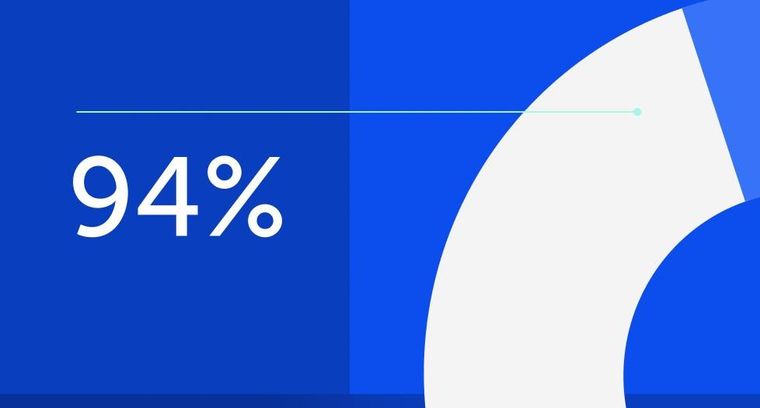
94% of researchers rate our articles as excellent or good
Learn more about the work of our research integrity team to safeguard the quality of each article we publish.
Find out more
REVIEW article
Front. Cell. Neurosci., 08 January 2024
Sec. Non-Neuronal Cells
Volume 17 - 2023 | https://doi.org/10.3389/fncel.2023.1322541
This article is part of the Research TopicRole of Glia in Neurodevelopmental DisordersView all 5 articles
Astrocytes have an important role in neuronal maturation and synapse function in the brain. The interplay between astrocytes and neurons is found to be altered in many neurodevelopmental disorders, including fragile X syndrome (FXS) that is the most common inherited cause of intellectual disability and autism spectrum disorder. Transcriptional, functional, and metabolic alterations in Fmr1 knockout mouse astrocytes, human FXS stem cell-derived astrocytes as well as in in vivo models suggest autonomous effects of astrocytes in the neurobiology of FXS. Abnormalities associated with FXS astrocytes include differentiation of central nervous system cell populations, maturation and regulation of synapses, and synaptic glutamate balance. Recently, FXS-specific changes were found more widely in astrocyte functioning, such as regulation of inflammatory pathways and maintenance of lipid homeostasis. Changes of FXS astrocytes impact the brain homeostasis and function both during development and in the adult brain and offer opportunities for novel types of approaches for intervention.
Glial cells comprise major cell populations in the human brain, and astrocytes account for a substantial portion of all glia (von Bartheld et al., 2016). Astroglial cells form functionally, structurally, and developmentally diverse cell populations (Clavreul et al., 2022; Yang et al., 2022). A crucial function of astrocytes is to provide metabolic support to neurons (Turner and Adamson, 2011). They display dynamic activity in the form of intracellular calcium levels, which control the release of neuroactive gliotransmitters (Goenaga et al., 2023). Astrocytes induce the development and destruction of both excitatory and inhibitory synapses and contribute to short- and long-term brain plasticity through distinct mechanisms (Perez-Catalan et al., 2021). Due to their complex arborisation, astrocytes form non-overlapping synaptic islands consisting of an astrocyte and all the synapses it contacts (Halassa et al., 2007). A single astrocyte can influence up to 2 million synapses in the human brain, which facilitates circuit level oversight. Human astrocytes are larger and structurally more complex, and synaptic islands in the human cortex are much larger than those of model animals (Oberheim et al., 2009). Gap junctions allow astrocytes to form networks, whose function is vital for cognition (Hösli et al., 2022). As astrocytes form the blood brain barrier (BBB), they regulate brain water balance through aquaporins (Satoh et al., 2007). By these means astrocytes are in an advanced position to interpret peripheral signals (Lee et al., 2022).
Astrocytes are implicated in various brain pathologies (Lee et al., 2022), including neurodevelopmental conditions such as fragile X syndrome (FXS, Fernández-Blanco and Dierssen, 2020). FXS, first described as an X chromosome-linked syndrome (Martin and Bell, 1943) is considered the most common cause of inherited intellectual disability. Prevalence of FXS is generally quoted as 1 in 4,000 males and 1 in every 6,000–8,000 females. Based on genetic screening studies, the prevalence was estimated to be approximately 1 in every 2,500 regardless of gender (Hagerman, 2008). The FXS symptomatology significantly overlaps with other neuropsychiatric conditions. Approximately a third of FXS patients fulfill diagnostic criteria for autism spectrum disorder (ASD, Harris et al., 2008), and 54–59% meet diagnostic criteria for attention deficit hyperactivity disorder (ADHD, Sullivan et al., 2006). Up to 44% have epilepsy (Cowley et al., 2016), which ranges from generalized seizures to benign focal epilepsy in childhood with centrotemporal spikes (Lozano et al., 2016). In addition, the FXS phenotype includes near-universal stereotypical physical features such as long and narrow face, prominent ears, high-arched palate, prominent jaw, and macroorchidism (Ciaccio et al., 2017).
A CGG trinucleotide repeat expansion in the 5′ untranslated region of the fragile X messenger ribonucleoprotein 1 (FMR1) gene is the most common genetic cause behind FXS (Fu et al., 1991; Verkerk et al., 1991). Varying lengths of the CGG triplet repeat exist. Repeats less than 55 are in the benign range, whereas over 200 repeats lead to embryonic methylation of the promoter, subsequent silencing of the gene, and therefore lack of the FMR1 protein (FMRP, Sutcliffe et al., 1992). Thus, there exist premutations between 55 and 200 repeats, whose carriers do not exhibit the FXS phenotype. The permutated CGG repeat sequence is prone to expand for offspring (Nolin et al., 1996), and premutation carriers themselves are susceptible to later in life develop conditions considered to be separate from FXS such as fragile X associated tremor and ataxia syndrome (FXTAS, Hagerman et al., 2003) and premature ovarian insufficiency (POI, Schwartz et al., 1994). FMRP is an RNA binding protein (Siomi et al., 1993) that is mainly expressed in the central nervous system (CNS) and gonads, and to lesser extent in other tissues (Hinds et al., 1993). In the CNS, it is expressed in neurons and during development in glial cells. Its expression in astrocytes shows highest levels at the time of vital growth, stabilization, and maturation of synapses, declining thereafter (Pacey and Doering, 2007). FMRP acts as an overall suppressor of protein synthesis (Laggerbauer et al., 2001), a function which it achieves by regulating nuclear-cytoplasmic translocation (Feng et al., 1997; Kim et al., 2009) and translation of various mRNAs. Many of these RNAs are associated with synapse function (Darnell et al., 2011). Hallmarks of neural circuit function in FXS include hyperexcitability and plasticity defects (Martin and Huntsman, 2012). The mouse model of FXS, the Fmr1 knockout (KO) mice, lacks FMRP and recapitulates the main phenotype, including deficiency in learning and memory, social behavior, and sensory processing (Bassell and Warren, 2008; Liu and Smith, 2009).
Despite several promising therapeutic targets and success with animal models, current therapeutic strategies of FXS remain symptomatic and no specific treatment has reached clinical use. Therapeutic approaches have been based on disturbed mechanisms observed to affect neuron function and the role of non-neuronal cells in these processes has been ignored. The theory around the metabotropic glutamate receptor 5 (mGluR5) proposed that inhibition of the glutamate receptor would rescue downstream ERK1/2 dependent excessive protein synthesis in FXS (Bear et al., 2004); however, treatment resistance likely occurs downstream of targeted factors (Stoppel et al., 2021). The hypercholesterolemia drug 3-hydroxy-3-methylglutaryl coenzyme A (HMG-CoA) reductase inhibitor lovastatin, which inhibits the mevalonate pathway and Ras activation upstream of ERK1/2, was beneficial in preclinical FXS models (Osterweil et al., 2013; Asiminas et al., 2019; Muscas et al., 2019), but did not succeed well in clinical trials (Çaku et al., 2014; Thurman et al., 2020; Champigny et al., 2022). Metformin, targeting ERK1/2 downstream of IGF-1, likewise appeared beneficial in an open-label study and increased GABAergic inhibition (Dy et al., 2018; Proteau-Lemieux et al., 2021). GABA mimetics were studied to influence changes in excitatory/inhibitory balance at circuit level in FXS, and several molecules appeared promising, but the matter of treatment window remains to be resolved (Milla et al., 2023). Selective serotonin uptake inhibitors (SSRIs) and memantine have been found to display beneficial effects (Winarni et al., 2012) and ZYN002 cannabidiol reduced symptoms in a randomized clinical trial (Berry-Kravis et al., 2022). Many therapeutic molecules have been examined on the rescue effects in FXS on dendritic spine abnormalities, which include an increased number of immature spines during early development as a consistent observation (He and Portera-Cailliau, 2013). However, it is unclear to what extent changes in dendritic spine morphology influences functional outcomes in FXS (Booker et al., 2019). Age-, region-, and cell type-specific alterations of structural dynamics of cortical spines are found to be associated with abnormal synaptic plasticity and behavioral deficit in the Fmr1 KO mice (Cruz-Martín et al., 2010; Pan et al., 2010; Padmashri et al., 2013; Hodges et al., 2017; Gredell et al., 2023). Minocycline was studied on the premise of inhibiting the overactive matrix metalloproteinase 9 (MMP-9) and correcting spine abnormalities in preclinical models with, however, little treatment effect in patients (Utari et al., 2010; Champigny et al., 2022). In general, development of successful treatment strategies in FXS is complicated by the disparity between critical periods of brain plasticity and the age of diagnosis, which is around 4 years for males and 9 years for females (Gabis et al., 2017). Many of the aforementioned treatment approaches for FXS are likely complicated by glial function, and future research on astrocytes has potential to benefit the development of therapies. Here we review astrocytic perspective in FXS.
Loss of FMRP-mediated translational control results in altered cell fate specification during early neural development (Tervonen et al., 2009; Saffary and Xie, 2011; Utami et al., 2020). Delayed cell cycle progression and extended maintenance of immature proliferating neural progenitor cells (NPCs) are found in both human and mouse FXS models (Edens et al., 2019; Raj et al., 2021). Studying human FMR1 KO differentiating astrocyte cell cycle dynamics, Ren et al. (2023) observed 2-fold increased cyclin D1 associating with reduced S phase duration in an otherwise unchanged cell cycle duration. The proteomics analysis revealed immature signals in differentiating human iPSC-derived FMR1 KO astrocytes when compared with controls (Ren et al., 2023). In a separate human FMR1 KO iPSC cortical model, KO NPCs produced larger organoids and displayed increased glial fibrillary acidic protein (GFAP) expression when compared with isogenic controls (Brighi et al., 2021). The protein kinase inhibitor LX7101 likely through AKT/the mammalian target of rapamycin (mTOR) inhibition was shown to suppress GFAP overexpression (Sunamura et al., 2018). Phospho-SMAD positive astrocytes are increased in Fmr1 KO cortex at P7, indicating increased bone morphogenetic protein (BMP) activity. Both Bmp6 RNA and protein are upregulated in rat Fmr1 KO astrocytes compared with wild type (WT) controls (Caldwell et al., 2022), which can promote astrocyte maturation and inhibit astrocyte proliferation (Scholze et al., 2014). Absence of FMRP appears to influence the dynamics of astrocyte maturation, and some of the mechanisms may be shared with other neurodevelopmental disorders (Caldwell et al., 2022).
In addition to its role in modulating haemostasis and thrombosis, the plasminogen system is implicated in translocation of cellular processes in the developing brain likely through the regulation of the proteolysis of the extracellular matrix (Goeke et al., 2022). Astrocytes regulate plasminogen activation and plasmin clearance in the brain (Briens et al., 2017). Cellular uptake and release processes control plasminogen activators (Cassé et al., 2012) and plasmin substrates (Bergami et al., 2008) in local neuronal environment. Expression of tissue plasminogen activator (tPA) is increased in differentiating Fmr1 KO neural progenitors, indicating increased tPA activity (Achuta et al., 2014). Colocalising with GFAP, tPA is increased in cortical supragranular layers I-III but decreased in layers IV-V at P7 in the Fmr1 KO brain (Achuta et al., 2014). Expression of the other main plasminogen activator urokinase-type plasminogen activator (uPA) and its receptor uPAR peak postnatally while being undetectable in the mature brain (Kalderon et al., 1990). uPA is increased in FXS hiPSC-derived forebrain astrocytes (Peteri et al., 2021). These immature human FXS astrocytes also secrete uPA more than healthy control astrocytes, and the increased uPA augments tyrosine receptor kinase B (TrkB) phosphorylation within the docking site for phospholipase-Cγ1 (PLCγ1) in cocultured rat primary neurons (Peteri et al., 2021). Increased production of diacylglycerol (DAG) by PLCγ1 might contribute to increased DAG levels observed in Fmr1 KO mouse neurons (Tabet et al., 2016) together with reduced diacylglycerol kinase kappa (DGKκ) activity, whose recovery restores behavior in Fmr1 KO mouse (Habbas et al., 2022). Since uPAR lacks transmembrane/cytoplasmic domains, it needs a partner protein to elicit intracellular signaling. Orphan receptor GPR124 (Chen et al., 2019) is differently expressed in FXS and control astrocytes and may represent a partner receptor that acts through WNT/β-catenin signaling, which is altered in FXS models (Peteri et al., 2021). Astrocytes mature in parallel with other CNS cell types (Cheng Y. T. et al., 2023) and studies of human FXS astrocytes have demonstrated an important impact of the plasminogen system on the differentiation and cell-to-cell interactions of CNS cell types.
FMRP expression in astrocytes appears essential for normal synapse formation and function (Cheng et al., 2012; Bagni and Zukin, 2019). Co-culturing studies of Fmr1 KO astrocytes with hippocampal neurons have revealed that FMRP-deficient astrocytes contribute to more complex dendritic arborisation when compared to co-culture with WT astrocytes (Jacobs and Doering, 2010). Dendritic spine immaturity (relatively increased length) and synaptic protein abnormalities of Fmr1 KO neurons are prevented by WT astrocyte conditioned media or a WT feeder layer (Cheng et al., 2016). Many astrocyte-secreted factors involved in the regulation of synapse formation and maturation are found to be dysregulated in Fmr1 KO mice. The studies of Fmr1 KO astrocytes suggest that FMRP deficiency particularly contributes to an abnormal temporal increase in excitatory synapses by modulating secretion of factors that regulate synapse formation and maturation. Tenascin C (TNC), an activator of TLR4 that can promote excitatory synapse formation and immature spine morphology, is overexpressed in Fmr1 KO astrocytes (Krasovska and Doering, 2018). Also, cortical expression of hevin that is necessary for the formation of thalamocortical excitatory synapses (Kucukdereli et al., 2011; Risher et al., 2014) is increased in P14 Fmr1 KO mice compared with WT controls consistent with an increase in the density of thalamocortical synapses when WT neurons are cultured with Fmr1 KO astrocytes (Wallingford et al., 2017). An increased number of thalamocortical synapses occur in layer IV of the somatosensory cortex of 4-month-old Fmr1 KO mice and altered thalamocortical connectivity is also implicated in ASD (Mizuno et al., 2006; Cheon et al., 2011; Nair et al., 2013). Conversely, expression of SPARC that inhibits the synaptogenic function of hevin and negatively regulates the formation of excitatory synapses (Risher et al., 2014) is reduced in the cortex and hippocampus of the Fmr1 KO mouse (Wallingford et al., 2017). Secretion of thrombospondin-1 (TSP-1) that as an extracellular matrix protein may induce cell adherence and induce synaptogenesis is also abnormally reduced from Fmr1 KO astrocytes (Cheng et al., 2016), whereas neurotrophin-3 (NT-3) levels are increased in the secretome of Fmr1 KO astrocytes as well as in the Fmr1 KO prefrontal cortex when compared with controls (Yang et al., 2012). Thus, differential spatial and temporal expression pattern of factors secreted from FMRP-deficient astrocytes may reflect astrocyte-dependent brain region-specific developmental processes. Dysregulation of secreted factors from FMRP-deficient astrocytes during critical periods for synapse formation, stabilization, and pruning are consistent with delayed critical period for thalamocortical plasticity in the barrel cortex (somatosensory layer IV) of Fmr1 KO mice and with increased silent synapses at earlier time points (Harlow et al., 2010).
Subcellular localization/expression of mRNAs and localization of ribosomes at perisynaptic astroglial processes (PAPs) are critical for localized astroglial signaling to synapses and regulation of neuronal activity (Bazargani and Attwell, 2016). There is evidence that astroglial processes have more contacts with synapses in the cortex of the Fmr1 KO mice than in WT controls (Men et al., 2022). FMRP deficiency affects localization of mRNAs in astrocytes and particularly alters levels of mRNAs localized at astrocytic processes. The mRNAs located at processes are enriched by mRNAs that encode surface proteins such as transporters, receptors, and channels involved in synaptic signaling (Men et al., 2022). Effects of the absence of FMRP on the total mRNA or on the local mRNA amount may serve as a critical factor for activity-dependent regulation of synapses.
Increased glutamatergic signaling and altered excitatory/inhibitory balance is recognized as a hallmark of FXS (Liu et al., 2022). The role of FXS astrocytes in abnormal neuronal firing was recently confirmed in co-cultures of cortical neurons and astrocytes derived from human stem cells generated from patients with FXS and a control donor (Das Sharma et al., 2023). FXS neurons showed a high frequency of short, spontaneous bursts and reduced persistent Na+ current in the presence of FXS astrocytes or media secreted by FXS astrocytes. Astrocyte-specific Ca2+ binding protein S100B was found to prevent abnormalities of Na+ current and firing pattern in FXS and control neurons co-cultured with FXS astrocytes or FXS astrocyte-derived conditioned medium. Furthermore, blocking antibody to S100B induced disordered firing in co-cultures of control neurons and control astrocytes (Das Sharma et al., 2023), demonstrating that astrocyte secreted factors can influence the firing patterns of glutamatergic neurons (Das Sharma et al., 2020).
An important role of astrocytes is to maintain glutamate homeostasis that is essential for brain physiology. Astrocyte-specific Fmr1 KO mouse displays reduced expression of the astrocytic glutamate transporter GLT-1, which leads to reduced astrocytic uptake of extracellular glutamate and increased cortical neuronal excitability (Higashimori et al., 2016). Astroglial glutamate transporters modulate activation of neuronal mGluR1/5 providing a mechanism, which may underlie enhanced neuronal mGluR5 activation in FXS. mGluR5 regulates GLT-1 expression in astrocytes and reduced mGluR5 expression has been found both in human and mouse FMRP-deficient astrocytes (Higashimori et al., 2013; Men et al., 2020). Selective astroglial overexpression of miRNA-128 results in reduced mGluR5 protein in FMRP-deficient astrocytes (Men et al., 2020). Regulation of astroglial transporter expression is strongly associated with astrocytic maturation and influenced by culturing conditions (Schlag et al., 1998; Peteri et al., 2021), which may explain that Ren and colleagues (Ren et al., 2023) did not observe reduced glutamate uptake in human FMR1 KO astrocytes. Species specific differences and differences in experimental approaches such as glutamate incubation time may explain different results in studies on astrocytic glutamate uptake (Higashimori et al., 2016; Ren et al., 2023). Astrocytic expression of mGluR5 is developmentally regulated and possibly several compensatory mechanisms also exist as mGluR5 inhibition can lead to increased GLT-1 expression under pathological conditions (Cheng S. et al., 2023). To what extent compensatory mechanisms in glutamate uptake by FXS astrocytes are dependent on cell-to-cell interactions and neuronal activity-dependent mechanisms remain to be studied.
In addition to the glutamatergic system, GABAergic circuits and GABA homeostasis are dysregulated in the absence of FMRP. Reduction of several GABA receptor subunits in various brain regions associates with reduced expression of GABA metabolizing enzymes and reduced numbers of parvalbumin (PV) positive neurons in the Fmr1 KO mouse (Gao et al., 2018; Van der Aa and Kooy, 2020). Contribution of FMRP-deficient astrocytes to the aberrant GABA phenotype was indicated by an astrocyte-specific postnatal Fmr1 KO model (Rais et al., 2022, preprint available). Loss of astrocytic FMRP recapitulated the reduced number of cortical PV cells and reduced synaptic GABAA receptor subunits (Rais et al., 2022, preprint available). These changes associated with increased GABA levels and increased glutamate decarboxylase in astrocytes, indicating increased GABA synthesis (Rais et al., 2022, preprint available). Astrocytes can sense extracellular GABA as well as release GABA through ion channels and transporters, controlling its extracellular levels (Kilb and Kirischuk, 2022), suggesting that increased astrocytic GABA in Fmr1 KO mice may be physiologically compensatory to maintain homeostasis.
Astrocytes exhibit intracellular Ca2+ currents which can modulate synapse function by altering gliotransmitter release (Goenaga et al., 2023). There is ample evidence that these Ca2+ signals are altered in the context of FMRP-deficient astrocytes. Ca2+ signals in response to extracellular adenosine triphosphate (ATP) in human FMR1 KO astrocytes have increased amplitude but neither the proportion of cells responding nor the duration of the response change (Ren et al., 2023). Augmented intracellular Ca2+ responses to membrane depolarization with high extracellular K+ are dependent on tPA in Fmr1 KO mouse NPCs (Achuta et al., 2014). In human FXS iPSC-derived astrocytes, L-type calcium channel-dependent Ca2+ responses to high extracellular K+ were reduced when compared with controls and these responses showed strong inverse correlation with ACM uPA levels (Peteri et al., 2021), demonstrating that altered Ca2+ signaling in FXS astrocytes modulates neuronal plasticity.
Astrocytes with abnormally high GFAP, have been observed in striatal, hippocampal, cortical, and cerebellar areas of adult Fmr1 KO mice (Yuskaitis et al., 2010; Pacey et al., 2015). Increased GFAP expression is also found in a human FMR1 KO iPSC cortical organoid model (Brighi et al., 2021). In human FXS cortical layer I GFAP and S100B positive cells are increased (Ren et al., 2023). Whether increased GFAP expression in FXS astrocytes reflects altered astrocyte fate determination, astrocyte immaturity or response of astrocytes to extracellular stressors remains to be elucidated.
Astrocytes with abnormally high GFAP reflecting astrocyte reactivity occurs in response to various genetic, environmental, and pathological stimuli (Escartin et al., 2021). Pacey et al. (2015) showed that astrocytes in the cerebellum of Fmr1 KO mice display chronic, persistent activation with little or no activation of microglia. Expression of glial cell markers tumor necrosis factor receptor 2 (TNFR2), leukemia inhibitory factor (LIF), and GFAP were found to be elevated in the Fmr1 KO mouse cerebellum at 2 weeks postnatally. GFAP, TNFR2, and S100B were increased in the adult Fmr1 KO brain (Pacey et al., 2015). The proinflammatory cytokine interleukin 1β (IL-1β) is an activator of human astrocytes leading to production of inflammatory mediators such as cytokines, chemokines, nitric oxide (NO), and reactive oxygen species (ROS) (Lee et al., 1993; Sharma et al., 2007) and to down-regulation of glutamate uptake (Hu et al., 2000). IL-1β treatment increases GFAP and chemokine CCL5 mRNA expression more in human FXS iPSC-derived astrocytes than in control astrocytes (Talvio et al., 2023), suggesting that FXS astrocytes are characterized by increased sensitivity to reactivity. This may be reflected in Fmr1 KO mice as impaired brain energy metabolism and increased ROS markers (el Bekay et al., 2007; D’Antoni et al., 2020). Mitochondrial respiration capacity and emission of reactive oxygen species are increased in Fmr1 KO mouse astrocytes under physiological hypoxia but not in atmospheric normoxia (Vandenberg et al., 2022). In the transcriptomics analysis of human FXS NPCs, MYD88 was amongst the most significantly overexpressed genes (Talvio et al., 2022) and there are several tentative arguments for MYD88-mediated system overactivity in FXS astrocytes. Expression of MMP-9, the proposed target of minocycline in FXS, is upregulated via a MYD88-dependent mechanism in astrocytes (Gorina et al., 2011), and reduced IL-10 in FXS astrocytes (Talvio et al., 2023) might contribute to increased MYD88 (Chang et al., 2009). TLR4/MyD88-mediated induction of IL-6 is overactive in Fmr1 KO astrocytes (Krasovska and Doering, 2018), implicating involvement of inflammatory factors and suggesting alteration in TLR4/MyD88/PI3K interactions (Laird et al., 2009) in FXS astrocytes.
Cholesterol is an essential component of cell membranes, where it influences membrane fluidity and the formation of signaling microdomains (signaling rafts), such as what occur in dendritic spines (Hering et al., 2003). Cholesterol is also the precursor of neurosteroids (Lloyd-Evans and Waller-Evans, 2020), and it participates in signaling on its own by binding membrane proteins (Sheng et al., 2012). Cholesterol does not pass the blood brain barrier, so the CNS pool is maintained locally. Neurons and astrocytes synthesize cholesterol in development, but as neurons acquire maturity and brain total cholesterol reaches a plateau stage, astrocytes become largely responsible for maintaining lipid homeostasis in the brain (Jin et al., 2019; Chen et al., 2023). There is evidence that astrocytic cholesterol stimulates the formation of synapses in radial glial cell cultures (Mauch et al., 2001).
A recently published extensive work by Ren and colleagues (Ren et al., 2023) on human stem cell-derived astrocytes identified cholesterol synthesis pathway to be downregulated in the proteomics analysis. Increased lanosterol synthase and lanosterol, an intermediate oxysteroid in the Bloch pathway of cholesterol synthesis, were associated with reduced downstream enzymes and total cholesterol in three out of the four FXS cell lines studied and compared with their isogenic controls. In studies of Fmr1 KO mouse astrocytes, cholesterol, and desmosterol (the last precursor of cholesterol in the Bloch pathway) accumulated in astrocytes (Talvio et al., 2023). Here, cholesterol levels did not differ between human or mouse FMRP-deficient and control astrocyte conditioned medium, suggesting that astrocytes maintained extracellular cholesterol homeostasis to avoid cholesterol toxicity (Adachi et al., 2022; Talvio et al., 2023). Both mouse and human FMRP-deficient astrocytes expressed less ATP-binding cassette transporter A1 (ABCA1, the main astrocytic cholesterol exporter) than their respective controls. Reduced ABCA1 expression associated with reduced secretion of IL-13 and IL-10, which are implicated in ABCA1 regulation (Cardilo-Reis et al., 2012; Ma et al., 2012). Since astrocyte culturing conditions and differentiation protocols for production of iPSC-derived astrocytes can affect lipidomics of astrocytes, they may explain differences in the cholesterol balance observed in different FXS astrocyte models (Ren et al., 2023; Talvio et al., 2023). Leukemia inhibitory factor LIF is a factor used to specify astrocytes during differentiation (Ren et al., 2023) and by inducing ABCA1 expression it might modulate cholesterol secretion (Trouillas et al., 2009).
There are several changes in the lipidome of the Fmr1 KO mouse astrocytes (Talvio et al., 2023). ABCA1 affects membrane structure by transporting cholesterol, sphingomyelin, phosphatidylserine, and phosphatidylcholine (PC) species from the cytoplasmic to the exocytoplasmic leaflet (Quazi and Molday, 2013). PC species, which are the most common membrane phospholipids, were the most differently regulated membrane lipid class in the Fmr1 KO astrocyte lipidomics analysis (Talvio et al., 2023). Fmr1 KO astrocytes contained more of the most unsaturated PC species (Talvio et al., 2023). Mutual aversion by cholesterol and polyunsaturated fatty acids (PUFA) drives the segregation of membrane microdomains (Wassall and Stillwell, 2009). The capacity of the Fmr1 KO astrocyte membranes to buffer cholesterol overload and prevent cholesterol toxicity was compromised due to the phospholipid profile containing highly unsaturated PC species and less sphingomyelin (Talvio et al., 2023). Further studies are needed to determine FXS-specific lipid changes in astrocytes more broadly.
Astrocytes express FMRP during development (Pacey and Doering, 2007; Gholizadeh et al., 2015), and its absence influences the way astrocytes differentiate in both human and murine models of FXS. As summarized in Figure 1, studies of the mouse Fmr1 KO astrocytes and human stem cell-derived astrocytes have shown a number of differences between FMRP-deficient and healthy control astrocytes, including changes in factors secreted from astrocytes as well as transcriptomics and protein expression profiles, lipidomics, Ca2+ signaling, and inflammatory activity of astrocytes. Both human and mouse FMRP-deficient astrocytes display modulation of the plasminogen system, which can influence the development of CNS cell types. Astrocytes sense and respond to changes in the extracellular milieu, and studies on the Fmr1 KO mouse astrocytes have revealed alterations in astrocyte released factors that differentially regulate excitatory synapse development and how astrocytes influence glutamatergic signaling in the absence of FMRP. Altered cholesterol balance in Fmr1 KO mouse astrocytes together with increased levels of PUFA may contribute to the capacity of astrocytes to maintain extracellular homeostasis by modulating membrane properties and influencing receptor functions.
Figure 1. Schematic presentation summarizing alterations observed in FMRP-deficient astrocytes and their secretome (h, human; m, mouse). In addition to changes in specific known astrocytic molecules influencing synapses, various signaling pathways (BMP, WNT/β-catenin, and integrin signaling) involved in brain development and many aspects of brain homeostasis are differently regulated in FXS astrocytes.
The Fmr1 KO mouse model of FXS parallels the phenotype of FXS, and studies on the mouse model have shed light on the role of FMRP in CNS development and function. However, for instance, cognitive impairment and anxiety of the FXS phenotype are not fully recapitulated in the mouse model (Kazdoba et al., 2014). Human astrocytes are more complex than their mouse counterparts, and many abnormalities observed in Fmr1 KO astrocytes need to be replicated in human models. Patient-derived iPSC and FMR1 KO human stem cells provide a tool to model human astrocytes. However, mature astrocytes represent heterogeneous populations (Oberheim et al., 2012) and they mature in conjunction with associated neurons (Cheng Y. T. et al., 2023), indicating that neuronal contacts and neuronal differentiation are necessary for astrocyte maturation. In the absence of full understanding of astrocytic specialization and the processes that govern it, astrocyte monocultures are likely to exhibit immature astrocytes and simple co-culture setups fail to model the range of astrocytes needed in order to form global conclusion about astrocytic dysfunction. In vitro human organoid models may overcome the issue of modeling astrocytic variability and the Fmr1 KO murine models facilitate replication of results in vivo.
KT: Writing – review & editing. MC: Writing – review & editing.
The authors declare financial support was received for the research, authorship, and/or publication of this article. Research of KT was supported by the grants from the Arvo and Lea Ylppö Foundation, and the Jane and Aatos Erkko Foundation.
The authors are grateful to FRAXA Research Foundation whose support enabled the continuous astrocyte research of the authors.
The authors declare that the research was conducted in the absence of any commercial or financial relationships that could be construed as a potential conflict of interest.
The authors declared that they were an editorial board member of Frontiers, at the time of submission. This had no impact on the peer review process and the final decision.
All claims expressed in this article are solely those of the authors and do not necessarily represent those of their affiliated organizations, or those of the publisher, the editors and the reviewers. Any product that may be evaluated in this article, or claim that may be made by its manufacturer, is not guaranteed or endorsed by the publisher.
Achuta, V. S., Rezov, V., Uutela, M., Louhivuori, V., Louhivuori, L., and Castrén, M. L. (2014). Tissue plasminogen activator contributes to alterations of neuronal migration and activity-dependent responses in fragile X mice. J. Neurosci. 34, 1916–1923. doi: 10.1523/JNEUROSCI.3753-13.2014
Adachi, C., Otsuka, S., and Inoue, T. (2022). Cholesterol-induced robust Ca oscillation in astrocytes required for survival and lipid droplet formation in high-cholesterol condition. iScience 25:105138. doi: 10.1016/j.isci.2022.105138
Asiminas, A., Jackson, A. D., Louros, S. R., Till, S. M., Spano, T., Dando, O., et al. (2019). Sustained correction of associative learning deficits after brief, early treatment in a rat model of Fragile X Syndrome. Sci. Transl. Med. 11:eaao0498. doi: 10.1126/scitranslmed.aao0498
Bagni, C., and Zukin, R. S. (2019). A synaptic perspective of fragile X syndrome and autism spectrum Disorders. Neuron 101, 1070–1088. doi: 10.1016/j.neuron.2019.02.041
Bassell, G. J., and Warren, S. T. (2008). Fragile X syndrome: Loss of local mRNA regulation alters synaptic development and function. Neuron 60, 201–214. doi: 10.1016/j.neuron.2008.10.004
Bazargani, N., and Attwell, D. (2016). Astrocyte calcium signaling: The third wave. Nat. Neurosci. 19, 182–189. doi: 10.1038/nn.4201
Bear, M. F., Huber, K. M., and Warren, S. T. (2004). The mGluR theory of fragile X mental retardation. Trends Neurosci. 27, 370–377. doi: 10.1016/j.tins.2004.04.009
Bergami, M., Santi, S., Formaggio, E., Cagnoli, C., Verderio, C., Blum, R., et al. (2008). Uptake and recycling of pro-BDNF for transmitter-induced secretion by cortical astrocytes. J. Cell Biol. 183, 213–221. doi: 10.1083/jcb.200806137
Berry-Kravis, E., Hagerman, R., Budimirovic, D., Erickson, C., Heussler, H., Tartaglia, N., et al. (2022). A randomized, controlled trial of ZYN002 cannabidiol transdermal gel in children and adolescents with fragile X syndrome (CONNECT-FX). J. Neurodev. Disord. 14:56. doi: 10.1186/s11689-022-09466-6
Booker, S. A., Domanski, A. P. F., Dando, O. R., Jackson, A. D., Isaac, J. T. R., Hardingham, G. E., et al. (2019). Altered dendritic spine function and integration in a mouse model of fragile X syndrome. Nat. Commun. 10, 4813. doi: 10.1038/s41467-019-11891-6
Briens, A., Bardou, I., Lebas, H., Miles, L. A., Parmer, R. J., Vivien, D., et al. (2017). Astrocytes regulate the balance between plasminogen activation and plasmin clearance via cell-surface actin. Cell Discov. 3:17001. doi: 10.1038/celldisc.2017.1
Brighi, C., Salaris, F., Soloperto, A., Cordella, F., Ghirga, S., de Turris, V., et al. (2021). Novel fragile X syndrome 2D and 3D brain models based on human isogenic FMRP-KO iPSCs. Cell Death Dis. 12:498. doi: 10.1038/s41419-021-03776-8
Çaku, A., Pellerin, D., Bouvier, P., Riou, E., and Corbin, F. (2014). Effect of lovastatin on behavior in children and adults with fragile X syndrome: An open-label study. Am. J. Med. Genet. A 164A, 2834–2842. doi: 10.1002/ajmg.a.36750
Caldwell, A. L. M., Sancho, L., Deng, J., Bosworth, A., Miglietta, A., Diedrich, J. K., et al. (2022). Aberrant astrocyte protein secretion contributes to altered neuronal development in multiple models of neurodevelopmental disorders. Nat. Neurosci. 25, 1163–1178. doi: 10.1038/s41593-022-01150-1
Cardilo-Reis, L., Gruber, S., Schreier, S. M., Drechsler, M., Papac-Milicevic, N., Weber, C., et al. (2012). Interleukin-13 protects from atherosclerosis and modulates plaque composition by skewing the macrophage phenotype. EMBO Mol. Med. 4, 1072–1086. doi: 10.1002/emmm.201201374
Cassé, F., Bardou, I., Danglot, L., Briens, A., Montagne, A., Parcq, J., et al. (2012). Glutamate controls tPA recycling by astrocytes, which in turn influences glutamatergic signals. J. Neurosci. 32, 5186–5199. doi: 10.1523/JNEUROSCI.5296-11.2012
Champigny, C., Morin-Parent, F., Bellehumeur-Lefebvre, L., Çaku, A., Lepage, J. F., and Corbin, F. (2022). Combining lovastatin and minocycline for the treatment of fragile X syndrome: Results from the LovaMiX clinical trial. Front. Psychiatry 12:762967. doi: 10.3389/fpsyt.2021.762967
Chang, J., Kunkel, S. L., and Chang, C. H. (2009). Negative regulation of MyD88-dependent signaling by IL-10 in dendritic cells. Proc. Natl. Acad. Sci. U.S.A. 106, 18327–18332. doi: 10.1073/pnas.0905815106
Chen, D. Y., Sun, N. H., Lu, Y. P., Hong, L. J., Cui, T. T., Wang, C. K., et al. (2019). GPR124 facilitates pericyte polarization and migration by regulating the formation of filopodia during ischemic injury. Theranostics 9, 5937–5955. doi: 10.7150/thno.34168
Chen, Z., Yuan, Z., Yang, S., Zhu, Y., Xue, M., Zhang, J., et al. (2023). Brain energy metabolism: Astrocytes in neurodegenerative diseases. CNS Neurosci. Ther. 29, 24–36. doi: 10.1111/cns.13982
Cheng, C., Lau, S. K., and Doering, L. C. (2016). Astrocyte-secreted thrombospondin-1 modulates synapse and spine defects in the fragile X mouse model. Mol. Brain 9:74. doi: 10.1186/s13041-016-0256-9
Cheng, C., Sourial, M., and Doering, L. C. (2012). Astrocytes and developmental plasticity in fragile X. Neural Plast. 2012:197491. doi: 10.1155/2012/197491
Cheng, S., Xu, J., Wang, W., Wang, R., Li, H., Jiang, Z., et al. (2023). Inhibition of mGluR5 alters BDNF/TrkB and GLT-1 expression in the prefrontal cortex and hippocampus and ameliorates PTSD-like behavior in rats. Psychopharmacology 240, 837–851. doi: 10.1007/s00213-023-06325-7
Cheng, Y. T., Woo, J., and Deneen, B. (2023). Sculpting Astrocyte Diversity through Circuits and Transcription. Neuroscientist 29, 445–460. doi: 10.1177/10738584221082620
Cheon, K. A., Kim, Y. S., Oh, S. H., Park, S. Y., Yoon, H. W., Herrington, J., et al. (2011). Involvement of the anterior thalamic radiation in boys with high functioning autism spectrum disorders: A Diffusion Tensor Imaging study. Brain Res. 1417, 77–86. doi: 10.1016/j.brainres.2011.08.020
Ciaccio, C., Fontana, L., Milani, D., Tabano, S., Miozzo, M., and Esposito, S. (2017). Fragile X syndrome: A review of clinical and molecular diagnoses. Ital. J. Pediatr. 43:39. doi: 10.1186/s13052-017-0355-y
Clavreul, S., Dumas, L., and Loulier, K. (2022). Astrocyte development in the cerebral cortex: Complexity of their origin, genesis, and maturation. Front. Neurosci. 16:916055. doi: 10.3389/fnins.2022.916055
Cowley, B., Kirjanen, S., Partanen, J., and Castrén, M. L. (2016). Epileptic electroencephalography profile associates with attention problems in children with fragile X syndrome: Review and case series. Front. Hum. Neurosci. 10:353. doi: 10.3389/fnhum.2016.00353
Cruz-Martín, A., Crespo, M., and Portera-Cailliau, C. (2010). Delayed stabilization of dendritic spines in fragile X mice. J. Neurosci. 30, 7793–7803. doi: 10.1523/JNEUROSCI.0577-10.2010
D’Antoni, S., de Bari, L., Valenti, D., Borro, M., Bonaccorso, C. M., Simmaco, M., et al. (2020). Aberrant mitochondrial bioenergetics in the cerebral cortex of the Fmr1 knockout mouse model of fragile X syndrome. Biol. Chem. 401, 497–503. doi: 10.1515/hsz-2019-0221
Darnell, J. C., Van Driesche, S. J., Zhang, C., Hung, K. Y., Mele, A., Fraser, C. E., et al. (2011). FMRP stalls ribosomal translocation on mRNAs linked to synaptic function and autism. Cell 146, 247–261. doi: 10.1016/j.cell.2011.06.013
Das Sharma, S., Pal, R., Reddy, B. K., Selvaraj, B. T., Raj, N., Samaga, K. K., et al. (2020). Cortical neurons derived from human pluripotent stem cells lacking FMRP display altered spontaneous firing patterns. Mol. Autism 11:52. doi: 10.1186/s13229-020-00351-4
Das Sharma, S., Reddy, B. K., Pal, R., Ritakari, T. E., Cooper, J. D., Selvaraj, B. T., et al. (2023). Astrocytes mediate cell non-autonomous correction of aberrant firing in human FXS neurons. Cell Rep. 42:112344. doi: 10.1016/j.celrep.2023.112344
Dy, A. B. C., Tassone, F., Eldeeb, M., Salcedo-Arellano, M. J., Tartaglia, N., and Hagerman, R. (2018). Metformin as targeted treatment in fragile X syndrome. Clin. Genet. 93, 216–222. doi: 10.1111/cge.13039
Edens, B. M., Vissers, C., Su, J., Arumugam, S., Xu, Z., Shi, H., et al. (2019). FMRP modulates neural differentiation through m6A-dependent mRNA nuclear export. Cell Rep. 28, 845–854.e5. doi: 10.1016/j.celrep.2019.06.072
el Bekay, R., Romero-Zerbo, Y., Decara, J., Sanchez-Salido, L., Del Arco-Herrera, I., Rodríguez-de Fonseca, F., et al. (2007). Enhanced markers of oxidative stress, altered antioxidants and NADPH-oxidase activation in brains from Fragile X mental retardation 1-deficient mice, a pathological model for Fragile X syndrome. Eur. J. Neurosci. 26, 3169–3180. doi: 10.1111/j.1460-9568.2007.05939.x
Escartin, C., Galea, E., Lakatos, A., O’Callaghan, J. P., Petzold, G. C., Serrano-Pozo, A., et al. (2021). Reactive astrocyte nomenclature, definitions, and future directions. Nat. Neurosci. 24, 312–325. doi: 10.1038/s41593-020-00783-4
Feng, Y., Gutekunst, C. A., Eberhart, D. E., Yi, H., Warren, S. T., and Hersch, S. M. (1997). Fragile X mental retardation protein: Nucleocytoplasmic shuttling and association with somatodendritic ribosomes. J. Neurosci. 17, 1539–1547. doi: 10.1523/JNEUROSCI.17-05-01539.1997
Fernández-Blanco, Á, and Dierssen, M. (2020). Rethinking intellectual disability from neuro- to astro-pathology. Int. J. Mol. Sci. 21:9039. doi: 10.3390/ijms21239039
Fu, Y. H., Kuhl, D. P., Pizzuti, A., Pieretti, M., Sutcliffe, J. S., Richards, S., et al. (1991). Variation of the CGG repeat at the fragile X site results in genetic instability: Resolution of the Sherman paradox. Cell 67, 1047–1058. doi: 10.1016/0092-8674(91)90283-5
Gabis, L. V., Hochberg, O., Leon Attia, O., Banet-Levi, Y., Topf, D., and Shefer, S. (2017). Prolonged time lag to final diagnosis of fragile X syndrome. J. Pediatr. 193, 217–221.e1. doi: 10.1016/j.jpeds.2017.10.008
Gao, F., Qi, L., Yang, Z., Yang, T., Zhang, Y., Xu, H., et al. (2018). Impaired GABA neural circuits are critical for fragile X syndrome. Neural. Plast. 2018:8423420. doi: 10.1155/2018/8423420
Gholizadeh, S., Halder, S. K., and Hampson, D. R. (2015). Expression of fragile X mental retardation protein in neurons and glia of the developing and adult mouse brain. Brain Res. 1596, 22–30. doi: 10.1016/j.brainres.2014.11.023
Goeke, C. M., Zhang, X., Hashimoto, J. G., and Guizzetti, M. (2022). Astrocyte tissue plasminogen activator expression during brain development and its role in pyramidal neuron neurite outgrowth. Neurosci. Lett. 769:136422. doi: 10.1016/j.neulet.2021.136422
Goenaga, J., Araque, A., Kofuji, P., and Herrera Moro Chao, D. (2023). Calcium signaling in astrocytes and gliotransmitter release. Front. Synaptic. Neurosci. 15:1138577. doi: 10.3389/fnsyn.2023.1138577
Gorina, R., Font-Nieves, M., Márquez-Kisinousky, L., Santalucia, T., and Planas, A. M. (2011). Astrocyte TLR4 activation induces a proinflammatory environment through the interplay between MyD88-dependent NFκB signaling, MAPK, and Jak1/Stat1 pathways. Glia 59, 242–255. doi: 10.1002/glia.21094
Gredell, M., Lu, J., and Zuo, Y. (2023). The effect of single-cell knockout of Fragile X Messenger Ribonucleoprotein on synaptic structural plasticity. Front. Synaptic Neurosci. 15:1135479. doi: 10.3389/fnsyn.2023.1135479
Habbas, K., Cakil, O., Zámbó, B., Tabet, R., Riet, F., Dembele, D., et al. (2022). AAV-delivered diacylglycerol kinase DGKk achieves long-term rescue of fragile X syndrome mouse model. EMBO Mol. Med. 14:e14649. doi: 10.15252/emmm.202114649
Hagerman, P. J. (2008). The fragile X prevalence paradox. J. Med. Genet. 45, 498–499. doi: 10.1136/jmg.2008.059055
Hagerman, P. J., Greco, C. M., and Hagerman, R. J. (2003). A cerebellar tremor/ataxia syndrome among fragile X premutation carriers. Cytogenet. Genome Res. 100, 206–212. doi: 10.1159/000072856
Halassa, M. M., Fellin, T., Takano, H., Dong, J. H., and Haydon, P. G. (2007). Synaptic islands defined by the territory of a single astrocyte. J. Neurosci. 27, 6473–6477. doi: 10.1523/JNEUROSCI.1419-07.2007
Harlow, E. G., Till, S. M., Russell, T. A., Wijetunge, L. S., Kind, P., and Contractor, A. (2010). Critical period plasticity is disrupted in the barrel cortex of FMR1 knockout mice. Neuron 65, 385–398. doi: 10.1016/j.neuron.2010.01.024
Harris, S. W., Hessl, D., Goodlin-Jones, B., Ferranti, J., Bacalman, S., Barbato, I., et al. (2008). Autism profiles of males with fragile X syndrome. Am. J. Ment. Retard. 113, 427–438. doi: 10.1352/2008.113:427-438
He, C. X., and Portera-Cailliau, C. (2013). The trouble with spines in fragile X syndrome: Density, maturity and plasticity. Neuroscience 251, 120–128. doi: 10.1016/j.neuroscience.2012.03.049
Hering, H., Lin, C. C., and Sheng, M. (2003). Lipid rafts in the maintenance of synapses, dendritic spines, and surface AMPA receptor stability. J. Neurosci. 23, 3262–3271. doi: 10.1523/JNEUROSCI.23-08-03262.2003
Higashimori, H., Morel, L., Huth, J., Lindemann, L., Dulla, C., Taylor, A., et al. (2013). Astroglial FMRP-dependent translational down-regulation of mGluR5 underlies glutamate transporter GLT1 dysregulation in the fragile X mouse. Hum. Mol. Genet. 22, 2041–2054. doi: 10.1093/hmg/ddt055
Higashimori, H., Schin, C. S., Chiang, M. S., Morel, L., Shoneye, T. A., Nelson, D. L., et al. (2016). Selective deletion of astroglial FMRP dysregulates glutamate transporter GLT1 and contributes to fragile X syndrome phenotypes in vivo. J. Neurosci. 36, 7079–7094. doi: 10.1523/JNEUROSCI.1069-16.2016
Hinds, H. L., Ashley, C. T., Sutcliffe, J. S., Nelson, D. L., Warren, S. T., Housman, D. E., et al. (1993). Tissue specific expression of FMR-1 provides evidence for a functional role in fragile X syndrome. Nat. Genet. 3, 36–43. doi: 10.1038/ng0193-36
Hodges, J. L., Yu, X., Gilmore, A., Bennett, H., Tjia, M., Perna, J. F., et al. (2017). Astrocytic contributions to synaptic and learning abnormalities in a mouse model of fragile X syndrome. Biol. Psychiatry 82, 139–149. doi: 10.1016/j.biopsych.2016.08.036
Hösli, L., Binini, N., Ferrari, K. D., Thieren, L., Looser, Z. J., Zuend, M., et al. (2022). Decoupling astrocytes in adult mice impairs synaptic plasticity and spatial learning. Cell Rep. 38:110484. doi: 10.1016/j.celrep.2022.110484
Hu, S., Sheng, W. S., Ehrlich, L. C., Peterson, P. K., and Chao, C. C. (2000). Cytokine effects on glutamate uptake by human astrocytes. Neuroimmunomodulation 7, 153–159. doi: 10.1159/000026433
Jacobs, S., and Doering, L. C. (2010). Astrocytes prevent abnormal neuronal development in the fragile X mouse. J. Neurosci. 30, 4508–4514. doi: 10.1523/JNEUROSCI.5027-09.2010
Jin, U., Park, S. J., and Park, S. M. (2019). Cholesterol metabolism in the brain and its association with Parkinson’s disease. Exp. Neurobiol. 28, 554–567. doi: 10.5607/en.2019.28.5.554
Kalderon, N., Ahonen, K., and Fedoroff, S. (1990). Developmental transition in plasticity properties of differentiating astrocytes: Age-related biochemical profile of plasminogen activators in astroglial cultures. Glia 3, 413–426. doi: 10.1002/glia.440030513
Kazdoba, T. M., Leach, P. T., Silverman, J. L., and Crawley, J. N. (2014). Modeling fragile X syndrome in the Fmr1 knockout mouse. Intractable Rar. Dis. Res. 3, 118–133. doi: 10.5582/irdr.2014.01024
Kilb, W., and Kirischuk, S. (2022). GABA release from astrocytes in health and disease. Int. J. Mol. Sci. 23:15859. doi: 10.3390/ijms232415859
Kim, M., Bellini, M., and Ceman, S. (2009). Fragile X mental retardation protein FMRP binds mRNAs in the nucleus. Mol. Cell. Biol. 29, 214–228. doi: 10.1128/MCB.01377-08
Krasovska, V., and Doering, L. C. (2018). Regulation of IL-6 secretion by astrocytes via TLR4 in the fragile X mouse model. Front. Mol. Neurosci. 11:272. doi: 10.3389/fnmol.2018.00272
Kucukdereli, H., Allen, N. J., Lee, A. T., Feng, A., Ozlu, M. I., Conatser, L. M., et al. (2011). Control of excitatory CNS synaptogenesis by astrocyte-secreted proteins Hevin and SPARC. Proc. Natl. Acad. Sci. U.S.A. 108, E440–E449. doi: 10.1073/pnas.1104977108
Laggerbauer, B., Ostareck, D., Keidel, E. M., Ostareck-Lederer, A., and Fischer, U. (2001). Evidence that fragile X mental retardation protein is a negative regulator of translation. Hum. Mol. Genet. 10, 329–338. doi: 10.1093/hmg/10.4.329
Laird, M. H., Rhee, S. H., Perkins, D. J., Medvedev, A. E., Piao, W., Fenton, M. J., et al. (2009). TLR4/MyD88/PI3K interactions regulate TLR4 signaling. J. Leukoc. Biol. 85, 966–977. doi: 10.1189/jlb.1208763
Lee, H. G., Wheeler, M. A., and Quintana, F. J. (2022). Function and therapeutic value of astrocytes in neurological diseases. Nat. Rev. Drug. Discov. 21, 339–358. doi: 10.1038/s41573-022-00390-x
Lee, S. C., Dickson, D. W., Liu, W., and Brosnan, C. F. (1993). Induction of nitric oxide synthase activity in human astrocytes by interleukin-1 beta and interferon-gamma. J. Neuroimmunol. 46, 19–24. doi: 10.1016/0165-5728(93)90229-r
Liu, X., Kumar, V., Tsai, N. P., and Auerbach, B. D. (2022). Hyperexcitability and homeostasis in fragile X Syndrome. Front. Mol. Neurosci. 14:805929. doi: 10.3389/fnmol.2021.805929
Liu, Z. H., and Smith, C. B. (2009). Dissociation of social and nonsocial anxiety in a mouse model of fragile X syndrome. Neurosci. Lett. 454, 62–66. doi: 10.1016/j.neulet.2009.02.066
Lloyd-Evans, E., and Waller-Evans, H. (2020). Biosynthesis and signalling functions of central and peripheral nervous system neurosteroids in health and disease. Essays Biochem. 64, 591–606. doi: 10.1042/EBC20200043
Lozano, R., Azarang, A., Wilaisakditipakorn, T., and Hagerman, R. J. (2016). Fragile X syndrome: A review of clinical management. Intractable Rare Dis. Res. 5, 145–157. doi: 10.5582/irdr.2016.01048
Ma, L., Dong, F., Zaid, M., Kumar, A., and Zha, X. (2012). ABCA1 protein enhances Toll-like receptor 4 (TLR4)-stimulated interleukin-10 (IL-10) secretion through protein kinase A (PKA) activation. J. Biol. Chem. 287, 40502–40512. doi: 10.1074/jbc.M112.413245
Martin, B. S., and Huntsman, M. M. (2012). Pathological plasticity in fragile X syndrome. Neural Plast. 2012:275630. doi: 10.1155/2012/275630
Martin, J. P., and Bell, J. (1943). A pedigree of mental defect showing sex-linkage. J. Neurol. Psychiatry 6, 154–157. doi: 10.1136/jnnp.6.3-4.154
Mauch, D. H., Nägler, K., Schumacher, S., Göritz, C., Müller, E. C., Otto, A., et al. (2001). CNS synaptogenesis promoted by glia-derived cholesterol. Science 294, 1354–1357. doi: 10.1126/science.294.5545.1354
Men, Y., Higashimori, H., Reynolds, K., Tu, L., Jarvis, R., and Yang, Y. (2022). Functionally clustered mRNAs are distinctly enriched at cortical astroglial processes and are preferentially affected by FMRP deficiency. J. Neurosci. 42, 5803–5814. doi: 10.1523/JNEUROSCI.0274-22.2022
Men, Y., Ye, L., Risgaard, R. D., Promes, V., Zhao, X., Paukert, M., et al. (2020). Astroglial FMRP deficiency cell-autonomously up-regulates miR-128 and disrupts developmental astroglial mGluR5 signaling. Proc. Natl. Acad. Sci. U.S.A. 117, 25092–25103. doi: 10.1073/pnas.2014080117
Milla, L. A., Corral, L., Rivera, J., Zuñiga, N., Pino, G., Nunez-Parra, A., et al. (2023). Neurodevelopment and early pharmacological interventions in Fragile X Syndrome. Front. Neurosci. 17:1213410. doi: 10.3389/fnins.2023.1213410
Mizuno, A., Villalobos, M. E., Davies, M. M., Dahl, B. C., and Müller, R. A. (2006). Partially enhanced thalamocortical functional connectivity in autism. Brain Res. 1104, 160–174. doi: 10.1016/j.brainres.2006.05.064
Muscas, M., Louros, S. R., and Osterweil, E. K. (2019). Lovastatin, not simvastatin, corrects core phenotypes in the fragile X mouse model. eNeuro 6, ENEURO.0097–19.2019. doi: 10.1523/ENEURO.0097-19.2019
Nair, A., Treiber, J. M., Shukla, D. K., Shih, P., and Müller, R. A. (2013). Impaired thalamocortical connectivity in autism spectrum disorder: A study of functional and anatomical connectivity. Brain 136(Pt. 6), 1942–1955. doi: 10.1093/brain/awt079
Nolin, S. L., Lewis, F. A. III, Ye, L. L., Houck, G. E. Jr., Glicksman, A. E., Limprasert, P., et al. (1996). Familial transmission of the FMR1 CGG repeat. Am. J. Hum. Genet. 59, 1252–1261.
Oberheim, N. A., Goldman, S. A., and Nedergaard, M. (2012). Heterogeneity of astrocytic form and function. Methods Mol. Biol. 814, 23–45. doi: 10.1007/978-1-61779-452-0_3
Oberheim, N. A., Takano, T., Han, X., He, W., Lin, J. H., Wang, F., et al. (2009). Uniquely hominid features of adult human astrocytes. J. Neurosci. 29, 3276–3287. doi: 10.1523/JNEUROSCI.4707-08.2009
Osterweil, E. K., Chuang, S. C., Chubykin, A. A., Sidorov, M., Bianchi, R., Wong, R. K., et al. (2013). Lovastatin corrects excess protein synthesis and prevents epileptogenesis in a mouse model of fragile X syndrome. Neuron 77, 243–250. doi: 10.1016/j.neuron.2012.01.034
Pacey, L. K., and Doering, L. C. (2007). Developmental expression of FMRP in the astrocyte lineage: Implications for fragile X syndrome. Glia 55, 1601–1609. doi: 10.1002/glia.20573
Pacey, L. K., Guan, S., Tharmalingam, S., Thomsen, C., and Hampson, D. R. (2015). Persistent astrocyte activation in the fragile X mouse cerebellum. Brain Behav. 5:e00400. doi: 10.1002/brb3.400
Padmashri, R., Reiner, B. C., Suresh, A., Spartz, E., and Dunaevsky, A. (2013). Altered structural and functional synaptic plasticity with motor skill learning in a mouse model of fragile X syndrome. J. Neurosci. 33, 19715–19723. doi: 10.1523/JNEUROSCI.2514-13.2013
Pan, F., Aldridge, G. M., Greenough, W. T., and Gan, W. B. (2010). Dendritic spine instability and insensitivity to modulation by sensory experience in a mouse model of fragile X syndrome. Proc. Natl. Acad. Sci. U.S.A. 107, 17768–17773. doi: 10.1073/pnas.1012496107
Perez-Catalan, N. A., Doe, C. Q., and Ackerman, S. D. (2021). The role of astrocyte-mediated plasticity in neural circuit development and function. Neural. Dev. 16:1. doi: 10.1186/s13064-020-00151-9
Peteri, U. K., Pitkonen, J., de Toma, I., Nieminen, O., Utami, K. H., Strandin, T. M., et al. (2021). Urokinase plasminogen activator mediates changes in human astrocytes modeling fragile X syndrome. Glia 69, 2947–2962. doi: 10.1002/glia.24080
Proteau-Lemieux, M., Lacroix, A., Galarneau, L., Corbin, F., Lepage, J. F., and Çaku, A. (2021). The safety and efficacy of metformin in fragile X syndrome: An open-label study. Prog. Neuropsychopharmacol. Biol. Psychiatry 110:110307. doi: 10.1016/j.pnpbp.2021.110307
Quazi, F., and Molday, R. S. (2013). Differential phospholipid substrates and directional transport by ATP-binding cassette proteins ABCA1, ABCA7, and ABCA4 and disease-causing mutants. J. Biol. Chem. 288, 34414–34426. doi: 10.1074/jbc.M113.508812
Rais, M., Kulinich, A. O., Wagner, V., Woodard, W., Shuai, X. S., Sutley, S. N., et al. (2022). Astrocytes regulate inhibition in Fragile X Syndrome. bioRxiv [Preprint] doi: 10.1101/2022.02.08.479618
Raj, N., McEachin, Z. T., Harousseau, W., Zhou, Y., Zhang, F., Merritt-Garza, M. E., et al. (2021). Cell-type-specific profiling of human cellular models of fragile X syndrome reveal PI3K-dependent defects in translation and neurogenesis. Cell Rep. 35:108991. doi: 10.1016/j.celrep.2021.108991
Ren, B., Burkovetskaya, M., Jung, Y., Bergdolt, L., Totusek, S., Martinez-Cerdeno, V., et al. (2023). Dysregulated cholesterol metabolism, aberrant excitability and altered cell cycle of astrocytes in fragile X syndrome. Glia 71, 1176–1196. doi: 10.1002/glia.24331
Risher, W. C., Patel, S., Kim, I. H., Uezu, A., Bhagat, S., Wilton, D. K., et al. (2014). Astrocytes refine cortical connectivity at dendritic spines. Elife 3:e04047. doi: 10.7554/eLife.04047
Saffary, R., and Xie, Z. (2011). FMRP regulates the transition from radial glial cells to intermediate progenitor cells during neocortical development. J. Neurosci. 31, 1427–1439. doi: 10.1523/JNEUROSCI.4854-10.2011
Satoh, J., Tabunoki, H., Yamamura, T., Arima, K., and Konno, H. (2007). Human astrocytes express aquaporin-1 and aquaporin-4 in vitro and in vivo. Neuropathology 27, 245–256. doi: 10.1111/j.1440-1789.2007.00774.x
Schlag, B. D., Vondrasek, J. R., Munir, M., Kalandadze, A., Zelenaia, O. A., Rothstein, J. D., et al. (1998). Regulation of the glial Na+-dependent glutamate transporters by cyclic AMP analogs and neurons. Mol. Pharmacol. 53, 355–369. doi: 10.1124/mol.53.3.355
Scholze, A. R., Foo, L. C., Mulinyawe, S., and Barres, B. A. (2014). BMP signaling in astrocytes downregulates EGFR to modulate survival and maturation. PLoS One 9:e110668. doi: 10.1371/journal.pone.0110668
Schwartz, C. E., Dean, J., Howard-Peebles, P. N., Bugge, M., Mikkelsen, M., Tommerup, N., et al. (1994). Obstetrical and gynecological complications in fragile X carriers: A multicenter study. Am. J. Med. Genet. 51, 400–402. doi: 10.1002/ajmg.1320510419
Sharma, V., Mishra, M., Ghosh, S., Tewari, R., Basu, A., Seth, P., et al. (2007). Modulation of interleukin-1beta mediated inflammatory response in human astrocytes by flavonoids: Implications in neuroprotection. Brain Res. Bull. 73, 55–63. doi: 10.1016/j.brainresbull.2007.01.016
Sheng, R., Chen, Y., Yung Gee, H., Stec, E., Melowic, H. R., Blatner, N. R., et al. (2012). Cholesterol modulates cell signaling and protein networking by specifically interacting with PDZ domain-containing scaffold proteins. Nat. Commun. 3:1249. doi: 10.1038/ncomms2221
Siomi, H., Siomi, M. C., Nussbaum, R. L., and Dreyfuss, G. (1993). The protein product of the fragile X gene, FMR1, has characteristics of an RNA-binding protein. Cell 74, 291–298. doi: 10.1016/0092-8674(93)90420-u
Stoppel, D. C., McCamphill, P. K., Senter, R. K., Heynen, A. J., and Bear, M. F. (2021). mGluR5 negative modulators for fragile X: Treatment resistance and persistence. Front. Psychiatry 12:718953. doi: 10.3389/fpsyt.2021.718953
Sullivan, K., Hatton, D., Hammer, J., Sideris, J., Hooper, S., Ornstein, P., et al. (2006). ADHD symptoms in children with FXS. Am. J. Med. Genet. A 140, 2275–2288. doi: 10.1002/ajmg.a.31388
Sunamura, N., Iwashita, S., Enomoto, K., Kadoshima, T., and Isono, F. (2018). Loss of the fragile X mental retardation protein causes aberrant differentiation in human neural progenitor cells. Sci. Rep. 8:11585. doi: 10.1038/s41598-018-30025-4
Sutcliffe, J. S., Nelson, D. L., Zhang, F., Pieretti, M., Caskey, C. T., Saxe, D., et al. (1992). DNA methylation represses FMR-1 transcription in fragile X syndrome. Hum. Mol. Genet. 1, 397–400. doi: 10.1093/hmg/1.6.397
Tabet, R., Moutin, E., Becker, J. A., Heintz, D., Fouillen, L., Flatter, E., et al. (2016). Fragile X Mental Retardation Protein (FMRP) controls diacylglycerol kinase activity in neurons. Proc. Natl. Acad. Sci. U.S.A. 113, E3619–E3628. doi: 10.1073/pnas.1522631113
Talvio, K., Minkeviciene, R., Townsley, K. G., Achuta, V. S., Huckins, L. M., Corcoran, P., et al. (2022). Reduced LYNX1 expression in transcriptome of human iPSC-derived neural progenitors modeling fragile X syndrome. Front. Cell. Dev. Biol. 10:1034679. doi: 10.3389/fcell.2022.1034679
Talvio, K., Wagner, V. A., Minkeviciene, R., Kirkwood, J. S., Kulinich, A. O., Umemori, J., et al. (2023). An iPSC-derived astrocyte model of fragile X syndrome exhibits dysregulated cholesterol homeostasis. Commun. Biol. 6:789. doi: 10.1038/s42003-023-05147-9
Tervonen, T. A., Louhivuori, V., Sun, X., Hokkanen, M. E., Kratochwil, C. F., Zebryk, P., et al. (2009). Aberrant differentiation of glutamatergic cells in neocortex of mouse model for fragile X syndrome. Neurobiol. Dis. 33, 250–259. doi: 10.1016/j.nbd.2008.10.010
Thurman, A. J., Potter, L. A., Kim, K., Tassone, F., Banasik, A., Potter, S. N., et al. (2020). Controlled trial of lovastatin combined with an open-label treatment of a parent-implemented language intervention in youth with fragile X syndrome. J. Neurodev. Disord. 12:12. doi: 10.1186/s11689-020-09315-4
Trouillas, M., Saucourt, C., Guillotin, B., Gauthereau, X., Ding, L., Buchholz, F., et al. (2009). Three LIF-dependent signatures and gene clusters with atypical expression profiles, identified by transcriptome studies in mouse ES cells and early derivatives. BMC Genomics 10:73. doi: 10.1186/1471-2164-10-73
Turner, D. A., and Adamson, D. C. (2011). Neuronal-astrocyte metabolic interactions: Understanding the transition into abnormal astrocytoma metabolism. J. Neuropathol. Exp. Neurol. 70, 167–176. doi: 10.1097/NEN.0b013e31820e1152
Utami, K. H., Skotte, N. H., Colaço, A. R., Yusof, N. A. B. M., Sim, B., Yeo, X. Y., et al. (2020). Integrative analysis identifies key molecular signatures underlying neurodevelopmental deficits in fragile X syndrome. Biol. Psychiatry 88, 500–511. doi: 10.1016/j.biopsych.2020.05.005
Utari, A., Chonchaiya, W., Rivera, S. M., Schneider, A., Hagerman, R. J., Faradz, S. M., et al. (2010). Side effects of minocycline treatment in patients with fragile X syndrome and exploration of outcome measures. Am. J. Intellect. Dev. Disabil. 115, 433–443. doi: 10.1352/1944-7558-115.5.433
Van der Aa, N., and Kooy, R. F. (2020). GABAergic abnormalities in the fragile X syndrome. Eur. J. Paediatr. Neurol. 24, 100–104. doi: 10.1016/j.ejpn.2019.12.022
Vandenberg, G. G., Thotakura, A., and Scott, A. L. (2022). Mitochondrial bioenergetics of astrocytes in Fragile X syndrome: New perspectives on culture conditions and sex effects. Am. J. Physiol. Cell Physiol. 322, C125–C135. doi: 10.1152/ajpcell.00130.2021
Verkerk, A. J. M. H., Pieretti, M., Sutcliffe, J. S., Fu, Y. H., Kuhl, D. P. A., Pizzuti, A., et al. (1991). Identification of a gene (FMR-1) containing a CGG repeat coincident with a breakpoint cluster region exhibiting length variation in fragile X syndrome. Cell 65, 905–914. doi: 10.1016/0092-8674(91)90397-h
von Bartheld, C. S., Bahney, J., and Herculano-Houzel, S. (2016). The search for true numbers of neurons and glial cells in the human brain: A review of 150 years of cell counting. J. Comp. Neurol. 524, 3865–3895. doi: 10.1002/cne.24040
Wallingford, J., Scott, A. L., Rodrigues, K., and Doering, L. C. (2017). Altered developmental expression of the astrocyte-secreted factors hevin and SPARC in the fragile X mouse model. Front. Mol. Neurosci. 10:268. doi: 10.3389/fnmol.2017.00268
Wassall, S. R., and Stillwell, W. (2009). Polyunsaturated fatty acid-cholesterol interactions: Domain formation in membranes. Biochim. Biophys. Acta 1788, 24–32. doi: 10.1016/j.bbamem.2008.10.011
Winarni, T. I., Schneider, A., Borodyanskara, M., and Hagerman, R. J. (2012). Early intervention combined with targeted treatment promotes cognitive and behavioral improvements in young children with fragile x syndrome. Case Rep. Genet. 2012:280813. doi: 10.1155/2012/280813
Yang, L., Li, Z., Liu, G., Li, X., and Yang, Z. (2022). Developmental origins of human cortical oligodendrocytes and astrocytes. Neurosci. Bull. 38, 47–68. doi: 10.1007/s12264-021-00759-9
Yang, Q., Feng, B., Zhang, K., Guo, Y. Y., Liu, S. B., Wu, Y. M., et al. (2012). Excessive astrocyte-derived neurotrophin-3 contributes to the abnormal neuronal dendritic development in a mouse model of fragile X syndrome. PLoS Genet. 8:e1003172. doi: 10.1371/journal.pgen.1003172
Keywords: fragile X syndrome, astrocytes, autism spectrum disorder, induced pluripotent stem cells, cholesterol, glutamate, calcium signaling, cell differentiation
Citation: Talvio K and Castrén ML (2024) Astrocytes in fragile X syndrome. Front. Cell. Neurosci. 17:1322541. doi: 10.3389/fncel.2023.1322541
Received: 16 October 2023; Accepted: 11 December 2023;
Published: 08 January 2024.
Edited by:
Marine A. Krzisch, University of Leeds, United KingdomReviewed by:
Caleb Andrew Doll, University of Colorado Anschutz Medical Campus, United StatesCopyright © 2024 Talvio and Castrén. This is an open-access article distributed under the terms of the Creative Commons Attribution License (CC BY). The use, distribution or reproduction in other forums is permitted, provided the original author(s) and the copyright owner(s) are credited and that the original publication in this journal is cited, in accordance with accepted academic practice. No use, distribution or reproduction is permitted which does not comply with these terms.
*Correspondence: Maija L. Castrén, bWFpamEuY2FzdHJlbkBoZWxzaW5raS5maQ==
Disclaimer: All claims expressed in this article are solely those of the authors and do not necessarily represent those of their affiliated organizations, or those of the publisher, the editors and the reviewers. Any product that may be evaluated in this article or claim that may be made by its manufacturer is not guaranteed or endorsed by the publisher.
Research integrity at Frontiers
Learn more about the work of our research integrity team to safeguard the quality of each article we publish.