- 1Department of Neurophysiology, Hirosaki University Graduate School of Medicine, Hirosaki, Japan
- 2Department of Neurophysiology, Hamamatsu University School of Medicine, Hamamatsu, Japan
During brain and spinal cord development, GABA and glycine, the inhibitory neurotransmitters, cause depolarization instead of hyperpolarization in adults. Since glycine and GABAA receptors (GABAARs) are chloride (Cl–) ion channel receptor, the conversion of GABA/glycine actions during development is influenced by changes in the transmembrane Cl– gradient, which is regulated by Cl– transporters, NKCC1 (absorption) and KCC2 (expulsion). In immature neurons, inhibitory neurotransmitters are released in a non-vesicular/non-synaptic manner, transitioning to vesicular/synaptic release as the neuron matures. In other word, in immature neurons, neurotransmitters generally act tonically. Thus, the glycine/GABA system is a developmentally multimodal system that is required for neurogenesis, differentiation, migration, and synaptogenesis. The endogenous agonists for these receptors are not fully understood, we address taurine. In this review, we will discuss about the properties and function of taurine during development of neocortex. Taurine cannot be synthesized by fetuses or neonates, and is transferred from maternal blood through the placenta or maternal milk ingestion. In developing neocortex, taurine level is higher than GABA level, and taurine tonically activates GABAARs to control radial migration as a stop signal. In the marginal zone (MZ) of the developing neocortex, endogenous taurine modulates the spread of excitatory synaptic transmission, activating glycine receptors (GlyRs) as an endogenous agonist. Thus, taurine affects information processing and crucial developmental processes such as axonal growth, cell migration, and lamination in the developing cerebral cortex. Additionally, we also refer to the possible mechanism of taurine-regulating Cl– homeostasis. External taurine is uptake by taurine transporter (TauT) and regulates NKCC1 and KCC2 mediated by intracellular signaling pathway, with-no-lysine kinase 1 (WNK1) and its subsequent kinases STE20/SPS1-related proline-alanine-rich protein kinase (SPAK) and oxidative stress response kinase-1 (OSR1). Through the regulation of NKCC1 and KCC2, mediated by the WNK-SPAK/OSR1 signaling pathway, taurine plays a role in maintaining Cl– homeostasis during normal brain development.
Introduction
Taurine (2-aminoethane-1-sulfonic acid), a sulfur-containing amino acid, is the most abundant amino acid in the central nervous system (CNS) and has been extensively studied (Kontro et al., 1984; Huxtable, 1989). Taurine functions as a partial activator of GABAAR and induces Cl– currents in neuronal cells (Ye et al., 1997). Compared to the adult brain, the immature brain contains higher levels of taurine, despite the limited ability to produce taurine during fetal development (Kaczmarek, 1976; Hayes and Sturman, 1981; Stipanuk et al., 1984; Ghisolfi, 1987; Benitez-Diaz et al., 2003). In mammals, taurine, an essential nutrient for fetal development, is acquired from the maternal source via the placenta during gestation, and neonates receive taurine through maternal milk ingestion (Sturman et al., 1977; Sturman, 1981). In addition, taurine concentrations are significantly higher in umbilical venous plasma than in the maternal artery, and taurine acts as a trophic factor and neuromodulator in the development of the CNS (Bernardi, 1985; Michel et al., 1994; Cetine et al., 1996; Chen et al., 1998). In kittens with a taurine deficiency, it was reported that a delay in the migration of granule cells from the outer layer of the cerebellum to the inner layers (Sturman et al., 1985). In addition to physiological functions of taurine in the developing brain, which have been established in previous studies, we demonstrated that endogenous taurine plays a role in activating GABAARs and influencing radial migration in the developing cerebral cortex (Furukawa et al., 2014).
In the developing brain, the primary inhibitory neurotransmitter GABA elicits depolarization, while in the adult brain, it induces hyperpolarization. This switch in the effects of GABA from depolarization (resulting in Cl– efflux) to hyperpolarization (resulting in Cl– influx) during development is attributed to changes in the Cl– gradient across the cell membrane. The regulation of this gradient involves cation-chloride cotransporters, such as NKCC1 (which facilitates Cl– uptake) and KCC2 (which promotes Cl– extrusion), specifically expressed in neurons. During the early stages of neuronal development, GABA is released through non-vesicular and non-synaptic mechanisms (Owens and Kriegstein, 2002; Manent et al., 2005). Consequently, the activation of GABAARs is typically tonic and primarily influenced by the ambient GABA present in the surrounding environment. In immature neurons, GABAAR-mediated tonic conductance is depolarizing (sometimes excitatory) because the intracellular Cl– concentration is maintained high by the balance of Cl– transporters (Owens et al., 1996; Ben-Ari, 2002; Yamada et al., 2004). It is believed that this tonic conductance through GABAARs plays a crucial role in various developmental processes, including neurogenesis (LoTurco et al., 1995; Haydar et al., 2000; Andang et al., 2008), neuronal migration (Behar et al., 1996, 1998, 2000, 2001; López-Bendito et al., 2003; Cuzon et al., 2006; Heck et al., 2007; Bortone and Polleux, 2009; Denter et al., 2010; Inada et al., 2011; Inoue et al., 2012), and synaptogenesis (Nakanishi et al., 2007; Wang and Kriegstein, 2008).
Altered GABAergic functions that arise during early brain growth are caused by variations in Cl– homeostasis and play important roles in the development of the neocortex by regulating processes such as synaptogenesis and laminar organization (Figure 1). During neural development, GABA, which is non-synaptically released from GABAergic neurons, has a paracrine effect on immature neurons (Demarque et al., 2002; Manent et al., 2005), which may influence both radial migration (Behar et al., 1996, 1998, 2000, 2001; Heck et al., 2007; Denter et al., 2010) and tangential migration (López-Bendito et al., 2003; Cuzon et al., 2006; Bortone and Polleux, 2009). The MZ plays a critical role in the developmental processes of cell migration and lamination within the cerebral cortex. Although Cajal-Retzius cells and non-Cajal-Retzius cells in the MZ are temporary cell populations, they both receive functional synaptic inputs and play a role in transient synaptic circuits. These synaptic interactions involving different cell types within the MZ are believed to be instrumental in the activity-dependent maturation of the neocortex, considering their vital contribution to structural development. Previous studies have highlighted the significance of these synaptic integrations in understanding the dynamic developmental processes occurring in the neocortex (Hestrin and Armstrong, 1996; Zhou and Hablitz, 1996; Radnikow et al., 2002; Luhmann et al., 2003; Soda et al., 2003). The intricate synaptic networks formed within the MZ are likely to contribute to the overall functional and structural organization of the cerebral cortex during development (Kilb et al., 2011).
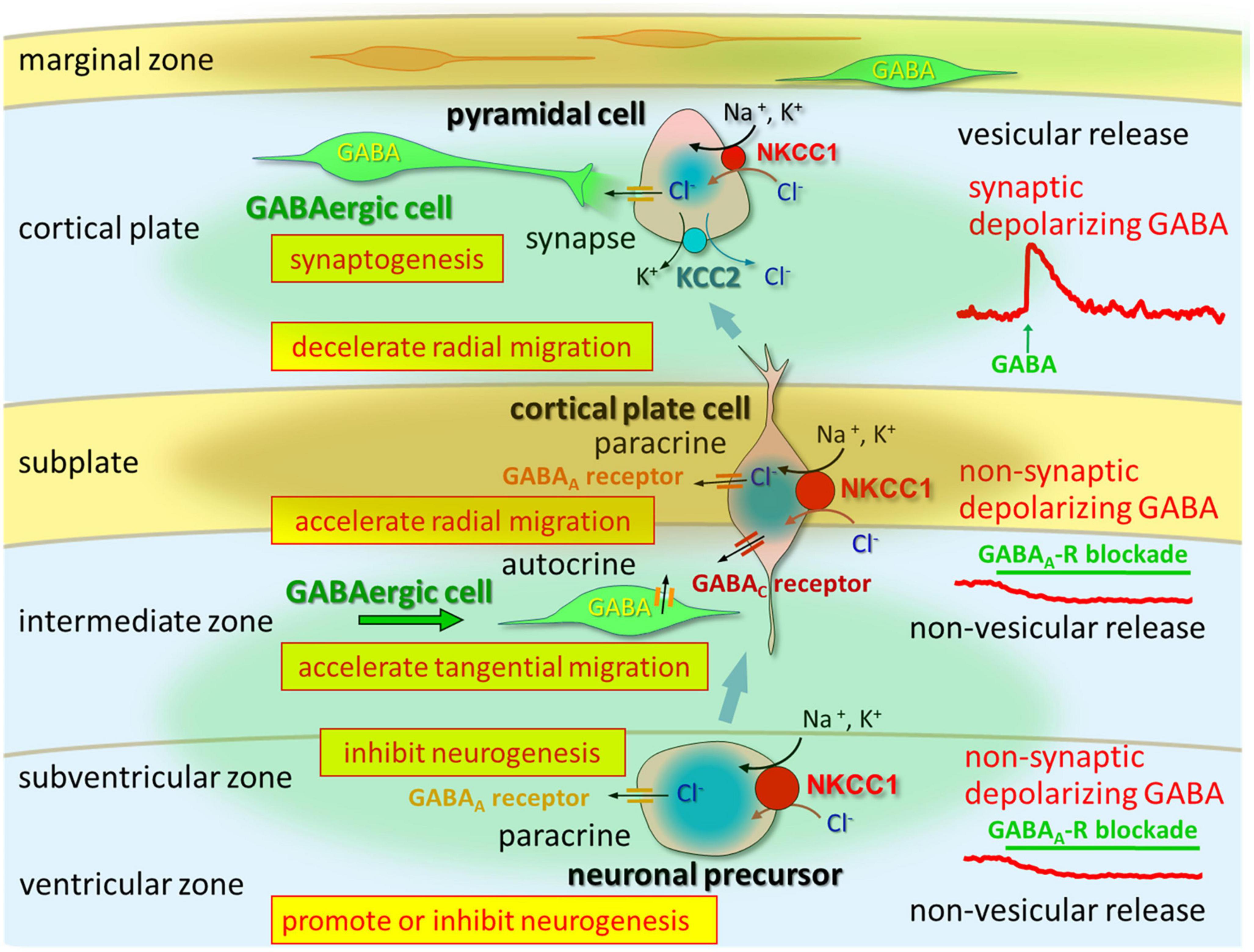
Figure 1. In the VZ and SVZ, ambient GABA affects neurogenesis. When post-mitotic neurons migrate to the CP, they are tonically depolarized by ambient GABA, which is released from tangentially migrating GABA neurons prior to forming synapses. Taurine taken-up and released by CP, subplate, and MZ neurons activates GABAA receptors in migrating cells. Vesicular release of GABA, which remains depolarizing, could contribute to synapse formation. Following establishment of GABAergic synapses and prior to hyperpolarization, GABA acts as an excitatory neurotransmitter. Up to this stage, intracellular chloride concentration levels are high due to NKCC1 and KCC2. Following up-regulation of KCC2 and down-regulation of NKCC1, GABA acts as an inhibitory neurotransmitter. Adapted from Fukuda and Wang (2013).
The transcriptional regulation of KCC2 is influenced by brain-derived neurotrophic factors (Aguado et al., 2003; Rivera et al., 2004; Coull et al., 2005) and GABA (Ganguly et al., 2001). Additionally, KCC2 function is modulated through post-translational mechanisms such as phosphorylation (Kelsch et al., 2001; Fiumelli et al., 2005; Khirug et al., 2005; Wake et al., 2007) and other pathways (Balakrishnan et al., 2003; Ikeda et al., 2004; Blaesse et al., 2006; Inoue et al., 2006). Previous studies suggest that the WNK-SPAK/OSR1 signaling pathway plays a role in the regulation and activation of NKCC1 through phosphorylation (Xu et al., 2000; Dowd and Forbush, 2003; Richardson and Alessi, 2008; Kahle et al., 2010). de Los Heros et al. (2006), Garzon-Muvdi et al. (2007), and Rinehart et al. (2009) proposed that the WNK signaling pathway is also responsible for the activation of KCCs, such as KCC2. Activation of WNKs is triggered by osmotic stress, but the upstream signaling mechanism is currently unknown (Anselmo et al., 2006; Richardson and Alessi, 2008; Zagórska et al., 2007).
Taurine is an agonist of both GABAA and glycine receptors
It is known that taurine structurally resembles GABA and glycine and interacts with both GABAAR and GlyRs to induce chloride currents in neuronal cells (Linne et al., 1996; Ye et al., 1997). GlyRs are pentameric proteins composed of α and β subunits. Five glycine receptor subunits have been identified consisting of 4 alpha (1–4) and one beta subunit. During development, homomeric α2 GlyRs are abundantly expressed in neurons (Kuhse et al., 1991) and are activated by taurine with lower affinity than glycine (Le-Corronc et al., 2011). GABAARs are composed of five subunits, and nineteen distinct subunits of GABAARs (α1–6, β1–3, γ1–3, δ, ε, φ, π, and ρ1–3) have been identified. Depending on the composition of subunits, GABAAR subtypes have different pharmacological and electrophysiological properties (Mehta and Ticku, 1999; Sieghart and Sperk, 2002). In addition, the expression patterns of GABAAR subunits are entirely different during development (Laurie et al., 1992; Fritschy et al., 1994). In the prenatal and early postnatal periods, neocortical neurons primarily express the α2–5, β2/3, and γ1/2 subunits (Laurie et al., 1992). The expression patterns of subunit transcripts of α2/3 and α5 were observed throughout the developing CNS. In the germinal matrix, specifically the ventricular zone (VZ), there was a high abundance of GABAAR α4, β1, and γ1 subunit mRNAs. However, these subunits were not detected in the intermediate zone (IZ) at embryonic day (E) 17 and E20 in rats, indicating that proliferating cells in the germinal matrix may express these specific subunits (Ma and Barker, 1995).
In rodents, during corticogenesis, GABAergic interneurons originate from the medial ganglionic eminence (MGE) and caudal ganglionic eminence (CGE) migrate tangentially toward the cortex (Marin, 2013; Lim et al., 2018). The MGE and CGE are the primary sources of cortical interneurons in the developing nervous system. As these cells migrate from the MGE to the neocortex, their sensitivity to GABA increases (Cuzon et al., 2006). Cuzon and Yeh (2011) demonstrated that migrating neurons in the MGE and cortex exhibit distinct response profiles to specific subunits, indicating the presence of different GABAAR isoforms. In their study, expression profiling of GABAAR subunits at the mRNA level (α1–5, β1–3, γ1–3, and δ) revealed elevated mRNA expression of α1, α2, α5, γ2, and γ3 subunits in the cortex.
Since E17, the mRNA levels of β subunits have been increasing at varying rates (with β3 exhibiting the highest increase, followed by β2 and then β1), with each subunit reaching its peak expression at different times. Specifically, β1 and β2 reach their peak expression levels at postnatal day (P) 12, while β3 reaches its peak expression level between E19 and P12. Additionally, the γ1 and γ2 subunits of GABAARs are upregulated at birth as they are expressed at low levels during E14. The mRNA expression of the δ and γ3 subunits appears around P0, with δ peaking at P12 and γ3 peaking at P6 (Laurie et al., 1992).
The functional characteristics and affinity of GABAAR for taurine vary depending on the subunit composition. Earlier investigations have established that taurine specifically affects β2 subunit containing GABAAR (Bureau and Olsen, 1991; Kash et al., 2004). Recombinant studies have also indicated that the effectiveness of taurine is not determined by the type of α subunit but rather by the specific β subunit. Furthermore, δ-containing receptors exhibit stronger and more effective activation by taurine compared to γ-containing receptors (Kletke et al., 2013). Based on these findings, it can be inferred that GABAARs containing αxβ1/2δ subunits are likely to be strongly activated by taurine. Immature cortical cells express GABAAR subunits α2–5, β1–3, γ1/2, and δ, and their expression is regulated during development (Gambarana et al., 1991; Araki et al., 1992; Cheng et al., 2006; Peden et al., 2008). Although the specific GABAAR subunits expressed in radially migrating cells are not completely clear, it is plausible that these cells are responsible for the effects of taurine. Additionally, our study suggests that GABAARs are activated tonically by ambient taurine rather than by ambient GABA in the fetal cerebral cortex. Thus, in the cerebral cortex of fetal mice, taurine may be the major agonist for tonic GABAAR, which is expressed by the radially migrating cells (Egawa and Fukuda, 2013).
Embryonic and fetal taurine is maternal origin
In adult mammals, taurine is synthesized in the liver through the conversion of methionine and cystine. However, due to the limited activities of taurine synthase, the synthesis of taurine is minimal in the livers and brains of human fetuses and newborn infants (Gaull et al., 1972; Zlotkin and Anderson, 1982; Sturman, 1988). Therefore, taurine is often referred to as a semi-essential amino acid (Lambert et al., 2015; Tochitani, 2017). As the ability of taurine synthesize in humans and rodents is limited, taurine deficiency is appears to be the result of reduced uptake of exogenous taurine (Miyazaki and Matsuzaki, 2014). TauT is responsible for the transport of taurine from the maternal blood to the developing brain of the embryo through the placenta (Ramamoorthy et al., 1994). In addition, fetuses consume amniotic fluid, which is abundant in taurine. Although fetal taurine levels decrease after birth, infants acquire taurine from breast milk, which contains a high concentration of taurine (Gaull, 1989; Sturman, 1993). Taurine is believed to play a role in osmoregulation and neuronal modulation through its interactions with GABAARs and GlyRs (Schmieden et al., 1992; del Olmo et al., 2000; Lambert, 2004). However, its precise role in brain development remains incompletely understood.
In TauT knockout (KO) mice, taurine level are strongly reduced in various tissues: in skeletal and heart muscle, brain, kidney, retina, and in liver (Heller-Stilb et al., 2002; Warskulat et al., 2004). TauT KO mice showed reduction of body weight gain during development and muscular endurance (Watanabe et al., 2022). Additionally, Hosoi et al. (2022) investigated the effect of taurine depletion during fetal and postnatal neocortical development on the functional properties of differentiated pyramidal neurons using TauT KO mice. They found that the depletion of taurine during development resulted in significant alteration in the firing responses of pyramidal neurons to external stimuli. These observations suggest that maternal and exogenous taurine is essential for normal development of neurons after birth.
Similar effect of taurine deficiency during perinatal period in other mammals was reported. The study using monkeys showed that infants fed taurine-free soy formula had impaired growth retardation (Hayes et al., 1980; Neuringer and Sturman, 1987). In human research, addition of taurine to formula increases fat absorption (Rigo and Senterre, 1977; Sturman, 1988). Nutritional studies of human premature infants showed that infants receiving taurine-enriched formula had more mature brain stem responses and a developmental advantage in motor function (Chesney et al., 1998). Additionally, premature infants who received breast milk showed an intelligence quotient advantage over infants who never received breast milk (Chesney et al., 1998). These evidences suggests that it is likely that there are feeding-dependent actions of taurine for normal development.
Paracrine taurine is essential for normal fetal development
Taurine plays various biological roles including ensuring tRNA stability (Suzuki et al., 2002) and promoting retinal development (Warskulat et al., 2007; Osakada et al., 2008). However, the precise signaling pathways that utilize intracellular taurine remain uncleared. During development, GABAAR-mediated signaling influences neurogenesis. LoTurco et al. (1995) found that GABA depolarize cells in the VZ of rat embryonic neocortex, ant that GABAAR antagonist application increase DNA synthesis. Their findings revealed that the depolarizing effects of GABA were associated with a reduction in progenitor cell proliferation in the developing neocortex of rats.
During the early phase of cortical neurogenesis in the VZ of rats, GABA causes cell depolarization and reduces DNA synthesis. This suggests that the presence of endogenous GABA downregulate the cell cycle and proliferation of neocortical progenitor cells (LoTurco et al., 1995; Haydar et al., 2000). GABA exhibits similar effects on neural progenitor cell populations in the subventricular zone (SVZ), where it also acts to suppress proliferation (Haydar et al., 2000). GABA exerts a significant influence on postnatal adult neurogenesis through its depolarizing effects, and the expression of NKCC1 is critical for proliferation (Liu et al., 2005; Ge et al., 2006). Notably, robust expression of NKCC1 has been observed in the neurepithelium, including the VZ and ganglionic eminence. When KCC2 was globally overexpressed in newly fertilized zebrafish embryos, it led to a reversed Cl– ion gradient, which subsequently led to hyperpolarization induced by glycine in all neurons. As a consequence, there was an observed decrease in the quantity of motoneurons and interneurons, suggesting a decline in the generation of new neurons (Reynolds et al., 2008). Hence, excitation mediated by Cl– ions plays a crucial role in facilitating neurogenesis during the early stages of embryonic development. Furthermore, Andang et al. (2008) provided evidence that autocrine/paracrine signaling of GABA through GABAARs inhibits the proliferation of embryonic and peripheral neural crest stem cells.
In the neocortex, different classes of neurons, such as cortical pyramidal neurons and GABAergic interneurons, arise from distinct origins and exhibit specific migration patterns. Cortical pyramidal neurons, which are glutamatergic neurons, undergo radial migration from the VZ of the dorsal telencephalon. During this process, they migrate along the radial glial scaffolding toward the cortical plate (CP). On the other hand, GABAergic interneurons, derived from the MGE and CGE, migrate tangentially within the cerebral wall, following a different path (Marín and Rubenstein, 2001). It is possible that GABAAR signaling can have different effects on neurons that migrate in radial or tangential directions. About radial migration, a number of studies, including pioneering in vitro research by Behar et al. (1996, 1998), demonstrated that GABA plays a role in cortical cell radial migration (Luján et al., 2005; Heng et al., 2007). Behar et al. (1996) conducted a study demonstrating that the modulation of neuronal migration by GABA is highly dependent on its concentration. They found that femtomolar concentrations of GABA promote migration along a chemical gradient, known as chemotaxis, while micromolar concentrations enhance random migration, known as chemokinesis. Additionally, continuous subdural application of a GABAAR antagonist to block GABAAR activity in vivo led to an acceleration of radial migration and the development of abnormal cortices similar to heterotopia (Heck et al., 2007). Similarly, the application of a GABAAR antagonist directly into the ventricles also resulted in an accelerated radial migration, indicating that the activation of GABAAR acts as a signaling mechanism to halt radial migration in the cortical plate. The signaling that is induced by the action of GABAARs could include Ca2+ signaling. Some studies demonstrated that GABAAR-induced depolarization in radial migration cells produced calcium influx through voltage-gated L-type Ca2+ channels (Maric et al., 2001; Soria and Valdeolmillos, 2002; de Lima et al., 2009). The depolarization is attributed to the high intracellular Cl– concentration, which is dependent on the activity of NKCC1. Therefore, disturbances in immature Cl– ion homeostasis could lead to abnormal migration patterns.
The activation of GABAB receptors in neuroblastic cells or GABAARs containing ρ-subunits promotes migration from the subventricular zone (SVZ) and IZ (Behar et al., 2000; Denter et al., 2010). GABA also plays a role in regulating the tangential migration of immature GABAergic cortical interneurons (Inada et al., 2011). Taurine has been identified as a potential candidate in this process, given its accumulation in immature neurons during cerebral cortex development (Shimada et al., 1984; Flint et al., 1998). Furthermore, certain studies suggest that taurine-deficient kittens exhibit abnormal neuronal migration in the cerebellum and cerebral cortex (Sturman et al., 1985; Palackal et al., 1986). As both radial and tangential migration are regulated via GABAAR, taurine may act on both radiating and tangential cell migration. Avila et al. (2013) suggested that glycine receptor activation is also involved in tangential migration. They speculated that glycine rather than taurine activates glycine receptor during neonatal period (Avila et al., 2013). Therefore, regarding cell migration in the developing neocortex, taurine regulation for tangential migration may be lower than that for radial migration.
Despite the absence of GAD65 or GAD67 expression in knockout mice, leading to a significant reduction in brain GABA levels, the structural integrity of the neocortex remains unaffected (Ji et al., 1999). This paradoxical observation prompted us to propose the existence of compensatory mechanisms that mitigate the effects of GABA deficiency. One potential solution is taurine, the dominant free amino acid during brain development, which functions as a partial activator of GABAARs (Jia et al., 2008). It was demonstrated that neuroblasts in the visual cortex of newborn kittens from taurine-depleted mothers failed to migrate and differentiate normally, indicating taurine’s critical role in regulating neuronal migration (Palackal et al., 1986). In neocortex of E17.5 mouse embryos of GAD67-GFP knock-in mice, GFP positive cells were mainly located in VZ, SVZ, and MZ. By immunohistochemical analysis, taurine signals located in MZ and SP (Furukawa et al., 2014). These results suggested that there was distinct distribution patterns of GABA and taurine in developing neocortex. When the ambient cerebral taurine concentration in homozygous embryos of GAD67-GFP knock-in mice was reduced to 50% by maternal administration of D-cysteine sulfinic acid (D-CSA), a taurine synthesis inhibitor (Figure 2), GABAAR-mediated tonic currents were disappeared, and radial migration of CP cells was accelerated (Furukawa et al., 2014). These findings suggest that taurine functions as an innate agonist of embryonic tonic GABAAR in the neocortex. Considering the distinct distribution patterns of GABA and taurine and the absence of significant differences in tonic GABAAR currents among the different genotypes of GAD67-GFP knock-in mice, it is plausible that maternally derived taurine acts as a stop signal for radially migrating CP cells.
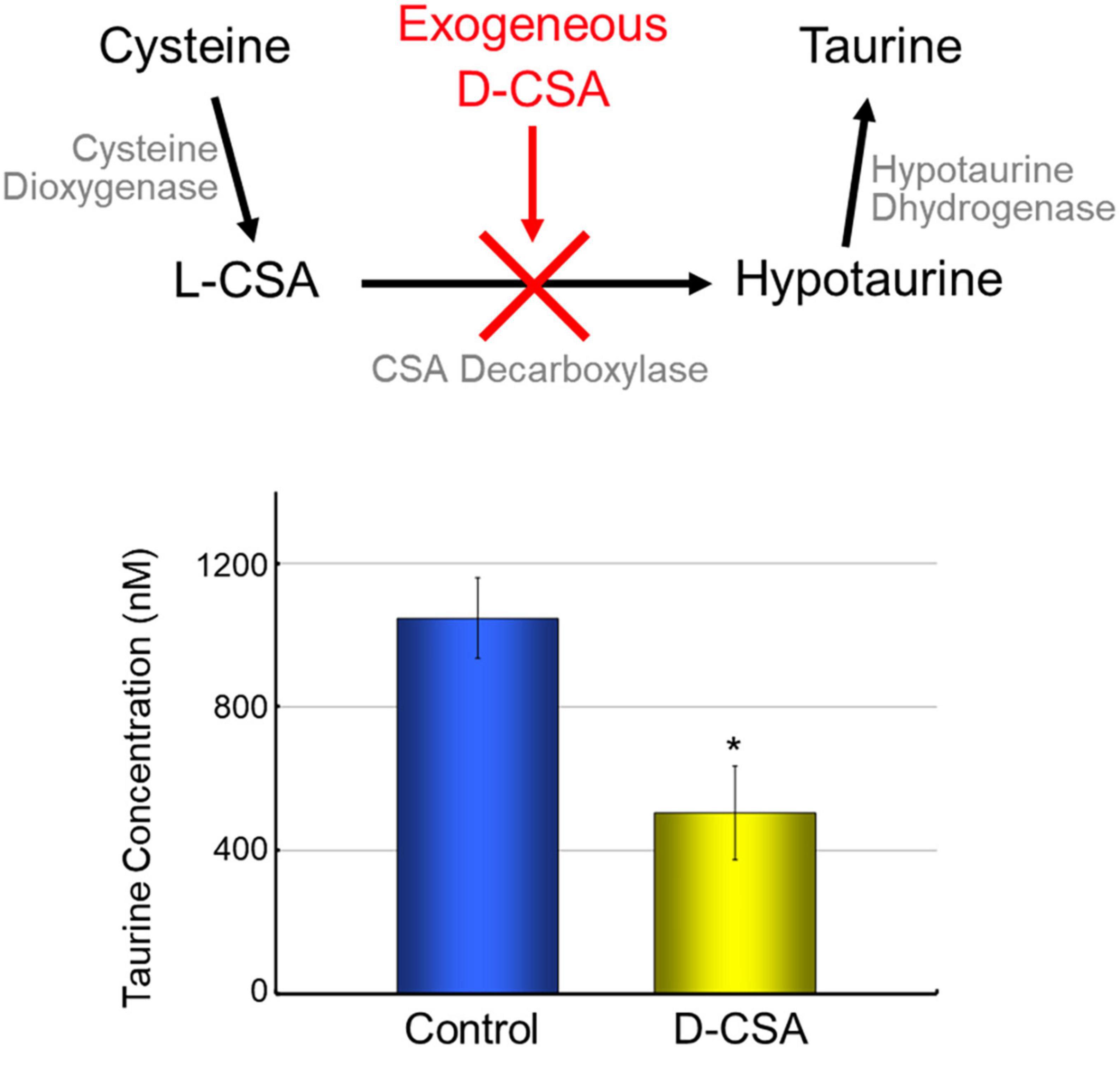
Figure 2. Maternal administration of D-CSA decreased ambient taurine in fetal cortex. Taurine is synthesized from cysteine via cysteine sulfinic acid (CSA) and hypotaurine. Cysteine dioxygenase and cysteine sulfinic acid decarboxylase mediates this pathway. As CSA is naturally L-type, administration of the optical isomer D-type CSA suppress taurine synthesis (Weinstein et al., 1988). The concentration of taurine released from fetal brain slices into the incubation medium was measured by HPLC, and the taurine level of brain slices from maternally D-CSA-injected fetuses was reduced by half. *P < 0.05, Student’s t-test.
In the neocortex, high expression of TauT has been detected (Smith et al., 1992). The distribution pattern of TauT in E17.5 mouse neocortex was similar to taurine distribution pattern, located in MZ and SP (Furukawa et al., 2014). TauT belong to the neurotransmitter transporter family, which relies on Na+ and Cl– and facilitates the uptake of taurine into cells during inactive states (Tappaz, 2004). The activity of TauTs can be reversed by stimuli that disrupt the Na+ and Cl– membrane gradient, as taurine uptake is dependent on this gradient (Oja et al., 1985; Oja and Kontro, 1987; Takuma et al., 1996). Inhibition of the TauT with the TauT inhibitor 2-(guanidino) ethanesulfonic acid (GES) led to elevated ambient taurine levels in fetal cortical slices. Furthermore, GES application during the taurine-loading phase decreased the release of taurine from cortical slices preloaded with 10 mM taurine. Additionally, GES application enhanced GABAAR-mediated tonic currents in subplate (SP) cells. These findings align with the expected function of TauTs in the uptake of extracellular taurine (Furukawa et al., 2014). Hence, the neocortex of fetal mice exhibits uptake of ambient taurine through the activity of TauTs, however, which types of cells in the cerebral cortex take up taurine is not yet clear.
Endogenous taurine modulate immature state of Cl homeostasis
KCC2 activity is known to be kinase-regulated (Kelsch et al., 2001; Lee et al., 2007; Wake et al., 2007; Rinehart et al., 2009; Watanabe et al., 2009). Taurine inhibit the KCC2 activity via serine/threonine phosphorylation (Inoue et al., 2012). When residues Thr-906 and Thr-1007 residues in KCC2 were replaced by Ala (KCC2T906A/T1007A), the facilitation of KCC2 activity was observed and the inhibitory effect of taurine was prevented (Inoue et al., 2012). Exogenous taurine activates WNK1, which in turn activates downstream SPAK/OSR1. The SPAK/OSR1 kinases regulate Thr906/Thr1007 phosphorylation sites of KCC2 (de Los Heros et al., 2014; Watanabe et al., 2019). The excessive expression of active WNK1 suppresses the function of KCC2. The phosphorylation of SPAK was consistently more pronounced in embryonic brains compared to neonatal brains and was reduced by inhibiting the TauT in vivo. Additionally, the radial migration of the cerebral cortex was disturbed by a variant of KCC2, known as KCC2T906A/T1007A, which is insensitive to taurine and can be regulated by the WNK-SPAK/OSR1 signaling pathway. Furthermore, activation of WNK-SPAK/OSR1 pathway leads to the activation of NKCC1. Taurine induced activation of the WNK-SPAK/OSR1 pathway suppresses KCC2 and activates NKCC1, resulting in increases intracellular Cl– influx and a positive shift in EGABA. These facts suggests that the taurine-WNK-SPAK/OSR1 signaling pathway may have a physiological role in maintaining embryonic Cl– homeostasis. Thus, taurine and WNK-SPAK/OSR1 signaling may contribute to the proper maintenance of neuronal Cl– homeostasis during embryonic development, which is crucial for normal brain development. Notably, the activation of WNK-SPAK/OSR1 signaling triggered by taurine may play a pivotal role in brain development (Figure 3).
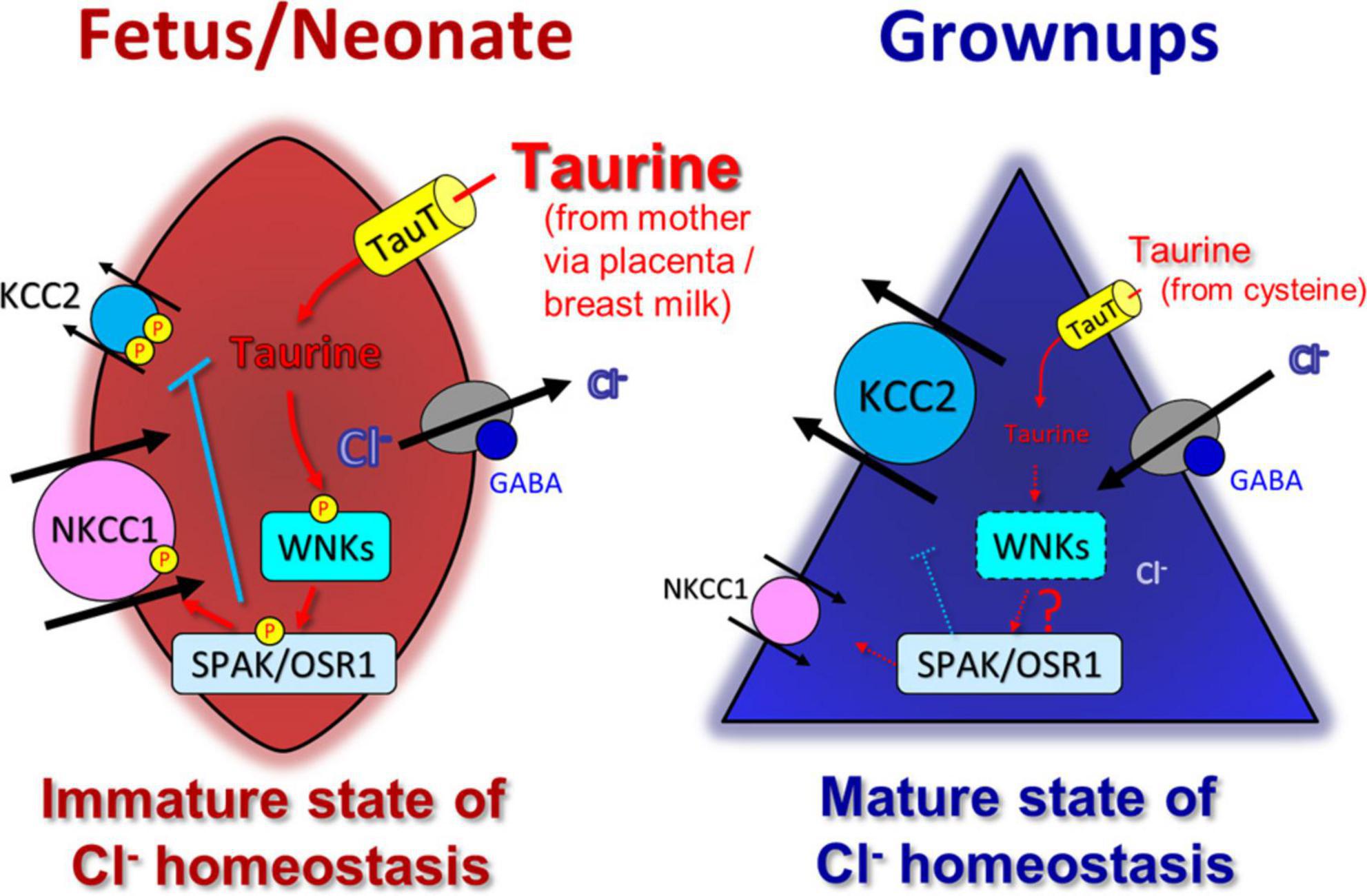
Figure 3. Taurine control WNK-SPAK/OSR1 signaling pathway and affect modulation of developmental switch in GABA actions induced by changes in Cl– homeostasis. In immature stage, taurine, taken up into cells by TauT, activates WNK-SPAK/OSR1 pathway (left). Both KCC2 and NKCC1 are phosphorylated, but their functions are oppositely regulated; KCC2 is inactivated, and NKCC1 is activated. Thus, the WNK-SPAK/OSR1 pathway maintains an immature stage of Cl– homeostasis with a high intracellular chloride concentration, rendering GABA-mediated depolarization that affects neural development. In mature stage, (possibly a while after birth), the decrease in taurine reduces the activity of WNK-SPAK/OSR1 pathway, together with the upregulation and downregulation of KCC2 and NKCC1 expression, respectively, and maintains the mature stage of Cl– homeostasis with a low intracellular Cl– concentration (right). Adapted from Fukuda and Watanabe (2019).
In the early stages of embryonic development, several types of neurons are generated, such as Cajal-Retzius and SP cells within the cerebral cortex. These particular cells have important functions in regulating the process of cell migration within the cerebral cortex. Several studies showed that these neurons, which are generated at an early stage in the MZ and SP, were activated by GABA and glycine (Mienville, 1998; Kilb et al., 2002, 2008; Hanganu et al., 2009). These early generated neurons are capable of expressing KCC2 at the embryonic and neonatal stages (Achilles et al., 2007). In addition, as taurine is abundant in these brain regions, our findings suggest that KCC2 is dysfunctional owing to taurine distribution, affecting WNK-SPAK/OSR1 signaling and preserving GABAergic excitation. This signaling cascade may play a more extensive and crucial role in the development of the brain than previously reported.
Nugent et al. (2012) report that administering estradiol to newborn rat offspring significantly increases the levels of SPAK and OSR1 proteins, two kinases upstream of the NKCC1 cotransporter. The elevation of estradiol levels leads to a significant increase in NKCC1 phosphorylation, and this effect is reliant on transcription. Notably, the time frame of the estradiol-induced rise in NKCC1 phosphorylation coincides with that of the estradiol-induced increase in NKCC1 expression. Intriguingly, unlike taurine, estradiol does not have an impact on the protein levels of WNK kinases or pSPAK. These findings suggest that estradiol does not modulate NKCC1 signaling by exerting its effects upstream of SPAK and OSR1.
Taurine regulate WNK1 phosphorylation because WNK1S382A and WNK1S382E mutant decrease the effect of taurine on positive EGABA shift (Inoue et al., 2012). The ratio of phosphor/total SPAK was downregulated at postnatal day 1 in cerebral cortex. It is suggested that WNK-SPAK/OSR1 signaling immediately decrease after birth. However, since the phosphorylated WNK1 level was not changed between embryo and postnatal rat, the phosphorylation of SPAK is not only regulated by intracellular taurine. The WNK signaling pathway can be activated by various stimuli, including osmotic stress, although the precise mechanisms underlying its activation remain unclear (Zagórska et al., 2007; Richardson and Alessi, 2008). Additionally, suppression of KCC2 function via WNK-SPAK/OSR1 signaling in rat is likely to decrease with development, however, the phosphorylation of KCC2 gradually diminishes as they progress into adulthood in mice (Rinehart et al., 2009). Further studies are necessary for the detail mechanism of WNK-SPAK/OSR1 signaling to KCC2 function.
Ambient taurine as an endogenous agonist of tonic GABA current
The persistent activation of GABAAR plays a role in the tonic depolarization observed in embryonic neurons. While numerous studies have investigated the involvement of GABAAR activation in neocortical development, particularly in migration, they mostly relied on exogenous inhibitors (Behar et al., 1996, 1998, 2000; Bolteus and Bordey, 2004; Heck et al., 2007; Denter et al., 2010). Using a different approach from previous reports, we accessed GABAAR activation in the developing neocortex. In previous study, we utilized GAD67-GFP knock-in mice lacking GABA synthesis (GAD67GFP/GFP) and performed in utero electroporation to label radially migrating cells originating from the VZ. A total of 3 days after electroporation, we found normal distribution of labeled-cells even in homozygous GAD67GFP/GFP mice (Furukawa et al., 2014). Furthermore, the sensitivity of labeled cells to GABA was also normal. Nonetheless, the accelerated radial migration observed in GAD67GFP/GFP mice upon continuous inhibition of GABAAR using the GABAAR antagonist SR95531 suggests the involvement of alternative endogenous agonists for GABAAR. Therefore, ambient taurine level was focused and measured in the fetal cerebral cortex using high-performance liquid chromatography (HPLC). In E17.5 wild-type mice, the taurine concentrations released from the cerebral cortex were measured to be approximately 50 fmol/μl⋅mg, while GABA was undetectable (Furukawa et al., 2014). Taking into account the measurement capabilities of the HPLC system (GABA is detected in 0.05 fmol/μl⋅mg), it is inferred that taurine levels in the cerebral cortex of E17.5 mice are more than 1000 times higher than those of GABA (Morishima et al., 2010). Furthermore, after taurine-loading to acute slices of the cerebral cortex, the released taurine concentration increased with a 10 mM taurine-loading, but after 1 mM taurine-loading did not affect the released taurine concentration (Furukawa et al., 2014). These findings suggest that the ambient taurine concentration in the fetal cerebral cortex may reach millimolar levels. The GES increased extracellular taurine concentration by blocking taurine uptake. The elevated extracellular taurine induced a GABAAR-mediated tonic current. In the taurine-deficient mouse model by maternal D-CSA administration, GABAAR-mediated tonic currents were abolished and radial migration was accelerated. Taurine, rather than GABA, may serve as an innate activator of embryonic tonic GABAAR conductance, as the tonic currents induced by GABAergic excitatory stimulation were indistinguishable between GAD67-GFP knock-in mice genotypes. Taurine is likely to exert agonistic effects on tonic GABAARs, potentially functioning as a signaling mechanism to halt the radial migration of neurons. The physiological significance of the suppression of migration by taurine is not yet known, and the benefit of this suppression is also not yet known. Such points should be addressed in future studies.
Given that taurine is taken up by TauT and that GABAAR is activated by taurine, there would be a mechanism of taurine release to modulate GABAAR activation in the developing cortical cells. The exact mechanism of taurine release is still remains unknown, but several lines of evidence have been reported suggesting non-vesicular and hypo-osmotic taurine release via taurine permeable channel. Immunoelectron microscopy analysis revealed the presence of taurine within immature neurons, while it was not detected in presynaptic structures in both mice and rats (Furukawa et al., 2014; Qian et al., 2014). These findings indicate that the release of taurine might be controlled by a non-vesicular process. This discovery is consistent with earlier research proposing that taurine can be released as an osmolyte using non-vesicular mechanisms in neurons and glial cells (Calvert and Shennan, 1998; Flint et al., 1998; Mongin et al., 1999; Mulligan and MacVicar, 2006). Taurine release mediated by volume-sensitive anion channels has been documented in numerous studies (Fugelli and Thoroed, 1986; Jackson and Strange, 1993; Hall, 1995; Shennan, 1999; Haskew-Layton et al., 2008). Despite the crucial role of taurine in functional growth, there is a lack of comprehensive knowledge regarding the release of taurine in the developing nervous system, although previous studies have reported non-synaptic and hypo-osmotic taurine release in the immature rat cortex (Flint et al., 1998; Kilb et al., 2008). The utilization of HPLC in acute neocortical slices demonstrated that the introduction of 4,4′-diisothiocyanatostilbene-2,2′-di-sulfonate (DIDS), a wide-ranging Cl– channel inhibitor, and 4-(2-butyl-6,7-dichlor-2-cyclopentylindan-1-on-5-yl) oxobutyric acid (DCPIB), a specific inhibitor of volume-sensitive anion channels, impeded the release of taurine. Conversely, taurine release was stimulated in a hypotonic medium (Furukawa et al., 2014). These findings provide compelling evidence that the release of taurine in the fetal cerebral cortex is facilitated through volume-sensitive anion channels (Jackson and Strange, 1993). However, it is still unclear which types of cells in the cerebral cortex release taurine, and how and when taurine release is modulated. Future studies will elucidate the detailed mechanism of taurine released in the developing cerebral cortex.
Activity-dependent taurine release modurate network excitability
Cajal-Retzius cells are a type of early generated neurons found in the MZ of the developing rat neocortex. These cells are crucial for cell migration and lamination processes in the cerebral cortex. Previous studies have indicated the presence of excitatory GABAergic neurotransmission in the MZ, and it has been observed that Cajal-Retzius cells express NKCC1 mRNA and protein (Mienville, 1998; Kilb and Luhmann, 2001; Achilles et al., 2007). This suggests that the uptake of Cl– is sufficient to maintain high intracellular Cl– concentrations, which are necessary for generating excitatory responses to GABA. Disruptions in the normal regulation of Cl– homeostasis and GABAergic signaling in the MZ may potentially contribute to cortical malformations, considering the MZ’s unique role in cell migration and lamination as well as its distinctive Cl– homeostasis mechanisms.
Excitatory GABAergic neurotransmission, depolarization mediated by GlyRs, and the functional expression of α2/β subunits of GlyRs in Cajal-Retzius cells have been substantiated by various studies (Hestrin and Armstrong, 1996; Mienville, 1998; Kilb and Luhmann, 2001; Kilb et al., 2002; Okabe et al., 2004). The propagation of action potentials across the MZ in rats remained unaffected by the administration of glutamate receptor blockers. On the other hand, inhibition of them was observed upon the administration of either GABAAR or glycine receptor antagonists. Notably, the combined application of blockers targeting GABAA and GlyRs resulted in the near-complete cessation of excitatory propagation. The impact of GABAA and glycine receptor antagonists on MZ neurotransmission was found to be additive, indicating the involvement of both GlyRs and GABAARs in synaptic transmission within the MZ (Qian et al., 2014). Bumetanide, an inhibitor of NKCC, has demonstrated the ability to attenuate excitation propagation in MZ. The cotransporter NKCC uptakes Cl– and facilitates the accumulation of Cl– in cells. Consequently, the activation of GABAAR can induce depolarization and occasional excitation in immature neurons. Additionally, the application of electrical stimulation to tangential slices including MZ followed by HPLC analysis revealed the release of GABA and taurine, while glycine or glutamate release was not observed (Qian et al., 2014). This suggests that the excitatory neurotransmission mediated by GABA in the rat MZ is facilitated through the activation of GlyRs by endogenous taurine. Although the release of taurine in response to depolarization and electrical stimulation has been observed in immature cortical regions, the specific mechanisms underlying activity-dependent taurine release remain unclear (Collins and Topiwala, 1974).
Several studies prove that taurine induces long-lasting enhancement of neurotransmission. In corticostriatal pathway, an involvement of taurine uptake by Na+-dependent TauTs accompanied by membrane depolarization has been considered (Chepkova et al., 2002, 2006; Sarkar et al., 2003; Sergeeva et al., 2003). This mechanism may accelerate the propagation of excitation, however, it is unlikely in the MZ because GES did not affect the spread of evoked signals induced by electrical stimulation. On the contrary, the induction of long-lasting synaptic transmission enhancement in the hippocampus by taurine relies on the presence of TauTs that are sensitive to GES (Galarreta et al., 1996; del Olmo et al., 2004; Dominy et al., 2004). In hippocampus, intracellular taurine accumulation rather than taurine uptake through TauT induces long-lasting synaptic potentiation (Galarreta et al., 1996; del Olmo et al., 2004; Dominy et al., 2004). While GES did not exhibit an impact on excitation within the MZ, inhibiting taurine uptake can lead to an elevation in extracellular taurine levels. It is possible that this discrepancy between the MZ and the hippocampus can be attributed to the possibility that electrical stimulation-induced extracellular taurine reaches a saturation point in facilitating excitatory propagation within the MZ. While the exact mechanism underlying the facilitatory effect of taurine on neurotransmission is yet to be fully understood, it is anticipated that taurine would augment excitatory propagation independent of GES-induced depolarization.
The possible effect of taurine action
There are some other excitatory or facilitatory effects of taurine. In retina, taurine is the most abundant amino acid (Pasantes-Morales et al., 1972). Bulley et al. (2013) demonstrated that taurine increases the firing rate of action potentials generated by current injection in ganglion cells of retina. The taurine-induced increase of firing rate was not affected by Cl– -permeable GABA and glycine receptor and GABAB receptor antagonists but was suppressed by voltage-gated potassium (KV) channel blockers. Furthermore, endogenous inward rectifier potassium current mediated by Kv channel was reduced by 5-HT2A serotonin receptor antagonist and PKC inhibitor. These results suggest that taurine facilitates neural activity by modulating serotonin system including 5-HT2A receptor and PKC pathway. There is possibility that taurine increased excitation through other intracellular pathways.
In addition to the developmental regulation of K-Cl cotransporters, Cl– homeostasis regulation in adult neurons has been reported. For example, the depolarizing EGABA shift was induced by tetanic stimulation, epileptic activity, hyperpolarizing current pulses, synaptic activity and BDNF application (Kapur and Coulter, 1995; Avoli, 1996; Kaila et al., 1997; Rivera et al., 2002, 2004; Wardle and Poo, 2003; Woodin et al., 2003; Fiumelli et al., 2005; Wang et al., 2006). After the depolarizing EGABA shift, GABA would promote neural activation and transmission. The EGABA shift in adult neurons would be caused by modification of KCC2 regulation. KCC2 regulation via WNK/SPAK signaling pathway in adult was reported (Conway et al., 2017; Lee et al., 2022). The possible effect of taurine on regulation of Cl– homeostasis in adults may be studied in future.
In this review, we have discussed about properties, functions, and role of maternal taurine including GABAAR- and GlyR-mediated actions. Otherwise, the various actions of GABA and taurine in immature neurons have been reported (Ben-Ari et al., 2007; Li et al., 2017). The GABAAR- and GlyR-mediated depolarization induced calcium elevation via voltage-gated calcium channels in immature neurons (Connor et al., 1987; Yuste and Katz, 1991; Owens et al., 1996). Whereas, taurine has been proposed to have antiexcitotoxic activity, and taurine diminish depolarization-induced intracellular calcium elevation by inhibition of reverse mode activity of Na+/Ca2+ exchanger in immature neuron (Zhao et al., 1999; Chen et al., 2001). This suggests that it is possible that taurine action is not simply, and other action of taurine may contribute to neural development beyond the taurine function described in this review article. Further research may reveal the detailed mechanism and novel effects of taurine on neural development.
Conclusion and perspectives
Even though it has been established that the activity of GABAARs can promote the growth of neurons, its impact appears to differ depending on the cell type or region: activation of GABAARs can either positively or negatively regulate the proliferation of neuronal progenitors or migration of neurons. Tonic and subsequent phasic depolarization mediated by GABAARs play a crucial role in the process of synaptogenesis. During development, the intracellular Cl– concentrations change, GABAAR containing tonically responsive subunit, and GABA and glycine receptor ligands (GABA or taurine), which is locally controlled by uptake or release mechanisms, allow the GABAAR-mediated actions to control the variety of developmental events in a mode and region-specific fashion. A disruption in the tonic conductance of GABA and glycine, which is regulated by the non-synaptic presence of GABA and taurine, can contribute to brain maldevelopment. Consequently, a range of pathological conditions such as epilepsy, psychiatric disorders, motor dysfunction, and neurodevelopmental disorders may be attributed, at least in part, to aberrant tonic GABA conductance. Therefore, maintaining the appropriate tone of tonic conductance and regulating the ambient GABA levels are crucial for normal brain function, and taurine, as an environmental factor acquired from the mother, is likely to play a modulatory role in CNS development.
Author contributions
AF contributed to conception, design, and wrote the first draft of the manuscript. TF wrote sections of the manuscript. Both authors contributed to manuscript revision, read, and approved the submitted version.
Funding
This publication cost of this review has been supported by Grants-in-Aid for Scientific Research (B) # 21H02661 and for Grant-in-Aid for Transformative Research Areas (A) #23H04159 from the Japan Society for the Promotion of Science (to AF).
Conflict of interest
The authors declare that the research was conducted in the absence of any commercial or financial relationships that could be construed as a potential conflict of interest.
Publisher’s note
All claims expressed in this article are solely those of the authors and do not necessarily represent those of their affiliated organizations, or those of the publisher, the editors and the reviewers. Any product that may be evaluated in this article, or claim that may be made by its manufacturer, is not guaranteed or endorsed by the publisher.
References
Achilles, K., Okabe, A., Ikeda, M., Shimizu-Okabe, C., Yamada, J., Fukuda, A., et al. (2007). Kinetic properties of Cl uptake mediated by Na+-dependent K+-2Cl cotransport in immature rat neocortical neurons. J. Neurosci. 27, 8616–8627. doi: 10.1523/JNEUROSCI.5041-06.2007
Aguado, F., Carmona, M. A., Pozas, E., Aguilo, A., Martinez-Guijarro, F. J., Alcantara, S., et al. (2003). BDNF regulates spontaneous correlated activity at early developmental stages by increasing synaptogenesis and expression of the K+/Cl– co-transporter KCC2. Development 130, 1267–1280. doi: 10.1242/dev.00351
Andang, M., Hjerling-Leffler, J., Moliner, A., Lundgren, T. K., Castelo-Branco, G., Nanou, E., et al. (2008). Histone H2AX-dependent GABAA receptor regulation of stem cell proliferation. Nature 451, 460–464. doi: 10.1038/nature06488
Anselmo, A. N., Earnest, S., Chen, W., Juang, Y. C., Kim, S. C., Zhao, Y., et al. (2006). WNK1 and OSR1 regulate the Na+. K+, 2Cl- cotransporter in HeLa cells. Proc. Natl. Acad. Sci .U.S.A. 103, 10883–10888. doi: 10.1073/pnas.0604607103
Araki, T., Kiyama, H., and Tohyama, M. (1992). GABAA receptor subunit messenger RNAs show differential expression during cortical development in the rat brain. Neuroscience 51, 583–591. doi: 10.1016/0306-4522(92)90298-g
Avila, A., Vidal, P. M., Dear, T. N., Harvey, R. J., Rigo, J. M., and Nguyen, L. (2013). Glycine receptor alpha2 subunit activation promotes cortical interneuron migration. Cell Rep. 4, 738–750. doi: 10.1016/j.celrep.2013.07.016
Avoli, M. (1996). GABA-mediated synchronous potentials and seizure generation. Epilepsia 37, 1035–1042. doi: 10.1111/j.1528-1157.1996.tb01022.x
Balakrishnan, V., Becker, M., Lohrke, S., Nothwang, H. G., Guresir, E., and Friauf, E. (2003). Expression and function of chloride transporters during development of inhibitory neurotransmission in the auditory brainstem. J. Neurosci. 23, 4134–4145. doi: 10.1523/JNEUROSCI.23-10-04134.2003
Behar, T. N., Li, Y. X., Tran, H. T., Ma, W., Dunlap, V., Scott, C., et al. (1996). GABA stimulates chemotaxis and chemokinesis of embryonic cortical neurons via calcium-dependent mechanisms. J. Neurosci. 16, 1808–1818. doi: 10.1523/JNEUROSCI.16-05-01808.1996
Behar, T. N., Schaffner, A. E., Scott, C. A., Greene, C. L., and Barker, J. L. (2000). GABA receptor antagonists modulate postmitotic cell migration in slice cultures of embryonic rat cortex. Cereb. Cortex 10, 899–909. doi: 10.1093/cercor/10.9.899
Behar, T. N., Schaffner, A. E., Scott, C. A., O’connell, C., and Barker, J. L. (1998). Differential response of cortical plate and ventricular zone cells to GABA as a migration stimulus. J. Neurosci. 18, 6378–6387. doi: 10.1523/JNEUROSCI.18-16-06378.1998
Behar, T. N., Smith, S. V., Kennedy, R. T., Mckenzie, J. M., Maric, I., and Barker, J. L. (2001). GABAB receptors mediate motility signals for migrating embryonic cortical cells. Cereb. Cortex 11, 744–753. doi: 10.1093/cercor/11.8.744
Ben-Ari, Y. (2002). Excitatory actions of gaba during development: The nature of the nurture. Nat. Rev. Neurosci. 3, 728–739. doi: 10.1038/nrn920
Ben-Ari, Y., Gaiarsa, J. L., Tyzio, R., and Khazipov, R. (2007). GABA: A pioneer transmitter that excites immature neurons and generates primitive oscillations. Physiol. Rev. 87, 1215–1284. doi: 10.1152/physrev.00017.2006
Benitez-Diaz, P., Miranda-Contreras, L., Mendoza-Briceno, R. V., Pena-Contreras, Z., and Palacios-Pru, E. (2003). Prenatal and postnatal contents of amino acid neurotransmitters in mouse parietal cortex. Dev. Neurosci. 25, 366–374. doi: 10.1159/000073514
Bernardi, N. (1985). On the role of taurine in the cerebellar cortex: A reappraisal. Acta Physiol. Pharmacol. Latinoam. 35, 153–164.
Blaesse, P., Guillemin, I., Schindler, J., Schweizer, M., Delpire, E., Khiroug, L., et al. (2006). Oligomerization of KCC2 correlates with development of inhibitory neurotransmission. J. Neurosci. 26, 10407–10419. doi: 10.1523/JNEUROSCI.3257-06.2006
Bolteus, A. J., and Bordey, A. (2004). GABA release and uptake regulate neuronal precursor migration in the postnatal subventricular zone. J. Neurosci. 24, 7623–7631. doi: 10.1523/JNEUROSCI.1999-04.2004
Bortone, D., and Polleux, F. (2009). KCC2 expression promotes the termination of cortical interneuron migration in a voltage-sensitive calcium-dependent manner. Neuron 62, 53–71. doi: 10.1016/j.neuron.2009.01.034
Bulley, S., Liu, Y., Ripps, H., and Shen, W. (2013). Taurine activates delayed rectifier Kv channels via a metabotropic pathway in retinal neurons. J. Physiol. 591, 123–132. doi: 10.1113/jphysiol.2012.243147
Bureau, M. H., and Olsen, R. W. (1991). Taurine acts on a subclass of GABAA receptors in mammalian brain in vitro. Eur. J. Pharmacol. 207, 9–16. doi: 10.1016/s0922-4106(05)80031-8
Calvert, D. T., and Shennan, D. B. (1998). Volume-activated taurine efflux from the in situ perfused lactating rat mammary gland. Acta Physiol. Scand. 162, 97–105. doi: 10.1046/j.1365-201X.1998.0267f.x
Cetine, I., Ronzoni, S., Marconi, A. M., Perugino, G., Corbetta, C., Battaglia, F. C., et al. (1996). Maternal concentrations and fetal-maternal concentration differences of plasma amino acids in normal and intrauterine growth-restricted pregnancies. Am. J. Obstet Gynecol. 174, 1575–1583. doi: 10.1016/s0002-9378(96)70609-9
Chen, W. Q., Jin, H., Nguyen, M., Carr, J., Lee, Y. J., Hsu, C. C., et al. (2001). Role of taurine in regulation of intracellular calcium level and neuroprotective function in cultured neurons. J. Neurosci. Res. 66, 612–619. doi: 10.1002/jnr.10027
Chen, X. C., Pan, Z. L., Liu, D. S., and Han, X. (1998). Effect of taurine on human fetal neuron cells: Proliferation and differentiation. Adv. Exp. Med. Biol. 442, 397–403.
Cheng, Q., Yeh, P. W., and Yeh, H. H. (2006). Cajal-Retzius cells switch from expressing gamma-less to gamma-containing GABA receptors during corticogenesis. Eur. J. Neurosci. 24, 2145–2151. doi: 10.1111/j.1460-9568.2006.05122.x
Chepkova, A. N., Doreulee, N., Yanovsky, Y., Mukhopadhyay, D., Haas, H. L., and Sergeeva, O. A. (2002). Long-lasting enhancement of corticostriatal neurotransmission by taurine. Eur. J. Neurosci. 16, 1523–1530. doi: 10.1046/j.1460-9568.2002.02223.x
Chepkova, A. N., Sergeeva, O. A., and Haas, H. L. (2006). Mechanisms of long-lasting enhancement of corticostriatal neurotransmission by taurine. Adv. Exp. Med. Biol. 583, 401–410. doi: 10.1007/978-0-387-33504-9_45
Chesney, R. W., Helms, R. A., Christensen, M., Budreau, A. M., Han, X., and Sturman, J. A. (1998). The role of taurine in infant nutrition. Adv. Exp. Med. Biol. 442, 463–476. doi: 10.1007/978-1-4899-0117-0_56
Collins, G. C., and Topiwala, S. H. (1974). Proceedings: The release of (14C)-taurine from slices of rat cerebral cortex and spinal cord evoked by electrical stimulation and high potassium ion concentrations. Br. J. Pharmacol. 50, 451–452.
Connor, J. A., Tseng, H. Y., and Hockberger, P. E. (1987). Depolarization- and transmitter-induced changes in intracellular Ca2+ of rat cerebellar granule cells in explant cultures. J. Neurosci. 7, 1384–1400. doi: 10.1523/JNEUROSCI.07-05-01384.1987
Conway, L. C., Cardarelli, R. A., Moore, Y. E., Jones, K., Mcwilliams, L. J., Baker, D. J., et al. (2017). N-Ethylmaleimide increases KCC2 cotransporter activity by modulating transporter phosphorylation. J. Biol. Chem. 292, 21253–21263. doi: 10.1074/jbc.M117.817841
Coull, J. A., Beggs, S., Boudreau, D., Boivin, D., Tsuda, M., Inoue, K., et al. (2005). BDNF from microglia causes the shift in neuronal anion gradient underlying neuropathic pain. Nature 438, 1017–1021. doi: 10.1038/nature04223
Cuzon, C. V. C., and Yeh, H. H. (2011). GABAA receptor subunit profiles of tangentially migrating neurons derived from the medial ganglionic eminence. Cereb. Cortex 21, 1792–1802. doi: 10.1093/cercor/bhq247
Cuzon, V. C., Yeh, P. W., Cheng, Q., and Yeh, H. H. (2006). Ambient GABA promotes cortical entry of tangentially migrating cells derived from the medial ganglionic eminence. Cereb. Cortex 16, 1377–1388. doi: 10.1093/cercor/bhj084
de Lima, A. D., Gieseler, A., and Voigt, T. (2009). Relationship between GABAergic interneurons migration and early neocortical network activity. Dev. Neurobiol. 69, 105–123. doi: 10.1002/dneu.20696
de Los Heros, P., Alessi, D. R., Gourlay, R., Campbell, D. G., Deak, M., Macartney, T. J., et al. (2014). The WNK-regulated SPAK/OSR1 kinases directly phosphorylate and inhibit the K+-Cl- co-transporters. Biochem. J. 458, 559–573. doi: 10.1042/BJ20131478
de Los Heros, P., Kahle, K. T., Rinehart, J., Bobadilla, N. A., Vazquez, N., San Cristobal, P., et al. (2006). WNK3 bypasses the tonicity requirement for K-Cl cotransporter activation via a phosphatase-dependent pathway. Proc. Natl. Acad. Sci .U.S.A. 103, 1976–1981. doi: 10.1073/pnas.0510947103
del Olmo, N., Bustamante, J., Del Rio, R. M., and Solis, J. M. (2000). Taurine activates GABAA but not GABAB receptors in rat hippocampal CA1 area. Brain Res. 864, 298–307. doi: 10.1016/s0006-8993(00)02211-3
del Olmo, N., Suarez, L. M., Orensanz, L. M., Suarez, F., Bustamante, J., Duarte, J. M., et al. (2004). Role of taurine uptake on the induction of long-term synaptic potentiation. Eur. J. Neurosci. 19, 1875–1886. doi: 10.1111/j.1460-9568.2004.03309.x
Demarque, M., Represa, A., Becq, H., Khalilov, I., Ben-Ari, Y., and Aniksztejn, L. (2002). Paracrine intercellular communication by a Ca2+– and SNARE-independent release of GABA and glutamate prior to synapse formation. Neuron 36, 1051–1061. doi: 10.1016/s0896-6273(02)01053-x
Denter, D. G., Heck, N., Riedemann, T., White, R., Kilb, W., and Luhmann, H. J. (2010). GABAC receptors are functionally expressed in the intermediate zone and regulate radial migration in the embryonic mouse neocortex. Neuroscience 167, 124–134. doi: 10.1016/j.neuroscience.2010.01.049
Dominy, J. Jr., Thinschmidt, J. S., Peris, J., Dawson, R. Jr., and Papke, R. L. (2004). Taurine-induced long-lasting potentiation in the rat hippocampus shows a partial dissociation from total hippocampal taurine content and independence from activation of known taurine transporters. J. Neurochem. 89, 1195–1205. doi: 10.1111/j.1471-4159.2004.02410.x
Dowd, B. F., and Forbush, B. (2003). PASK (proline-alanine-rich STE20-related kinase), a regulatory kinase of the Na-K-Cl cotransporter (NKCC1). J. Biol. Chem. 278, 27347–27353. doi: 10.1074/jbc.M301899200
Egawa, K., and Fukuda, A. (2013). Pathophysiological power of improper tonic GABAA conductances in mature and immature models. Front. Neural Circuits 7:170. doi: 10.3389/fncir.2013.00170
Fiumelli, H., Cancedda, L., and Poo, M. M. (2005). Modulation of GABAergic transmission by activity via postsynaptic Ca2+–dependent regulation of KCC2 function. Neuron 48, 773–786. doi: 10.1016/j.neuron.2005.10.025
Flint, A. C., Liu, X., and Kriegstein, A. R. (1998). Nonsynaptic glycine receptor activation during early neocortical development. Neuron 20, 43–53. doi: 10.1016/s0896-6273(00)80433-x
Fritschy, J. M., Paysan, J., Enna, A., and Mohler, H. (1994). Switch in the expression of rat GABAA-receptor subtypes during postnatal development: An immunohistochemical study. J. Neurosci. 14, 5302–5324. doi: 10.1523/JNEUROSCI.14-09-05302.1994
Fugelli, K., and Thoroed, S. M. (1986). Taurine transport associated with cell volume regulation in flounder erythrocytes under anisosmotic conditions. J. Physiol. 374, 245–261. doi: 10.1113/jphysiol.1986.sp016077
Fukuda, A., and Wang, T. (2013). A perturbation of multimodal GABA functions underlying the formation of focal cortical malformations: Assessments by using animal models. Neuropathology 33, 480–486. doi: 10.1111/neup.12021
Fukuda, A., and Watanabe, M. (2019). Pathogenic potential of human SLC12A5 variants causing KCC2 dysfunction. Brain Res. 1710, 1–7. doi: 10.1016/j.brainres.2018.12.025
Furukawa, T., Yamada, J., Akita, T., Matsushima, Y., Yanagawa, Y., and Fukuda, A. (2014). Roles of taurine-mediated tonic GABAA receptor activation in the radial migration of neurons in the fetal mouse cerebral cortex. Front. Cell Neurosci. 8:88. doi: 10.3389/fncel.2014.00088
Galarreta, M., Bustamante, J., Martin Del Rio, R., and Solis, J. M. (1996). Taurine induces a long-lasting increase of synaptic efficacy and axon excitability in the hippocampus. J. Neurosci. 16, 92–102. doi: 10.1523/JNEUROSCI.16-01-00092.1996
Gambarana, C., Beattie, C. E., Rodriguez, Z. R., and Siegel, R. E. (1991). Region-specific expression of messenger RNAs encoding GABAA receptor subunits in the developing rat brain. Neuroscience 45, 423–432. doi: 10.1016/0306-4522(91)90238-j
Ganguly, K., Schinder, A. F., Wong, S. T., and Poo, M. (2001). GABA itself promotes the developmental switch of neuronal GABAergic responses from excitation to inhibition. Cell 105, 521–532. doi: 10.1016/s0092-8674(01)00341-5
Garzon-Muvdi, T., Pacheco-Alvarez, D., Gagnon, K. B., Vazquez, N., Ponce-Coria, J., Moreno, E., et al. (2007). WNK4 kinase is a negative regulator of K+-Cl– cotransporters. Am. J. Physiol. Renal. Physiol. 292, F1197–F1207. doi: 10.1152/ajprenal.00335.2006
Gaull, G., Sturman, J. A., and Raiha, N. C. (1972). Development of mammalian sulfur metabolism: Absence of cystathionase in human fetal tissues. Pediatr Res. 6, 538–547. doi: 10.1203/00006450-197206000-00002
Ge, S., Goh, E. L., Sailor, K. A., Kitabatake, Y., Ming, G. L., and Song, H. (2006). GABA regulates synaptic integration of newly generated neurons in the adult brain. Nature 439, 589–593. doi: 10.1038/nature04404
Hall, A. C. (1995). Volume-sensitive taurine transport in bovine articular chondrocytes. J. Physiol. 484(Pt 3), 755–766. doi: 10.1113/jphysiol.1995.sp020701
Hanganu, I. L., Okabe, A., Lessmann, V., and Luhmann, H. J. (2009). Cellular mechanisms of subplate-driven and cholinergic input-dependent network activity in the neonatal rat somatosensory cortex. Cereb. Cortex 19, 89–105. doi: 10.1093/cercor/bhn061
Haskew-Layton, R. E., Rudkouskaya, A., Jin, Y., Feustel, P. J., Kimelberg, H. K., and Mongin, A. A. (2008). Two distinct modes of hypoosmotic medium-induced release of excitatory amino acids and taurine in the rat brain in vivo. PLoS One 3:e3543. doi: 10.1371/journal.pone.0003543
Haydar, T. F., Wang, F., Schwartz, M. L., and Rakic, P. (2000). Differential modulation of proliferation in the neocortical ventricular and subventricular zones. J. Neurosci. 20, 5764–5774.
Hayes, K. C., Stephan, Z. F., and Sturman, J. A. (1980). Growth depression in taurine-depleted infant monkeys. J. Nutr. 110, 2058–2064. doi: 10.1093/jn/110.10.2058
Hayes, K. C., and Sturman, J. A. (1981). Taurine in metabolism. Annu. Rev. Nutr. 1, 401–425. doi: 10.1146/annurev.nu.01.070181.002153
Heck, N., Kilb, W., Reiprich, P., Kubota, H., Furukawa, T., Fukuda, A., et al. (2007). GABA-A receptors regulate neocortical neuronal migration in vitro and in vivo. Cereb. Cortex 17, 138–148. doi: 10.1093/cercor/bhj135
Heller-Stilb, B., van Roeyen, C., Rascher, K., Hartwig, H. G., Huth, A., Seeliger, M. W., et al. (2002). Disruption of the taurine transporter gene (taut) leads to retinal degeneration in mice. FASEB J. 16, 231–233. doi: 10.1096/fj.01-0691fje
Heng, J. I., Moonen, G., and Nguyen, L. (2007). Neurotransmitters regulate cell migration in the telencephalon. Eur. J. Neurosci. 26, 537–546. doi: 10.1111/j.1460-9568.2007.05694.x
Hestrin, S., and Armstrong, W. E. (1996). Morphology and physiology of cortical neurons in layer I. J. Neurosci. 16, 5290–5300. doi: 10.1523/JNEUROSCI.16-17-05290.1996
Hosoi, Y., Akita, T., Watanabe, M., Ito, T., Miyajima, H., and Fukuda, A. (2022). Taurine depletion during fetal and postnatal development blunts firing responses of neocortical layer II/III pyramidal neurons. Front. Mol. Neurosci. 15:806798. doi: 10.3389/fnmol.2022.806798
Huxtable, R. J. (1989). Taurine in the central nervous system and the mammalian actions of taurine. Prog. Neurobiol. 32, 471–533.
Ikeda, K., Onimaru, H., Yamada, J., Inoue, K., Ueno, S., Onaka, T., et al. (2004). Malfunction of respiratory-related neuronal activity in Na+, K+-ATPase alpha2 subunit-deficient mice is attributable to abnormal Cl– homeostasis in brainstem neurons. J. Neurosci. 24, 10693–10701. doi: 10.1523/JNEUROSCI.2909-04.2004
Inada, H., Watanabe, M., Uchida, T., Ishibashi, H., Wake, H., Nemoto, T., et al. (2011). GABA regulates the multidirectional tangential migration of GABAergic interneurons in living neonatal mice. PLoS One 6:e27048. doi: 10.1371/journal.pone.0027048
Inoue, K., Furukawa, T., Kumada, T., Yamada, J., Wang, T., Inoue, R., et al. (2012). Taurine inhibits K+-Cl– cotransporter KCC2 to regulate embryonic Cl– homeostasis via with-no-lysine (WNK) protein kinase signaling pathway. J. Biol. Chem. 287, 20839–20850. doi: 10.1074/jbc.M111.319418
Inoue, K., Yamada, J., Ueno, S., and Fukuda, A. (2006). Brain-type creatine kinase activates neuron-specific K+-Cl– co-transporter KCC2. J. Neurochem. 96, 598–608. doi: 10.1111/j.1471-4159.2005.03560.x
Jackson, P. S., and Strange, K. (1993). Volume-sensitive anion channels mediate swelling-activated inositol and taurine efflux. Am. J. Physiol. 265, C1489–C1500. doi: 10.1152/ajpcell.1993.265.6.C1489
Ji, F., Kanbara, N., and Obata, K. (1999). GABA and histogenesis in fetal and neonatal mouse brain lacking both the isoforms of glutamic acid decarboxylase. Neurosci. Res. 33, 187–194. doi: 10.1016/s0168-0102(99)00011-5
Jia, F., Yue, M., Chandra, D., Keramidas, A., Goldstein, P. A., Homanics, G. E., et al. (2008). Taurine is a potent activator of extrasynaptic GABAA receptors in the thalamus. J. Neurosci. 28, 106–115. doi: 10.1523/JNEUROSCI.3996-07.2008
Kaczmarek, L. (1976). A comparison of the evidence for taurine and GABA as neurotransmitters. New York, NY: Raven Press, 283–292.
Kahle, K. T., Rinehart, J., and Lifton, R. P. (2010). Phosphoregulation of the Na-K-2Cl and K-Cl cotransporters by the WNK kinases. Biochim. Biophys. Acta 1802, 1150–1158. doi: 10.1016/j.bbadis.2010.07.009
Kaila, K., Lamsa, K., Smirnov, S., Taira, T., and Voipio, J. (1997). Long-lasting GABA-mediated depolarization evoked by high-frequency stimulation in pyramidal neurons of rat hippocampal slice is attributable to a network-driven, bicarbonate-dependent K+ transient. J. Neurosci. 17, 7662–7672. doi: 10.1523/JNEUROSCI.17-20-07662.1997
Kapur, J., and Coulter, D. A. (1995). Experimental status epilepticus alters gamma-aminobutyric acid type A receptor function in CA1 pyramidal neurons. Ann. Neurol. 38, 893–900. doi: 10.1002/ana.410380609
Kash, T. L., Dizon, M. J., Trudell, J. R., and Harrison, N. L. (2004). Charged residues in the beta2 subunit involved in GABAA receptor activation. J. Biol. Chem. 279, 4887–4893. doi: 10.1074/jbc.M311441200
Kelsch, W., Hormuzdi, S., Straube, E., Lewen, A., Monyer, H., and Misgeld, U. (2001). Insulin-like growth factor 1 and a cytosolic tyrosine kinase activate chloride outward transport during maturation of hippocampal neurons. J. Neurosci. 21, 8339–8347. doi: 10.1523/JNEUROSCI.21-21-08339.2001
Khirug, S., Huttu, K., Ludwig, A., Smirnov, S., Voipio, J., Rivera, C., et al. (2005). Distinct properties of functional KCC2 expression in immature mouse hippocampal neurons in culture and in acute slices. Eur. J. Neurosci. 21, 899–904. doi: 10.1111/j.1460-9568.2005.03886.x
Kilb, W., Hanganu, I. L., Okabe, A., Sava, B. A., Shimizu-Okabe, C., Fukuda, A., et al. (2008). Glycine receptors mediate excitation of subplate neurons in neonatal rat cerebral cortex. J. Neurophysiol. 100, 698–707. doi: 10.1152/jn.00657.2007
Kilb, W., Ikeda, M., Uchida, K., Okabe, A., Fukuda, A., and Luhmann, H. J. (2002). Depolarizing glycine responses in Cajal-Retzius cells of neonatal rat cerebral cortex. Neuroscience 112, 299–307. doi: 10.1016/s0306-4522(02)00071-4
Kilb, W., Kirischuk, S., and Luhmann, H. J. (2011). Electrical activity patterns and the functional maturation of the neocortex. Eur. J. Neurosci. 34, 1677–1686. doi: 10.1111/j.1460-9568.2011.07878.x
Kilb, W., and Luhmann, H. J. (2001). Spontaneous GABAergic postsynaptic currents in Cajal-Retzius cells in neonatal rat cerebral cortex. Eur. J. Neurosci. 13, 1387–1390. doi: 10.1046/j.0953-816x.2001.01514.x
Kletke, O., Gisselmann, G., May, A., Hatt, H., and Sergeeva, O. A. (2013). Partial agonism of taurine at gamma-containing native and recombinant GABAA receptors. PLoS One 8:e61733. doi: 10.1371/journal.pone.0061733
Kontro, P., Marnela, K. M., and Oja, S. S. (1984). GABA, taurine and hypotaurine in developing mouse brain. Acta Physiol. Scand. Suppl. 537, 71–74.
Kuhse, J., Kuryatov, A., Maulet, Y., Malosio, M. L., Schmieden, V., and Betz, H. (1991). Alternative splicing generates two isoforms of the alpha 2 subunit of the inhibitory glycine receptor. FEBS Lett. 283, 73–77. doi: 10.1016/0014-5793(91)80557-j
Lambert, I. H. (2004). Regulation of the cellular content of the organic osmolyte taurine in mammalian cells. Neurochem. Res. 29, 27–63. doi: 10.1023/b:nere.0000010433.08577.96
Lambert, I. H., Kristensen, D. M., Holm, J. B., and Mortensen, O. H. (2015). Physiological role of taurine–from organism to organelle. Acta Physiol. 213, 191–212. doi: 10.1111/apha.12365
Laurie, D. J., Wisden, W., and Seeburg, P. H. (1992). The distribution of thirteen GABAA receptor subunit mRNAs in the rat brain. III. Embryonic and postnatal development. J. Neurosci. 12, 4151–4172. doi: 10.1523/JNEUROSCI.12-11-04151.1992
Le-Corronc, H., Rigo, J. M., Branchereau, P., and Legendre, P. (2011). GABAA receptor and glycine receptor activation by paracrine/autocrine release of endogenous agonists: More than a simple communication pathway. Mol. Neurobiol. 44, 28–52. doi: 10.1007/s12035-011-8185-1
Lee, H. H., Walker, J. A., Williams, J. R., Goodier, R. J., Payne, J. A., and Moss, S. J. (2007). Direct protein kinase C-dependent phosphorylation regulates the cell surface stability and activity of the potassium chloride cotransporter KCC2. J. Biol. Chem. 282, 29777–29784. doi: 10.1074/jbc.M705053200
Lee, K. L., Abiraman, K., Lucaj, C., Ollerhead, T. A., Brandon, N. J., Deeb, T. Z., et al. (2022). Inhibiting with-no-lysine kinases enhances K+/Cl- cotransporter 2 activity and limits status epilepticus. Brain 145, 950–963. doi: 10.1093/brain/awab343
Li, X. W., Gao, H. Y., and Liu, J. (2017). The role of taurine in improving neural stem cells proliferation and differentiation. Nutr. Neurosci. 20, 409–415. doi: 10.1080/1028415X.2016.1152004
Lim, L., Mi, D., Llorca, A., and Marin, O. (2018). Development and Functional Diversification of Cortical Interneurons. Neuron 100, 294–313. doi: 10.1016/j.neuron.2018.10.009
Linne, M. L., Jalonen, T. O., Saransaari, P., and Oja, S. S. (1996). Taurine-induced single-channel currents in cultured rat cerebellar granule cells. Adv. Exp. Med. Biol. 403, 455–462. doi: 10.1007/978-1-4899-0182-8_49
Liu, X., Wang, Q., Haydar, T. F., and Bordey, A. (2005). Nonsynaptic GABA signaling in postnatal subventricular zone controls proliferation of GFAP-expressing progenitors. Nat. Neurosci. 8, 1179–1187. doi: 10.1038/nn1522
López-Bendito, G., Lujan, R., Shigemoto, R., Ganter, P., Paulsen, O., and Molnar, Z. (2003). Blockade of GABAB receptors alters the tangential migration of cortical neurons. Cereb. Cortex 13, 932–942. doi: 10.1093/cercor/13.9.932
LoTurco, J. J., Owens, D. F., Heath, M. J., Davis, M. B., and Kriegstein, A. R. (1995). GABA and glutamate depolarize cortical progenitor cells and inhibit DNA synthesis. Neuron 15, 1287–1298. doi: 10.1016/0896-6273(95)90008-x
Luhmann, H. J., Hanganu, I., and Kilb, W. (2003). Cellular physiology of the neonatal rat cerebral cortex. Brain Res. Bull. 60, 345–353. doi: 10.1016/s0361-9230(03)00059-5
Luján, R., Shigemoto, R., and Lopez-Bendito, G. (2005). Glutamate and GABA receptor signalling in the developing brain. Neuroscience 130, 567–580. doi: 10.1016/j.neuroscience.2004.09.042
Ma, W., and Barker, J. L. (1995). Complementary expressions of transcripts encoding GAD67 and GABAA receptor alpha 4, beta 1, and gamma 1 subunits in the proliferative zone of the embryonic rat central nervous system. J. Neurosci. 15, 2547–2560. doi: 10.1523/JNEUROSCI.15-03-02547.1995
Manent, J. B., Demarque, M., Jorquera, I., Pellegrino, C., Ben-Ari, Y., Aniksztejn, L., et al. (2005). A noncanonical release of GABA and glutamate modulates neuronal migration. J. Neurosci. 25, 4755–4765. doi: 10.1523/JNEUROSCI.0553-05.2005
Maric, D., Liu, Q. Y., Maric, I., Chaudry, S., Chang, Y. H., Smith, S. V., et al. (2001). GABA expression dominates neuronal lineage progression in the embryonic rat neocortex and facilitates neurite outgrowth via GABAA autoreceptor/Cl– channels. J. Neurosci. 21, 2343–2360. doi: 10.1523/JNEUROSCI.21-07-02343.2001
Marin, O. (2013). Cellular and molecular mechanisms controlling the migration of neocortical interneurons. Eur. J. Neurosci. 38, 2019–2029. doi: 10.1111/ejn.12225
Marín, O., and Rubenstein, J. L. (2001). A long, remarkable journey: Tangential migration in the telencephalon. Nat. Rev. Neurosci. 2, 780–790. doi: 10.1038/35097509
Mehta, A. K., and Ticku, M. K. (1999). An update on GABAA receptors. Brain Res. Brain Res. Rev. 29, 196–217. doi: 10.1016/s0165-0173(98)00052-6
Michel, F., Fossat, B., Porthe-Nibelle, J., Lahlou, B., and Saint-Marc, P. (1994). Effects of hyposmotic shock on taurine transport in isolated trout hepatocytes. Exp. Physiol. 79, 983–995. doi: 10.1113/expphysiol.1994.sp003823
Mienville, J. M. (1998). Persistent depolarizing action of GABA in rat Cajal-Retzius cells. J. Physiol. 512(Pt 3), 809–817. doi: 10.1111/j.1469-7793.1998.809bd.x
Miyazaki, T., and Matsuzaki, Y. (2014). Taurine and liver diseases: A focus on the heterogeneous protective properties of taurine. Amino Acids 46, 101–110. doi: 10.1007/s00726-012-1381-0
Mongin, A. A., Reddi, J. M., Charniga, C., and Kimelberg, H. K. (1999). [3H]taurine and D-[3H]aspartate release from astrocyte cultures are differently regulated by tyrosine kinases. Am. J. Physiol. 276, C1226–C1230. doi: 10.1152/ajpcell.1999.276.5.C1226
Morishima, T., Uematsu, M., Furukawa, T., Yanagawa, Y., Fukuda, A., and Yoshida, S. (2010). GABA imaging in brain slices using immobilized enzyme-linked photoanalysis. Neurosci. Res. 67, 347–353. doi: 10.1016/j.neures.2010.04.005
Mulligan, S. J., and MacVicar, B. A. (2006). VRACs CARVe a path for novel mechanisms of communication in the CNS. Sci. STKE 2006:e42. doi: 10.1126/stke.3572006pe42
Nakanishi, K., Yamada, J., Takayama, C., Oohira, A., and Fukuda, A. (2007). NKCC1 activity modulates formation of functional inhibitory synapses in cultured neocortical neurons. Synapse 61, 138–149. doi: 10.1002/syn.20352
Neuringer, M., and Sturman, J. (1987). Visual acuity loss in rhesus monkey infants fed a taurine-free human infant formula. J. Neurosci. Res. 18, 597–601. doi: 10.1002/jnr.490180413
Nugent, B. M., Valenzuela, C. V., Simons, T. J., and Mccarthy, M. M. (2012). Kinases SPAK and OSR1 are upregulated by estradiol and activate NKCC1 in the developing hypothalamus. J. Neurosci. 32, 593–598. doi: 10.1523/JNEUROSCI.5415-11.2012
Oja, S. S., and Kontro, P. (1987). Cation effects on taurine release from brain slices: Comparison to GABA. J. Neurosci. Res. 17, 302–311. doi: 10.1002/jnr.490170316
Oja, S. S., Korpi, E. R., Holopainen, I., and Kontro, P. (1985). Mechanisms of stimulated taurine release from nervous tissue. Prog. Clin. Biol. Res. 179, 237–247.
Okabe, A., Kilb, W., Shimizu-Okabe, C., Hanganu, I. L., Fukuda, A., and Luhmann, H. J. (2004). Homogenous glycine receptor expression in cortical plate neurons and Cajal-Retzius cells of neonatal rat cerebral cortex. Neuroscience 123, 715–724. doi: 10.1016/j.neuroscience.2003.10.014
Osakada, F., Ikeda, H., Mandai, M., Wataya, T., Watanabe, K., Yoshimura, N., et al. (2008). Toward the generation of rod and cone photoreceptors from mouse, monkey and human embryonic stem cells. Nat. Biotechnol. 26, 215–224. doi: 10.1038/nbt1384
Owens, D. F., Boyce, L. H., Davis, M. B., and Kriegstein, A. R. (1996). Excitatory GABA responses in embryonic and neonatal cortical slices demonstrated by gramicidin perforated-patch recordings and calcium imaging. J. Neurosci. 16, 6414–6423. doi: 10.1523/JNEUROSCI.16-20-06414.1996
Owens, D. F., and Kriegstein, A. R. (2002). Is there more to GABA than synaptic inhibition? Nat. Rev. Neurosci. 3, 715–727. doi: 10.1038/nrn919
Palackal, T., Moretz, R., Wisniewski, H., and Sturman, J. (1986). Abnormal visual cortex development in the kitten associated with maternal dietary taurine deprivation. J. Neurosci. Res. 15, 223–239. doi: 10.1002/jnr.490150212
Pasantes-Morales, H., Klethi, J., Ledig, M., and Mandel, P. (1972). Free amino acids of chicken and rat retina. Brain Res. 41, 494–497. doi: 10.1016/0006-8993(72)90523-9
Peden, D. R., Petitjean, C. M., Herd, M. B., Durakoglugil, M. S., Rosahl, T. W., Wafford, K., et al. (2008). Developmental maturation of synaptic and extrasynaptic GABAA receptors in mouse thalamic ventrobasal neurones. J. Physiol. 586, 965–987. doi: 10.1113/jphysiol.2007.145375
Qian, T., Chen, R., Nakamura, M., Furukawa, T., Kumada, T., Akita, T., et al. (2014). Activity-dependent endogenous taurine release facilitates excitatory neurotransmission in the neocortical marginal zone of neonatal rats. Front. Cell Neurosci. 8:33. doi: 10.3389/fncel.2014.00033
Radnikow, G., Feldmeyer, D., and Lubke, J. (2002). Axonal projection, input and output synapses, and synaptic physiology of Cajal-Retzius cells in the developing rat neocortex. J. Neurosci. 22, 6908–6919. doi: 10.1523/JNEUROSCI.22-16-06908.2002
Ramamoorthy, S., Leibach, F. H., Mahesh, V. B., Han, H., Yang-Feng, T., Blakely, R. D., et al. (1994). Functional characterization and chromosomal localization of a cloned taurine transporter from human placenta. Biochem. J. 300(Pt 3), 893–900. doi: 10.1042/bj3000893
Reynolds, A., Brustein, E., Liao, M., Mercado, A., Babilonia, E., Mount, D. B., et al. (2008). Neurogenic role of the depolarizing chloride gradient revealed by global overexpression of KCC2 from the onset of development. J. Neurosci. 28, 1588–1597. doi: 10.1523/JNEUROSCI.3791-07.2008
Richardson, C., and Alessi, D. R. (2008). The regulation of salt transport and blood pressure by the WNK-SPAK/OSR1 signalling pathway. J. Cell Sci. 121, 3293–3304. doi: 10.1242/jcs.029223
Rigo, J., and Senterre, J. (1977). Is taurine essential for the neonates? Biol. Neonate 32, 73–76. doi: 10.1159/000240997
Rinehart, J., Maksimova, Y. D., Tanis, J. E., Stone, K. L., Hodson, C. A., Zhang, J., et al. (2009). Sites of regulated phosphorylation that control K-Cl cotransporter activity. Cell 138, 525–536. doi: 10.1016/j.cell.2009.05.031
Rivera, C., Li, H., Thomas-Crusells, J., Lahtinen, H., Viitanen, T., Nanobashvili, A., et al. (2002). BDNF-induced TrkB activation down-regulates the K+-Cl- cotransporter KCC2 and impairs neuronal Cl- extrusion. J. Cell Biol. 159, 747–752. doi: 10.1083/jcb.200209011
Rivera, C., Voipio, J., Thomas-Crusells, J., Li, H., Emri, Z., Sipila, S., et al. (2004). Mechanism of activity-dependent downregulation of the neuron-specific K-Cl cotransporter KCC2. J. Neurosci. 24, 4683–4691. doi: 10.1523/JNEUROSCI.5265-03.2004
Sarkar, H. K., Wright, E. M., Boorer, K. J., and Loo, D. D. (2003). Electrophysiological properties of the mouse Na+/Cl–-dependent taurine transporter (mTauT-1): Steady-state kinetics: Stoichiometry of taurine transport. Adv. Exp. Med. Biol. 526, 197–204. doi: 10.1007/978-1-4615-0077-3_25
Schmieden, V., Kuhse, J., and Betz, H. (1992). Agonist pharmacology of neonatal and adult glycine receptor alpha subunits: Identification of amino acid residues involved in taurine activation. EMBO J. 11, 2025–2032. doi: 10.1002/j.1460-2075.1992.tb05259.x
Sergeeva, O. A., Chepkova, A. N., Doreulee, N., Eriksson, K. S., Poelchen, W., Monnighoff, I., et al. (2003). Taurine-induced long-lasting enhancement of synaptic transmission in mice: Role of transporters. J. Physiol. 550, 911–919. doi: 10.1113/jphysiol.2003.045864
Shennan, D. B. (1999). Properties of volume-activated taurine and iodide efflux from term human placental tissue. Placenta 20, 485–491. doi: 10.1053/plac.1999.0391
Shimada, M., Shimono, R., Watanabe, M., Imahayashi, T., Ozaki, H. S., Kihara, T., et al. (1984). Distribution of 35S-taurine in rat neonates and adults. A whole-body autoradiographic study. Histochemistry 80, 225–230. doi: 10.1007/BF00495770
Sieghart, W., and Sperk, G. (2002). Subunit composition, distribution and function of GABA(A) receptor subtypes. Curr. Top. Med. Chem. 2, 795–816. doi: 10.2174/1568026023393507
Smith, K. E., Borden, L. A., Wang, C. H., Hartig, P. R., Branchek, T. A., and Weinshank, R. L. (1992). Cloning and expression of a high affinity taurine transporter from rat brain. Mol. Pharmacol. 42, 563–569.
Soda, T., Nakashima, R., Watanabe, D., Nakajima, K., Pastan, I., and Nakanishi, S. (2003). Segregation and coactivation of developing neocortical layer 1 neurons. J. Neurosci. 23, 6272–6279. doi: 10.1523/JNEUROSCI.23-15-06272.2003
Soria, J. M., and Valdeolmillos, M. (2002). Receptor-activated calcium signals in tangentially migrating cortical cells. Cereb. Cortex 12, 831–839. doi: 10.1093/cercor/12.8.831
Stipanuk, M. H., Kuo, S. M., and Hirschberger, L. L. (1984). Changes in maternal taurine levels in response to pregnancy and lactation. Life Sci. 35, 1149–1155. doi: 10.1016/0024-3205(84)90185-1
Sturman, J. A. (1993). Taurine in development. Physiol. Rev. 73, 119–147. doi: 10.1152/physrev.1993.73.1.119
Sturman, J. A., Moretz, R. C., French, J. H., and Wisniewski, H. M. (1985). Taurine deficiency in the developing cat: Persistence of the cerebellar external granule cell layer. J. Neurosci. Res. 13, 405–416. doi: 10.1002/jnr.490130307
Sturman, J. A., Rassin, D. K., and Gaull, G. E. (1977). Taurine in developing rat brain: Maternal-fetal transfer of [35 S] taurine and its fate in the neonate. J. Neurochem. 28, 31–39. doi: 10.1111/j.1471-4159.1977.tb07705.x
Suzuki, T., Suzuki, T., Wada, T., Saigo, K., and Watanabe, K. (2002). Taurine as a constituent of mitochondrial tRNAs: New insights into the functions of taurine and human mitochondrial diseases. EMBO J. 21, 6581–6589. doi: 10.1093/emboj/cdf656
Takuma, K., Matsuda, T., Kishida, Y., Asano, S., Azuma, J., and Baba, A. (1996). Ca2+ depletion facilitates taurine release in cultured rat astrocytes. Jpn. J. Pharmacol. 72, 75–78. doi: 10.1254/jjp.72.75
Tappaz, M. L. (2004). Taurine biosynthetic enzymes and taurine transporter: Molecular identification and regulations. Neurochem. Res. 29, 83–96. doi: 10.1023/b:nere.0000010436.44223.f8
Tochitani, S. (2017). Functions of maternally-derived taurine in fetal and neonatal brain development. Adv. Exp. Med. Biol. 975(Pt 1), 17–25. doi: 10.1007/978-94-024-1079-2_2
Wake, H., Watanabe, M., Moorhouse, A. J., Kanematsu, T., Horibe, S., Matsukawa, N., et al. (2007). Early changes in KCC2 phosphorylation in response to neuronal stress result in functional downregulation. J. Neurosci. 27, 1642–1650. doi: 10.1523/JNEUROSCI.3104-06.2007
Wang, D. D., and Kriegstein, A. R. (2008). GABA regulates excitatory synapse formation in the neocortex via NMDA receptor activation. J. Neurosci. 28, 5547–5558. doi: 10.1523/JNEUROSCI.5599-07.2008
Wang, L., Kitai, S. T., and Xiang, Z. (2006). Activity-dependent bidirectional modification of inhibitory synaptic transmission in rat subthalamic neurons. J. Neurosci. 26, 7321–7327. doi: 10.1523/JNEUROSCI.4656-05.2006
Wardle, R. A., and Poo, M. M. (2003). Brain-derived neurotrophic factor modulation of GABAergic synapses by postsynaptic regulation of chloride transport. J. Neurosci. 23, 8722–8732. doi: 10.1523/JNEUROSCI.23-25-08722.2003
Warskulat, U., Borsch, E., Reinehr, R., Heller-Stilb, B., Roth, C., Witt, M., et al. (2007). Taurine deficiency and apoptosis: Findings from the taurine transporter knockout mouse. Arch. Biochem. Biophys. 462, 202–209. doi: 10.1016/j.abb.2007.03.022
Warskulat, U., Flogel, U., Jacoby, C., Hartwig, H. G., Thewissen, M., Merx, M. W., et al. (2004). Taurine transporter knockout depletes muscle taurine levels and results in severe skeletal muscle impairment but leaves cardiac function uncompromised. FASEB J. 18, 577–579. doi: 10.1096/fj.03-0496fje
Watanabe, M., Ito, T., and Fukuda, A. (2022). Effects of taurine depletion on body weight and mouse behavior during development. Metabolites 12:631. doi: 10.3390/metabo12070631
Watanabe, M., Wake, H., Moorhouse, A. J., and Nabekura, J. (2009). Clustering of neuronal K+-Cl- cotransporters in lipid rafts by tyrosine phosphorylation. J. Biol. Chem. 284, 27980–27988. doi: 10.1074/jbc.M109.043620
Watanabe, M., Zhang, J., Mansuri, M. S., Duan, J., Karimy, J. K., Delpire, E., et al. (2019). Developmentally regulated KCC2 phosphorylation is essential for dynamic GABA-mediated inhibition and survival. Sci. Signal. 12:eaaw9315. doi: 10.1126/scisignal.aaw9315
Weinstein, C. L., Haschemeyer, R. H., and Griffith, O. W. (1988). In vivo studies of cysteine metabolism. Use of D-cysteinesulfinate, a novel cysteinesulfinate decarboxylase inhibitor, to probe taurine and pyruvate synthesis. J. Biol. Chem. 263, 16568–16579.
Woodin, M. A., Ganguly, K., and Poo, M. M. (2003). Coincident pre- and postsynaptic activity modifies GABAergic synapses by postsynaptic changes in Cl- transporter activity. Neuron 39, 807–820. doi: 10.1016/s0896-6273(03)00507-5
Xu, B., English, J. M., Wilsbacher, J. L., Stippec, S., Goldsmith, E. J., and Cobb, M. H. (2000). WNK1, a novel mammalian serine/threonine protein kinase lacking the catalytic lysine in subdomain II. J. Biol. Chem. 275, 16795–16801. doi: 10.1074/jbc.275.22.16795
Yamada, J., Okabe, A., Toyoda, H., Kilb, W., Luhmann, H. J., and Fukuda, A. (2004). Cl– uptake promoting depolarizing GABA actions in immature rat neocortical neurones is mediated by NKCC1. J. Physiol. 557, 829–841. doi: 10.1113/jphysiol.2004.062471
Ye, G., Tse, A. C., and Yung, W. (1997). Taurine inhibits rat substantia nigra pars reticulata neurons by activation of GABA- and glycine-linked chloride conductance. Brain Res. 749, 175–179. doi: 10.1016/s0006-8993(96)01427-8
Yuste, R., and Katz, L. C. (1991). Control of postsynaptic Ca2+ influx in developing neocortex by excitatory and inhibitory neurotransmitters. Neuron 6, 333–344. doi: 10.1016/0896-6273(91)90243-s
Zagórska, A., Pozo-Guisado, E., Boudeau, J., Vitari, A. C., Rafiqi, F. H., Thastrup, J., et al. (2007). Regulation of activity and localization of the WNK1 protein kinase by hyperosmotic stress. J. Cell Biol. 176, 89–100. doi: 10.1083/jcb.200605093
Zhao, P., Huang, Y. L., and Cheng, J. S. (1999). Taurine antagonizes calcium overload induced by glutamate or chemical hypoxia in cultured rat hippocampal neurons. Neurosci. Lett. 268, 25–28. doi: 10.1016/s0304-3940(99)00373-0
Zhou, F. M., and Hablitz, J. J. (1996). Postnatal development of membrane properties of layer I neurons in rat neocortex. J. Neurosci. 16, 1131–1139. doi: 10.1523/JNEUROSCI.16-03-01131.1996
Keywords: GABA, chloride, transporter, migration, cortex
Citation: Furukawa T and Fukuda A (2023) Maternal taurine as a modulator of Cl– homeostasis as well as of glycine/GABAA receptors for neocortical development. Front. Cell. Neurosci. 17:1221441. doi: 10.3389/fncel.2023.1221441
Received: 12 May 2023; Accepted: 20 July 2023;
Published: 03 August 2023.
Edited by:
Claudia Lodovichi, National Research Council (CNR), ItalyReviewed by:
Corette J. Wierenga, Radboud University, NetherlandsRustem Khazipov, Institut National de la Santé et de la Recherche Médicale (INSERM), France
Copyright © 2023 Furukawa and Fukuda. This is an open-access article distributed under the terms of the Creative Commons Attribution License (CC BY). The use, distribution or reproduction in other forums is permitted, provided the original author(s) and the copyright owner(s) are credited and that the original publication in this journal is cited, in accordance with accepted academic practice. No use, distribution or reproduction is permitted which does not comply with these terms.
*Correspondence: Atsuo Fukuda, YXhmdWt1ZGFAaGFtYS1tZWQuYWMuanA=, YXhmdWt1ZGE1N0BnbWFpbC5jb20=