, S. H., et al. (2021). The economic burden of adults with major depressive disorder in the United States (2010 and 2018). PharmacoEconomics 39, 653–665. doi: 10.1007/s40273-021-01019-4
Gruver-Yates, A. L., Quinn, M. A., and Cidlowski, J. A. (2014). Analysis of glucocorticoid receptors and their apoptotic response to dexamethasone in male murine B cells during development. Endocrinology 155, 463–474. doi: 10.1210/en.2013-1473
Guilloux, J. P., Douillard-Guilloux, G., Kota, R., Wang, X., Gardier, A. M., Martinowich, K., et al. (2012). Molecular evidence for BDNF- and GABA-related dysfunctions in the amygdala of female subjects with major depression. Mol. Psychiatry 17, 1130–1142. doi: 10.1038/mp.2011.113
Gurfein, B. T., Davidenko, O., Premenko-Lanier, M., Milush, J. M., Acree, M., Dallman, M. F., et al. (2014). Environmental enrichment alters splenic immune cell composition and enhances secondary influenza vaccine responses in mice. Mol. Med. 20, 179–190. doi: 10.2119/molmed.2013.00158
Gurfein, B. T., Hasdemir, B., Milush, J. M., Touma, C., Palme, R., Nixon, D. F., et al. (2017). Enriched environment and stress exposure influence splenic B lymphocyte composition. PLoS One 12:e0180771. doi: 10.1371/journal.pone.0180771
Guyon, A. (2014). CXCL12 chemokine and GABA neurotransmitter systems crosstalk and their putative roles. Front. Cell. Neurosci. 5:115. doi: 10.3389/fncel.2014.00115
Hamaguchi, Y., Uchida, J., Cain, D. W., Venturi, G. M., Poe, J. C., Haas, K. M., et al. (2005). The peritoneal cavity provides a protective niche for B1 and conventional B lymphocytes during anti-CD20 immunotherapy in mice. J. Immunol. 174, 4389–4399. doi: 10.4049/jimmunol.174.7.4389
Hansen, N. (2022). NMDAR autoantibodies in psychiatric disease - an immunopsychiatric continuum and potential predisposition for disease pathogenesis. J Transl Autoimmun 5:100165. doi: 10.1016/j.jtauto.2022.100165
Harrer, C., Otto, F., Radlberger, R. F., Moser, T., Pilz, G., Wipfler, P., et al. (2022). The CXCL13/CXCR5 immune Axis in health and disease-implications for intrathecal B cell activities in Neuroinflammation. Cells 11:2649. doi: 10.3390/cells11172649
Hauser, S. L., Waubant, E., Arnold, D. L., Vollmer, T., Antel, J., Fox, R. J., et al. (2008). B-cell depletion with rituximab in relapsing-remitting multiple sclerosis. N. Engl. J. Med. 358, 676–688. doi: 10.1056/NEJMoa0706383
Henning, U., Krieger, K., Loeffler, S., Rivas, F., Orozco, G., de Castro, M. G., et al. (2005). Increased levels of glucocorticoid receptors and enhanced glucocorticoid receptor auto-regulation after hydrocortisone challenge in B-lymphoblastoids from patients with affective disorders. Psychoneuroendocrinology 30, 325–332. doi: 10.1016/j.psyneuen.2004.09.004
Herkenham, M., and Kigar, S. L. (2017). Contributions of the adaptive immune system to mood regulation: mechanisms and pathways of neuroimmune interactions. Prog. Neuro-Psychopharmacol. Biol. Psychiatry 79, 49–57. doi: 10.1016/j.pnpbp.2016.09.003
Hernandez, M. E., Martinez-Fong, D., Perez-Tapia, M., Estrada-Garcia, I., Estrada-Parra, S., and Pavón, L. (2010). Evaluation of the effect of selective serotonin-reuptake inhibitors on lymphocyte subsets in patients with a major depressive disorder. Eur. Neuropsychopharmacol. 20, 88–95. doi: 10.1016/j.euroneuro.2009.11.005
Herr, N., Bode, C., and Duerschmied, D. (2017). The effects of serotonin in immune cells. Front Cardiovasc Med 4:48. doi: 10.3389/fcvm.2017.00048
Higashida, H., Liang, M., Yoshihara, T., Akther, S., Fakhrul, A., Stanislav, C., et al. (2017). An immunohistochemical, enzymatic, and behavioral study of CD157/BST-1 as a neuroregulator. BMC Neurosci. 18:35. doi: 10.1186/s12868-017-0350-7
Hillis, J., O'Dwyer, M., and Gorman, A. M. (2016). Neurotrophins and B-cell malignancies. Cell. Mol. Life Sci. 73, 41–56. doi: 10.1007/s00018-015-2046-4
Hoffman, W., Lakkis, F. G., and Chalasani, G. (2016). B cells, antibodies, and more. Clin. J. Am. Soc. Nephrol. 11, 137–154. doi: 10.2215/CJN.09430915
Homann, D., Tishon, A., Berger, D. P., Weigle, W. O., von Herrath, M. G., and Oldstone, M. B. (1998). Evidence for an underlying CD4 helper and CD8 T-cell defect in B-cell-deficient mice: failure to clear persistent virus infection after adoptive immunotherapy with virus-specific memory cells from muMT/muMT mice. J. Virol. 72, 9208–9216. doi: 10.1128/JVI.72.11.9208-9216.1998
Hua, C., Audo, R., Yeremenko, N., Baeten, D., Hahne, M., Combe, B., et al. (2016). A proliferation inducing ligand (APRIL) promotes IL-10 production and regulatory functions of human B cells. J. Autoimmun. 73, 64–72. doi: 10.1016/j.jaut.2016.06.002
Huang, M. W., Stock, A. D., and Putterman, C. (2021). CXCL13 neutralization attenuates neuropsychiatric manifestations in lupus-prone mice. Front. Immunol. 12:763065. doi: 10.3389/fimmu.2021.763065
Igarashi, H., Medina, K. L., Yokota, T., Rossi, M. I. D., Sakaguchi, N., Comp, P. C., et al. (2005). Early lymphoid progenitors in mouse and man are highly sensitive to glucocorticoids. Int. Immunol. 17, 501–511. doi: 10.1093/intimm/dxh230
Iken, K., Chheng, S., Fargin, A., Goulet, A. C., and Kouassi, E. (1995). Serotonin upregulates mitogen-stimulated B lymphocyte proliferation through 5-HT1A receptors. Cell. Immunol. 163, 1–9. doi: 10.1006/cimm.1995.1092
Ince, L. M., Weber, J., and Scheiermann, C. (2018). Control of leukocyte trafficking by stress-associated hormones. Front. Immunol. 9:3143. doi: 10.3389/fimmu.2018.03143
Irani, D. N. (2016). Regulated production of CXCL13 within the central nervous system. J Clin Cell Immunol 7:460. doi: 10.4172/2155-9899.1000460
Itoh, M., Ishihara, K., Hiroi, T., Lee, B. O., Maeda, H., Iijima, H., et al. (1998). Deletion of bone marrow stromal cell antigen-1 (CD157) gene impaired systemic thymus independent-2 antigen-induced IgG3 and mucosal TD antigen-elicited IgA responses. J. Immunol. 161, 3974–3983.
Jain, R. W., and Yong, V. W. (2022). B cells in central nervous system disease: diversity, locations and pathophysiology. Nat. Rev. Immunol. 22, 513–524. doi: 10.1038/s41577-021-00652-6
Jauhar, S., Cowen, P. J., and Browning, M. (2023). Fifty years on: serotonin and depression. J. Psychopharmacol. 37, 237–241. doi: 10.1177/02698811231161813
Jiang, B. C., Cao, D. L., Zhang, X., Zhang, Z. J., He, L. N., Li, C. H., et al. (2016). CXCL13 drives spinal astrocyte activation and neuropathic pain via CXCR5. J. Clin. Invest. 126, 745–761. doi: 10.1172/JCI81950
Johnson, J. D., O'Connor, K. A., Deak, T., Stark, M., Watkins, L. R., and Maier, S. F. (2002). Prior stressor exposure sensitizes LPS-induced cytokine production. Brain Behav. Immun. 16, 461–476. doi: 10.1006/brbi.2001.0638
Joyce, P. R., Hawes, C. R., Mulder, R. T., Sellman, J. D., Wilson, D. A., and Boswell, D. R. (1992). Elevated levels of acute phase plasma proteins in major depression. Biol. Psychiatry 32, 1035–1041. doi: 10.1016/0006-3223(92)90065-8
Kappos, L., Hartung, H. P., Freedman, M. S., Boyko, A., Radü, E. W., Mikol, D. D., et al. (2014). Atacicept in multiple sclerosis (ATAMS): a randomised, placebo-controlled, double-blind, phase 2 trial. Lancet Neurol. 13, 353–363. doi: 10.1016/S1474-4422(14)70028-6
Kerage, D., Sloan, E. K., Mattarollo, S. R., and McCombe, P. A. (2019). Interaction of neurotransmitters and neurochemicals with lymphocytes. J. Neuroimmunol. 332, 99–111. doi: 10.1016/j.jneuroim.2019.04.006
Kerschensteiner, M., Gallmeier, E., Behrens, L., Leal, V. V., Misgeld, T., Klinkert, W. E., et al. (1999). Activated human T cells, B cells, and monocytes produce brain-derived neurotrophic factor in vitro and in inflammatory brain lesions: a neuroprotective role of inflammation? J. Exp. Med. 189, 865–870. doi: 10.1084/jem.189.5.865
Khan, S. R., van der Burgh, A. C., Peeters, R. P., van Hagen, P. M., Dalm, V. A. S. H., and Chaker, L. (2021). Determinants of serum immunoglobulin levels: a systematic review and meta-analysis. Front. Immunol. 12:664526. doi: 10.3389/fimmu.2021.664526
Khedri, M., Samei, A., Fasihi-Ramandi, M., and Taheri, R. A. (2020). The immunopathobiology of T cells in stress condition: a review. Cell Stress Chaperones 25, 743–752. doi: 10.1007/s12192-020-01105-0
Kiecolt-Glaser, J. K., Heffner, K. L., Glaser, R., Malarkey, W. B., Porter, K., Atkinson, C., et al. (2009). How stress and anxiety can alter immediate and late phase skin test responses in allergic rhinitis. Psychoneuroendocrinology 34, 670–680. doi: 10.1016/j.psyneuen.2008.11.010
Kin, N. W., and Sanders, V. M. (2006). It takes nerve to tell T and B cells what to do. J. Leukoc. Biol. 79, 1093–1104. doi: 10.1189/jlb.1105625
Kivisäkk, P., Mahad, D. J., Callahan, M. K., Sikora, K., Trebst, C., Tucky, B., et al. (2004). Expression of CCR7 in multiple sclerosis: implications for CNS immunity. Ann. Neurol. 55, 627–638. doi: 10.1002/ana.20049
Köhler-Forsberg, O., Buttenschøn, H. N., Tansey, K. E., Maier, W., Hauser, J., Dernovsek, M. Z., et al. (2017). Association between C-reactive protein (CRP) with depression symptom severity and specific depressive symptoms in major depression. Brain Behav. Immun. 62, 344–350. doi: 10.1016/j.bbi.2017.02.020
Köhler‐Forsberg, O. N., Lydholm, C., Hjorthøj, C., Nordentoft, M., Mors, O., and Benros, M. E. (2019). Efficacy of anti-inflammatory treatment on major depressive disorder or depressive symptoms: meta-analysis of clinical trials. Acta Psychiatr. Scand. 139, 404–419. doi: 10.1111/acps.13016
Kohm, A. P., Tang, Y., Sanders, V. M., and Jones, S. B. (2000). Activation of antigen-specific CD4+ Th2 cells and B cells in vivo increases norepinephrine release in the spleen and bone marrow. J. Immunol. 165, 725–733. doi: 10.4049/jimmunol.165.2.725
Kolan, S. S., Lidström, T., Mediavilla, T., Dernstedt, A., Degerman, S., Hultdin, M., et al. (2019). Growth-inhibition of cell lines derived from B cell lymphomas through antagonism of serotonin receptor signaling. Sci. Rep. 9:4276. doi: 10.1038/s41598-019-40825-x
Koolschijn, P. C., van Haren, N. E., Lensvelt-Mulders, G. J., Hulshoff Pol, H. E., and Kahn, R. S. (2009). Brain volume abnormalities in major depressive disorder: a meta-analysis of magnetic resonance imaging studies. Hum. Brain Mapp. 30, 3719–3735. doi: 10.1002/hbm.20801
Korf, J. M., Honarpisheh, P., Mohan, E. C., Banerjee, A., Blasco-Conesa, M. P., Honarpisheh, P., et al. (2022). CD11b(high) B cells increase after stroke and regulate microglia. J. Immunol. 209, 288–300. doi: 10.4049/jimmunol.2100884
Korin, B., Ben-Shaanan, T. L., Schiller, M., Dubovik, T., Azulay-Debby, H., Boshnak, N. T., et al. (2017). High-dimensional, single-cell characterization of the brain's immune compartment. Nat. Neurosci. 20, 1300–1309. doi: 10.1038/nn.4610
Kovacs, W. J. (2014). To B or not to B? Glucocorticoid impact on B lymphocyte fate and function. Endocrinology 155, 339–342. doi: 10.1210/en.2013-2085
Kronfol, Z., and House, J. D. (1989). Lymphocyte mitogenesis, immunoglobulin and complement levels in depressed patients and normal controls. Acta Psychiatr. Scand. 80, 142–147. doi: 10.1111/j.1600-0447.1989.tb01316.x
Krumbholz, M., Theil, D., Derfuss, T., Rosenwald, A., Schrader, F., Monoranu, C. M., et al. (2005). BAFF is produced by astrocytes and up-regulated in multiple sclerosis lesions and primary central nervous system lymphoma. J. Exp. Med. 201, 195–200. doi: 10.1084/jem.20041674
Krumbholz, M., Theil, D., Steinmeyer, F., Cepok, S., Hemmer, B., Hofbauer, M., et al. (2007). CCL19 is constitutively expressed in the CNS, up-regulated in neuroinflammation, active and also inactive multiple sclerosis lesions. J. Neuroimmunol. 190, 72–79. doi: 10.1016/j.jneuroim.2007.07.024
Kuhn, C., and Weiner, H. L. (2016). Therapeutic anti-CD3 monoclonal antibodies: from bench to bedside. Immunotherapy 8, 889–906. doi: 10.2217/imt-2016-0049
Kwon, D. Y., Xu, B., Hu, P., Zhao, Y.-T., Beagan, J. A., Nofziger, J. H., et al. (2022). Neuronal Yin Yang1 in the prefrontal cortex regulates transcriptional and behavioral responses to chronic stress in mice. Nat. Commun. 13:55. doi: 10.1038/s41467-021-27571-3
Lalor, S. J., and Segal, B. M. (2010). Lymphoid chemokines in the CNS. J. Neuroimmunol. 224, 56–61. doi: 10.1016/j.jneuroim.2010.05.017
Laumet, G., Edralin, J. D., Chiang, A. C., Dantzer, R., Heijnen, C. J., and Kavelaars, A. (2018). Resolution of inflammation-induced depression requires T lymphocytes and endogenous brain interleukin-10 signaling. Neuropsychopharmacology 43, 2597–2605. doi: 10.1038/s41386-018-0154-1
Lee, D. S. W., Rojas, O. L., and Gommerman, J. L. (2021). B cell depletion therapies in autoimmune disease: advances and mechanistic insights. Nat. Rev. Drug Discov. 20, 179–199. doi: 10.1038/s41573-020-00092-2
Leighton, S. P., Nerurkar, L., Krishnadas, R., Johnman, C., Graham, G. J., and Cavanagh, J. (2018). Chemokines in depression in health and in inflammatory illness: a systematic review and meta-analysis. Mol. Psychiatry 23, 48–58. doi: 10.1038/mp.2017.205
Leo, R., Di Lorenzo, G., Tesauro, M., Razzini, C., Forleo, G. B., Chiricolo, G., et al. (2006). Association between enhanced soluble CD40 ligand and proinflammatory and prothrombotic states in major depressive disorder: pilot observations on the effects of selective serotonin reuptake inhibitor therapy. J. Clin. Psychiatry 67, 1760–1766. doi: 10.4088/jcp.v67n1114
Leypoldt, F., Höftberger, R., Titulaer, M. J., Armangue, T., Gresa-Arribas, N., Jahn, H., et al. (2015). Investigations on CXCL13 in anti-N-methyl-D-aspartate receptor encephalitis: a potential biomarker of treatment response. JAMA Neurol. 72, 180–186. doi: 10.1001/jamaneurol.2014.2956
Li, H., Chang, H., Song, X., Liu, W., Li, L., Wang, L., et al. (2019). Integrative analyses of major histocompatibility complex loci in the genome-wide association studies of major depressive disorder. Neuropsychopharmacology 44, 1552–1561. doi: 10.1038/s41386-019-0346-3
Li, M., and Ransohoff, R. M. (2008). Multiple roles of chemokine CXCL12 in the central nervous system: a migration from immunology to neurobiology. Prog. Neurobiol. 84, 116–131. doi: 10.1016/j.pneurobio.2007.11.003
Li, K., Yu, W., Cao, R., Zhu, Z., and Zhao, G. (2017). Microglia-mediated BAFF-BAFFR ligation promotes neuronal survival in brain ischemia injury. Neuroscience 363, 87–96. doi: 10.1016/j.neuroscience.2017.09.007
Lill-Elghanian, D., Schwartz, K., King, L., and Fraker, P. (2002). Glucocorticoid-induced apoptosis in early B cells from human bone marrow. Exp. Biol. Med. (Maywood) 227, 763–770. doi: 10.1177/153537020222700907
Liu, Y. Z., Wang, Y. X., and Jiang, C. L. (2017). Inflammation: the common pathway of stress-related diseases. Front. Hum. Neurosci. 11:316. doi: 10.3389/fnhum.2017.00316
Lobo-Silva, D., Carriche, G. M., Castro, A. G., Roque, S., and Saraiva, M. (2016). Balancing the immune response in the brain: IL-10 and its regulation. J. Neuroinflammation 13:297. doi: 10.1186/s12974-016-0763-8
Lokensgard, J. R., Mutnal, M. B., Prasad, S., Sheng, W., and Hu, S. (2016). Glial cell activation, recruitment, and survival of B-lineage cells following MCMV brain infection. J. Neuroinflammation 13:114. doi: 10.1186/s12974-016-0582-y
Londoño, A. C., and Mora, C. A. (2018). Role of CXCL13 in the formation of the meningeal tertiary lymphoid organ in multiple sclerosis. F1000Res 7:514. doi: 10.12688/f1000research.14556.3
Lopatina, O. L., Komleva, Y. K., Malinovskaya, N. A., Panina, Y. A., Morgun, A. V., and Salmina, A. B. (2020). CD157 and brain immune system in (Patho)physiological conditions: focus on brain plasticity. Front. Immunol. 11:585294. doi: 10.3389/fimmu.2020.585294
Lopatina, O., Yoshihara, T., Nishimura, T., Zhong, J., Akther, S., Fakhrul, A. A. K. M., et al. (2014). Anxiety- and depression-like behavior in mice lacking the CD157/BST1 gene, a risk factor for Parkinson's disease. Front. Behav. Neurosci. 8:133. doi: 10.3389/fnbeh.2014.00133
Luykx, J. J., Laban, K. G., van den Heuvel, M. P., Boks, M. P., Mandl, R. C., Kahn, R. S., et al. (2012). Region and state specific glutamate downregulation in major depressive disorder: a meta-analysis of (1)H-MRS findings. Neurosci. Biobehav. Rev. 36, 198–205. doi: 10.1016/j.neubiorev.2011.05.014
Lynall, M. E., Kigar, S. L., Lehmann, M. L., DePuyt, A. E., Tuong, Z. K., Listwak, S. J., et al. (2021). B-cells are abnormal in psychosocial stress and regulate meningeal myeloid cell activation. Brain Behav. Immun. 97, 226–238. doi: 10.1016/j.bbi.2021.08.002
Ma, N., Liu, X., Xing, C., Wang, X., Wei, Y., Han, G., et al. (2015). Ligation of metabotropic glutamate receptor 3 (Grm3) ameliorates lupus-like disease by reducing B cells. Clin. Immunol. 160, 142–154. doi: 10.1016/j.clim.2015.05.016
Maes, M. (2011). Depression is an inflammatory disease, but cell-mediated immune activation is the key component of depression. Prog. Neuro-Psychopharmacol. Biol. Psychiatry 35, 664–675. doi: 10.1016/j.pnpbp.2010.06.014
Maes, M., Bosmans, E., Suy, E., Vandervorst, C., De Jonckheere, C., and Raus, J. (1990). Immune disturbances during major depression: upregulated expression of interleukin-2 receptors. Neuropsychobiology 24, 115–120.
Maes, M., Lambrechts, J., Bosmans, E., Jacobs, J., Suy, E., Vandervorst, C., et al. (1992). Evidence for a systemic immune activation during depression: results of leukocyte enumeration by flow cytometry in conjunction with monoclonal antibody staining. Psychol. Med. 22, 45–53. doi: 10.1017/s0033291700032712
Maes, M., van Bockstaele, D. R., Gastel, A. V., Song, C., Schotte, C., Neels, H., et al. (1999). The effects of psychological stress on leukocyte subset distribution in humans: evidence of immune activation. Neuropsychobiology 39, 1–9. doi: 10.1159/000026552
Magliozzi, R., Howell, O., Vora, A., Serafini, B., Nicholas, R., Puopolo, M., et al. (2007). Meningeal B-cell follicles in secondary progressive multiple sclerosis associate with early onset of disease and severe cortical pathology. Brain 130, 1089–1104. doi: 10.1093/brain/awm038
Maheshwari, S., Dwyer, L. J., and Sîrbulescu, R. F. (2023). Inflammation and immunomodulation in central nervous system injury - B cells as a novel therapeutic opportunity. Neurobiol. Dis. 180:106077. doi: 10.1016/j.nbd.2023.106077
Malone, M. K., Ujas, T. A., Britsch, D. R. S., Cotter, K. M., Poinsatte, K., and Stowe, A. M. (2023). The immunopathology of B lymphocytes during stroke-induced injury and repair. Semin. Immunopathol. 45, 315–327. doi: 10.1007/s00281-022-00971-3
Marazziti, D., Catena Dell'osso, M., Baroni, S., Masala, I., Dell'Osso, B., Consoli, G., et al. (2010). Alterations of the dopamine transporter in resting lymphocytes of patients with different psychotic disorders. Psychiatry Res. 175, 54–57. doi: 10.1016/j.psychres.2009.03.009
Marchetti, L., and Engelhardt, B. (2020). Immune cell trafficking across the blood-brain barrier in the absence and presence of neuroinflammation. Vasc. Biol. 2, H1–h18. doi: 10.1530/vb-19-0033
Marella, M., Yao, X., Carreira, V., Bustamante, M. F., Clark, H. B., Jackson, C. C., et al. (2022). Comprehensive BCMA expression profiling in adult Normal human brain suggests a low risk of on-target neurotoxicity in BCMA-targeting multiple myeloma therapy. J. Histochem. Cytochem. 70, 273–287. doi: 10.1369/00221554221079579
Martinez-Hernandez, E., Horvath, J., Shiloh-Malawsky, Y., Sangha, N., Martinez-Lage, M., and Dalmau, J. (2011). Analysis of complement and plasma cells in the brain of patients with anti-NMDAR encephalitis. Neurology 77, 589–593. doi: 10.1212/WNL.0b013e318228c136
Mata, S., Urbina, M., Manzano, E., Ortiz, T., and Lima, L. (2005). Noradrenaline transporter and its turnover rate are decreased in blood lymphocytes of patients with major depression. J. Neuroimmunol. 170, 134–140. doi: 10.1016/j.jneuroim.2005.08.007
Matos-Gomes, N., Katsurayama, M., Makimoto, F. H., Santana, L. L., Paredes-Garcia, E., Becker, M. A., et al. (2010). Psychological stress and its influence on salivary flow rate, total protein concentration and IgA, IgG and IgM titers. Neuroimmunomodulation 17, 396–404. doi: 10.1159/000292064
Matthews, K. A., Caggiula, A. R., McAllister, C. G., Berga, S. L., Owens, J. F., Flory, J. D., et al. (1995). Sympathetic reactivity to acute stress and immune response in women. Psychosom. Med. 57, 564–571. doi: 10.1097/00006842-199511000-00009
McEwen, B. S. (2017). Neurobiological and systemic effects of chronic stress. Chronic Stress 1:2470547017692328. doi: 10.1177/2470547017692328
McEwen, B. S., and Karatsoreos, I. N. (2020). “What is stress?” in Stress challenges and immunity in space: from mechanisms to monitoring and preventive strategies. ed. A. Choukèr (Cham: Springer International Publishing)
McGregor, B. A., Antoni, M. H., Ceballos, R. M., and Blomberg, B. B. (2008). Very low CD19+ B-lymphocyte percentage is associated with high levels of academic stress among healthy graduate students. Stress. Health 24, 413–418. doi: 10.1002/smi.1188
McKenna, F., McLaughlin, P. J., Lewis, B. J., Sibbring, G. C., Cummerson, J. A., Bowen-Jones, D., et al. (2002). Dopamine receptor expression on human T- and B-lymphocytes, monocytes, neutrophils, eosinophils and NK cells: a flow cytometric study. J. Neuroimmunol. 132, 34–40. doi: 10.1016/s0165-5728(02)00280-1
McKim, D. B., Yin, W., Wang, Y., Cole, S. W., Godbout, J. P., and Sheridan, J. F. (2018). Social stress mobilizes hematopoietic stem cells to establish persistent splenic Myelopoiesis. Cell Rep. 25, 2552–2562.e3. doi: 10.1016/j.celrep.2018.10.102
McWilliams, T. G., Howard, L., Wyatt, S., and Davies, A. M. (2017). TNF superfamily member APRIL enhances midbrain dopaminergic axon growth and contributes to the nigrostriatal projection in vivo. Exp. Neurol. 298, 97–103. doi: 10.1016/j.expneurol.2017.09.007
Medina-Rodriguez, E. M., Cruz, A. A., De Abreu, J. C., and Beurel, E. (2023). Stress, inflammation, microbiome and depression. Pharmacol. Biochem. Behav. 227-228:173561. doi: 10.1016/j.pbb.2023.173561
Medina-Rodriguez, E. M., Han, D., Lowell, J., and Beurel, E. (2023). Stress promotes the infiltration of peripheral immune cells to the brain. Brain Behav. Immun. 111, 412–423. doi: 10.1016/j.bbi.2023.05.003
Meinl, E., Krumbholz, M., and Hohlfeld, R. (2006). B lineage cells in the inflammatory central nervous system environment: migration, maintenance, local antibody production, and therapeutic modulation. Ann. Neurol. 59, 880–892. doi: 10.1002/ana.20890
Meredith, E. J., Holder, M. J., Chamba, A., Challa, A., Drake-Lee, A., Bunce, C. M., et al. (2005). The serotonin transporter (SLC6A4) is present in B-cell clones of diverse malignant origin: probing a potential anti-tumor target for psychotropics. FASEB J. 19, 1187–1189. doi: 10.1096/fj.04-3477fje
Mesquita, A. R., Correia-Neves, M., Roque, S., Castro, A. G., Vieira, P., Pedrosa, J., et al. (2008). IL-10 modulates depressive-like behavior. J. Psychiatr. Res. 43, 89–97. doi: 10.1016/j.jpsychires.2008.02.004
Michel, L., Grasmuck, C., Charabati, M., Lécuyer, M. A., Zandee, S., Dhaeze, T., et al. (2019). Activated leukocyte cell adhesion molecule regulates B lymphocyte migration across central nervous system barriers. Sci. Transl. Med. 11:eaaw0475. doi: 10.1126/scitranslmed.aaw0475
Milenkovic, V. M., Stanton, E. H., Nothdurfter, C., Rupprecht, R., and Wetzel, C. H. (2019). The role of chemokines in the pathophysiology of major depressive disorder. Int. J. Mol. Sci. 20:2283. doi: 10.3390/ijms20092283
Monson, N. L., Ortega, S. B., Ireland, S. J., Meeuwissen, A. J. M., Chen, D., Plautz, E. J., et al. (2014). Repetitive hypoxic preconditioning induces an immunosuppressed B cell phenotype during endogenous protection from stroke. J. Neuroinflammation 11:22. doi: 10.1186/1742-2094-11-22
Moriguchi, S., Takamiya, A., Noda, Y., Horita, N., Wada, M., Tsugawa, S., et al. (2019). Glutamatergic neurometabolite levels in major depressive disorder: a systematic review and meta-analysis of proton magnetic resonance spectroscopy studies. Mol. Psychiatry 24, 952–964. doi: 10.1038/s41380-018-0252-9
Murray, S. E., Lallman, H. R., Heard, A. D., Rittenberg, M. B., and Stenzel-Poore, M. P. (2001). A genetic model of stress displays decreased lymphocytes and impaired antibody responses without altered susceptibility to Streptococcus pneumoniae1. J. Immunol. 167, 691–698. doi: 10.4049/jimmunol.167.2.691
Myung, W., Lim, S. W., Woo, H. I., Park, J. H., Shim, S., Lee, S. Y., et al. (2016). Serum cytokine levels in major depressive disorder and its role in antidepressant response. Psychiatry Investig. 13, 644–651. doi: 10.4306/pi.2016.13.6.644
Negron, A., Stüve, O., and Forsthuber, T. G. (2020). Ectopic lymphoid follicles in multiple sclerosis: centers for disease control? Front. Neurol. 11:607766. doi: 10.3389/fneur.2020.607766
Nelson, P. A., Sage, J. R., Wood, S. C., Davenport, C. M., Anagnostaras, S. G., and Boulanger, L. M. (2013). MHC class I immune proteins are critical for hippocampus-dependent memory and gate NMDAR-dependent hippocampal long-term depression. Learn. Mem. 20, 505–517. doi: 10.1101/lm.031351.113
Neubauer, H., Petrak, F., Zahn, D., Pepinghege, F., Hägele, A. K., Pirkl, P. A., et al. (2013). Newly diagnosed depression is associated with increased beta-thromboglobulin levels and increased expression of platelet activation markers and platelet derived CD40-CD40L. J. Psychiatr. Res. 47, 865–871. doi: 10.1016/j.jpsychires.2013.03.011
Nguyen, A. V., Young, K. A., and Bourgeois, J. A. (2020). Psychiatric Prodrome in anti-NMDAR-associated encephalopathy: clinical and pathophysiologic considerations. Prim Care Companion CNS Disord 22:19r02563. doi: 10.4088/PCC.19r02563
Nishiyama, A., Shimizu, T., Sherafat, A., and Richardson, W. D. (2021). Life-long oligodendrocyte development and plasticity. Semin. Cell Dev. Biol. 116, 25–37. doi: 10.1016/j.semcdb.2021.02.004
Nolan, J. P. (2022). The evolution of spectral flow cytometry. Cytometry A 101, 812–817. doi: 10.1002/cyto.a.24566
Noor, S., and Wilson, E. H. (2012). Role of C-C chemokine receptor type 7 and its ligands during neuroinflammation. J. Neuroinflammation 9:77. doi: 10.1186/1742-2094-9-77
Nugent, A. L., Houghtling, R. A., and Bayer, B. M. (2011). Morphine suppresses MHC-II expression on circulating B lymphocytes via activation of the HPA. J. Neuroimmune Pharmacol. 6, 130–141. doi: 10.1007/s11481-010-9218-7
O'Connor, J. C., Lawson, M. A., André, C., Moreau, M., Lestage, J., Castanon, N., et al. (2009). Lipopolysaccharide-induced depressive-like behavior is mediated by indoleamine 2,3-dioxygenase activation in mice. Mol. Psychiatry 14, 511–522. doi: 10.1038/sj.mp.4002148
Ogłodek, E. A., Szota, A., Just, M. J., Moś, D., and Araszkiewicz, A. (2014). Comparison of chemokines (CCL-5 and SDF-1), chemokine receptors (CCR-5 and CXCR-4) and IL-6 levels in patients with different severities of depression. Pharmacol. Rep. 66, 920–926. doi: 10.1016/j.pharep.2014.06.001
Ortega, S. B., Torres, V. O., Latchney, S. E., Whoolery, C. W., Noorbhai, I. Z., Poinsatte, K., et al. (2020). B cells migrate into remote brain areas and support neurogenesis and functional recovery after focal stroke in mice. Proc. Natl. Acad. Sci. U. S. A. 117, 4983–4993. doi: 10.1073/pnas.1913292117
Osório, C., Chacón, P. J., White, M., Kisiswa, L., Wyatt, S., Rodríguez-Tébar, A., et al. (2014). Selective regulation of axonal growth from developing hippocampal neurons by tumor necrosis factor superfamily member APRIL. Mol. Cell. Neurosci. 59, 24–36. doi: 10.1016/j.mcn.2014.01.002
Otsuki, K., Uchida, S., Watanuki, T., Wakabayashi, Y., Fujimoto, M., Matsubara, T., et al. (2008). Altered expression of neurotrophic factors in patients with major depression. J. Psychiatr. Res. 42, 1145–1153. doi: 10.1016/j.jpsychires.2008.01.010
Pan, H., Steixner-Kumar, A. A., Seelbach, A., Deutsch, N., Ronnenberg, A., Tapken, D., et al. (2021). Multiple inducers and novel roles of autoantibodies against the obligatory NMDAR subunit NR1: a translational study from chronic life stress to brain injury. Mol. Psychiatry 26, 2471–2482. doi: 10.1038/s41380-020-0672-1
Papa, I., Saliba, D., Ponzoni, M., Bustamante, S., Canete, P. F., Gonzalez-Figueroa, P., et al. (2017). TFH-derived dopamine accelerates productive synapses in germinal centres. Nature 547, 318–323. doi: 10.1038/nature23013
Pavlov, V. A., Chavan, S. S., and Tracey, K. J. (2018). Molecular and functional neuroscience in immunity. Annu. Rev. Immunol. 36, 783–812. doi: 10.1146/annurev-immunol-042617-053158
Pavón, L., Sandoval-López, G., Eugenia Hernández, M., Loría, F., Estrada, I., Pérez, M., et al. (2006). Th2 cytokine response in major depressive disorder patients before treatment. J. Neuroimmunol. 172, 156–165. doi: 10.1016/j.jneuroim.2005.08.014
Peña, S., Baccichet, E., Urbina, M., Carreira, I., and Lima, L. (2005). Effect of mirtazapine treatment on serotonin transporter in blood peripheral lymphocytes of major depression patients. Int. Immunopharmacol. 5, 1069–1076. doi: 10.1016/j.intimp.2005.02.005
Peng, Z., Peng, S., Lin, K., Zhao, B., Wei, L., Tuo, Q., et al. (2022). Chronic stress-induced depression requires the recruitment of peripheral Th17 cells into the brain. J. Neuroinflammation 19:186. doi: 10.1186/s12974-022-02543-6
Petersone, L., Edner, N. M., Ovcinnikovs, V., Heuts, F., Ross, E. M., Ntavli, E., et al. (2018). T cell/B cell collaboration and autoimmunity: an intimate relationship. Front. Immunol. 9:1941. doi: 10.3389/fimmu.2018.01941
Pilz, G., Steger, R., Wipfler, P., Otto, F., Afazel, S., Haschke-Becher, E., et al. (2020). Beyond LNB: real life data on occurrence and extent of CSF CXCL13 in neuroinflammatory diseases. J. Neuroimmunol. 338:577087. doi: 10.1016/j.jneuroim.2019.577087
Plantone, D., Pardini, M., Locci, S., Nobili, F., and De Stefano, N. (2022). B lymphocytes in Alzheimer's disease-A comprehensive review. J. Alzheimers Dis. 88, 1241–1262. doi: 10.3233/JAD-220261
Poller, W. C., Downey, J., Mooslechner, A. A., Khan, N., Li, L., Chan, C. T., et al. (2022). Brain motor and fear circuits regulate leukocytes during acute stress. Nature 607, 578–584. doi: 10.1038/s41586-022-04890-z
Prado, C., Osorio-Barrios, F., Falcón, P., Espinoza, A., Saez, J. J., Yuseff, M. I., et al. (2021). Dopaminergic stimulation leads B-cell infiltration into the central nervous system upon autoimmunity. J. Neuroinflammation 18:292. doi: 10.1186/s12974-021-02338-1
Pröbstel, A. K., Zhou, X., Baumann, R., Wischnewski, S., Kutza, M., Rojas, O. L., et al. (2020). Gut microbiota-specific IgA(+) B cells traffic to the CNS in active multiple sclerosis. Sci. Immunol. 5:eabc7191. doi: 10.1126/sciimmunol.abc7191
Pryce, C. R., and Fontana, A. (2017). Depression in autoimmune diseases. Curr. Top. Behav. Neurosci. 31, 139–154. doi: 10.1007/7854_2016_7
Rajkowska, G., O'Dwyer, G., Teleki, Z., Stockmeier, C. A., and Miguel-Hidalgo, J. J. (2007). GABAergic neurons immunoreactive for calcium binding proteins are reduced in the prefrontal cortex in major depression. Neuropsychopharmacology 32, 471–482. doi: 10.1038/sj.npp.1301234
Rattazzi, L., Piras, G., Ono, M., Deacon, R., Pariante, C. M., and D'Acquisto, F. (2013). CD4(+) but not CD8(+) T cells revert the impaired emotional behavior of immunocompromised RAG-1-deficient mice. Transl. Psychiatry 3:e280. doi: 10.1038/tp.2013.54
Rayasam, A., Hsu, M., Kijak, J. A., Kissel, L., Hernandez, G., Sandor, M., et al. (2018). Immune responses in stroke: how the immune system contributes to damage and healing after stroke and how this knowledge could be translated to better cures? Immunology 154, 363–376. doi: 10.1111/imm.12918
Remus, J. L., and Dantzer, R. (2016). Inflammation models of depression in rodents: relevance to psychotropic drug discovery. Int. J. Neuropsychopharmacol. 19:pyw028. doi: 10.1093/ijnp/pyw028
Ren, X., Akiyoshi, K., Dziennis, S., Vandenbark, A. A., Herson, P. S., Hurn, P. D., et al. (2011). Regulatory B cells limit CNS inflammation and neurologic deficits in murine experimental stroke. J. Neurosci. 31, 8556–8563. doi: 10.1523/JNEUROSCI.1623-11.2011
Rock, K. L., Reits, E., and Neefjes, J. (2016). Present yourself! By MHC class I and MHC class II molecules. Trends Immunol. 37, 724–737. doi: 10.1016/j.it.2016.08.010
Romeo, B., Choucha, W., Fossati, P., and Rotge, J. Y. (2018). Meta-analysis of central and peripheral γ-aminobutyric acid levels in patients with unipolar and bipolar depression. J. Psychiatry Neurosci. 43, 58–66. doi: 10.1503/jpn.160228
Romero-Sanchiz, P., Nogueira-Arjona, R., Araos, P., Serrano, A., Barrios, V., Argente, J., et al. (2020). Variation in chemokines plasma concentrations in primary care depressed patients associated with internet-based cognitive-behavioral therapy. Sci. Rep. 10:1078. doi: 10.1038/s41598-020-57967-y
Roumestan, C., Michel, A., Bichon, F., Portet, K., Detoc, M., Henriquet, C., et al. (2007). Anti-inflammatory properties of desipramine and fluoxetine. Respir. Res. 8:35. doi: 10.1186/1465-9921-8-35
Russo, S. J., and Nestler, E. J. (2013). The brain reward circuitry in mood disorders. Nat. Rev. Neurosci. 14, 609–625. doi: 10.1038/nrn3381
Sánchez-Alcañiz, J. A., Haege, S., Mueller, W., Pla, R., Mackay, F., Schulz, S., et al. (2011). Cxcr7 controls neuronal migration by regulating chemokine responsiveness. Neuron 69, 77–90. doi: 10.1016/j.neuron.2010.12.006
Sarawagi, A., Soni, N. D., and Patel, A. B. (2021). Glutamate and GABA homeostasis and Neurometabolism in major depressive disorder. Front. Psych. 12:637863. doi: 10.3389/fpsyt.2021.637863
Schafflick, D., Wolbert, J., Heming, M., Thomas, C., Hartlehnert, M., Börsch, A. L., et al. (2021). Single-cell profiling of CNS border compartment leukocytes reveals that B cells and their progenitors reside in non-diseased meninges. Nat. Neurosci. 24, 1225–1234. doi: 10.1038/s41593-021-00880-y
Scheinert, R. B., Haeri, M. H., Lehmann, M. L., and Herkenham, M. (2016). Therapeutic effects of stress-programmed lymphocytes transferred to chronically stressed mice. Prog. Neuro-Psychopharmacol. Biol. Psychiatry 70, 1–7. doi: 10.1016/j.pnpbp.2016.04.010
Schlaaff, K., Dobrowolny, H., Frodl, T., Mawrin, C., Gos, T., Steiner, J., et al. (2020). Increased densities of T and B lymphocytes indicate neuroinflammation in subgroups of schizophrenia and mood disorder patients. Brain Behav. Immun. 88, 497–506. doi: 10.1016/j.bbi.2020.04.021
Schmidt, F. M., Koch, J., Nowak, C., Holdt, L. M., Teupser, D., Hegerl, U., et al. (2019). Ligands and receptors of the TNF superfamily are decreased in major depression and during early antidepressant therapy. J. Psychiatr. Res. 119, 116–121. doi: 10.1016/j.jpsychires.2019.09.010
Schoenfeld, T. J., and Cameron, H. A. (2015). Adult neurogenesis and mental illness. Neuropsychopharmacology 40, 113–128. doi: 10.1038/npp.2014.230
Schönemeier, B., Kolodziej, A., Schulz, S., Jacobs, S., Hoellt, V., and Stumm, R. (2008). Regional and cellular localization of the CXCl12/SDF-1 chemokine receptor CXCR7 in the developing and adult rat brain. J. Comp. Neurol. 510, 207–220. doi: 10.1002/cne.21780
Schuhmann, B., Dietrich, A., Sel, S., Hahn, C., Klingenspor, M., Lommatzsch, M., et al. (2005). A role for brain-derived neurotrophic factor in B cell development. J. Neuroimmunol. 163, 15–23. doi: 10.1016/j.jneuroim.2005.01.023
Serafeim, A., Grafton, G., Chamba, A., Gregory, C. D., Blakely, R. D., Bowery, N. G., et al. (2002). 5-Hydroxytryptamine drives apoptosis in biopsylike Burkitt lymphoma cells: reversal by selective serotonin reuptake inhibitors. Blood 99, 2545–2553. doi: 10.1182/blood.v99.7.2545
Shatz, C. J. (2009). MHC class I: an unexpected role in neuronal plasticity. Neuron 64, 40–45. doi: 10.1016/j.neuron.2009.09.044
Simma, N., Bose, T., Kahlfuß, S., Mankiewicz, J., Lowinus, T., Lühder, F., et al. (2014). NMDA-receptor antagonists block B-cell function but foster IL-10 production in BCR/CD40-activated B cells. Cell Commun. Signal. 12:75. doi: 10.1186/s12964-014-0075-5
Ślusarczyk, J., Trojan, E., Głombik, K., Budziszewska, B., Kubera, M., Lasoń, W., et al. (2015). Prenatal stress is a vulnerability factor for altered morphology and biological activity of microglia cells. Front. Cell. Neurosci. 9:82. doi: 10.3389/fncel.2015.00082
Song, C., Dinan, T., and Leonard, B. E. (1994). Changes in immunoglobulin, complement and acute phase protein levels in the depressed patients and normal controls. J. Affect. Disord. 30, 283–288. doi: 10.1016/0165-0327(94)90135-x
Steel, Z., Marnane, C., Iranpour, C., Chey, T., Jackson, J. W., Patel, V., et al. (2014). The global prevalence of common mental disorders: a systematic review and meta-analysis 1980-2013. Int. J. Epidemiol. 43, 476–493. doi: 10.1093/ije/dyu038
Stefanski, V., and Engler, H. (1998). Effects of acute and chronic social stress on blood cellular immunity in rats. Physiol. Behav. 64, 733–741. doi: 10.1016/s0031-9384(98)00127-9
Stein, J., and Nombela-Arrieta, C. (2005). Chemokine control of lymphocyte trafficking: a general overview. Immunology 116, 1–12. doi: 10.1111/j.1365-2567.2005.02183.x
Stenzel-Poore, M. P., Cameron, V. A., Vaughan, J., Sawchenko, P. E., and Vale, W. (1992). Development of Cushing's syndrome in corticotropin-releasing factor transgenic mice. Endocrinology 130, 3378–3386. doi: 10.1210/endo.130.6.1597149
Stenzel-Poore, M. P., Heinrichs, S. C., Rivest, S., Koob, G. F., and Vale, W. W. (1994). Overproduction of corticotropin-releasing factor in transgenic mice: a genetic model of anxiogenic behavior. J. Neurosci. 14, 2579–2584. doi: 10.1523/jneurosci.14-05-02579.1994
Stock, A. D., Der, E., Gelb, S., Huang, M., Weidenheim, K., Ben-Zvi, A., et al. (2019). Tertiary lymphoid structures in the choroid plexus in neuropsychiatric lupus. JCI Insight 4:e124203. doi: 10.1172/jci.insight.124203
Stuart, M. J., Singhal, G., and Baune, B. T. (2015). Systematic review of the neurobiological relevance of chemokines to psychiatric disorders. Front. Cell. Neurosci. 9:357. doi: 10.3389/fncel.2015.00357
Sturgill, J. L., Mathews, J., Scherle, P., and Conrad, D. H. (2011). Glutamate signaling through the kainate receptor enhances human immunoglobulin production. J. Neuroimmunol. 233, 80–89. doi: 10.1016/j.jneuroim.2010.11.014
Sun, B., Ramberger, M., O'Connor, K. C., Bashford-Rogers, R. J. M., and Irani, S. R. (2020). The B cell immunobiology that underlies CNS autoantibody-mediated diseases. Nat. Rev. Neurol. 16, 481–492. doi: 10.1038/s41582-020-0381-z
Sun, W., Zhang, L., Lin, L., Wang, W., Ge, Y., Liu, Y., et al. (2019). Chronic psychological stress impairs germinal center response by repressing miR-155. Brain Behav. Immun. 76, 48–60. doi: 10.1016/j.bbi.2018.11.002
Szpakowski, P., Ksiazek-Winiarek, D., Turniak-Kusy, M., Pacan, I., and Glabinski, A. (2022). Human primary astrocytes differently respond to pro- and anti-inflammatory stimuli. Biomedicines 10:1769. doi: 10.3390/biomedicines10081769
Tada, S., Yasui, T., Nakatsuji, Y., Okuno, T., Koda, T., Mochizuki, H., et al. (2013). BAFF controls neural cell survival through BAFF receptor. PLoS One 8:e70924. doi: 10.1371/journal.pone.0070924
Tanabe, S., and Yamashita, T. (2018). B-1a lymphocytes promote oligodendrogenesis during brain development. Nat. Neurosci. 21, 506–516. doi: 10.1038/s41593-018-0106-4
Tarcic, N., Levitan, G., Ben-Yosef, D., Prous, D., Ovadia, H., and Weiss, D. W. (1995). Restraint stress-induced changes in lymphocyte subsets and the expression of adhesion molecules. Neuroimmunomodulation 2, 249–257. doi: 10.1159/000097203
ter Heegde, F., De Rijk, R. H., and Vinkers, C. H. (2015). The brain mineralocorticoid receptor and stress resilience. Psychoneuroendocrinology 52, 92–110. doi: 10.1016/j.psyneuen.2014.10.022
Tesfagiorgis, Y., Zhu, S. L., Jain, R., and Kerfoot, S. M. (2017). Activated B cells participating in the anti-myelin response are excluded from the inflamed central nervous system in a model of autoimmunity that allows for B cell recognition of autoantigen. J. Immunol. 199, 449–457. doi: 10.4049/jimmunol.1602042
Thangarajh, M., Masterman, T., Hillert, J., Moerk, S., and Jonsson, R. (2007). A proliferation-inducing ligand (APRIL) is expressed by astrocytes and is increased in multiple sclerosis. Scand. J. Immunol. 65, 92–98. doi: 10.1111/j.1365-3083.2006.01867.x
Torres, V. O., Turchan-Cholewo, J., Kong, X., Plautz, E. J., Monson, N. L., Goldberg, M. P., et al. (2021). “The B cell response to extracellular glutamate in the ischemic brain” in International Stroke Conference (New Orleans: Stroke)
Touil, H., Kobert, A., Lebeurrier, N., Rieger, A., Saikali, P., Lambert, C., et al. (2018). For the Canadian: human central nervous system astrocytes support survival and activation of B cells: implications for MS pathogenesis. J. Neuroinflammation 15:114. doi: 10.1186/s12974-018-1136-2
Tripp, A., Kota, R. S., Lewis, D. A., and Sibille, E. (2011). Reduced somatostatin in subgenual anterior cingulate cortex in major depression. Neurobiol. Dis. 42, 116–124. doi: 10.1016/j.nbd.2011.01.014
Trivedi, M. H., Rush, A. J., Wisniewski, S. R., Nierenberg, A. A., Warden, D., Ritz, L., et al. (2006). Evaluation of outcomes with citalopram for depression using measurement-based care in STAR*D: implications for clinical practice. Am. J. Psychiatry 163, 28–40. doi: 10.1176/appi.ajp.163.1.28
Trzeciak, P., and Herbet, M. (2021). Role of the intestinal microbiome, intestinal barrier and Psychobiotics in depression. Nutrients 13:927. doi: 10.3390/nu13030927
Turbic, A., Leong, S. Y., and Turnley, A. M. (2011). Chemokines and inflammatory mediators interact to regulate adult murine neural precursor cell proliferation, survival and differentiation. PLoS One 6:e25406. doi: 10.1371/journal.pone.0025406
Tüzün, E., Zhou, L., Baehring, J. M., Bannykh, S., Rosenfeld, M. R., and Dalmau, J. (2009). Evidence for antibody-mediated pathogenesis in anti-NMDAR encephalitis associated with ovarian teratoma. Acta Neuropathol. 118, 737–743. doi: 10.1007/s00401-009-0582-4
Uchida, J., Hamaguchi, Y., Oliver, J. A., Ravetch, J. V., Poe, J. C., Haas, K. M., et al. (2004). The innate mononuclear phagocyte network depletes B lymphocytes through fc receptor-dependent mechanisms during anti-CD20 antibody immunotherapy. J. Exp. Med. 199, 1659–1669. doi: 10.1084/jem.20040119
Urbina, M., Pineda, S., Piñango, L., Carreira, I., and Lima, L. (1999). [3H]paroxetine binding to human peripheral lymphocyte membranes of patients with major depression before and after treatment with fluoxetine. Int. J. Immunopharmacol. 21, 631–646. doi: 10.1016/s0192-0561(99)00035-1
Utset, H. A., Guthmiller, J. J., and Wilson, P. C. (2021). Bridging the B cell gap: novel technologies to study antigen-specific human B cell responses. Vaccines 9:711. doi: 10.3390/vaccines9070711
Vahabi, Z., Etesam, F., Zandifar, A., and Badrfam, R. (2021). Psychosocial stress, blood brain barrier and the development of anti N-methyl-D-aspartate receptor (NMDAR) encephalitis. Mult. Scler. Relat. Disord. 50:102876. doi: 10.1016/j.msard.2021.102876
Vidal, P. M., and Pacheco, R. (2020). Targeting the dopaminergic system in autoimmunity. J. Neuroimmune Pharmacol. 15, 57–73. doi: 10.1007/s11481-019-09834-5
Vincent, F. B., Saulep-Easton, D., Figgett, W. A., Fairfax, K. A., and Mackay, F. (2013). The BAFF/APRIL system: emerging functions beyond B cell biology and autoimmunity. Cytokine Growth Factor Rev. 24, 203–215. doi: 10.1016/j.cytogfr.2013.04.003
Vladutiu, A. O. (2000). Immunoglobulin D: properties, measurement, and clinical relevance. Clin. Diagn. Lab. Immunol. 7, 131–140. doi: 10.1128/CDLI.7.2.131-140.2000
Wang, H., He, Y., Sun, Z., Ren, S., Liu, M., Wang, G., et al. (2022). Microglia in depression: an overview of microglia in the pathogenesis and treatment of depression. J. Neuroinflammation 19:132. doi: 10.1186/s12974-022-02492-0
Wang, A. A., Luessi, F., Neziraj, T., Pössnecker, E., Zuo, M., Engel, S., et al. (2024). B cell depletion with anti-CD20 promotes neuroprotection in a BAFF-dependent manner in mice and humans. Sci. Transl. Med. 16:eadi0295. doi: 10.1126/scitranslmed.adi0295
Wang, Y. T., Wang, X. L., Feng, S. T., Chen, N. H., Wang, Z. Z., and Zhang, Y. (2021). Novel rapid-acting glutamatergic modulators: targeting the synaptic plasticity in depression. Pharmacol. Res. 171:105761. doi: 10.1016/j.phrs.2021.105761
Wasiak, J., and Gawlik-Kotelnicka, O. (2023). Intestinal permeability and its significance in psychiatric disorders - A narrative review and future perspectives. Behav. Brain Res. 448:114459. doi: 10.1016/j.bbr.2023.114459
Wei, Y., Wang, T., Liao, L., Fan, X., Chang, L., and Hashimoto, K. (2022). Brain-spleen axis in health and diseases: a review and future perspective. Brain Res. Bull. 182, 130–140. doi: 10.1016/j.brainresbull.2022.02.008
Wei, L., Zhang, C., Chen, H. Y., Zhang, Z. J., Ji, Z. F., Yue, T., et al. (2015). Dopamine receptor DR2 expression in B cells is negatively correlated with disease activity in rheumatoid arthritis patients. Immunobiology 220, 323–330. doi: 10.1016/j.imbio.2014.10.016
Wieber, K., Fleige, L., Tsiami, S., Reinders, J., Braun, J., Baraliakos, X., et al. (2022). Dopamine receptor 1 expressing B cells exert a proinflammatory role in female patients with rheumatoid arthritis. Sci. Rep. 12:5985. doi: 10.1038/s41598-022-09891-6
Wieczorek, M., Abualrous, E. T., Sticht, J., Alvaro-Benito, M., Stolzenberg, S., Noe, F., et al. (2017). Major histocompatibility complex (MHC) class I and MHC class II proteins: conformational plasticity in antigen presentation. Front. Immunol. 8:292. doi: 10.3389/fimmu.2017.00292
Wikenheiser, D. J., and Stumhofer, J. S. (2016). ICOS co-stimulation: friend or foe? Front. Immunol. 7:304. doi: 10.3389/fimmu.2016.00304
Wohleb, E. S., Franklin, T., Iwata, M., and Duman, R. S. (2016). Integrating neuroimmune systems in the neurobiology of depression. Nat. Rev. Neurosci. 17, 497–511. doi: 10.1038/nrn.2016.69
Wolf, S. A., Steiner, B., Akpinarli, A., Kammertoens, T., Nassenstein, C., Braun, A., et al. (2009). CD4-positive T lymphocytes provide a neuroimmunological link in the control of adult hippocampal neurogenesis. J. Immunol. 182, 3979–3984. doi: 10.4049/jimmunol.0801218
Woo, H. I., Lim, S. W., Myung, W., Kim, D. K., and Lee, S. Y. (2018). Differentially expressed genes related to major depressive disorder and antidepressant response: genome-wide gene expression analysis. Exp. Mol. Med. 50, 1–11. doi: 10.1038/s12276-018-0123-0
World Health Organization (2017). Depression and other common mental disorders: global health estimates. Geneva: World Health Organization.
Wray, N. R., Ripke, S., Mattheisen, M., Trzaskowski, M., Byrne, E. M., Abdellaoui, A., et al. (2018). Consortium: genome-wide association analyses identify 44 risk variants and refine the genetic architecture of major depression. Nat. Genet. 50, 668–681. doi: 10.1038/s41588-018-0090-3
Wu, X. B., He, L. N., Jiang, B. C., Wang, X., Lu, Y., and Gao, Y. J. (2019). Increased CXCL13 and CXCR5 in anterior cingulate cortex contributes to neuropathic pain-related conditioned place aversion. Neurosci. Bull. 35, 613–623. doi: 10.1007/s12264-019-00377-6
Wu, C. Y., Sarfati, M., Heusser, C., Fournier, S., Rubio-Trujillo, M., Peleman, R., et al. (1991). Glucocorticoids increase the synthesis of immunoglobulin E by interleukin 4-stimulated human lymphocytes. J. Clin. Invest. 87, 870–877. doi: 10.1172/JCI115092
Zhang, E., Huang, Z., Zang, Z., Qiao, X., Yan, J., and Shao, X. (2023). Identifying circulating biomarkers for major depressive disorder. Front. Psych. 14:1230246. doi: 10.3389/fpsyt.2023.1230246
Zhang, X., Lei, B., Yuan, Y., Zhang, L., Hu, L., Jin, S., et al. (2020). Brain control of humoral immune responses amenable to behavioural modulation. Nature 581, 204–208. doi: 10.1038/s41586-020-2235-7
Zhang, X., Li, J., Sha, W., and Bu, R. (2015). “Neurotrophic factors and major depressive disorder” in Major depressive disorder - cognitive and neurobiological mechanisms. ed. Y.-K. Kim (South Korea: InTech Open).
Zhang, J., Ma, L., Chang, L., Pu, Y., Qu, Y., and Hashimoto, K. (2020). A key role of the subdiaphragmatic vagus nerve in the depression-like phenotype and abnormal composition of gut microbiota in mice after lipopolysaccharide administration. Transl. Psychiatry 10:186. doi: 10.1038/s41398-020-00878-3
Zhang, K., Sakamoto, A., Chang, L., Qu, Y., Wang, S., Pu, Y., et al. (2021). Splenic NKG2D confers resilience versus susceptibility in mice after chronic social defeat stress: beneficial effects of (R)-ketamine. Eur. Arch. Psychiatry Clin. Neurosci. 271, 447–456. doi: 10.1007/s00406-019-01092-z
Zhang, B., Vogelzang, A., Miyajima, M., Sugiura, Y., Wu, Y., Chamoto, K., et al. (2021). B cell-derived GABA elicits IL-10+ macrophages to limit anti-tumour immunity. Nature 599, 471–476. doi: 10.1038/s41586-021-04082-1
Zhang, Z., Xu, Q., and Huang, L. (2023). B cell depletion therapies in autoimmune diseases: monoclonal antibodies or chimeric antigen receptor-based therapy? Front. Immunol. 14:1126421. doi: 10.3389/fimmu.2023.1126421
Zhang, L., Zheng, S., Wu, H., Wu, Y., Liu, S., Fan, M., et al. (2009). Identification of BLyS (B lymphocyte stimulator), a non-myelin-associated protein, as a functional ligand for Nogo-66 receptor. J. Neurosci. 29, 6348–6352. doi: 10.1523/jneurosci.5040-08.2009
Zogorean, R., and Wirtz, S. (2023). The yin and yang of B cells in a constant state of battle: intestinal inflammation and inflammatory bowel disease. Front. Immunol. 14:1260266. doi: 10.3389/fimmu.2023.1260266
Zong, S., Hoffmann, C., Mané-Damas, M., Molenaar, P., Losen, M., and Martinez-Martinez, P. (2017). Neuronal surface autoantibodies in neuropsychiatric disorders: are there implications for depression? Front. Immunol. 8:752. doi: 10.3389/fimmu.2017.00752
Zwilling, B. S., Dinkins, M., Christner, R., Faris, M., Griffin, A., Hilburger, M., et al. (1990). Restraint stress-induced suppression of major histocompatibility complex class II expression by murine peritoneal macrophages. J. Neuroimmunol. 29, 125–130. doi: 10.1016/0165-5728(90)90154-f
Glossary
Keywords: stress, major depression, immune system, B lymphocyte, brain
Citation: Engler-Chiurazzi E (2024) B cells and the stressed brain: emerging evidence of neuroimmune interactions in the context of psychosocial stress and major depression. Front. Cell. Neurosci. 18:1360242. doi: 10.3389/fncel.2024.1360242
Edited by:
Olga Rojas, University Health Network (UHN), CanadaReviewed by:
Ruxandra F. Sirbulescu, Massachusetts General Hospital and Harvard Medical School, United StatesKenji Hashimoto, Chiba University, Japan
Copyright © 2024 Engler-Chiurazzi. This is an open-access article distributed under the terms of the Creative Commons Attribution License (CC BY). The use, distribution or reproduction in other forums is permitted, provided the original author(s) and the copyright owner(s) are credited and that the original publication in this journal is cited, in accordance with accepted academic practice. No use, distribution or reproduction is permitted which does not comply with these terms.
*Correspondence: Elizabeth Engler-Chiurazzi, ZWVuZ2xlcmNoaXVyYXp6aUB0dWxhbmUuZWR1