- 1Faculty of Medicine and Dentistry and the School of Biological and Behavioural Sciences, Queen Mary University of London, London, United Kingdom
- 2Gastrointestinal and Liver Diseases Research Center, Guilan University of Medical Sciences, Rasht, Iran
- 3Department of Microbiology, School of Medicine, Guilan University of Medical Sciences, Rasht, Iran
- 4Department of Neurology, Thomas Jefferson University, Philadelphia, PA, United States
Alzheimer’s disease (AD) is a complex, multifactorial disorder, influenced by a multitude of variables ranging from genetic factors, age, and head injuries to vascular diseases, infections, and various other environmental and demographic determinants. Among the environmental factors, the role of the microbiome in the genesis of neurodegenerative disorders (NDs) is gaining increased recognition. This paradigm shift is substantiated by an extensive body of scientific literature, which underscores the significant contributions of microorganisms, encompassing viruses and gut-derived bacteria, to the pathogenesis of AD. The mechanism by which microbial infection exerts its influence on AD hinges primarily on inflammation. Neuroinflammation, activated in response to microbial infections, acts as a defense mechanism for the brain but can inadvertently lead to unexpected neuropathological perturbations, ultimately contributing to NDs. Given the ongoing uncertainty surrounding the genetic factors underpinning ND, comprehensive investigations into environmental factors, particularly the microbiome and viral agents, are imperative. Recent advances in neuroscientific research have unveiled the pivotal role of non-coding RNAs (ncRNAs) in orchestrating various pathways integral to neurodegenerative pathologies. While the upstream regulators governing the pathological manifestations of microorganisms remain elusive, an in-depth exploration of the nuanced role of ncRNAs holds promise for the development of prospective therapeutic interventions. This review aims to elucidate the pivotal role of ncRNAs as master modulators in the realm of neurodegenerative conditions, with a specific focus on Alzheimer’s disease.
1 Introduction
Alzheimer’s disease (AD) represents a devastating and globally prevalent neurodegenerative disorder, characterized as the most common form of dementia. Recent statistics from the Centers for Disease Control and Prevention (CDC) reveal that nearly 6 million individuals in the United States alone are afflicted by AD (Dorszewska et al., 2016). The etiology of AD remains enigmatic due to its intricate interplay with various organ systems and multicellular interactions, often initiated by microbial infections. Infections targeting the central nervous system (CNS) present a grave and frequently fatal health concern, with survivors often grappling with enduring neurological impairments (Pluta et al., 2020). To unravel the intricate mechanisms governing the impact of microbes on CNS function, it becomes paramount to delve into the regulatory role of non-coding RNAs (ncRNAs) as their significance in numerous disease states has garnered increasing recognition. Notably, the precise mechanisms underlying the contribution of human viral infections or perturbations in the host microbiome to the onset of AD remain elusive, potentially involving intricate ncRNA-mediated processes. Perturbations in ncRNAs can exert profound effects on disease states, thus emphasizing the critical importance of comprehending these molecules and the factors influencing them. Such insights hold promise for manipulating the mechanisms that influence brain function, offering prospects for ameliorating or delaying the progression of neurodegenerative pathology (Li et al., 2021).
2 Clinical and cellular mechanisms in Alzheimer’s disease
Alzheimer’s disease (AD) is characterized by a spectrum of clinical and pathological features, encompassing synaptic loss, neuronal damage, alterations in plasticity, and the aggregation of senile plaques composed of Aβ and tau proteins, ultimately culminating in progressive neural system dysfunction over time (Valenza and Scuderi, 2022). Predominantly, AD patients grapple with memory loss as the cardinal clinical manifestation (Barichello et al., 2013). Additionally, AD patients often exhibit mild language impairments and judgment deficits (Miguel et al., 2020). Neuropsychiatric symptoms, including behavioral changes, can also be observed in individuals with AD (Lyketsos et al., 2011). The pathophysiological landscape of AD is multifaceted, underpinned by various hypotheses, including the roles of (a) amyloid-β, (b) Tau pathology leading to neurofibrillary tangles, (c) mitochondrial dysfunction, and reactive oxygen species (ROS) generation, among others (Alqahtani et al., 2023). Amyloidosis, a hallmark feature of Alzheimer’s disease (AD), is characterized by the aggregation of amyloid peptides within cells and tissues, giving rise to disruptive plaques. Aβ peptides can further self-aggregate through complex mechanisms, leading to organ damage. A central player in the amyloidogenic cascade is the beta-site amyloid precursor protein cleaving enzyme 1 (BACE1) enzyme. It cleaves the amyloid precursor protein (APP) at the β site, leading to the production of amyloid β-1 (Sayad et al., 2022). This event is pivotal in AD as the excessive accumulation of amyloid plaques underlies disease pathogenesis. Intriguingly, alterations in BACE1 expression have been linked to neuropathological conditions, suggesting a tightly regulated process governed by non-coding RNAs (ncRNAs; Zhou et al., 2021; Hao et al., 2022; Sayad et al., 2022).
Neural cells have been reported to produce higher quantities of Aβ compared to other cell types. Aβ is a crucial component involved in intracellular signaling cascades and various physiological functions within the central nervous system (CNS; Bernabeu-Zornoza et al., 2022). Another key aspect of AD is tau pathology. As a microtubule-associated protein, tau contributes to microtubule assembly and related protein interactions. Aberrant tau phosphorylation disrupts microtubule integrity, resulting in synaptic dysfunction and increased neurite branching (Guo et al., 2017). Tau oligomers have been implicated as initiators of deficits in axonal transport, resulting in neural damage and eventual cell death. The mitochondrial damage hypothesis suggests that Aβ accumulation within brain mitochondria can alter their structure and function. These changes are associated with increased Aβ levels, leading to energy generation imbalances and respiratory deficits (Fišar, 2022). Aβ oligomer buildup within the mitochondria can also stimulate bidirectional reactive oxygen species (ROS) production, which can be toxic to neural cells (Araki, 2023). Methylation of mitochondrial tRNA, a small non-coding RNA, has been observed in elderly individuals, whether they are healthy or have Alzheimer’s disease (AD). This methylation can potentially impact mitochondrial protein biosynthesis and contribute to mitochondrial dysfunction, which is an early hallmark of neurodegeneration and age-related disorders (Silzer et al., 2020). Changes in the expressions of tRNA-related fragments (TRFs) have been noted in the hippocampus of AD patients, and these changes appear to be age- and disease-stage dependent (Wu et al., 2021). Gender differences in brain development have significant implications for the investigation of neuropsychiatric and neurodegenerative disorders, including AD. Brain pathology in female patients with AD, compared to male patients, underscores the role of sex differences in the progression of AD (Castro-Aldrete et al., 2023). Aberrant expressions of corticotropin-releasing factor (CRF) in female patients have been correlated with increased tau phosphorylation, a pathological step in the formation of neurofibrillary tangles seen in AD (Bangasser et al., 2017). Gender-associated long non-coding RNAs (lncRNAs), such as SLC25A25-AS1, LY86-AS1, and PWRN1, have been identified as highly dysregulated in AD brains although further studies are warranted to elucidate their precise roles in AD pathology (Cao et al., 2019). Observations through brain MRI reveal ventricle enlargement and cerebral cortex shrinkage, indicative of Alzheimer’s pathology. These structural changes impact memory, intelligence, judgment, behavior, and language in Alzheimer’s patients (Lombardi et al., 2020). Furthermore, disruptions in the blood–brain barrier (BBB) are evident, characterized by altered endothelial cell connections, leading to enhanced fluid transcytosis, pericyte loss, compromised tight junction proteins, and cerebral microhemorrhages (Figure 1; Aragón-González et al., 2022).
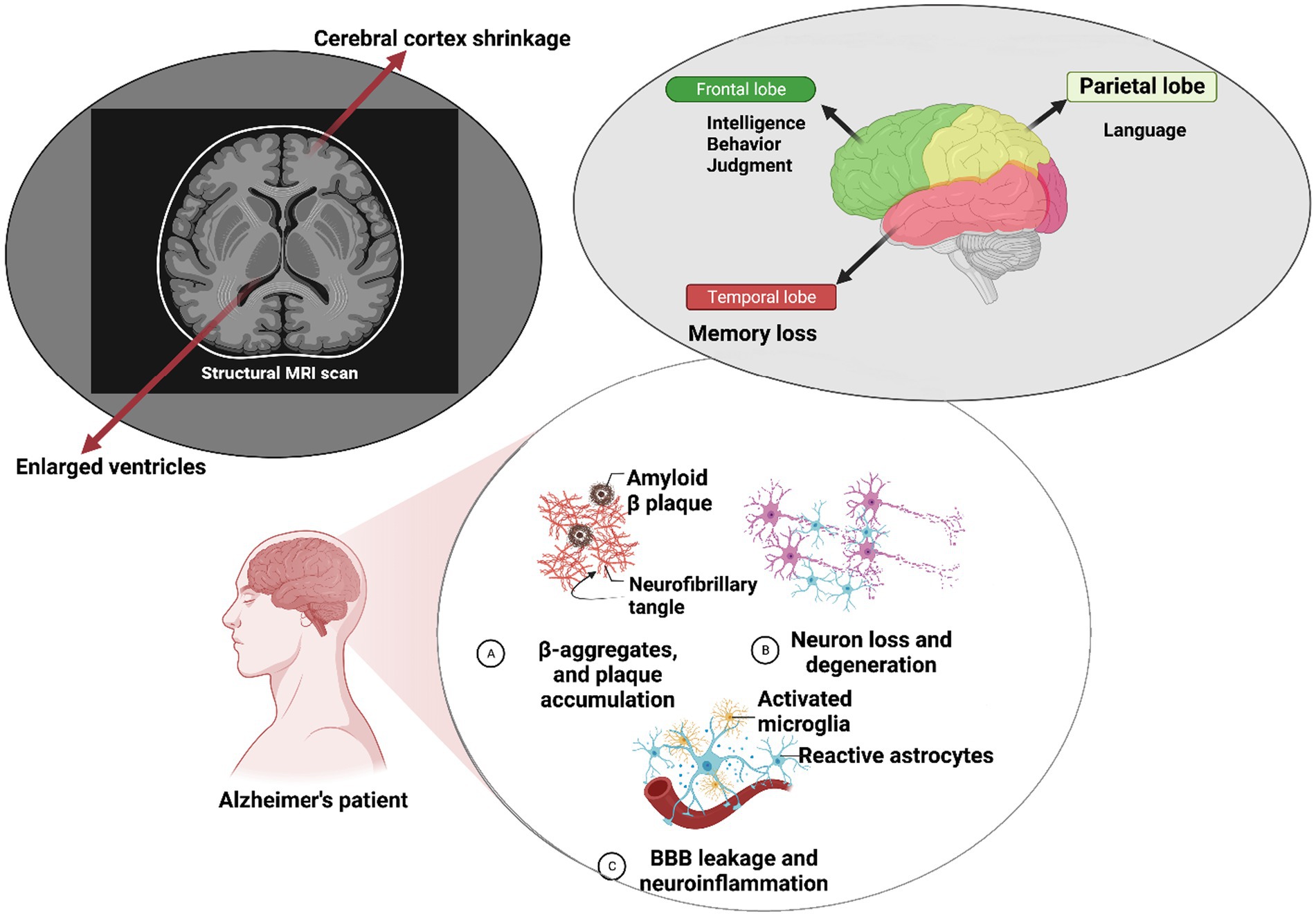
Figure 1. Molecular mechanisms underlying Alzheimer’s disease pathology. At the molecular level within neurons, Alzheimer’s disease (AD) is characterized by the self-aggregation of amyloid-β peptides into plaques and hyperphosphorylation of the tau protein. These pathological events can trigger microglial activation, leading to an increase in blood–brain barrier (BBB) permeability and subsequent neuroinflammation, which results in neural impairments. Clinically, individuals with AD often exhibit a range of symptoms such as deficits in intelligence and judgment behavior, speech difficulties, and memory loss. Brain magnetic resonance imaging (MRI) reveals significant structural changes, including cerebral cortex shrinkage and ventricular enlargement, highlighting the structural alterations associated with the progression of AD.
3 Non-coding RNAs
Non-coding RNAs (ncRNAs) constitute a diverse group of RNA molecules that, primarily, do not encode proteins. Their multifaceted roles in cellular processes and pathophysiological conditions have garnered increasing attention in contemporary molecular biology. These ncRNAs participate in gene regulation, epigenetic modifications, and RNA processing, contributing significantly to normal neuronal function (Das et al., 2021). They can be broadly categorized into two major groups: circular RNAs and linear RNAs, as illustrated in Figure 2. Linear RNAs further branch into housekeeping and regulatory elements (Fu, 2014). The housekeeping group encompasses transfer RNA (tRNA), ribosomal RNA (rRNA), small nuclear RNA (snRNA), and small nucleolar RNA (snoRNA). Regulatory elements, based on their lengths, segregate into long ncRNAs (lncRNAs) and short ncRNAs or small ncRNAs (sncRNAs; Zhang et al., 2019). Intergenic and intronic divisions are found within lncRNAs (Ma et al., 2013). Pioneering studies have unveiled that conserved regions within intergenic lncRNAs are significantly enriched in protein-RNA interaction signatures, contrasting non-conserved counterparts such as Malat1, Neat1, and Meg3 (Ruiz-Orera and Albà, 2019). SncRNAs encompass various subsets such as microRNAs (miRNAs), small-interfering RNAs (siRNAs), and piwi-interacting RNAs (piRNAs), among others (Gomes et al., 2013). MiRNAs, highly conserved in evolution, modulate nearly 50% of genes post-transcriptionally, serving as critical regulators in brain development, neuronal processes including apoptosis, proliferation, and differentiation (Gurtan and Sharp, 2013). SiRNAs are involved in posttranscriptional gene silencing although not exclusively (Carthew and Sontheimer, 2009). PiRNAs contribute to diverse neural events, including genomic heterogeneity, neuronal response to injuries, behavior, and memory formation (Kim, 2019; Sato et al., 2023). Regulatory RNAs exhibit the potential to manipulate cellular processes, and their aberrant expressions can underpin disease etiology in specific cases (Statello et al., 2021). In the context of diagnostics, ncRNAs offer valuable tools for molecular biology due to their relative stability in the bloodstream. For example, NEAT1, an ncRNA, plays a pivotal role in chromatin remodeling and microtubule integrity. The downregulation of NEAT1 may enhance the expression of phosphorylated Tau protein, leading to microtubule dysfunction via the Frizzled Class Receptor 3/CSK3β/p-tau pathway (Zhao et al., 2020). Linc00507, another long intergenic non-protein coding RNA, has been associated with AD pathology through enhanced tau phosphorylation (Ni et al., 2022). The dysregulation of NDM29, BC200, 17A, and 51A ncRNAs has been correlated with AD pathology linked to Aβ accumulation (Luo and Chen, 2016). Recent scientific endeavors have spotlighted the hypothesis that microorganisms, particularly those of viral and bacterial origin, may significantly contribute to neurodegenerative processes, potentially employing ncRNAs as mediators in the manifestation of brain impairments.
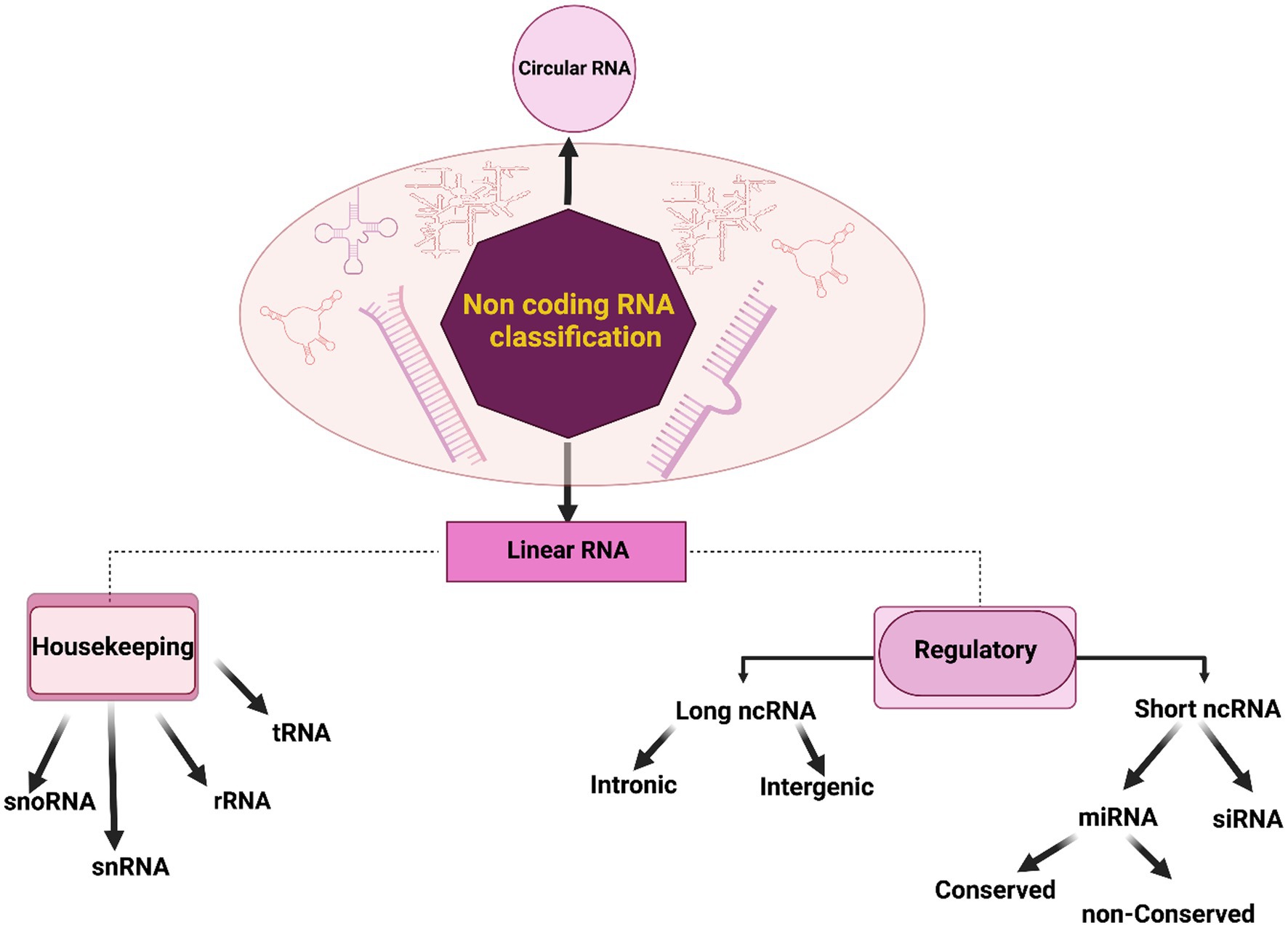
Figure 2. Classification of non-coding RNAs (ncRNAs). In a general categorization, non-coding RNAs (ncRNAs) can be systematically classified into two fundamental groups: circular and linear RNAs. Linear RNAs, further delineated based on their functional roles and expression patterns, segregate into two categories: housekeeping and regulatory elements. The housekeeping group consists of elements that are typically expressed at a constant level, including transfer RNA (tRNA), ribosomal RNA (rRNA), small nuclear RNA (snRNA), and small nucleolar RNA (snoRNA). In contrast, regulatory elements are subdivided into short and long ncRNAs based on their respective lengths. This classification provides a foundational framework for understanding the diverse landscape of ncRNAs and their pivotal roles in cellular processes and disease pathogenesis.
4 Gut microbiome and Alzheimer’s disease
Recent research has shed light on the intricate relationship between the gut microbiome and Alzheimer’s disease (AD) pathology, yet several crucial gaps in understanding remain to be addressed. Human studies, particularly interventional investigations, are needed to draw definitive conclusions regarding the mediating role of non-coding RNAs (ncRNAs) in the initiation and progression of neurodegenerative diseases, including Alzheimer’s, ultimately providing practical avenues for therapeutic interventions (Johnson et al., 2023).
Mounting evidence suggests that dysbiosis of gut microbiota can contribute to AD pathogenesis through microbial metabolites, such as Bacteroides fragilis toxins (BFT) and lipopolysaccharide (LPS; Sampson et al., 2016), which actively engage neurotransmitters and ncRNAs in gut–central nervous system (CNS) communication (Huang and Wu, 2021). BFT, for instance, influences the CNS by modulating the NF-κB pathway and upregulating miRNA-146a expression, potentially inciting neuroinflammation and neurodegeneration (Zhao and Lukiw, 2018a). LPS, on the other hand, can increase the expression of miRNAs-34a and 146a, targeting genes implicated in sporadic AD (Sil et al., 2020). While the precise role of miRNAs in gut–brain communication remains elusive, the potential interplay between microbial metabolites and ncRNAs is evident. For instance, butyrate induces miR-375 expression and regulates tryptophan metabolism, a process associated with AD neuropathogenesis and cognitive dysfunction (Moloney et al., 2017).
Exploring the connection between bacterial toxins, gastrointestinal (GI) barrier permeability, and the role of different ncRNAs in facilitating the passage of molecules through the blood–brain barrier (BBB) is essential (Sochocka et al., 2019; Tang et al., 2020). The role of BFT in GI barrier disruption has been documented through synaptic E-cadherin cleavage in GI epithelial cells, emphasizing the need to examine bacterial metabolites with neurotoxic potential (Zhao and Lukiw, 2018b). Additionally, the severity of inflammation in sepsis is closely linked to miR-486-5p expression, a diagnostic biomarker of sepsis, which may enhance microglial activity and contribute to persistent inflammation (Bhattacharyya et al., 2021; Zhang M. et al., 2021; Liu et al., 2022).
In recent research, specific miRNAs have emerged as critical regulators of innate responses to infections, and symbiotic bacteria have been found to downregulate the expression of miRNA-10a in dendritic cells through interactions with toll-like receptors (TLRs) and ligands (Xue et al., 2011; Sil et al., 2020; Arzua et al., 2021; Zhao et al., 2021). Another type of non-coding RNA, H19, plays a significant role in pro-inflammatory responses induced by pathogens. It achieves this through modulation of the NF-κB signaling pathway. Moreover, H19 has implications for glucose metabolism and insulin-dependent signaling, potentially linking diabetes and Alzheimer’s disease (Datta et al., 2018; Li et al., 2019).
The altered composition of the gut microbiota is a common observation and can significantly impact the progression of Alzheimer’s disease (Barandouzi et al., 2020; Romanenko et al., 2021). The complex relationship between the gut microbiota composition and specific miRNA expression in the brain is of particular interest. This bidirectional interaction has implications for anxiety and depression-related behaviors, highlighting the potential role of non-coding RNAs as mediators in the gut–brain axis. Studies suggest that disturbances in the gut microbiota can trigger processes such as apoptosis, ROS generation, and neurodegeneration (Khan et al., 2020). It is worth noting that miRNAs influenced by pathogens may play a role in neuropsychiatric disorders, further supporting the theory of the gut microbiota–ncRNA–brain axis (Rosa et al., 2022).
In summary, disturbances in the gut microbiota can lead to fluctuations in ncRNA modulation profiles, ultimately resulting in brain dysfunction (Hou et al., 2022). Table 1 provides a more detailed exploration of gut microbiome derivatives implicated in AD pathology.
4.1 Positive effects of microbiome changes
The human gut microbiome (GM) plays a pivotal role in maintaining overall health and can significantly impact various disease states, as depicted in Figure 3. Notably, certain Lactobacillus strains originating from the GM have a positive impact on human brain health. Extensive research has provided compelling evidence that a metabolite called lactate, produced by the Lactobacillus family, contributes to the upregulation of sirtuin1 deacetylase (Sirt1) through the mediation of Sirt1 antisense (AS) lncRNA. This particular lncRNA engages in competitive interactions with miRNA-34a, ultimately rescuing the Sirt1 mRNA transcript from repression. The intricate mechanism involving lactate-induced neurotrophic factors in the hippocampus, facilitated by the Sirt1-AS lncRNA, indirectly leads to enhanced synaptic plasticity (Wang et al., 2016; Bostanciklioğlu, 2019; El Hayek et al., 2019; Ahmed et al., 2022). This connection underscores the significance of the gut–brain axis in neurodegenerative conditions and opens avenues for therapeutic interventions (Figure 3).
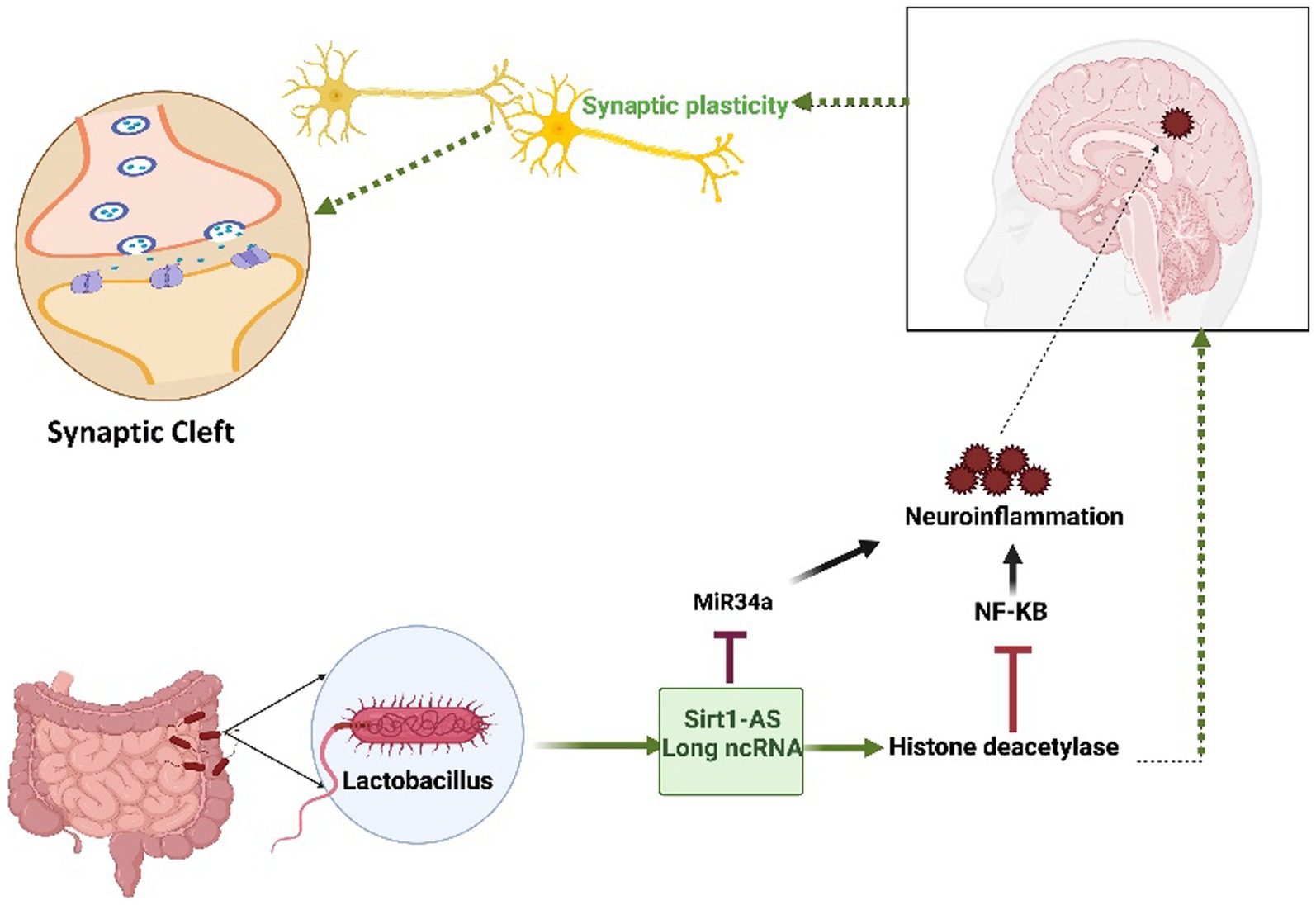
Figure 3. The Impact of the Lactobacillus-mediated regulation of Sirt1-AS, a long non-coding RNA, on human brain health. Lactobacillus-produced lactate plays a crucial role in orchestrating the activation of Sirt1-AS, a long non-coding RNA, effectively outcompeting miRNA-34a to protect the Sirt-1 mRNA transcript. This transcript encodes the histone deacetylase enzyme, which is known for its pivotal role in enhancing synaptic plasticity. Furthermore, this enzyme has the ability to effectively reduce neuroinflammation by inhibiting NF-KB signaling. Given its reliance on nicotinamide adenine dinucleotide (NAD+) and predominant localization within mitochondria, any disruption in mitochondrial function may exacerbate neurodegenerative pathologies. The figure underscores the intricate interplay between microbiota-derived molecules, RNA regulation, and neuroprotective mechanisms, offering promising therapeutic prospects.
4.2 Negative effects of microbiome changes
There is growing interest in understanding how the GM might impact neurodegenerative disorders such as Alzheimer’s disease (AD), as indicated by Kaur et al. (2019), Leblhuber et al. (2021), and Hasavci and Blank (2022). The connection between the GM and AD involves a range of pathological mechanisms, including deposition of amyloid-β, hyperphosphorylation of tau, neuroinflammation, mitochondrial dysfunction, and metabolic alterations (Wang et al., 2016; Leblhuber et al., 2021; Chandra et al., 2023). Non-coding RNAs (ncRNAs) have emerged as key regulators of the host’s responses to pathogenic microbiota. However, it is crucial to underscore the importance of conducting rigorous investigations to validate the interactions between pathogens and ncRNAs within the context of the brain.
5 Human bacterial infections and Alzheimer’s disease
Bacterial metabolites and components, including substances such as LPS, peptidoglycan (PG), and lipoprotein, possess the ability to influence the expression of host non-coding RNAs (ncRNAs), particularly microRNAs (miRNAs), which can lead to the development of pro-inflammatory conditions that indirectly impact neurodegeneration. An example of this is miRNA-1224, which significantly increases following exposure to LPS, thereby contributing to LPS-induced inflammation by regulating tumor necrosis factor-alpha or (TNF-α) through an SP1 binding site, associated with increased levels of miRNA-1224 in AD patients, suggesting a potential link between LPS, a component of Gram-negative bacteria, and the pathogenesis of AD (Niu et al., 2011; Candido et al., 2019). Furthermore, it is noteworthy that, microbial agents often employ the NF-κB signaling pathway, mediated by miRNA-146a, as a means to promote neurodegeneration.
5.1 Periodontitis, nasal microbiome, and Alzheimer’s disease
Severe periodontitis can have a significant impact on tooth health and increase the risk of various diseases, including AD. Periodontitis can trigger the release of pro-inflammatory cytokines, potentially leading to increased deposition of Aβ in the brain. However, the intricate relationship between periodontitis and Alzheimer’s disease requires further research for a comprehensive understanding. In particular, Porphyromonas gingivalis (P. gingivalis), a periodontal pathogen, has been detected in the brains of AD patients and is associated with neurotoxicity. P. gingivalis triggers toll-like receptor (TLR-2) and TLR-4 signaling, leading to the activation of TNF-α and interleukine-1 (IL-1) through bacterial LPS and an increase in miRNA-9 expression (upregulation), ultimately alleviating inflammation (Ishida et al., 2017; Dominy et al., 2019; Bashir and Khan, 2022). LPS also induces the long non-coding RNA (lncRNA) MALAT1 in gingival fibroblasts, leading to pro-inflammatory states. Paradoxically, enhancing MALAT1 expression in AD brains has been linked to the inhibition of neuroinflammation (Gholami et al., 2020; Liu et al., 2022). Moreover, the lncRNA ANRIL, which is altered in periodontitis, is associated with AD-related neuroinflammation by binding to miRNA-125a, leading to its increased expression (Liu et al., 2022). While both MALAT1 and ANRIL are influenced by periodontitis, MALAT1 appears to contribute to neuroprotection, whereas ANRIL has neurodegenerative consequences (Liu et al., 2022). It is important to note that dysfunctions in olfaction can significantly impact mood and behavior. The miRNA-200 family plays a crucial role in olfactory neurogenesis, and there is an increasing interest in the role of the nasal microbiome in olfactory function and its potential connection to neurodegenerative disorders (Choi et al., 2008). Some bacteria in the nasal cavity may have links to the central nervous system. For instance, Chlamydia pneumoniae, associated with sinusitis, has been found in the brains of Alzheimer’s disease patients. Additionally, Corynebacterium diphtheriae, known to produce diphtheria toxin, can enter the CNS, potentially contributing to sporadic AD (Thangaleela et al., 2022a). Dysbiosis in the nasal microbiome may lead to inflammation, exacerbating neurodegeneration (Thangaleela et al., 2022a,b). Non-coding RNAs (ncRNAs), particularly miRNAs, may serve as key mediators in the nasal microbiota-brain axis. Studies have shown that elevated levels of miRNA-206 and inflammatory mediators are observed in human astrocytes exposed to LPS, and in early dementia patients, overexpression of miRNA-206 is associated with suppressed brain-derived neurotropic factor (BDNF), potentially aggravating neuropathogenesis in AD patients (Duan et al., 2015).
6 Human virus infection and Alzheimer’s disease
The role of viruses in neurodegenerative diseases, including AD, is garnering attention. Non-coding RNAs, particularly miRNAs, are crucial in regulating viral replication and infection outcomes. Certain infectious agents, particularly those affecting the central nervous system (CNS), are associated with Alzheimer’s disease (AD) pathology, some of which can remain latent in the CNS and reactivate with age and stress, leading to subsequent inflammation, synaptic dysfunction, and neural loss (Itzhaki et al., 2016).
6.1 Human immunodeficiency viruses
In a study conducted in 2020, HIV-infected patients exhibited elevated BACE1 enzyme expression and increased production of Aβ (Moloney et al., 2017; Zhao and Lukiw, 2018a) in human astrocytes exposed to Tat, an early gene product of HIV-1 continually secreted from latently infected cells. This mechanism involved the upregulation of hypoxia-inducible factors-1-alfa (HIF-1α), leading to the formation of the lncRNA BACE1-antisense (AS/BACE1) RNA complex. Subsequently, the complex increased BACE1 enzyme activity (Sil et al., 2020). Figure 4 illustrates how BACE1-AS functions as a competing endogenous RNA (ceRNA), sequestering miRNA-485-5p and relieving its inhibitory effect on BACE1 mRNA, ultimately resulting in increased BACE1 mRNA expression (Khan et al., 2020; Canseco-Rodriguez et al., 2022; Ryu et al., 2023). Research on lentiviruses in the CNS revealed that these viruses can induce the expression of miRNA-31, which significantly reduces Aβ deposition in the subiculum and hippocampus. This finding suggests a potential role for non-coding RNAs (ncRNAs) in Alzheimer’s disease (AD) neuropathology (Barros-Viegas et al., 2020). Contradictory effects of different lentiviruses on Alzheimer’s disease (AD) call for further comprehensive investigations.
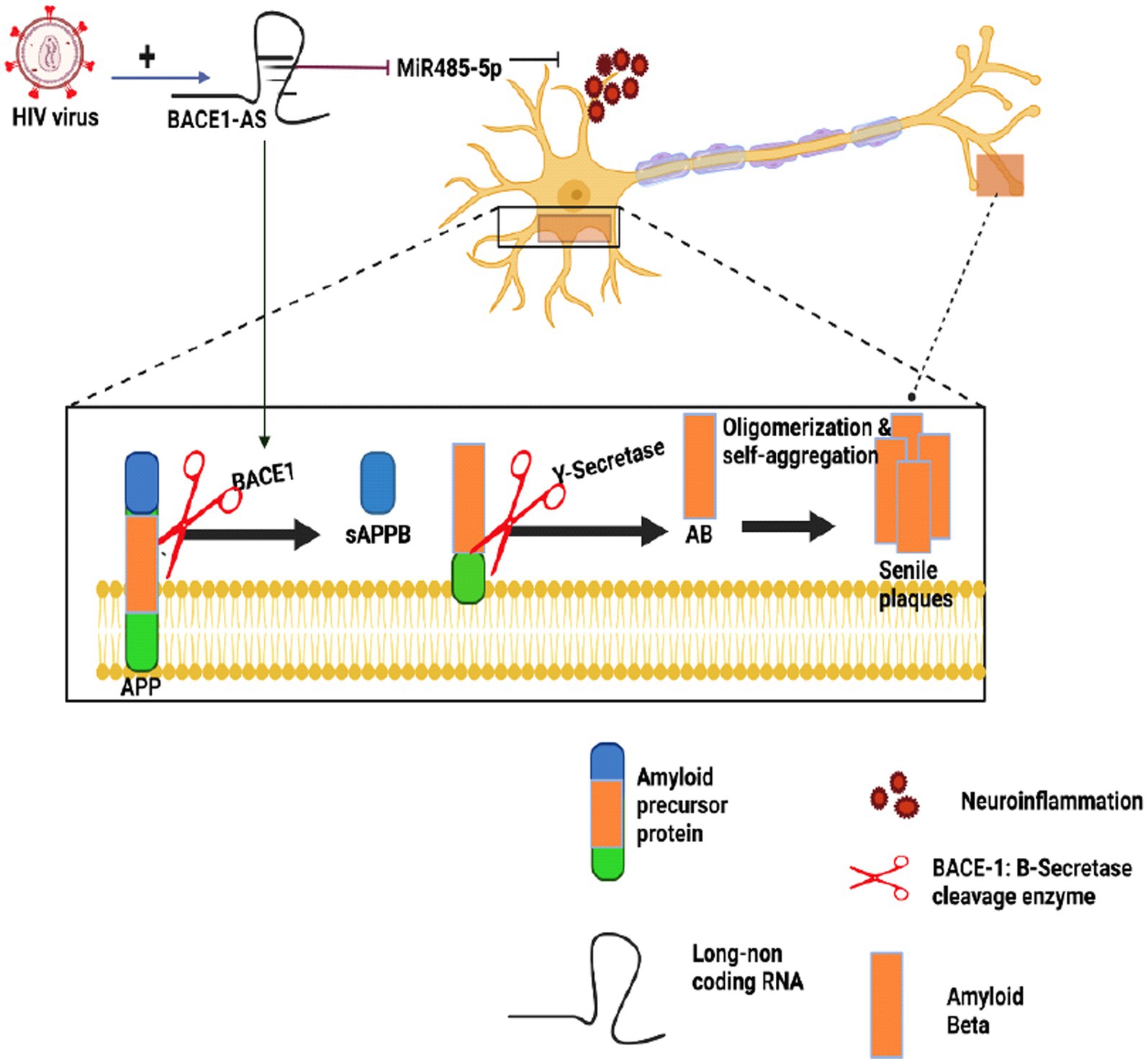
Figure 4. The impact of HIV on Alzheimer’s amyloidogenic pathway. This figure provides an overview of how HIV infection can influence Alzheimer’s disease (AD) mechanisms, particularly the amyloidogenic pathway. BACE1-AS acts as a competing endogenous RNA (ceRNA), sequestering miRNA-485-5p, which in turn, enhances BACE1 mRNA expression, ultimately contributing to Aβ generation in AD. Studies on CNS lentiviruses have revealed their ability to induce the expression of miRNA-31, which reduces Aβ deposition in brain regions such as the subiculum and hippocampus, and this suggests a potential role for non-coding RNAs (ncRNAs) in the pathology of AD. However, due to the intricate and sometimes contradictory effects of diverse lentiviruses on AD, further comprehensive investigations remain essential to fully understand this complex interaction.
6.2 Hepatitis B viruses
In patients infected with hepatitis B virus (HBV), a study observed a significant induction of miRNA-125b-5p in serum, which was associated with the persistence of the HBV infection. Another study revealed that the upregulation of miRNA-125b is linked to an increase in tau protein hyperphosphorylation in AD, which contributes to the progression of the disease (Singh et al., 2018).
6.3 Severe acute respiratory coronavirus 2
In the context of viral infections, it has been reported that during Severe acute respiratory coronavirus 2 (SARS-CoV2) infection, miRNA-200c-3p disrupts the regulation of the angiotensin-converting enzyme (ACE), indirectly inhibiting viral infection (Sodagar et al., 2022). Furthermore, a study in 2022 suggested that miR-200c suppression increases tau hyperphosphorylation by targeting 14-3-3y at the early-stage AD in a mouse model (5xFAD; Park et al., 2022). These findings highlight the bidirectional impact of ncRNAs on microbes in disease development or suppression (Abu-Izneid et al., 2021).
6.4 Herpes simplex viruses
Herpes Simplex Virus type 1 (HSV-1) infection is recognized as a contributing factor to the progression of Alzheimer’s disease (AD). It is found in the latent form within the brains of many older individuals, leading to neural and glial cell damage and immune dysfunction. HSV-1 can enter the CNS without clinical symptoms, and its reactivation can accelerate the pathogenesis of AD (Mielcarska et al., 2021). HSV-1 expresses its own miRNAs abundantly during latency and has the capacity to manipulate host miRNAs to facilitate either replication or latency (Cokarić Brdovčak et al., 2018). The co-evolution of the host and virus enables HSV-1 to hijack host cellular processes for its growth and survival. Non-coding RNAs, particularly miRNAs, play a crucial role in viral pathogenesis by balancing latency and reactivation in response to various stimuli. For example, cellular miRNA-101 targets mitochondrial ATP synthase, reducing the lytic replication of HSV-1 and promoting latency (Zheng et al., 2011). In contrast, miRNA-23a supports viral replication by inhibiting the host’s antiviral innate immune response (Ru et al., 2014). Another study revealed that HSV-1 infection of human neuronal glial cells induced miRNA-146a, which targets key components of the arachidonic acid pathway and fumarate hydratase (FH), enabling HSV-1 to evade the complement system, exacerbating neuropathological changes in AD (Hill et al., 2009; Zhao and Lukiw, 2018a; Slota and Booth, 2019). HSV-1 infection has also been associated with tau hyperphosphorylation, contributing to neurotoxicity in AD brains (Alvarez et al., 2012). In summary, viral and cellular miRNAs and their interplay appear to play a significant role in viral latency and reactivation, thereby influencing virus replication and pathogenesis (Bernier and Sagan, 2018). Consequently, miRNAs are strongly associated with viral-related pathological events (Piedade and Azevedo-Pereira, 2016).
6.5 Human cytomegalovirus
Human cytomegalovirus (HCMV) causes severe disease, especially in individuals with compromised immune systems. It is linked to the early onset of systemic sclerosis and has the capability to enter the CNS, causing inflammation and histopathological changes in various brain cells. CMV-induced inflammation mediated by the host can exacerbate immunopathology and neuropathological symptoms (Krstanović et al., 2021). Studies have reported several AD pathological hallmarks associated with neuro-invasive HCMV, including the accumulation of amyloid-beta (Aβ), astrogliosis, neuroinflammation, and neural damage. In vitro experiments have shown that HCMV can induce Aβ production and astrocyte reactivity, resembling AD pathological features (Adelman et al., 2023). HCMV encodes its own microRNAs (miRNAs) that target viral genes and host cellular ones, including immunomodulators. Additionally, it can modify cellular miRNAs to facilitate their replication (Dhuruvasan et al., 2011). HCMV can manipulate various cellular mechanisms to its advantage, affecting host metabolic pathways and cell apoptosis (Das et al., 2007; Leng et al., 2017). Notably, downregulation of the miRNA-199a/214 cluster has been observed in HCMV-infected cells (Dhuruvasan et al., 2011). In a study from 2020, miRNA-199a was found to decrease neuritin expression, contributing to AD progression in mouse models. Researchers noted significant miRNA-199a expression at the early stages of AD, suggesting its potential as a biomarker for early disease diagnosis (Song et al., 2020).
7 Conclusion
The early diagnosis of Alzheimer’s disease is critical for delaying or mitigating its progression. Non-coding RNAs (ncRNAs) present in blood circulation and the cerebrospinal fluid hold promise as reliable diagnostic biomarkers due to their stability and ease of detection. Moreover, ncRNAs have therapeutic potential, particularly in the preclinical stages of the disease. However, the development of effective drugs remains challenging due to a limited understanding of AD pathogenesis, and current drugs only offer symptomatic relief. Therefore, in-depth research into the causes of AD is essential. The regulatory role of ncRNAs in various human diseases, including the potential influence of microorganisms within the human body on host ncRNAs, presents a promising avenue for investigation. Studying the molecular basis of AD pathology, especially the mechanisms involving ncRNAs, is crucial for advancing rational treatment approaches. Accumulated amyloid-beta (Aβ), a hallmark of AD, can trigger microglia activation and subsequent neuroinflammation, with many ncRNAs, including miRNAs, playing key roles as modulators of neuroinflammation. Some ncRNAs also regulate enzymes involved in Aβ processing, highlighting their potential impact on AD pathology. Dysregulations of these ncRNAs, influenced by external factors such as viruses and bacteria, can significantly alter disease trajectories. Recent research has shed light on the role of gut and nasal microbiota in neurodegenerative diseases, emphasizing the role of ncRNAs in modulating immune responses and inflammation. Investigating the profiles of ncRNAs responsible for controlling pro-inflammatory genes associated with AD is essential for effective treatment or early disease diagnosis. Perturbations in neuroinflammation-related ncRNAs within various microbiome communities may lead to the dysregulation of specific signal transduction pathways, contributing to disease development. To improve treatment and diagnosis methodologies for Alzheimer’s disease, further research is needed to explore the roles of ncRNAs in regulating genes involved in the progression of the disease.
Author contributions
HM-P: Visualization, Writing – original draft, Conceptualization. MS: Supervision, Writing – review & editing, Conceptualization, Project administration. FJ: Data curation, Supervision, Writing – review & editing. HN: Data curation, Writing – review & editing. FM-G: Writing – review & editing, Supervision.
Acknowledgments
The authors gratefully acknowledge the support from contributors to this study.
Conflict of interest
The authors declare that the research was conducted in the absence of any commercial or financial relationships that could be construed as a potential conflict of interest.
Publisher’s note
All claims expressed in this article are solely those of the authors and do not necessarily represent those of their affiliated organizations, or those of the publisher, the editors and the reviewers. Any product that may be evaluated in this article, or claim that may be made by its manufacturer, is not guaranteed or endorsed by the publisher.
References
Abu-Izneid, T., AlHajri, N., Ibrahim, A. M., Javed, M. N., Salem, K. M., Pottoo, F. H., et al. (2021). Micro-RNAs in the regulation of immune response against SARS CoV-2 and other viral infections. J. Adv. Res. 30, 133–145. doi: 10.1016/j.jare.2020.11.013
Adelman, J. W., Rosas-Rogers, S., Schumacher, M. L., Mokry, R. L., Terhune, S. S., and Ebert, A. D. Human Cytomegalovirus Induces Significant Structural and Functional Changes in Terminally Differentiated Human Cortical Neurons. bioRxiv [Preprint]. (2023).
Ahmed, H., Leyrolle, Q., Koistinen, V., Kärkkäinen, O., Layé, S., Delzenne, N., et al. (2022). Microbiota-derived metabolites as drivers of gut-brain communication. Gut Microbes 14:2102878. doi: 10.1080/19490976.2022.2102878
Alqahtani, T., Deore, S. L., Kide, A. A., Shende, B. A., Sharma, R., Dadarao Chakole, R., et al. (2023). Mitochondrial dysfunction and oxidative stress in Alzheimer’s disease, and Parkinson’s disease, Huntington’s disease and amyotrophic lateral sclerosis-an updated review. Mitochondrion 71, 83–92. doi: 10.1016/j.mito.2023.05.007
Alvarez, G., Aldudo, J., Alonso, M., Santana, S., and Valdivieso, F. (2012). Herpes simplex virus type 1 induces nuclear accumulation of hyperphosphorylated tau in neuronal cells. J. Neurosci. Res. 90, 1020–1029. doi: 10.1002/jnr.23003
Aragón-González, A., Shaw, P. J., and Ferraiuolo, L. (2022). Blood-brain barrier disruption and its involvement in neurodevelopmental and neurodegenerative disorders. Int. J. Mol. Sci. 23:5271. doi: 10.3390/ijms232315271
Araki, W. (2023). Aβ oligomer toxicity-reducing therapy for the prevention of Alzheimer's disease: importance of the Nrf2 and PPARγ pathways. Cells 12:1386. doi: 10.3390/cells12101386
Arzua, T., Jiang, C., Yan, Y., and Bai, X. (2021). The importance of non-coding RNAs in environmental stress-related developmental brain disorders: a systematic review of evidence associated with exposure to alcohol, anesthetic drugs, nicotine, and viral infections. Neurosci. Biobehav. Rev. 128, 633–647. doi: 10.1016/j.neubiorev.2021.06.033
Bangasser, D. A., Dong, H., Carroll, J., Plona, Z., Ding, H., Rodriguez, L., et al. (2017). Corticotropin-releasing factor overexpression gives rise to sex differences in Alzheimer's disease-related signaling. Mol. Psychiatry 22, 1126–1133. doi: 10.1038/mp.2016.185
Barandouzi, Z. A., Starkweather, A. R., Henderson, W. A., Gyamfi, A., and Cong, X. S. (2020). Altered composition of gut microbiota in depression: a systematic review. Front. Psych. 11:541. doi: 10.3389/fpsyt.2020.00541
Barichello, T., Generoso, J. S., Milioli, G., Elias, S. G., and Teixeira, A. L. (2013). Pathophysiology of bacterial infection of the central nervous system and its putative role in the pathogenesis of behavioral changes. Braz J Psychiatry. 35, 81–87. doi: 10.1016/j.rbp.2012.11.003
Barros-Viegas, A. T., Carmona, V., Ferreiro, E., Guedes, J., Cardoso, A. M., Cunha, P., et al. (2020). miRNA-31 improves cognition and abolishes amyloid-β pathology by targeting APP and BACE1 in an animal model of Alzheimer's disease. Mol Ther Nucleic Acids. 19, 1219–1236. doi: 10.1016/j.omtn.2020.01.010
Bashir, Y., and Khan, A. U. (2022). The interplay between the gut-brain axis and the microbiome: a perspective on psychiatric and neurodegenerative disorders. Front. Neurosci. 16:1030694. doi: 10.3389/fnins.2022.1030694
Bernabeu-Zornoza, A., Coronel, R., Palmer, C., Martín, A., López-Alonso, V., and Liste, I. (2022). Neurogenesis is increased in human neural stem cells by Aβ40 peptide. Int. J. Mol. Sci. 23:5820. doi: 10.3390/ijms23105820
Bernier, A., and Sagan, S. M. (2018). The diverse roles of microRNAs at the host−Virus Interface. Viruses 10:440. doi: 10.3390/v10080440
Bhattacharyya, N., Pandey, V., Bhattacharyya, M., and Dey, A. (2021). Regulatory role of long non coding RNAs (lncRNAs) in neurological disorders: from novel biomarkers to promising therapeutic strategies. Asian J Pharm Sci. 16, 533–550. doi: 10.1016/j.ajps.2021.02.006
Bostanciklioğlu, M. (2019). The role of gut microbiota in pathogenesis of Alzheimer's disease. J. Appl. Microbiol. 127, 954–967. doi: 10.1111/jam.14264
Candido, S., Lupo, G., Pennisi, M., Basile, M. S., Anfuso, C. D., Petralia, M. C., et al. (2019). The analysis of miRNA expression profiling datasets reveals inverse microRNA patterns in glioblastoma and Alzheimer's disease. Oncol. Rep. 42, 911–922. doi: 10.3892/or.2019.7215
Canseco-Rodriguez, A., Masola, V., Aliperti, V., Meseguer-Beltran, M., Donizetti, A., and Sanchez-Perez, A. M. (2022). Long non-coding RNAs, extracellular vesicles and inflammation in Alzheimer's disease. Int. J. Mol. Sci. 23:3171. doi: 10.3390/ijms232113171
Cao, M., Li, H., Zhao, J., Cui, J., and Hu, G. (2019). Identification of age-and gender-associated long noncoding RNAs in the human brain with Alzheimer's disease. Neurobiol. Aging 81, 116–126. doi: 10.1016/j.neurobiolaging.2019.05.023
Carthew, R. W., and Sontheimer, E. J. (2009). Origins and mechanisms of miRNAs and siRNAs. Cells 136, 642–655. doi: 10.1016/j.cell.2009.01.035
Castro-Aldrete, L., Moser, M. V., Putignano, G., Ferretti, M. T., Schumacher Dimech, A., and Santuccione, C. A. (2023). Sex and gender considerations in Alzheimer's disease: the Women's brain project contribution. Front. Aging Neurosci. 15:1105620. doi: 10.3389/fnagi.2023.1105620
Chandra, S., Sisodia, S. S., and Vassar, R. J. (2023). The gut microbiome in Alzheimer's disease: what we know and what remains to be explored. Mol. Neurodegener. 18:9. doi: 10.1186/s13024-023-00595-7
Choi, P. S., Zakhary, L., Choi, W. Y., Caron, S., Alvarez-Saavedra, E., Miska, E. A., et al. (2008). Members of the miRNA-200 family regulate olfactory neurogenesis. Neuron 57, 41–55. doi: 10.1016/j.neuron.2007.11.018
Cokarić Brdovčak, M., Zubković, A., and Jurak, I. (2018). Herpes simplex virus 1 deregulation of host MicroRNAs. Noncoding. RNA 4:36. doi: 10.3390/ncrna4040036
Das, T., Das, T. K., Khodarkovskaya, A., and Dash, S. (2021). Non-coding RNAs and their bioengineering applications for neurological diseases. Bioengineered. 12, 11675–11698. doi: 10.1080/21655979.2021.2003667
Das, S., Vasanji, A., and Pellett, P. E. (2007). Three-dimensional structure of the human cytomegalovirus cytoplasmic virion assembly complex includes a reoriented secretory apparatus. J. Virol. 81, 11861–11869. doi: 10.1128/JVI.01077-07
Datta, M., Staszewski, O., Raschi, E., Frosch, M., Hagemeyer, N., Tay, T. L., et al. (2018). Histone deacetylases 1 and 2 regulate microglia function during development, homeostasis, and neurodegeneration in a context-dependent manner. Immunity 48, 514–29.e6. doi: 10.1016/j.immuni.2018.02.016
Dhuruvasan, K., Sivasubramanian, G., and Pellett, P. E. (2011). Roles of host and viral microRNAs in human cytomegalovirus biology. Virus Res. 157, 180–192. doi: 10.1016/j.virusres.2010.10.011
Dominy, S. S., Lynch, C., Ermini, F., Benedyk, M., Marczyk, A., Konradi, A., et al. (2019). Porphyromonas gingivalis in Alzheimer's disease brains: evidence for disease causation and treatment with small-molecule inhibitors. Sci. Adv. 5:eaau3333. doi: 10.1126/sciadv.aau3333
Dorszewska, J., Prendecki, M., Oczkowska, A., Dezor, M., and Kozubski, W. (2016). Molecular basis of familial and sporadic Alzheimer's disease. Curr. Alzheimer Res. 13, 952–963. doi: 10.2174/1567205013666160314150501
Duan, X., Zohaib, A., Li, Y., Zhu, B., Ye, J., Wan, S., et al. (2015). miR-206 modulates lipopolysaccharide-mediated inflammatory cytokine production in human astrocytes. Cell. Signal. 27, 61–68. doi: 10.1016/j.cellsig.2014.10.006
El Hayek, L., Khalifeh, M., Zibara, V., Abi Assaad, R., Emmanuel, N., Karnib, N., et al. (2019). Lactate mediates the effects of exercise on learning and memory through SIRT1-dependent activation of hippocampal brain-derived neurotrophic factor (BDNF). J. Neurosci. 39, 2369–2382. doi: 10.1523/JNEUROSCI.1661-18.2019
Fišar, Z. (2022). Linking the amyloid, tau, and mitochondrial hypotheses of Alzheimer's disease and identifying promising drug targets. Biomol. Ther. 12:1676. doi: 10.3390/biom12111676
Fu, X. D. (2014). Non-coding RNA: a new frontier in regulatory biology. Natl. Sci. Rev. 1, 190–204. doi: 10.1093/nsr/nwu008
Gholami, L., Ghafouri-Fard, S., Mirzajani, S., Arsang-Jang, S., Taheri, M., Dehbani, Z., et al. (2020). The lncRNA ANRIL is down-regulated in peripheral blood of patients with periodontitis. Noncoding RNA Res. 5, 60–66. doi: 10.1016/j.ncrna.2020.04.001
Gomes, A. Q., Nolasco, S., and Soares, H. (2013). Non-coding RNAs: multi-tasking molecules in the cell. Int. J. Mol. Sci. 14, 16010–16039. doi: 10.3390/ijms140816010
Guo, T., Noble, W., and Hanger, D. P. (2017). Roles of tau protein in health and disease. Acta Neuropathol. 133, 665–704. doi: 10.1007/s00401-017-1707-9
Gurtan, A. M., and Sharp, P. A. (2013). The role of miRNAs in regulating gene expression networks. J. Mol. Biol. 425, 3582–3600. doi: 10.1016/j.jmb.2013.03.007
Hao, Y., Xie, B., Fu, X., Xu, R., and Yang, Y. (2022). New insights into lncRNAs in Aβ Cascade hypothesis of Alzheimer's disease. Biomol. Ther. 12:1802. doi: 10.3390/biom12121802
Hasavci, D., and Blank, T. (2022). Age-dependent effects of gut microbiota metabolites on brain resident macrophages. Front. Cell. Neurosci. 16:944526. doi: 10.3389/fncel.2022.944526
Hill, J. M., Zhao, Y., Clement, C., Neumann, D. M., and Lukiw, W. J. (2009). HSV-1 infection of human brain cells induces miRNA-146a and Alzheimer-type inflammatory signaling. Neuroreport 20, 1500–1505. doi: 10.1097/WNR.0b013e3283329c05
Hou, K., Wu, Z. X., Chen, X. Y., Wang, J. Q., Zhang, D., Xiao, C., et al. (2022). Microbiota in health and diseases. Signal Transduct. Target. Ther. 7:135. doi: 10.1038/s41392-022-00974-4
Huang, F., and Wu, X. (2021). Brain neurotransmitter modulation by gut microbiota in anxiety and depression. Front. Cell Dev. Biol. 9:649103. doi: 10.3389/fcell.2021.649103
Ishida, N., Ishihara, Y., Ishida, K., Tada, H., Funaki-Kato, Y., Hagiwara, M., et al. (2017). Periodontitis induced by bacterial infection exacerbates features of Alzheimer's disease in transgenic mice. NPJ Aging Mech Dis. 3:15. doi: 10.1038/s41514-017-0015-x
Itzhaki, R. F., Lathe, R., Balin, B. J., Ball, M. J., Bearer, E. L., Braak, H., et al. (2016). Microbes and Alzheimer's disease. J. Alzheimers Dis. 51, 979–984. doi: 10.3233/JAD-160152
Johnson, D., Letchumanan, V., Thum, C. C., Thurairajasingam, S., and Lee, L. H. (2023). A microbial-based approach to mental health: the potential of probiotics in the treatment of depression. Nutrients 15:1382. doi: 10.3390/nu15061382
Kaur, H., Bose, C., and Mande, S. S. (2019). Tryptophan metabolism by gut microbiome and gut-brain-Axis: an in silico analysis. Front. Neurosci. 13:1365. doi: 10.3389/fnins.2019.01365
Khan, S., Barve, K. H., and Kumar, M. S. (2020). Recent advancements in pathogenesis, diagnostics and treatment of Alzheimer's disease. Curr. Neuropharmacol. 18, 1106–1125. doi: 10.2174/1570159X18666200528142429
Kim, K. W. (2019). PIWI proteins and piRNAs in the nervous system. Mol. Cells 42, 828–835. doi: 10.14348/molcells.2019.0241
Krstanović, F., Britt, W. J., Jonjić, S., and Brizić, I. (2021). Cytomegalovirus infection and inflammation in developing brain. Viruses 13:1078. doi: 10.3390/v13061078
Leblhuber, F., Ehrlich, D., Steiner, K., Geisler, S., Fuchs, D., Lanser, L., et al. (2021). The immunopathogenesis of Alzheimer's disease is related to the composition of gut microbiota. Nutrients 13:361. doi: 10.3390/nu13020361
Leng, S. X., Kamil, J., Purdy, J. G., Lemmermann, N. A., Reddehase, M. J., and Goodrum, F. D. (2017). Recent advances in CMV tropism, latency, and diagnosis during aging. Geroscience. 39, 251–259. doi: 10.1007/s11357-017-9985-7
Li, F., Hearn, M., and Bennett, L. E. (2021). The role of microbial infection in the pathogenesis of Alzheimer's disease and the opportunity for protection by anti-microbial peptides. Crit. Rev. Microbiol. 47, 240–253. doi: 10.1080/1040841X.2021.1876630
Li, X., Wang, H., Zhang, Y., Zhang, J., Qi, S., Zhang, Y., et al. (2019). Overexpression of lncRNA H19 changes basic characteristics and affects immune response of bovine mammary epithelial cells. PeerJ. 7:e6715. doi: 10.7717/peerj.8265
Liu, Y., Cheng, X., Li, H., Hui, S., Zhang, Z., Xiao, Y., et al. (2022). Non-coding RNAs as novel regulators of neuroinflammation in Alzheimer's disease. Front. Immunol. 13:908076. doi: 10.3389/fimmu.2022.947136
Lombardi, G., Crescioli, G., Cavedo, E., Lucenteforte, E., Casazza, G., Bellatorre, A. G., et al. (2020). Structural magnetic resonance imaging for the early diagnosis of dementia due to Alzheimer's disease in people with mild cognitive impairment. Cochrane Database Syst. Rev. 3:Cd009628. doi: 10.1002/14651858.CD009628.pub2
Lukiw, W. J., Arceneaux, L., Li, W., Bond, T., and Zhao, Y. (2021). Gastrointestinal (GI)-tract microbiome derived neurotoxins and their potential contribution to inflammatory neurodegeneration in Alzheimer's disease (AD). J Alzheimers Dis Parkinsonism. 11:525.
Luo, Q., and Chen, Y. (2016). Long noncoding RNAs and Alzheimer's disease. Clin. Interv. Aging 11, 867–872. doi: 10.2147/CIA.S107037
Lyketsos, C. G., Carrillo, M. C., Ryan, J. M., Khachaturian, A. S., Trzepacz, P., Amatniek, J., et al. (2011). Neuropsychiatric symptoms in Alzheimer's disease. Alzheimers Dement. 7, 532–539. doi: 10.1016/j.jalz.2011.05.2410
Ma, L., Bajic, V. B., and Zhang, Z. (2013). On the classification of long non-coding RNAs. RNA Biol. 10, 925–933. doi: 10.4161/rna.24604
Mielcarska, M. B., Skowrońska, K., Wyżewski, Z., and Toka, F. N. (2021). Disrupting neurons and glial cells oneness in the brain-the possible causal role of herpes simplex virus type 1 (HSV-1) in Alzheimer's disease. Int. J. Mol. Sci. 23:242. doi: 10.3390/ijms23010242
Miguel, V., Lamas, S., and Espinosa-Diez, C. (2020). Role of non-coding-RNAs in response to environmental stressors and consequences on human health. Redox Biol. 37:101580:101580. doi: 10.1016/j.redox.2020.101580
Moloney, G. M., O’Leary OF, Salvo-Romero, E., Desbonnet, L., Shanahan, F., Dinan, T. G., et al. (2017). Microbial regulation of hippocampal miRNA expression: implications for transcription of kynurenine pathway enzymes. Behav. Brain Res. 334, 50–54. doi: 10.1016/j.bbr.2017.07.026
Ni, Y. Q., Xu, H., and Liu, Y. S. (2022). Roles of long non-coding RNAs in the development of aging-related neurodegenerative diseases. Front. Mol. Neurosci. 15:844193. doi: 10.3389/fnmol.2022.844193
Niu, Y., Mo, D., Qin, L., Wang, C., Li, A., Zhao, X., et al. (2011). Lipopolysaccharide-induced miR-1224 negatively regulates tumour necrosis factor-α gene expression by modulating Sp1. Immunology 133, 8–20. doi: 10.1111/j.1365-2567.2010.03374.x
Park, H., Lee, Y. B., and Chang, K. A. (2022). miR-200c suppression increases tau hyperphosphorylation by targeting 14-3-3γ in early stage of 5xFAD mouse model of Alzheimer's disease. Int. J. Biol. Sci. 18, 2220–2234. doi: 10.7150/ijbs.66604
Piedade, D., and Azevedo-Pereira, J. M. (2016). The role of microRNAs in the pathogenesis of herpesvirus infection. Viruses 8:156. doi: 10.3390/v8060156
Pluta, R., Ułamek-Kozioł, M., Januszewski, S., and Czuczwar, S. J. (2020). Gut microbiota and pro/prebiotics in Alzheimer's disease. Aging 12, 5539–5550. doi: 10.18632/aging.102930
Romanenko, M., Kholin, V., Koliada, A., and Vaiserman, A. (2021). Nutrition, gut microbiota, and Alzheimer's disease. Front. Psych. 12:712673. doi: 10.3389/fpsyt.2021.712673
Rosa, J. M., Formolo, D. A., Yu, J., Lee, T. H., and Yau, S. Y. (2022). The role of MicroRNA and microbiota in depression and anxiety. Front. Behav. Neurosci. 16:828258. doi: 10.3389/fnbeh.2022.828258
Ru, J., Sun, H., Fan, H., Wang, C., Li, Y., Liu, M., et al. (2014). MiR-23a facilitates the replication of HSV-1 through the suppression of interferon regulatory factor 1. PloS One 9:e114021. doi: 10.1371/journal.pone.0114021
Ruiz-Orera, J., and Albà, M. M. (2019). Conserved regions in long non-coding RNAs contain abundant translation and protein-RNA interaction signatures. NAR Genom Bioinform. 1:e2. doi: 10.1093/nargab/lqz002
Ryu, I. S., Kim, D. H., Cho, H. J., and Ryu, J. H. (2023). The role of microRNA-485 in neurodegenerative diseases. Rev. Neurosci. 34, 49–62. doi: 10.1515/revneuro-2022-0039
Sampson, T. R., Debelius, J. W., Thron, T., Janssen, S., Shastri, G. G., Ilhan, Z. E., et al. (2016). Gut microbiota regulate motor deficits and neuroinflammation in a model of Parkinson's disease. Cells 167, 1469–1480. doi: 10.1016/j.cell.2016.11.018
Sato, K., Takayama, K. I., and Inoue, S. (2023). Role of piRNA biogenesis and its neuronal function in the development of neurodegenerative diseases. Front. Aging Neurosci. 15:1157818. doi: 10.3389/fnagi.2023.1157818
Sayad, A., Najafi, S., Hussen, B. M., Abdullah, S. T., Movahedpour, A., Taheri, M., et al. (2022). The emerging roles of the β-secretase BACE1 and the long non-coding RNA BACE1-AS in human diseases: a focus on neurodegenerative diseases and cancer. Front. Aging Neurosci. 14:853180. doi: 10.3389/fnagi.2022.853180
Sil, S., Hu, G., Liao, K., Niu, F., Callen, S., Periyasamy, P., et al. (2020). HIV-1 tat-mediated astrocytic amyloidosis involves the HIF-1α/lncRNA BACE1-AS axis. PLoS Biol. 18:e3000660. doi: 10.1371/journal.pbio.3000660
Silzer, T. K., Pathak, G. A., and Phillips, N. R. (2020). Mitochondrial tRNA methylation in Alzheimer's disease and progressive supranuclear palsy. BMC Med. Genet. 13:71. doi: 10.1186/s12920-020-0727-9
Singh, A. K., Rooge, S. B., Varshney, A., Vasudevan, M., Bhardwaj, A., Venugopal, S. K., et al. (2018). Global microRNA expression profiling in the liver biopsies of hepatitis B virus-infected patients suggests specific microRNA signatures for viral persistence and hepatocellular injury. Hepatology 67, 1695–1709. doi: 10.1002/hep.29690
Slota, J. A., and Booth, S. A. (2019). MicroRNAs in neuroinflammation: implications in disease pathogenesis, biomarker discovery and therapeutic applications. Noncoding RNA 5:35. doi: 10.3390/ncrna5020035
Sochocka, M., Donskow-Łysoniewska, K., Diniz, B. S., Kurpas, D., Brzozowska, E., and Leszek, J. (2019). The gut microbiome alterations and inflammation-driven pathogenesis of Alzheimer's disease-a critical review. Mol. Neurobiol. 56, 1841–1851. doi: 10.1007/s12035-018-1188-4
Sodagar, H., Alipour, S., Hassani, S., Aziz, S. G., Ansari, M. H. K., and Asghari, R. (2022). The role of microRNAs in COVID-19 with a focus on miR-200c. J Circ Biomark. 11, 14–23. doi: 10.33393/jcb.2022.2356
Song, D., Li, G., Hong, Y., Zhang, P., Zhu, J., Yang, L., et al. (2020). miR-199a decreases Neuritin expression involved in the development of Alzheimer's disease in APP/PS1 mice. Int. J. Mol. Med. 46, 384–396. doi: 10.3892/ijmm.2020.4602
Statello, L., Guo, C.-J., Chen, L.-L., and Huarte, M. (2021). Gene regulation by long non-coding RNAs and its biological functions. Nat. Rev. Mol. Cell Biol. 22, 96–118. doi: 10.1038/s41580-020-00315-9
Swer, N. M., Venkidesh, B. S., Murali, T. S., and Mumbrekar, K. D. (2023). Gut microbiota-derived metabolites and their importance in neurological disorders. Mol. Biol. Rep. 50, 1663–1675. doi: 10.1007/s11033-022-08038-0
Tang, W., Zhu, H., Feng, Y., Guo, R., and Wan, D. (2020). The impact of gut microbiota disorders on the blood-brain barrier. Infect Drug Resist. 13, 3351–3363. doi: 10.2147/IDR.S254403
Thangaleela, S., Sivamaruthi, B. S., Kesika, P., Bharathi, M., and Chaiyasut, C. (2022a). Nasal microbiota, olfactory health, neurological disorders and aging: a review. Microorganisms. 10:1405. doi: 10.3390/microorganisms10071405
Thangaleela, S., Sivamaruthi, B. S., Kesika, P., and Chaiyasut, C. (2022b). Role of probiotics and diet in the Management of Neurological Diseases and Mood States: a review. Microorganisms. 10:2268. doi: 10.3390/microorganisms10112268
Valenza, M., and Scuderi, C. (2022). How useful are biomarkers for the diagnosis of Alzheimer's disease and especially for its therapy? Neural Regen. Res. 17, 2205–2207. doi: 10.4103/1673-5374.335791
Wang, G. Q., Wang, Y., Xiong, Y., Chen, X. C., Ma, M. L., Cai, R., et al. (2016). Sirt1 AS lncRNA interacts with its mRNA to inhibit muscle formation by attenuating function of miR-34a. Sci. Rep. 6:21865.
Wu, W., Lee, I., Spratt, H., Fang, X., and Bao, X. (2021). tRNA-derived fragments in Alzheimer's disease: implications for new disease biomarkers and neuropathological mechanisms. J. Alzheimers Dis. 79, 793–806. doi: 10.3233/JAD-200917
Xue, X., Feng, T., Yao, S., Wolf, K. J., Liu, C. G., Liu, X., et al. (2011). Microbiota downregulates dendritic cell expression of miR-10a, which targets IL-12/IL-23p40. J. Immunol. 187, 5879–5886. doi: 10.4049/jimmunol.1100535
Zhang, Y., Geng, R., and Tu, Q. (2021). Gut microbial involvement in Alzheimer's disease pathogenesis. Aging 13, 13359–13371. doi: 10.18632/aging.202994
Zhang, M., He, P., and Bian, Z. (2021). Long noncoding RNAs in neurodegenerative diseases: pathogenesis and potential implications as clinical biomarkers. Front. Mol. Neurosci. 14:685143. doi: 10.3389/fnmol.2021.685143
Zhang, P., Wu, W., Chen, Q., and Chen, M. (2019). Non-coding RNAs and their integrated networks. J. Integr. Bioinform. 16:27. doi: 10.1515/jib-2019-0027
Zhao, Y., and Lukiw, W. J. (2018a). Microbiome-mediated upregulation of MicroRNA-146a in sporadic Alzheimer’s disease. Front. Neurol. 9:649103. doi: 10.3389/fneur.2018.00145
Zhao, Y., and Lukiw, W. J. (2018b). Bacteroidetes neurotoxins and inflammatory neurodegeneration. Mol. Neurobiol. 55, 9100–9107. doi: 10.1007/s12035-018-1015-y
Zhao, Y., Wang, Z., Mao, Y., Li, B., Zhu, Y., Zhang, S., et al. (2020). NEAT1 regulates microtubule stabilization via FZD3/GSK3β/P-tau pathway in SH-SY5Y cells and APP/PS1 mice. Aging 12, 23233–23250. doi: 10.18632/aging.104098
Zhao, Y., Zeng, Y., Zeng, D., Wang, H., Zhou, M., Sun, N., et al. (2021). Probiotics and MicroRNA: their roles in the host–microbe interactions. Front. Microbiol. 11:604462. doi: 10.3389/fmicb.2020.604462
Zheng, S. Q., Li, Y. X., Zhang, Y., Li, X., and Tang, H. (2011). MiR-101 regulates HSV-1 replication by targeting ATP5B. Antiviral Res. 89, 219–226. doi: 10.1016/j.antiviral.2011.01.008
Keywords: Alzheimer’s disease, non-coding RNAs, gut microbiome, viral disease, gut–brain axis, neurodegenerative disorders
Citation: Mohammadi-Pilehdarboni H, Shenagari M, Joukar F, Naziri H and Mansour-Ghanaei F (2024) Alzheimer’s disease and microorganisms: the non-coding RNAs crosstalk. Front. Cell. Neurosci. 17:1256100. doi: 10.3389/fncel.2023.1256100
Edited by:
Nouha Bouayed Abdelmoula, University of Sfax, TunisiaReviewed by:
Sabrina Petralla, Heidelberg University, GermanySaivageethi Nuthikattu, University of California, Davis, United States
Copyright © 2024 Mohammadi-Pilehdarboni, Shenagari, Joukar, Naziri and Mansour-Ghanaei. This is an open-access article distributed under the terms of the Creative Commons Attribution License (CC BY). The use, distribution or reproduction in other forums is permitted, provided the original author(s) and the copyright owner(s) are credited and that the original publication in this journal is cited, in accordance with accepted academic practice. No use, distribution or reproduction is permitted which does not comply with these terms.
*Correspondence: Mohammad Shenagari, c2hlbmFnYXJpQGdtYWlsLmNvbQ==; Farahnaz Joukar, ZmFyYWpvdkBnbWFpbC5jb20=