- 1Department of Molecular and Cellular Neurobiology, Center for Neurogenomics and Cognitive Research, Amsterdam Neuroscience, Vrije Universiteit Amsterdam, Amsterdam, Netherlands
- 2Research Master’s Programme Brain and Cognitive Sciences, University of Amsterdam, Amsterdam, Netherlands
Designer Receptors Exclusively Activated by Designer Drugs (DREADDs) have proven themselves as one of the key in vivo techniques of modern neuroscience, allowing for unprecedented access to cellular manipulations in living animals. With respect to astrocyte research, DREADDs have become a popular method to examine the functional aspects of astrocyte activity, particularly G-protein coupled receptor (GPCR)-mediated intracellular calcium (Ca2+) and cyclic adenosine monophosphate (cAMP) dynamics. With this method it has become possible to directly link the physiological aspects of astrocytic function to cognitive processes such as memory. As a result, a multitude of studies have explored the impact of DREADD activation in astrocytes on synaptic activity and memory. However, the emergence of varying results prompts us to reconsider the degree to which DREADDs expressed in astrocytes accurately mimic endogenous GPCR activity. Here we compare the major downstream signaling mechanisms, synaptic, and behavioral effects of stimulating Gq-, Gs-, and Gi-DREADDs in hippocampal astrocytes of adult mice to those of endogenously expressed GPCRs.
1. Introduction
While astrocytes were traditionally seen as simple, supportive cells within the brain, the advent of Ca2+ imaging technologies in the 1990s led to the understanding that astrocytes exhibit a non-electrical form of excitability mediated by intracellular Ca2+ signaling (Cornell-Bell et al., 1990; Dani et al., 1992). Following this discovery, it was shown that astrocytes are intricately associated with neurons at the synapse, forming what is often termed the “tripartite synapse”: a functional unit consisting of pre- and post-synaptic neurons and peri-synaptic astrocyte processes (PAPs) (Araque et al., 1999; Papouin et al., 2017). Although the extent of gliotransmission remains debated (Bazargani and Attwell, 2016; Fiacco and McCarthy, 2018; Savtchouk and Volterra, 2018; Durkee et al., 2019), astrocytes are known to express a plethora of receptors which are also present on neurons, and a multitude of research now illustrates the contribution of astrocytes to synaptic activity and behavior, through dynamic interactions with neurons (Durkee and Araque, 2019; Lawal et al., 2022; Lyon and Allen, 2022).
G-protein coupled receptors (GPCRs) are a major family of metabotropic receptors abundantly expressed on both neurons and astrocytes. The presence of GPCRs on astrocytes is thought to allow these cells to receive and respond to local synaptic activity and environmental cues such as hormones and extracellular matrix molecules (Roux and Cottrell, 2014; Durkee and Araque, 2019; Kofuji and Araque, 2021). Astrocytic GPCRs can be activated by neurotransmitters such as glutamate, gamma-aminobutyric acid (GABA), adenosine triphosphate (ATP) and endocannabinoids, triggering a variety of downstream signaling pathways by elevation of intracellular Ca2+ (Durkee and Araque, 2019) or cyclic adenosine monophosphate (cAMP) (Zhou et al., 2019). There is a growing body of evidence suggesting that astrocytic GPCRs are instrumental in mediating memory processes (Santello et al., 2019; Kol and Goshen, 2021; Lyon and Allen, 2022), although much of this research utilizes artificial rather than endogenously expressed GPCRs. Indeed, methodological challenges in studying endogenous GPCR activity in astrocytes in vivo has limited our understanding of how they impact cognition. For instance, while a wide variety of techniques are available to study the function of endogenous GPCRs in astrocytes, assessing behavioral outcomes of astrocytic GPCR modulation often requires sacrificing the physiological plausibility of the intervention (Xie et al., 2015).
Chemogenetics has become a popular remedy to the difficulties in assessing the behavioral consequences of astrocytic GPCR activity. This methodology allows for the activity of genetically engineered proteins to be altered by a biologically inert chemical ligand (Roth, 2016). Designer Receptors Exclusively Activated by Designer Drugs (DREADDs) (Armbruster et al., 2007) are the most commonly used chemogenetic tool for this purpose. DREADDs aim to mimic endogenous GPCR activity (Atasoy and Sternson, 2018), and confer a number of advantages to other techniques, including increased physiological plausibility and access to behavioral effects (Xie et al., 2015). However, studies using this method to uncover the role of astrocytic GPCRs in synaptic activity and behavior have produced a number of varying results, provoking controversy both as to the roles of astrocytic GPCRs and the utility of DREADDs for investigating their activity. In this review we question how accurately DREADDs can replicate endogenous GPCR stimulation in adult mouse hippocampal astrocytes, and, by extension, how the use of DREADDs in hippocampal astrocytes may inform us on the roles astrocytic GPCRs in cognitive processes such as learning and memory.
2. Astrocyte participation at the synapse
2.1. Astrocyte morphological and functional responses to synaptic activity
Astrocytes, originally named for their “star”-like appearance, extend thousands of highly ramified processes across the neuropil, with a single mouse astrocyte capable of contacting 300–600 dendrites and over 100,000 synapses (Halassa et al., 2007). The abundance of ultrathin PAPs across the entire cell give astrocytes a sponge-like morphology (Arizono et al., 2020) that mediates interactions with neurons across largely non-overlapping spatial domains of interconnected astrocytes (Bushong et al., 2002). The nanoscopic scale of PAPs and their close physical proximity to the synaptic cleft makes them perfectly situated to mediate bidirectional communication with neurons (Ghézali et al., 2016), and by extension, survey and influence the activity of a well-defined volume of the neuropil (Papouin et al., 2017). Astrocytes express a wide range of cell surface receptors, including GPCRs (Verkhratsky, 2009), some of which allow for the transduction of neuronal signals into intracellular Ca2+ and cAMP elevations when stimulated by neurotransmitters (such as glutamate and ATP) released by neurons (Zhou et al., 2019; Park and Lee, 2020; Kofuji and Araque, 2021). Astrocytic cAMP levels are regulated primarily by activation of different astrocytic GPCRs that can stimulate or inhibit the generation of cAMP from ATP by action of adenylyl cyclase activity (Figure 1; Zhou et al., 2019). Astrocytic cAMP levels can modulate morphological and functional characteristics of these cells. For example, elevated intracellular cAMP by activation of beta-adrenoceptors (βARs) is known to influence morphology of cultured astrocytes (Vardjan et al., 2014). Functionally, astrocytic cAMP is known to trigger a number of signal transduction pathways leading to glycogenolysis, glucose uptake and lactate release, all of which have proposed functional roles in mediating gliotransmission and plasticity mechanisms (Zhou et al., 2019, 2021). Indeed, cAMP elevations during memory formation are known to induce synaptic plasticity mechanisms in the adult mouse hippocampus, resulting in improved memory acquisition (Zhou et al., 2021). The cAMP pathway is also known to regulate the neuroinflammatory profile of astrocytes, whereby increased intracellular cAMP levels protects against proinflammatory insults by impairing upregulation of pro-inflammatory cytokines (Christiansen et al., 2011; Zhou et al., 2019; Kim et al., 2021).
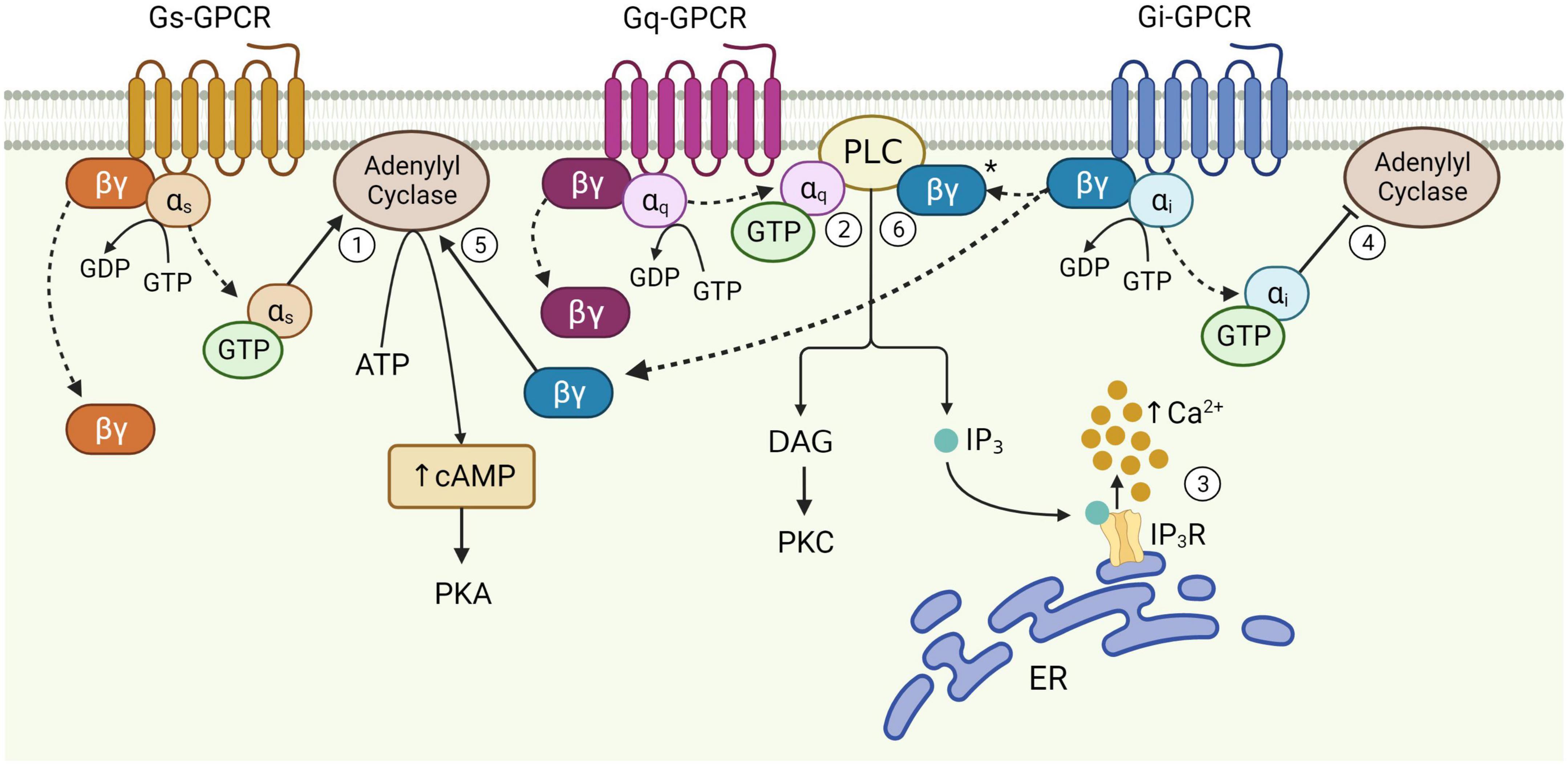
Figure 1. Schematic of astrocytic G-protein coupled receptor (GPCR) signaling. Activation of membrane-bound astrocytic GPCRs triggers the exchange of GDP for GTP at the α subunit and dissociation of α and βγ subunits from the receptor (dotted arrows). Once released, αs stimulates adenylyl cyclase activity leading to the production of cAMP from ATP (1). αq binds PLC, catalyzing diacylglycerol (DAG) and IP3 synthesis (2). IP3 binds its receptor on the endoplasmic reticulum (ER) to release Ca2+ from internal stores (3). αi inhibits adenylyl cyclase activity (4). The Gi βγ subunit can potentiate cAMP elevations mediated by Gs-GPCRs (5), and is speculated to enhance Gq-GPCR-mediated Ca2+ release from stores via the PLC-IP3 pathway (6), although this has yet to be proven in astrocytes (*). Figure created with Biorender.com.
While cAMP levels seem to impact astrocytic GPCR-related synaptic and behavioral effects, much more is known about the effects of GPCRs on astrocytic Ca2+ signaling. Activation of GPCRs allow astrocytes to produce activity-dependent Ca2+ signaling in response to synaptic activity, which is thought to be a key mediator of astrocyte-neuron interactions, including mechanisms that influence synaptic transmission (Perea and Araque, 2005; Di Castro et al., 2011) and plasticity (Guerra-Gomes et al., 2018; Liu J.-H. et al., 2022). A major source of astrocytic Ca2+ is that released from the endoplasmic reticulum, which is mediated primarily by activation of intracellular inositol trisphosphate (IP3) receptors via the phospholipase C (PLC) signaling pathway (Figure 1; Volterra and Meldolesi, 2005; Agulhon et al., 2008). It has recently been shown that endoplasmic reticulum can be found locally in PAPs of the mouse cortex (Aboufares El Alaoui et al., 2021), suggesting that PLC-IP3-dependent Ca2+ elevations can occur locally within PAPs, without necessarily involving the cell soma. Changes to intracellular Ca2+ levels are therefore dependent upon diverse inputs received at receptors and the intracellular signaling pathways that mediate Ca2+ elevations – both of which are subject to intrinsic properties of the receptor, astrocyte and the neural circuit to which they belong (Durkee and Araque, 2019).
Accordingly, compartmentalized Ca2+ events at PAP microdomains appear to be much more prevalent than global Ca2+ signaling involving the soma and proximal processes (Shigetomi et al., 2013; Haustein et al., 2014; Srinivasan et al., 2015; Rungta et al., 2016; Bindocci et al., 2017; Lia et al., 2021; Sherwood et al., 2021). Indeed, Ca2+ events within astrocytic somata likely originate from the fine processes (Oe et al., 2020). The basal distribution patterns of intracellular Ca2+ are therefore heterogenous both within and between cells (Zheng et al., 2015) whereby these basal concentrations appear to be functionally relevant to evoked Ca2+ signaling (King et al., 2020). That is, resting state Ca2+ concentrations in PAPs are correlated with the amplitudes of evoked Ca2+ elevations in the same PAPs, inviting the possibility that PAPs can encode information regarding the physiological demands of the surrounding neuropil. Astrocyte morphology is also shown to be highly heterogenous across different brain regions, according to the functional demands of nearby circuits (Chai et al., 2017; Matias et al., 2019). For example, while hippocampal and striatal astrocytes in the adult mouse both show equivalent somatic volumes and number of primary branches, striatal astrocytes occupy larger spatial territories, while hippocampal astrocytes contact more excitatory synapses and have PAPs positioned much closer to the post-synaptic densities than their striatal counterparts (Chai et al., 2017).
Not only do PAPs exhibit the ability to sense and adapt to the functional demands of their surroundings, morphological plasticity of PAPs is also associated with synaptic remodeling, with functional consequences to memory (Badia-Soteras et al., 2022; Lawal et al., 2022). β-adrenoceptors have been shown to affect the morphology of astrocyte in vitro (Vardjan et al., 2014), while metabotropic glutamate receptor 3 (mGluR3) and 5 (mGluR5) and IP3R2-mediated calcium release are involved in PAP plasticity (Lavialle et al., 2011; Perez-Alvarez et al., 2014). Accordingly, stimulation of PLC-IP3-dependent Ca2+ signaling, a key effect of astrocytic GPCR activation, has been shown to mediate structural remodeling of PAP-neuronal contacts in vivo (Tanaka et al., 2013; Bernardinelli et al., 2014; Perez-Alvarez et al., 2014). Indeed, PAP motility is also shown to be functionally relevant to long-term potentiation (LTP) whereby PAPs transiently alter their coverage of postsynaptic spines during LTP induction (Bernardinelli et al., 2014; Santello et al., 2019; Henneberger et al., 2020). This suggests that PAP-spine contacts are selectively restructured in an activity-dependent manner as a function of LTP. Given synaptic remodeling is associated with learning and memory (Caroni et al., 2012; Badia-Soteras et al., 2022), and morphological plasticity of PAPs was found to be impaired in a mouse model of Alzheimer Disease (Kater et al., 2023), astrocytic GPCR-mediated PAP motility is likely a key mechanism mediating learning and memory processes.
2.2. GPCRs and associated signaling pathways
The functional responses of astrocytes to synaptic activity described above are evoked primarily through activation of cell surface GPCRs. GPCRs are a large group of membrane-bound, metabotropic receptors, whose activation stimulates several cellular signaling pathways (Rosenbaum et al., 2009). They are characterized by seven transmembrane α-helix domains, the intracellular portions of which interact with heterotrimeric G proteins, composed of α, β, and γ subunits (Wettschureck and Offermanns, 2005). In the resting, inactive state, the α-subunit is bound to guanosine diphosphate (GDP) and remains associated with the βγ complex. Activation of the GPCR triggers exchange of GDP for guanosine triphosphate (GTP) on the α-subunit, resulting in its dissociation from the βγ-dimer and the receptor (Figure 1). This dissociation allows for the Gα subunit and βγ dimer to separately interact with other membrane proteins, such as adenylyl cyclase and PLC, and stimulate downstream signaling pathways (Weis and Kobilka, 2018). The structural nature of this system allows for substantial functional versatility. That is, all three components; receptor, G-protein and downstream effectors, are subject to regulation by additional mechanisms, while interactions between each component may also introduce variation, allowing GPCRs to evoke a wide variety of functions through stimulation of signaling pathways in multiple cell types – including astrocytes (Wettschureck and Offermanns, 2005).
G-protein coupled receptors are often categorized as Gq, Gs, or Gi, according to structural and functional similarities of the heterotrimeric G-protein α-subunit with which they preferentially interact (Syrovatkina et al., 2016). However, the effects of GPCR activity are made significantly more complex given that the same GPCR can couple to multiple Gα isoforms with different affinities, according to their specific receptor conformations (Rosenbaum et al., 2009; Okashah et al., 2019; Sandhu et al., 2019). This binding promiscuity allows for the same receptor to stimulate multiple signaling pathways to different extents. Nevertheless, the downstream effects of each GPCR subtype vary according to the major signaling pathways they preferentially activate (Figure 1). Gi-GPCR stimulation typically inhibits cAMP production by adenylyl cyclase inhibition. This has an inhibitory effect on neurons, whereby downregulation of cAMP and PKA mediate membrane hyperpolarization by increasing the open probability of G-protein inward rectifier K+ channels (Armbruster et al., 2007; Witkowski et al., 2012; Durkee et al., 2019). However, Gi-GPCR stimulation in astrocytes can elicit Ca2+ elevations, a marker of cellular activation, alongside reduced (Sobolczyk and Boczek, 2022) or elevated cAMP levels (Tang and Gilman, 1991; Walker et al., 2017). Conversely, Gs-GPCRs upregulate cAMP production and are therefore regarded as stimulatory in neurons – however are generally reported to not mediate Ca2+ elevations in astrocytes (Shigetomi et al., 2019; Oe et al., 2020). Finally, astrocytic Gq-GPCRs stimulate the PLC signaling pathway, mediating IP3 production and subsequent Ca2+ release from the endoplasmic reticulum (Rhee and Bae, 1997; Volterra and Meldolesi, 2005; Agulhon et al., 2008).
2.3. Investigating the effects of astrocytic GPCRs on synapse and behavior
A variety of GPCRs are endogenously expressed on hippocampal astrocytes (Verkhratsky et al., 2019), and studying their activation effects provides insight into key functional characteristics of astrocytes, including their effects on neuronal activity. Astrocytes exhibit substantial morphological and functional heterogeneity across different brain regions (Matias et al., 2019; Batiuk et al., 2020), species (Falcone, 2022) and developmental time (Sun et al., 2013; Farhy-Tselnicker and Allen, 2018; Matias et al., 2019). As a result, understanding the functional roles of astrocytic GPCRs requires careful consideration of the species, brain region and developmental timepoints under investigation. To limit confounds due to astrocyte functional heterogeneity and GPCR expression, here we compare literature on DREADDs and endogenous GPCRs primarily in adult mouse hippocampal astrocytes.
A major limitation to our understanding of the role of astrocytic GPCRs in synaptic activity and memory has been the methodological challenge of targeting these receptors to specific cell types within the intact brain. Classical pharmacological studies modulating astrocytic GPCR activity by agonist application (Angulo et al., 2004; Fiacco and McCarthy, 2004; Rae and Irving, 2004) are criticized for being unable to specify these interventions to astrocytes without impacting activity of the same receptors present on nearby neurons and other glia. Hence, although these studies indicate a role for astrocytic GPCRs in synaptic activity, their limitations hamper the drawing of proper conclusions. Selective elevation of Ca2+ by photochemical uncaging in astrocytes circumvented these issues, and was shown by multiple groups to have impacts on neuronal function in hippocampal slice preparations (Fellin et al., 2004; Liu et al., 2004). However, this technique lacks physiological plausibility, given that Ca2+ elevations are evoked in somata, failing to replicate endogenous spatiotemporal dynamics of Ca2+ signaling in PAPs (Shigetomi et al., 2013; Bindocci et al., 2017; Lia et al., 2021). Furthermore, because the uncaging of Ca2+ bypasses GPCR activation and subsequent signaling cascades, the complexity of this mechanism is lost and resulting Ca2+ dynamics are likely incomparable to those elicited through receptor activation.
3. DREADDs
3.1. Next generation methodology
The advent of transgenic mouse models and chemogenetic tools has enabled the selective activation of GPCRs in astrocytes. The first of these systems utilized the Mas-related genes A1 receptor (MrgA1R), a GPCR endogenously expressed in nociceptive sensory neurons in the dorsal root and trigeminal ganglia, but not in the brain (Dong et al., 2001). Chemogenetic expression of MrgA1R in hippocampal astrocytes thereby allowed for selective activation of Gq-GPCR-mediated Ca2+ signaling by exogenous ligand application (Fiacco et al., 2007; Agulhon et al., 2010). However, since agonists for MrgA1R cross the blood brain barrier with very poor efficacy, in vivo applications of this technique remained somewhat out of reach (Xie et al., 2015). Very soon after, a new family of engineered GPCRs – DREADDs – were developed, making in vivo modulation of GPCR signaling specifically in astrocytes a reality (Armbruster et al., 2007). DREADDs were engineered through molecular evolution of human muscarinic cholinergic receptors. The Gq-DREADD hM3Dq, and the Gi-DREADD hM4Di, were derived from the human M3 and M4 receptors respectively, while the Gs-DREADD rM3Ds was derived from the rat M3 receptor, mutated to contain the 2nd and 3rd intracellular loop of the turkey beta adrenoceptor (βAR) to allow for interaction with Gs proteins (Armbruster et al., 2007; Guettier et al., 2009; Farrell et al., 2013; Shen et al., 2021). Endogenous GPCRs show binding promiscuity between subtypes whereby receptor binding to each G protein subtype is reflected by conformational changes to the third intracellular loop of the GPCR structure (Sandhu et al., 2019). DREADDs on the other hand, are often assumed to activate generalized downstream signaling mechanisms specific to Gq-, Gs-, and Gi-GPCRs. However, since DREADD-mediated effects on Gq-, Gs-, and Gi-signaling are based upon the structure of their parent receptor (M3, M3/β1AR or M4), it is likely that the specificity of their G-protein coupling mechanisms reflect those of M3, M4 and β1A receptors, which have been shown to also couple multiple G-protein subtypes, albeit with lower affinities to their preferentially bound Gα subunit (Rubenstein et al., 1991; Dittman et al., 1994; Ilyaskina et al., 2018). As a result, researchers should consider that DREADDs do not interact specifically with Gq-, Gs-, and Gi-protein subtypes, but rather can couple multiple subtypes with varied affinities, much like their endogenous parent receptors.
Designer Receptors Exclusively Activated by Designer Drugs can be incorporated into cells of interest in living animals by viral expression using a cell-specific promotor [e.g., for astrocytic expression, using GFAP promotors (Chai et al., 2017; Adamsky et al., 2018; Durkee et al., 2019; Nagai et al., 2019; Lei et al., 2022)], and activated by systemic ligand injection (Adamsky et al., 2018; Atasoy and Sternson, 2018). The DREADD technique was first applied to study astrocytic GPCR signaling in vivo by Agulhon et al. (2013), with a number of major advantages. First, it allows for the modulation of activity in specific cell populations, whilst also retaining the complexity of GPCR-mediated signaling cascades (Xie et al., 2015; Atasoy and Sternson, 2018). This method is also relatively non-invasive compared to other in vivo experimental systems such as optogenetics. This is because activation of the transfected DREADD can be achieved by intraperitoneal ligand injection, while optogenetics requires the application of light directly to brain tissue (Xie et al., 2015). Second, the development of DREADDs mimicking different GPCR subtypes increased the number of experimental applications of this method compared to Gq-coupled MrgA1R (Fiacco et al., 2007; Atasoy and Sternson, 2018). Finally, unlike MrgA1R ligands, CNO ligands can cross the blood brain barrier very efficiently (Bender et al., 1994), allowing for in vivo DREADD activation and the assessment of subsequent behavioral effects (Xie et al., 2015).
3.2. Experimental findings from DREADD studies in astrocytes
Despite boasting increased physiological plausibility compared to other methods, recent reports highlight variable effects upon DREADD activation in astrocytes, including effects on Ca2+ dynamics, synaptic activity (e.g., Chai et al., 2017; Durkee et al., 2019) and behavior (e.g., Nam et al., 2019; Kol et al., 2020), even when the same DREADD is used in the same brain region, species and during the same developmental period. This evokes important questions yet to be assessed: Why do results from studies using DREADDs vary? Is this variability relevant to our understanding of endogenous GPCRs, or must we consider in greater depth the limitations of DREADDs? Here we question the assumption that astrocytic DREADD stimulation accurately mimics endogenous GPCR activity in astrocytes and subsequent synaptic and behavioral effects. To do this, we will first discuss the known intracellular, synaptic and behavioral effects of experimental activation of astrocytic Gq, Gs-, and Gi-DREADDs in the adult mouse hippocampus.
3.2.1. Gq-DREADDs
Gq-DREADDs such as hM3Dq have been experimentally expressed in astrocytes to replicate Gq-GPCR activation and investigate the subsequent intracellular, synaptic and behavioral effects in vivo (Roth, 2016). Stimulation of Gq-DREADDs has been shown to elicit Ca2+ signaling in astrocytic somata and processes in the mouse hippocampus, via IP3-dependent mechanisms (Agulhon et al., 2013; Chai et al., 2017; Adamsky et al., 2018; Durkee et al., 2019; Van Den Herrewegen et al., 2021; Liu J.-H. et al., 2022). Interestingly, Liu J.-H. et al. (2022) showed that while the majority of evoked somatic Ca2+ elevations are IP3 receptor 2 (IP3R2)-dependent, those in processes may be largely IP3R2-independent. Gq-DREADD-induced Ca2+ events are relatively long lasting compared to Ca2+ events evoked by Gi-DREADD stimulation (Kim et al., 2021). However, evoked and spontaneous Ca2+ events are shown to be completely abolished after initial elevations upon CNO injection (Vaidyanathan et al., 2021) despite CNO remaining available for binding for much longer (about 2 h). Together, these studies indicate that while Gq-DREADD ligand binding increases Ca2+ signaling in vivo by IP3R2-dependent and -independent mechanisms, Ca2+ activity is silenced upon prolonged stimulation.
While it is clear that astrocytic Gq-DREADD activations evoke intracellular Ca2+ elevations, the impact of these Ca2+ events to synaptic activity has become a point of controversy (Bazargani and Attwell, 2016). A number of studies using the MrgA1R chemogenetic tool in adult mouse hippocampal astrocytes have shown that although Gq pathway stimulation evokes robust Ca2+ elevations, it has no effect on neuronal activity in situ (Fiacco et al., 2007; Agulhon et al., 2010). However, since then, other studies have shown that Gq-DREADD activation in adult mouse hippocampal astrocytes can impact synaptic activity through interactions with nearby neurons. It was shown by Adamsky et al. (2018) that astrocytic Gq-DREADD stimulation increases evoked miniature excitatory post-synaptic currents (mEPSCs) by approximately 50%. Furthermore, Durkee et al. (2019) showed that Gq-DREADD activation in astrocytes robustly increases the frequency of slow inward currents (SICs) in neighboring neurons. This finding opposes that from Chai et al. (2017) who did not detect changes to SICs upon Gq-DREADD activation in astrocytes. However, Gq-DREADD-induced astrocytic activity in CA1 has been shown to be necessary and sufficient to induce NMDA-dependent LTP in CA3-CA1 synapses and enhance memory processes including acquisition and early consolidation (Adamsky et al., 2018). SICs are known to mediate NMDAR activity and plasticity mechanisms (Papouin and Oliet, 2014), substantiating claims that Gq-DREADD activation on hippocampal astrocytes alters synaptic activity. Indeed, more recent studies have also shown that Gq-DREADD stimulation on CA1 astrocytes mediates long-lasting potentiation at synapses (Van Den Herrewegen et al., 2021; Liu J.-H. et al., 2022).
These Gq-DREADD-mediated synaptic effects are also shown to influence memory. Adamsky et al. (2018) show that astrocytic Gq-DREADD activation in mouse CA1 selectively increased neuronal activity during fear memory acquisition, leading to memory enhancement. Furthermore, blocking astrocytic Gq-DREADD-induced Ca2+ elevations in the hippocampus using IP3R2 knockout mice evoked significant impairments to LTP and remote fear memory compared to wild type (Liu J.-H. et al., 2022).
Together, it seems that various in situ and in vivo studies corroborate the view that Gq-DREADD activation in astrocytes and subsequent Ca2+ signaling can (i) impact synaptic activity by generation of mEPSCs, SICs and LTP, and (ii) plays a role in memory formation. Indeed, as research in this area progresses, astrocytic Gq-DREADD activation and the synaptic and behavioral consequences are becoming increasingly established. However, the specific mechanisms by which astrocytes exert these effects, downstream of Ca2+ elevations, remains a debated topic for future research.
3.2.2. Gs-DREADDs
A limited number of studies have examined the activity of Gs-DREADD activation in astrocytes. Oe et al. (2020) showed that astrocytic Gs-DREADD activation in the adult mouse hippocampus mediates sustained elevation of cAMP levels and subsequently stimulates glycogenolysis. In addition, Gs-DREADD activation in hippocampal astrocytes elicits Ca2+ elevations (Chai et al., 2017; Nagai et al., 2021). Other studies have used alternative chemogenetic means to probe Gs-GPCR activity, including use of receptors activated solely by synthetic ligands (RASSL) such as RASSL serotonin 1 (Rs1), a Gs-coupled receptor based on the human 5-HT4b receptor. In one such study, stimulation of Rs1 in hippocampal astrocytes of adult mice was seen to increase cAMP levels, with no effect on Ca2+ signaling, and was shown to impair memory consolidation, but not learning (Orr et al., 2015). Together, these studies confirm that Gs-DREADDs expressed in the hippocampus of adult mice evoke increased cAMP levels and subsequent memory impairment. However, while Gs-DREADDs can indeed elicit Ca2+ elevations, this is not always the case. The cause of this inconsistent Ca2+ response to Gs-DREADD stimulation is unknown, and further research is required to elucidate the mechanisms responsible.
3.2.3. Gi-DREADDs
Several studies have shown that Gi-DREADD activation in mouse hippocampal astrocytes elicits Ca2+ elevations (Durkee et al., 2019; Kol et al., 2020; Kim et al., 2021), while others show limited to no effects on astrocytic Ca2+ levels (Chai et al., 2017; Van Den Herrewegen et al., 2021), despite all studies utilizing the same Gi-DREADD construct (hM4Di). It was shown by two studies that Gi-DREADD-evoked Ca2+ elevations are IP3R2-dependent (Kol et al., 2020; Vaidyanathan et al., 2021), indicating that Gi-DREADD activation evokes Ca2+ release from intracellular stores. Furthermore, when present, Gi-DREADD-evoked Ca2+ activity in hippocampal astrocytes is distinct to that of Gq-DREADDs, whereby Gi-mediated Ca2+ fluctuations are more transient in nature (Kim et al., 2021). Indeed, Kol et al. (2020) showed that Gi-DREADD activation in CA1 astrocytes evokes a moderate decrease in astrocytic Ca2+ levels with respect to baseline, after an initial transient peak in activity. These Ca2+ fluctuations also seem to regulate the reactive, proinflammatory astrocyte phenotype, such that prolonged Gi-DREADD activation results in inhibition of Ca2+ transients triggered by proinflammatory environments, and is associated with ameliorating neuroinflammation and associated cognitive impairment (Kim et al., 2021).
Gi-DREADD-evoked increases in intracellular Ca2+ levels in adult mouse hippocampal astrocytes is accompanied by profound effects on nearby neurons, including increased neuronal firing rates and SIC frequency (Durkee et al., 2019). Activation of astrocytic Gi-DREADDs in the adult mouse during learning has also been shown to potentiate NMDAR-dependent synaptic plasticity in Schaffer collaterals by lowering the stimulation threshold for LTP, ultimately resulting in enhanced recent contextual memory (Nam et al., 2019). Astrocytic Gi-DREADD-mediated effects on synaptic activity in Schaffer collaterals has also been shown in the absence of evoked Ca2+ signaling, whereby Gi-DREADD activation increased field excitatory post-synaptic potential (fEPSP) amplitude and LTP at CA1 synapses in adult mice (Van Den Herrewegen et al., 2021). However, in contrast, behavioral results from Kol et al. (2020) indicate that Gi-DREADD activation in CA1 astrocytes of adult mice during learning has no effect on recent memory retrieval, but does disrupt remote memory retrieval. It is noted that no effects on local CA1 neuronal activity were observed, but rather impaired remote memory retrieval resulted from impaired recruitment of CA1 projections during acquisition and disrupted synaptic transmission from the hippocampus to the cortex (Kol et al., 2020). This stark contrast between the results of Nam et al. (2019) and Kol et al. (2020) could be due to differences in (i) cellular pathways evoked by Gi-DREADD stimulation (Gα or Gβγ subunit affecting Ca2+ or cAMP), (ii) type of hippocampal synapse targeted (CA3-CA1 vs. CA1-cortex) or (iii) experimental design, whereby the potentiation shown by Nam et al. (2019) and Van Den Herrewegen et al. (2021) was dependent upon electrical stimulation of nearby neurons, while Kol et al. (2020) did not provide this stimulation.
Relatively little is known about Gi-DREADD effects on cAMP activity. However, what has been shown is that Gi-DREADD activation in rat hippocampal astrocytes inhibits cAMP production and attenuates stress-enhanced fear learning (Jones et al., 2018). Further studies are required to confirm that this also occurs in the adult mouse hippocampus.
Together, these studies show that Gi-DREADD activation can evoke at least two effector pathways – one that is Ca2+-dependent and another, Ca2+-independent pathway, likely relying on cAMP inhibition to evoke downstream effects. In addition, these studies show, similarly to Gs-DREADDs, that astrocytic Gi-DREADD stimulation can evoke Ca2+ signaling in many, but not all cases, sparking controversy on the mechanism of action of Gi-DREADD activation in astrocytes. However, the finding that Gi-DREADD activation in adult mouse hippocampal astrocytes evokes synaptic changes by both Ca2+-dependent and -independent mechanisms, illustrates the complexity of astrocytic GPCR functionality with respect to intracellular Ca2+ dynamics and resulting synaptic effects. Despite reduced cAMP production being a hallmark of this GPCR subtype, very little is known about the effects of Gi-DREADD stimulation on cAMP levels in hippocampal astrocytes. However, we speculate that reduced cAMP levels in the mouse hippocampus mediate Ca2+-independent synaptic and behavioral effects of astrocytic Gi-DREADD stimulation, whereby the type and frequency of stimulation determines downstream effects.
Taken together, the current understanding of the intracellular, synaptic and behavioral effects of DREADD expression in adult mouse hippocampal astrocytes is reaching a loose consensus. What many of these DREADD studies in astrocytes have made clear, is that much more research is needed to unravel the exceptional complexity of astrocytic GPCR systems and fully characterize their downstream effects on synapses and behavior. To understand these mechanisms, it is therefore important to evaluate what is known about endogenous GPCR signaling in astrocytes, and determine to what extent the effects of DREADD and endogenous GPCR stimulation are comparable in astrocytes.
4. Endogenous astrocytic GPCRs
4.1. Endogenous GPCR expression in astrocytes
Endogenously expressed GPCRs can be categorized as Gq, Gs, and Gi, thereby corresponding to the DREADD subtypes previously described. Here we provide evidence for the presence of some of the most frequently studied GPCRs in the adult mouse hippocampus (summarized in Tables 1–3) and describe the known intracellular, synaptic and behavioral effects of their activation, such that we may later compare these effects to those of DREADD stimulation in section “5. Comparative assessment of endogenous GPCRs and DREADDs.”
4.1.1. Endogenous Gq-GPCRs
Metabotropic glutamate receptors (mGuRs) are a core group of GPCRs present in hippocampal astrocytes of the adult mouse. Multiple mGluR subtypes exist, two of which are Gq-coupled; mGluR1 and mGluR5, whose activation elicits IP3-dependent Ca2+ signaling as previously described (Table 1). mGluR1 and mGluR5 are both group I mGluRs (mGluR1/5) and are therefore studied within this grouping, as well as individually. Substantial evidence shows the presence of mGluR1 (Fellin et al., 2004; Xie et al., 2012; Devaraju et al., 2013; Chai et al., 2017; Batiuk et al., 2020) and mGluR5 (Fellin et al., 2004; Chai et al., 2017; Batiuk et al., 2020; Liu C. et al., 2022) in rodent hippocampal astrocytes in vitro and in situ. However, relatively few studies have illustrated mGluR1/5 expression specifically in hippocampal astrocytes in the adult mouse (Chai et al., 2017; Batiuk et al., 2020; Liu C. et al., 2022). Immunoelectron microscopy by Sun et al. (2013) provided strong evidence that mGluR5 expression is downregulated over developmental time to near absence in the adult mouse brain. Indeed, although many studies have detected mGluR1/5 expression in rodent hippocampal astrocytes, single cell RNA sequencing (scRNA-seq) data also shows that expression tends to be very limited in the adult mouse hippocampus (Chai et al., 2017; Batiuk et al., 2020), indicating that mGluR5 is more relevant to developmental processes than those in the mature mouse. However, expression of astrocytic mGluR5 has since been shown by immunohistochemistry in the adult mouse hippocampus (Liu C. et al., 2022). Expression of astrocytic mGluR5 is also shown in the adult rat hippocampus, whereby expression is localized predominantly in PAPs (Lavialle et al., 2011). Here, Lavialle et al. (2011) also implicate PAP mGluR5 expression in PAP motility indicating that (i) expression levels may be higher in PAPs compared to somata, and (ii) astrocytic mGluR5 is functionally significant to synaptic activity through effects on PAP motility. It is possible that high degrees of heterogeneity in astrocytic gene expression impacts detection of GPCRs in whole cell analysis and minimizes potential contributions of mGluR5 to astrocyte-synapse interactions. Functionally, mGluR1/5s are also shown to exhibit dose-dependent response heterogeneity to agonist application, whereby low concentrations of agonists elicit single peak signals, and progressively higher concentrations produce multi-peak and plateaued responses in hippocampal astrocytes of young mice (Xie et al., 2012); differences which are illustrative of how Ca2+ dynamics vary according to GPCR receptor stimulation frequency and duration (Tang et al., 2015). However, the downstream implications of these differences in evoked Ca2+ responses are not fully understood and require further investigation, particularly in the adult mouse. Astrocytic mGluR1/5 activation and subsequent Ca2+ activity is also required for the generation of NMDAR-dependent SICs in neurons, thereby facilitating LTP in the rodent hippocampus (Fellin et al., 2004; Perea and Araque, 2005). Downregulated expression of astrocytic mGluR5 in the adult mouse CA1 has been shown to reduce the frequency of miniature excitatory post-synaptic currents (mEPSCs), thereby impairing excitatory synaptic function in this region (Liu C. et al., 2022). Furthermore, mGluR5 overexpression rescued these impairments. Although the mechanism by which this occurs is debated, it may involve increased astrocytic Ca2+ elevations and probability of gliotransmitter release. Accordingly, mGluR1/5 are frequently cited as likely mediators of astrocytic glutamate exocytosis and gliotransmission (Volterra and Meldolesi, 2005; Hamilton and Attwell, 2010; Mahmoud et al., 2019).
The P2Y purinoceptor 1 (P2YR1) is another Gq-coupled GPCR shown to be present in PAPs located at excitatory synapses in mouse hippocampal slices (Domercq et al., 2006; Santello et al., 2011; Shigetomi et al., 2018). It has been shown that P2YR1-PLC-IP3- mediated Ca2+ elevations induce gliotransmission and NMDAR-dependent synaptic potentiation. However, these synaptic effects of astrocytic P2YR1-mediated Ca2+ elevations are not sufficient on their own but require the presence of permissive, homeostatic factors like TNFα (Domercq et al., 2006; Santello et al., 2011). Furthermore, while mGluR1 antagonist application reduced Ca2+ signaling in PAPs, combined application of both mGluR1 and P2YR1 antagonists reduced Ca2+ signaling even further (Tang et al., 2015). Hence, it is likely that both receptors contribute to Gq-mediated synaptic effects at mouse CA3-CA1 synapses in accordance with surrounding neuronal activity (Tang et al., 2015) and the presence of supporting proteins such as TNFα, controlling stimulus-secretion coupling in astrocytes (Santello et al., 2011). However, while the above evidence indicates that endogenous Gq-GPCR activation in astrocytes promotes intracellular and synaptic processes that are expected to enhance memory, Reichenbach et al. (2018) show that inhibition of astrocytic P2YR1 is protective against spatial learning and memory impairment in the APP/PS1 mouse model of Alzheimer’s Disease (AD). In this case, the maladaptive impact of astrocytic P2YR1 on memory is likely due to the pathology of this AD model (including P2YR1 hyperactivity and gliosis), as opposed to being a reflection of the role of astrocytic P2YR1 in memory under physiological conditions – which remains to be determined.
Cannabinoid receptor 1 (CB1R) is a GPCR that depending upon agonist availability, can couple to either Gi or Gq proteins (Lauckner et al., 2005), but preferentially to Gi proteins, and will therefore be discussed in more detail below (section “4.1.3. Endogenous Gi-GPCRs”).
The presence of the Gq-coupled α1-adrenoceptor (α1AR) on adult mouse hippocampal astrocytes has been illustrated in vivo (Srinivasan et al., 2015), in situ (Chai et al., 2017) and through scRNA-seq (Batiuk et al., 2020). This receptor seems to be necessary for the initial peak in startle-evoked intracellular Ca2+ activity, but not for slow rising elevations thereafter (Srinivasan et al., 2015). This finding is substantiated by those of Oe et al. (2020) who show α1AR stimulation in cortical astrocytes mediates sharp elevations in intracellular Ca2+ upon short stimulus durations, and surges of Ca2+ activity upon neuronal burst firing. While this was observed in cortical regions, we speculate that this mechanism also holds true in the hippocampus given the findings from Srinivasan et al. (2015), who showed that α1AR-mediated Ca2+ elevations display peaks of activity as opposed to prolonged elevations. Astrocytic α1AR are also associated with regulating inhibitory dynamics in neural circuits (Wahis and Holt, 2021). Indeed, astrocytic α1ARs are implicated (alongside those expressed in interneurons) in increasing GABAergic neurotransmission.
Together, these studies suggest that Ca2+ elevations are a hallmark of astrocytic Gq-GPCR activation in the adult mouse hippocampus, whereby these dynamics directly impact synaptic activity. Indeed, astrocytic mGluR1/5 activation stimulates generation of mEPSCs and SICs in nearby neurons, thereby facilitating synaptic potentiation. Activation of astrocytic P2YR1s also seem to contribute to potentiating excitatory synapses, while astrocytic α1AR stimulation appears to regulate inhibitory neurotransmission. Overall, very little is known about the behavioral consequences of endogenous Gq-GPCR activity under physiological conditions (Table 1), prompting the requirement of in vivo techniques to fill this gap in the literature.
4.1.2. Endogenous Gs-GPCRs
A number of different Gs-GPCRs are expressed in adult mouse hippocampal astrocytes, including the adenosine 2A receptor (A2AR) and βARs (Table 2). A2AR to be present at low levels in hippocampal astrocytes of adult mice (Batiuk et al., 2020). Furthermore, A2AR expression is known to increase upon neuroinflammatory brain insults whereby astrocytic A2AR activation increases cell proliferation and activation, indicating roles for A2AR in mediating astrogliosis, a known hallmark of AD (Boison et al., 2010; Lopes et al., 2021). Astrocytic A2ARs have indeed been linked to AD such that mRNA expression of A2AR in human hippocampal astrocytes is upregulated with disease progression (while expression is downregulated during normal aging) and strongly correlated with pathological amyloid beta and microtubule-associated protein tau accumulation (Orr et al., 2015). Furthermore, Orr et al. (2015) show that increased activity of astrocytic A2ARs across the whole brain impairs memory retention in a mouse model of AD. Functionally, activation of A2AR in cultured rat astrocytes is shown to increase cAMP levels (Murphy et al., 1991; Cristóvão-Ferreira et al., 2013). This typical Gs-coupled effect of cAMP elevations has also been associated with inhibiting nitric oxide production in astrocytes via A2AR activation (Brodie et al., 1998; Boison et al., 2010). Nitric oxide is an important retrograde messenger for LTP and LTD induction whereby extracellular nitric oxide plays a role in NMDAR-dependent LTP by action on postsynaptic Ca2+ channels (Paul and Ekambaram, 2011; Pigott and Garthwaite, 2016). We speculate that memory impairment upon activation of astrocytic A2ARs may be due to nitric oxide inhibition and subsequent impairment in LTP induction. However, the role of astrocytic A2AR activation under physiological conditions remains to be determined.
The presence of another group of Gs-GPCRs, βARs, has also been illustrated pharmacologically in hippocampal astrocytes of adult rodents (Salm and McCarthy, 1992; Gao et al., 2016; Oe et al., 2020). βAR activation is also known to mediate cAMP accumulation (Catus et al., 2011; Vardjan et al., 2014) leading to increased process branching in primary rat cultures (Vardjan et al., 2014), indicating a role for βARs in morphological plasticity. Astrocytic βAR activity in the mouse hippocampus is also shown to enhance LTP (Walker et al., 2017). In the rat hippocampus, astrocytic βAR antagonism prior to learning had no effect on short-term memory but significantly impaired recent and remote long-term memory, indicating activation of astrocytic βARs promotes memory consolidation (Gao et al., 2016). Furthermore, the role of βAR in long term memory appears to be mediated specifically by a β2AR-dependent increase in lactate release during learning. However, most studies do not discriminate between different βARs, limiting our understanding of subtype-specific effects.
Together, these studies suggest that endogenous A2AR and βAR activation in hippocampal astrocytes of the adult mouse mediate increased cAMP production with no effect on Ca2+ levels. However, Gs-GPCR effects on learning and memory appear to be receptor specific, whereby A2AR stimulation results in memory impairment in an AD mouse model (with so far unknown effects under physiological conditions), but βAR stimulation enhances memory.
4.1.3. Endogenous Gi-GPCRs
Various Gi-GPCRs are also endogenously expressed in hippocampal astrocytes of adult mice (Table 3), including mGluR3, a group II mGluR (mGluR2/3). Strong expression profiles have been shown for mGluR3 in hippocampal astrocytes of adult mice by immunostaining (Sun et al., 2013) and scRNA-seq data (Batiuk et al., 2020). mGluR3 expression has also been observed in hippocampal astrocytes of the rhesus monkey, and was shown by immunoelectron microscopy to be located primarily at PAPs (Jin et al., 2018). This observation is congruent with findings by Lavialle et al. (2011) in adult rats, who showed that in vivo mGluR3 expression is largely confined to PAPs. Together, findings from Lavialle et al. (2011) and Jin et al. (2018) indicate that mGluR3 expression on hippocampal PAPs is conserved across species, although this is yet to be proven in adult mice. Another key Gi-coupled GPCR, the GABAb receptor (GABAbR), was also shown to be present in mouse hippocampal astrocytes by RNA sequencing and by pharmacological induction of Ca2+ signaling (Chai et al., 2017; Durkee et al., 2019; Batiuk et al., 2020). GABAbR expression has also been observed in PAPs of hippocampal astrocytes in the adult rat by immunostaining (Charles et al., 2003), but is yet to be observed in the adult mouse hippocampus.
Generally, activation of astrocytic Gi-GPCRs stimulates signaling pathways that elevate Ca2+ levels and inhibit cAMP production (Figure 1 and Table 3). Indeed, activation of astrocytic mGluR2/3, by a non-specific agonist in hippocampal mossy fiber circuits (DG-CA3) in vivo, elevates Ca2+ (Haustein et al., 2014). However, using the same agonist Chai et al. (2017) were unable to detect Ca2+ elevations in CA1 astrocytes. This could reflect regional differences for astrocytes in the hippocampus, concerning (i) differences in mGluR2/3 expression levels, (ii) interactions of mGluR2/with different G proteins or (iii) functional heterogeneity of the astrocytes themselves. For example, it was recently shown in HEK cells that Gi-GPCR-induced Ca2+ elevations are mediated by the Gi-βγ subunit, and that mobilization of the Gi-βγ subunit requires Gq co-activation (Pfeil et al., 2020). Thus, Gi-GPCR activation amplifies Gq-mediated Ca2+ responses via the PLC-IP3 signaling pathway – although the degree to which Gq co-activation is required for Gi-GPCR-induced Ca2+ signaling specifically in astrocytes, remains to be determined (Figure 1). Astrocytic GABAbR activation in mossy fiber circuits is also evokes Ca2+ events in parallel with mGluR2/3 (Haustein et al., 2014), with substantial impacts on neural circuit activity. For example, Covelo and Araque (2018) found that astrocyte GABAbR-dependent Ca2+ elevations are triggered in situ by interneuron stimulation in the mouse stratum radiatum and mediate IP3-dependent synaptic potentiation and depression. Furthermore, astrocyte GABAbR activity in mouse hippocampal slices mediated interneuron-induced potentiation of excitatory neurotransmission (Perea et al., 2016).
The effects of Gi-GPCR stimulation on cAMP levels is less studied than effects on Ca2+ levels. However, GABAbR activation is known to be negatively coupled to cAMP in rat primary astrocyte cultures (Patte et al., 1999). It has also been shown for hippocampal astrocytes that, quite counterintuitively, mGluR3 activity can potentiate cAMP elevations elicited by Gs-coupled βARs via Gi-βγ subunits as illustrated in Figure 1 (Tang and Gilman, 1991; Walker et al., 2017). Interestingly, mGluR3 potentiation of βAR-induced cAMP elevations disrupts βAR-mediated LTP induction and contextual fear learning, to a similar degree as direct βAR antagonism. It is suggested that accumulation of astrocytic cAMP has this antagonistic effect through subsequent activation of presynaptic adenosine receptors, ultimately attenuating LTP induction. Indeed, βARs and mGluR3 are co-localized on hippocampal astrocytes and are therefore optimally located to cooperate in regulating cAMP signaling, synaptic potentiation, and memory (Walker et al., 2017).
Cannabinoid receptor 1 (CB1R) is a GPCR thought to preferentially couple to Gi proteins. However, depending upon agonist availability, CB1R ligand binding stabilizes distinct receptor conformations thereby favoring coupling to either Gi or Gq proteins (Lauckner et al., 2005; Tables 2, 3). Particular agonists (for example WIN55, 212-2) stabilize CB1R confirmations in favor of Gq-coupling (Lauckner et al., 2005). However, disentangling the contributions of Gi- and Gq-coupled CB1R pathways requires further detailed investigation. CB1R expression has been observed in astrocytes of the adult mouse hippocampus by immunostaining, electron microscopy, and by functional responses to application of endogenous and synthetic agonists (Navarrete and Araque, 2008; Han et al., 2012; Gutiérrez-Rodríguez et al., 2018). CB1R activation is known to stimulate the PLC-IP3 signaling pathway and mediate Ca2+ release from intracellular stores (Navarrete and Araque, 2008), which could be due to Gi- and/or Gq-coupling, as both are shown to mediate IP3-dependent Ca2+ signaling by action of the Gi-βγ and Gq-α subunits respectively (Zeng et al., 2003; Figure 1). These CB1R-evoked Ca2+ elevations have been shown to increase extracellular D-serine levels (Robin et al., 2018) and induce NMDAR-dependent SICs in nearby excitatory neurons in the mouse CA1 (Navarrete and Araque, 2008), indicating an important role for astrocytic CB1Rs in synaptic potentiation in the mouse hippocampus. Indeed, temporal coincidence of CB1R-evoked Ca2+ signaling in astrocytes with postsynaptic activity is shown to induce LTD at synapses close to the source of endocannabinoid, but LTP at relatively more distant synapses in mouse hippocampal slices (Navarrete and Araque, 2010; Min and Nevian, 2012; Gómez-Gonzalo et al., 2015). It has also been shown exogenous cannabinoid stimulation of astrocytic CB1Rs in vivo evokes LTD and spatial working memory impairments in mice by activation of NMDAR and internalization of AMPAR in CA1 post-synaptic neurons (Han et al., 2012). Together with the observation that total CB1R deletion in hippocampus impairs memory extinction (Marsicano et al., 2002), the above studies show that CB1Rs in hippocampal astrocytes have a physiological role in regulating memory retention/extinction processes.
In summary, endogenously expressed astrocytic CB1R, GABAbR and mGluR2/3 evoke Ca2+ elevations by Gi-βγ subunit stimulation of the PLC-IP3 signaling pathway, but it remains unclear whether Gi-βγ-mediated Ca2+ elevations can occur independently of Gq-GPCR activity. Endogenous Gi-GPCRs also downregulate cAMP production in astrocytes via Gi-α subunit activity. However, co-activation of mGluR3 with βAR seems to potentiate βAR-Gs-mediated elevation of cAMP levels. A number of studies also show astrocytic Gi-GPCR activity impacts synaptic potentiation and memory processes.
5. Comparative assessment of endogenous GPCRs and DREADDs
5.1. Endogenous Gq-GPCRs vs. Gq-DREADDs
A number of studies have directly compared endogenous Gq-GPCR activation to that of Gq-DREADDs in astrocytes. Here, we integrate these studies with those investigating either endogenous Gq-GPCRs or Gq-DREADDs individually, to obtain more insight into the astrocytic response characteristics, and the synaptic and behavioral effects of endogenous Gq-GPCR versus Gq-DREADD stimulation.
It has been shown that the amplitude and temporal characteristics of evoked Ca2+ responses in hippocampal astrocytes are comparable for Gq-DREADD and endogenous Gq-GPCR activation (Agulhon et al., 2013; Durkee et al., 2019; Vaidyanathan et al., 2021). Indeed, endogenous mGluR1/5 stimulation evokes Ca2+ elevations in the mouse hippocampus with stimulus-strength response heterogeneity (Xie et al., 2012), and similarly, astrocytic Gq-DREADD stimulation in the cortex produces multi-peak Ca2+ signals at low CNO concentrations (0.2 mg/kg) and plateaued responses at higher doses (1 mg/kg) (Bonder and McCarthy, 2014). Furthermore, chronic stimulation of endogenous Gq-GPCRs is associated with receptor desensitization (Rajagopal and Shenoy, 2018; Dyer-Reaves et al., 2019). This aligns with observations that Gq-DREADD activation (due to chronic ligand availability) silences evoked Ca2+ responses after just a few minutes of repeated stimulation (Vaidyanathan et al., 2021). Stimulation of astrocytic Gq-DREADDs has yet to illustrate the transient, single peak Ca2+ elevations which can be observed upon endogenous α1AR stimulation (Srinivasan et al., 2015; Oe et al., 2020). It is unclear at present why these α1AR effects cannot be replicated by Gq-DREADDs (hM3Dq), however it could be a reflection of the systemic ligand delivery system resulting in chronic ligand availability, as opposed to acute spikes in endogenous, locally released, ligand concentrations (Claes et al., 2022), or due to underlying functional differences between endogenous α1ARs and the muscarinic-derived DREADDs.
Direct comparisons by Durkee et al. (2019) show that stimulation of Gq-DREADDs or endogenous Gq-GPCRs both evoke Ca2+ elevations by PLC-IP3 signaling cascades in the somata and processes of hippocampal astrocytes (Figure 2). This observation is verified by other investigations of Gq-DREADDs (Chai et al., 2017; Vaidyanathan et al., 2021) and endogenous Gq-GPCRs (Xie et al., 2012; Srinivasan et al., 2015; Oe et al., 2020; Lia et al., 2021; Lawal et al., 2022). Interestingly, the specific IP3R subtype mediating astrocytic Ca2+ fluctuations may indicate the subcellular origin of these fluctuations (Sherwood et al., 2021), thereby influencing the impact of astrocytic Ca2+ elevations on synaptic function and behavior. The predominant narrative is that IP3R2 is responsible for Gq-GPCR Ca2+ elevations, with early reports showing that genetic knock-out of IP3R2 abolished Ca2+ transients in astrocytes, without impacting SIC generation, neuronal ionotropic glutamate receptor activation (Fiacco et al., 2007), neuronal Ca2+ activity (Fiacco et al., 2007; Petravicz et al., 2008), spontaneous or evoked EPSCs (Petravicz et al., 2008; Agulhon et al., 2010) or long-term synaptic plasticity mechanisms (Petravicz et al., 2014). However, these studies do not account for Ca2+ signaling in PAPs, which likely has a more significant impact on neuronal function than somatic Ca2+ fluctuations (Haustein et al., 2014; Srinivasan et al., 2015; Sherwood et al., 2021). Indeed, more recent studies indicate that PAP Ca2+ activity relies primarily upon IP3R2-independent mechanisms (Srinivasan et al., 2015; Liu J.-H. et al., 2022). For example, IP3R1 and IP3R3 are shown to contribute to Ca2+ signaling in PAPs, alongside IP3R2 (Sherwood et al., 2017). As a result, it could be that IP3R2 knockout does not significantly impact neuronal function due to the compensating ability of IP3R1 and IP3R3 in PAPs (Bazargani and Attwell, 2016). Accordingly, Sherwood et al. (2021) speculate that astrocytic IP3 receptors have subtype-specific cellular distributions according to their functional properties, however this has yet to be empirically assessed. The predominance and relative importance of each IP3R subtype within PAPs remains an interesting open question – one which could contribute to our understanding of how endogenous Gq-GPCR and Gq-DREADD subcellular localization and evoked Ca2+ signaling impacts downstream synaptic and behavioral effects.
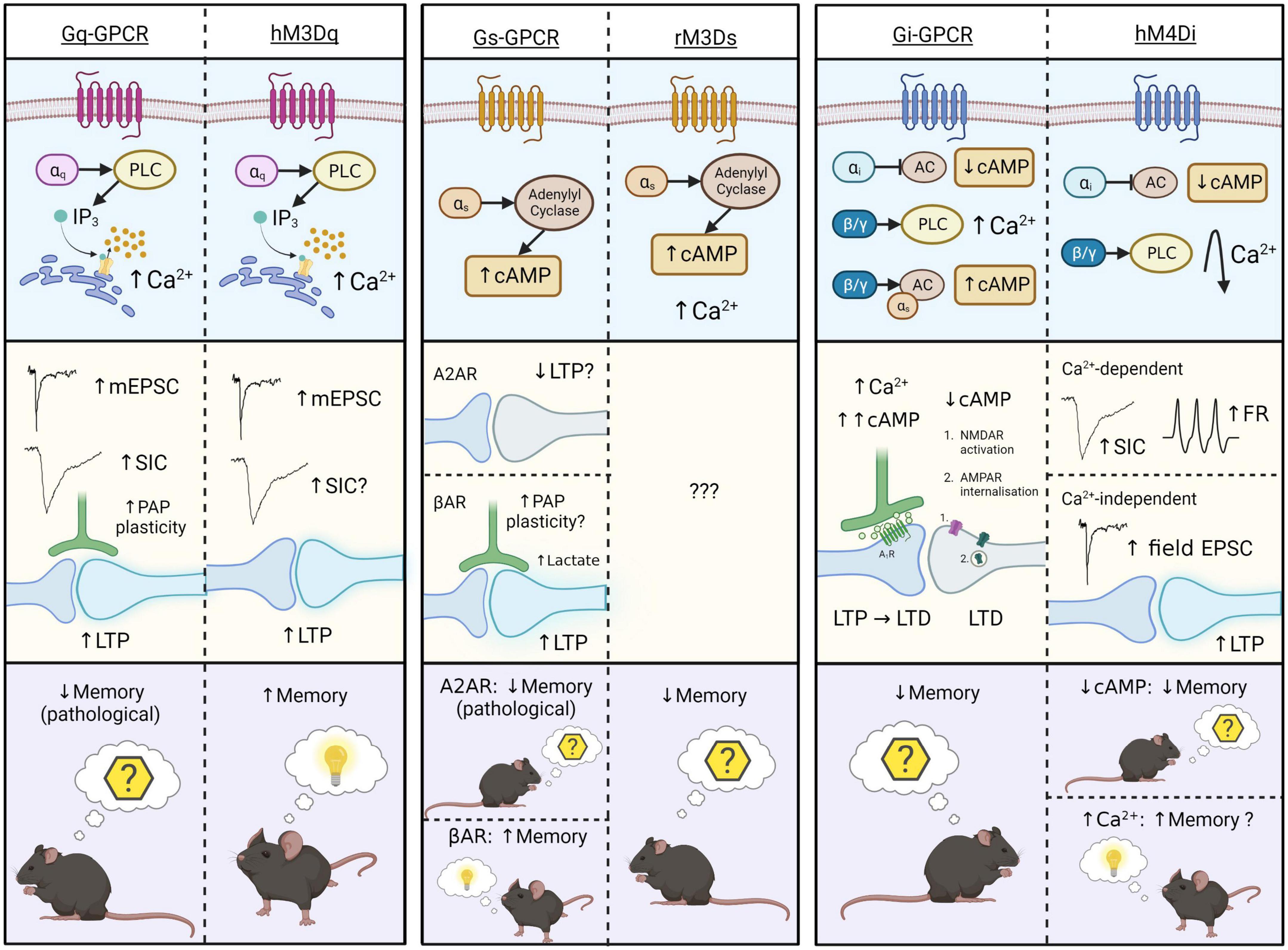
Figure 2. Schematic representation of intracellular (top), synaptic (middle), and behavioral (bottom) effects of endogenous Gq-, Gs-, and Gi-GPCR stimulation in astrocytes, and that of corresponding Gq-, Gs-, and Gi-DREADDs (hM3Dq, rM3Ds, and hM4Di). Astrocytic Gq-GPCRs/hM3Dq activation (left) evokes Ca2+ elevations via the PLC-IP3 signaling pathway. This increases mEPSCs, SICs (conflicting evidence for hM3Dq), LTP and PAP plasticity at synapses. Only one study illustrates memory-related behavioral effects of Gq-GPCR activation in astrocytes – in pathological condition (AD) Gq-GPCR activation mediated memory impairment, while hM3Dq activations mediate memory enhancement under physiological conditions. Astrocytic Gs-GPCR and rM3Ds activation (centre) evokes cAMP elevations through stimulation of adenylyl cyclase (AC), and rM3Ds activations evoke Ca2+ elevations through unknown mechanisms. We speculate that astrocytic A2AR activation impairs LTP. Astrocytic βAR activation is shown to mediate morphological plasticity, possibly including the PAPs, and lactate release, thereby stimulating LTP at synapses. These synaptic effects have not been shown for the Gs-DREADD hM3Ds, illustrating a major gap in the literature. Behavioral effects of astrocytic A2AR and rM3Ds activation is on memory extinction, however βAR stimulation evokes memory enhancement. Astrocytic Gi-GPCR and hM4D activation (right) evokes reductions in cAMP levels by inhibition of AC. However, the endogenous Gi-GPCR mGluR3 can also elevate cAMP levels by action of the βγ subunit, through potentiation of Gs-coupled elevations. Stimulation of Gi-GPCRs evokes Ca2+ elevations via the PLC signaling pathway. Corresponding synaptic effects of cAMP- and Ca2+-elevations include synaptic potentiation and depression, whereby cAMP accumulation leads to activation of presynaptic adenosine 1 receptors (A1R) and LTP disruption. Downregulated cAMP by astrocytic Gi-GPCRs mediate post-synaptic NMDAR activation and AMPAR internalization, thereby evoking LTD. Together these intracellular and synaptic effects, evoked by lowering astrocytic cAMP-levels, have a negative effect on memory retention. Astrocytic Gi-DREADD (hM4Di) activation is also shown to evoke cAMP reductions and thereby inhibition of fear learning. In addition, other studies reported that astrocytic hM4Di activation can also evoke transient Ca2+ elevations that return to levels below baseline thereafter. These Ca2+ effects are shown to increase frequency of SICs, firing rates (FR) and LTP in nearby neurons, potentially having a positive effect on memory. The final effect of Gi-DREADD signaling on memory therefore seems to depend on the net outcome of cAMP and Ca2+ effects. Figure created with www.biorender.com.
A major, unresolved issue on Gq-DREADD activation effects in astrocytes, is their Ca2+-dependent effects on neurons. Endogenous Gq-GPCR-mediated Ca2+ activity is consistently shown to impact synaptic activity in the adult mouse hippocampus (Figure 2). However, early MrgA1 (Fiacco et al., 2007; Agulhon et al., 2010) and IP3R2 knockout studies (Fiacco et al., 2007; Petravicz et al., 2008, 2014; Agulhon et al., 2010; Bonder and McCarthy, 2014) contradict the hypothesis that astrocytic Gq-GPCR activation and subsequent Ca2+ elevations impact synaptic activity and plasticity mechanisms. To our knowledge, the source of this discrepancy is unknown, but likely represents (i) the uncharacterized complexity in the spatiotemporal dynamics of evoked Ca2+ signaling (e.g., subcellular localization of GPCRs in PAP domains), (ii) interactions between receptors [e.g., synergistic effects of mGluR3 (Gi) with βAR (Gs)] and (iii) the presence (or absence) of functionally relevant neuromodulators (e.g., TNFα). It is also worth noting that the inability of some studies to detect synaptic changes upon astrocytic Gq-DREADD stimulation is not strong evidence of absence for this hypothesis – rather, it simply shows that synaptic effects were not observed in this case. Indeed, there is substantial evidence in favor of the hypothesis that Ca2+ elevations evoked both by endogenously expressed astrocytic Gq-GPCRs, and Gq-DREADDs, alter synaptic activity in the adult mouse hippocampus (Figure 2).
At a behavioral level, Gq-DREADDs and endogenous Gq-GPCRs in hippocampal astrocytes are shown to impact memory (Adamsky et al., 2018; Reichenbach et al., 2018). However, while astrocytic Gq-DREADD activation enhances memory (Adamsky et al., 2018), endogenous astrocytic Gq-GPCR activation contributes to memory impairment in an AD mouse model (Reichenbach et al., 2018). While these results appear contradictory (Figure 2), the severity of pathology in this model impedes direct comparisons between endogenous and synthetic receptors. However, it could be that these maladaptive effects on memory are induced upon chronic, pathological Gq-GPCR activation in astrocytes, whereas the physiological activations have memory enhancing effects, similar to those observed by Adamsky et al. (2018). The impact of endogenous Gq-GPCR activation in astrocytes in the healthy brain is yet to be observed, and therefore the degree to which the behavioral consequences of endogenous and synthetic receptor stimulation are comparable in the healthy brain remains an open question.
Overall, these results suggest that improved methodologies and theoretical understanding has generated a loose consensus that astrocytic Gq-coupled receptor stimulation in the adult mouse hippocampus elevates Ca2+ levels and potentiates synaptic activity (Figure 2). However, it remains unclear whether subsequent behavioral effects are equally comparable (Figure 2).
5.2. Endogenous Gs-GPCRs vs. Gs-DREADDs
To our knowledge, Orr et al. (2015) is the only study to directly compare Gs-GPCR and Gs-DREADD activation effects in astrocytes. Activation of endogenous, and synthetic Gs-GPCRs in adult mouse hippocampal astrocytes, mediates increased cAMP levels without impacting intracellular Ca2+ concentrations (Orr et al., 2015). This comparison is substantiated by other studies, on hippocampal astrocytes, showing cAMP elevation upon Gs-DREADD activation (Oe et al., 2020) and upon Gs-coupled βAR activation in vitro (Catus et al., 2011) and in situ (Walker et al., 2017). Activation of endogenous βARs stimulates astrocyte protrusions in vitro (Vardjan et al., 2014), lactate release (Zhou et al., 2019) and LTP induction (Walker et al., 2017) at synapses. Furthermore, astrocytic A2AR activity is thought to impair LTP induction via inhibition of nitric oxide production (Boison et al., 2010). However, while the intracellular effects of stimulating endogenous Gs-GPCRs and Gs-DREADDs in astrocytes are comparable, synaptic effects of endogenous Gs-GPCR activity have not yet been replicated by Gs-DREADD experiments (Figure 2), representing a major gap in the literature yet to be addressed.
Astrocytic Gs-DREADD activation has also been shown to elicit Ca2+ elevations (Chai et al., 2017; Nagai et al., 2021), however an effect of endogenous Gs-GPCRs on calcium elevation has not been reported so far. Accordingly, we speculate that Gs-DREADD-evoked Ca2+ elevations are DREADD-specific, and due to promiscuous binding to Gq proteins, given the relative structural homogeneity between rM3Ds and hM3Dq.
At the behavioral level, astrocytic Gs-DREADD stimulation is associated with impaired memory consolidation (Orr et al., 2015), in line with our understanding of astrocytic A2AR activation effects on memory (Boison et al., 2010; Paul and Ekambaram, 2011; Orr et al., 2015). However, activation of βAR is shown to promote long term memory consolidation in rats (Gao et al., 2016). Hence, while both βAR and A2AR evoke cAMP elevations (Catus et al., 2011; Orr et al., 2015), the effects of βAR activation on memory oppose that of A2AR. This discrepancy could be explained by manipulating A2AR expression in whole brain (Orr et al., 2015) compared to just in hippocampus (Gao et al., 2016), or the presence of divergent downstream mechanisms eliciting distinct synaptic effects (Figure 2). Given this, further receptor-specific research is required to uncover the precise nature of signaling pathways downstream of cAMP elevations, including the potential influence of different receptor expression levels, subcellular localization and interactions with Gi and Gq proteins.
5.3. Endogenous Gi-GPCRs vs. Gi-DREADDs
Finally, we compare the intracellular signaling mechanisms, synaptic and behavioral consequences of endogenous Gi-GPCR and Gi-DREADD activation in astrocytes. We will first discuss these effects in relation to cAMP, followed by those mediated by Ca2+ signaling.
Endogenously expressed Gi-GPCRs in astrocytes are characterized by their inhibitory effects on adenylyl cyclase activity and subsequent reductions to cAMP levels (Patte et al., 1999; Han et al., 2012; Walker et al., 2017), an effect that has been replicated by Gi-DREADDs in the rodent hippocampus (Jones et al., 2018). This Gi-DREADD-mediated attenuation of intracellular cAMP signaling is also shown to impair stress-enhanced fear learning (Jones et al., 2018). Impaired remote memory retrieval has also been observed upon astrocytic Gi-DREADD activation (Kol et al., 2020), although the contribution of cAMP was not measured in this study. Similar behavioral effects were observed upon stimulation of endogenous mGluR3 in the mouse hippocampus by Walker et al. (2017), which, in contrast to the effects of Gi-DREADD activation, were associated with cAMP upregulation due to interactions between the mGluR3 βγ subunit and coactivated, Gs-coupled βARs (Walker et al., 2017). Testing whether this effect is conserved in DREADDs signaling would further strengthen the notion that DREADDs can accurately mimic their endogenous counterparts. However, receptor expression levels and sub-cellular localization may impact the ability of Gi-DREADDs to interact with other receptors, limited their ability to replicate this particular effect. Overall, there seems to be a shared effect of endogenous and synthetic astrocytic Gi-coupled receptors in that both impair contextual and stress-enhanced fear memory and remote retrieval, however the precise role of cAMP in this remains unclear (Figure 2).
Gi-DREADD activation in astrocytes has also been shown to elicit Ca2+-independent potentiation of excitatory CA1 synapses (Van Den Herrewegen et al., 2021; Figure 2). These potentiating effects on excitatory synapes, while Ca2+-independent and thus possibly cAMP-related, are expected to cause memory enhancement – however Ca2+-independent memory enhancement has not been empirically proven for endogenous Gi-GPCRs or Gi-DREADDs in astrocytes. As a result, it is unclear if Ca2+-independent, Gi-DREADD-evoked synaptic potentiation is (i) due to cAMP downregulation or other mechanisms, (ii) can evoke memory enhancement, and (iii) whether these effects mimic endogenous processes or are Gi-DREADD-specific. The disparity between synaptic and behavioral effects of Gi-DREADD and endogenous Gi-GPCR activation in astrocytes indicate that future studies are needed to further establish the downstream effects of cAMP modulation by astrocytic Gi-GPCRs and the precise manner in which these effects diverge from those elicited by Gi-DREADDs. Increased understanding of brain region- and receptor-specific differences, expression levels and interactions with other G proteins will likely aid in determining how Gi-coupled receptor activation modulates cAMP and memory processes.
In accordance with the literature outlining the effects of endogenous Gi-GPCR stimulation, some studies show that Gi-DREADDs evoke Ca2+ elevations (Haustein et al., 2014; Durkee et al., 2019; Kol et al., 2020; Vaidyanathan et al., 2021), while others indicate that they do not (Chai et al., 2017; Nam et al., 2019; Van Den Herrewegen et al., 2021). However, as shown in Figure 2, both Gi-GPCRs and Gi-DREADDs have been shown to elicit Ca2+ elevations via the PLC-IP3 signaling pathway (Navarrete and Araque, 2008; Haustein et al., 2014; Covelo and Araque, 2018; Kol et al., 2020; Vaidyanathan et al., 2021). However, while astrocytic Gi-DREADD activation in mouse hippocampus evokes moderate reductions in astrocytic Ca2+ levels after initial transient peaks (Kol et al., 2020), this has not been observed upon endogenous Gi-GPCR stimulation in astrocytes (Figure 2). The finding that at least some Gi-GPCRs require co-activation of Gq-GPCRs to evoke Ca2+ elevations by action of the βγ subunit on the PLC-IP3 signaling pathway (Pfeil et al., 2020) could explain why some studies show robust Gi-evoked Ca2+ signaling, while others show little to no effect – if constitutive Gq activity is low, this could theoretically impact the capacity of Gi-GPCRs to stimulate Ca2+ signaling. Whether or not Gq-dependence is also a feature of Gi-GPCR and Gi-DREADD-mediated Ca2+ activity specifically in mouse hippocampal astrocytes has yet to be determined. However, further investigation of this mechanism in endogenous Gi-GPCRs and Gi-DREADDs could clarify the degree to which Ca2+ signaling mechanisms in Gi-DREADDs mimic the endogenous process even further.
Taken together, these studies show that astrocytic Gi-DREADDs mimic astrocytic Gi-GPCR activity in many aspects. This includes cAMP downregulation, PLC-IP3-induced Ca2+ elevations (in some but not all instances), synaptic potentiation and memory impairment (Figure 2). However, there remain some distinguishing factors. It has not yet been shown whether endogenous Gi-GPCRs in astrocytes evoke Ca2+ elevations which share the same temporal profile as observed upon Gi-DREADD stimulation. Unlike endogenous Gi-GPCRs in astrocytes, astrocytic Gi-DREADDs can evoke increases to cAMP levels, as is shown to occur upon co-activation of mGluR3 and Gs-coupled βAR. Furthermore, while activation of astrocytic Gi-DREADDs elicits synaptic effects indicative of memory enhancement, this has not yet been shown for endogenous Gi-GPCRs (Figure 2).
6. Concluding remarks
The most frequently studied GPCR subtype, Gq, seems to show a high level of congruence between DREADD-induced and endogenous GPCR effects. This is particularly true in relation to downstream mechanisms elicited by their activation. Indeed, PLC-IP3 signaling mechanisms evoke Ca2+ elevations with similar physical and temporal properties upon activation of both endogenous Gq-GPCRs and Gq-DREADDs. Subsequent effects on neuronal activity are also comparable, indicating DREADDs accurately mimic astrocytic GPCR-mediated intracellular and synaptic effects in the adult mouse hippocampus. Limited available data show memory-related behaviors were not comparable; however, this likely relates to difficulties directly comparing behaviors between physiological and pathological conditions. With respect to Gs-coupled receptors, activation of Gs-DREADDs appears to replicate intracellular cAMP elevations associated with endogenous A2AR and βAR stimulation in astrocytes. However, the behavioral consequences of Gs-DREADD stimulation in astrocytes mimic only those observed upon βAR activation, opposing that of A2AR. This is likely the result of diverging downstream signaling pathways and synaptic effects which require further region-specific characterization. Similarly, while activation of Gi-DREADDs mimics that of endogenous Gi-GPCRs in some ways, there are a number of exceptions. Both appear to inhibit cAMP production, inconsistently evoke Ca2+ elevations, and induce memory impairment. However, the ability of mGluR3 to potentiate cAMP has not yet been reported in Gi-DREADD studies, while the Gi-DREADD-mediated enhancement of synaptic potentiation and memory acquisition has not yet been shown for endogenously expressed GPCRs.
Mounting evidence suggests that the relationship between intracellular Ca2+ elevations, synaptic changes and behavior is not a black and white binary, but rather depends upon activation of specific receptor subtypes, likely at specific sub-cellular locations and timepoints – the complexity of which is yet to be fully understood (Shigetomi et al., 2008; Bazargani and Attwell, 2016). With this in mind, disparities between the effects of astrocytic DREADDs and their endogenous counterparts could be due to a variety of factors. First, we must consider that although DREADDs are often assumed to bind specifically to Gq-, Gs- or Gi-proteins, it is likely that they mimic downstream mechanisms specific to their respective parent muscarinic receptors, which are not necessarily Gq-, Gs-, or Gi-specific. While this question of DREADD specificity may complicate the interpretation of experiments aiming to elucidate the mechanisms of specific G-protein activation, it does increase the degree to which DREADDs accurately mimic endogenous GPCR activity. Indeed, these considerations may explain observed functional differences between DREADDs and some endogenous receptors. That is, although DREADDs aim to simulate generalized downstream mechanisms of Gq-, Gs-, and Gi-GPCR activation, imperfect reflections of other receptor types such as the α1AR, may simply reflect their muscarinic lineage. Thus, a certain degree of functional distinction between DREADDs and endogenous GPCRs is likely inherent to experimental technique itself, without nullifying its utility. Furthermore, it is known that current methods cannot accurately mimic endogenous spatiotemporal expression (McNeill et al., 2021; Shen et al., 2021) or excitation patterns (Nimmerjahn and Bergles, 2015; Sherwood et al., 2021). Indeed, the stochastic spatial distribution of DREADD expression likely does not reflect endogenous distributions of GPCRs. By extension, this could impact synaptic and behavioral effects of astrocytic DREADD stimulation, limiting their equivalence to the effects of endogenous GPCR activity. Incorporation of morphological examinations in future DREADD experiments in astrocytes could shed light on this issue. Furthermore, the chronic availability of DREADD ligands could be contributing factor to inconsistent results between DREADD-mediated effects and those evoked by acute and local stimulation of endogenous GPCRs, given differing temporal dynamics of receptor stimulation (Claes et al., 2022).
This comparative assessment suggests that controversies arising from astrocytic DREADD experiments are largely due to the sheer complexity of the system at hand. Increased research in recent years has, to a certain extent, clarified the physiological plausibility of DREADDs in the study of astrocytic GPCRs and their roles at the synapse and in memory. All GPCR subtypes assessed here show some degree of similarity between effects of DREADDs and endogenous GPCRs. Furthermore, it seems that a higher degree of consistency between DREADDS and endogenous receptors is achieved with increased research. We therefore expect the current understanding of GPCR activity in astrocytes to improve accordingly. Manipulating astrocyte activity using DREADDs can and has illustrated important roles for these cells at synapses, within larger networks and in behavior. This method, in combination with the study of endogenous GPCRs, can therefore provide indications as to the molecular and cellular mechanisms mediating synaptic and behavioral effects. Overall, the use of DREADDs in astrocytes is at least, hypothesis generating. But, at best, can provide us unprecedented access to the intersection between astrocytic GPCR physiology and synaptic function and behavior.
Author contributions
SL contributed to the conceptualization, manuscript selection and reading, visualization, and writing of the draft. AM contributed to the manuscript selection and editing. MV contributed to the conceptualization, review, editing, and supervision. All authors contributed to the article and approved the submitted version.
Conflict of interest
The authors declare that the research was conducted in the absence of any commercial or financial relationships that could be construed as a potential conflict of interest.
Publisher’s note
All claims expressed in this article are solely those of the authors and do not necessarily represent those of their affiliated organizations, or those of the publisher, the editors and the reviewers. Any product that may be evaluated in this article, or claim that may be made by its manufacturer, is not guaranteed or endorsed by the publisher.
References
Aboufares El Alaoui, A., Jackson, M., Fabri, M., de Vivo, L., and Bellesi, M. (2021). Characterization of subcellular organelles in cortical perisynaptic astrocytes. Front. Cell. Neurosci. 14:573944. doi: 10.3389/fncel.2020.573944
Adamsky, A., Kol, A., Kreisel, T., Doron, A., Ozeri-Engelhard, N., Melcer, T., et al. (2018). Astrocytic activation generates de novo neuronal potentiation and memory enhancement. Cell 174, 59–71.e14. doi: 10.1016/j.cell.2018.05.002
Agulhon, C., Boyt, K. M., Xie, A. X., Friocourt, F., Roth, B. L., and McCarthy, K. D. (2013). Modulation of the autonomic nervous system and behaviour by acute glial cell Gq protein-coupled receptor activation in vivo. J. Physiol. 591, 5599–5609. doi: 10.1113/jphysiol.2013.261289
Agulhon, C., Fiacco, T. A., and McCarthy, K. D. (2010). Hippocampal short- and long-term plasticity are not modulated by astrocyte Ca 2+ signaling. Science 327, 1250–1254. doi: 10.1126/science.1184821
Agulhon, C., Petravicz, J., McMullen, A. B., Sweger, E. J., Minton, S. K., Taves, S. R., et al. (2008). What is the role of astrocyte calcium in neurophysiology? Neuron 59, 932–946. doi: 10.1016/j.neuron.2008.09.004
Angulo, M. C., Kozlov, A. S., Charpak, S., and Audinat, E. (2004). Glutamate released from glial cells synchronizes neuronal activity in the hippocampus. J. Neurosci. 24, 6920–6927. doi: 10.1523/JNEUROSCI.0473-04.2004
Araque, A., Parpura, V., Sanzgiri, R. P., and Haydon, P. G. (1999). Tripartite synapses: Glia, the unacknowledged partner. Trends Neurosci. 22, 208–215. doi: 10.1016/s0166-2236(98)01349-6
Arizono, M., Inavalli, V. V. G. K., Panatier, A., Pfeiffer, T., Angibaud, J., Levet, F., et al. (2020). Structural basis of astrocytic Ca2+ signals at tripartite synapses. Nat. Commun. 11:1906. doi: 10.1038/s41467-020-15648-4
Armbruster, B. N., Li, X., Pausch, M. H., Herlitze, S., and Roth, B. L. (2007). Evolving the lock to fit the key to create a family of G protein-coupled receptors potently activated by an inert ligand. Proc. Natl. Acad. Sci. U.S.A. 104, 5163–5168. doi: 10.1073/pnas.0700293104
Atasoy, D., and Sternson, S. M. (2018). Chemogenetic tools for causal cellular and neuronal biology. Physiol. Rev. 98, 391–418. doi: 10.1152/physrev.00009.2017
Badia-Soteras, A., Heistek, T. S., Kater, M. S. J., Mak, A., Negrean, A., Oever, M. C., et al. (2022). Retraction of astrocyte leaflets from the synapse enhances fear memory. Biol. Psychiatry doi: 10.1016/j.biopsych.2022.10.013
Batiuk, M. Y., Martirosyan, A., Wahis, J., de Vin, F., Marneffe, C., Kusserow, C., et al. (2020). Identification of region-specific astrocyte subtypes at single cell resolution. Nat. Commun. 11:1. doi: 10.1038/s41467-019-14198-8
Bazargani, N., and Attwell, D. (2016). Astrocyte calcium signaling: The third wave. Nat. Neurosci. 19, 182–189. doi: 10.1038/nn.4201
Bender, D., Holschbach, M., and Stöcklin, G. (1994). Synthesis of n.c.a. Carbon-11 labelled clozapine and its major metabolite clozapine-N-oxide and comparison of their biodistribution in mice. Nuclear Med. Biol. 21, 921–925. doi: 10.1016/0969-8051(94)90080-9
Bernardinelli, Y., Randall, J., Janett, E., Nikonenko, I., König, S., Jones, E. V., et al. (2014). Activity-dependent structural plasticity of perisynaptic astrocytic domains promotes excitatory synapse stability. Curr. Biol. 24, 1679–1688. doi: 10.1016/j.cub.2014.06.025
Bindocci, E., Savtchouk, I., Liaudet, N., Becker, D., Carriero, G., and Volterra, A. (2017). Three-dimensional Ca2+ imaging advances understanding of astrocyte biology. Science 356:eaai8185. doi: 10.1126/science.aai8185
Boison, D., Chen, J.-F., and Fredholm, B. B. (2010). Adenosine signalling and function in glial cells. Cell Death Diff. 17, 1071–1082. doi: 10.1038/cdd.2009.131
Bonder, D. E., and McCarthy, K. D. (2014). Astrocytic Gq-GPCR-linked IP3R-dependent Ca2+ signaling does not mediate neurovascular coupling in mouse visual cortex in vivo. J. Neurosci. 34, 13139–13150. doi: 10.1523/JNEUROSCI.2591-14.2014
Brodie, C., Blumberg, P. M., and Jacobson, K. A. (1998). Activation of the A2A adenosine receptor inhibits nitric oxide production in glial cells. FEBS Lett. 429, 139–142. doi: 10.1016/s0014-5793(98)00556-0
Bushong, E. A., Martone, M. E., Jones, Y. Z., and Ellisman, M. H. (2002). Protoplasmic astrocytes in CA1 stratum radiatum occupy separate anatomical domains. J. Neurosci. 22, 183–192. doi: 10.1523/JNEUROSCI.22-01-00183.2002
Caroni, P., Donato, F., and Muller, D. (2012). Structural plasticity upon learning: Regulation and functions. Nat. Rev. Neurosci. 13:7. doi: 10.1038/nrn3258
Catus, S. L., Gibbs, M. E., Sato, M., Summers, R. J., and Hutchinson, D. S. (2011). Role of β-adrenoceptors in glucose uptake in astrocytes using β-adrenoceptor knockout mice. Br. J. Pharmacol. 162, 1700–1715. doi: 10.1111/j.1476-5381.2010.01153.x
Chai, H., Diaz-Castro, B., Shigetomi, E., Monte, E., Octeau, J. C., Yu, X., et al. (2017). Neural circuit-specialized astrocytes: Transcriptomic, proteomic, morphological, and functional evidence. Neuron 95, 531–549.e9. doi: 10.1016/j.neuron.2017.06.029
Charles, K. J., Deuchars, J., Davies, C. H., and Pangalos, M. N. (2003). GABA B receptor subunit expression in glia. Mol. Cell. Neurosci. 24, 214–223. doi: 10.1016/s1044-7431(03)00162-3
Christiansen, S. H., Selige, J., Dunkern, T., Rassov, A., and Leist, M. (2011). Combined anti-inflammatory effects of β2-adrenergic agonists and PDE4 inhibitors on astrocytes by upregulation of intracellular cAMP. Neurochem. Int. 59, 837–846. doi: 10.1016/j.neuint.2011.08.012
Claes, M., De Groef, L., and Moons, L. (2022). The DREADDful hurdles and opportunities of the chronic chemogenetic toolbox. Cells 11:1110. doi: 10.3390/cells11071110
Cornell-Bell, A. H., Finkbeiner, S. M., Cooper, M. S., and Smith, S. J. (1990). Glutamate induces calcium waves in cultured astrocytes: Long-range glial signaling. Science 247, 470–473. doi: 10.1126/science.1967852
Covelo, A., and Araque, A. (2018). Neuronal activity determines distinct gliotransmitter release from a single astrocyte. eLife 7:e32237. doi: 10.7554/eLife.32237
Cristóvão-Ferreira, S., Navarro, G., Brugarolas, M., Pérez-Capote, K., Vaz, S. H., Fattorini, G., et al. (2013). A1R–A2AR heteromers coupled to Gs and Gi/0 proteins modulate GABA transport into astrocytes. Purinergic Signal. 9, 433–449. doi: 10.1007/s11302-013-9364-5
Dani, J. W., Chernjavsky, A., and Smith, S. J. (1992). Neuronal activity triggers calcium waves in hippocampal astrocyte networks. Neuron 8, 429–440. doi: 10.1016/0896-6273(92)90271-E
Devaraju, P., Sun, M.-Y., Myers, T. L., Lauderdale, K., and Fiacco, T. A. (2013). Astrocytic group I mGluR-dependent potentiation of astrocytic glutamate and potassium uptake. J. Neurophysiol. 109, 2404–2414. doi: 10.1152/jn.00517.2012
Di Castro, M. A., Chuquet, J., Liaudet, N., Bhaukaurally, K., Santello, M., Bouvier, D., et al. (2011). Local Ca2+ detection and modulation of synaptic release by astrocytes. Nat. Neurosci. 14:10. doi: 10.1038/nn.2929
Dittman, A. H., Weber, J. P., Hinds, T. R., Choi, E. J., Migeon, J. C., Nathanson, N. M., et al. (1994). A novel mechanism for coupling of m4 muscarinic acetylcholine receptors to calmodulin-sensitive adenylyl cyclases: Crossover from G protein-coupled inhibition to stimulation. Biochemistry 33, 943–951. doi: 10.1021/bi00170a013
Domercq, M., Brambilla, L., Pilati, E., Marchaland, J., Volterra, A., and Bezzi, P. (2006). P2Y1 receptor-evoked glutamate exocytosis from astrocytes: Control by tumor necrosis factor-α and prostaglandins*. J. Biol. Chem. 281, 30684–30696. doi: 10.1074/jbc.M606429200
Dong, X., Han, S., Zylka, M. J., Simon, M. I., and Anderson, D. J. (2001). A diverse family of GPCRs expressed in specific subsets of nociceptive sensory neurons. Cell 106, 619–632. doi: 10.1016/s0092-8674(01)00483-4
Durkee, C. A., and Araque, A. (2019). Diversity and specificity of astrocyte–neuron communication. Neuroscience 396, 73–78. doi: 10.1016/j.neuroscience.2018.11.010
Durkee, C. A., Covelo, A., Lines, J., Kofuji, P., Aguilar, J., and Araque, A. (2019). Gi/o protein-coupled receptors inhibit neurons but activate astrocytes and stimulate gliotransmission. Glia 67, 1076–1093. doi: 10.1002/glia.23589
Dyer-Reaves, K., Goodman, A. M., Nelson, A. R., and McMahon, L. L. (2019). Alpha1-adrenergic receptor mediated long-term depression at CA3-CA1 synapses can be induced via accumulation of endogenous norepinephrine and is preserved following noradrenergic denervation. Front. Synaptic Neurosci. 11:27. doi: 10.3389/fnsyn.2019.00027
Falcone, C. (2022). Evolution of astrocytes: From invertebrates to vertebrates. Front. Cell Dev. Biol. 10:931311. doi: 10.3389/fcell.2022.931311
Farhy-Tselnicker, I., and Allen, N. J. (2018). Astrocytes, neurons, synapses: A tripartite view on cortical circuit development. Neural Dev. 13:7. doi: 10.1186/s13064-018-0104-y
Farrell, M. S., Pei, Y., Wan, Y., Yadav, P. N., Daigle, T. L., Urban, D. J., et al. (2013). A Gαs DREADD mouse for selective modulation of cAMP production in striatopallidal neurons. Neuropsychopharmacology 38, 854–862. doi: 10.1038/npp.2012.251
Fellin, T., Pascual, O., Gobbo, S., Pozzan, T., Haydon, P. G., and Carmignoto, G. (2004). Neuronal synchrony mediated by astrocytic glutamate through activation of extrasynaptic NMDA receptors. Neuron 43, 729–743. doi: 10.1016/j.neuron.2004.08.011
Fiacco, T. A., Agulhon, C., Taves, S. R., Petravicz, J., Casper, K. B., Dong, X., et al. (2007). Selective stimulation of astrocyte calcium in situ does not affect neuronal excitatory synaptic activity. Neuron 54, 611–626. doi: 10.1016/j.neuron.2007.04.032
Fiacco, T. A., and McCarthy, K. D. (2004). Intracellular astrocyte calcium waves in situ increase the frequency of spontaneous AMPA receptor currents in CA1 pyramidal neurons. J. Neurosci. 24, 722–732. doi: 10.1523/JNEUROSCI.2859-03.2004
Fiacco, T. A., and McCarthy, K. D. (2018). Multiple lines of evidence indicate that gliotransmission does not occur under physiological conditions. J. Neurosci. 38, 3–13. doi: 10.1523/JNEUROSCI.0016-17.2017
Gao, V., Suzuki, A., Magistretti, P. J., Lengacher, S., Pollonini, G., Steinman, M. Q., et al. (2016). Astrocytic β2-adrenergic receptors mediate hippocampal long-term memory consolidation. Proc. Natl. Acad. Sci. U.S.A. 113, 8526–8531. doi: 10.1073/pnas.1605063113
Ghézali, G., Dallérac, G., and Rouach, N. (2016). Perisynaptic astroglial processes: Dynamic processors of neuronal information. Brain Struct. Funct. 221, 2427–2442. doi: 10.1007/s00429-015-1070-3
Gómez-Gonzalo, M., Navarrete, M., Perea, G., Covelo, A., Martín-Fernández, M., Shigemoto, R., et al. (2015). Endocannabinoids induce lateral long-term potentiation of transmitter release by stimulation of gliotransmission. Cereb. Cortex 25, 3699–3712. doi: 10.1093/cercor/bhu231
Guerra-Gomes, S., Sousa, N., Pinto, L., and Oliveira, J. F. (2018). Functional roles of astrocyte calcium elevations: From synapses to behavior. Front. Cell. Neurosci. 11:427. doi: 10.3389/fncel.2017.00427
Guettier, J.-M., Gautam, D., Scarselli, M., de Azua, I. R., Li, J. H., Rosemond, E., et al. (2009). A chemical-genetic approach to study G protein regulation of β cell function in vivo. Proc. Natl. Acad. Sci. U.S.A. 106, 19197–19202. doi: 10.1073/pnas.0906593106
Gutiérrez-Rodríguez, A., Bonilla-Del Río, I., Puente, N., Gómez-Urquijo, S. M., Fontaine, C. J., Egaña-Huguet, J., et al. (2018). Localization of the cannabinoid type-1 receptor in subcellular astrocyte compartments of mutant mouse hippocampus. Glia 66, 1417–1431. doi: 10.1002/glia.23314
Halassa, M. M., Fellin, T., Takano, H., Dong, J.-H., and Haydon, P. G. (2007). Synaptic islands defined by the territory of a single astrocyte. J. Neurosci. 27, 6473–6477. doi: 10.1523/JNEUROSCI.1419-07.2007
Hamilton, N. B., and Attwell, D. (2010). Do astrocytes really exocytose neurotransmitters? Nat. Rev. Neurosci. 11:4. doi: 10.1038/nrn2803
Han, J., Kesner, P., Metna-Laurent, M., Duan, T., Xu, L., Georges, F., et al. (2012). Acute cannabinoids impair working memory through astroglial CB1 receptor modulation of hippocampal LTD. Cell 148, 1039–1050. doi: 10.1016/j.cell.2012.01.037
Haustein, M. D., Kracun, S., Lu, X.-H., Shih, T., Jackson-Weaver, O., Tong, X., et al. (2014). Conditions and constraints for astrocyte calcium signaling in the hippocampal mossy fiber pathway. Neuron 82, 413–429. doi: 10.1016/j.neuron.2014.02.041
Henneberger, C., Bard, L., Panatier, A., Reynolds, J. P., Kopach, O., Medvedev, N. I., et al. (2020). LTP induction boosts glutamate spillover by driving withdrawal of perisynaptic astroglia. Neuron 108, 919–936.e11. doi: 10.1016/j.neuron.2020.08.030
Ilyaskina, O. S., Lemoine, H., and Bünemann, M. (2018). Lifetime of muscarinic receptor–G-protein complexes determines coupling efficiency and G-protein subtype selectivity. Proc. Natl. Acad. Sci. U.S.A. 115, 5016–5021. doi: 10.1073/pnas.1715751115
Jin, L. E., Wang, M., Galvin, V. C., Lightbourne, T. C., Conn, P. J., Arnsten, A. F. T., et al. (2018). mGluR2 versus mGluR3 metabotropic glutamate receptors in primate dorsolateral prefrontal cortex: Postsynaptic mGluR3 Strengthen working memory networks. Cereb. Cortex 28, 974–987. doi: 10.1093/cercor/bhx005
Jones, M. E., Paniccia, J. E., Lebonville, C. L., Reissner, K. J., and Lysle, D. T. (2018). Chemogenetic manipulation of dorsal hippocampal astrocytes protects against the development of stress-enhanced fear learning. Neuroscience 388, 45–56. doi: 10.1016/j.neuroscience.2018.07.015
Kater, M. S. J., Badia-Soteras, A., van Weering, J. R. T., Smit, A. B., and Verheijen, M. H. G. (2023). Electron microscopy analysis of astrocyte-synapse interactions shows altered dynamics in an Alzheimer’s disease mouse model. Front. Cell. Neurosci. 17:1085690. doi: 10.3389/fncel.2023.1085690
Kim, J.-H., Rahman, M. H., Lee, W. H., and Suk, K. (2021). Chemogenetic stimulation of the Gi pathway in astrocytes suppresses neuroinflammation. Pharmacol. Res. Perspect. 9:e00822. doi: 10.1002/prp2.822
King, C. M., Bohmbach, K., Minge, D., Delekate, A., Zheng, K., Reynolds, J., et al. (2020). Local resting Ca2+ controls the scale of astroglial Ca2+ signals. Cell Rep. 30, 3466–3477.e4. doi: 10.1016/j.celrep.2020.02.043
Kofuji, P., and Araque, A. (2021). G-protein-coupled receptors in astrocyte–neuron communication. Neuroscience 456, 71–84. doi: 10.1016/j.neuroscience.2020.03.025
Kol, A., Adamsky, A., Groysman, M., Kreisel, T., London, M., and Goshen, I. (2020). Astrocytes contribute to remote memory formation by modulating hippocampal–cortical communication during learning. Nat. Neurosci. 23:10. doi: 10.1038/s41593-020-0679-6
Kol, A., and Goshen, I. (2021). The memory orchestra: The role of astrocytes and oligodendrocytes in parallel to neurons. Curr. Opin. Neurobiol. 67, 131–137. doi: 10.1016/j.conb.2020.10.022
Lauckner, J. E., Hille, B., and Mackie, K. (2005). The cannabinoid agonist WIN55,212-2 increases intracellular calcium via CB1 receptor coupling to Gq/11 G proteins. Proc. Natl. Acad. Sci. U.S.A. 102, 19144–19149. doi: 10.1073/pnas.0509588102
Lavialle, M., Aumann, G., Anlauf, E., Pröls, F., Arpin, M., and Derouiche, A. (2011). Structural plasticity of perisynaptic astrocyte processes involves ezrin and metabotropic glutamate receptors. Proc. Natl. Acad. Sci. U.S.A. 108, 12915–12919. doi: 10.1073/pnas.1100957108
Lawal, O., Ulloa Severino, F. P., and Eroglu, C. (2022). The role of astrocyte structural plasticity in regulating neural circuit function and behavior. Glia 70, 1467–1483. doi: 10.1002/glia.24191
Lei, Z., Xie, L., Li, C. H., Lam, Y. Y., Ramkrishnan, A. S., Fu, Z., et al. (2022). Chemogenetic activation of astrocytes in the basolateral amygdala contributes to fear memory formation by modulating the amygdala–prefrontal cortex communication. Int. J. Mol. Sci. 23:6092. doi: 10.3390/ijms23116092
Lia, A., Henriques, V. J., Zonta, M., Chiavegato, A., Carmignoto, G., Gómez-Gonzalo, M., et al. (2021). Calcium signals in astrocyte microdomains, a decade of great advances. Front. Cell. Neurosci. 15:673433. doi: 10.3389/fncel.2021.673433
Liu, C., Yang, T.-Q., Zhou, Y.-D., and Shen, Y. (2022). Reduced astrocytic mGluR5 in the hippocampus is associated with stress-induced depressive-like behaviors in mice. Neurosci. Lett. 784:136766. doi: 10.1016/j.neulet.2022.136766
Liu, J.-H., Zhang, M., Wang, Q., Wu, D.-Y., Jie, W., Hu, N.-Y., et al. (2022). Distinct roles of astroglia and neurons in synaptic plasticity and memory. Mol. Psychiatry 27, 873–885. doi: 10.1038/s41380-021-01332-6
Liu, Q.-S., Xu, Q., Kang, J., and Nedergaard, M. (2004). Astrocyte activation of presynaptic metabotropic glutamate receptors modulates hippocampal inhibitory synaptic transmission. Neuron Glia Biol. 1, 307–316. doi: 10.1017/S1740925X05000190
Lopes, C. R., Cunha, R. A., and Agostinho, P. (2021). Astrocytes and adenosine A2A receptors: Active players in Alzheimer’s disease. Front. Neurosci. 15:666710. doi: 10.3389/fnins.2021.666710
Lyon, K. A., and Allen, N. J. (2022). From synapses to circuits, astrocytes regulate behavior. Front. Neural Circuits 15:786293. doi: 10.3389/fncir.2021.786293
Mahmoud, S., Gharagozloo, M., Simard, C., and Gris, D. (2019). Astrocytes maintain glutamate homeostasis in the CNS by controlling the balance between glutamate uptake and release. Cells 8:184. doi: 10.3390/cells8020184
Marsicano, G., Wotjak, C. T., Azad, S. C., Bisogno, T., Rammes, G., Cascio, M. G., et al. (2002). The endogenous cannabinoid system controls extinction of aversive memories. Nature 418, 530–534. doi: 10.1038/nature00839
Matias, I., Morgado, J., and Gomes, F. C. A. (2019). Astrocyte heterogeneity: Impact to brain aging and disease. Front. Aging Neurosci. 11:59. doi: 10.3389/fnagi.2019.00059
McNeill, J., Rudyk, C., Hildebrand, M. E., and Salmaso, N. (2021). Ion channels and electrophysiological properties of astrocytes: Implications for emergent stimulation technologies. Front. Cell. Neurosci. 15:644126. doi: 10.3389/fncel.2021.644126
Min, R., and Nevian, T. (2012). Astrocyte signaling controls spike timing–dependent depression at neocortical synapses. Nature Neurosci. 15:5. doi: 10.1038/nn.3075
Murphy, M. G., Moak, C. M., Byczko, Z., and MacDonald, W. F. (1991). Adenosine-dependent regulation of cyclic AMP accumulation in primary cultures of rat astrocytes and neurons. J. Neurosci. Res. 30, 631–640. doi: 10.1002/jnr.490300406
Nagai, J., Bellafard, A., Qu, Z., Yu, X., Ollivier, M., Gangwani, M. R., et al. (2021). Specific and behaviorally consequential astrocyte Gq GPCR signaling attenuation in vivo with iβARK. Neuron 109, 2256–2274.e9. doi: 10.1016/j.neuron.2021.05.023
Nagai, J., Rajbhandari, A. K., Gangwani, M. R., Hachisuka, A., Coppola, G., Masmanidis, S. C., et al. (2019). Hyperactivity with disrupted attention by activation of an astrocyte synaptogenic cue. Cell 177, 1280–1292.e20. doi: 10.1016/j.cell.2019.03.019
Nam, M.-H., Han, K.-S., Lee, J., Won, W., Koh, W., Bae, J. Y., et al. (2019). Activation of astrocytic μ-opioid receptor causes conditioned place preference. Cell Reports 28, 1154–1166.e5. doi: 10.1016/j.celrep.2019.06.071
Navarrete, M., and Araque, A. (2008). Endocannabinoids mediate neuron-astrocyte communication. Neuron 57, 883–893. doi: 10.1016/j.neuron.2008.01.029
Navarrete, M., and Araque, A. (2010). Endocannabinoids potentiate synaptic transmission through stimulation of astrocytes. Neuron 68, 113–126. doi: 10.1016/j.neuron.2010.08.043
Navarrete, M., Díez, A., and Araque, A. (2014). Astrocytes in endocannabinoid signalling. Philos. Trans. R. Soc. B Biol. Sci. 369:20130599. doi: 10.1098/rstb.2013.0599
Nimmerjahn, A., and Bergles, D. E. (2015). Large-scale recording of astrocyte activity. Curr. Opin. Neurobiol. 32, 95–106. doi: 10.1016/j.conb.2015.01.015
Oe, Y., Wang, X., Patriarchi, T., Konno, A., Ozawa, K., Yahagi, K., et al. (2020). Distinct temporal integration of noradrenaline signaling by astrocytic second messengers during vigilance. Nat. Commun. 11:1. doi: 10.1038/s41467-020-14378-x
Okashah, N., Wan, Q., Ghosh, S., Sandhu, M., Inoue, A., Vaidehi, N., et al. (2019). Variable G protein determinants of GPCR coupling selectivity. Proc. Natl. Acad. Sci. U.S.A. 116, 12054–12059. doi: 10.1073/pnas.1905993116
Orr, A. G., Hsiao, E. C., Wang, M. M., Ho, K., Kim, D. H., Wang, X., et al. (2015). Astrocytic adenosine receptor A2A and Gs-coupled signaling regulate memory. Nat. Neurosci. 18, 423–434. doi: 10.1038/nn.3930
Papouin, T., and Oliet, S. H. R. (2014). Organization, control and function of extrasynaptic NMDA receptors. Philos. Trans. R. Soc. B Biol. Sci. 369:20130601. doi: 10.1098/rstb.2013.0601
Papouin, T., Dunphy, J., Tolman, M., Foley, J. C., and Haydon, P. G. (2017). Astrocytic control of synaptic function. Philos. Trans. R. Soc. Lond. B Biol. Sci. 372:20160154. doi: 10.1098/rstb.2016.0154
Park, K., and Lee, S. J. (2020). Deciphering the star codings: Astrocyte manipulation alters mouse behavior. Exp. Mol. Med. 52:7. doi: 10.1038/s12276-020-0468-z
Patte, C., Gandolfo, P., Leprince, J., Thoumas, J. L., Fontaine, M., Vaudry, H., et al. (1999). GABA inhibits endozepine release from cultured rat astrocytes. Glia 25, 404–411.
Paul, V., and Ekambaram, P. (2011). Involvement of nitric oxide in learning & memory processes. Indian J. Med. Res. 133, 471–478.
Perea, G., and Araque, A. (2005). Glial calcium signaling and neuron–glia communication. Cell Calc. 38, 375–382. doi: 10.1016/j.ceca.2005.06.015
Perea, G., Gómez, R., Mederos, S., Covelo, A., Ballesteros, J. J., Schlosser, L., et al. (2016). Activity-dependent switch of GABAergic inhibition into glutamatergic excitation in astrocyte-neuron networks. eLife 5:e20362. doi: 10.7554/eLife.20362
Perez-Alvarez, A., Navarrete, M., Covelo, A., Martin, E. D., and Araque, A. (2014). Structural and functional plasticity of astrocyte processes and dendritic spine interactions. J. Neurosci. 34, 12738–12744. doi: 10.1523/JNEUROSCI.2401-14.2014
Petravicz, J., Boyt, K. M., and McCarthy, K. D. (2014). Astrocyte IP3R2-dependent Ca2+ signaling is not a major modulator of neuronal pathways governing behavior. Front. Behav. Neurosci. 8:384. doi: 10.3389/fnbeh.2014.00384
Petravicz, J., Fiacco, T. A., and McCarthy, K. D. (2008). Loss of IP3 receptor-dependent Ca2+ increases in hippocampal astrocytes does not affect baseline CA1 pyramidal neuron synaptic activity. J. Neurosci. 28, 4967–4973. doi: 10.1523/JNEUROSCI.5572-07.2008
Pfeil, E. M., Brands, J., Merten, N., Vögtle, T., Vescovo, M., Rick, U., et al. (2020). Heterotrimeric G protein subunit Gαq is a master switch for gβγ-mediated calcium mobilization by Gi-coupled GPCRs. Mol. Cell 80, 940–954.e6. doi: 10.1016/j.molcel.2020.10.027
Pigott, B. M., and Garthwaite, J. (2016). Nitric oxide is required for L-Type Ca2+ channel-dependent long-term potentiation in the hippocampus. Front. Synaptic Neurosci. 8:17. doi: 10.3389/fnsyn.2016.00017
Rae, M. G., and Irving, A. J. (2004). Both mGluR1 and mGluR5 mediate Ca2+ release and inward currents in hippocampal CA1 pyramidal neurons. Neuropharmacology 46, 1057–1069. doi: 10.1016/j.neuropharm.2004.02.002
Rajagopal, S., and Shenoy, S. K. (2018). GPCR desensitization: Acute and prolonged phases. Cell. Signal. 41, 9–16. doi: 10.1016/j.cellsig.2017.01.024
Reichenbach, N., Delekate, A., Breithausen, B., Keppler, K., Poll, S., Schulte, T., et al. (2018). P2Y1 receptor blockade normalizes network dysfunction and cognition in an Alzheimer’s disease model. J. Exp. Med. 215, 1649–1663. doi: 10.1084/jem.20171487
Rhee, S. G., and Bae, Y. S. (1997). Regulation of phosphoinositide-specific phospholipase C isozymes *. J. Biol. Chem. 272, 15045–15048. doi: 10.1074/jbc.272.24.15045
Robin, L. M., Oliveira da Cruz, J. F., Langlais, V. C., Martin-Fernandez, M., Metna-Laurent, M., Busquets-Garcia, A., et al. (2018). Astroglial CB1 Receptors Determine Synaptic D-Serine Availability to Enable Recognition Memory. Neuron 98, 935–944.e5. doi: 10.1016/j.neuron.2018.04.034
Rosenbaum, D. M., Rasmussen, S. G. F., and Kobilka, B. K. (2009). The structure and function of G-protein-coupled receptors. Nature 459, 356–363. doi: 10.1038/nature08144
Roth, B. L. (2016). DREADDs for neuroscientists. Neuron 89, 683–694. doi: 10.1016/j.neuron.2016.01.040
Roux, B. T., and Cottrell, G. S. (2014). G protein-coupled receptors: What a difference a ‘partner’ makes. Int. J. Mol. Sci. 15, 1112–1142. doi: 10.3390/ijms15011112
Rubenstein, R. C., Linder, M. E., and Ross, E. M. (1991). Selectivity of the .beta.-adrenergic receptor among Gs, Gi’s, and go: Assay using recombinant .alpha. Subunits in reconstituted phospholipid vesicles. Biochemistry 30, 10769–10777. doi: 10.1021/bi00108a023
Rungta, R. L., Bernier, L.-P., Dissing-Olesen, L., Groten, C. J., LeDue, J. M., Ko, R., et al. (2016). Ca2+ transients in astrocyte fine processes occur via Ca2+ influx in the adult mouse hippocampus. Glia 64, 2093–2103. doi: 10.1002/glia.23042
Salm, A. K., and McCarthy, K. D. (1992). The evidence for astrocytes as a target for central noradrenergic activity: Expression of adrenergic receptors. Brain Res. Bull. 29, 265–275. doi: 10.1016/0361-9230(92)90056-4
Sandhu, M., Touma, A. M., Dysthe, M., Sadler, F., Sivaramakrishnan, S., and Vaidehi, N. (2019). Conformational plasticity of the intracellular cavity of GPCR-G-protein complexes leads to G-protein promiscuity and selectivity. Proc. Natl. Acad. Sci. U.S.A. 116, 11956–11965. doi: 10.1073/pnas.1820944116
Santello, M., Bezzi, P., and Volterra, A. (2011). TNFα controls glutamatergic gliotransmission in the hippocampal dentate gyrus. Neuron 69, 988–1001. doi: 10.1016/j.neuron.2011.02.003
Santello, M., Toni, N., and Volterra, A. (2019). Astrocyte function from information processing to cognition and cognitive impairment. Nat. Neurosci. 22, 154–166. doi: 10.1038/s41593-018-0325-8
Savtchouk, I., and Volterra, A. (2018). Gliotransmission: Beyond black-and-white. J. Neurosci. 38, 14–25. doi: 10.1523/JNEUROSCI.0017-17.2017
Shen, W., Chen, S., Liu, Y., Han, P., Ma, T., and Zeng, L.-H. (2021). Chemogenetic manipulation of astrocytic activity: Is it possible to reveal the roles of astrocytes? Biochem. Pharmacol. 186:114457. doi: 10.1016/j.bcp.2021.114457
Sherwood, M. W., Arizono, M., Hisatsune, C., Bannai, H., Ebisui, E., Sherwood, J. L., et al. (2017). Astrocytic IP 3 Rs: Contribution to Ca 2+ signalling and hippocampal LTP: Astrocytic IP 3 Rs: Ca 2+ signalling and LTP. Glia 65, 502–513. doi: 10.1002/glia.23107
Sherwood, M. W., Arizono, M., Panatier, A., Mikoshiba, K., and Oliet, S. H. R. (2021). Astrocytic IP3Rs: Beyond IP3R2. Front. Cell. Neurosci. 15:695817. doi: 10.3389/fncel.2021.695817
Shigetomi, E., Bowser, D. N., Sofroniew, M. V., and Khakh, B. S. (2008). Two forms of astrocyte calcium excitability have distinct effects on NMDA receptor-mediated slow inward currents in pyramidal neurons. J. Neurosci. 28, 6659–6663. doi: 10.1523/JNEUROSCI.1717-08.2008
Shigetomi, E., Bushong, E. A., Haustein, M. D., Tong, X., Jackson-Weaver, O., Kracun, S., et al. (2013). Imaging calcium microdomains within entire astrocyte territories and endfeet with GCaMPs expressed using adeno-associated viruses. J. Gen. Physiol. 141, 633–647. doi: 10.1085/jgp.201210949
Shigetomi, E., Hirayama, Y. J., Ikenaka, K., Tanaka, K. F., and Koizumi, S. (2018). Role of purinergic receptor P2Y1 in spatiotemporal Ca2+ dynamics in astrocytes. J. Neurosci. 38, 1383–1395. doi: 10.1523/JNEUROSCI.2625-17.2017
Shigetomi, E., Saito, K., Sano, F., and Koizumi, S. (2019). Aberrant calcium signals in reactive astrocytes: A key process in neurological disorders. Int. J. Mol. Sci. 20:996. doi: 10.3390/ijms20040996
Sobolczyk, M., and Boczek, T. (2022). Astrocytic calcium and cAMP in neurodegenerative diseases. Front. Cell. Neurosci. 16:889939. doi: 10.3389/fncel.2022.889939
Srinivasan, R., Huang, B. S., Venugopal, S., Johnston, A. D., Chai, H., Zeng, H., et al. (2015). Ca2+ signaling in astrocytes from Ip3r2-/- mice in brain slices and during startle responses in vivo. Nat. Neurosci. 18:5. doi: 10.1038/nn.4001
Sun, W., McConnell, E., Pare, J.-F., Xu, Q., Chen, M., Peng, W., et al. (2013). Glutamate-dependent neuroglial calcium signaling differs between young and adult brain. Science 339, 197–200. doi: 10.1126/science.1226740
Syrovatkina, V., Alegre, K. O., Dey, R., and Huang, X.-Y. (2016). Regulation signaling and physiological functions of G-proteins. J. Mol. Biol. 428, 3850–3868. doi: 10.1016/j.jmb.2016.08.002
Tanaka, M., Shih, P.-Y., Gomi, H., Yoshida, T., Nakai, J., Ando, R., et al. (2013). Astrocytic Ca2+ signals are required for the functional integrity of tripartite synapses. Mol. Brain 6:6. doi: 10.1186/1756-6606-6-6
Tang, W. J., and Gilman, A. G. (1991). Type-specific regulation of adenylyl cyclase by G protein beta gamma subunits. Science 254, 1500–1503. doi: 10.1126/science.1962211
Tang, W., Szokol, K., Jensen, V., Enger, R., Trivedi, C. A., Hvalby, Ø, et al. (2015). Stimulation-evoked Ca2+ signals in astrocytic processes at hippocampal CA3-CA1 synapses of adult mice are modulated by glutamate and ATP. J. Neurosci. 35, 3016–3021. doi: 10.1523/JNEUROSCI.3319-14.2015
Vaidyanathan, T. V., Collard, M., Yokoyama, S., Reitman, M. E., and Poskanzer, K. E. (2021). Cortical astrocytes independently regulate sleep depth and duration via separate GPCR pathways. eLife 10:e63329. doi: 10.7554/eLife.63329
Van Den Herrewegen, Y., Sanderson, T. M., Sahu, S., De Bundel, D., Bortolotto, Z. A., and Smolders, I. (2021). Side-by-side comparison of the effects of Gq- and Gi-DREADD-mediated astrocyte modulation on intracellular calcium dynamics and synaptic plasticity in the hippocampal CA1. Mol. Brain 14:144. doi: 10.1186/s13041-021-00856-w
Vardjan, N., Kreft, M., and Zorec, R. (2014). Dynamics of β-adrenergic/cAMP signaling and morphological changes in cultured astrocytes. Glia 62, 566–579. doi: 10.1002/glia.22626
Verkhratsky, A. (2009). “Neurotransmitter receptors in astrocytes,” in Astrocytes in (Patho)Physiology of the Nervous System, eds P. G. Haydon and V. Parpura (New York, NY: Springer US), 49–67. doi: 10.1007/978-0-387-79492-1_3
Verkhratsky, A., Parpura, V., Vardjan, N., and Zorec, R. (2019). Physiology of astroglia. Adv. Exp. Med. Biol. 1175, 45–91. doi: 10.1007/978-981-13-9913-8_3
Volterra, A., and Meldolesi, J. (2005). Astrocytes, from brain glue to communication elements: The revolution continues. Nat. Rev. Neurosci. 6, 626–640. doi: 10.1038/nrn1722
Wahis, J., and Holt, M. G. (2021). Astrocytes, noradrenaline, α1-adrenoreceptors, and neuromodulation: Evidence and unanswered questions. Front. Cell. Neurosci. 15:645691. doi: 10.3389/fncel.2021.645691
Walker, A. G., Sheffler, D. J., Lewis, A. S., Dickerson, J. W., Foster, D. J., Senter, R. K., et al. (2017). Co-activation of metabotropic glutamate receptor 3 and beta-adrenergic receptors modulates cyclic-AMP and long-term potentiation, and disrupts memory reconsolidation. Neuropsychopharmacology 42, 2553–2566. doi: 10.1038/npp.2017.136
Weis, W. I., and Kobilka, B. K. (2018). The molecular basis of G protein–coupled receptor activation. Annual Rev. Biochem. 87, 897–919. doi: 10.1146/annurev-biochem-060614-033910
Wettschureck, N., and Offermanns, S. (2005). Mammalian G proteins and their cell type specific functions. Physiol. Rev. 85, 1159–1204. doi: 10.1152/physrev.00003.2005
Witkowski, G., Rola, R., and Szulczyk, P. (2012). Effect of cyclic adenosine monophosphate on the G protein-dependent inward rectifier K(+)-like channel current in medial prefrontal cortex pyramidal neurons. J. Physiol. Pharmacol. 63, 457–462.
Xie, A. X., Petravicz, J., and McCarthy, K. D. (2015). Molecular approaches for manipulating astrocytic signaling in vivo. Front. Cell. Neurosci. 9:144. doi: 10.3389/fncel.2015.00144
Xie, A. X., Sun, M.-Y., Murphy, T., Lauderdale, K., Tiglao, E., and Fiacco, T. A. (2012). Bidirectional scaling of astrocytic metabotropic glutamate receptor signaling following long-term changes in neuronal firing rates. PLoS One 7:e49637. doi: 10.1371/journal.pone.0049637
Zeng, W., Mak, D.-O. D., Li, Q., Shin, D. M., Foskett, J. K., and Muallem, S. (2003). A new mode of Ca2+ signaling by G protein-coupled receptors: Gating of IP3 receptor Ca2+ release channels by Gβγ. Curr. Biol. 13, 872–876. doi: 10.1016/S0960-9822(03)00330-0
Zheng, K., Bard, L., Reynolds, J. P., King, C., Jensen, T. P., Gourine, A. V., et al. (2015). Time-resolved imaging reveals heterogeneous landscapes of nanomolar Ca2+ in neurons and astroglia. Neuron 88, 277–288. doi: 10.1016/j.neuron.2015.09.043
Zhou, Z., Ikegaya, Y., and Koyama, R. (2019). The astrocytic cAMP pathway in health and disease. Int. J. Mol. Sci. 20:779. doi: 10.3390/ijms20030779
Keywords: glia, tripartite synapse, signal transduction, signaling pathways, behavior
Citation: Lee SH, Mak A and Verheijen MHG (2023) Comparative assessment of the effects of DREADDs and endogenously expressed GPCRs in hippocampal astrocytes on synaptic activity and memory. Front. Cell. Neurosci. 17:1159756. doi: 10.3389/fncel.2023.1159756
Received: 06 February 2023; Accepted: 13 March 2023;
Published: 27 March 2023.
Edited by:
Edna Suarez Pozos, Virginia Commonwealth University, United StatesReviewed by:
Marta Navarrete, Spanish National Research Council (CSIC), SpainMarcello Melone, Marche Polytechnic University, Italy
Copyright © 2023 Lee, Mak and Verheijen. This is an open-access article distributed under the terms of the Creative Commons Attribution License (CC BY). The use, distribution or reproduction in other forums is permitted, provided the original author(s) and the copyright owner(s) are credited and that the original publication in this journal is cited, in accordance with accepted academic practice. No use, distribution or reproduction is permitted which does not comply with these terms.
*Correspondence: Mark Verheijen, bWFyay52ZXJoZWlqZW5AdnUubmw=