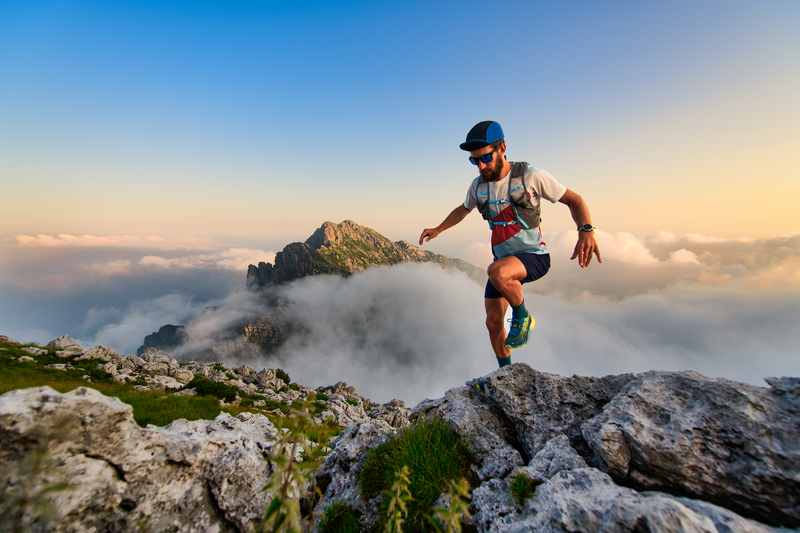
95% of researchers rate our articles as excellent or good
Learn more about the work of our research integrity team to safeguard the quality of each article we publish.
Find out more
ORIGINAL RESEARCH article
Front. Cell. Neurosci. , 30 March 2023
Sec. Cellular Neuropathology
Volume 17 - 2023 | https://doi.org/10.3389/fncel.2023.1154772
This article is part of the Research Topic Rising Stars in Cellular Neuropathology 2022 View all 8 articles
Background: Creatine supplementation during pregnancy is a promising prophylactic treatment for perinatal hypoxic brain injury. Previously, in near-term sheep we have shown that fetal creatine supplementation reduces cerebral metabolic and oxidative stress induced by acute global hypoxia. This study investigated the effects of acute hypoxia with or without fetal creatine supplementation on neuropathology in multiple brain regions.
Methods: Near-term fetal sheep were administered continuous intravenous infusion of either creatine (6 mg kg–1 h–1) or isovolumetric saline from 122 to 134 days gestational age (dGA; term is approx. 145 dGA). At 131 dGA, global hypoxia was induced by a 10 min umbilical cord occlusion (UCO). Fetuses were then recovered for 72 h at which time (134 dGA) cerebral tissue was collected for either RT-qPCR or immunohistochemistry analyses.
Results: UCO resulted in mild injury to the cortical gray matter, thalamus and hippocampus, with increased cell death and astrogliosis and downregulation of genes involved in regulating injury responses, vasculature development and mitochondrial integrity. Creatine supplementation reduced astrogliosis within the corpus callosum but did not ameliorate any other gene expression or histopathological changes induced by hypoxia. Of importance, effects of creatine supplementation on gene expression irrespective of hypoxia, including increased expression of anti-apoptotic (BCL-2) and pro-inflammatory (e.g., MPO, TNFa, IL-6, IL-1β) genes, particularly in the gray matter, hippocampus, and striatum were identified. Creatine treatment also effected oligodendrocyte maturation and myelination in white matter regions.
Conclusion: While supplementation did not rescue mild neuropathology caused by UCO, creatine did result in gene expression changes that may influence in utero cerebral development.
Creatine is an amino acid derivate and its phosphorylated form, phosphocreatine, is a compound with the important role in supporting high-energy metabolism through the spatial and temporal buffering of cellular ATP during periods of high metabolic demand (Wyss and Kaddurah-Daouk, 2000). An increase of creatine by exogenous administration can result in an increase in tissue total creatine content (creatine plus phosphocreatine), allowing for a greater and more prolonged provision of phosphocreatine within cells (Wallimann et al., 2011). This has the effect of limiting cellular energy failure and sustaining redox homeostasis during periods of oxygen deprivation (Andres et al., 2005; Shen and Goldberg, 2012). Accordingly, dietary creatine supplementation is proposed as a prophylactic treatment strategy for pathologies that involve hypoxia-mediated energy failure such as perinatal hypoxic-ischemic encephalopathy (HIE) (Ellery et al., 2016).
The near-term fetal brain is particularly vulnerable to hypoxic-ischemic (HI) injury with even mild HIE events associated with neurodevelopmental deficits and delays (Hayes et al., 2018). A complex interplay between tissue oxygenation, cellular and mitochondrial function, and the generation of neurotoxic cascades leads to increased cell death, neuro-inflammation, oxidative stress (Gunn and Bennet, 2009), and ultimately, the clinical manifestation of HIE (Vannucci, 2000; Perlman, 2007). Targeting one or some of these mechanisms may be beneficial in reducing or even preventing the development of HIE. Of note, near-term HIE often presents as injury to the cerebral cortex, hippocampus, thalamus, and basal ganglia (striatum) (Barkovich et al., 1995). This increased injury susceptibility is attributable to the high metabolic needs of these particular brain regions (Martin et al., 1997; Sie et al., 2000; Okereafor et al., 2008).
Increasing the extracellular and intracellular availability of creatine and thus phosphocreatine to support cerebral metabolism within vulnerable brain regions may thereby attenuate the severity of HIE. Indeed, previous preclinical investigations have documented the neuroprotective effects of creatine following perinatal hypoxic events, having demonstrated reduced cerebral cell death and the size of infarcts, and improved behavioral function (Tran et al., 2021a). We have previously reported in near-term fetal sheep that higher circulating creatine levels were associated with reduced degrees of hypoxemia, reduced efflux of cerebral extracellular reactive oxygen species (ROS), and reduced extracellular lactate and pyruvate following global hypoxia induced by umbilical cord occlusion (UCO) (Tran et al., 2022). Despite indications of improved cerebral metabolism during the phase of secondary energy failure, we found in the same fetal sheep cohort that creatine supplementation did not reduce the dysregulation of mitochondrial respiration within hippocampal and white matter tissue samples, when studied in vitro at 72 h after the UCO insult (Muccini et al., 2022). This suggests that fetal creatine supplementation may be neuroprotective via other mechanisms. Indeed, a range of studies encompassing juvenile through to adult brain analyses suggest creatine has anti-inflammatory and antioxidant effects and can support cerebral vasculature perfusion (Prass et al., 2006; Beal, 2011; Riesberg et al., 2016; Sestili et al., 2016), see review (Muccini et al., 2021). While there is much promise that creatine might reduce the cellular pathology following HI, these neuroprotective outcomes have yet to be demonstrated in the near-term fetal brain.
Using the same cohort of fetal sheep where we previously demonstrated regional creatine loading in the fetal brain following direct intravenous infusion (Tran et al., 2021b,2022; Muccini et al., 2022), the aim of the present study was to identify the neuropathological effects induced by mild UCO within brain regions typically affected by hypoxia. We then assessed if fetal creatine supplementation could ameliorate such hypoxia-induced effects. To do this, we assessed changes in mRNA expression of multiple genes relating to key biological processes and pathological mechanisms involved in HI. We also investigated the effects on the key cell populations within the brain (neurons, oligodendrocytes, microglia, astrocytes, blood vessels) using immunohistochemical markers.
The second aim was to assess the effects of creatine itself on the fetal brain, irrespective of hypoxia. The brain possesses both a capacity to load creatine but also an inherent capacity to synthesis creatine (Braissant et al., 2007; Béard and Braissant, 2010). While it is not yet clear at what point in gestation the human fetus is able to independently synthesize creatine de novo, and in the brain in particular, it seems that the fetal requirements of creatine depend heavily on maternal creatine levels (Bianchi et al., 2000; Dickinson et al., 2014). Interestingly, studies have indicated that maternal creatine supplementation influences the morphological and functional development of neurons in rodent offspring, enhancing overall neuronal excitability and improving long-term potentiation (Sartini et al., 2016, 2019). Other than these studies by Sartini et al. (2016, 2019) there is limited data on the effects of increased creatine levels within the immature brain. Investigating the mechanisms by which creatine supplementation could alter basal biological processes in the fetal brain is important for establishing its safety profile for use in pregnancy and during the perinatal period.
This study utilized post-mortem fetal brain tissue collected from previously published studies detailing the experimental model and physiological outcomes (Tran et al., 2021b,2022). The use of animals was approved by Monash Medical Centre Animal Ethics Committee (MMCA-2017-02) and was conducted in accordance with the National Health and Medical Research Council Code of Practice for the Care and Use of Animals for Scientific Purposes (Eighth Edition).
Briefly, 28 time-mated pregnant Border–Leicester/Merino cross ewes carrying singleton fetuses at 118 days’ gestational age (dGA; term is ∼145–147 dGA) were placed under general anesthesia using 2% isoflurane in oxygen. Under strict sterile conditions, surgery involved exposure of the fetal head and forelimbs via a midline maternal abdominal incision and approximately a 10 cm incision of the uterus. The fetal left brachial artery and vein were catheterized for blood sampling and creatine or saline infusion, respectively. A microdialysis probe was also inserted into the right cerebral hemisphere as detailed elsewhere (Tran et al., 2022). The fetus was then withdrawn further from the uterus and an inflatable silastic vascular occluder (type-OC 16 mm, in vivo Metric, Healdsburg, CA, USA) was placed around the umbilical cord and anchored to the fetal abdomen. The fetus was then returned to the uterus, and all fetal, uterine and maternal incisions were repaired, and catheters exteriorized via a small (1–1.5 cm) incision in the maternal right flank. Ewes were recovered from surgery and placed in individual pens and in the company of other sheep at the Monash Medical Centre animal house holding facility, maintained on a 12 h light/dark cycle (8:00 a.m.–8:00 p.m.) at an ambient temperature of ∼20°C. Ewes were fed twice daily with a lucerne chaff mixture and had access to water ab libitum. The overall wellbeing of each ewe, and food and water intake was monitored daily throughout the entire experiment.
Fetuses were randomly assigned to one of the following four treatment groups: sham UCO with saline (n = 6; SalCon) or creatine infusion (n = 7; CrCon); or UCO for 10 min with saline (n = 8; SalUCO) or creatine infusion (n = 7; CrUCO). All creatine-infused fetuses were given a continuous intravenous infusion of creatine from 121 dGA at 6 mg kg–1.h–1 (18 mg h–1, based on the historical observation of an average fetal weight of ∼3 kg) diluted in saline and delivered at 1.5 ml h–1 and continued until the end of the experiment at 134 dGA when post-mortem was performed. Saline-infused fetuses received an isovolumetric administration of 0.9% NaCl, pH 7.4 for the same duration. Mild global fetal hypoxia was induced transiently at 131 dGA where the umbilical cord was completely occluded (UCO) for 10 min, followed by recovery in utero for 72 h until post-mortem. At post-mortem, the ewe and fetus were humanely killed using an overdose of pentobarbitone (100 mg kg–1; Lethabarb; Virbac, Milperra, NSW, Australia) injected intravenously to the ewe, as previously described (Tran et al., 2021b). The fetal brain was rapidly removed from the skull by cutting the cranial nerves, pituitary stalk, and the cervical spinal cord, and then divided into right and left hemispheres along the midline. Samples of cortical gray matter (GM), white matter (WM; combined sampling from the subcortical and periventricular white matter), hippocampus, striatum (combined sampling from the putamen and caudate nucleus), and thalamus were dissected from the left hemisphere and snap frozen in liquid nitrogen and stored at −80°C until analysis of mRNA expression. The right hemisphere was left intact and fixed by immersion in 4% paraformaldehyde (0.1 M; pH 7.4) for 72 h and then cut coronally into 5 mm-thick rostro-caudal blocks (∼10 blocks per brain) and embedded in paraffin.
Total RNA extraction and cDNA preparation was conducted as previously described (Muccini et al., 2022). Briefly, RNA was extracted from frozen brain tissue (60–80 mg) using an RNA Mini Kit (PureLink RNA Mini Kite, Thermofisher, Melbourne, VIC, Australia) following the manufacturer’s instructions. RNA yield was determined by spectrophotometry (Nanodrop, Analytical Technologies, Biolab, Concord, ON, Canada). cDNA was transcribed from RNA, then pre-amplified at 25 ng μl–1 with a mixture of pooled 20X TaqMan gene expression assay probes (Life Technologies, ThermoFisher, USA), Taqman PreAmp Master Mix (2X; Applied Biosystems; Thermo Fisher Scientific, Lithuania).
Genes involved in cellular regulation, inflammation, metabolism, oxidative stress and vasculature development are listed in Table 1. Expression of these genes was analysed across all of the flash-frozen brain regions. Genes associated with mitochondrial integrity, cell death and metabolic pathways (Table 1) were also assessed in the striatum and thalamus, adding to previous data published on the gray matter, white matter and hippocampus by Muccini et al. (2022). Gene expression was analysed using a Fluidigm Dynamic array Biomark HD system (Fluidigm, USA). Gene expression was determined as relative expression calculated by change in cycle threshold (ΔCt) between Ct of each gene of interest and the geometric average of three endogenous housekeeping genes, RPL32, RPS16, and OAZ1. Levels of mRNA expression relative to geometrical average of house-keeping genes were determined using the 2–ΔΔCT method (Livak and Schmittgen, 2001). Due to technical issues, frozen samples from one animal in each of the SalCon and CrUCO groups could not be analysed; thus, the revised group numbers for RT-qPCR analysis are, SalCon, n = 5; CrUCO, n = 6.
Paraffin-embedded blocks were sectioned coronally at 8 μm thickness using a microtome across the parietal-temporal lobe and tissue sections placed on glass slides. For each animal, a total of two slides, corresponding to section 720 and section 1,120 according to the Michigan State University Sheep Atlas [Cx4 (rostral) and Cx7 (caudal); Supplementary Figure 1] were utilized per immunohistochemical marker. Slides were dewaxed in xylene and rehydrated in decreasing concentrations of ethanol. Slides were then washed in phosphate buffered saline (PBS; pH 7.4) and prepared for individual protocols for histochemical staining.
Details of specific immunohistochemistry protocols are provided in Supplementary Table 1. After antigen retrieval was conducted, slides were rinsed with PBS and blocked for endogenous peroxidases and then blocked for non-specific binding. Slides were then incubated overnight at 4°C in the presence of one of the following primary antibodies: mouse anti-NeuN (1:500; Millipore, Germany; CAT#: MAB377) for mature neurons; rabbit anti-ionized calcium binding adaptor molecule 1 (IBA-1; 1:1000; Wako Chemicals, USA; CAT#: 019-19741) for microglia; rabbit anti-glial fibrillary acidic protein (GFAP; 1:500; Dako, USA; CAT#: Z033401-2) for astrocytes; mouse anti-CNPase (1:500; Sigma-Aldrich, USA; CAT#: C5922) for pre-myelinating and myelinating oligodendrocytes and myelin; rabbit anti-myelin basic protein (MBP; 1:200; Chemicon International, USA; CAT# AB5864) for mature oligodendrocytes and myelin; and rabbit anti-Olig-2 (1:500; Millipore, Germany; CAT#: MABN50) for the entire oligodendrocyte lineage; rabbit anti-sheep serum (1:700; Sigma-Aldrich, USA; CAT#: S4265) for serum protein extravasation to detect blood brain barrier (BBB) permeability. Slides were then washed in PBS and incubated in secondary biotinylated IgG antibody raised in corresponding species (1:200). The slides were washed again and incubated with avidin-biotin complex (ABC Elite kit; 1:1:200 in PBS; Vectastain®, Vector Laboratories, UK) then visualized with 3,3’-diamniobenzidine solution (DAB; 1 tablet in 10 ml dH2O; MP Biomedicals, Australia). In addition, to assess cell death, a “Terminal deoxynucleotidyl transferase dUTP nick end labelling” (TUNEL) assay (ApopTag® Peroxidase in situ Apoptosis Detection Kit, Millipore, USA) was conducted according to manufacturer’s instructions. All slides were then dehydrated and then cover-slipped using DPX (Merck, Germany). IBA-1 and Olig-2 immunohistochemical stains were counterstained with hematoxylin. Negative control sections where the primary antibody was omitted, were conducted for each staining protocol. No positive cellular staining and minimal background staining was observed for all these negative control sections.
All analyses were undertaken by investigators blinded to the treatment groups. Slides were scanned at 20 × magnification using an Aperio Scanscope AT Turbo (Leica Biosystems, Germany). Regions of interest included the cortical grey matter (GM), subcortical white matter (SCWM), periventricular white matter (PVWM), corpus callosum (CC), caudate, putamen, internal and external capsule, thalamic nuclei and hippocampus [dorsal region: cornu ammonis (CA) 1–3 region and dentate gyrus (DG)] (Supplementary Figure 1). Regions of interest were outlined using Aperio Image Scope (Leica Biosystems, Germany). Six to ten fields of interest (FOI; 200 μm × 200 μm) were randomly placed within each brain region of interest but placed at similar locations for each animal; for smaller brain regions, i.e., hippocampus (dorsal region), FOI of 100 μm × 100 μm were used. For all analyses in which the same brain regions were present within both the rostral and caudal sections (GM, SCWM, PVWM, CC), statistical analysis (paired t-test) was undertaken to assess whether there was an effect of section sampling; if no sampling effect was found, analysis parameters were averaged for each animal. Only MBP-positive area coverage analysis within the SCWM revealed a section sampling difference and therefore results are presented separately (i.e., rostral and caudal).
For quantitative assessments of TUNEL-positive cell density, TUNEL-positive cells were manually counted in each FOI and then expressed as cells/mm2; means were calculated for each animal and a mean of means for each experimental group determined. Note that TUNEL data is only reported for the caudate, thalamus, CC and putamen adding to previous data published on the GM, WM and hippocampus (Muccini et al., 2022).
Analysis of sheep serum extravasation from blood vessels into the surrounding parenchyma was used as an indicator of blood brain barrier (BBB) breakdown or compromise. FOI boxes were not used for sheep serum analysis, instead, whole brain regions of interest were outlined on each scanned section. Blood vessels were manually identified as either being intact: positively-stained serum limited to within blood vessel, or compromised: positively-stained serum within the parenchyma surrounding a blood vessel. A vessel with sheep serum extravasation was counted as one vessel regardless of the number of areas of serum leakage around a single connected blood vessel. Positively-stained serum without a visible blood vessel was not counted. The number of vessels exhibiting serum extravasation was counted for each brain region per fetus, and an average calculated for each experimental group.
For assessment of NeuN-positive cell density in the GM, counts were conducted in four bins each roughly equivalent to cortical layer I (Bin 1), layer II and III (Bin 2), layer IV and V (Bin 3), and layer VI (Bin 4). If no differences were found between experimental groups for each bin, cell counts from all bins were summated and expressed as cell density (cells/mm2) within the entire GM sampling for each animal, and then an average calculated for each experimental group.
Changes to microglia and astrocytes that indicate a neuroinflammatory state were assessed using antibodies specific for microglia (IBA-1) and astrocytes (GFAP). IBA-1 and GFAP-immunoreactive cells were manually counted in each FOI and then an average calculated for each animal; the cell density (cells/mm2) for each was then determined. For quantitative assessments of IBA-1 and GFAP-positive area coverage to assess the proportion of the area occupied by IBA-1 and GFAP-positive cell bodies and branching processes, each FOI was exported, and the image processed using ImageJ software (version 2.0.0-rc-69/1.52p, National Institutes of Health). The “area coverage tool” in ImageJ was used to automatically quantify percentage of positive staining in each FOI; an average area coverage of multiple FOI was then calculated for each brain region in each fetus.
White matter development and integrity was assessed using oligodendrocyte lineage and myelination markers, e.g., Olig-2, a pan-oligodendrocyte marker for the entire oligodendrocyte lineage, CNPase for pre-myelinating and myelinating oligodendrocytes and myelin, and MBP for mature myelinating oligodendrocytes and myelin (see Figure 4). For Olig2-positive cell density in the GM, cell counts were conducted in Layer VI. As above, Olig-2- and MBP-positive cells were manually counted in each FOI and the cell density (cells/mm2) determined. For quantitative assessments of CNPase- and MBP-positive area coverage, an average area coverage (%) of multiple FOI was then calculated for each brain region in each fetus.
All statistical analyses and data visualization were conducted using GraphPad Prism (version 8.1.2; GraphPad Software, CA, United States). Data were assessed for normality using the Shapiro-Wilk Test with an alpha of 0.01 due to small sample sizes. RT-qPCR data expressed as relative change (2–ΔΔCT) were log-transformed to maintain normal distribution, and all statistical analysis and conducted on log transformed 2–ΔΔCT data. For all analyses, data sets were assessed for main effects of UCO (PUCO), main effects of creatine treatment (PTREAT) and interactions between the main effects (PINT) by two-way ANOVA. Where a significant interaction was observed, post-hoc analysis was performed using Tukey’s multiple comparison test. RT-qPCR volcano plot data visualizations present the main effect of UCO, main effect of creatine, and post-hoc comparisons of SalUCO vs. CrUCO as fold changes. Data are presented as mean ± SD and P < 0.05 was considered statistically significant and a trend for significance was considered when P < 0.07.
The systemic changes of blood gases, pH, cardiovascular responses, cerebral metabolism and oxidative responses to UCO and creatine supplementation have been reported elsewhere (Tran et al., 2022). Of note, all physiological and metabolic parameters had returned to pre-UCO levels by the time of the post-mortem, 72 h post-UCO.
The effect of acute global hypoxia and creatine supplementation on genes of interest in the cortical GM, WM, hippocampus, striatum, and thalamus of the fetal sheep brain are summarized as volcano plots (Figures 1A–C) and a heat map (Figure 1D). Values are reported in Supplementary Table 2.
Figure 1. Volcano plot visualizations of (A) umbilical cord occlusion (UCO) and (B) creatine main effects, and (C) SalUCO vs. CrUCO post-hoc comparisons. Volcano plots show fold change [log2(fold change); i.e., log2(fold change = 2) = 1; x-axis] and all gene expression changes [−log2(adjusted P-value); y axis] in all brain regions: GM (●), WM (■), hippocampus (▲), striatum (◆) and thalamus (▼). Gray dashed line represents a P-value of 0.05 [−log10(0.05)≈4.139]. Names of genes with P < 0.05 are included. Genes that were downregulated are presented in red and those upregulated in blue. (D) Heat map representation of gene expression determined by reverse transcription-quantitative polymerase chain reaction (RT-qPCR) within saline control (SalCon; n = 5), creatine control (CrCon; n = 7), saline UCO (SalUCO; n = 7) and creatine UCO (CrUCO; n = 6) fetuses. Brain regions analysed include cortical grey matter (GM); white matter (WM); hippocampus (Hipp); striatum; and thalamus. Red indicates downregulation of mRNA expression; blue indicates upregulation relative to saline controls. Data are average log2 transformed fold change.
Umbilical cord occlusion resulted in significant downregulation of mRNA expression of multiple genes in the GM and thalamus (Figure 1A). Within the cortical GM, UCO resulted in downregulation of NFκB1 [F(1, 18) = 4.69, PUCO = 0.044], VEGFA [F(1, 18) = 4.86, PUCO = 0.041] and FASN expression [F(1, 18) = 10.23, PUCO = 0.005]. These genes are involved in cellular regulation, vasculature growth and fatty acid synthesis, respectively (Figure 1D and Supplementary Table 2). In the thalamus, UCO resulted in decreased expression of cellular regulatory genes: TGF-β [F(1, 19) = 8.04, PUCO = 0.011], NFκB1 [F(1, 17) = 13.23, PUCO = 0.002], NRG1 [F(1, 19) = 5.986, PUCO = 0.024] and PPARγ [F(1, 19) = 4.3, PUCO = 0.050]; the choline transporter, SLC5A7 [F(1, 17) = 8.62, PUCO = 0.009]; genes involved in mitochondrial biogenesis MFF [F(1, 19) = 5.23, PUCO = 0.034], SIRT1 [F(1, 19) = 5.11, PUCO = 0.036], and TFAM [F(1, 19) = 4.45, PUCO = 0.049]; and the gene encoding mitochondrial complex III [F(1, 19) = 4.94, PUCO = 0.039]. Expression of the angiogenic gene, ANGPT2 [F(1, 19) = 13.98, PUCO = 0.001] and tight-junction protein OCLN [F(1, 19) = 9.86, PUCO = 0.005] were also downregulated with UCO in the thalamus (Figure 1D and Supplementary Table 2). No gene expression changes were found in the WM, hippocampus and striatum as a result of the UCO.
Creatine treatment irrespective of hypoxia resulted in altered gene expression within the cortical GM, hippocampus and striatum (Figure 1B). Within the cortical GM, creatine treatment upregulated the pro-inflammatory genes TNFa [F(1, 12) = 6.28, PTREAT = 0.028] and IL-6 [F(1, 17) = 7.45, PTREAT = 0.014], and downregulated TLR3 expression [F(1, 18) = 5.52, PTREAT = 0.031] (Figure 1D and Supplementary Table 2). Within the hippocampus, NOX1 expression, a gene encoding NADPH oxidase 1, which has a primary function in generating free radicals, was downregulated with creatine treatment [F(1, 15) = 7.64, PTREAT = 0.015]. Also within the hippocampus, SLC5A7, a gene encoding the choline uptake transporter, was upregulated with creatine supplementation [F(1, 16) = 4.636, PTREAT = 0.0469] (Figure 1D and Supplementary Table 2). Lastly, in the striatum, creatine treatment upregulated anti-apoptotic BCL2 [F(1, 20) = 5.66, PTREAT = 0.028], pro-inflammatory cytokine IL-1β [F(1, 20) = 5.80, PTREAT = 0.026], MPO, a gene encoding myeloperoxidase protein, an inflammatory enzyme that triggers oxidative stress and neuroinflammatory processes [F(1, 17) = 12.34, PTREAT = 0.003], and creatine kinase CKMT [F(1, 20) = 6.77, PTREAT = 0.017] (Figure 1D and Supplementary Table 2).
Within the striatum, there was a UCO and creatine interaction effect on both PPARγ [F(1, 20) = 5.01, PINT = 0.037] and PTGS1 [F(1, 20) = 4.41, PINT = 0.049] with post-hoc test revealing that in the absence of UCO, both PPARγ (1.7-fold; P = 0.042) and PTGS1 (2.2-fold; P = 0.043) gene expression was increased in CrCon fetuses compared to SalCon fetuses (Figures 1B, D and Supplementary Table 2). Also in the striatum, there was a UCO and creatine interaction effect on the expression of pro-apoptotic BAK1 [F(1, 20) = 9.12, PINT = 0.0068], but post-hoc tests revealed no significant differences between groups (Supplementary Table 2).
In the hippocampus, there was a UCO and creatine interaction effect on NOX1 [F(1, 15) = 7.33, PINT = 0.016] expression, where in the absence of UCO, creatine treatment (CrCon) downregulated the expression of NOX1 (compared to SalCon; P = 0.017; Supplementary Table 2). OCLN expression in the hippocampus was also affected by UCO and creatine treatment combined [F(1, 19) = 5.32, PINT = 0.033], however, post-hoc tests revealed no significant differences between groups (Supplementary Table 2). For all the genes examined, no significant effect of creatine following UCO was revealed (Figure 1C).
In addition to the increase in the density of TUNEL-positive cells within the hippocampal CA1-3 region reported previously in the same fetuses (Muccini et al., 2022), this study found that UCO increased cell death in the thalamus 72 h after hypoxia [F(1, 24) = 5.68; PUCO = 0.025; Figure 2A and Table 2], compared to controls. No effect of UCO was observed in the caudate, CC or putamen. Neither creatine treatment alone nor creatine and UCO affected the density of TUNEL-positive cells for any brain region (Table 2).
Figure 2. (A) Representative images of TUNEL-positive cells (black arrows) indicating cell death in thalamus. (B) Representative images of sheep serum extravasation from blood vessels into the brain parenchyma (arrows), and sheep serum contained within the blood vessels (arrowhead) in the subcortical white matter (SCWM) and cortical grey matter (GM). Scale bar represents 100 μm.
There were no effects of UCO with or without creatine on sheep serum extravasation within any brain region (Table 2). Within the SCWM and GM, there was a decrease in the average number of sheep serum-positive blood vessel profiles with creatine treatment alone [F(1, 24) = 6.71, PTREAT = 0.016 and F(1, 24) = 4.39, PTREAT = 0.047, respectively, Figure 2B and Table 2].
No group differences were found within each GM binned analysis and therefore NeuN cell density is expressed as a total summation within the GM. The cell density of NeuN-positive neurons was similar in all groups for all brain regions examined (Figure 3A and Table 2).
Figure 3. (A) Representative images of NeuN-positive cells indicating mature neurons in the cortical grey matter (GM). GM counts were conducted in four bins roughly equivalent to cortical layer I (Bin 1), layer II and III (Bin 2), layer IV and V (Bin 3), and layer VI (Bin 4). No significant differences of bins were found between groups, therefore data were combined and presented as a total average for GM. (B) Representative images of IBA-1-positive cells indicating microglia in the subcortical white matter (SCWM). Insert demonstrates ramified microglia. (C) Representative images of glial fibrillary acidic protein (GFAP)-positive cells and processes indicating astrocytes in the dorsal hippocampal (Hipp) CA1-3, thalamus and corpus callosum (CC). Scale bar represents 100 μm.
Figure 4. (A) Representative images of Olig2-positive oligodendrocytes in the subcortical white matter (SCWM). (B) Representative images of CNPase-positive staining in the periventricular white matter (PVWM) and external capsule. (C) Representative images of MBP-positive oligodendrocytes and fibers indicating mature myelination in the rostral SCWM. Scale bar represents 100 μm.
There were no significant changes in the cell density and area coverage of IBA-1-positive staining within any of the brain regions (Table 2). There was, however, a near to significant trend for a UCO effect on increasing IBA-1 cell density in both the SCWM and hippocampal DG (PUCO = 0.056 and PUCO = 0.055, respectively, Figure 3B and Table 2).
Umbilical cord occlusion increased GFAP-positive cell density in the hippocampal CA1-3 region [F(1, 24) = 4.31, PUCO = 0.049; Figure 3C and Table 2] with a trend to also increase astrocyte density within the hippocampal DG [F(1, 24) = 3.60; PUCO = 0.070; Table 2]. UCO also increased GFAP-positive area coverage in the thalamus compared to controls [F(1, 24) = 4.77, PUCO = 0.039; Table 2 and Figure 3C]. In the CC of saline-treated fetuses, UCO increased GFAP-positive area coverage 50.5% compared to SalCon (P = 0.03). This increase in GFAP-positive area coverage caused by the UCO was reduced in creatine-treated fetuses by 20.6% [F(1, 24) = 9.468,PINT = 0.005; Table 2 and Figure 3C].
White matter development and integrity was assessed using oligodendrocyte lineage and myelination markers (see Figure 4). There was no effect of UCO or creatine treatment on Olig-2-positive cell density in any brain region (Table 2 and Figure 4A). Analysis of the CNPase-positive area coverage revealed that creatine treatment decreased CNPase-positive area coverage in the PVWM by 14.5% [F(1, 24) = 4.65, PTREAT = 0.041], but in contrast, the CNPase area coverage was increased by 18% in the external capsule [F(1, 22) = 4.37, PTREAT = 0.048; Table 2 and Figure 4B]. Assessment of mature oligodendrocytes using MBP-positive cell density revealed no effects of creatine nor UCO (Table 2). However, MBP-positive area coverage in the SCWM showed a rostro-caudal difference, with a significant creatine treatment main effect as well as a significant interaction of treatment and UCO at the more rostral levels of the brain [F(1, 24) = 5.70, PTREAT = 0.025; F(1, 24) = 4.28, PINT = 0.050; Table 2 and Figure 4C]. Post-hoc test revealed that creatine treatment alone significantly increased the MBP-positive area coverage in the SCWM (P = 0.027).
This study examined brain region-specific neuropathology 72 h after a brief (10 min) in utero acute hypoxia at a gene expression and histopathology level, and assessed whether creatine treatment commenced before the hypoxic episode affected these outcomes. The cortical GM, thalamus and hippocampus were particularly susceptible to neuropathology following UCO irrespective of creatine treatment, with increased evidence of cell death and neuroinflammation, and changes in expression of many genes associated with the regulation of injury responses, the vasculature, and mitochondrial function. Creatine treatment prior to hypoxia did not have a large impact on any of the indices of neuropathology induced by the UCO, except for reducing the astrogliosis in the corpus callosum. Interestingly, there were numerous effects of creatine treatment alone on gene expression, particularly of apoptotic, inflammatory, and oxidative stress-related pathways in the cortical GM, hippocampus and striatum. However, these transcriptional changes were not reflected at a histopathological level.
It was evident that specific brain regions, namely, the cortical GM, thalamus, and hippocampus were particularly vulnerable to the mild hypoxia caused by UCO in these near-term fetal sheep. This is consistent with clinical presentations in which term human infants have a predilection for brain injury patterns involving watershed regions; i.e., cortical gray matter and the basal ganglia (Miller et al., 2005). In experimental newborn animals, the hippocampus also appears to be particularly vulnerable to hypoxic-ischemic injury (Martin et al., 1997; Roland et al., 1998; Kreisman et al., 2000). This pattern of regional vulnerability in the term newborn is thought to be due to the presence of dense neuronal populations, their high energy requirements and inherent susceptibility to excitotoxicity, oxidative stress, and apoptosis (Billiards et al., 2006).
Whilst the hypoxic injury evident at 72 h post-UCO was relatively mild there were indications of persisting injury within the GM and thalamus. Modulation of neuropathological processes such as apoptosis, inflammation, perturbed energy metabolism and angiogenesis following hypoxia are predominately mediated by HIF-1α induction (Liang et al., 2018). In the present study, there were no significant changes in HIF-1α gene expression 72 h after UCO. Despite this, after UCO we observed reduced gene expression in the cortical GM and thalamus of factors downstream to HIF-1α, such as the pluripotent transcription factor NFκB1, pleotropic cytokine TGF-β, synaptogenic protein NRG1, the pleotropic ligand-inducible nuclear transcription factor PPARγ, and vascular growth factors VEGFA and ANGPT2 – all key factors associated with HIF-1α function (Klempt et al., 1992; Zhu et al., 2002; Pichiule et al., 2004; Zhao et al., 2009; Akwii et al., 2019; Navarro-González et al., 2019; Yoo et al., 2019). Given their individual roles in maintaining cellular homeostasis and neuroprotection, the decreased expression of these genes may suggest reduced cellular protection and the potential for persisting cellular perturbations. It is important to note that the physiological changes provoked by UCO had all returned to pre-UCO levels by 24 h post-UCO (Tran et al., 2021b,2022), so that decreased gene expression after 72 h suggests persisting cellular effects within the cortical GM and thalamus following acute in utero hypoxia, and perhaps an increased susceptibility to the effects of any subsequent hypoxic episode that might arise before or after birth.
We observed minimal histopathology and no effect on gene expression following UCO in WM regions, contrary to our previous indications of disturbed mitochondrial respiration in the WM of the same fetuses (Muccini et al., 2022). We were unable to separate cellular populations within our procedure for mitochondria isolation in this previous study (Muccini et al., 2022), and therefore cannot be certain which cell type may, or may not have had, disruption of mitochondrial respiration. Though, it has been shown that mature oligodendrocytes are not particularly vulnerable to perturbations to the electron transport chain (Fünfschilling et al., 2012). Moreover, given the trend for increased microglial density within the SCWM, it is likely another cell type within the WM were affected by the UCO at 72 h which requires further investigation. Collectively, the neuropathology present 72 h after even a mild hypoxic insult suggests a potential progression or development of delayed neurodegeneration over a long period of time (Miller and Ferriero, 2009; Baburamani et al., 2021).
We observed that creatine treatment reduced astrocyte area coverage following UCO in the corpus callosum, suggesting a potential protective effect following hypoxic injury (Raivich et al., 1999; Chiareli et al., 2021). The reason why this creatine effect was only seen in the corpus callosum, and not elsewhere such as in the thalamus and hippocampus and white matter regions, requires further elucidation. The finding is of clinical interest as callosal injuries have been identified in newborns diagnosed with HIE, and this kind of injury contributes to poor neurological outcomes (Mañeru et al., 2003; Epelman et al., 2012). It is possible that this protective effect of creatine in the corpus callosum may be present only after mild and brief hypoxia as induced in this study, and therefore further study of a more severe hypoxic injury is warranted.
In this study, the continuous infusion of creatine to the fetus for 13 days resulted in multiple effects that indicate changes to the innate immune system. We found that creatine treatment downregulated gene expression of TLR3 in the GM. A similar downregulation of toll-like receptor expression has been observed in vitro in a mouse macrophage cell line (Leland et al., 2011). Moreover, in the GM and striatum we observed upregulated expression of major cytokine genes (TNFα, IL-6 and IL-1β), of myeloperoxidase (MPO), a critical inflammatory enzyme involved in triggering oxidative stress responses, and of PTGS1, the gene encoding cyclooxygenase 1 involved in producing prostaglandins. The increased expression of pro-inflammatory and oxidative stress mediators suggests an increasing pro-inflammatory environment as has been indicated by studies in the lungs showing that creatine supplementation can exacerbate pulmonary inflammation by inducing airway inflammation, remodeling and hyper-responsiveness (Vieira et al., 2007; Garcia et al., 2019).
However, alternatively, these results could actually indicate changes in the physiological resting state that could be protective, as observed in studies where creatine supplementation has anti-inflammatory effects following in vitro oxidative and in vivo exercise injury (Nomura et al., 2003; Bassit et al., 2008). Creatine may potentially aid in controlling particular proinflammatory states (Bredahl et al., 2021), which may have implications for perinatal diseases such as cerebral palsy (Gouveia et al., 2022). The downregulation of NADPH oxidase 1 (NOX1) expression in the hippocampus supports this hypothesis of improved resistance to hypoxic injury, as genetic deletion or inhibition of NOX1 reduces ischemic lesions, preserves BBB integrity (Kahles et al., 2010), reduces ROS production (Coyoy et al., 2008), and reduces the extent of neuronal degeneration after stroke (Choi et al., 2015). Regardless, whether these gene expression changes are reflected in changes in the expressed proteins needs to be ascertained. Importantly, we did not find protein immunostaining evidence that creatine supplementation alone increases the presence of neuroglia (microglia and astrocytes) nor increases markers of oxidative stress, as reported previously (Tran et al., 2022). Therefore, these creatine effects on inflammatory pathways need to be investigated further, as we cannot conclusively say if these changes have adverse or protective effects overall. Though, the latter is more likely as these creatine-induced inflammatory transcriptional changes did not present as adverse changes in protein expression (i.e., no changes in microglia and astrocyte cell density and improved vascular integrity).
Importantly, we show here for the first time that creatine supplementation irrespective of hypoxia did have effects on creatine metabolism in the fetal brain. Creatine supplementation significantly increased gene expression for mitochondrial creatine kinase (CKMT) in the striatum, with a trend for an increase in the GM and hippocampus but decreased expression in the WM as reported previously (Muccini et al., 2022). These results complement the increases in total creatine content found within the striatum, GM, and hippocampus, and no increase in the WM in the same fetuses (Tran et al., 2021b). The increased expression of CKMT is consistent with an increased need for creatine phosphorylation to maintain the 1:3 ratio of creatine to phosphocreatine within cells as creatine levels increase (Wallimann et al., 1992). Indeed, endogenous creatine concentrations are known to be higher in GM regions (McLean et al., 2000), and CKMT is more highly expressed in neuronal populations due to their high oxidative metabolism (Tachikawa et al., 2004; Lowe et al., 2013). Moreover, the decreased expression of CKMT but no change in creatine tissue content in the WM is consistent with the findings of Chamberlain et al. (Chamberlain et al., 2017) who reported that creatine treatment of primary oligodendrocyte cultures enhanced mitochondria density and ATP production, suggesting a reduced need for creatine phosphorylation and therefore a decreased need for CKMT expression. Moreover, in the present study there were no expression changes to SLC6A8, the gene that encodes the creatine transporter with creatine supplementation, consistent with other reports (Brault et al., 2003; Tarnopolsky et al., 2003; Andre et al., 2015).
Interestingly, there were also indications that prolonged creatine supplementation may have long term effects on cellular function. In the hippocampus creatine supplementation increased SLC5A7 expression. SLC5A7 encodes the high-affinity choline transporter that maintains cholinergic neurotransmission by supporting the synthesis of acetylcholine (Okuda et al., 2000). Cholinergic input to the hippocampus is involved in cognitive processing and extinction memory (Ballinger et al., 2016; Knox and Keller, 2016). Recently, multiple studies have investigated the use of creatine supplementation to enhance cognitive performance which, in part, may be due to increased choline availability and utilization see review (Dolan et al., 2019). We also observed reduced CNPase area coverage in the PVWM, increased MBP-positive area coverage in the rostral SCWM, and increased CNPase in the external capsule after the 13 days of creatine treatment irrespective of hypoxia. Creatine kinase activity and density is inherently high in oligodendrocytes suggesting their cellular function and response to injury could also be modified by increased creatine levels (Manos and Bryan, 1993). Appropriate myelinogenesis is integral for the establishment and consolidation of functional neuronal networks (Mathis et al., 2001; Doretto et al., 2011), and our study indicates a potential for creatine to enhance myelination in the developing brain. The increased amount of mature myelin observed in creatine-treated fetuses was primarily within the rostral SCWM section, i.e., post-central sulci, involving the somatosensory cortex and not in the caudal SCWM section, which includes the occipital lobe cortex (see Supplementary Figure 1). This finding suggests an effect on sensory information processing. Early and accelerated abnormal maturation of white matter has been observed in adolescents with autism spectrum disorder (Ben Bashat et al., 2007), therefore the increased myelin maturation may indicate an increased susceptibility to neurodevelopmental disorders with sensory disabilities. However, recent work supports the idea that increased in utero myelin maturation is coupled with a reduced rate of maturation after birth, meaning overall myelination remains balanced (Grotheer et al., 2022), and thus the increased myelin maturation may have no long-term effects or rather improved cognition (Chevalier et al., 2015). Regardless, further studies with and without increased in utero creatine exposure are required to understand these myelin maturation processes and subsequent consequences on neurodevelopment.
Together, this study highlights potential neuroprotective but also potentially adverse neurodevelopmental effects of increased creatine exposure in utero. Human data demonstrates that there are no toxicity or adverse risks associated with high and prolonged exposure to oral creatine monohydrate in non-pregnant females (de Guingand et al., 2020), and investigations in spiny mice that followed offspring until sexual maturity (3 months of age) found no difference in development between pups exposed to creatine during pregnancy and controls (LaRosa et al., 2016; Ellery et al., 2017). However, continuing to include assessments of the potential effects of creatine supplementation alone in preclinical studies will provide important safety and efficacy data to ensure effective and appropriate translation of promising findings.
A limitation of this study is the assessment of neuropathology at a single time point post-UCO and creatine treatment. Indeed, the pathogenesis of hypoxia-ischemia encephalopathy involves an evolution of injury and recovery over time. Therefore, an assessment at a single time point cannot completely reflect the dynamic and complex development of injury and repair. By allowing 72 h to elapse after UCO there may already be partial or near complete restoration of cellular homeostasis, by which time some of the effects of creatine treatment would no longer be apparent. Indeed, a follow-up analysis of protein levels is needed to confirm the biological importance of observed gene expression changes. However, as differences in gene expression persisted 72 h post UCO and 13 days of creatine infusion suggests that there is likely changes to protein levels and thus cellular metabolism and function.
Also important to note is that this study evidently reflects a quite mild hypoxic insult given the absence of profound brain damage (Tran et al., 2021b). Whilst mild neonatal HIE cases are now increasingly recognized as a significant risk factor for an impaired neurodevelopmental trajectory (Conway et al., 2018), clinically, abnormalities are detectable by cerebral magnetic resonance imaging by 3 days of life (Walsh et al., 2017). Therefore, the 10 min UCO within this cohort of fetal sheep may not properly reflect these clinical mild HIE cases that are at risk of developing into cases of only mild neurodevelopmental delays. Nonetheless, the creatine effects irrespective of UCO found within this study may reflect neuroprotective effects that were not identifiable due to the mild mature of the hypoxic insult and the timing of assessment. Future studies assessing different time points (i.e., during primary, secondary, and tertiary energy failure) and following up assessments of protein levels reflecting the gene expression data are needed. Moreover, extending the analyses presented in this study into a more moderate-to-severe model of hypoxia would not only be clinically relevant but may also provide a better understanding and consolidation of the potential neuroprotective effects of creatine.
Another limitation of this study was the small sample size akin to large animal studies. Small group sizes may reduce the effect size of observed differences between groups and can underpower effects that would have otherwise been significant with larger group sizes. Nonetheless, this study has highlighted multiple cellular pathways affected by UCO and creatine, and in detail investigations are now required to elucidate these findings.
Finally, in this study it was necessary to infuse creatine directly into the fetus, as the ovine placenta does not transfer creatine in a maternal-fetal direction (Baharom et al., 2017). It is possible that, in human studies where it would be envisaged that fetal creatine supplementation would follow increased maternal intake of creatine, that there are effects on the placenta that may or may not improve fetal health. The full clinical evaluation of creatine supplementation in an obstetric population is yet to be undertaken.
In the present study, brain region-specific changes of mRNA expression and histopathology were present 72 h after a transient, mild global hypoxia in the near-term fetal sheep. Hypoxia resulted in significant astrogliosis and down-regulation of genes involved in cellular regulation, vasculature integrity and mitochondrial dynamics in the cortical GM and thalamus. Creatine supplementation ameliorated astrogliosis within the corpus callosum caused by hypoxia, however, no other obvious protective effects were found. Of particular interest is that creatine supplementation alone induced transcriptional changes in apoptotic and inflammatory pathways in the cortical gray matter, striatum and hippocampus, with evidence of increased myelination. This creatine effect warrants further investigation, as understanding any effects of creatine supplementation on normal fetal brain development will be essential if the proposal to give creatine prophylactically to pregnant women is to be a realistic clinical option.
The original contributions presented in this study are included in the article/Supplementary material, further inquiries can be directed to the corresponding author.
The animal study was reviewed and approved by Monash Medical Centre Animal Ethics Committee.
NT, NH, AM, RS, DW, MT, and SE: study conception. NT, NH, MT, and SE: methodology. NT, AM, NH, and SE: data curation. NT, AM, and NH: data analysis. NT: writing—original draft preparation. NT, NH, RS, DW, MT, and SE: writing—review and editing. All authors contributed to the article and approved the submitted version.
NT was supported by a Ph.D. scholarship from the School of Health and Biomedical Sciences, RMIT University. This research was supported by a grant to DW, RS, and SE from the National Health and Medical Research Council of Australia (1124493), and to DW and SE from the Victorian Government Infrastructure Support Scheme. SE was supported by Australian National Health and Medical Research Council (NHMRC) Early Career Fellowship (1125539). MT was an Australian Research Council Future Fellow (FT180100082).
We acknowledge the support provided by Ilias Nitsos, Dalibor Stanojkovic and the Monash University animal staff for their assistance in animal handling and care.
The authors declare that the research was conducted in the absence of any commercial or financial relationships that could be construed as a potential conflict of interest.
All claims expressed in this article are solely those of the authors and do not necessarily represent those of their affiliated organizations, or those of the publisher, the editors and the reviewers. Any product that may be evaluated in this article, or claim that may be made by its manufacturer, is not guaranteed or endorsed by the publisher.
The Supplementary Material for this article can be found online at: https://www.frontiersin.org/articles/10.3389/fncel.2023.1154772/full#supplementary-material
Akwii, R., Sajib, M., Zahra, F., and Mikelis, C. (2019). Role of angiopoietin-2 in vascular physiology and pathophysiology. Cells 8:471. doi: 10.3390/cells8050471
Andre, T., McKinley-Barnard, S., Gann, J., and Willoughby, D. (2015). The effects of creatine monohydrate supplementation on creatine transporter activity and creatine metabolism in resistance trained males. J. Int. Soc. Sports Nutr. 12(Suppl. 1):43. doi: 10.1186/1550-2783-12-S1-P43
Andres, R., Huber, A., Schlattner, U., Perez-Bouza, A., Krebs, S., Seiler, R., et al. (2005). Effects of creatine treatment on the survival of dopaminergic neurons in cultured fetal ventral mesencephalic tissue. Neuroscience 133, 701–713. doi: 10.1016/j.neuroscience.2005.03.004
Baburamani, A., Tran, N., Castillo-Melendez, M., Yawno, T., and Walker, D. (2021). Brief hypoxia in late gestation sheep causes prolonged disruption of fetal electrographic, breathing behaviours and can result in early labour. J. Physiol. 599, 3221–3236. doi: 10.1113/JP281266
Baharom, S., Matteo, R., Ellery, S., Gatta, P., Bruce, C., Kowalski, G., et al. (2017). Does maternal-fetal transfer of creatine occur in pregnant sheep? Am. J. Physiol. Endocrinol. Metab. 313, E75–E83. doi: 10.1152/ajpendo.00450.2016
Ballinger, E., Ananth, M., Talmage, D., and Role, L. (2016). Basal forebrain cholinergic circuits and signaling in cognition and cognitive decline. Neuron 91, 1199–1218. doi: 10.1016/j.neuron.2016.09.006
Barkovich, A., Westmark, K., Partridge, C., Sola, A., and Ferriero, D. (1995). Perinatal asphyxia: MR findings in the first 10 days. AJNR Am. J. Neuroradiol. 16, 427–438.
Bassit, R., Curi, R., and Costa Rosa, L. (2008). Creatine supplementation reduces plasma levels of pro-inflammatory cytokines and PGE2 after a half-ironman competition. Amino Acids 35, 425–431. doi: 10.1007/s00726-007-0582-4
Béard, E., and Braissant, O. (2010). Synthesis and transport of creatine in the CNS: Importance for cerebral functions. J. Neurochem. 115, 297–313. doi: 10.1111/j.1471-4159.2010.06935.x
Ben Bashat, D., Kronfeld-Duenias, V., Zachor, D., Ekstein, P., Hendler, T., Tarrasch, R., et al. (2007). Accelerated maturation of white matter in young children with autism: A high b value DWI study. Neuroimage 37, 40–47. doi: 10.1016/j.neuroimage.2007.04.060
Bianchi, M., Tosetti, M., Fornai, F., Alessandri, M., Cipriani, P., De Vito, G., et al. (2000). Reversible brain creatine deficiency in two sisters with normal blood creatine level. Ann. Neurol. 47, 511–513. doi: 10.1002/1531-8249(200004)47:4<511::AID-ANA15>3.0.CO;2-N
Billiards, S., Pierson, C., Haynes, R., Folkerth, R., and Kinney, H. (2006). Is the late preterm infant more vulnerable to gray matter injury than the term infant? Clin. Perinatol. 33, 915–933. doi: 10.1016/j.clp.2006.10.003
Braissant, O., Bachmann, C., and Henry, H. (2007). Expression and function of AGAT, GAMT and CT1 in the mammalian brain. Subcell. Biochem. 46, 67–81.
Brault, J., Abraham, K., and Terjung, R. (2003). Muscle creatine uptake and creatine transporter expression in response to creatine supplementation and depletion. J. Appl. Physiol. 94, 2173–2180. doi: 10.1152/japplphysiol.01171.2002
Bredahl, E., Eckerson, J., Tracy, S., McDonald, T., and Drescher, K. (2021). The role of creatine in the development and activation of immune responses. Nutrients 13:751. doi: 10.3390/nu13030751
Chamberlain, K., Chapey, K., Nanescu, S., and Huang, J. (2017). Creatine enhances mitochondrial-mediated oligodendrocyte survival after demyelinating injury. J. Neurosci. 37, 1479–1492. doi: 10.1523/JNEUROSCI.1941-16.2016
Chevalier, N., Kurth, S., Doucette, M., Wiseheart, M., Deoni, S., Dean, D. III, et al. (2015). Myelination is associated with processing speed in early childhood: Preliminary insights. PLoS One 10:e0139897. doi: 10.1371/journal.pone.0139897
Chiareli, R., Carvalho, G., Marques, B., Mota, L., Oliveira-Lima, O., Gomes, R., et al. (2021). The role of astrocytes in the neurorepair process. Front. Cell Dev. Biol. 9:665795. doi: 10.3389/fcell.2021.665795
Choi, D., Kim, J., Lee, K., Kim, H., Kim, Y., Choi, W., et al. (2015). Role of neuronal NADPH oxidase 1 in the peri-infarct regions after stroke. PLoS One 10:e0116814. doi: 10.1371/journal.pone.0116814
Conway, J., Walsh, B., Boylan, G., and Murray, D. (2018). Mild hypoxic ischaemic encephalopathy and long term neurodevelopmental outcome - A systematic review. Early Hum. Dev. 120, 80–87. doi: 10.1016/j.earlhumdev.2018.02.007
Coyoy, A., Valencia, A., Guemez-Gamboa, A., and Morán, J. (2008). Role of NADPH oxidase in the apoptotic death of cultured cerebellar granule neurons. Free Radic. Biol. Med. 45, 1056–1064. doi: 10.1016/j.freeradbiomed.2008.06.027
de Guingand, D., Palmer, K., Snow, R., Davies-Tuck, M., and Ellery, S. (2020). Risk of adverse outcomes in females taking oral creatine monohydrate: A systematic review and meta-analysis. Nutrients 12:1780. doi: 10.3390/nu12061780
Dickinson, H., Ellery, S., Ireland, Z., LaRosa, D., Snow, R., and Walker, D. (2014). Creatine supplementation during pregnancy: Summary of experimental studies suggesting a treatment to improve fetal and neonatal morbidity and reduce mortality in high-risk human pregnancy. BMC Pregnancy Childbirth 14:150. doi: 10.1186/1471-2393-14-150
Dolan, E., Gualano, B., and Rawson, E. (2019). Beyond muscle: The effects of creatine supplementation on brain creatine, cognitive processing, and traumatic brain injury. Eur. J. Sport Sci. 19, 1–14. doi: 10.1080/17461391.2018.1500644
Doretto, S., Malerba, M., Ramos, M., Ikrar, T., Kinoshita, C., De Mei, C., et al. (2011). Oligodendrocytes as regulators of neuronal networks during early postnatal development. PLoS One 6:e19849. doi: 10.1371/journal.pone.0019849
Ellery, S., Dickinson, H., McKenzie, M., and Walker, D. (2016). Dietary interventions designed to protect the perinatal brain from hypoxic-ischemic encephalopathy–Creatine prophylaxis and the need for multi-organ protection. Neurochem. Int. 95, 15–23. doi: 10.1016/j.neuint.2015.11.002
Ellery, S., LaRosa, D., Cullen-McEwen, L., Brown, R., Snow, R., Walker, D., et al. (2017). Renal dysfunction in early adulthood following birth asphyxia in male spiny mice, and its amelioration by maternal creatine supplementation during pregnancy. Pediatr. Res. 81, 646–653. doi: 10.1038/pr.2016.268
Epelman, M., Daneman, A., Halliday, W., Whyte, H., and Blaser, S. (2012). Abnormal corpus callosum in neonates after hypoxic-ischemic injury. Pediatr. Radiol. 42, 321–330.
Fünfschilling, U., Supplie, L., Mahad, D., Boretius, S., Saab, A., Edgar, J., et al. (2012). Glycolytic oligodendrocytes maintain myelin and long-term axonal integrity. Nature 485, 517–521. doi: 10.1038/nature11007
Garcia, M., Santos-Dias, A., Bachi, A., Oliveira-Junior, M., Andrade-Souza, A., Ferreira, S., et al. (2019). Creatine supplementation impairs airway inflammation in an experimental model of asthma involving P2 × 7 receptor. Eur. J. Immunol. 49, 928–939. doi: 10.1002/eji.201847657
Gouveia, H., Manhães-de-Castro, R., Lacerda, D., and Toscano, A. (2022). Creatine supplementation to improve the peripheral and central inflammatory profile in cerebral palsy. Clin. Nutr. ESPEN 52, 254–256. doi: 10.1016/j.clnesp.2022.11.016
Grotheer, M., Rosenke, M., Wu, H., Kular, H., Querdasi, F., Natu, V., et al. (2022). White matter myelination during early infancy is linked to spatial gradients and myelin content at birth. Nat. Commun. 13:997. doi: 10.1038/s41467-022-28326-4
Gunn, A., and Bennet, L. (2009). Fetal hypoxia insults and patterns of brain injury: Insights from animal models. Clin. Perinatol. 36, 579–593. doi: 10.1016/j.clp.2009.06.007
Hayes, B., Doherty, E., Grehan, A., Madigan, C., McGarvey, C., Mulvany, S., et al. (2018). Neurodevelopmental outcome in survivors of hypoxic ischemic encephalopathy without cerebral palsy. Eur. J. Pediatr. 177, 19–32. doi: 10.1007/s00431-017-3028-3
Kahles, T., Kohnen, A., Heumueller, S., Rappert, A., Bechmann, I., Liebner, S., et al. (2010). NADPH oxidase Nox1 contributes to ischemic injury in experimental stroke in mice. Neurobiol. Dis. 40, 185–192. doi: 10.1016/j.nbd.2010.05.023
Klempt, N., Sirimanne, E., Gunn, A., Klempt, M., Singh, K., Williams, C., et al. (1992). Hypoxia-ischemia induces transforming growth factor beta 1 mRNA in the infant rat brain. Brain Res. Mol. Brain Res. 13, 93–101. doi: 10.1016/0169-328x(92)90048-g
Knox, D., and Keller, S. (2016). Cholinergic neuronal lesions in the medial septum and vertical limb of the diagonal bands of Broca induce contextual fear memory generalization and impair acquisition of fear extinction. Hippocampus 26, 718–726. doi: 10.1002/hipo.22553
Kreisman, N., Soliman, S., and Gozal, D. (2000). Regional differences in hypoxic depolarization and swelling in hippocampal slices. J. Neurophysiol. 83, 1031–1038.
LaRosa, D., Ellery, S., Snow, R., Walker, D., and Dickinson, H. (2016). Maternal creatine supplementation during pregnancy prevents acute and long-term deficits in skeletal muscle after birth asphyxia: A study of structure and function of hind limb muscle in the spiny mouse. Pediatr. Res. 80, 852–860. doi: 10.1038/pr.2016.153
Leland, K., McDonald, T., and Drescher, K. (2011). Effect of creatine, creatinine, and creatine ethyl ester on TLR expression in macrophages. Int. Immunopharmacol. 11, 1341–1347. doi: 10.1016/j.intimp.2011.04.018
Liang, X., Liu, X., Lu, F., Zhang, Y., Jiang, X., and Ferriero, D. (2018). HIF1α signaling in the endogenous protective responses after neonatal brain hypoxia-ischemia. Dev. Neurosci. 40, 617–626. doi: 10.1159/000495879
Livak, K., and Schmittgen, T. (2001). Analysis of relative gene expression data using real-time quantitative PCR and the 2(−Delta Delta C(T)) Method. Methods 25, 402–408.
Lowe, M., Kim, E., Faull, R., Christie, D., and Waldvogel, H. (2013). Dissociated expression of mitochondrial and cytosolic creatine kinases in the human brain: A new perspective on the role of creatine in brain energy metabolism. J. Cereb. Blood Flow Metab. 33, 1295–1306. doi: 10.1038/jcbfm.2013.84
Mañeru, C., Junqué, C., Salgado-Pineda, P., Serra-Grabulosa, J., Bartrés-Faz, D., Ramirez-Ruiz, B., et al. (2003). Corpus callosum atrophy in adolescents with antecedents of moderate perinatal asphyxia. Brain Inj. 17, 1003–1009. doi: 10.1080/0269905031000110454
Manos, P., and Bryan, G. (1993). Cellular and subcellular compartmentation of creatine kinase in brain. Dev. Neurosci. 15, 271–279.
Martin, L., Brambrink, A., Koehler, R., and Traystman, R. (1997). Primary sensory and forebrain motor systems in the newborn brain are preferentially damaged by hypoxia-ischemia. J. Comp. Neurol. 377, 262–285. doi: 10.1002/(SICI)1096-9861(19970113)377:2<262::AID-CNE8>3.0.CO;2-1
Mathis, C., Denisenko-Nehrbass, N., Girault, J., and Borrelli, E. (2001). Essential role of oligodendrocytes in the formation and maintenance of central nervous system nodal regions. Development 128, 4881–4890. doi: 10.1242/dev.128.23.4881
McLean, M., Woermann, F., Barker, G., and Duncan, J. (2000). Quantitative analysis of short echo time (1)H-MRSI of cerebral gray and white matter. Magn. Reson. Med. 44, 401–411. doi: 10.1002/1522-2594(200009)44:3<401::AID-MRM10>3.0.CO;2-W
Miller, S., and Ferriero, D. (2009). From selective vulnerability to connectivity: Insights from newborn brain imaging. Trends Neurosci. 32, 496–505. doi: 10.1016/j.tins.2009.05.010
Miller, S., Ramaswamy, V., Michelson, D., Barkovich, A., Holshouser, B., Wycliffe, N., et al. (2005). Patterns of brain injury in term neonatal encephalopathy. J. Pediatr. 146, 453–460. doi: 10.1016/j.jpeds.2004.12.026
Muccini, A., Tran, N., de Guingand, D., Philip, M., Della Gatta, P., Galinsky, R., et al. (2021). Creatine metabolism in female reproduction, pregnancy and newborn health. Nutrients 13:490. doi: 10.3390/nu13020490
Muccini, A., Tran, N., Hale, N., McKenzie, M., Snow, R., Walker, D., et al. (2022). The effects of in utero fetal hypoxia and creatine treatment on mitochondrial function in the late gestation fetal sheep brain. Oxid. Med. Cell. Longev. 2022:3255296. doi: 10.1155/2022/3255296
Navarro-González, C., Huerga-Gómez, A., and Fazzari, P. (2019). Nrg1 intracellular signaling is neuroprotective upon stroke. Oxid. Med. Cell. Longev. 2019:3930186. doi: 10.1155/2019/3930186
Nomura, A., Zhang, M., Sakamoto, T., Ishii, Y., Morishima, Y., Mochizuki, M., et al. (2003). Anti-inflammatory activity of creatine supplementation in endothelial cells in vitro. Br. J. Pharmacol. 139, 715–720.
Okereafor, A., Allsop, J., Counsell, S., Fitzpatrick, J., Azzopardi, D., Rutherford, M., et al. (2008). Patterns of brain injury in neonates exposed to perinatal sentinel events. Pediatrics 121, 906–914. doi: 10.1542/peds.2007-0770
Okuda, T., Haga, T., Kanai, Y., Endou, H., Ishihara, T., and Katsura, I. (2000). Identification and characterization of the high-affinity choline transporter. Nat. Neurosci. 3, 120–125. doi: 10.1038/72059
Pichiule, P., Chavez, J., and LaManna, J. (2004). Hypoxic regulation of angiopoietin-2 expression in endothelial cells. J. Biol. Chem. 279, 12171–12180.
Prass, K., Royl, G., Lindauer, U., Freyer, D., Megow, D., Dirnagl, U., et al. (2006). Improved reperfusion and neuroprotection by creatine in a mouse model of stroke. J. Cereb. Blood Flow Metab. 27, 452–459. doi: 10.1038/sj.jcbfm.9600351
Raivich, G., Bohatschek, M., Kloss, C., Werner, A., Jones, L., and Kreutzberg, G. (1999). Neuroglial activation repertoire in the injured brain: Graded response, molecular mechanisms and cues to physiological function. Brain Res. Brain Res. Rev. 30, 77–105. doi: 10.1016/s0165-0173(99)00007-7
Riesberg, L., Weed, S., McDonald, T., Eckerson, J., and Drescher, K. (2016). Beyond muscles: The untapped potential of creatine. Int. Immunopharmacol. 37, 31–42. doi: 10.1016/j.intimp.2015.12.034
Roland, E., Poskitt, K., Rodriguez, E., Lupton, B., and Hill, A. (1998). Perinatal hypoxic-ischemic thalamic injury: Clinical features and neuroimaging. Ann. Neurol. 44, 161–166.
Sartini, S., Lattanzi, D., Ambrogini, P., Di Palma, M., Galati, C., Savelli, D., et al. (2016). Maternal creatine supplementation affects the morpho-functional development of hippocampal neurons in rat offspring. Neuroscience 312, 120–129. doi: 10.1016/j.neuroscience.2015.11.017
Sartini, S., Lattanzi, D., Di Palma, M., Savelli, D., Eusebi, S., Sestili, P., et al. (2019). Maternal creatine supplementation positively affects male rat hippocampal synaptic plasticity in adult offspring. Nutrients 11:2014. doi: 10.3390/nu11092014
Sestili, P., Ambrogini, P., Barbieri, E., Sartini, S., Fimognari, C., Calcabrini, C., et al. (2016). New insights into the trophic and cytoprotective effects of creatine in in vitro and in vivo models of cell maturation. Amino Acids 48, 1897–1911.
Shen, H., and Goldberg, M. (2012). Creatine pretreatment protects cortical axons from energy depletion in vitro. Neurobiol. Dis. 47, 184–193. doi: 10.1016/j.nbd.2012.03.037
Sie, L., Van der Knaap, M., Oosting, J., De Vries, L., Lafeber, H., and Valk, J. (2000). MR patterns of hypoxic-ischemic brain damage after prenatal, perinatal or postnatal asphyxia. Neuropediatrics 31, 128–136. doi: 10.1055/s-2000-7496
Tachikawa, M., Fukaya, M., Terasaki, T., Ohtsuki, S., and Watanabe, M. (2004). Distinct cellular expressions of creatine synthetic enzyme GAMT and creatine kinases uCK-Mi and CK-B suggest a novel neuron–glial relationship for brain energy homeostasis. Eur. J. Neurosci. 20, 144–160. doi: 10.1111/j.1460-9568.2004.03478.x
Tarnopolsky, M., Parise, G., Fu, M., Brose, A., Parshad, A., Speer, O., et al. (2003). Acute and moderate-term creatine monohydrate supplementation does not affect creatine transporter mRNA or protein content in either young or elderly humans. Mol. Cell. Biochem. 244, 159–166.
Tran, N., Kelly, S., Snow, R., Walker, D., Ellery, S., and Galinsky, R. (2021a). Assessing creatine supplementation for neuroprotection against perinatal hypoxic-ischaemic encephalopathy: A systematic review of perinatal and adult pre-clinical studies. Cells 10:2902. doi: 10.3390/cells10112902
Tran, N., Kowalski, G., Muccini, A., Nitsos, I., Hale, N., Snow, R., et al. (2022). Creatine supplementation reduces the cerebral oxidative and metabolic stress responses to acute in utero hypoxia in the late-gestation fetal sheep. J. Physiol. 600, 3193–3210. doi: 10.1113/JP282840
Tran, N., Muccini, A., Snow, R., Nitsos, I., Hale, N., Walker, D., et al. (2021b). The physiological effects of creatine supplementation in fetal sheep before, during, and after umbilical cord occlusion and global hypoxia. J. Appl. Physiol. 131, 1088–1099. doi: 10.1152/japplphysiol.00092.2021
Vannucci, R. (2000). Hypoxic-ischemic encephalopathy. Am. J. Perinatol. 17, 113–120. doi: 10.1055/s-2000-9293
Vieira, R., Duarte, A., Claudino, R., Perini, A., Santos, Â, Moriya, H., et al. (2007). Creatine supplementation exacerbates allergic lung inflammation and airway remodeling in mice. Am. J. Respir. Cell Mol. Biol. 37, 660–667. doi: 10.1165/rcmb.2007-0108OC
Wallimann, T., Tokarska-Schlattner, M., and Schlattner, U. (2011). The creatine kinase system and pleiotropic effects of creatine. Amino Acids 40, 1271–1296. doi: 10.1007/s00726-011-0877-3
Wallimann, T., Wyss, M., Brdiczka, D., Nicolay, K., and Eppenberger, H. (1992). Intracellular compartmentation, structure and function of creatine kinase isoenzymes in tissues with high and fluctuating energy demands: The ‘phosphocreatine circuit’ for cellular energy homeostasis. Biochem. J. 281(Pt 1), 21–40. doi: 10.1042/bj2810021
Walsh, B., Neil, J., Morey, J., Yang, E., Silvera, M., Inder, T., et al. (2017). The frequency and severity of magnetic resonance imaging abnormalities in infants with mild neonatal encephalopathy. J. Pediatr. 187, 26–33.e1. doi: 10.1016/j.jpeds.2017.03.065
Wyss, M., and Kaddurah-Daouk, R. (2000). Creatine and creatinine metabolism. Physiol. Rev. 80, 1107–1213. doi: 10.1152/physrev.2000.80.3.1107
Yoo, S., Yoo, J., Kim, H., Baik, T., Lee, J., and Woo, R. (2019). Neuregulin-1 protects neuronal cells against damage due to CoCl2-induced hypoxia by suppressing hypoxia-inducible factor-1α and P53 in SH-SY5Y cells. Int. Neurourol. J. 23(Suppl. 2), S111–S118. doi: 10.5213/inj.1938190.095
Zhao, X., Strong, R., Zhang, J., Sun, G., Tsien, J., Cui, Z., et al. (2009). Neuronal PPARgamma deficiency increases susceptibility to brain damage after cerebral ischemia. J. Neurosci. 29, 6186–6195. doi: 10.1523/JNEUROSCI.5857-08.2009
Keywords: hypoxia-ischemia encephalopathy, creatine metabolism, neuroprotection, perinatal asphyxia (PNA), perinatal brain injury
Citation: Tran NT, Muccini AM, Hale N, Tolcos M, Snow RJ, Walker DW and Ellery SJ (2023) Creatine in the fetal brain: A regional investigation of acute global hypoxia and creatine supplementation in a translational fetal sheep model. Front. Cell. Neurosci. 17:1154772. doi: 10.3389/fncel.2023.1154772
Received: 31 January 2023; Accepted: 15 March 2023;
Published: 30 March 2023.
Edited by:
Mario Valentino, University of Malta, MaltaReviewed by:
Olivier Braissant, Centre Hospitalier Universitaire Vaudois (CHUV), SwitzerlandCopyright © 2023 Tran, Muccini, Hale, Tolcos, Snow, Walker and Ellery. This is an open-access article distributed under the terms of the Creative Commons Attribution License (CC BY). The use, distribution or reproduction in other forums is permitted, provided the original author(s) and the copyright owner(s) are credited and that the original publication in this journal is cited, in accordance with accepted academic practice. No use, distribution or reproduction is permitted which does not comply with these terms.
*Correspondence: Nhi T. Tran, bmhpLnRyYW5AaHVkc29uLm9yZy5hdQ==
Disclaimer: All claims expressed in this article are solely those of the authors and do not necessarily represent those of their affiliated organizations, or those of the publisher, the editors and the reviewers. Any product that may be evaluated in this article or claim that may be made by its manufacturer is not guaranteed or endorsed by the publisher.
Research integrity at Frontiers
Learn more about the work of our research integrity team to safeguard the quality of each article we publish.