- 1Department of Developmental, Molecular and Chemical Biology, Tufts University School of Medicine, Boston, MA, United States
- 2Graduate Program in Cell, Molecular and Developmental Biology, Tufts University School of Medicine, Boston, MA, United States
- 3Department of Biology, Simmons University, Boston, MA, United States
- 4Graduate Program in Cell, Molecular Biology, Developmental Biology, Graduate School of Biomedical Sciences, Tufts University School of Medicine, Boston, MA, United States
AMPA receptors (AMPARs) mediate the majority of fast excitatory transmission in the brain. Regulation of AMPAR levels at synapses controls synaptic strength and underlies information storage and processing. Many proteins interact with the intracellular domain of AMPARs to regulate their trafficking and synaptic clustering. However, a growing number of extracellular factors important for glutamatergic synapse development, maturation and function have emerged that can also regulate synaptic AMPAR levels. This mini-review highlights extracellular protein factors that regulate AMPAR trafficking to control synapse development and plasticity. Some of these factors regulate AMPAR clustering and mobility by interacting with the extracellular N-terminal domain of AMPARs whereas others regulate AMPAR trafficking indirectly via their respective signaling receptors. While several of these factors are secreted from neurons, others are released from non-neuronal cells such as glia and muscle. Although it is apparent that secreted factors can act locally on neurons near their sites of release to coordinate individual synapses, it is less clear if they can diffuse over longer ranges to coordinate related synapses within a circuit or region of the brain. Given that there are hundreds of factors that can be secreted from neuronal and non-neuronal cells, it will not be surprising if more extracellular factors that modulate AMPARs and glutamatergic synapses are discovered. Many open questions remain including where and when the factors are expressed, what regulates their secretion from different cell types, what controls their diffusion, stability, and range of action, and how their cognate receptors influence intracellular signaling to control AMPAR trafficking.
Introduction
Forming and maintaining functional glutamatergic synapses is crucial for proper brain function as glutamatergic transmission underlies vital processes such as cognition, motor coordination, learning and memory. Dysfunction in glutamatergic synapse formation, maintenance and function has been implicated in conditions ranging from autism spectrum disorders to epilepsy to neurodegenerative disorders, highlighting the importance of glutamate signaling for brain function.
AMPA receptors (AMPARs) are the principal ionotropic glutamate receptors that mediate fast, excitatory synaptic transmission. AMPARs are tetrameric cation channels that are assembled from a combination of 4 different subunits, GluA1-GluA4 (Hansen et al., 2021). Subunit composition and association with intracellular proteins and auxiliary subunits in the membrane, like Transmembrane Associated Receptor Proteins (TARPs), confers distinct trafficking characteristics. Both composition and expression levels vary across brain regions, adding another layer of complexity. Synaptic AMPAR abundance is a major determinant of synaptic strength and is tightly regulated via multiple mechanisms including receptor synthesis, trafficking, membrane insertion, clustering, internalization, recycling, and degradation, as well as modulation of channel function (Anggono and Huganir, 2012). Many forms of synaptic plasticity engage one or more of these mechanisms to precisely control the number and function of AMPARs at the synapse.
Much progress has been made in identifying proteins that interact with the intracellular domain of AMPARs and the mechanisms by which they regulate clustering and trafficking (Diering and Huganir, 2018). However, these processes can also be regulated by extracellular, secreted factors that impact synaptic strength and plasticity (Allen and Eroglu, 2017; Yuzaki, 2018). One class of secreted factors directly binds to the extracellular N-terminus of AMPARs and clusters receptors at synapses (i.e., Neuronal Pentraxins, Noelins), whereas another class are diffusible ligands that control AMPAR trafficking indirectly via their cognate signaling receptors (i.e., Netrin-1, BDNF, VEGF, TNFα) (Figure 1). Some of these diffusible factors are secreted from neurons, whereas others are secreted from non-neuronal cells such as glia and, intriguingly, from distal tissues such as muscle. In this mini-review, we discuss what is known about secreted proteins that regulate AMPAR trafficking and highlight some open questions that remain to be addressed.
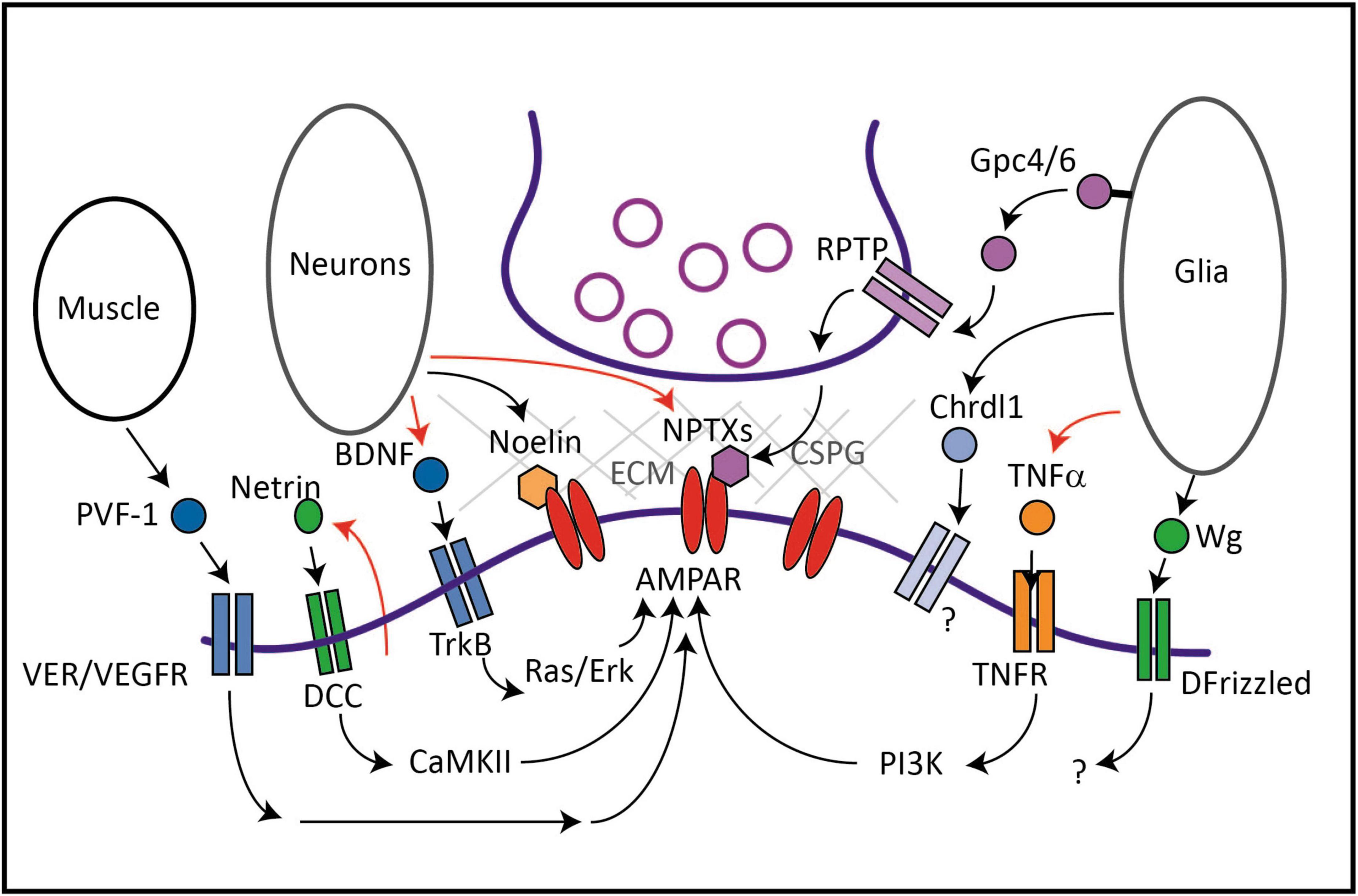
Figure 1. Protein factors secreted from neurons, glia or muscle regulate AMPAR trafficking and synaptic levels. Netrin is released in an activity-dependent manner from neurons and results in an autocrine activation of its receptor DCC. Downstream activation of CaMKII leads to increased delivery of GluA1-containing AMPARs to synapses. BDNF is also secreted from neurons in an activity-dependent manner and activates the tyrosine kinase receptor TrkB and downstream Ras and Erk signaling to increase AMPAR transcription, translation and synaptic incorporation. BDNF can also be secreted from glia (not shown). Noelin and NPTX are secreted from neurons, and act as extracellular scaffolds by interacting with the N-terminal domain of AMPARs and a network of proteins including ECM components. Glia such as astrocytes secrete Gpc4/6 which acts on presynaptic RPTPs leading to the release of NPTX. Chrdl1 acts via an unidentified receptor and signaling pathway to promote synapse maturation during development by inducing the switch to calcium-impermeable GluA2-containing AMPARs. TNFα is secreted from glia in an activity-dependent manner and activates the TNFR and downstream PI3 kinase to upregulate synaptic AMPARs during homeostatic synaptic scaling. In Drosophila, Wg is released from specialized glia that regulate glutamate receptor cluster size and synaptic localization via its receptor DFrizzled. In C. elegans, PVF-1/VEGF secreted by muscle promotes surface levels of AMPARs in distal neurons via its receptor VER-1 and VER-4. PVF-1 and the VERs are thought to promote surface levels of AMPARs via long-distance recycling via retromer. In mammals, VEGF also promotes AMPAR levels at synapses and can be secreted from many cells including neurons, glia and muscle, although it is not known if muscle-derived VEGF regulates AMPARs in mammals. Red arrows represent activity-dependent regulation of the secreted factor.
Factors secreted from neurons
Neuronal pentraxins (NPTXs)
The neuronal pentraxin family consists of two secreted glycoproteins, NPTX1/NP1 and NPTX2/NP2/NARP, and the NPTX receptor (NPTXR). NPTX1 and NPTX2 cluster AMPARs at excitatory synapses onto inhibitory neurons, interacting directly with the N-terminus of AMPARs or indirectly via NPTXR (Table 1). Secreted NPTX1 interacts with NPTXR and GluA4 at shaft synapses resulting in the clustering of NPTX1 and GluA4-containing AMPARs, which are highly expressed on parvalbumin-positive (PV +) interneurons in the forebrain (Sia et al., 2007; Pelkey et al., 2015). The selective effect of NPTXs on these interneurons can be attributed to their almost exclusive interaction with GluA4 subunits (Sia et al., 2007; Pelkey et al., 2015). NPTX2 is an immediate early gene whose expression is correlated with neuronal activity (O’Brien et al., 1999; Xu et al., 2003; Chang et al., 2010) and mediates homeostatic synaptic scaling (Chang et al., 2010). NPTX2 is released from dense-core vesicles (DCVs) near glutamatergic synapses, multimerizes, and becomes trapped in the Extra-Cellular Matrix (ECM)-containing perineuronal nets that surround PV + inhibitory neuron cell bodies (O’Brien et al., 1999; Reti et al., 2008; Chang et al., 2010). Thus, NPTXs act as extracellular scaffolds that interact with and cluster AMPARs and mediate an activity-dependent mechanism to recruit inhibitory neurons into circuits to dampen excitation. Interestingly, NPTX2 can be detected in the circulatory system (Reti et al., 2008), suggesting it may have other functions and act far beyond its sites of release.
Netrin-1
Netrin-1 was discovered as a regulator of neurodevelopmental processes including cell migration, axon guidance, and synapse formation in invertebrates and vertebrates (Ishii et al., 1992; Kennedy et al., 1994; Colón-Ramos et al., 2007). More recently, Netrin-1 was shown to promote excitatory synapse formation (Goldman et al., 2013) and activity-dependent insertion of AMPARs into synapses (Glasgow et al., 2018). NMDAR-mediated Ca2+ influx stimulates the release of Netrin-1 from hippocampal dendrites, resulting in autocrine activation of the Netrin receptor Deleted in Colorectal Cancer (DCC), activation of Calcium/Calmodulin Kinase II (CaMKII) and increased surface abundance of GluA1-containing AMPARs (Glasgow et al., 2018, 2020). Netrin-1 released from dopaminergic and GABAergic neurons in the ventral tegmental area similarly acts in an autocrine manner to promote AMPAR-mediated currents (Cline et al., 2023). The Netrin co-receptor Down Syndrome Cell Adhesion Molecule (DSCAM) also promotes AMPAR levels and clustering in cultured Aplysia neurons (Li et al., 2009). Additionally, long-term potentiation (LTP) occludes Netrin-1-induced potentiation of AMPAR EPSCs, and vice versa, indicating that activity-dependent secretion of Netrin-1 is a mechanism of LTP (Glasgow et al., 2018). Conditional deletion of Netrin or DCC from excitatory neurons in the hippocampus results in defects in spatial memory in rodents (Horn et al., 2013; Wong et al., 2019), and shRNA-mediated knockdown of Netrin-1 from spinal dorsal horn neurons impairs pain sensation and decreases the membrane fraction of GluA1-containing AMPARs (Cui et al., 2021). Thus, Netrin is secreted in an activity-dependent manner from dendrites of many cell types and promotes synaptic AMPAR retention, providing an example of a secreted factor being used in an autocrine fashion to strengthen glutamatergic signaling in response to postsynaptic activity. It remains to be seen whether the effects of Netrin-1 are restricted to stimulated synapses or if secreted Netrin-1 can diffuse further away to reach neighboring unstimulated synapses.
Noelin
Noelin-1/Olfactomedin-1 is a glycoprotein secreted from neurons in the cortex, cerebellum and hippocampus. Noelin-1 and the related Noelin-2 regulate AMPAR trafficking and function in zebrafish and rodents (Sultana et al., 2014; Nakaya et al., 2017). Noelins are conserved in all vertebrates but do not appear to have an obvious homolog in invertebrates (Boudkkazi et al., 2023). Noelin-1 was originally isolated as an AMPAR-interacting protein from rodent synaptosomes (von Engelhardt et al., 2010; Schwenk et al., 2012). Noelin-1 interacts with GluA1 and GluA2 in heterologous cells and, similar to NPTXs, binds directly to the N-terminal domain of GluA2 (Pandya et al., 2018), resulting in the stabilization of synaptic AMPARs (Díaz-Alonso et al., 2017). Interestingly, the effects of Noelin-1 are modified by the presence of the ECM because Noelin-1 reduces AMPAR mobility on young neurons but not on mature neurons which are surrounded by ECM. Noelin-1 may also link AMPARs with Chondroitin sulfate proteoglycans (CSPGs) in the ECM via Nogo, a Noelin-1 receptor (Frischknecht et al., 2009). A recent study shows that Noelins 1-3 act as polyvalent extracellular scaffolds that stabilize AMPARs by anchoring them to a network of secreted and transmembrane proteins (Boudkkazi et al., 2023). Using high-resolution immuno-EM and electrophysiology recordings, neurons lacking Noelins 1-3 were observed to have 40 and 70% reductions in AMPARs at synapses on excitatory and inhibitory neurons, respectively, and defects in hippocampal LTP. This study suggests that secreted factors like Noelins and their extracellular protein networks are major contributors to LTP (Boudkkazi et al., 2023). Interestingly, chemical LTP increases Noelin-1 levels in the synapse suggesting that activity may increase Noelin-1 secretion to stabilize synaptic AMPARs during plasticity (Pandya et al., 2018).
Brain-derived neurotrophic factor (BDNF)
Brain-derived neurotrophic factor, a member of the neurotrophin family, signals through its tyrosine-kinase receptor, tropomyosin-related Kinase B (TrkB) to regulate several processes including neurogenesis, neuronal survival, axon outgrowth, and synapse formation (Messaoudi et al., 1998; Lee and Hempstead, 2018). LTP stimulates BDNF transcription via the transcription factor CREB (Castrén et al., 1993; Dragunow et al., 1993; Tao et al., 1998, 2002; Hong et al., 2008) and promotes BDNF secretion (Balkowiec and Katz, 2000; Aicardi et al., 2004). Conversely, application of mature BDNF induces LTP at hippocampal synapses (Kang and Schuman, 1995a,b). These early studies suggested that BDNF could regulate AMPAR expression or trafficking during LTP. Indeed, BDNF/TrkB signaling increases transcription and protein expression of GluA1, GluA2, and GluA3, and AMPAR colocalization with PSD-95 in dendrites (Jourdi et al., 2003; Caldeira et al., 2007; Nakata and Nakamura, 2007). Of all secreted factors, the intracellular signaling pathways that mediate the effects of BDNF on synaptic AMPAR levels are probably the best understood. Specifically, BDNF/TrkB complexes activate the Ras/ERK pathway that is involved in surface delivery of AMPARs (Li and Keifer, 2008, 2009; Li and Wolf, 2011; Reimers et al., 2014) and phosphorylates S831 on GluA1 subunits, a critical site for CaMKII and protein kinase C (PKC) binding, promoting AMPAR synaptic delivery and retention (Caldeira et al., 2007; Keifer, 2022). Thus, BDNF is a secreted factor whose expression and secretion are regulated by activity that promotes AMPAR levels at synapses via multiple intracellular mechanisms.
Factors secreted from glia
Thrombospondin-1
The large glycoprotein Thrombospondin-1 is secreted by astrocytes and was originally shown to induce synapse formation during development, although these synapses initially lack AMPARs and are silent (Christopherson et al., 2005). Thrombospondin-1 also functions in the mature nervous system to reduce synaptic transmission: treatment of cultured rat spinal cord neurons with Thrombospondin-1 increases lateral diffusion and endocytosis of AMPARs and increases levels of inhibitory glycine receptors at synapses (Hennekinne et al., 2013). Thus, Thrombospondin-1 is a glia-secreted factor that regulates synapse development and AMPAR trafficking in mature neurons.
Secreted protein acidic and rich in cysteines (SPARC)
Astrocytes and microglia secrete the protein SPARC which antagonizes synapse formation induced by hevin, another astrocyte-secreted factor (Jones et al., 2011; Kucukdereli et al., 2011). Consistent with a role for SPARC in negatively regulating excitatory transmission, hippocampal neurons from SPARC knock-out mice exhibit increased mESPC amplitude and frequency. Moreover, when cultured with SPARC knock-out astrocytes, hippocampal neurons exhibit increased surface levels of GluA1 and GluA2. Intriguingly, secretion of SPARC from astrocytes is dependent on synaptic activity, suggesting a model whereby increased synaptic activity leads to SPARC release and downregulation of AMPAR surface levels and signaling (Jones et al., 2011). These studies indicate that SPARC is an activity-regulated secreted factor that inhibits glutamatergic signaling by reducing surface levels of AMPARs at synapses, although how SPARC controls intracellular signaling pathways in neurons to impact AMPAR trafficking remains to be determined.
Chondroitin-sulfate proteoglycans (CSPGs)
Astrocytes contribute to the ECM surrounding neurons by secreting proteoglycans such as CSPG. Frischknecht et al. (2009) showed that digestion of the ECM backbone with hyaluronidase results in increased AMPAR mobility in the membrane and increased short-term plasticity (STP), suggesting that the ECM restricts exchange of desensitized synaptic AMPARs with naïve extrasynaptic AMPARs during STP (Frischknecht et al., 2009). Since CSPG levels increase during postnatal development, they may contribute to synapse maturation by limiting plasticity (Pizzorusso et al., 2002). Consistent with this, digestion of ECM increased synapse number in a hippocampal/astrocyte co-culture system and electrophysiological recordings indicated that the synapses were immature (Pyka et al., 2011). Thus, components of the ECM like CSPG and its interacting proteins are required to stabilize synaptic AMPARs and promote synapse maturation. It will be interesting to determine how CSPG expression or secretion from astrocytes is regulated to control synaptic AMPARs and maturation.
Glypicans (Gpcs)
Glypicans are conserved heparan sulfate proteoglycans (HSPGs) comprising 6 family members in mammals that are anchored to the plasma membrane via glycosylphosphatidylinositol links. Allen et al. (2012) biochemically isolated Gpc4 and Gpc6 from astrocyte-conditioned media as factors that induce functional retinal ganglion cell (RGC) synapses. Both Gpc4 and Gpc6 promote cell surface clustering of GluA1-containing AMPARs and increased excitatory transmission (Allen et al., 2012). Knock-out of Gpc4 in mice decreases GluA1 and reduces excitatory transmission in the developing hippocampus. The authors proposed that glypicans induce immature synapses containing GluA1 which then subsequently mature by incorporating GluA2/3 and GluA4 subunit-containing AMPARs. Although glypicans can be proteolytically cleaved and released from the plasma membrane, it is not clear how astrocytes regulate the release of glypicans.
Other studies have revealed the mechanism by which Gpc4 regulates AMPARs. Mammalian glypicans and the Drosophila glypican homolog Dally like interacts with Leukocyte common antigen-related (LAR) receptor tyrosine phosphatases (RPTPs) family members (Johnson et al., 2006; Takahashi and Craig, 2013; Ko et al., 2015) and postsynaptic Leucine-Rich Repeat TM4 (LRRTM4) (de Wit et al., 2013; Siddiqui et al., 2013). These interactors promote excitatory synapse formation and function in mice (Dunah et al., 2005; Linhoff et al., 2009; Siddiqui et al., 2013; Soler-Llavina et al., 2013) by increasing surface GluA1-containing AMPARs (Schwenk et al., 2012; Shanks et al., 2012; Siddiqui et al., 2013). Additionally, astrocyte-secreted Gpc4 stimulates release of NPTX1 to promote AMPAR clustering at synapses (see above). Disruption of the NPTX1-AMPAR interaction with antibodies blocks Gpc4-mediated synapse formation, and loss of Gpc4 or LAR family member RPTPδ results in accumulation of NPTX1 in presynaptic terminals, reduced GluA1 clusters and decreased synapse number (Farhy-Tselnicker et al., 2017). These data suggest a model whereby astrocyte-released Gpc4 interacts with presynaptic RPTPδ resulting in secretion of NPTX1 from neurons and postsynaptic AMPAR clustering.
Chordin-like-1 (Chrdl1)
Excitatory synapse maturation is marked by a switch from calcium-permeable GluA1-containing to calcium-impermeable GluA2-containing AMPARs. Blanco-Suarez et al. (2018) identified Chordin-like 1 (Chrdl1) as an astrocyte-secreted factor that promotes synapse maturation by increasing surface levels of GluA2-containing AMPARs at synapses on rat RGCs, thus inducing the switch from calcium-permeable to calcium-impermeable AMPARs (Blanco-Suarez et al., 2018). Interestingly, Chrdl1 knock-out mice exhibit a shift toward immature synapses which is associated with increased plasticity in the visual cortex. The authors propose a model where Gpcs act first to promote clustering of GluA1-containing AMPARs at immature synapses followed by Chrdl-1 which promotes the switch to GluA2-containing AMPARs typically associated with mature synapses. Consistent with this model, astrocyte expression of Gpc4 and Gpc6 is highest in the first two postnatal weeks when immature synapses are developing while Chrdl1 peaks in the cortex later at the time of synapse maturation in mice (Cahoy et al., 2008; Allen et al., 2012). It remains to be determined how Chrdl1 secretion is regulated and how Chrdl1 regulates intracellular trafficking to promote GluA2-containing AMPARs. Given that CSPGs also promote synapse maturation, it will be informative to investigate how Chrdl-1 and CSPG interact to regulate maturation.
Tumor necrosis factor α (TNF-α)
The proinflammatory cytokine TNFα is secreted from astrocytes or microglia and promotes surface levels of AMPARs in hippocampal neurons in vitro and in vivo (Beattie et al., 2002). TNFα regulation of AMPARs is not involved in hippocampal LTP or LTD, but is required for homeostatic synaptic scaling (Stellwagen and Malenka, 2006) in which chronic changes in synaptic activity result in a global adjustment of synaptic strength by altering AMPAR levels (O’Brien et al., 1998; Turrigiano et al., 1998). Synaptic scaling up is blocked if TNFα is scavenged by soluble TNFR1 or if neurons are co-cultured with TNFα knock out glia (Stellwagen and Malenka, 2006). Mechanistically, TNFα increases the exocytosis of GluA2-lacking AMPARs in hippocampal and cortical neurons (Ogoshi et al., 2005; Stellwagen and Malenka, 2006; He et al., 2012) via PI3 kinase (Stellwagen and Malenka, 2006) while simultaneously promoting the internalization of GABA receptors (Stellwagen et al., 2005). The effects of TNFα are brain region-specific. TNFα has the opposite effect on AMPARs in the mouse dorsolateral striatum where it promotes internalization of GluA1-containing AMPARs from medium spiny neurons and reduces corticostriatal synaptic strength (Lewitus et al., 2014). In the striatum, TNFα treatment activates protein phosphatase 1 (PP1) via DARPP-32 which promotes AMPAR endocytosis by increasing the dephosphorylation of GluA1 on S845 and S831.
Wingless (Wg)/Wnt
Wingless (Wg)/Wnt is secreted from neurons and glia and regulates pre- and postsynaptic development via the receptor Frizzled at the glutamatergic Drosophila neuromuscular junction (NMJ) (Packard et al., 2002; Mathew et al., 2005; Miech et al., 2008). Loss of Wg results in decreased synapse numbers and abnormal glutamate receptor (GluR) distribution (Packard et al., 2002). Interestingly, Wg is secreted from glia in addition to motor neurons at the Drosophila NMJ. Loss of Wg in neurons or subperineurial glia results in increased GluR cluster size and intensity, however, these synapses are correlated with reduced quantal content. Thus, Wg secreted from both glia and neurons is required for the normal distribution and function of GluRs. Interestingly, the source of Wg matters because Wg secreted from motor neurons, but not glia, regulates NMJ size, suggesting that neuron-derived Wg regulates NMJ structure. Although it is not clear how the Wg receptor Frizzled signals to regulate GluR trafficking, vertebrate Wnt7a promotes synapse formation in hippocampal neurons via postsynaptic CaMKII (Ciani et al., 2011) which is known to promote synaptic AMPAR levels during plasticity (Hayashi et al., 2000).
Factors secreted from other tissues
Vascular endothelial growth factor (VEGF)
A recent study in C. elegans showed that the VEGF homolog PVF-1 is secreted from muscle and regulates surface levels of the calcium-permeable AMPAR GLR-1 in upstream neurons (Luth et al., 2021). Interestingly, PVF-1/VEGF is expressed and released from muscle to mediate GLR-1 surface levels in pre-motor interneurons that reside two synaptic layers upstream of the neuromuscular junction. Loss of the VEGF Receptor (VEGFR) homologs VER-1 and VER-4, or PVF-1 reduces GLR-1 cell surface levels, resulting in impaired glutamate-dependent locomotor behaviors (Luth et al., 2021). Local recycling of GLR-1 to the plasma membrane is notably unchanged in VER mutants, consistent with the idea that VEGFR signaling in C. elegans promotes long-range AMPA receptor trafficking, perhaps via retromer (Luth et al., 2021).
In vertebrates, VEGF is expressed in vascular endothelial cells throughout the body including in the brain and spinal cord (Licht et al., 2011), but also in neurons (Ogunshola et al., 2002; Schiera et al., 2007), microglia (Bartholdi et al., 1997) and astrocytes (Ijichi et al., 1995). VEGF is also expressed in multiple muscle cell-types such as cardiac myocytes (Levy et al., 1995), vascular smooth muscle (Ishida et al., 2001) and skeletal muscle (Germani et al., 2003). VEGF expression can be induced by ischemia and, in the case of skeletal muscle, is known to both promote angiogenesis and act in an autocrine manner to regulate myoblast function to promote new muscle growth (Germani et al., 2003). Interestingly, VEGFR family members are expressed in neurons and glia and can be induced in astrocytes and microglia following injury (Ogunshola et al., 2002; Choi et al., 2007a,b).
Several studies indicate that VEGF regulates glutamatergic transmission in vertebrates (Cammalleri et al., 2011; Licht et al., 2011; De Rossi et al., 2016). Inhibiting or stimulating VEGF signaling in adult mice has respective effects on LTP in the hippocampus and glutamate-dependent fear conditioning (Licht et al., 2011). VEGFRs localize to the postsynaptic density of mouse hippocampal neurons, and although VEGF alone failed to increase AMPA receptor trafficking or LTP (Cammalleri et al., 2011; De Rossi et al., 2016), co-administration of VEGF and NMDA activated PKC and CaMKII, increased the delivery of calcium-permeable GluA1-containing AMPARs to synapses, and increased excitatory synapse number. Conversely, conditional knockout of VEGFR2 in neurons impaired LTP and fear-related memory consolidation, without altering basal transmission (De Rossi et al., 2016). Collectively, these findings suggest that in the hippocampus, VEGFR2 signaling may promote activity-dependent strengthening of glutamatergic transmission and resultant behavior by promoting the synaptic incorporation of AMPARs. Together these studies indicate that VEGF regulates AMPAR levels and trafficking in vertebrates and invertebrates, however, the source of VEGF in vertebrates is not clear. Given that PVF-1/VEGF is secreted from muscle to regulate AMPARs in C. elegans, it is tempting to speculate that muscle-secreted VEGF may, under some circumstances, also act at a distance to regulate AMPARs in the brain in vertebrates.
Fibronectin type III domain-containing protein 5 (FNDC5)/Irisin
Muscle activity during exercise has beneficial effects on energy metabolism and brain function (Cotman et al., 2007; Mattson, 2012). Interestingly, previous studies identified irisin as a muscle-released factor that signals to the brain. FNDC5 is a transmembrane protein expressed in the brain and skeletal muscle that is cleaved and released as a peptide called irisin during exercise. Irisin enters the circulation and directs fat metabolism and thermogenesis and also mediates many of the beneficial effects of exercise on cognition (Boström et al., 2012). Peripheral injection of FNDC5 or irisin increases irisin protein levels in the hippocampus without altering irisin mRNA levels in the brain (Wrann et al., 2013; Lourenco et al., 2019; Islam et al., 2021), suggesting that irisin can cross the blood-brain barrier. Once in the brain, irisin induces the expression of BDNF in the hippocampus (Wrann et al., 2013), which as discussed above, can regulate AMPAR expression and trafficking. Interestingly, BDNF expression is increased after exercise in the hippocampus (Vaynman et al., 2004; El Hayek et al., 2019; Lourenco et al., 2019) and also in skeletal muscle (Cuppini et al., 2007; Szuhany et al., 2015) and can be released into the circulatory system (Pan et al., 1998; Matthews et al., 2009).
Discussion
This mini-review highlights secreted protein factors that regulate trafficking or clustering of AMPAR subunits and discusses their effects on glutamatergic transmission and plasticity (Table 1). Several factors are secreted in response to activity either from neurons or glia and are thus ideal for coordinating synapse development or plasticity in an activity-dependent manner. Regulation of the expression and release of secreted factors potentially provides a multitude of spatio-temporal mechanisms to control neuronal development and function. Depending on their range of action, secreted factors could act locally to coordinate subsets of synapses within a neuron or more globally to coordinate groups of glia or neurons in a circuit or region of the brain. Longer range factors may act more distally in another region of the brain and still signal to a restricted subset of neurons that express their specific cognate receptor, enabling precise long-distance signaling. However, since most studies described in this review typically focus on one neuronal cell type or one region of the brain, their full complement of target cells within the brain are not known.
We also describe a role for PVF-1/VEGF as a muscle-secreted factor in C. elegans that regulates AMPAR levels in a distal neuron that coordinates locomotion. Although VEGF is also expressed and released from muscle in vertebrates, it’s role in signaling to the brain has not been investigated. However, the fact that muscle cells release hundreds of factors, many of which are stimulated by contraction that may mediate the beneficial effects of exercise on metabolism and brain function (Görgens et al., 2015) and that studies in Drosophila and mammals have revealed hundreds of secreted proteins that may mediate inter-organ communication including between peripheral tissues and the brain (Droujinine and Perrimon, 2016; Severinsen and Pedersen, 2020), raises the intriguing possibility that there may be other factors secreted from peripheral tissues that regulate glutamatergic synapses in the brain.
Many open questions remain. Although neuronal activity can regulate the release of some of these factors, we do not understand how they are packaged into vesicles, the mechanisms that regulate their secretion, or how their range of action might be modulated. Similarly, although we have some idea how a few of these factors regulate AMPAR trafficking, for the vast majority, the molecular mechanisms by which they impinge on intracellular trafficking pathways (lateral diffusion, clustering, insertion, removal, degradation, transport, etc.) to control synaptic AMPAR levels are unknown. It will also be interesting to determine when these factors are expressed and released either during development or in the mature brain and if they are expressed in a region-specific manner. Finally, although this review largely focused on the secretion of soluble factors, there are many membrane-associated or transmembrane proteins, like FNDC5 and glypicans that undergo cleavage to release extracellular domains (Tien et al., 2017) that may have signaling or regulatory functions that remain to be discovered. It will not be surprising if more extracellular factors that regulate AMPAR trafficking and glutamatergic synapses will be discovered that are secreted from both neuronal and non-neuronal cell types in the brain, and perhaps also from peripheral tissues.
Author contributions
BR: Writing – original draft, Writing – review and editing. EL: Writing – original draft, Writing – review and editing. SM: Writing – original draft, Writing – review and editing. PJ: Conceptualization, Writing – original draft, Writing – review and editing.
Funding
The author(s) declare financial support was received for the research, authorship, and/or publication of this article. This research was funded in part by a National Science Foundation Award (IOS#1941073) and a Tufts University Springboard Award.
Acknowledgments
We apologize for not being able to cite all the relevant primary research articles due to space restrictions.
Conflict of interest
The authors declare that the research was conducted in the absence of any commercial or financial relationships that could be construed as a potential conflict of interest.
Publisher’s note
All claims expressed in this article are solely those of the authors and do not necessarily represent those of their affiliated organizations, or those of the publisher, the editors and the reviewers. Any product that may be evaluated in this article, or claim that may be made by its manufacturer, is not guaranteed or endorsed by the publisher.
References
Aicardi, G., Argilli, E., Cappello, S., Santi, S., Riccio, M., Thoenen, H., et al. (2004). Induction of long-term potentiation and depression is reflected by corresponding changes in secretion of endogenous brain-derived neurotrophic factor. Proc. Natl. Acad. Sci. U. S. A. 101, 15788–15792. doi: 10.1073/pnas.0406960101
Allen, N. J., Bennett, M. L., Foo, L. C., Wang, G. X., Chakraborty, C., Smith, S. J., et al. (2012). Astrocyte glypicans 4 and 6 promote formation of excitatory synapses via GluA1 AMPA receptors. Nature 486, 410–414. doi: 10.1038/nature11059
Allen, N. J., and Eroglu, C. (2017). Cell Biology of Astrocyte-Synapse Interactions. Neuron 96, 697–708. doi: 10.1016/j.neuron.2017.09.056
Anggono, V., and Huganir, R. (2012). Regulation of AMPA receptor trafficking and synaptic plasticity. Curr. Opin. Neurobiol. 22, 461–469. doi: 10.1016/j.conb.2011.12.006
Bae, J. J., Xiang, Y. Y., Martinez-Canabal, A., Frankland, P. W., Yang, B. B., and Lu, W.Y. (2011). Increased transforming growth factor-β1 modulates glutamate receptor expression in the hippocampus. Int. J. Physiol. Pathophysiol. Pharmacol. 3, 9–20.
Balkowiec, A., and Katz, D. (2000). Activity-dependent release of endogenous brain-derived neurotrophic factor from primary sensory neurons detected by ELISA in situ. J. Neurosci. 20, 7417–7423. doi: 10.1523/JNEUROSCI.20-19-07417.2000
Bartholdi, D., Rubin, B. P., and Schwab, M. E. (1997). VEGF mRNA induction correlates with changes in the vascular architecture upon spinal cord damage in the rat. Eur. J. Neurosci. 9, 2549–2560. doi: 10.1111/j.1460-9568.1997.tb01684.x
Beattie, E. C., Stellwagen, D., Morishita, W., Bresnahan, J. C., Ha, B. K., Von Zastrow, M., et al. (2002). Control of synaptic strength by glial TNFalpha. Science 295, 2282–2285. doi: 10.1126/science.1067859
Blanco-Suarez, E., Liu, T. F., Kopelevich, A., and Allen, N. (2018). Astrocyte-secreted chordin-like 1 drives synapse maturation and limits plasticity by increasing synaptic GluA2 AMPA Receptors. Neuron 100, 1116–1132.e1.
Boström, P., Wu, J., Jedrychowski, M. P., Korde, A., Ye, L., Lo, J. C., et al. (2012). A PGC1-α-dependent myokine that drives brown-fat-like development of white fat and thermogenesis. Nature 481, 463–468. doi: 10.1038/nature10777
Boudkkazi, S., Schwenk, J., Nakaya, N., Brechet, A., Kollewe, A., Harada, H., et al. (2023). A Noelin-organized extracellular network of proteins required for constitutive and context-dependent anchoring of AMPA-receptors. Neuron 111, 2544–2556.e1.
Cahoy, J. D., Emery, B., Kaushal, A., Foo, L. C., Zamanian, J. L., Christopherson, K. S., et al. (2008). A transcriptome database for astrocytes, neurons, and oligodendrocytes: a new resource for understanding brain development and function. J. Neurosci. 28, 264–278. doi: 10.1523/JNEUROSCI.4178-07.2008
Caldeira, M. V., Melo, C. V., Pereira, D. B., Carvalho, R., Correia, S. S., Backos, D. S., et al. (2007). Brain-derived neurotrophic factor regulates the expression and synaptic delivery of alpha-amino-3-hydroxy-5-methyl-4-isoxazole propionic acid receptor subunits in hippocampal neurons. J. Biol. Chem. 282, 12619–12628. doi: 10.1074/jbc.M700607200
Cammalleri, M., Martini, D., Ristori, C., Timperio, A. M., and Bagnoli, P. (2011). Vascular endothelial growth factor up-regulation in the mouse hippocampus and its role in the control of epileptiform activity. Eur. J. Neurosci. 33, 482–498. doi: 10.1111/j.1460-9568.2010.07529.x
Castrén, E., Pitkänen, M., Sirviö, J., Parsadanian, A., Lindholm, D., Thoenen, H., et al. (1993). The induction of LTP increases BDNF and NGF mRNA but decreases NT-3 mRNA in the dentate gyrus. Neuroreport 4, 895–898. doi: 10.1097/00001756-199307000-00014
Chang, M. C., Park, J. M., Pelkey, K. A., Grabenstatter, H. L., Xu, D., Linden, D. J., et al. (2010). Narp regulates homeostatic scaling of excitatory synapses on parvalbumin-expressing interneurons. Nat. Neurosci. 13, 1090–1097. doi: 10.1038/nn.2621
Choi, J. S., Kim, H. Y., Cha, J. H., Choi, J. Y., Chun, M. H., and Lee, M. (2007a). Upregulation of vascular endothelial growth factor receptors Flt-1 and Flk-1 in rat hippocampus after transient forebrain ischemia. J. Neurotrauma 24, 521–531. doi: 10.1089/neu.2006.0139
Choi, J. S., Kim, H. Y., Cha, J. H., Choi, J. Y., Park, S. I., Jeong, C., et al. (2007b). Upregulation of vascular endothelial growth factor receptors Flt-1 and Flk-1 following acute spinal cord contusion in rats. J. Histochem. Cytochem. 55, 821–830. doi: 10.1369/jhc.6A7139.2007
Christopherson, K. S., Ullian, E. M., Stokes, C. C., Mullowney, C. E., Hell, J., Agah, A., et al. (2005). Thrombospondins are astrocyte-secreted proteins that promote CNS synaptogenesis. Cell 120, 421–433. doi: 10.1016/j.cell.2004.12.020
Ciani, L., Boyle, K. A., Dickins, E., Sahores, M., Anane, D., Lopes, D. M., et al. (2011). Wnt7a signaling promotes dendritic spine growth and synaptic strength through Ca2 +/Calmodulin-dependent protein kinase II. Proc. Natl. Acad. Sci. U. S. A. 108, 10732–10737. doi: 10.1073/pnas.1018132108
Cline, M. M., Juarez, B., Hunker, A., Regiarto, E. G., Hariadi, B., Soden, M. E., et al. (2023). Netrin-1 regulates the balance of synaptic glutamate signaling in the adult ventral tegmental area. Elife 12, e83760. doi: 10.7554/eLife.83760
Colón-Ramos, D. A., Margeta, M. A., and Shen, K. (2007). Glia promote local synaptogenesis through UNC-6 (netrin) signaling in C. elegans. Science 318, 103–106. doi: 10.1126/science.1143762
Cotman, C. W., Berchtold, N. C., and Christie, L. (2007). Exercise builds brain health: key roles of growth factor cascades and inflammation. Trends Neurosci. 30, 464–472. doi: 10.1016/j.tins.2007.06.011
Cui, W., Li, Y., Wang, Z., Song, C., Yu, Y., Wang, G., et al. (2021). Spinal caspase-6 regulates AMPA receptor trafficking and dendritic spine plasticity through netrin-1 in postoperative pain after orthopedic surgery for tibial fracture in mice. Pain 162, 124–134. doi: 10.1097/j.pain.0000000000002021
Cuppini, R., Sartini, S., Agostini, D., Guescini, M., Ambrogini, P., Betti, M., et al. (2007). Bdnf expression in rat skeletal muscle after acute or repeated exercise. Arch. Ital. Biol. 145, 99–110.
De Rossi, P., Harde, E., Dupuis, J. P., Martin, L., Chounlamountri, N., Bardin, M., et al. (2016). A critical role for VEGF and VEGFR2 in NMDA receptor synaptic function and fear-related behavior. Mol. Psychiatry 21, 1768–1780. doi: 10.1038/mp.2015.195
de Wit, J., O’Sullivan, M. L., Savas, J. N., Condomitti, G., Caccese, M. C., Vennekens, K. M., et al. (2013). Unbiased discovery of glypican as a receptor for LRRTM4 in regulating excitatory synapse development. Neuron 79, 696–711. doi: 10.1016/j.neuron.2013.06.049
Díaz-Alonso, J., Sun, Y. J., Granger, A. J., Levy, J. M., Blankenship, S. M., and Nicoll, R. (2017). Subunit-specific role for the amino-terminal domain of AMPA receptors in synaptic targeting. Proc. Natl. Acad. Sci. U. S. A. 114, 7136–7141. doi: 10.1073/pnas.1707472114
Diering, G. H., and Huganir, R. (2018). The AMPA Receptor Code of Synaptic Plasticity. Neuron 100, 314–329. doi: 10.1016/j.neuron.2018.10.018
Ding, Q., Vaynman, S., Akhavan, M., Ying, Z., and Gomez-Pinilla, F. (2006). Insulin-like growth factor I interfaces with brain-derived neurotrophic factor-mediated synaptic plasticity to modulate aspects of exercise-induced cognitive function. Neuroscience 140, 823–833.
Dragunow, M., Beilharz, E., Mason, B., Lawlor, P., Abraham, W., and Gluckman, P. (1993). Brain-derived neurotrophic factor expression after long-term potentiation. Neurosci. Lett. 160, 232–236. doi: 10.1016/0304-3940(93)90420-p
Droujinine, I. A., and Perrimon, N. (2016). Interorgan communication pathways in physiology: Focus on Drosophila. Annu. Rev. Genet. 50, 539–570. doi: 10.1146/annurev-genet-121415-122024
Dunah, A. W., Hueske, E., Wyszynski, M., Hoogenraad, C. C., Jaworski, J., Pak, D. T., et al. (2005). LAR receptor protein tyrosine phosphatases in the development and maintenance of excitatory synapses. Nat. Neurosci. 8, 458–467. doi: 10.1038/nn1416
El Hayek, L., Khalifeh, M., Zibara, V., Abi Assaad, R., Emmanuel, N., Karnib, N., et al. (2019). Lactate mediates the effects of exercise on learning and memory through SIRT1-dependent activation of hippocampal brain-derived neurotrophic factor (BDNF). J. Neurosci. 39, 2369–2382. doi: 10.1523/JNEUROSCI.1661-18.2019
Farhy-Tselnicker, I., van Casteren, A. C., Lee, A., Chang, V. T., Aricescu, A. R., and Allen, N. (2017). Astrocyte-secreted glypican 4 regulates release of neuronal pentraxin 1 from axons to induce functional synapse formation. Neuron 96, 428–445.e2.
Frischknecht, R., Heine, M., Perrais, D., Seidenbecher, C. I., Choquet, D., and Gundelfinger, E. (2009). Brain extracellular matrix affects AMPA receptor lateral mobility and short-term synaptic plasticity. Nat. Neurosci. 12, 897–904. doi: 10.1038/nn.2338
Fukata, Y., Adesnik, H., Iwanaga, T., Bredt, D. S., Nicoll, R. A., and Fukata, M. (2006). Epilepsy-related ligand/receptor complex LGI1 and ADAM22 regulate synaptic transmission. Science 313, 1792–1795.
Fukata, Y., Lovero, K. L., Iwanaga, T., Watanabe, A., Yokoi, N., Tabuchi, K. R., et al. (2010). Disruption of LGI1-linked synaptic complex causes abnormal synaptic transmission and epilepsy. Proc. Natl. Acad. Sci. U.S.A. 107, 3799–3804.
Germani, A., Di Carlo, A., Mangoni, A., Straino, S., Giacinti, C., Turrini, P., et al. (2003). Vascular endothelial growth factor modulates skeletal myoblast function. Am. J. Pathol. 163, 1417–1428. doi: 10.1016/S0002-9440(10)63499-2
Glasgow, S. D., Labrecque, S., Beamish, I. V., Aufmkolk, S., Gibon, J., Han, D., et al. (2018). Activity-dependent netrin-1 secretion drives synaptic insertion of GluA1-containing AMPA receptors in the hippocampus. Cell. Rep. 25, 168–182.e3.
Glasgow, S. D., Wong, E. W., Thompson-Steckel, G., Marcal, N., Séguéla, P., Ruthazer, E. S., et al. (2020). Pre- and post-synaptic roles for DCC in memory consolidation in the adult mouse hippocampus. Mol. Brain 13:56. doi: 10.1186/s13041-020-00597-2
Goldman, J. S., Ashour, M. A., Magdesian, M. H., Tritsch, N. X., Harris, S. N., Christofi, N., et al. (2013). Netrin-1 promotes excitatory synaptogenesis between cortical neurons by initiating synapse assembly. J. Neurosci. 33, 17278–17289. doi: 10.1523/JNEUROSCI.1085-13.2013
Görgens, S. W., Eckardt, K., Jensen, J., Drevon, C. A., and Eckel, J. (2015). Exercise and Regulation of Adipokine and Myokine Production. Prog. Mol. Biol. Transl. Sci. 135, 313–336. doi: 10.1016/bs.pmbts.2015.07.002
Hansen, K. B., Wollmuth, L., Bowie, D., Furukawa, H., Menniti, F., Sobolevsky, A., et al. (2021). Structure, Function, and Pharmacology of Glutamate Receptor Ion Channels. Pharmacol. Rev. 73, 298–487. doi: 10.1124/pharmrev.120.000131
Hayashi, Y., Shi, S., Esteban, J., Piccini, A., Poncer, J., and Malinow, R. (2000). Driving AMPA receptors into synapses by LTP and CaMKII: requirement for GluR1 and PDZ domain interaction. Science 287, 2262–2267. doi: 10.1126/science.287.5461.2262
He, P., Liu, Q., Wu, J., and Shen, Y. (2012). Genetic deletion of TNF receptor suppresses excitatory synaptic transmission via reducing AMPA receptor synaptic localization in cortical neurons. FASEB J. 26, 334–345. doi: 10.1096/fj.11-192716
Hennekinne, L., Colasse, S., Triller, A., and Renner, M. (2013). Differential control of thrombospondin over synaptic glycine and AMPA receptors in spinal cord neurons. J. Neurosci. 33, 11432–11439. doi: 10.1523/JNEUROSCI.5247-12.2013
Hong, E., McCord, A., and Greenberg, M. E. (2008). A biological function for the neuronal activity-dependent component of Bdnf transcription in the development of cortical inhibition. Neuron 60, 610–624. doi: 10.1016/j.neuron.2008.09.024
Horn, K., Glasgow, S., Gobert, D., Bull, S., Luk, T., Girgis, J., et al. (2013). DCC expression by neurons regulates synaptic plasticity in the adult brain. Cell Rep. 3, 173–185. doi: 10.1016/j.celrep.2012.12.005
Ijichi, A., Sakuma, S., and Tofilon, P. (1995). Hypoxia-induced vascular endothelial growth factor expression in normal rat astrocyte cultures. Glia 14, 87–93. doi: 10.1002/glia.440140203
Ishida, A., Murray, J., Saito, Y., Kanthou, C., Benzakour, O., Shibuya, M., et al. (2001). Expression of vascular endothelial growth factor receptors in smooth muscle cells. J. Cell Physiol. 188, 359–368. doi: 10.1002/jcp.1121
Ishii, N., Wadsworth, W., Stern, B., Culotti, J., and Hedgecock, E. M. (1992). UNC-6, a laminin-related protein, guides cell and pioneer axon migrations in C. elegans. Neuron 9, 873–881. doi: 10.1016/0896-6273(92)90240-e
Islam, M. R., Valaris, S., Young, M. F., Haley, E. B., Luo, R., Bond, S. F., et al. (2021). Exercise hormone irisin is a critical regulator of cognitive function. Nat. Metab. 3, 1058–1070. doi: 10.1038/s42255-021-00438-z
Johnson, K. G., Tenney, A. P., Ghose, A., Duckworth, A. M., Higashi, M. E., Parfitt, K., et al. (2006). The HSPGs syndecan and dallylike bind the receptor phosphatase LAR and exert distinct effects on synaptic development. Neuron 49, 517–531. doi: 10.1016/j.neuron.2006.01.026
Jones, E. V., Bernardinelli, Y., Tse, Y. C., Chierzi, S., Wong, T. P., and Murai, K. K. (2011). Astrocytes control glutamate receptor levels at developing synapses through SPARC-beta-integrin interactions. J. Neurosci. 31, 4154–4165. doi: 10.1523/JNEUROSCI.4757-10.2011
Jourdi, H., Iwakura, Y., Narisawa-Saito, M., Ibaraki, K., Xiong, H., Watanabe, M., et al. (2003). Brain-derived neurotrophic factor signal enhances and maintains the expression of AMPA receptor-associated PDZ proteins in developing cortical neurons. Dev. Biol. 263, 216–230. doi: 10.1016/j.ydbio.2003.07.008
Kang, H. J., and Schuman, E. M. (1995a). Long-lasting neurotrophin-induced enhancement of synaptic transmission in the adult hippocampus. Science 267, 1658–1662. doi: 10.1126/science.7886457
Kang, H. J., and Schuman, E. M. (1995b). Neurotrophin-induced modulation of synaptic transmission in the adult hippocampus. J. Physiol. Paris. 89, 11–22. doi: 10.1016/0928-4257(96)80547-x
Keifer, J. (2022). Regulation of AMPAR trafficking in synaptic plasticity by BDNF and the impact of neurodegenerative disease. J. Neurosci. Res. 100, 979–991. doi: 10.1002/jnr.25022
Kennedy, T. E., Serafini, T., de la Torre, J. R., and Tessier-Lavigne, M. (1994). Netrins are diffusible chemotropic factors for commissural axons in the embryonic spinal cord. Cell 78, 425–435. doi: 10.1016/0092-8674(94)90421-9
Ko, J., Pramanik, G., Um, J., Shim, J., Lee, D., Kim, K., et al. (2015). PTPσ functions as a presynaptic receptor for the glypican-4/LRRTM4 complex and is essential for excitatory synaptic transmission. Proc. Natl. Acad. Sci. U. S. A. 112, 1874–1879. doi: 10.1073/pnas.1410138112
Kucukdereli, H., Allen, N. J., Lee, A. T., Feng, A., Ozlu, M. I., Conatser, L. M., et al. (2011). Control of excitatory CNS synaptogenesis by astrocyte-secreted proteins Hevin and SPARC. Proc. Natl. Acad. Sci. U. S. A. 108, E440–E449. doi: 10.1073/pnas.1104977108
Lee, F., and Hempstead, B. (2018). New roles for an ancient factor. Trends Neurosci. 41, 765–767. doi: 10.1016/j.tins.2018.08.012
Levy, A., Levy, N., Loscalzo, J., Calderone, A., Takahashi, N., Yeo, K., et al. (1995). Regulation of vascular endothelial growth factor in cardiac myocytes. Circ. Res. 76, 758–766. doi: 10.1161/01.res.76.5.758
Lewitus, G., Pribiag, H., Duseja, R., St-Hilaire, M., and Stellwagen, D. (2014). An adaptive role of TNFα in the regulation of striatal synapses. J. Neurosci. 34, 6146–6155. doi: 10.1523/JNEUROSCI.3481-13.2014
Li, H., Huang, B., Vishwasrao, H., Sutedja, N., Chen, W., Jin, I., et al. (2009). Dscam mediates remodeling of glutamate receptors in Aplysia during de novo and learning-related synapse formation. Neuron 61, 527–540. doi: 10.1016/j.neuron.2009.01.010
Li, W., and Keifer, J. (2008). Coordinate action of pre- and postsynaptic brain-derived neurotrophic factor is required for AMPAR trafficking and acquisition of in vitro classical conditioning. Neuroscience 155, 686–697. doi: 10.1016/j.neuroscience.2008.06.043
Li, W., and Keifer, J. (2009). BDNF-induced synaptic delivery of AMPAR subunits is differentially dependent on NMDA receptors and requires ERK. Neurobiol. Learn Mem. 91, 243–249. doi: 10.1016/j.nlm.2008.10.002
Li, X., and Wolf, M. (2011). Brain-derived neurotrophic factor rapidly increases AMPA receptor surface expression in rat nucleus accumbens. Eur. J. Neurosci. 34, 190–198. doi: 10.1111/j.1460-9568.2011.07754.x
Licht, T., Goshen, I., Avital, A., Kreisel, T., Zubedat, S., Eavri, R., et al. (2011). Reversible modulations of neuronal plasticity by VEGF. Proc. Natl. Acad. Sci. U. S. A. 108, 5081–5086. doi: 10.1073/pnas.1007640108
Lin, J. W., Ju, W., Foster, K., Lee, S. H., Ahmadian, G., Wyszynski, M., et al. (2000). Distinct molecular mechanisms and divergent endocytotic pathways of AMPA receptor internalization. Nat. Neurosci. 3, 1282–1290.
Linhoff, M., Laurén, J., Cassidy, R., Dobie, F., Takahashi, H., Nygaard, H., et al. (2009). An unbiased expression screen for synaptogenic proteins identifies the LRRTM protein family as synaptic organizers. Neuron 61, 734–749. doi: 10.1016/j.neuron.2009.01.017
Lourenco, M., Frozza, R., de Freitas, G., Zhang, H., Kincheski, G., Ribeiro, F., et al. (2019). Exercise-linked FNDC5/irisin rescues synaptic plasticity and memory defects in Alzheimer’s models. Nat. Med. 25, 165–175. doi: 10.1038/s41591-018-0275-4
Luth, E., Hodul, M., Rennich, B., Riccio, C., Hofer, J., Markoja, K., et al. (2021). VER/VEGF receptors regulate AMPA receptor surface levels and glutamatergic behavior. PLoS Genet. 17:e1009375. doi: 10.1371/journal.pgen.1009375
Man, H. Y., Lin, J. W., Ju, W. H., Ahmadian, G., Liu, L., Becker, L. E., et al. (2000). Regulation of AMPA receptor-mediated synaptic transmission by clathrin-dependent receptor internalization. Neuron 25, 649–662.
Mathew, D., Ataman, B., Chen, J., Zhang, Y., Cumberledge, S., and Budnik, V. (2005). Wingless signaling at synapses is through cleavage and nuclear import of receptor DFrizzled2. Science 310, 1344–1347. doi: 10.1126/science.1117051
Matthews, V., Aström, M., Chan, M., Bruce, C., Krabbe, K., Prelovsek, O., et al. (2009). Brain-derived neurotrophic factor is produced by skeletal muscle cells in response to contraction and enhances fat oxidation via activation of AMP-activated protein kinase. Diabetologia 52, 1409–1418. doi: 10.1007/s00125-009-1364-1
Mattson, M. (2012). Energy intake and exercise as determinants of brain health and vulnerability to injury and disease. Cell Metab. 16, 706–722. doi: 10.1016/j.cmet.2012.08.012
Messaoudi, E., Bârdsen, K., Srebro, B., and Bramham, C. (1998). Acute intrahippocampal infusion of BDNF induces lasting potentiation of synaptic transmission in the rat dentate gyrus. J. Neurophysiol. 79, 496–499. doi: 10.1152/jn.1998.79.1.496
McGehee, A. M., Moss, B. J., and Juo, P. (2015). The DAF-7/TGF-β signaling pathway regulates abundance of the Caenorhabditis elegans glutamate receptor GLR-1. Mol. Cell. Neurosci. 67, 66–74.
Miech, C., Pauer, H., He, X., and Schwarz, T. (2008). Presynaptic local signaling by a canonical wingless pathway regulates development of the Drosophila neuromuscular junction. J. Neurosci. 28, 10875–10884. doi: 10.1523/JNEUROSCI.0164-08.2008
Nakata, H., and Nakamura, S. (2007). Brain-derived neurotrophic factor regulates AMPA receptor trafficking to post-synaptic densities via IP3R and TRPC calcium signaling. FEBS Lett. 581, 2047–2054. doi: 10.1016/j.febslet.2007.04.041
Nakaya, N., Sultana, A., and Tomarev, S. (2017). Impaired AMPA receptor trafficking by a double knockout of zebrafish olfactomedin1a/b. J. Neurochem. 143, 635–644. doi: 10.1111/jnc.14231
O’Brien, R., Kamboj, S., Ehlers, M., Rosen, K., Fischbach, G., and Huganir, R. (1998). Activity-dependent modulation of synaptic AMPA receptor accumulation. Neuron 21, 1067–1078. doi: 10.1016/s0896-6273(00)80624-8
O’Brien, R., Xu, D., Petralia, R., Steward, O., Huganir, R., and Worley, P. (1999). Synaptic clustering of AMPA receptors by the extracellular immediate-early gene product Narp. Neuron 23, 309–323. doi: 10.1016/s0896-6273(00)80782-5
Ogoshi, F., Yin, H., Kuppumbatti, Y., Song, B., Amindari, S., and Weiss, J. (2005). Tumor necrosis-factor-alpha (TNF-alpha) induces rapid insertion of Ca2+-permeable alpha-amino-3-hydroxyl-5-methyl-4-isoxazole-propionate (AMPA)/kainate (Ca-A/K) channels in a subset of hippocampal pyramidal neurons. Exp. Neurol. 193, 384–393. doi: 10.1016/j.expneurol.2004.12.026
Ogunshola, O., Antic, A., Donoghue, M., Fan, S., Kim, H., Stewart, W., et al. (2002). Paracrine and autocrine functions of neuronal vascular endothelial growth factor (VEGF) in the central nervous system. J. Biol. Chem. 277, 11410–11415. doi: 10.1074/jbc.M111085200
Ohkawa, T., Fukata, Y., Yamasaki, M., Miyazaki, T., Yokoi, N., Takashima, H., et al. (2013). Autoantibodies to epilepsy-related LGI1 in limbic encephalitis neutralize LGI1-ADAM22 interaction and reduce synaptic AMPA receptors. J. Neurosci. 33, 18161–18174.
Packard, M., Koo, E., Gorczyca, M., Sharpe, J., Cumberledge, S., and Budnik, V. (2002). The Drosophila Wnt, wingless, provides an essential signal for pre- and postsynaptic differentiation. Cell 111, 319–330. doi: 10.1016/s0092-8674(02)01047-4
Pan, W., Banks, W., Fasold, M., Bluth, J., and Kastin, A. (1998). Transport of brain-derived neurotrophic factor across the blood-brain barrier. Neuropharmacology 37, 1553–1561. doi: 10.1016/s0028-3908(98)00141-5
Pandya, N., Seeger, C., Babai, N., Gonzalez-Lozano, M., Mack, V., Lodder, J., et al. (2018). Noelin1 Affects Lateral Mobility of Synaptic AMPA Receptors. Cell Rep. 24, 1218–1230. doi: 10.1016/j.celrep.2018.06.102
Pelkey, K., Barksdale, E., Craig, M., Yuan, X., Sukumaran, M., Vargish, G., et al. (2015). Pentraxins coordinate excitatory synapse maturation and circuit integration of parvalbumin interneurons. Neuron 85, 1257–1272. doi: 10.1016/j.neuron.2015.02.020
Pizzorusso, T., Medini, P., Berardi, N., Chierzi, S., Fawcett, J., and Maffei, L. (2002). Reactivation of ocular dominance plasticity in the adult visual cortex. Science 298, 1248–1251. doi: 10.1126/science.1072699
Pyka, M., Wetzel, C., Aguado, A., Geissler, M., Hatt, H., and Faissner, A. (2011). Chondroitin sulfate proteoglycans regulate astrocyte-dependent synaptogenesis and modulate synaptic activity in primary embryonic hippocampal neurons. Eur. J. Neurosci. 33, 2187–2182. doi: 10.1111/j.1460-9568.2011.07690.x
Reimers, J., Loweth, J., and Wolf, M. E. (2014). BDNF contributes to both rapid and homeostatic alterations in AMPA receptor surface expression in nucleus accumbens medium spiny neurons. Eur. J. Neurosci. 39, 1159–1169. doi: 10.1111/ejn.12422
Reti, I., Miskimon, M., Dickson, M., Petralia, R., Takamiya, K., Bland, R., et al. (2008). Activity-dependent secretion of neuronal activity regulated pentraxin from vasopressin neurons into the systemic circulation. Neuroscience 151, 352–360. doi: 10.1016/j.neuroscience.2007.10.040
Schiera, G., Proia, P., Alberti, C., Mineo, M., Savettieri, G., and Di Liegro, I. (2007). Neurons produce FGF2 and VEGF and secrete them at least in part by shedding extracellular vesicles. J. Cell Mol. Med. 11, 1384–1394. doi: 10.1111/j.1582-4934.2007.00100.x
Schwenk, J., Harmel, N., Brechet, A., Zolles, G., Berkefeld, H., Müller, C., et al. (2012). High-resolution proteomics unravel architecture and molecular diversity of native AMPA receptor complexes. Neuron 74, 621–633. doi: 10.1016/j.neuron.2012.03.034
Severinsen, M., and Pedersen, B. (2020). Muscle-Organ Crosstalk: The Emerging Roles of Myokines. Endocr. Rev. 41, 594–609. doi: 10.1210/endrev/bnaa016
Shanks, N., Savas, J., Maruo, T., Cais, O., Hirao, A., Oe, S., et al. (2012). Differences in AMPA and kainate receptor interactomes facilitate identification of AMPA receptor auxiliary subunit GSG1L. Cell Rep. 1, 590–598. doi: 10.1016/j.celrep.2012.05.004
Sia, G., Béïque, J., Rumbaugh, G., Cho, R., Worley, P., and Huganir, R. (2007). Interaction of the N-terminal domain of the AMPA receptor GluR4 subunit with the neuronal pentraxin NP1 mediates GluR4 synaptic recruitment. Neuron 55, 87–102. doi: 10.1016/j.neuron.2007.06.020
Siddiqui, T., Tari, P., Connor, S., Zhang, P., Dobie, F., She, K., et al. (2013). An LRRTM4-HSPG complex mediates excitatory synapse development on dentate gyrus granule cells. Neuron 79, 680–695. doi: 10.1016/j.neuron.2013.06.029
Soler-Llavina, G., Arstikaitis, P., Morishita, W., Ahmad, M., Südhof, T., and Malenka, R. (2013). Leucine-rich repeat transmembrane proteins are essential for maintenance of long-term potentiation. Neuron 79, 439–446. doi: 10.1016/j.neuron.2013.06.007
Stellwagen, D., Beattie, E., Seo, J., and Malenka, R. (2005). Differential regulation of AMPA receptor and GABA receptor trafficking by tumor necrosis factor-alpha. J. Neurosci. 25, 3219–3228. doi: 10.1523/JNEUROSCI.4486-04.2005
Stellwagen, D., and Malenka, R. (2006). Synaptic scaling mediated by glial TNF-alpha. Nature 440, 1054–1059. doi: 10.1038/nature04671
Sultana, A., Nakaya, N., Dong, L., Abu-Asab, M., Qian, H., and Tomarev, S. (2014). Deletion of olfactomedin 2 induces changes in the AMPA receptor complex and impairs visual, olfactory, and motor functions in mice. Exp. Neurol. 261, 802–811. doi: 10.1016/j.expneurol.2014.09.002
Szuhany, K., Bugatti, M., and Otto, M. W. (2015). A meta-analytic review of the effects of exercise on brain-derived neurotrophic factor. J. Psychiatr. Res. 60, 56–64. doi: 10.1016/j.jpsychires.2014.10.003
Takahashi, H., and Craig, A. (2013). Protein tyrosine phosphatases PTPδ, PTPσ, and LAR: presynaptic hubs for synapse organization. Trends Neurosci. 36, 522–534. doi: 10.1016/j.tins.2013.06.002
Tao, X., Finkbeiner, S., Arnold, D., Shaywitz, A., and Greenberg, M. (1998). Ca2+ influx regulates BDNF transcription by a CREB family transcription factor-dependent mechanism. Neuron 20, 709–726. doi: 10.1016/s0896-6273(00)81010-7
Tao, X., West, A., Chen, W., Corfas, G., and Greenberg, M. E. (2002). A calcium-responsive transcription factor, CaRF, that regulates neuronal activity-dependent expression of BDNF. Neuron 33, 383–395. doi: 10.1016/s0896-6273(01)00561-x
Tien, W., Chen, J., and Wu, K. (2017). SheddomeDB: the ectodomain shedding database for membrane-bound shed markers. BMC Bioinformatics 18:42. doi: 10.1186/s12859-017-1465-7
Turrigiano, G., Leslie, K., Desai, N., Rutherford, L., and Nelson, S. (1998). Activity-dependent scaling of quantal amplitude in neocortical neurons. Nature 391, 892–896. doi: 10.1038/36103
Vaynman, S., Ying, Z., and Gomez-Pinilla, F. (2004). Hippocampal BDNF mediates the efficacy of exercise on synaptic plasticity and cognition. Eur. J. Neurosci. 20, 2580–2590. doi: 10.1111/j.1460-9568.2004.03720.x
von Engelhardt, J., Mack, V., Sprengel, R., Kavenstock, N., Li, K., Stern-Bach, Y., et al. (2010). CKAMP44: a brain-specific protein attenuating short-term synaptic plasticity in the dentate gyrus. Science 327, 1518–1522. doi: 10.1126/science.1184178
Wong, E., Glasgow, S., Trigiani, L., Chitsaz, D., Rymar, V., Sadikot, A., et al. (2019). Spatial memory formation requires netrin-1 expression by neurons in the adult mammalian brain. Learn Mem. 26, 77–83. doi: 10.1101/lm.049072.118
Wrann, C., White, J., Salogiannnis, J., Laznik-Bogoslavski, D., Wu, J., Ma, D., et al. (2013). Exercise induces hippocampal BDNF through a PGC-1α/FNDC5 pathway. Cell Metab. 18, 649–659. doi: 10.1016/j.cmet.2013.09.008
Xu, D., Hopf, C., Reddy, R., Cho, R., Guo, L., Lanahan, A., et al. (2003). Narp and NP1 form heterocomplexes that function in developmental and activity-dependent synaptic plasticity. Neuron 39, 513–528. doi: 10.1016/s0896-6273(03)00463-x
Keywords: AMPAR, trafficking, secreted factors, regulation, synapse
Citation: Rennich BJ, Luth ES, Moores S and Juo P (2023) Regulation of AMPA receptor trafficking by secreted protein factors. Front. Cell. Neurosci. 17:1271169. doi: 10.3389/fncel.2023.1271169
Received: 01 August 2023; Accepted: 03 November 2023;
Published: 27 November 2023.
Edited by:
Frederic John Hoerndli, Colorado State University, United StatesReviewed by:
Igor Delvendahl, University of Zurich, SwitzerlandGiusy Laudati, University of Naples Federico II, Italy
Dion Dickman, University of Southern California, United States
Copyright © 2023 Rennich, Luth, Moores and Juo. This is an open-access article distributed under the terms of the Creative Commons Attribution License (CC BY). The use, distribution or reproduction in other forums is permitted, provided the original author(s) and the copyright owner(s) are credited and that the original publication in this journal is cited, in accordance with accepted academic practice. No use, distribution or reproduction is permitted which does not comply with these terms.
*Correspondence: Peter Juo, cGV0ZXIuanVvQHR1ZnRzLmVkdQ==