- 1Centre for Immunology and Infection Control, School of Biomedical Sciences, Faculty of Health, Queensland University of Technology (QUT), Brisbane, QLD, Australia
- 2Mental Health and Neuroscience Program, QIMR Berghofer Medical Research Institute, Herston, QLD, Australia
- 3Centre for Vision and Eye Research, School of Biomedical Sciences, Faculty of Health, Queensland University of Technology (QUT), Brisbane, QLD, Australia
Microglia play crucial roles in immune responses and contribute to fundamental biological processes within the central nervous system (CNS). In neurodegenerative diseases, microglia undergo functional changes and can have both protective and pathogenic roles. Microglia in the retina, as an extension of the CNS, have also been shown to be affected in many neurological diseases. While our understanding of how microglia contribute to pathological conditions is incomplete, non-invasive in vivo imaging of brain and retinal microglia in living subjects could provide valuable insights into their role in the neurodegenerative diseases and open new avenues for diagnostic biomarkers. This mini-review provides an overview of the current brain and retinal imaging tools for studying microglia in vivo. We focus on microglia targets, the advantages and limitations of in vivo microglia imaging approaches, and applications for evaluating the pathogenesis of neurological conditions, such as Alzheimer’s disease and multiple sclerosis.
Introduction
The central nervous system (CNS) parenchyma is populated with resident macrophages called microglia, which contribute to regulation of neurodevelopment, CNS homeostasis, inflammation and injury repair (Michell-Robinson et al., 2015; McMenamin et al., 2019). Microglia are implicated in the pathogenesis of several neurodegenerative conditions, including Alzheimer’s disease (AD) (Cherry et al., 2015; Shi et al., 2019), multiple sclerosis (MS) (Voet et al., 2019) and Parkinson’s disease (Guo et al., 2020), with recent studies suggesting that different microglia subtypes with varying functional responses may be involved in CNS diseases (Olah et al., 2011; Flowers et al., 2017; Keren-Shaul et al., 2017; Hammond et al., 2019; Masuda et al., 2020).
Much of our understanding of microglia in humans and animal models comes from studies of fixed or ex vivo tissue, in vitro cell cultures or ‘omics’ analysis of microglia isolated from CNS tissue. However, tissue processing methods may artificially shift microglia into various reactive states that are not representative of their in vivo status (Marsh et al., 2022). Approaches to non-invasively study microglia in their physiological environment in living subjects are therefore of interest to advance our understanding of these cells and their involvement in CNS diseases. As ‘first line’ responders in CNS immune defense, non-invasive in vivo evaluation of microglia has been proposed as a tool for the diagnosis and monitoring of neuroinflammation in neuropathological conditions (Tucker et al., 2016; Beaino et al., 2017, 2020; Ardaya et al., 2020; Coda et al., 2021). An altered CNS inflammatory state is postulated to occur prior to the onset of pathology in many neurodegenerative conditions (Hansen et al., 2017; Gazestani et al., 2023), suggesting that non-invasive in vivo microglia imaging could be used to identify early signs of disease (Tucker et al., 2016; Beaino et al., 2017, 2020; Ardaya et al., 2020; Coda et al., 2021). Furthermore, the ability to monitor microglia in a non-invasive manner may also inform patient treatment, especially considering that these cells are being investigated as immunotherapeutic targets for several neurological and ocular conditions (Liu et al., 2022; Gao et al., 2023).
Here, we review non-invasive techniques that have been used to image microglia in the brain and retina of living subjects, including positron emission tomography (PET), optical coherence tomography (OCT), confocal scanning laser ophthalmoscopy (cSLO) and adaptive optics. We discuss these imaging approaches in the context of AD, MS, and their animal models.
Non-invasive approaches for in vivo microglia imaging
Positron emission tomography
Positron emission tomography is the most commonly employed non-invasive approach for imaging brain inflammation; used for quantitative assessment of neuroinflammation and longitudinal visualization of CNS immune cells in clinical studies and animal models (Politis et al., 2012b). This technique uses radiolabelled tracers, which comprise a ligand that binds to protein targets, and a positron-emitting isotope that is detected using nuclear medicine. PET radiotracers for imaging of targets in the brain must meet basic requirements, such as the ability to cross the blood-brain barrier, specific binding to the target with high affinity, and metabolic stability (Pike, 2009). A limitation of commercial PET scanners is the relatively low spatial resolution, reported to be 2–6 mm in dedicated brain PET imaging devices (Catana, 2019).
Positron emission tomography targets for imaging neuroinflammation have been reviewed elsewhere (Tronel et al., 2017; Beaino et al., 2021), and their application in neurodegenerative diseases will be covered in greater detail in subsequent sections. The most widely used target for PET imaging of neuroinflammation is Translocator protein 18 kDa (TSPO) (Jain et al., 2020). Although highly expressed by activated microglia, TSPO lacks specificity as it is also expressed by other brain cell types during disease, including astrocytes, endothelial cells and infiltrating immune cells (Kaunzner et al., 2019; Nutma et al., 2019; Gui et al., 2020). Other targets that have been investigated for PET imaging of neuroinflammation include cyclooxygenase (COX) isoforms (Shrestha et al., 2020), cannabinoid receptor type 2 (CB2R) (Evens et al., 2012) and sphingosine-1-phosphate receptor 1 (S1PR1) (Liu et al., 2016). Whilst none of these targets are exclusively expressed by microglia, they have been shown to be upregulated in the brain during pathological conditions and therefore can indicate a broad neuroinflammatory state.
The ability to selectively target microglia and their subtypes using PET imaging would be a significant step forward for in vivo brain imaging, potentially enabling new insights into the contribution of these cells to the pathogenesis of neurodegenerative diseases. Limited progress toward the goal of microglia-specific PET imaging has been made using radiotracers targeting Purinergic 2Y receptor type 12 (P2RY12). P2RY12 is highly expressed in homeostatic conditions and can distinguish microglia from other brain cells and border-associated macrophages (Sasaki et al., 2003; Beaino et al., 2017; Mildner et al., 2017). The expression of P2RY12 is altered during CNS diseases, with immunohistochemical studies of human brain tissue suggesting that reduced microglial P2RY12 expression occurs in regions of neuropathology and inflammation (Zrzavy et al., 2017; Walker et al., 2020). In contrast, P2RY12 expression may be increased by microglia involved in anti-inflammatory repair processes (Beaino et al., 2017). These properties make P2RY12 an attractive target for PET imaging; however, attempts to develop radiotracers targeting this receptor have largely been unsuccessful to date, demonstrating poor penetration of the blood-brain barrier (Villa et al., 2018; van der Wildt et al., 2021). Further studies to understand the expression of P2RY12 across different neurological diseases and develop radiotracers with improved brain penetration are therefore required.
An alternative marker that has been investigated for PET imaging of brain microglia is colony stimulating factor-1 receptor (CSF-1R) (Horti et al., 2019). Similar to other macrophage populations, microglial development (Ginhoux et al., 2010) and survival (Elmore et al., 2014) are controlled by colony stimulating factor-1 (CSF-1) and its receptor, CSF-1R. In the healthy neural parenchyma, microglia are the sole cells that express CSF-1R, and Horti et al. (2019) developed a CSF-1R targeting PET radiotracer (11C-CPPC) that demonstrated high levels of uptake in mice, non-human primates and post-mortem brain tissue of human AD patients. First-in-human use of 11C-CPPC revealed promising pharmacokinetic properties and good brain uptake in healthy individuals (Coughlin et al., 2022); whilst these findings were regarded as exciting developments in microglial imaging, an important caveat is that perivascular macrophages and peripheral cells of the monocytic lineage also express CSF-1R (Chitu and Stanley, 2006; Kerkhofs et al., 2020). Therefore, CSF-1R PET imaging of neuroinflammatory conditions involving infiltration of monocytes is unlikely to be truly microglia-specific.
Recent work has focused on Purinergic 2X receptor type 7 (P2RX7) as a promising target for PET imaging of so-called “pro-inflammatory microglia.” In vitro studies of primary human microglia polarized into either a pro-inflammatory or anti-inflammatory phenotype demonstrated that P2RX7 is highly expressed by pro-inflammatory (but not anti-inflammatory) microglia (Beaino et al., 2017). Several radiotracers targeting P2RX7 have been evaluated in preclinical and clinical studies, with many showing good pharmacokinetics and brain uptake [reviewed in Beaino et al. (2021)]. A limitation of targeting P2RX7 is that it may also be expressed by astrocytes and oligodendrocytes (albeit at low levels) (Zhao et al., 2021); however, immunostaining of brain tissue with MS active lesions demonstrated that P2RX7 antibodies labeled MHC class II + cells with a microglia-like morphology (Beaino et al., 2017). These findings suggest that P2RX7 is predominantly expressed by microglia.
Overall, combinations of subtype specific markers would be ideal for investigating microglial activation in neurological diseases. Future selection of targets for microglia PET imaging should be guided by the wealth of microglial subtypes (and their markers) that have been identified using transcriptomic approaches in recent years. For example, Keren-Shaul et al. (2017) identified a unique microglia subtype termed ‘disease-associated microglia’ (DAM) in a mouse model of AD and in human brain slices in AD patients. DAM are localized near AD plaques and the transition of homeostatic microglia to DAM begins during early disease (Keren-Shaul et al., 2017). Therefore, non-invasive PET imaging of DAM could be used to detect early disease and monitor progression. The challenge for the field is to identify robust microglia subtype-specific markers that can be used to develop PET radiotracers.
Retinal imaging techniques
The retina is part of the CNS and enables the visualization and assessment of neurological disease progression through non-invasive imaging (Zhang et al., 2021). Pathological changes occur in the retina in neurodegenerative diseases, and these can be examined using traditional ophthalmic imaging approaches including optical coherence tomography (OCT) and confocal laser scanning ophthalmoscopy (cSLO) (Koronyo et al., 2017; Vij and Arora, 2022; Vujosevic et al., 2023). OCT generates cross-sectional images (typically 4–7 μm axial resolution, 15–20 μm transverse resolution) of the retina by detecting light reflection from the different tissue layers and enables assessment of retinal layer thickness (Bajwa et al., 2015). Techniques such as en face OCT and OCT angiography (OCTA) produce transverse retinal images and 3D reconstructions of the retinal vasculature, respectively (Van Velthoven et al., 2006; de Carlo et al., 2015). Confocal scanning laser ophthalmoscopy (cSLO) is used for fundus imaging and offers several modalities, including angiography and retro-illumination. In addition to conventional fundus imaging, cSLO uses lasers with differing wavelengths to produce images of different retinal layers or structural features (Bajwa et al., 2015); however, this technique is limited by a lower axial resolution (∼300 μm) compared to OCT (Mainster et al., 2022). Whilst these techniques enable excellent visualization of the retina for clinical and diagnostic purposes, they are unable to capture detailed information at the cellular and sub-cellular level due to the monochromatic wavefront aberrations of the eye, and therefore studying retinal microglia and their processes in living subjects has been elusive.
To address this challenge, adaptive optics (AO) has been combined with SLO to correct the optical aberrations, enabling fine cellular structures within the retina to be resolved. Geng et al. (2012) developed a custom AO-SLO instrument for non-invasive imaging of the mouse retina and generated the first in vivo images of the photoreceptor mosaic in mice. The AO-SLO instrument had a reported axial resolution of ∼10 μm and a submicron transverse resolution, and also enabled individual nerve fiber bundles, blood vessels and capillaries within the mouse retinal nerve fiber layer to be resolved. Furthermore, AO-SLO imaging of transgenic reporter mice enabled visualization of fluorescently labeled ganglion cell bodies, dendrites and axons (Geng et al., 2012). Recent studies have applied this technique to non-invasive imaging of fluorescent microglia in mice (Miller et al., 2019; Joseph et al., 2021). Important advances in near infra-red phase contrast AO-SLO have also enabled label-free imaging of mouse retinal microglia and their process dynamics over time (Joseph et al., 2021). This provides proof-of-concept that phase contrast AO-SLO could be translated to perform in vivo microglia imaging in the human eye.
Adaptive optics combined with OCT (AO-OCT) has a resolution of 4.7 μm (axial) and 2.4 μm (lateral) (Liu et al., 2017) and also has potential applications for direct visualization of microglia in the human retina. Several publications have demonstrated that AO-OCT can be used to resolve macrophages (hyalocytes) at the inner limiting membrane (ILM) located at the vitreoretinal interface in the human eye (Liu et al., 2017; Hammer et al., 2020; Kazuhiro et al., 2020). However, ILM macrophages are distinct from microglia, and due to their location exterior to the CNS these cells are not suitable surrogates for studying microglia. To date, AO-OCT studies have been unable to resolve retinal microglia (Hammer et al., 2020), which reside within the outer plexiform layer, inner plexiform layer and ganglion cell layer of the neural retina (McMenamin et al., 2019). Taken together, the recent application of AO to traditional ophthalmic imaging approaches has significantly enhanced retinal imaging capabilities by enabling visualization of cells and cellular structures. Future development in this space will likely lead to non-invasive methods for in vivo microglia imaging in the human eye, providing a window into the immune landscape of the CNS. Although, given these are label-free approaches, it is unlikely that they could be adapted to enable targeted imaging of immune cell subtypes in the human retina without the involvement of tracers.
In vivo imaging of brain and retinal microglia in neurodegenerative diseases
Alzheimer’s disease
Alzheimer’s disease is the most common form of dementia (Gabandé-Rodríguez et al., 2020), characterized by the pathological hallmarks of extracellular deposition of amyloid-β (Aβ) plaques resulting from impairment of Aβ clearance from the CNS (Mawuenyega et al., 2010), and intraneuronal hyperphosphorylated tau protein tangles (Johnson and Stoothoff, 2004). Microglial activation and inflammatory responses are also increasingly recognized as a central feature of AD (Kinney et al., 2018). Microglia undergo a number of functional changes in AD and have beneficial roles, including phagocytosis of Aβ (Gabandé-Rodríguez et al., 2020), lipid metabolism (Claes et al., 2021) and regulation of tau pathology via autophagy (Xu et al., 2021). However, sustained microglial activation and pro-inflammatory signaling can lead to reduced Aβ phagocytosis, exacerbated neuroinflammation and suppression of homeostatic microglia, which contribute to neurodegeneration (Kinney et al., 2018).
Microglia PET imaging in AD
Positron emission tomography has been extensively used to study neuroinflammation and microglia activation in AD, with a large number of studies reporting that microglial PET target levels are increased in the brains of AD patients (Table 1). TSPO PET in particular has advanced our understanding of the role of microglia in AD, although these findings need to be interpreted carefully due to the non-specificity of TSPO. Increased TSPO levels are positively correlated with Aβ accumulation (Parbo et al., 2017; Dani et al., 2018; Zou et al., 2020) and tau aggregation (Dani et al., 2018) in mild cognitive impairment (MCI) and AD, supporting a role for microglia activation and neuroinflammation in AD. A recent study examined the spatial relationships between microglial activation (determined by TSPO PET), Aβ deposition and tau accumulation in 130 individuals across the spectrum of aging and AD disease progression. This study revealed that microglial activation, potentiated by interactions with Aβ, initiated the spread of tau tangles in the neocortex in a Braak-like pattern (Pascoal et al., 2021). In line with these findings, Rauchmann et al. (2022) reported that microglial activation in AD patients followed a similar spatial distribution to tau along functional connectivity pathways. Taken together, these findings suggest that microglia directly contribute to the pathological hallmarks of AD and highlight the valuable contributions of in vivo brain imaging to understanding the pathogenesis of neurodegenerative diseases.
Interestingly, a longitudinal PET study suggested that microglial activation occurs in two waves during AD disease progression, whereby TSPO signal is initially increased during MCI, then undergoes a longitudinal reduction, followed by a second increase in TSPO signal during AD (Fan et al., 2017). The authors hypothesized that the early peak represents expansion of microglia with a protective phenotype and the later peak represents expansion of pro-inflammatory microglia. However, the ability to study microglia subtypes in living patients remains challenging using existing PET targets. This represents a current limitation of in vivo brain imaging, especially considering molecular studies have identified several microglia subtypes with unique functional roles in AD (Kamphuis et al., 2016; Krasemann et al., 2017; Frigerio et al., 2019; Olah et al., 2020; Prater et al., 2021). This includes disease-associated microglia (DAM), which have enhanced phagocytic and lipid metabolism pathways (Keren-Shaul et al., 2017). In mouse models of AD, the switch from a microglial homeostatic phenotype to a disease-associated phenotype involves upregulation of a set of genes, including the AD-associated gene APOE, and downregulation of the core microglial transcriptomic signature (Keren-Shaul et al., 2017; Krasemann et al., 2017). The second phase of DAM activation (stage 2 DAM) is mediated by microglial Trem2 (Keren-Shaul et al., 2017). Interestingly, loss-of-function mutations in Trem2 increase the risk of late onset AD, and this may be partially due to the inability of Trem2-deficient microglia to transition to stage 2 DAM (Lewcock et al., 2020). Whist DAM appear to have a neuroprotective role, other microglia subtypes may negatively contribute to neurodegeneration. For example, microglial subtypes enriched in type 1 interferon genes (‘interferon-responsive’ microglia) have been identified in mouse models of AD and in human AD brains (Frigerio et al., 2019; Olah et al., 2020). Roy et al. (2022) demonstrated that microglial type 1 interferon signaling is involved in post-synaptic loss in a model of AD, suggesting a pathogenic role for the interferon-responsive microglia subtype.
The ability to perform non-invasive imaging of functionally distinct microglia subtypes would significantly enhance our understanding of microglial involvement in AD and spatiotemporal changes associated with disease progression. Using PET, this could be achieved with microglia subtype-specific radiotracers. Bartolo et al. (2022) reported an in silico approach for identifying microglial candidate genes for PET radiotracer development that could be adapted for this purpose. These authors interrogated published -omics datasets to identify microglia-specific genes that have increased expression in post-mortem AD brain tissue and are associated with neuropathological characteristics (Bartolo et al., 2022). Using this approach, 19 microglia genes were identified and ranked for PET target prioritization. A similar strategy could be employed to determine candidate genes for microglia subtypes, although further studies are first required to obtain a more detailed understanding of microglia subtypes and their transcriptomic signatures in AD.
Retinal imaging biomarkers and microglia in AD
In recent years there has been significant interest in developing retinal imaging biomarkers for AD. Deposits of Aβ and tau protein have been found in the retina of AD patients, along with other retinal changes including vascular alterations, inflammation and thinning of retinal layers (Ramirez et al., 2017; Snyder et al., 2021; Zhang et al., 2022; Ashraf et al., 2023). Interestingly, in the early stages of disease, preceding Aβ plaque formation in the brain, Aβ plaques were detected in the retina in AD mouse models (Koronyo-Hamaoui et al., 2011; Koronyo et al., 2012), suggesting that retinal imaging may be useful as an early diagnostic tool.
Consistent with observations in the brain suggesting a close spatial relationship between microglia and tau, mouse and human AD studies have shown that retinal tau accumulated in the inner and outer plexiform layers (Chiasseu et al., 2017; den Haan et al., 2018) where microglia are known to be localized. However, unlike brain microglia, retinal microglia have not been widely investigated in AD. Increased microglial density has been reported in the retinae of AD patients compared to controls (Grimaldi et al., 2019; Xu et al., 2022), and it has been proposed that retinal microglia acquire a DAM phenotype during AD based on the expression of a small number of markers (Grimaldi et al., 2019). Studies in mice have also demonstrated changes in retinal microglial phenotypes in AD models, including changes in morphology and spatial distribution (Salobrar-García et al., 2020). Grimaldi et al. (2018) reported that retinal microglia co-localized with Aβ plaques prior to onset of symptoms in 3xTg-AD mice, and that microglia transitioned from a ramified anti-inflammatory phenotype to a pro-inflammatory phenotype as disease progressed. Conversely, in a study of post-mortem donor eyes Xu et al. (2022) demonstrated reduced co-localization of microglia and Aβ in AD retinae compared to control retinae, despite there being an overall increase in retinal microglia immunolabeling in AD. The authors posited that similar to brain microglia, retinal microglia in AD become dysfunctional and have diminished capacity to migrate toward and phagocytose Aβ (Xu et al., 2022).
Combined, these studies provide a clear indication of retinal microglial involvement and ocular pathology in AD (summarized in Figure 1). Given the early involvement of the retina in AD, there is a significant need for researchers and clinicians to develop standardized imaging approaches for the assessment of retinal biomarkers, including microglia.
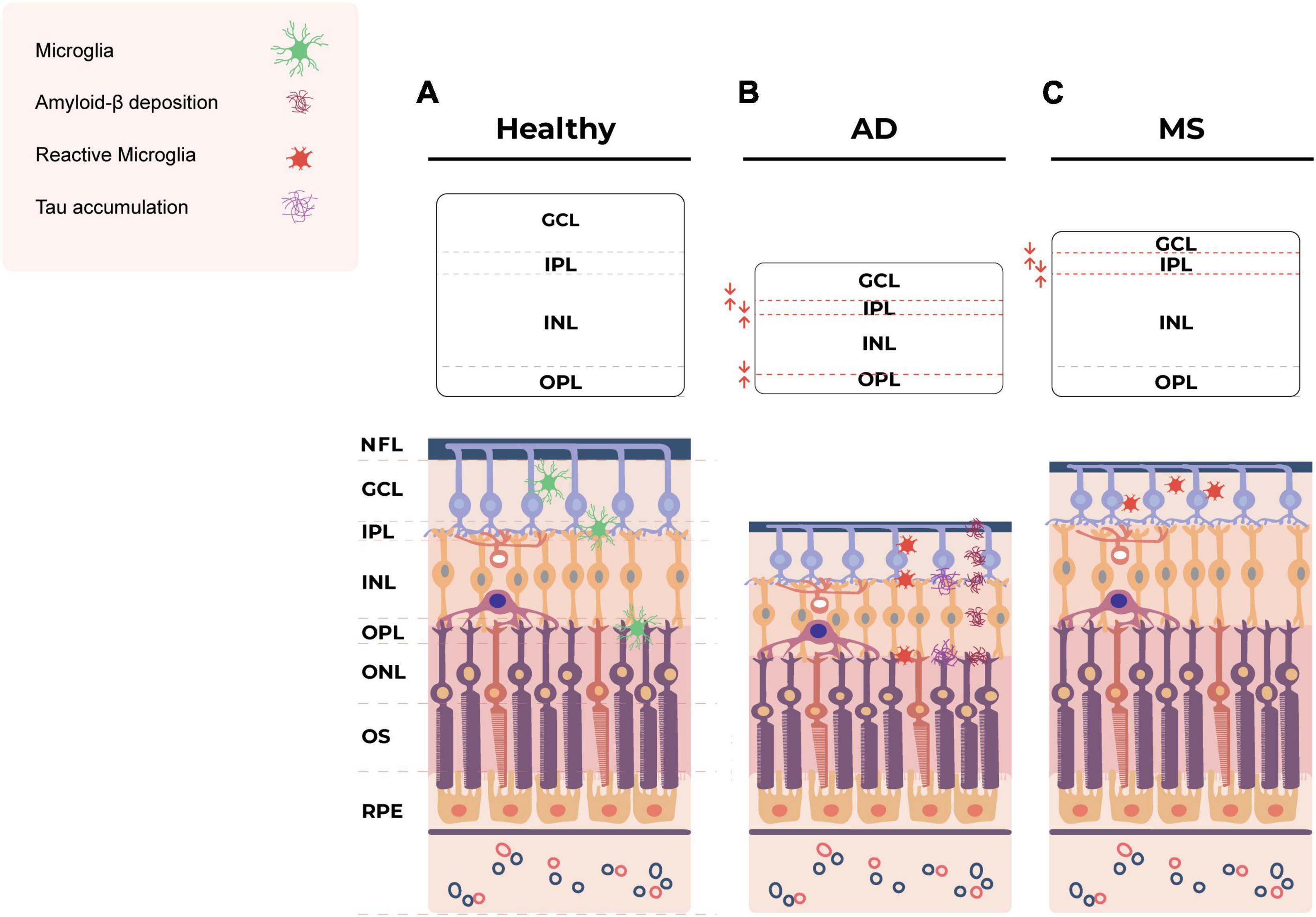
Figure 1. Schematic of the layers of the healthy retina (A) and the hypothetical pathological changes in the retina in AD (B) and MS (C). (B) In AD, Tau accumulation is observed in the inner and outer plexiform layers of the retina (Chiasseu et al., 2017; den Haan et al., 2018). Retinal Aβ plaques are distributed across various layers, including the NFL, GCL, IPL, INL, OPL and even externally to the retina within the sclera (Koronyo-Hamaoui et al., 2011). The eye exhibits structural abnormalities, including decreased thickness of the inner and outer layers (Salobrar-Garcia et al., 2015; Cabrera DeBuc et al., 2019; Jáñez-Escalada et al., 2019; Czakó et al., 2020). Pro-inflammatory, less ramified, neurotoxic microglia are also observed in retina in AD (Zhang et al., 2005; Noailles et al., 2014; Grimaldi et al., 2018; Salobrar-García et al., 2020; Guo et al., 2022). (C) In MS, ocular manifestations lead to the thinning of the NFL, GCL and IPL (Cennamo et al., 2016; Choi et al., 2021) and atrophy of the GCL and IPL (Saidha et al., 2015; Petzold et al., 2017). Microglial cell numbers increase in the GCL but decrease in the IPL (Namekata et al., 2019). Amoeboid microglia are present in the inner retinal layers (Jin et al., 2019). AD, Alzheimer’s disease; MS, multiple sclerosis; NFL, nerve fiber layer; GCL, ganglion cell layer; IPL, inner plexiform layer; INL, inner nuclear layer; OPL, outer plexiform layer; ONL, outer nuclear layer; OS, outer segment; RPE, retinal pigment epithelium.
Multiple sclerosis
Multiple sclerosis, characterized by demyelination and multiple focal lesions, is the most common chronic neurological disease in young adults, affecting 2.8 million people worldwide in 2020 (Walton et al., 2020). MS pathogenesis is thought to be driven by infiltrating autoreactive T cells but also involves a plethora of other infiltrating adaptive and innate immune cell types, as well as resident microglia (Attfield et al., 2022). Strong evidence for microglial involvement in MS was provided by a large genome-wide association study of 47,429 MS and 68,374 control subjects, which revealed that MS susceptibility genes were enriched in microglia but not in other brain cell types (International Multiple Sclerosis Genetics Consortium et al., 2019). Similar findings were reported by Ma et al. (2023), who demonstrated that MS risk genes were significantly enriched in microglial regulatory regions.
Histologically, activated microglia are found in high numbers in active MS lesions and form a rim around mixed active/inactive lesions. Interestingly, microglia are absent in inactive lesions suggesting they play a role in active disease processes (Kuhlmann et al., 2017). Studies of brain tissue from human MS and the experimental autoimmune encephalomyelitis (EAE) animal model have demonstrated that microglia exhibit diverse functions, phenotypes and gene expression profiles in different CNS regions and across different stages of disease (Peferoen et al., 2015; Jordão et al., 2019; Masuda et al., 2019; Schirmer et al., 2019; van der Poel et al., 2019; Miedema et al., 2022). They are thought to contribute to immune-mediated tissue damage during lesion development through various mechanisms including (i) release of reactive oxygen/nitrogen species and toxic levels of glutamate; (ii) sustained pro-inflammatory cytokine production resulting in neuronal and glial dysfunction, and recruitment of infiltrating immune cells; and (iii) antigen presentation to encephalitogenic T cells (Zrzavy et al., 2017; Haimon et al., 2022; Kamma et al., 2022; Montilla et al., 2023). Conversely, microglia also contribute to tissue repair and remyelination in MS by phagocytosing myelin debris, secreting trophic factors, promoting oligodendrocyte maturation, and presenting antigen to regulatory T cells (Lloyd and Miron, 2019; Haimon et al., 2022).
Microglia PET imaging in MS
In MS, enhanced detection of TSPO in PET imaging studies is correlated with disease severity and clinical disability, indicating that microglial activation/neuroinflammation can be used as a general biomarker of MS disease progression (Banati et al., 2000; Politis et al., 2012a; Rissanen et al., 2014; Sucksdorff et al., 2020). Increased TSPO signal is observed within active lesions, at the rim of mixed active/inactive lesions and has the potential to differentiate chronic active and chronic inactive lesions (Rissanen et al., 2014). Whilst it has been assumed that this is due to increased TSPO expression by activated pro-inflammatory microglia, recent studies of human MS brain tissue have shown that TSPO is expressed in a range of microglia phenotypes and that the increased TSPO signal in lesions predominantly reflects microglia/macrophage density rather than activation status or phenotype (Nutma et al., 2019, 2021).
Elevated levels of the potential PET targets P2 × 7R, COX-2, CB2R and CSF-1R have been demonstrated immunohistochemically in lesions in human MS and EAE (Yiangou et al., 2006; Beaino et al., 2017; Hagan et al., 2020). Of these only P2 × 7R has been evaluated as a PET target (using the novel PET tracer [11C]SMW139) in MS and was reported to identify neuroinflammation in lesions and normal appearing brain tissue in patients with active relapsing remitting MS (Hagens et al., 2020). Similar to AD, microglia in MS brains exhibit a marked downregulation of microglial core genes such as P2RY12 and Tmem119 (Zrzavy et al., 2017; Masuda et al., 2019). Therefore, an area for future research is to develop PET tracers that can distinguish between homeostatic microglia and those associated with MS pathogenesis. Single cell RNA sequencing showed that brain tissue from MS patients with early active multiple sclerosis contained a mixture of microglia clusters, including three homeostatic microglia clusters and four clusters with unique disease-related molecular signatures (Masuda et al., 2019). The ability to discriminate these microglia subtypes using PET would allow for exquisite imaging of microglial dynamics in MS patients and provide new insights into disease pathogenesis. However, this remains a challenging concept as most of the genes that are enriched in MS-specific microglial clusters are also expressed by infiltrating myeloid cells and are not suitable targets for microglia-specific imaging.
Retinal imaging biomarkers and microglia in MS
Multiple sclerosis also affects the eyes, causing thinning of the nerve fiber layer (NFL), ganglion cell layer (GCL), inner plexiform layer (IPL) and inner nuclear layer (INL) of the retina, reduced macular volume and optic neuritis (Cennamo et al., 2016; Petzold et al., 2017; Pearson et al., 2022; Usta and Gunay, 2023; Vujosevic et al., 2023) (summarized in Figure 1). Therefore, retinal imaging biomarkers are a growing area of interest for early detection and monitoring of MS. Spectral domain OCT has emerged as a valuable tool for investigating neurodegeneration in the retina and has demonstrated that increased thinning of the inner retinal layers is associated with worsening long-term disability in MS (Lambe et al., 2021; Bsteh et al., 2023; Gernert et al., 2023). OCT studies have also revealed that atrophy of the retinal NFL and GCL reflect brain atrophy in MS patients, particularly grey matter loss (Saidha et al., 2015; Cagol et al., 2023).
MS-associated ocular changes are most prominent in the inner retina; however, a recent AO-OCT study demonstrated that the outer retina is also affected. McIlwaine et al. (2023) reported that MS patients had a significantly lower cone outer-segment density compared to healthy controls; these authors also observed an increase in the thickness of the photoreceptor layer in MS patients who had a history of optic neuritis. Thickening of the combined outer plexiform and outer nuclear layers is also a feature in MS-associated optic neuritis, and this is thought to occur due to inflammation (Petzold et al., 2017). Another non-invasive indicator of inflammation in the retina is the presence of hyper-reflecting foci, which are increased in the retinae of MS patients compared to healthy controls (Pilotto et al., 2020; Pengo et al., 2022). These are thought to represent clusters of activated and proliferating retinal microglia and are associated with cortical pathology, suggesting that retinal microglia may be useful biomarkers in MS (Pengo et al., 2022).
Retinal microglia have not been well studied in MS. In the EAE model, retinal microglia undergo morphological changes consistent with an activated phenotype (Jin et al., 2019). Using cSLO, Cruz-Herranz et al. (2021) demonstrated that the density of retinal myeloid cells markedly increased during the acute phase of EAE and then decreased during the chronic phase. The same authors performed single cell transcriptomic profiling of retinal microglia and reported that these cells existed in a pro-inflammatory state prior to the onset of disease and then switched to a protective state in chronic EAE (Cruz-Herranz et al., 2021). Future applications of AO-SLO/AO-OCT may enable non-invasive characterization of retinal microglia in distinct tissue layers, and provide additional imaging biomarkers for MS.
Conclusion
Microglia are involved in the pathogenesis of neurodegenerative diseases. Therefore, non-invasive brain and retinal imaging techniques to visualize microglia in living patients can be used to monitor disease progression. A major limitation of current imaging approaches is they lack specificity for microglia and cannot distinguish the unique microglial subtypes that have been identified in conditions such as AD and MS. To overcome these limitations, further research is needed to identify microglia subtype-specific imaging targets during different stages of neurodegeneration. Moreover, advances in in vivo imaging are essential to establish standardized approaches for diagnosing and monitoring the progression of neurological diseases.
Author contributions
FE: Writing—original draft, Writing—review and editing. DH: Supervision, Writing—review and editing. AW: Writing—review and editing. SD: Funding acquisition, Supervision, Writing—original draft, Writing—review and editing.
Funding
The author(s) declare that no financial support was received for the research, authorship, and/or publication of this article.
Conflict of interest
The authors declare that the research was conducted in the absence of any commercial or financial relationships that could be construed as a potential conflict of interest.
The author(s) declared that they were an editorial board member of Frontiers, at the time of submission. This had no impact on the peer review process and the final decision.
Publisher’s note
All claims expressed in this article are solely those of the authors and do not necessarily represent those of their affiliated organizations, or those of the publisher, the editors and the reviewers. Any product that may be evaluated in this article, or claim that may be made by its manufacturer, is not guaranteed or endorsed by the publisher.
References
Ahmad, R., Postnov, A., Bormans, G., Versijpt, J., Vandenbulcke, M., and Van Laere, K. (2016). Decreased in vivo availability of the cannabinoid type 2 receptor in Alzheimer’s disease. Eur. J. Nucl. Med. Mol. Imaging 43, 2219–2227.
Ardaya, M., Joya, A., Padro, D., Plaza-García, S., Gómez-Vallejo, V., Sánchez, M., et al. (2020). In vivo PET imaging of gliogenesis after cerebral ischemia in rats. Front. Neurosci. 14:793. doi: 10.3389/fnins.2020.00793
Ashraf, G., McGuinness, M., Khan, M. A., Obtinalla, C., Hadoux, X., and van Wijngaarden, P. (2023). Retinal imaging biomarkers of Alzheimer’s disease: a systematic review and meta-analysis of studies using brain amyloid beta status for case definition. Alzheimers Dement. (Amst.) 15:e12421. doi: 10.1002/dad2.12421
Attfield, K. E., Jensen, L. T., Kaufmann, M., Friese, M. A., and Fugger, L. (2022). The immunology of multiple sclerosis. Nat. Rev. Immunol. 22, 734–750.
Bajwa, A., Aman, R., and Reddy, A. K. (2015). A comprehensive review of diagnostic imaging technologies to evaluate the retina and the optic disk. Int. Ophthalmol. 35, 733–755.
Banati, R. B., Newcombe, J., Gunn, R. N., Cagnin, A., Turkheimer, F., Heppner, F., et al. (2000). The peripheral benzodiazepine binding site in the brain in multiple sclerosis: quantitative in vivo imaging of microglia as a measure of disease activity. Brain 123, 2321–2337. doi: 10.1093/brain/123.11.2321
Bartolo, N. D., Mortimer, N., Manter, M. A., Sanchez, N., Riley, M., O’Malley, T. T., et al. (2022). Identification and prioritization of PET neuroimaging targets for microglial phenotypes associated with microglial activity in Alzheimer’s disease. ACS Chem. Neurosci. 13, 3641–3660. doi: 10.1021/acschemneuro.2c00607
Beaino, W., Janssen, B., Kooij, G., van der Pol, S. M. A., van Het Hof, B., van Horssen, J., et al. (2017). Purinergic receptors P2Y12R and P2X7R: potential targets for PET imaging of microglia phenotypes in multiple sclerosis. J. Neuroinflammation 14:259. doi: 10.1186/s12974-017-1034-z
Beaino, W., Janssen, B., Kooijman, E., Vos, R., Schuit, R. C., O’Brien-Brown, J., et al. (2020). PET imaging of P2X(7)R in the experimental autoimmune encephalomyelitis model of multiple sclerosis using [(11)C]SMW139. J. Neuroinflammation 17:300.
Beaino, W., Janssen, B., Vugts, D. J., de Vries, H. E., and Windhorst, A. D. (2021). Towards PET imaging of the dynamic phenotypes of microglia. Clin. Exp. Immunol. 206, 282–300.
Benito, C., Núñez, E., Tolón, R. M., Carrier, E. J., Rábano, A., Hillard, C. J., et al. (2003). Cannabinoid CB2 receptors and fatty acid amide hydrolase are selectively overexpressed in neuritic plaque-associated glia in Alzheimer’s disease brains. J. Neurosci. 23, 11136–11141. doi: 10.1523/JNEUROSCI.23-35-11136.2003
Bsteh, G., Hegen, H., Altmann, P., Auer, M., Berek, K., Di Pauli, F., et al. (2023). Retinal layer thickness predicts disability accumulation in early relapsing multiple sclerosis. Eur. J. Neurol. 30, 1025–1034. doi: 10.1111/ene.15718
Cabrera DeBuc, D., Gaca-Wysocka, M., Grzybowski, A., and Kanclerz, P. (2019). Identification of retinal biomarkers in Alzheimer’s disease using optical coherence tomography: recent insights, challenges, and opportunities. J. Clin. Med. 8:996. doi: 10.3390/jcm8070996
Cagol, A., Fuertes, N. C., Stoessel, M., Barakovic, M., Schaedelin, S., D’Souza, M., et al. (2023). Optical coherence tomography reflects clinically relevant gray matter damage in patients with multiple sclerosis. J. Neurol. 270, 2139–2148. doi: 10.1007/s00415-022-11535-8
Catana, C. (2019). Development of dedicated brain PET imaging devices: recent advances and future perspectives. J. Nucl. Med. 60, 1044–1052. doi: 10.2967/jnumed.118.217901
Cennamo, G., Romano, M. R., Vecchio, E. C., Minervino, C., della Guardia, C., Velotti, N., et al. (2016). Anatomical and functional retinal changes in multiple sclerosis. Eye 30, 456–462.
Chandra, A., Valkimadi, P.-E., Pagano, G., Cousins, O., Dervenoulas, G., Politis, M., et al. (2019). Applications of amyloid, tau, and neuroinflammation PET imaging to Alzheimer’s disease and mild cognitive impairment. Hum. Brain Mapp. 40, 5424–5442.
Cherry, J. D., Olschowka, J. A., and O’Banion, M. K. (2015). Arginase 1+ microglia reduce Aβ plaque deposition during IL-1β-dependent neuroinflammation. J. Neuroinflammation 12:203.
Chiasseu, M., Alarcon-Martinez, L., Belforte, N., Quintero, H., Dotigny, F., Destroismaisons, L., et al. (2017). Tau accumulation in the retina promotes early neuronal dysfunction and precedes brain pathology in a mouse model of Alzheimer’s disease. Mol. Neurodegener. 12:58. doi: 10.1186/s13024-017-0199-3
Chitu, V., and Stanley, E. R. (2006). Colony-stimulating factor-1 in immunity and inflammation. Curr. Opin. Immunol. 18, 39–48.
Choi, S., Guo, L., and Cordeiro, M. F. (2021). Retinal and brain microglia in multiple sclerosis and neurodegeneration. Cells 10:1507.
Claes, C., Danhash, E. P., Hasselmann, J., Chadarevian, J. P., Shabestari, S. K., England, W. E., et al. (2021). Plaque-associated human microglia accumulate lipid droplets in a chimeric model of Alzheimer’s disease. Mol. Neurodegener. 16:50. doi: 10.1186/s13024-021-00473-0
Coda, A. R., Anzilotti, S., Boscia, F., Greco, A., Panico, M., Gargiulo, S., et al. (2021). In vivo imaging of CNS microglial activation/macrophage infiltration with combined [(18)F]DPA-714-PET and SPIO-MRI in a mouse model of relapsing remitting experimental autoimmune encephalomyelitis. Eur. J. Nucl. Med. Mol. Imaging 48, 40–52.
Coughlin, J. M., Du, Y., Lesniak, W. G., Harrington, C. K., Brosnan, M. K., O’Toole, R., et al. (2022). First-in-human use of 11C-CPPC with positron emission tomography for imaging the macrophage colony-stimulating factor 1 receptor. EJNMMI Res. 12:64. doi: 10.1186/s13550-022-00929-4
Cruz-Herranz, A., Oertel, F. C., Kim, K., Cantó, E., Timmons, G., Sin, J. H., et al. (2021). Distinctive waves of innate immune response in the retina in experimental autoimmune encephalomyelitis. JCI Insight 6:e149228. doi: 10.1172/jci.insight.149228
Czakó, C., Kovács, T., Ungvari, Z., Csiszar, A., Yabluchanskiy, A., Conley, S., et al. (2020). Retinal biomarkers for Alzheimer’s disease and vascular cognitive impairment and dementia (VCID): implication for early diagnosis and prognosis. Geroscience 42, 1499–1525. doi: 10.1007/s11357-020-00252-7
Dani, M., Wood, M., Mizoguchi, R., Fan, Z., Walker, Z., Morgan, R., et al. (2018). Microglial activation correlates in vivo with both tau and amyloid in Alzheimer’s disease. Brain 141, 2740–2754.
de Carlo, T. E., Romano, A., Waheed, N. K., and Duker, J. S. (2015). A review of optical coherence tomography angiography (OCTA). Int. J. Retina Vitreous 1:5.
den Haan, J., Morrema, T. H. J., Verbraak, F. D., de Boer, J. F., Scheltens, P., Rozemuller, A. J., et al. (2018). Amyloid-beta and phosphorylated tau in post-mortem Alzheimer’s disease retinas. Acta Neuropathol. Commun. 6:147.
Elmore, M. R., Najafi, A. R., Koike, M. A., Dagher, N. N., Spangenberg, E. E., Rice, R. A., et al. (2014). Colony-stimulating factor 1 receptor signaling is necessary for microglia viability, unmasking a microglia progenitor cell in the adult brain. Neuron 82, 380–397. doi: 10.1016/j.neuron.2014.02.040
Evens, N., Vandeputte, C., Coolen, C., Janssen, P., Sciot, R., Baekelandt, V., et al. (2012). Preclinical evaluation of [11C]NE40, a type 2 cannabinoid receptor PET tracer. Nucl. Med. Biol. 39, 389–399. doi: 10.1016/j.nucmedbio.2011.09.005
Fan, Z., Brooks, D. J., Okello, A., and Edison, P. (2017). An early and late peak in microglial activation in Alzheimer’s disease trajectory. Brain 140, 792–803. doi: 10.1093/brain/aww349
Flowers, A., Bell-Temin, H., Jalloh, A., Stevens, S. M., and Bickford, P. C. (2017). Proteomic analysis of aged microglia: shifts in transcription, bioenergetics, and nutrient response. J. Neuroinflammation 14:96. doi: 10.1186/s12974-017-0840-7
Francistiová, L., Bianchi, C., Di Lauro, C., Sebastián-Serrano, Á, de Diego-García, L., Kobolák, J., et al. (2020). The role of P2X7 receptor in Alzheimer’s disease. Front. Mol. Neurosci. 13:94. doi: 10.3389/fnmol.2020.00094
Frigerio, C. S., Wolfs, L., Fattorelli, N., Thrupp, N., Voytyuk, I., Schmidt, I., et al. (2019). The major risk factors for Alzheimer’s disease: age, sex, and genes modulate the microglia response to Aβ plaques. Cell Rep. 27, 1293–1306.e6.
Gabandé-Rodríguez, E., Keane, L., and Capasso, M. (2020). Microglial phagocytosis in aging and Alzheimer’s disease. J. Neurosci. Res. 98, 284–298.
Gao, C., Jiang, J., Tan, Y., and Chen, S. (2023). Microglia in neurodegenerative diseases: mechanism and potential therapeutic targets. Signal Transduct. Target. Ther. 8:359.
Gazestani, V., Kamath, T., Nadaf, N. M., Dougalis, A., Burris, S. J., Rooney, B., et al. (2023). Early Alzheimer’s disease pathology in human cortex involves transient cell states. Cell 186, 4438–4453.e23. doi: 10.1016/j.cell.2023.08.005
Geng, Y., Dubra, A., Yin, L., Merigan, W. H., Sharma, R., Libby, R. T., et al. (2012). Adaptive optics retinal imaging in the living mouse eye. Biomed. Opt. Express 3, 715–734.
Gernert, J. A., Böhm, L., Starck, M., Buchka, S., Kümpfel, T., Kleiter, I., et al. (2023). Inner retinal layer changes reflect changes in ambulation score in patients with primary progressive multiple sclerosis. Int. J. Mol. Sci. 24:12872. doi: 10.3390/ijms241612872
Ginhoux, F., Greter, M., Leboeuf, M., Nandi, S., See, P., Gokhan, S., et al. (2010). Fate mapping analysis reveals that adult microglia derive from primitive macrophages. Science 330, 841–845. doi: 10.1126/science.1194637
Grimaldi, A., Brighi, C., Peruzzi, G., Ragozzino, D., Bonanni, V., Limatola, C., et al. (2018). Inflammation, neurodegeneration and protein aggregation in the retina as ocular biomarkers for Alzheimer’s disease in the 3xTg-AD mouse model. Cell Death Dis. 9:685. doi: 10.1038/s41419-018-0740-5
Grimaldi, A., Pediconi, N., Oieni, F., Pizzarelli, R., Rosito, M., Giubettini, M., et al. (2019). Neuroinflammatory processes, A1 astrocyte activation and protein aggregation in the retina of Alzheimer’s disease patients, possible biomarkers for early diagnosis. Front. Neurosci. 13:925. doi: 10.3389/fnins.2019.00925
Gui, Y., Marks, J. D., Das, S., Hyman, B. T., and Serrano-Pozo, A. (2020). Characterization of the 18 kDa translocator protein (TSPO) expression in post-mortem normal and Alzheimer’s disease brains. Brain Pathol. 30, 151–164. doi: 10.1111/bpa.12763
Guo, L., Choi, S., Bikkannavar, P., and Cordeiro, M. F. (2022). Microglia: key players in retinal ageing and neurodegeneration. Front. Cell. Neurosci. 16:804782. doi: 10.3389/fncel.2022.804782
Guo, M., Wang, J., Zhao, Y., Feng, Y., Han, S., Dong, Q., et al. (2020). Microglial exosomes facilitate α-synuclein transmission in Parkinson’s disease. Brain 143, 1476–1497. doi: 10.1093/brain/awaa090
Hagan, N., Kane, J. L., Grover, D., Woodworth, L., Madore, C., Saleh, J., et al. (2020). CSF1R signaling is a regulator of pathogenesis in progressive MS. Cell Death Dis. 11:904.
Hagens, M. H. J., Golla, S. S. V., Janssen, B., Vugts, D. J., Beaino, W., Windhorst, A. D., et al. (2020). The P2X7 receptor tracer [11C]SMW139 as an in vivo marker of neuroinflammation in multiple sclerosis: a first-in man study. Eur. J. Nucl. Med. Mol. Imaging 47, 379–389.
Haimon, Z., Frumer, G. R., Kim, J.-S., Trzebanski, S., Haffner-Krausz, R., Ben-Dor, S., et al. (2022). Cognate microglia–T cell interactions shape the functional regulatory T cell pool in experimental autoimmune encephalomyelitis pathology. Nat. Immunol. 23, 1749–1762. doi: 10.1038/s41590-022-01360-6
Hammer, D. X., Agrawal, A., Villanueva, R., Saeedi, O., and Liu, Z. (2020). Label-free adaptive optics imaging of human retinal macrophage distribution and dynamics. Proc. Natl. Acad. Sci. U.S.A. 117, 30661–30669. doi: 10.1073/pnas.2010943117
Hammond, T. R., Dufort, C., Dissing-Olesen, L., Giera, S., Young, A., Wysoker, A., et al. (2019). Single-cell RNA sequencing of microglia throughout the mouse lifespan and in the injured brain reveals complex cell-state changes. Immunity 50, 253–271.e6. doi: 10.1016/j.immuni.2018.11.004
Hansen, D. V., Hanson, J. E., and Sheng, M. (2017). Microglia in Alzheimer’s disease. J. Cell Biol. 217, 459–472.
Hoozemans, J. J., Rozemuller, A. J., Janssen, I., De Groot, C. J., Veerhuis, R., and Eikelenboom, P. (2001). Cyclooxygenase expression in microglia and neurons in Alzheimer’s disease and control brain. Acta Neuropathol. 101, 2–8.
Horti, A. G., Naik, R., Foss, C. A., Minn, I., Misheneva, V., Du, Y., et al. (2019). PET imaging of microglia by targeting macrophage colony-stimulating factor 1 receptor (CSF1R). Proc. Natl. Acad. Sci. U.S.A. 116, 1686–1691.
Hu, B., Duan, S., Wang, Z., Li, X., Zhou, Y., Zhang, X., et al. (2021). Insights into the role of CSF1R in the central nervous system and neurological disorders. Front. Aging Neurosci. 13:789834. doi: 10.3389/fnagi.2021.789834
International Multiple Sclerosis Genetics Consortium, Patsopoulos, N. A., Baranzini, S. E., Santaniello, A., Shoostari, P., Cotsapas, C., et al. (2019). Multiple sclerosis genomic map implicates peripheral immune cells and microglia in susceptibility. Science 365:eaav7188. doi: 10.1126/science.aav7188
Jain, P., Chaney, A., Carlson, M. L., Jackson, I. M., Rao, A., and James, M. L. (2020). Neuroinflammation PET imaging: current opinion and future directions. J. Nucl. Med. 61, 1107–1112. doi: 10.2967/jnumed.119.229443
Jáñez-Escalada, L., Jáñez-García, L., Salobrar-García, E., Santos-Mayo, A., de Hoz, R., Yubero, R., et al. (2019). Spatial analysis of thickness changes in ten retinal layers of Alzheimer’s disease patients based on optical coherence tomography. Sci. Rep. 9:13000. doi: 10.1038/s41598-019-49353-0
Janssen, B., Vugts, D. J., Wilkinson, S. M., Ory, D., Chalon, S., Hoozemans, J. J. M., et al. (2018). Identification of the allosteric P2X7 receptor antagonist [11C]SMW139 as a PET tracer of microglial activation. Sci. Rep. 8:6580. doi: 10.1038/s41598-018-24814-0
Jin, J., Smith, M. D., Kersbergen, C. J., Kam, T.-I., Viswanathan, M., Martin, K., et al. (2019). Glial pathology and retinal neurotoxicity in the anterior visual pathway in experimental autoimmune encephalomyelitis. Acta Neuropathol. Commun. 7:125. doi: 10.1186/s40478-019-0767-6
Johnson, G. V., and Stoothoff, W. H. (2004). Tau phosphorylation in neuronal cell function and dysfunction. J. Cell Sci. 117(Pt 24), 5721–5729.
Jordão, M. J. C., Sankowski, R., Brendecke, S. M., Locatelli, G., Tai, Y.-H., Tay, T. L., et al. (2019). Single-cell profiling identifies myeloid cell subsets with distinct fates during neuroinflammation. Science 363:eaat7554. doi: 10.1126/science.aat7554
Joseph, A., Power, D., and Schallek, J. (2021). Imaging the dynamics of individual processes of microglia in the living retina in vivo. Biomed. Opt. Express 12, 6157–6183. doi: 10.1364/BOE.426157
Jung, Y., Lopez-Benitez, J., Tognoni, C. M., Carreras, I., and Dedeoglu, A. (2023). Dysregulation of sphingosine-1-phosphate (S1P) and S1P receptor 1 signaling in the 5xFAD mouse model of Alzheimer’s disease. Brain Res. 1799:148171. doi: 10.1016/j.brainres.2022.148171
Kamma, E., Lasisi, W., Libner, C., Ng, H. S., and Plemel, J. R. (2022). Central nervous system macrophages in progressive multiple sclerosis: relationship to neurodegeneration and therapeutics. J. Neuroinflammation 19:45. doi: 10.1186/s12974-022-02408-y
Kamphuis, W., Kooijman, L., Schetters, S., Orre, M., and Hol, E. M. (2016). Transcriptional profiling of CD11c-positive microglia accumulating around amyloid plaques in a mouse model for Alzheimer’s disease. Biochim. Biophys. Acta 1862, 1847–1860. doi: 10.1016/j.bbadis.2016.07.007
Kaunzner, U. W., Kang, Y., Zhang, S., Morris, E., Yao, Y., Pandya, S., et al. (2019). Quantitative susceptibility mapping identifies inflammation in a subset of chronic multiple sclerosis lesions. Brain 142, 133–145. doi: 10.1093/brain/awy296
Kazuhiro, K., James, A. C., Furu, Z., and Donald, T. M. (2020). Suite of methods for assessing inner retinal temporal dynamics across spatial and temporal scales in the living human eye. Neurophotonics 7:015013. doi: 10.1117/1.NPh.7.1.015013
Keren-Shaul, H., Spinrad, A., Weiner, A., Matcovitch-Natan, O., Dvir-Szternfeld, R., Ulland, T. K., et al. (2017). A unique microglia type associated with restricting development of Alzheimer’s disease. Cell 169, 1276–1290.e17. doi: 10.1016/j.cell.2017.05.018
Kerkhofs, D., van Hagen, B. T., Milanova, I. V., Schell, K. J., van Essen, H., Wijnands, E., et al. (2020). Pharmacological depletion of microglia and perivascular macrophages prevents Vascular Cognitive Impairment in Ang II-induced hypertension. Theranostics 10, 9512–9527. doi: 10.7150/thno.44394
Kinney, J. W., Bemiller, S. M., Murtishaw, A. S., Leisgang, A. M., Salazar, A. M., and Lamb, B. T. (2018). Inflammation as a central mechanism in Alzheimer’s disease. Alzheimers Dement. (N. Y.) 4, 575–590.
Koronyo, Y., Biggs, D., Barron, E., Boyer, D. S., Pearlman, J. A., Au, W. J., et al. (2017). Retinal amyloid pathology and proof-of-concept imaging trial in Alzheimer’s disease. JCI Insight 2:e93621. doi: 10.1172/jci.insight.93621
Koronyo, Y., Salumbides, B. C., Black, K. L., and Koronyo-Hamaoui, M. (2012). Alzheimer’s disease in the retina: imaging retinal aβ plaques for early diagnosis and therapy assessment. Neurodegener. Dis. 10, 285–293.
Koronyo-Hamaoui, M., Koronyo, Y., Ljubimov, A. V., Miller, C. A., Ko, M. K., Black, K. L., et al. (2011). Identification of amyloid plaques in retinas from Alzheimer’s patients and noninvasive in vivo optical imaging of retinal plaques in a mouse model. Neuroimage 54(Suppl. 1), S204–S217. doi: 10.1016/j.neuroimage.2010.06.020
Krasemann, S., Madore, C., Cialic, R., Baufeld, C., Calcagno, N., El Fatimy, R., et al. (2017). The TREM2-APOE pathway drives the transcriptional phenotype of dysfunctional microglia in neurodegenerative diseases. Immunity 47, 566–581.e9. doi: 10.1016/j.immuni.2017.08.008
Kuhlmann, T., Ludwin, S., Prat, A., Antel, J., Brück, W., and Lassmann, H. (2017). An updated histological classification system for multiple sclerosis lesions. Acta Neuropathol. 133, 13–24.
Lambe, J., Fitzgerald, K. C., Murphy, O. C., Filippatou, A. G., Sotirchos, E. S., Kalaitzidis, G., et al. (2021). Association of spectral-domain OCT with long-term disability worsening in multiple sclerosis. Neurology 96, e2058–e2069. doi: 10.1212/WNL.0000000000011788
Lewcock, J. W., Schlepckow, K., Di Paolo, G., Tahirovic, S., Monroe, K. M., and Haass, C. (2020). Emerging microglia biology defines novel therapeutic approaches for Alzheimer’s disease. Neuron 108, 801–821.
Liu, H., Jin, H., Yue, X., Luo, Z., Liu, C., Rosenberg, A. J., et al. (2016). PET imaging study of S1PR1 expression in a rat model of multiple sclerosis. Mol. Imaging Biol. 18, 724–732. doi: 10.1007/s11307-016-0944-y
Liu, H., Luo, Z., Gu, J., Jiang, H., Joshi, S., Shoghi, K. I., et al. (2020). In vivo characterization of four (18)F-labeled S1PR1 tracers for neuroinflammation. Mol. Imaging Biol. 22, 1362–1369. doi: 10.1007/s11307-020-01514-8
Liu, Y., Zhao, C., Meng, J., Li, N., Xu, Z., Liu, X., et al. (2022). Galectin-3 regulates microglial activation and promotes inflammation through TLR4/MyD88/NF-kB in experimental autoimmune uveitis. Clin. Immunol. 236:108939. doi: 10.1016/j.clim.2022.108939
Liu, Z., Kurokawa, K., Zhang, F., Lee, J. J., and Miller, D. T. (2017). Imaging and quantifying ganglion cells and other transparent neurons in the living human retina. Proc. Natl. Acad. Sci. U.S.A. 114, 12803–12808. doi: 10.1073/pnas.1711734114
Lloyd, A. F., and Miron, V. E. (2019). The pro-remyelination properties of microglia in the central nervous system. Nat. Rev. Neurol. 15, 447–458. doi: 10.1038/s41582-019-0184-2
Ma, Q., Shams, H., Didonna, A., Baranzini, S. E., Cree, B. A. C., Hauser, S. L., et al. (2023). Integration of epigenetic and genetic profiles identifies multiple sclerosis disease-critical cell types and genes. Commun. Biol. 6:342. doi: 10.1038/s42003-023-04713-5
Maeda, J., Minamihisamatsu, T., Shimojo, M., Zhou, X., Ono, M., Matsuba, Y., et al. (2021). Distinct microglial response against Alzheimer’s amyloid and tau pathologies characterized by P2Y12 receptor. Brain Commun. 3:fcab011. doi: 10.1093/braincomms/fcab011
Mainster, M. A., Desmettre, T., Querques, G., Turner, P. L., and Ledesma-Gil, G. (2022). Scanning laser ophthalmoscopy retroillumination: applications and illusions. Int. J. Retina Vitreous 8:71. doi: 10.1186/s40942-022-00421-0
Marsh, S. E., Walker, A. J., Kamath, T., Dissing-Olesen, L., Hammond, T. R., de Soysa, T. Y., et al. (2022). Dissection of artifactual and confounding glial signatures by single-cell sequencing of mouse and human brain. Nat. Neurosci. 25, 306–316. doi: 10.1038/s41593-022-01022-8
Martin, E., Amar, M., Dalle, C., Youssef, I., Boucher, C., Le Duigou, C., et al. (2019). New role of P2X7 receptor in an Alzheimer’s disease mouse model. Mol. Psychiatry 24, 108–125. doi: 10.1038/s41380-018-0108-3
Masuda, T., Sankowski, R., Staszewski, O., and Prinz, M. (2020). Microglia heterogeneity in the single-cell era. Cell Rep. 30, 1271–1281.
Masuda, T., Sankowski, R., Staszewski, O., Böttcher, C., Amann, L., Sagar, et al. (2019). Spatial and temporal heterogeneity of mouse and human microglia at single-cell resolution. Nature 566, 388–392.
Mawuenyega, K. G., Sigurdson, W., Ovod, V., Munsell, L., Kasten, T., Morris, J. C., et al. (2010). Decreased clearance of CNS beta-amyloid in Alzheimer’s disease. Science 330:1774.
McIlwaine, G., Csincsik, L., Coey, R., Wang, L., Fitzgerald, D., Moffat, J., et al. (2023). Reduced cone density is associated with multiple sclerosis. Ophthalmol. Sci. 3:100308. doi: 10.1016/j.xops.2023.100308
McMenamin, P. G., Saban, D. R., and Dando, S. J. (2019). Immune cells in the retina and choroid: two different tissue environments that require different defenses and surveillance. Prog. Retin. Eye Res. 70, 85–98. doi: 10.1016/j.preteyeres.2018.12.002
Michell-Robinson, M. A., Touil, H., Healy, L. M., Owen, D. R., Durafourt, B. A., Bar-Or, A., et al. (2015). Roles of microglia in brain development, tissue maintenance and repair. Brain 138(Pt 5), 1138–1159.
Miedema, A., Gerrits, E., Brouwer, N., Jiang, Q., Kracht, L., Meijer, M., et al. (2022). Brain macrophages acquire distinct transcriptomes in multiple sclerosis lesions and normal appearing white matter. Acta Neuropathol. Commun. 10:8. doi: 10.1186/s40478-021-01306-3
Mildner, A., Huang, H., Radke, J., Stenzel, W., and Priller, J. (2017). P2Y12 receptor is expressed on human microglia under physiological conditions throughout development and is sensitive to neuroinflammatory diseases. Glia 65, 375–387. doi: 10.1002/glia.23097
Miller, E. B., Zhang, P., Ching, K., Pugh, E. N., and Burns, M. E. (2019). In vivo imaging reveals transient microglia recruitment and functional recovery of photoreceptor signaling after injury. Proc. Natl. Acad. Sci. U.S.A. 116, 16603–16612. doi: 10.1073/pnas.1903336116
Montilla, A., Zabala, A., Er-Lukowiak, M., Rissiek, B., Magnus, T., Rodriguez-Iglesias, N., et al. (2023). Microglia and meningeal macrophages depletion delays the onset of experimental autoimmune encephalomyelitis. Cell Death Dis. 14:16. doi: 10.1038/s41419-023-05551-3
Namekata, K., Guo, X., Kimura, A., Arai, N., Harada, C., and Harada, T. (2019). DOCK8 is expressed in microglia, and it regulates microglial activity during neurodegeneration in murine disease models. J. Biol. Chem. 294, 13421–13433. doi: 10.1074/jbc.RA119.007645
Noailles, A., Fernández-Sánchez, L., Lax, P., and Cuenca, N. (2014). Microglia activation in a model of retinal degeneration and TUDCA neuroprotective effects. J. Neuroinflammation 11:186. doi: 10.1186/s12974-014-0186-3
Nutma, E., Gebro, E., Marzin, M. C., van der Valk, P., Matthews, P. M., Owen, D. R., et al. (2021). Activated microglia do not increase 18 kDa translocator protein (TSPO) expression in the multiple sclerosis brain. Glia 69, 2447–2458.
Nutma, E., Stephenson, J. A., Gorter, R. P., de Bruin, J., Boucherie, D. M., Donat, C. K., et al. (2019). A quantitative neuropathological assessment of translocator protein expression in multiple sclerosis. Brain 142, 3440–3455. doi: 10.1093/brain/awz287
Olah, M., Biber, K., Vinet, J., and Boddeke, H. W. (2011). Microglia phenotype diversity. CNS Neurol. Disord. Drug Targets 10, 108–118.
Olah, M., Menon, V., Habib, N., Taga, M. F., Ma, Y., Yung, C. J., et al. (2020). Single cell RNA sequencing of human microglia uncovers a subset associated with Alzheimer’s disease. Nat. Commun. 11:6129. doi: 10.1038/s41467-020-19737-2
Parbo, P., Ismail, R., Hansen, K. V., Amidi, A., Mårup, F. H., Gottrup, H., et al. (2017). Brain inflammation accompanies amyloid in the majority of mild cognitive impairment cases due to Alzheimer’s disease. Brain 140, 2002–2011. doi: 10.1093/brain/awx120
Pascoal, T. A., Benedet, A. L., Ashton, N. J., Kang, M. S., Therriault, J., Chamoun, M., et al. (2021). Microglial activation and tau propagate jointly across Braak stages. Nat. Med. 27, 1592–1599.
Pearson, T., Chen, Y., Dhillon, B., Chandran, S., van Hemert, J., and MacGillivray, T. (2022). Multi-modal retinal scanning to measure retinal thickness and peripheral blood vessels in multiple sclerosis. Sci. Rep. 12:20472. doi: 10.1038/s41598-022-24312-4
Peferoen, L. A. N., Vogel, D. Y. S., Ummenthum, K., Breur, M., Heijnen, P. D. A. M., Gerritsen, W. H., et al. (2015). Activation status of human microglia is dependent on lesion formation stage and remyelination in multiple sclerosis. J. Neuropathol. Exp. Neurol. 74, 48–63. doi: 10.1097/NEN.0000000000000149
Pengo, M., Miante, S., Franciotta, S., Ponzano, M., Torresin, T., Bovis, F., et al. (2022). Retinal hyperreflecting foci associate with cortical pathology in multiple sclerosis. Neurol. Neuroimmunol. Neuroinflammation 9:e1180.
Petzold, A., Balcer, L. J., Calabresi, P. A., Costello, F., Frohman, T. C., Frohman, E. M., et al. (2017). Retinal layer segmentation in multiple sclerosis: a systematic review and meta-analysis. Lancet Neurol. 16, 797–812.
Pike, V. W. (2009). PET radiotracers: crossing the blood–brain barrier and surviving metabolism. Trends Pharmacol. Sci. 30, 431–440. doi: 10.1016/j.tips.2009.05.005
Pilotto, E., Miante, S., Torresin, T., Puthenparampil, M., Frizziero, L., Federle, L., et al. (2020). Hyperreflective foci in the retina of active relapse-onset multiple sclerosis. Ophthalmology 127, 1774–1776. doi: 10.1016/j.ophtha.2020.03.024
Politis, M., Su, P., and Piccini, P. (2012b). Imaging of microglia in patients with neurodegenerative disorders. Front. Pharmacol. 3:96. doi: 10.3389/fphar.2012.00096
Politis, M., Giannetti, P., Su, P., Turkheimer, F., Keihaninejad, S., Wu, K., et al. (2012a). Increased PK11195 PET binding in the cortex of patients with MS correlates with disability. Neurology 79, 523–530. doi: 10.1212/WNL.0b013e3182635645
Prater, K. E., Green, K. J., Sun, W., Smith, C. L., Chiou, K. L., Heath, L., et al. (2021). Transcriptomic profiling of myeloid cells in Alzheimer’s Disease brain illustrates heterogeneity of microglia endolysosomal subtypes. bioRxiv [preprint]. doi: 10.1101/2021.10.25.465802
Ramirez, A. I., de Hoz, R., Salobrar-Garcia, E., Salazar, J. J., Rojas, B., Ajoy, D., et al. (2017). The role of microglia in retinal neurodegeneration: Alzheimer’s disease, parkinson, and glaucoma. Front. Aging Neurosci. 9:214. doi: 10.3389/fnagi.2017.00214
Rauchmann, B.-S., Brendel, M., Franzmeier, N., Trappmann, L., Zaganjori, M., Ersoezlue, E., et al. (2022). Microglial activation and connectivity in Alzheimer disease and aging. Ann. Neurol. 92, 768–781.
Rissanen, E., Tuisku, J., Rokka, J., Paavilainen, T., Parkkola, R., Rinne, J. O., et al. (2014). In Vivo detection of diffuse inflammation in secondary progressive multiple sclerosis using PET imaging and the radioligand 11C-PK11195. J. Nucl. Med. 55, 939–944. doi: 10.2967/jnumed.113.131698
Roy, E. R., Chiu, G., Li, S., Propson, N. E., Kanchi, R., Wang, B., et al. (2022). Concerted type I interferon signaling in microglia and neural cells promotes memory impairment associated with amyloid β plaques. Immunity 55, 879–894.e6.
Saidha, S., Al-Louzi, O., Ratchford, J. N., Bhargava, P., Oh, J., Newsome, S. D., et al. (2015). Optical coherence tomography reflects brain atrophy in multiple sclerosis: a four-year study. Ann. Neurol. 78, 801–813. doi: 10.1002/ana.24487
Salobrar-Garcia, E., Hoyas, I., Leal, M., de Hoz, R., Rojas, B., Ramirez, A. I., et al. (2015). Analysis of retinal peripapillary segmentation in early Alzheimer’s disease patients. Biomed Res. Int. 2015:636548. doi: 10.1155/2015/636548
Salobrar-García, E., Rodrigues-Neves, A. C., Ramírez, A. I., de Hoz, R., Fernández-Albarral, J. A., López-Cuenca, I., et al. (2020). Microglial activation in the retina of a triple-transgenic Alzheimer’s disease mouse model (3xTg-AD). Int. J. Mol. Sci. 21:816. doi: 10.3390/ijms21030816
Sasaki, Y., Hoshi, M., Akazawa, C., Nakamura, Y., Tsuzuki, H., Inoue, K., et al. (2003). Selective expression of Gi/o-coupled ATP receptor P2Y12 in microglia in rat brain. Glia 44, 242–250. doi: 10.1002/glia.10293
Savonenko, A. V., Melnikova, T., Wang, Y., Ravert, H., Gao, Y., Koppel, J., et al. (2015). Cannabinoid CB2 receptors in a mouse model of Aβ amyloidosis: immunohistochemical analysis and suitability as a PET biomarker of neuroinflammation. PLoS One 10:e0129618. doi: 10.1371/journal.pone.0129618
Schirmer, L., Velmeshev, D., Holmqvist, S., Kaufmann, M., Werneburg, S., Jung, D., et al. (2019). Neuronal vulnerability and multilineage diversity in multiple sclerosis. Nature 573, 75–82. doi: 10.1038/s41586-019-1404-z
Shi, Y., Manis, M., Long, J., Wang, K., Sullivan, P. M., Remolina Serrano, J., et al. (2019). Microglia drive APOE-dependent neurodegeneration in a tauopathy mouse model. J. Exp. Med. 216, 2546–2561. doi: 10.1084/jem.20190980
Shrestha, S., Kim, M.-J., Eldridge, M., Lehmann, M. L., Frankland, M., Liow, J.-S., et al. (2020). PET measurement of cyclooxygenase-2 using a novel radioligand: upregulation in primate neuroinflammation and first-in-human study. J. Neuroinflammation 17:140. doi: 10.1186/s12974-020-01804-6
Shukuri, M., Mawatari, A., Ohno, M., Suzuki, M., Doi, H., Watanabe, Y., et al. (2016). Detection of cyclooxygenase-1 in activated microglia during amyloid plaque progression: PET studies in Alzheimer’s disease model mice. J. Nucl. Med. 57, 291–296. doi: 10.2967/jnumed.115.166116
Snyder, P. J., Alber, J., Alt, C., Bain, L. J., Bouma, B. E., Bouwman, F. H., et al. (2021). Retinal imaging in Alzheimer’s and neurodegenerative diseases. Alzheimers Dement. 17, 103–111.
Sucksdorff, M., Matilainen, M., Tuisku, J., Polvinen, E., Vuorimaa, A., Rokka, J., et al. (2020). Brain TSPO-PET predicts later disease progression independent of relapses in multiple sclerosis. Brain 143, 3318–3330. doi: 10.1093/brain/awaa275
Tournier, B. B., Tsartsalis, S., Ceyzériat, K., Garibotto, V., and Millet, P. (2020). In vivo TSPO signal and neuroinflammation in Alzheimer’s disease. Cells 9:1941.
Tronel, C., Largeau, B., Santiago Ribeiro, M. J., Guilloteau, D., Dupont, A.-C., and Arlicot, N. (2017). Molecular targets for PET imaging of activated microglia: the current situation and future expectations. Int. J. Mol. Sci. 18:802. doi: 10.3390/ijms18040802
Tucker, E. W., Pokkali, S., Zhang, Z., DeMarco, V. P., Klunk, M., Smith, E. S., et al. (2016). Microglia activation in a pediatric rabbit model of tuberculous meningitis. Dis. Model. Mech. 9, 1497–1506. doi: 10.1242/dmm.027326
Usta, N. C., and Gunay, B. O. (2023). Is the ganglion cell layer thickness to macular thickness ratio a new biomarker for multiple sclerosis? Int. Ophthalmol. 43, 3841–3852. doi: 10.1007/s10792-023-02839-3
van der Poel, M., Ulas, T., Mizee, M. R., Hsiao, C.-C., Miedema, S. S. M., Adelia, et al. (2019). Transcriptional profiling of human microglia reveals grey–white matter heterogeneity and multiple sclerosis-associated changes. Nat. Commun. 10:1139. doi: 10.1038/s41467-019-08976-7
van der Wildt, B., Janssen, B., Pekošak, A., Stéen, E. J. L., Schuit, R. C., Kooijman, E. J. M., et al. (2021). Novel thienopyrimidine-based PET tracers for P2Y12 receptor imaging in the brain. ACS Chem. Neurosci. 12, 4465–4474.
Van Velthoven, M. E. J., Verbraak, F. D., Yannuzzi, L. A., Rosen, R. B., Podoleanu, A. G. H., and De Smet, M. D. (2006). Imaging the retina by en face optical coherence tomography. Retina 26, 129–136.
Vij, R., and Arora, S. (2022). A systematic survey of advances in retinal imaging modalities for Alzheimer’s disease diagnosis. Metab. Brain Dis. 37, 2213–2243. doi: 10.1007/s11011-022-00927-4
Villa, A., Klein, B., Janssen, B., Pedragosa, J., Pepe, G., Zinnhardt, B., et al. (2018). Identification of new molecular targets for PET imaging of the microglial anti-inflammatory activation state. Theranostics 8, 5400–5418. doi: 10.7150/thno.25572
Voet, S., Prinz, M., and van Loo, G. (2019). Microglia in central nervous system inflammation and multiple sclerosis pathology. Trends Mol. Med. 25, 112–123.
Vujosevic, S., Parra, M. M., Hartnett, M. E., O’Toole, L., Nuzzi, A., Limoli, C., et al. (2023). Optical coherence tomography as retinal imaging biomarker of neuroinflammation/neurodegeneration in systemic disorders in adults and children. Eye 37, 203–219.
Walker, D. G., Tang, T. M., Mendsaikhan, A., Tooyama, I., Serrano, G. E., Sue, L. I., et al. (2020). Patterns of expression of purinergic receptor P2RY12, a putative marker for non-activated microglia, in aged and Alzheimer’s disease brains. Int. J. Mol. Sci. 21:678. doi: 10.3390/ijms21020678
Walton, C., King, R., Rechtman, L., Kaye, W., Leray, E., Marrie, R. A., et al. (2020). Rising prevalence of multiple sclerosis worldwide: insights from the Atlas of MS, third edition. Mult. Scler. J. 26, 1816–1821. doi: 10.1177/1352458520970841
Xu, Q. A., Boerkoel, P., Hirsch-Reinshagen, V., Mackenzie, I. R., Hsiung, G.-Y. R., Charm, G., et al. (2022). Müller cell degeneration and microglial dysfunction in the Alzheimer’s retina. Acta Neuropathol. Commun. 10:145.
Xu, Y., Propson, N. E., Du, S., Xiong, W., and Zheng, H. (2021). Autophagy deficiency modulates microglial lipid homeostasis and aggravates tau pathology and spreading. Proc. Natl. Acad. Sci. U.S.A. 118:e2023418118. doi: 10.1073/pnas.2023418118
Yiangou, Y., Facer, P., Durrenberger, P., Chessell, I. P., Naylor, A., Bountra, C., et al. (2006). COX-2, CB2 and P2X7-immunoreactivities are increased in activated microglial cells/macrophages of multiple sclerosis and amyotrophic lateral sclerosis spinal cord. BMC Neurol. 6:12. doi: 10.1186/1471-2377-6-12
Zhang, C., Shen, J. K., Lam, T. T., Zeng, H. Y., Chiang, S. K., Yang, F., et al. (2005). Activation of microglia and chemokines in light-induced retinal degeneration. Mol. Vis. 11, 887–895.
Zhang, J., Shi, L., and Shen, Y. (2022). The retina: a window in which to view the pathogenesis of Alzheimer’s disease. Ageing Res. Rev. 77:101590.
Zhang, Y., Wang, Y., Shi, C., Shen, M., and Lu, F. (2021). Advances in retina imaging as potential biomarkers for early diagnosis of Alzheimer’s disease. Transl. Neurodegener. 10:6.
Zhao, Y.-F., Tang, Y., and Illes, P. (2021). Astrocytic and oligodendrocytic P2X7 receptors determine neuronal functions in the CNS. Front. Mol. Neurosci. 14:641570. doi: 10.3389/fnmol.2021.641570
Zhou, R., Ji, B., Kong, Y., Qin, L., Ren, W., Guan, Y., et al. (2021). PET imaging of neuroinflammation in Alzheimer’s disease. Front. Immunol. 12:739130. doi: 10.3389/fimmu.2021.739130
Zou, J., Tao, S., Johnson, A., Tomljanovic, Z., Polly, K., Klein, J., et al. (2020). Microglial activation, but not tau pathology, is independently associated with amyloid positivity and memory impairment. Neurobiol. Aging 85, 11–21.
Keywords: microglia, non-invasive in vivo imaging, positron emission tomography, optical coherence tomography, confocal scanning laser ophthalmoscopy, adaptive optics, Alzheimer’s disease, multiple sclerosis
Citation: Etebar F, Harkin DG, White AR and Dando SJ (2024) Non-invasive in vivo imaging of brain and retinal microglia in neurodegenerative diseases. Front. Cell. Neurosci. 18:1355557. doi: 10.3389/fncel.2024.1355557
Received: 14 December 2023; Accepted: 10 January 2024;
Published: 29 January 2024.
Edited by:
Ana María Espinosa Oliva, Seville University, SpainReviewed by:
Shweta Pradip Jadhav, Consultant, Carlsbad, CA, United StatesCopyright © 2024 Etebar, Harkin, White and Dando. This is an open-access article distributed under the terms of the Creative Commons Attribution License (CC BY). The use, distribution or reproduction in other forums is permitted, provided the original author(s) and the copyright owner(s) are credited and that the original publication in this journal is cited, in accordance with accepted academic practice. No use, distribution or reproduction is permitted which does not comply with these terms.
*Correspondence: Samantha J. Dando, c2FtYW50aGEuZGFuZG9AcXV0LmVkdS5hdQ==