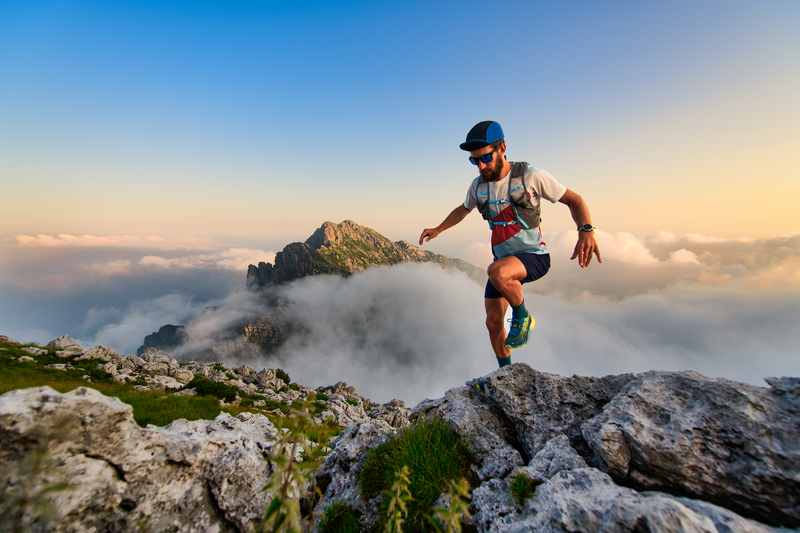
95% of researchers rate our articles as excellent or good
Learn more about the work of our research integrity team to safeguard the quality of each article we publish.
Find out more
MINI REVIEW article
Front. Cell. Neurosci. , 11 January 2024
Sec. Cellular Neurophysiology
Volume 17 - 2023 | https://doi.org/10.3389/fncel.2023.1329095
This article is part of the Research Topic Cellular and Molecular Mechanisms in Social and Repetitive Behavior: a Focus on Cortico-Striatal Circuitry View all 10 articles
The basal ganglia are major targets of cortical inputs and, in turn, modulate cortical function via their projections to the motor and prefrontal cortices. The role of the basal ganglia in motor control and reward is well documented and there is also extensive evidence that they play a key role in social and repetitive behaviors. The basal ganglia influence the activity of the cerebral cortex via two major projections from the striatum to the output nuclei, the globus pallidus internus and the substantia nigra, pars reticulata. This modulation involves a direct projection known as the direct pathway and an indirect projection via the globus pallidus externus and the subthalamic nucleus, known as the indirect pathway. This review discusses the respective contribution of the direct and indirect pathways to social and repetitive behaviors in neurotypical conditions and in autism spectrum disorders.
It is well documented that the cortico-striatal projection is altered in experimental models of Autism Spectrum Disorders (ASD) and in humans with ASD (reviews in Shepherd, 2013; Fuccillo, 2016; Kuo and Liu, 2019; Masuda et al., 2019; Li and Pozzo-Miller, 2020; Leisman et al., 2023). Imaging studies have found both hyper- and hypo-connectivity of cortico-striatal projections but most studies at the cellular level in rodent models suggest that cortico-striatal projections are depressed in ASD (reviews in Shepherd, 2013; Fuccillo, 2016; Masuda et al., 2019; Leisman et al., 2023). Cortico-striato-thalamo-cortical pathways are organized into distinct parallel limbic, associative and motor circuits (Alexander and Crutcher, 1990; Haber, 2003; Kim and Hikosaka, 2013; Lee et al., 2020). Limbic circuits primarily involve projections from the hippocampus, amygdala and limbic-associated cortices to the ventral-most striatum (i.e., nucleus accumbens) and are involved in reward and motivational aspects of behavior. Associative circuits primarily involve projections from prefrontal and other associative cortical areas to dorso-medial striatal regions (and anterior regions in primates) and have been associated with action-value learning and goal-directed behavior. Motor circuits primarily involve projections from motor and pre-motor cortices to dorso-lateral striatal regions (and posterior regions in primates) and have been associated with automatic movements and habits (e.g., Balleine et al., 2007; Kim and Hikosaka, 2013). There is also evidence that these circuits interact with each other and may contribute to several aspects of action-value learning and motor behavior. For instance, both the nucleus accumbens and the dorsomedial striatum are involved in the motivational and performance aspects of cued and non-cued generated, sequential actions (Fraser et al., 2023). The cerebral cortex controls basal ganglia outputs by modulating the activity of the so-called direct and indirect pathways in each of these circuits (reviews in Surmeier et al., 2010; Lanciego et al., 2012). Evidence for a contribution of the indirect pathway to repetitive behaviors in ASD has been discussed elsewhere (i.e., Lovinger, 2017; Tian et al., 2022). The objective in this review is to discuss the relative contribution of the direct and indirect pathways to behaviors relevant to ASD.
The direct pathway involves a projection from medium spiny neurons (dMSNs) to the globus pallidus internus (Gpi or rodent entopeduncular nucleus) and pars reticulata of the substantia nigra (SNr) and a projection from medium spiny neurons (iMSNs) to the globus pallidus externus (Gpe or rodent globus pallidus) (review in Lanciego et al., 2012). It is noteworthy that MSNs of the nucleus accumbens primarily project to limbic regions and the ventral pallidum and mesencephalon (review in Groenewegen et al., 2016). In addition, dMSNs exert a key control on both the ventral pallidum and ventral mesencephalon while iMSNs primarily project to the ventral pallidum (Kupchik et al., 2015). Based on the circuitry, the concept of direct and indirect pathway in the nucleus accumbens has been re-evaluated (Kupchik et al., 2015). Dopamine is a key modulator of excitatory corticostriatal and thalamostriatal projections. It enhances the responsiveness of dMSNs to glutamatergic inputs by binding to dopamine D1 receptors whereas it depresses the responsiveness of iMSNs by binding to D2 receptors (Cepeda et al., 2001; Surmeier et al., 2010; Planert et al., 2013). Some striatal projection neurons co-express the two types of receptors and/or the D3 receptor but their function is unclear (Gagnon et al., 2017; Gayden et al., 2023 also reviewed in Soghomonian, 2016). Current evidence indicates that the basal ganglia direct and indirect pathways are components of different cortico-striatal circuits, which may play distinct roles in ASD behavioral phenotypes. Earlier gene expression studies have shown that the sensorimotor cortex preferentially activates iMSNs (Berretta et al., 1997; Parthasarathy and Graybiel, 1997; Miyachi et al., 2005). Recent studies using genetically engineered viral tracers in rodents show that dMSNs neurons receive preferential projections from secondary motor, secondary visual, and limbic cortices while iMSNs receive input preferentially from motor cortical regions (Wall et al., 2013; Lu et al., 2021). Both the limbic and motor cortices are involved in ASD behaviors (Nebel et al., 2014; Subramanian et al., 2017; An et al., 2021). Cortico-striatal projections arise from two distinct populations of pyramidal-tract and intra-telencephalic neurons. ASD has been indirectly linked to an imbalance between these two projections (Shepherd, 2013) and morphological differences have been documented in some pyramidal-tract neurons in a ltgb3-mouse model of ASD (Celora et al., 2023). Inhibition of pyramidal-tract neurons reduces drug-induced conditioned taste aversion while inhibition of intra-telencephalic neurons increases drug-induced conditioned place preference, suggesting a different contribution to reward and aversion (Garcia et al., 2021). Altogether, these data suggest that dMSNs and iMSNs, integrate signals from several regional and cellular cortico-striatal sources but how this integration occurs is still poorly understood.
Several studies have found decreased amplitude and/or frequency of mEPSCs and field population spikes, altered ratio of NMDA/AMPA receptors and altered glutamate-dependent LTP and LTD, in the striatum of ASD rodent models (Peça et al., 2011; Wang et al., 2017; reviews in Kim et al., 2016 and Li and Pozzo-Miller, 2020) and imaging studies have reported reduced fronto-striatal connectivity, as measured in Shank3 mutant mice (Pagani et al., 2019). Proton magnetic resonance spectroscopy studies have also documented decreased cortico-striatal glutamate levels in ASD subjects and in rodents with mutations of the ASD-implicated gene, neuroligin 3 (Horder et al., 2018). Decreased ratio between cortical glutamate and GABA is associated with social behavior deficits in Cntnap2 mutant mice, another ASD model (Park et al., 2022) although another study failed to detect changes in GABA or glutamate levels in the sensory or sensorimotor cortex in ASD compared to neurotypical adults (Kolodny et al., 2020). There is also conflicting information regarding the activity of limbic-striatal circuits in ASD with evidence for increased limbic flow in cortico-limbic-striatal connectivity (Whi et al., 2020) and decreased metabolic activity in cortical limbic regions (Haznedar et al., 2000). It is unclear if these apparently conflicting reports are based on methodological differences or other variables. Different frontal cortico-striatal circuits contribute to ASD behaviors (review in Leisman et al., 2023). Interestingly, when ASD subjects are subdivided into groups exhibiting low and high repetitive behaviors, high repetitive behaviors are associated with increased limbic but reduced sensori-motor cortico-striatal connectivity (Abbott et al., 2018), consistent with the hypothesis that distinct cortico-striatal circuits are differentially impaired in relation to ASD-related behaviors.
Social and goal-directed behaviors involve the activation of brain reward circuits (e.g., Báez-Mendoza and Schultz, 2013), which are implicated in ASD (i.e., Scott-Van Zeeland et al., 2010; Dichter et al., 2012; Kohls et al., 2013). Current evidence indicates that activation of dMSNs facilitates the acquisition of reward-based and goal-directed behaviors while activation of iMSNs facilitates avoidance as well as the ability to shift goal-directed behaviors in response to changes in external and/or internal cues (review in Nakanishi et al., 2014). For instance, toxin-induced lesion of dMSNs, but not iMSNs, in the nucleus accumbens impairs preference for natural rewards, and reward-based learning (Hikida et al., 2010, 2016; Yawata et al., 2012), and loss of dMSNs in the dorsal striatum impairs goal-directed learning (Peak et al., 2020). Consistent with these data, the blockade of excitatory dopamine D1 receptors in the nucleus accumbens impairs the acquisition of appetitive rewards (Hikida et al., 2013). Conversely, toxin-induced loss of iMSNs, but not dMSNs, in the nucleus accumbens, impairs learning flexibility and the acquisition of a new task strategy in a reward-based learning visual task (Yawata et al., 2012), an effect reproduced by pharmacological stimulation of inhibitory dopamine D2 receptors (Yawata et al., 2012). Furthermore, inhibition of iMSNs in the dorsal striatum impairs the updating of goal-directed learning (Peak et al., 2020) and pharmacological activation of inhibitory D2 receptors impairs conditioned avoidance to an aversive electric shock (Hikida et al., 2013). The key results discussed in this paragraph are summarized in Table 1.
Table 1. Summarizes the data documenting the distinct role of dMSNs and iMSNs in the nucleus accumbens and dorsal striatum in reward and avoidance behavior.
Social behavior deficits in ASD may likewise involve an imbalance between dMSNs and iMSNs. Activation of the ventral tegmental-nucleus accumbens projection in control rodents facilitates social approach via the activation of dopamine D1 receptors (Gunaydin et al., 2014). In the zebrafish, social approach is paralleled by an increase in dopaminergic activity (Mahabir et al., 2013) while blockade of dopamine D1 receptors impairs social preferences (Scerbina et al., 2012). Targeted loss of the endocannabinoid receptor-mediated signaling pathway in the dorsal striatum, but not the nucleus accumbens, impairs social behavior, an effect reproduced by a targeted loss of the signaling pathway in dMSNs but not iMSNs (Shonesy et al., 2018). Loss of dMSNs in the accumbens nucleus, but not the dorsal striatum, also blunts social behavior (Le Merrer et al., 2024). Interestingly, the detrimental effect of loss of dMSNs on social behavior in mice can be reversed by the pharmacological inactivation of iMSNs (Le Merrer et al., 2024), suggesting a complementary role of the two pathways. This conclusion is also supported by evidence that optogenetic activation of iMSNs in the nucleus accumbens of stress-naïve mice induces social avoidance following a subthreshold exposure to a social defeat stressor while chronic social defeat stress is paralleled by a decreased activity of dMSNs that parallels social avoidance (Francis et al., 2015). Conversely, pharmacogenetic inhibition of iMSNs increases social interaction in social-stressed resilient mice (Francis et al., 2015). A contribution of iMSNs neurons to social behavior is further supported by studies on Gpr88, an orphan G-protein-coupled receptor intensely expressed in the striatum. Global Gpr88 knockout mice show increased locomotion, stereotypies and motor learning deficits (Quintana et al., 2012) and conditional knockout of the GABA-synthetizing enzyme, Gad67, in Gp88-expressing neurons is associated with social approach and object recognition deficits (Zhang et al., 2014). Selective deletion of Gpr88 in iMSNs, but not dMSNs, facilitates social approach (Meirsman et al., 2019). Interestingly, loss of dopamine D2 receptors in the dorsal striatum, which would presumably result in increased iMSNs activity, blunts social behavior (Lee et al., 2018) and optogenetic activation of nigro-striatal projections to the dorsal striatum impairs social preference (Lee et al., 2018), suggesting that activation of iMSNs or dMSNs in the nucleus accumbens has a distinct and possibly opposite effect on social behavior than activation in the dorsal striatum. In any case, these studies indicate that both dMSNs and iMSNs in the nucleus accumbens and dorsal striatum contribute to modulate social behavior. Because the nucleus accumbens is a key component in the limbic reward circuitry, its role in the modulation of social behaviors is not surprising. However, evidence reviewed here, as well as other studies (e.g., review in Báez-Mendoza and Schultz, 2013), indicate that the dorsal striatum also controls social behaviors. The dorsal striatum responds to reward and punishment signals and is involved in motivated behavior (Delgado et al., 2003) and tonically-active neurons in the limbic, associative and motor regions of the striatum respond to reward-associated stimuli (Marche et al., 2017). It is possible that the contribution of the dorsal striatum to social behavior involves a processing of reward as well as contingency- and motor-related signals. However, it is unclear how the information processed by limbic, associative and motor basal ganglia circuits is integrated in order to implement successful social behaviors.
The hypothesis that ASD behaviors are associated with depressed cortico-striatal inputs is supported by studies in Shank3B mutant mice (Peça et al., 2011) and adult restauration of Shank3 rescues both behavioral phenotypes and the depression in MSNs excitability (Mei et al., 2016). In addition, in Shank3 mutant mice, optical stimulation of dopamine VTA neurons, which primarily stimulate ventral striatum dMSNs, reverses social approach deficits (Bariselli et al., 2016). Similarly, impaired social behavior in the valproate model of ASD, is associated with an altered activity of dMSNs in the dorso-medial striatum (Di et al., 2022). A specific contribution of iMSNs to social behavior differences in ASD remains to be documented but, based on studies described here, one can hypothesize that an imbalance toward depressed dMSNs and/or enhanced iMSNs in the nucleus accumbens contributes to social deficits (Figure 1). Interestingly, a recent study suggests that too low or too high activation of dMSNs by D1 receptors in the nucleus accumbens impairs social behaviors (Tzanoulinou et al., 2022). This raises the intriguing possibility that the direction of altered activation of cortico-striatal projections may be less relevant to ASD behaviors than the magnitude of changes in the activity of dMSNs or iMSNs. The results described in the preceding paragraphs on social behavior are summarized in Table 2.
Figure 1. Illustrates hypothetical changes in the direct and indirect basal ganglia motor circuit associated with excessive repetitive behaviors in ASD and hypothetical changes in the limbic circuit associated with social deficits. (A) Canonical circuitry of the motor basal ganglia circuit. (B) Hypothetical changes in the motor circuit in ASD. Excessive repetitive movements would involve dysfunctional inhibition of PV + Gpe neurons by iMSNs, which provide inhibitory input to the STN. This would contribute to a deficient STN excitatory output. A possible depression of the excitatory hyper-direct pathway from the motor cortex to the STN may further contribute to depress the STN output. Although there is some evidence for depressed motor cortico-striatal input in ASD, the physiological impact of ASD on the activity of dMSNs and iMSNs in the dorsal striatum is still unclear (question mark) (C) hypothetical changes in the limbic striatal circuit in ASD. In the nucleus accumbens, depressed dMSNs activation relative to iMSNs may contribute to social behavior deficits. The impact of ASD on limbic-associated inputs onto striatal MSNs in the nucleus accumbens remains unclear (question mark). V.Mes, ventral mesencephalon. Blue lines are presumably excitatory. Red lines are presumably inhibitory. Brown lines are modulated by an interplay of inhibitory/excitatory mechanisms and the effects of ASD on these projections are not fully characterized. In addition, the targets of the ventral pallidum and ventral mesencephalon are diffuse and can modulate multiple regions that may also contribute to social differences in ASD. Broken lines indicate depressed projection.
Table 2. Summarizes the data documenting the distinct role of dMSNs and iMSNs in the nucleus accumbens and dorsal striatum in social behavior.
The role of dMSNs or iMSNs in ASD behaviors has been mainly inferred from studies that manipulate these two populations as homogenous entities. However, recent evidence suggests that different subsets of dMSNs and/or iMSNs neurons may play different roles in ASD-related behaviors. Rewarding stimuli inhibit nucleus accumbens dMSNs that project to the ventral pallidum but excite nucleus accumbens dMSNs that project to the ventral mesencephalon (Liu et al., 2022). On the other hand, only stimulation of dMSNs that project to the ventral pallidum results in place aversion (Liu et al., 2022). The possibility that different subsets of dMSNs or iMSNs as well as subsets of basal ganglia output neurons are involved in specific and different aspects of behavior is also supported by evidence that behavior-active dMSNs but behavior-inactive iMSNs encode for natural behaviors in the rodent (Varin et al., 2023) or that both increased or decreased activation of SNr projections can facilitate avoidance behavior (Hormigo et al., 2016; Almada et al., 2018). Thus, elucidating the exact contribution of dMSNs and iMSNs to social behaviors and their contribution to ASD calls for studies that can discriminate between subsets of neurons in each of these two major subpopulations of striatal efferent neurons.
Altered cortico-striatal connectivity has been associated with restricted repetitive behaviors in ASD (review in Wilkes and Lewis, 2018) and repetitive behaviors in several rodent models of ASD are associated with depressed glutamatergic activity in the striatum (reviewed in Kuo and Liu, 2019). Current evidence suggests that excessive repetitive behavior involves an imbalance between dMSNs and iMSNs. Using a DREADD approach in the Shank3 mutant mouse, excessive repetitive grooming behavior is reversed by selectively enhancing the activity of iMSN (Wang et al., 2017). Altered activity of iMSNs is also associated with repetitive behavior in the valproate model of ASD (Di et al., 2022). Spontaneous stereotypies in the deer mice are negatively correlated with enkephalin content, a marker of iMSNs but not dMSNs, and administration of an adenosine agonist, which stimulates iMSNs, attenuates repetitive behavior (Tanimura et al., 2010). Environmental enrichment in the deer mouse attenuates excessive grooming and this effect is paralleled by increased metabolic activity and dendritic spine density in the Gpe and the STN (Bechard et al., 2016), emphasizing the contribution of the basal ganglia indirect pathway to repetitive behaviors (further discussed in the following section). Deletion of Gpr88 in iMSNs, but not dMSNs, increases locomotion and stereotypies and decreases anxiety whereas deletion in dMSNs results in a deficit in motor habituation in a rotarod task (Meirsman et al., 2019). Other studies, however, indicate that dMSNs are also implicated in repetitive behavior. Mutations of different neuroligin-3, another ASD-associated gene, enhance repetitive motor routines via an effect on dMSNs but not iMSNs (Rothwell et al., 2014). Although the dorsal striatum is traditionally considered a key player in the generation of automatic repetitive movements, there is evidence that the nucleus accumbens also influences these behaviors in neurotypical brains and in ASD. For instance, the selective deletion of neuroligin-3 in dMSNs of the nucleus accumbens, but not dorsal striatum, nor deletion in iMSNs, reproduces the effect of the mutation on repetitive behavior as measured using a rotarod test (Rothwell et al., 2014). This effect is paralleled by a decreased synaptic inhibition of dMSNs (Rothwell et al., 2014). Similarly, impairment of the endocannabinoid pathway in nucleus accumbens dMSNs results in excessive repetitive grooming (Shonesy et al., 2018) and inactivation of dMSNs in the nucleus accumbens, but iMSNs in the dorsal striatum, induces stereotypies (Le Merrer et al., 2024). A contribution of the nucleus accumbens to repetitive behaviors appears consistent with recent evidence that both the nucleus accumbens and the dorsal striatum are implicated in the generation of sequential movements (Fraser et al., 2023). In addition to an imbalance between dMSNs and iMSNs, there is evidence that different subdivisions of the striatum may contribute differently to repetitive behavior. Indeed, excessive grooming behavior has been associated with an imbalance between striosome and matrix striatal compartments (Kuo and Liu, 2020; Ferhat et al., 2023). Altogether, these studies indicate that excessive repetitive behaviors involve an imbalance between iMSNs and dMSNs, with most studies supporting the hypothesis that depressed iMSNs activity and/or excessive dMSNs activity contributes to excessive repetitive behaviors.
Imaging studies show that high ASD scores are associated with decreased Gpe volume, shape or neuronal volume (Wegiel et al., 2014; Sussman et al., 2015; O’Dwyer et al., 2016; Schuetze et al., 2016; however, see also Sato et al., 2014). Excessive repetitive and stereotyped behaviors are inversely correlated with Gpe volume in ASD children 3–4 years of age (Estes et al., 2011). Two distinct subpopulations of neurons are present in the GPe. One expresses the calcium-binding protein parvalbumin (PV +) and preferentially targets the STN and SNr while a smaller subpopulation expresses the transcription factor, Npas1 and preferentially projects to the striatum (Hegeman et al., 2016; Courtney et al., 2023). A small population of PV + neurons also project to low threshold spiking NPY and fast spiking PV + striatal interneurons (Saunders et al., 2016; Courtney et al., 2023), providing a route for feedback control of MSNs. PV + GPe neurons receive a major input from iMSNs whereas Npas1 + neurons receive an input from dMSNs (Ketzef and Silberberg, 2021) and a direct input from the motor cortex (Karube et al., 2019). PV + neurons slow or pause firing when the cerebral cortex is in an upstate (Ketzef and Silberberg, 2021), consistent with a major inhibitory role of cortico-striatal projections onto Gpe neurons. Recent evidence indicates that the density of perineuronal nets is decreased in the Gpe and Gpi in post-mortem ASD brains (Brandenburg and Blatt, 2022). Perineuronal nets play a key role in the closure of critical periods, synaptic plasticity and neuronal activity and impaired perineuronal nets formation is paralleled by decreased GABAergic inhibition (Sorg et al., 2016; Fawcett et al., 2019). A deficit in perineuronal nets around PV + neurons in ASD would likely impair cortico-striatal inhibition of PV + neurons and may lead to Gpe hyperactivity. In keeping with earlier evidence that Gpe lesions disrupt grooming in rodents (Cromwell and Berridge, 1996), the possibility that excessive Gpe activity, secondary to a deficit in iMSNs-dependent inhibition, is involved in ASD deserves further investigation.
Excessive repetitive behaviors and stereotypies in ASD may also be linked to depressed STN activation. High-frequency stimulation of the STN dampens excessive grooming in ASD mutant mice models (Chang et al., 2016) and reduces stereotypies induced by the infusion of a GABA antagonist in the monkey Gpe (Baup et al., 2008). On the other hand, cytochrome oxidase activity in the STN is significantly lower in mice that exhibit high level stereotypies and is negatively correlated with the frequency of stereotypy (Tanimura et al., 2010, 2011). Repetitive behavior in C58 inbred mice is paralleled by fewer dendritic spines and decreased cytochrome oxidase levels in the STN (Lewis et al., 2018) and toxin-induced loss of the excitatory hyper-direct pathway from the motor cortex results in motor hyperactivity in mice (Koketsu et al., 2021). The hypothesis that depressed STN activity plays a role in excessive repetitive movements or stereotypies in ASD is also supported by pharmacological studies (Muehlmann et al., 2020). In addition to its role on repetitive behavior, depressed STN activity in ASD may also contribute to social deficits as loss of the STN in rats impairs social interaction (Reymann et al., 2013). Based on the evidence discussed above, one can propose a model in which excessive repetitive behaviors in ASD may involve deficient striato-Gpe inhibition leading to enhanced activity of the Gpe-STN projection and over-inhibition of the STN in motor basal ganglia circuits (Figure 1).
Gene variants associated with dopaminergic markers and receptors have been identified in ASD (De Krom et al., 2009; Staal et al., 2012; Gangi et al., 2016; Mariggiò et al., 2021). Several ASD animal models show increased gene expression of dopamine D2 receptors in iMSNs (Maisterrena et al., 2022Chhabra et al., 2023; reviewed in Gandhi and Lee, 2021). Increased striatal levels of dopamine D2 receptors was documented in Rett syndrome (Chiron et al., 1993) and more recently, increased striatal D2 receptor gene expression has been reported in ASD post-mortem brains (Brandenburg et al., 2020). In mice, knock-down of neuroligin 2, another ASD-associated gene, results in a reduction in the density of dopaminergic synapses and an increase in the number of GABAergic synapses onto MSN (Uchigashima et al., 2016). In the valproic model of ASD, dopamine levels are decreased and dopamine turnover is increased in the dorsal striatum while dopamine receptor expression is increased in the nucleus accumbens (Maisterrena et al., 2022). These convergent findings suggest that altered dopaminergic modulation of corticostriatal inputs onto MSNs is a key pathophysiological feature of ASD. Extensive loss of striatal dopaminergic innervation in models of Parkinson’s disease is paralleled by an increased gene expression of D2 receptors and an increased excitability of iMSNs (Surmeier et al., 2010; review in Soghomonian, 2016). Thus, increased expression of D2 receptors in iMSNs in ASD could be compensatory to a decreased dopaminergic input. Consistent with this possibility, in ASD, fluoro-DOPA uptake is decreased in the striatum and medial prefrontal cortex (Ernst et al., 1997; Schalbroeck et al., 2021) and phasic dopamine release is reduced in the striatum in response to a monetary incentive task (Zürcher et al., 2021). Attention deficit hyperactivity disorder, a common co-morbidity in ASD, is paralleled by decreased tonic and increased phasic dopamine release (Badgaiyan et al., 2015). In contrast to these studies, no changes in dopaminergic activity were detected in other studies of ASD (Dichter et al., 2012; Pavãl, 2017; Wang et al., 2017; Schalbroeck et al., 2021). Thus, current research suggests that an impairment in striatal dopaminergic activity in ASD would involve subtle metabolic changes in release mechanisms, which may explain the conflicting results reported in the literature.
Current research in rodent models and in ASD subjects suggests that altered cortico-striatal projections in ASD contribute to social and repetitive behaviors deficits via a dysregulation of dMSNs and iMSNs in limbic, associative and motor basal ganglia circuits. Altered dopaminergic activity would further contribute to an imbalance in the coordinated modulation of dMSNs and iMSNs. Evidence discussed in this review suggests that social behavior deficits in ASD may involve a tilted balance toward increased activity of dMSNs relative to iMSNs in the nucleus accumbens while excessive repetitive behaviors may involve a tilted balance toward decreased activity of iMSNs relative to dMSNs in the dorsal striatum. Whether such imbalances differentially involve changes in pyramidal-tract and intra-telencephalic cortico-striatal projections onto dMSNs and iMSNs is unclear and deserves further attention. In addition, although a contribution of the ventral and dorsal striatum to social and repetitive behaviors has been documented, it remains to be determined if these regions play competing or synergistic roles in these behaviors.
J-JS: Writing –original draft, Writing –review and editing.
The author(s) declare that no financial support was received for the research, authorship, and/or publication of this article.
The authors declare that the research was conducted in the absence of any commercial or financial relationships that could be construed as a potential conflict of interest.
The author(s) declared that they were an editorial board member of Frontiers, at the time of submission. This had no impact on the peer review process and the final decision.
All claims expressed in this article are solely those of the authors and do not necessarily represent those of their affiliated organizations, or those of the publisher, the editors and the reviewers. Any product that may be evaluated in this article, or claim that may be made by its manufacturer, is not guaranteed or endorsed by the publisher.
Abbott, A. E., Linke, A. C., Nair, A., Jahedi, A., Alba, L. A., Keown, C. L., et al. (2018). Repetitive behaviors in autism are linked to imbalance of corticostriatal connectivity: A functional connectivity MRI study. Soc. Cogn. Affect. Neurosci. 13, 32–42. doi: 10.1093/scan/nsx129
Alexander, G. E., and Crutcher, M. D. (1990). Functional architecture of basal ganglia circuits: Neural substrates of parallel processing. Trends Neurosci. 13, 266–271. doi: 10.1016/0166-2236(90)90107-L
Almada, R. C., Genewsky, A. J., Heinz, D. E., Kaplick, P. M., Coimbra, N. C., and Wotjak, C. T. (2018). Stimulation of the nigrotectal pathway at the level of the superior colliculus reduces threat recognition and causes a shift from avoidance to approach behavior. Front. Neural Circ. 12:36. doi: 10.3389/fncir.2018.00036
An, K. M., Ikeda, T., Hasegawa, C., Yoshimura, Y., Tanaka, S., Saito, D. N., et al. (2021). Aberrant brain oscillatory coupling from the primary motor cortex in children with autism spectrum disorders. Neuroimage Clin. 29:102560. doi: 10.1016/j.nicl.2021.102560
Badgaiyan, R. D., Sinha, S., Sajjad, M., and Wack, D. S. (2015). Attenuated tonic and enhanced phasic release of dopamine in attention deficit hyperactivity disorder. PLoS One 10:e0137326. doi: 10.1371/journal.pone.0137326
Báez-Mendoza, R., and Schultz, W. (2013). The role of the striatum in social behavior. Front. Neurosci. 7:233. doi: 10.3389/fnins.2013.00233
Balleine, B. W., Delgado, M. R., and Hikosaka, O. (2007). The role of the dorsal striatum in reward and decision-making. J. Neurosci. 27, 8161–8165.
Bariselli, S., Tzanoulinou, S., Glangetas, C., Prévost-Solié, C., Pucci, L., Viguié, J., et al. (2016). SHANK3 controls maturation of social reward circuits in the VTA. Nat. Neurosci. 19, 926–934. doi: 10.1038/nn.4319
Baup, N., Grabli, D., Karachi, C., Mounayar, S., François, C., Yelnik, J., et al. (2008). High-frequency stimulation of the anterior subthalamic nucleus reduces stereotyped behaviors in primates. J. Neurosci. 28, 8785–8788. doi: 10.1523/JNEUROSCI.2384-08.2008
Bechard, A. R., Cacodcar, N., King, M. A., and Lewis, M. H. (2016). How does environmental enrichment reduce repetitive motor behaviors? Neuronal activation and dendritic morphology in the indirect basal ganglia pathway of a mouse model. Behav. Brain Res. 299, 122–131. doi: 10.1016/j.bbr.2015.11.029
Berretta, S., Parthasarathy, H. B., and Graybiel, A. M. (1997). Local release of GABAergic inhibition in the motor cortex induces immediate-early gene expression in indirect pathway neurons of the striatum. J. Neurosci. 17, 4752–4763. doi: 10.1523/JNEUROSCI.17-12-04752.1997
Brandenburg, C., and Blatt, G. J. (2022). Region-specific alterations of perineuronal net expression in postmortem autism brain tissue. Front. Mol. Neurosci. 15:838918. doi: 10.3389/fnmol.2022.838918
Brandenburg, C., Soghomonian, J. J., Zhang, K., Sulkaj, I., Randolph, B., Kachadoorian, M., et al. (2020). Increased dopamine type 2 gene expression in the dorsal striatum in individuals with autism spectrum disorder suggests alterations in indirect pathway signaling and circuitry. Front. Cell Neurosci. 14:577858. doi: 10.3389/fncel.2020.577858
Celora, L., Jaudon, F., Vitale, C., and Cingolani, L. A. (2023). Regulation of dendritic spine length in corticopontine layer V pyramidal neurons by autism risk gene β3 integrin. Mol. Brain 16:49. doi: 10.1186/s13041-023-01031-z
Cepeda, C., Hurst, R. S., Altemus, K. L., Flores-Hernández, J., Calvert, C. R., Jokel, E. S., et al. (2001). Facilitated glutamatergic transmission in the striatum of D 2 dopamine receptor-deficient mice. J. Neurophysiol. 85, 659–670. doi: 10.1152/jn.2001.85.2.659
Chang, A. D., Berges, V. A., Chung, S. J., Fridman, G. Y., Baraban, J. M., and Reti, I. M. (2016). High-frequency stimulation at the subthalamic nucleus suppresses excessive self-grooming in autism-like mouse models. Neuropsychopharmacology 41, 1813–1821. doi: 10.1038/npp.2015.350
Chhabra, S., Nardi, L., Leukel, P., Sommer, C. J., and Schmeisser, M. J. (2023). Striatal increase of dopamine receptor 2 density in idiopathic and syndromic mouse models of autism spectrum disorder. Front. Psychiatry 14:1110525. doi: 10.3389/fpsyt.2023.1110525
Chiron, C., Bulteau, C., Loc’h, C., Raynaud, C., Garreau, B., Syrota, A., et al. (1993). Dopaminergic D2 receptor SPECT imaging in rett syndrome: Increase of specific binding in striatum. J Nucl Med. 34, 1717–1721. doi: 10.1111/j.1528-1157.1993.tb00450.x
Courtney, C. D., Pamukcu, A., and Chan, C. S. (2023). Cell and circuit complexity of the external globus pallidus. Na.t Neurosci. 26, 1147–1159. doi: 10.1038/s41593-023-01368-7
Cromwell, H. C., and Berridge, K. C. (1996). Implementation of action sequences by a neostriatal site: A lesion mapping study of grooming syntax. J. Neurosci. 16, 3444–3458. doi: 10.1523/JNEUROSCI.16-10-03444.1996
De Krom, M., Staal, W. G., Ophoff, R. A., Hendriks, J., Buitelaar, J., Franke, B., et al. (2009). A common variant in DRD3 receptor is associated with autism spectrum disorder. Biol. Psychiatry 65, 625–630. doi: 10.1016/j.biopsych.2008.09.035
Delgado, M. R., Locke, H. M., Stenger, V. A., and Fiez, J. A. (2003). Dorsal striatum responses to reward and punishment: Effects of valence and magnitude manipulations. Cogn. Affect. Behav. Neurosci. 3, 27–38. doi: 10.3758/CABN.3.1.27
Di, Y., Diao, Z., Zheng, Q., Li, J., Cheng, Q., Li, Z., et al. (2022). Differential alterations in striatal direct and indirect pathways mediate two autism-like behaviors in valproate-exposed mice. J. Neurosci. 42, 7833–7847. doi: 10.1523/JNEUROSCI.0623-22.2022
Dichter, G. S., Felder, J. N., Green, S. R., Rittenberg, A. M., Sasson, N. J., and Bodfish, J. W. (2012). Reward circuitry function in autism spectrum disorders. Soc. Cogn. Affect. Neurosci. 7, 160–172. doi: 10.1093/scan/nsq095
Ernst, M., Zametkin, A., Matochik, J., Pascualvaca, D., and Cohen, R. (1997). Low medial prefrontal dopaminergic activity in autistic children. Lancet 350:638. doi: 10.1016/S0140-6736(05)63326-0
Estes, A., Shaw, D. W., Sparks, B. F., Friedman, S., Giedd, J. N., Dawson, G., et al. (2011). Basal ganglia morphometry and repetitive behavior in young children with autism spectrum disorder. Autism Res. 4, 212–220. doi: 10.1002/aur.193
Fawcett, J. W., Oohashi, T., and Pizzorusso, T. (2019). The roles of perineuronal nets and the perinodal extracellular matrix in neuronal function. Nat. Rev. Neurosci. 20, 451–465. doi: 10.1038/s41583-019-0196-3
Ferhat, A. T., Verpy, E., Biton, A., Forget, B., De Chaumont, F., Mueller, F., et al. (2023). Excessive self-grooming, gene dysregulation and imbalance between the striosome and matrix compartments in the striatum of Shank3 mutant mice. Front. Mol. Neurosci. 16:1139118. doi: 10.3389/fnmol.2023.1139118
Francis, T., Chandra, R., Friend, D., Finkel, E., Dayrit, G., Miranda, J., et al. (2015). Nucleus accumbens medium spiny neuron subtypes mediate depression-related outcomes to social defeat stress. Biol. Psychiatry 77, 212–222. doi: 10.1016/j.biopsych.2014.07.021
Fraser, K. M., Chen, B. J., and Janak, P. H. (2023). Nucleus accumbens and dorsal medial striatal dopamine and neural activity are essential for action sequence performance. Neuroscience [Epub ahead of print] doi: 10.1101/2023.04.17.537212
Fuccillo, M. V. (2016). Striatal circuits as a common node for autism pathophysiology. Front. Neurosci. 10:27. doi: 10.3389/fnins.2016.00027
Gagnon, D., Petryszyn, S., Sanchez, M. G., Bories, C., Beaulieu, J. M., De Koninck, Y., et al. (2017). Striatal neurons expressing D1 and D2 receptors are morphologically distinct and differently affected by dopamine denervation in mice. Sci Rep. 7:41432. doi: 10.1038/srep41432
Gandhi, T., and Lee, C. C. (2021). Neural mechanisms underlying repetitive behaviors in rodent models of autism spectrum disorders. Front. Cell Neurosci. 14:592710. doi: 10.3389/fncel.2020.592710
Gangi, D. N., Messinger, D. S., Martin, E. R., and Cuccaro, M. L. (2016). Dopaminergic variants in siblings at high risk for autism: Associations with initiating joint attention. Autism Res. 9, 1142–1150. doi: 10.1002/aur.1623
Garcia, A. F., Crummy, E. A., Webb, I. G., Nooney, M. N., and Ferguson, S. M. (2021). Distinct populations of cortical pyramidal neurons mediate drug reward and aversion. Nat. Commun. 12:182. doi: 10.1038/s41467-020-20526-0
Gayden, J., Puig, S., Srinivasan, C., Buck, S. A., Gamble, C., Glausier, J. R., et al. (2023). Three-dimensional characterization of medium spiny neuron heterogeneity in the adult mouse striatum. bioRXiv [Preprint] doi: 10.1101/2023.05.04.539488
Groenewegen, H. J., Voorn, P., and Scheel-Krüger, J. (2016). “Limbic-basal ganglia circuits parallel and integrative aspects,” in The Basal Ganglia: Novel perspectives on motor and cognitive functions, ed. J. Soghomonian (Cham: Springer International Publishing), 11–45. doi: 10.1007/978-3-319-42743-0_2
Gunaydin, L. A., Grosenick, L., Finkelstein, J. C., Kauvar, I. V., Fenno, L. E., Adhikari, A., et al. (2014). Natural neural projection dynamics underlying social behavior. Cell 157, 1535–1551. doi: 10.1016/j.cell.2014.05.017
Haber, S. N. (2003). The primate basal ganglia: Parallel and integrative networks. J. Chem. Neuroanat. 26, 317–330. doi: 10.1016/j.jchemneu.2003.10.003
Haznedar, M. M., Buchsbaum, M. S., Wei, T. C., Hof, P. R., Cartwright, C., Bienstock, C. A., et al. (2000). Limbic circuitry in patients with autism spectrum disorders studied with positron emission tomography and magnetic resonance imaging. Am. J. Psychiatry 157, 1994–2001. doi: 10.1176/appi.ajp.157.12.1994
Hegeman, D. J., Hong, E. S., Hernández, V. M., and Chan, C. S. (2016). The external globus pallidus: Progress and perspectives. Eur. J. Neurosci. 43, 1239–1265. doi: 10.1111/ejn.13196
Hikida, T., Kimura, K., Wada, N., Funabiki, K., and Nakanishi, S. (2010). Distinct roles of synaptic transmission in direct and indirect striatal pathways to reward and aversive behavior. Neuron 66, 896–907. doi: 10.1016/j.neuron.2010.05.011
Hikida, T., Morita, M., and Macpherson, T. (2016). Neural mechanisms of the nucleus accumbens circuit in reward and aversive learning. Neurosci. Res. 108, 1–5. doi: 10.1016/j.neures.2016.01.004
Hikida, T., Yawata, S., Yamaguchi, T., Danjo, T., Sasaoka, T., Wang, Y., et al. (2013). Pathway-specific modulation of nucleus accumbens in reward and aversive behavior via selective transmitter receptors. Proc. Natl. Acad. Sci. U.S.A. 110, 342–347. doi: 10.1073/pnas.1220358110
Horder, J., Petrinovic, M. M., Mendez, M. A., Bruns, A., Takumi, T., Spooren, W., et al. (2018). Glutamate and GABA in autism spectrum disorder—a translational magnetic resonance spectroscopy study in man and rodent models. Transl. Psychiatry 8:106. doi: 10.1038/s41398-018-0155-1
Hormigo, S., Vega-Flores, G., and Castro-Alamancos, M. A. (2016). Basal Ganglia output controls active avoidance behavior. J. Neurosci. 36, 10274–10284. doi: 10.1523/JNEUROSCI.1842-16.2016
Karube, F., Takahashi, S., Kobayashi, K., and Fujiyama, F. (2019). Motor cortex can directly drive the globus pallidus neurons in a projection neuron type-dependent manner in the rat. eLife 8:e49511. doi: 10.7554/eLife.49511
Ketzef, M., and Silberberg, G. (2021). Differential synaptic input to external globus pallidus neuronal subpopulations in vivo. Neuron 109, 516–529.e4. doi: 10.1016/j.neuron.2020.11.006
Kim, H. F., and Hikosaka, O. (2013). Distinct Basal Ganglia circuits controlling behaviors guided by flexible and stable values. Neuron 79, 1001–1010. doi: 10.1016/j.neuron.2013.06.044
Kim, H., Lim, C. S., and Kaang, B. K. (2016). Neuronal mechanisms and circuits underlying repetitive behaviors in mouse models of autism spectrum disorder. Behav. Brain Funct. 12:3. doi: 10.1186/s12993-016-0087-y
Kohls, G., Schulte-Rüther, M., Nehrkorn, B., Müller, K., Fink, G. R., Kamp-Becker, I., et al. (2013). Reward system dysfunction in autism spectrum disorders. Soc. Cogn. Affect. Neurosci. 8, 565–572. doi: 10.1093/scan/nss033
Koketsu, D., Chiken, S., Hisatsune, T., Miyachi, S., and Nambu, A. (2021). Elimination of the cortico-subthalamic hyperdirect pathway induces motor hyperactivity in mice. J. Neurosci. 41, 5502–5510. doi: 10.1523/JNEUROSCI.1330-20.2021
Kolodny, T., Schallmo, M., Gerdts, J., Edden, R. A., Bernier, R. A., and Murray, S. O. (2020). Concentrations of cortical GABA and glutamate in young adults with autism spectrum disorder. Autism Res. 13, 1111–1129. doi: 10.1002/aur.2300
Kuo, H. Y., and Liu, F. C. (2019). Synaptic wiring of corticostriatal circuits in Basal Ganglia: Insights into the pathogenesis of neuropsychiatric disorders. eNeuro 6, ENEURO.76–ENEURO.19. doi: 10.1523/ENEURO.0076-19.2019
Kuo, H. Y., and Liu, F. C. (2020). Pathological alterations in striatal compartments in the human brain of autism spectrum disorder. Mol. Brain 13:83. doi: 10.1186/s13041-020-00624-2
Kupchik, Y. M., Brown, R. M., Heinsbroek, J. A., Lobo, M. K., Schwartz, D. J., and Kalivas, P. W. (2015). Coding the direct/indirect pathways by D1 and D2 receptors is not valid for accumbens projections. Nat. Neurosci. 18, 1230–1232. doi: 10.1038/nn.4068
Lanciego, J. L., Luquin, N., and Obeso, J. A. (2012). Functional neuroanatomy of the Basal Ganglia. Cold Spring Harb. Perspect. Med. 2:a009621. doi: 10.1101/cshperspect.a009621
Le Merrer, J., Detraux, B., Gandía, J., De Groote, A., Fonteneau, M., De Kerchove d’Exaerde, A., et al. (2024). Balance between projecting neuronal populations of the nucleus accumbens controls social behavior in mice. Biol. Psychiatry. 95, 123–135. doi: 10.1101/2022.11.28.518147
Lee, J., Wang, W., and Sabatini, B. L. (2020). Anatomically segregated basal ganglia pathways allow parallel behavioral modulation. Nat. Neurosci. 23, 1388–1398. doi: 10.1038/s41593-020-00712-5
Lee, Y., Kim, H., Kim, J. E., Park, J. Y., Choi, J., Lee, J. E., et al. (2018). Excessive D1 dopamine receptor activation in the dorsal striatum promotes autistic-like behaviors. Mol. Neurobiol. 55, 5658–5671. doi: 10.1007/s12035-017-0770-5
Leisman, G., Melillo, R., and Melillo, T. (2023). Prefrontal functional connectivities in autism spectrum disorders: A connectopathic disorder affecting movement, interoception, and cognition. Brain Res. Bull. 198, 65–76. doi: 10.1016/j.brainresbull.2023.04.004
Lewis, M. H., Lindenmaier, Z., Boswell, K., Edington, G., King, M. A., and Muehlmann, A. M. (2018). Subthalamic nucleus pathology contributes to repetitive behavior expression and is reversed by environmental enrichment. Genes Brain Behav. 17:e12468. doi: 10.1111/gbb.12468
Li, W., and Pozzo-Miller, L. (2020). Dysfunction of the corticostriatal pathway in autism spectrum disorders. J. Neurosci. Res. 98, 2130–2147. doi: 10.1002/jnr.24560
Liu, Z., Le, Q., Lv, Y., Chen, X., Cui, J., Zhou, Y., et al. (2022). A distinct D1-MSN subpopulation down-regulates dopamine to promote negative emotional state. Cell Res. 32, 139–156. doi: 10.1038/s41422-021-00588-5
Lovinger, D. M. (2017). An indirect route to repetitive actions. J. Clin. Investig. 127, 1618–1621. doi: 10.1172/JCI93918
Lu, J., Cheng, Y., Xie, X., Woodson, K., Bonifacio, J., Disney, E., et al. (2021). Whole-brain mapping of direct inputs to dopamine D1 and D2 receptor-expressing medium spiny neurons in the posterior dorsomedial striatum. eNeuro 8, ENEURO.348–ENEURO.320. doi: 10.1523/ENEURO.0348-20.2020
Mahabir, S., Chatterjee, D., Buske, C., and Gerlai, R. (2013). Maturation of shoaling in two zebrafish strains: A behavioral and neurochemical analysis. Behav. Brain Res. 247, 1–8. doi: 10.1016/j.bbr.2013.03.013
Maisterrena, A., Matas, E., Mirfendereski, H., Balbous, A., Marchand, S., and Jaber, M. (2022). The state of the dopaminergic and glutamatergic systems in the valproic acid mouse model of autism spectrum disorder. Biomolecules 12:1691. doi: 10.3390/biom12111691
Marche, K., Martel, A., and Apicella, P. (2017). Differences between dorsal and ventral striatum in the sensitivity of tonically active neurons to rewarding events. Front. Syst. Neurosci. 11:52. doi: 10.3389/fnsys.2017.00052
Mariggiò, M. A., Palumbi, R., Vinella, A., Laterza, R., Petruzzelli, M. G., Peschechera, A., et al. (2021). DRD1 and DRD2 receptor polymorphisms: Genetic neuromodulation of the dopaminergic system as a risk factor for ASD, ADHD and ASD/ADHD overlap. Front. Neurosci. 15:705890. doi: 10.3389/fnins.2021.705890
Masuda, F., Nakajima, S., Miyazaki, T., Yoshida, K., Tsugawa, S., Wada, M., et al. (2019). Motor cortex excitability and inhibitory imbalance in autism spectrum disorder assessed with transcranial magnetic stimulation: A systematic review. Transl. Psychiatry 9:110. doi: 10.1038/s41398-019-0444-3
Mei, Y., Monteiro, P., Zhou, Y., Kim, J. A., Gao, X., Fu, Z., et al. (2016). Adult restoration of Shank3 expression rescues selective autistic-like phenotypes. Nature 530, 481–484. doi: 10.1038/nature16971
Meirsman, A. C., Ben Hamida, S., Clarke, E., De Kerchove d’Exaerde, A., Darcq, E., and Kieffer, B. L. (2019). GPR88 in D1R-type and D2R-type medium spiny neurons differentially regulates affective and motor behavior. eNeuro 6, ENEURO.35–ENEURO.19. doi: 10.1523/ENEURO.0035-19.2019
Miyachi, S., Hasegawa, Y. T., and Gerfen, C. R. (2005). Coincident stimulation of convergent cortical inputs enhances immediate early gene induction in the striatum. Neuroscience 134, 1013–1022. doi: 10.1016/j.neuroscience.2005.02.051
Muehlmann, A. M., Maletz, S., King, M. A., and Lewis, M. H. (2020). Pharmacological targeting of striatal indirect pathway neurons improves subthalamic nucleus dysfunction and reduces repetitive behaviors in C58 mice. Behav. Brain Res. 391:112708. doi: 10.1016/j.bbr.2020.112708
Nakanishi, S., Hikida, T., and Yawata, S. (2014). Distinct dopaminergic control of the direct and indirect pathways in reward-based and avoidance learning behaviors. Neuroscience 282, 49–59. doi: 10.1016/j.neuroscience.2014.04.026
Nebel, M. B., Joel, S. E., Muschelli, J., Barber, A. D., Caffo, B. S., Pekar, J. J., et al. (2014). Disruption of functional organization within the primary motor cortex in children with autism. Hum. Brain Mapp. 35, 567–580. doi: 10.1002/hbm.22188
O’Dwyer, L., Tanner, C., Van Dongen, E. V., Greven, C. U., Bralten, J., Zwiers, M. P., et al. (2016). Decreased left caudate volume is associated with increased severity of autistic-like symptoms in a cohort of ADHD patients and their unaffected siblings. PLoS One 11:e0165620. doi: 10.1371/journal.pone.0165620
Pagani, M., Bertero, A., Liska, A., Galbusera, A., Sabbioni, M., Barsotti, N., et al. (2019). Deletion of autism risk gene shank3 disrupts prefrontal connectivity. J. Neurosci. 39, 5299–5310. doi: 10.1523/JNEUROSCI.2529-18.2019
Park, G., Jeon, S. J., Ko, I. O., Park, J. H., Lee, K. C., Kim, M. S., et al. (2022). Decreased in vivo glutamate/GABA ratio correlates with the social behavior deficit in a mouse model of autism spectrum disorder. Mol. Brain 15:19. doi: 10.1186/s13041-022-00904-z
Parthasarathy, H. B., and Graybiel, A. M. (1997). Cortically driven immediate-early gene expression reflects modular influence of sensorimotor cortex on identified striatal neurons in the squirrel monkey. J. Neurosci. 17, 2477–2491. doi: 10.1523/JNEUROSCI.17-07-02477.1997
Pavãl, D. A. (2017). Dopamine hypothesis of autism spectrum disorder. Dev. Neurosci. 39, 355–360. doi: 10.1159/000478725
Peak, J., Chieng, B., Hart, G., and Balleine, B. (2020). Striatal direct and indirect pathway neurons differentially control the encoding and updating of goal-directed learning. eLife 9:e58544. doi: 10.7554/eLife.58544
Peça, J., Feliciano, C., Ting, J., Wang, W., Wells, M., Venkatraman, T., et al. (2011). Shank3 mutant mice display autistic-like behaviours and striatal dysfunction. Nature 472, 437–442. doi: 10.1038/nature09965
Planert, H., Berger, T. K., and Silberberg, G. (2013). Membrane properties of striatal direct and indirect pathway neurons in mouse and rat slices and their modulation by dopamine. PLoS One 8:e57054. doi: 10.1371/journal.pone.0057054
Quintana, A., Sanz, E., Wang, W., Storey, G. P., Güler, A. D., Wanat, M. J., et al. (2012). Lack of GPR88 enhances medium spiny neuron activity and alters motor- and cue-dependent behaviors. Nat. Neurosci. 15, 1547–1555. doi: 10.1038/nn.3239
Reymann, J., Naudet, F., Pihan, M., Saïkali, S., Laviolle, B., Bentué-Ferrer, D., et al. (2013). Subthalamic nucleus modulates social and anxogenic-like behaviors. Behav. Brain Res. 252C, 356–362. doi: 10.1016/j.bbr.2013.05.059
Rothwell, P. E., Fuccillo, M. V., Maxeiner, S., Hayton, S. J., Gokce, O., Lim, B. K., et al. (2014). Autism-associated neuroligin-3 mutations commonly impair striatal circuits to boost repetitive behaviors. Cell 158, 198–212. doi: 10.1016/j.cell.2014.04.045
Sato, W., Kubota, Y., Kochiyama, T., Uono, S., Yoshimura, S., Sawada, R., et al. (2014). Increased putamen volume in adults with autism spectrum disorder. Front. Hum. Neurosci. 8:957. doi: 10.3389/fnhum.2014.00957
Saunders, A., Huang, K. W., and Sabatini, B. L. (2016). Globus pallidus externus neurons expressing parvalbumin interconnect the subthalamic nucleus and striatal interneurons. PLoS One 11:e0149798. doi: 10.1371/journal.pone.0149798
Scerbina, T., Chatterjee, D., and Gerlai, R. (2012). Dopamine receptor antagonism disrupts social preference in zebrafish: A strain comparison study. Amino Acids 43, 2059–2072. doi: 10.1007/s00726-012-1284-0
Schalbroeck, R., Van Velden, F. H., De Geus-Oei, L. F., Yaqub, M., Van Amelsvoort, T., Booij, J., et al. (2021). Striatal dopamine synthesis capacity in autism spectrum disorder and its relation with social defeat: An [18F]-FDOPA PET/CT study. Transl. Psychiatry 11:47. doi: 10.1038/s41398-020-01174-w
Schuetze, M., Park, M. T., Cho, I. Y., MacMaster, F. P., Chakravarty, M. M., and Bray, S. L. (2016). Morphological alterations in the thalamus, striatum, and pallidum in autism spectrum disorder. Neuropsychopharmacology 41, 2627–2637. doi: 10.1038/npp.2016.64
Scott-Van Zeeland, A. A., Dapretto, M., Ghahremani, D. G., Poldrack, R. A., and Bookheimer, S. Y. (2010). Reward processing in autism. Autism Res. 3, 53–67. doi: 10.1002/aur.122
Shepherd, G. M. (2013). Corticostriatal connectivity and its role in disease. Nat. Rev. Neurosci. 14, 278–291. doi: 10.1038/nrn3469
Shonesy, B. C., Parrish, W. P., Haddad, H. K., Stephenson, J. R., Báldi, R., Bluett, R. J., et al. (2018). Role of striatal direct pathway 2-arachidonoylglycerol signaling in sociability and repetitive behavior. Biol. Psychiatry 84, 304–315. doi: 10.1016/j.biopsych.2017.11.036
Soghomonian, J. J. (2016). “Anatomy and function of the direct and indirect striatal pathways,” in The Basal Ganglia. innovations in cognitive neuroscience, ed. J. J. Soghomonian (Cham: Springer). doi: 10.1007/978-3-319-42743-0_3
Sorg, B. A., Berretta, S., Blacktop, J. M., Fawcett, J. W., Kitagawa, H., Kwok, J. C., et al. (2016). Casting a wide net: Role of perineuronal nets in neural plasticity. J. Neurosci. 36, 11459–11468. doi: 10.1523/JNEUROSCI.2351-16.2016
Staal, W. G., De Krom, M., and De Jonge, M. V. (2012). Brief report: The dopamine-3-receptor gene (DRD3) is Associated with specific repetitive behavior in Autism Spectrum Disorder (ASD). J. Autism Dev. Disord. 42, 885–888. doi: 10.1007/s10803-011-1312-z
Subramanian, K., Brandenburg, C., Orsati, F., Soghomonian, J.-J., Hussman, J. P., and Blatt, G. J. (2017). Basal ganglia and autism – a translational perspective. Autism Res. 10, 1751–1775. doi: 10.1002/aur.1837
Surmeier, D. J., Shen, W., Day, M., Gertler, T., Chan, S., Tian, X., et al. (2010). “The role of dopamine in modulating the structure and function of striatal circuits,” in Progress in Brain Research [Internet], eds S. Waxman, D. G. Stein, D. Swaab, and H. Fields (Amsterdam: Elsevier), 148–167. doi: 10.1016/S0079-6123(10)83008-0
Sussman, D., Leung, R. C., Vogan, V. M., Lee, W., Trelle, S., Lin, S., et al. (2015). The autism puzzle: Diffuse but not pervasive neuroanatomical abnormalities in children with ASD. Neuroimage Clin. 8, 170–179. doi: 10.1016/j.nicl.2015.04.008
Tanimura, Y., King, M. A., Williams, D. K., and Lewis, M. H. (2011). Development of repetitive behavior in a mouse model: Roles of indirect and striosomal basal ganglia pathways. Int. J. Dev. Neurosci. 29, 461–467. doi: 10.1016/j.ijdevneu.2011.02.004
Tanimura, Y., Vaziri, S., and Lewis, M. H. (2010). Indirect basal ganglia pathway mediation of repetitive behavior: Attenuation by adenosine receptor agonists. Behav. Brain Res. 210, 116–122. doi: 10.1016/j.bbr.2010.02.030
Tian, J., Gao, X., and Yang, L. (2022). Repetitive restricted behaviors in autism spectrum disorder: From mechanism to development of therapeutics. Front. Neurosci. 16:780407. doi: 10.3389/fnins.2022.780407
Tzanoulinou, S., Musardo, S., Contestabile, A., Bariselli, S., Casarotto, G., Magrinelli, E., et al. (2022). Inhibition of Trpv4 rescues circuit and social deficits unmasked by acute inflammatory response in a Shank3 mouse model of Autism. Mol. Psychiatry 27, 2080–2094. doi: 10.1038/s41380-021-01427-0
Uchigashima, M., Ohtsuka, T., Kobayashi, K., and Watanabe, M. (2016). Dopamine synapse is a neuroligin-2–mediated contact between dopaminergic presynaptic and GABAergic postsynaptic structures. Proc. Natl. Acad. Sci. U.S.A. 113, 4206–4211. doi: 10.1073/pnas.1514074113
Varin, C., Cornil, A., Houtteman, D., Bonnavion, P., and De Kerchove d’Exaerde, A (2023). The respective activation and silencing of striatal direct and indirect pathway neurons support behavior encoding. Nat. Commun. 14:4982. doi: 10.1038/s41467-023-40677-0
Wall, N. R., De La Parra, M., Callaway, E. M., and Kreitzer, A. C. (2013). Differential innervation of direct- and indirect-pathway striatal projection neurons. Neuron 79, 347–360. doi: 10.1016/j.neuron.2013.05.014
Wang, W., Li, C., Chen, Q., Van Der Goes, M. S., Hawrot, J., Yao, A. Y., et al. (2017). Striatopallidal dysfunction underlies repetitive behavior in Shank3-deficient model of autism. J. Clin. Investig. 127, 1978–1990. doi: 10.1172/JCI87997
Wegiel, J., Flory, M., Kuchna, I., Nowicki, K., Ma, S. Y., Imaki, H., et al. (2014). Brain-region–specific alterations of the trajectories of neuronal volume growth throughout the lifespan in autism. Acta Neuropathol. Commun. 2:28. doi: 10.1186/2051-5960-2-28
Whi, W., Ha, S., Kang, H., Lee, H., Kim, B., and Lee, D. S. (2020). Alteration of cortico-limbic-striatal connectivity in ASD patients: Topological brain network analysis. J. Nucl. Med. 61, (Suppl. 1), 1542.
Wilkes, B. J., and Lewis, M. H. (2018). The neural circuitry of restricted repetitive behavior: Magnetic resonance imaging in neurodevelopmental disorders and animal models. Neurosci. Biobehav. Rev. 92, 152–171. doi: 10.1016/j.neubiorev.2018.05.022
Yawata, S., Yamaguchi, T., Danjo, T., Hikida, T., and Nakanishi, S. (2012). Pathway-specific control of reward learning and its flexibility via selective dopamine receptors in the nucleus accumbens. Proc. Natl. Acad. Sci. U.S.A. 109, 12764–12769. doi: 10.1073/pnas.1210797109
Zhang, K., Hill, K., Labak, S., Blatt, G. J., and Soghomonian, J. J. (2014). Loss of glutamic acid decarboxylase (Gad67) in Gpr88-expressing neurons induces learning and social behavior deficits in mice. Neuroscience 275, 238–247. doi: 10.1016/j.neuroscience.2014.06.020
Zürcher, N. R., Walsh, E. C., Phillips, R. D., Cernasov, P. M., Tseng, C. E., Dharanikota, A., et al. (2021). A simultaneous [11C] raclopride positron emission tomography and functional magnetic resonance imaging investigation of striatal dopamine binding in autism. Transl. Psychiatry 11:33. doi: 10.1038/s41398-020-01170-0
Keywords: striatum, indirect pathway, direct pathway, social behavior, repetitive behavior, autism spectrum disorders
Citation: Soghomonian J-J (2024) The cortico-striatal circuitry in autism-spectrum disorders: a balancing act. Front. Cell. Neurosci. 17:1329095. doi: 10.3389/fncel.2023.1329095
Received: 27 October 2023; Accepted: 18 December 2023;
Published: 11 January 2024.
Edited by:
Ilaria Morella, Cardiff University, United KingdomReviewed by:
A. J. Baucum, Indiana University Bloomington, United StatesCopyright © 2024 Soghomonian. This is an open-access article distributed under the terms of the Creative Commons Attribution License (CC BY). The use, distribution or reproduction in other forums is permitted, provided the original author(s) and the copyright owner(s) are credited and that the original publication in this journal is cited, in accordance with accepted academic practice. No use, distribution or reproduction is permitted which does not comply with these terms.
*Correspondence: Jean-Jacques Soghomonian, ampzb2dob0BidS5lZHU=
Disclaimer: All claims expressed in this article are solely those of the authors and do not necessarily represent those of their affiliated organizations, or those of the publisher, the editors and the reviewers. Any product that may be evaluated in this article or claim that may be made by its manufacturer is not guaranteed or endorsed by the publisher.
Research integrity at Frontiers
Learn more about the work of our research integrity team to safeguard the quality of each article we publish.