- 1Department of Genetics, University of Texas MD Anderson Cancer Center, Houston, TX, United States
- 2Program in Genetics and Epigenetics, Graduate School of Biomedical Sciences, The University of Texas Health Science Center at Houston, Houston, TX, United States
- 3Department of Neurobiology and Anatomy, McGovern Medical School, The University of Texas Health Science Center at Houston, Houston, TX, United States
- 4Program in Neuroscience, Graduate School of Biomedical Sciences, The University of Texas Health Science Center at Houston, Houston, TX, United States
Dendritic arborization is essential for proper neuronal connectivity and function. Conversely, abnormal dendrite morphology is associated with several neurological pathologies like Alzheimer’s disease and schizophrenia. Among major intrinsic mechanisms that determine the extent of the dendritic arbor is cytoskeletal remodeling. Here, we characterize and compare the impact of the four proteins involved in cytoskeletal remodeling–vertebrate members of the p120-catenin subfamily–on neuronal dendrite morphology. In relation to each of their own distributions, we find that p120-catenin and delta-catenin are expressed at relatively higher proportions in growth cones compared to ARVCF-catenin and p0071-catenin; ARVCF-catenin is expressed at relatively high proportions in the nucleus; and all catenins are expressed in dendritic processes and the soma. Through altering the expression of each p120-subfamily catenin in neurons, we find that exogenous expression of either p120-catenin or delta-catenin correlates with increased dendritic length and branching, whereas their respective depletion decreases dendritic length and branching. While increasing ARVCF-catenin expression also increases dendritic length and branching, decreasing expression has no grossly observable morphological effect. Finally, increasing p0071-catenin expression increases dendritic branching, but not length, while decreasing expression decreases dendritic length and branching. These distinct localization patterns and morphological effects during neuron development suggest that these catenins have both shared and distinct roles in the context of dendrite morphogenesis.
Introduction
Dendrites were discovered over 100 years ago, and perhaps their most notable feature is their elaborate range of morphologies that make up the dendritic arbor. Through precise extension, branching, elongation, retraction, and pruning, dendritic arbors allow neurons to connect with each other to form neural circuits (Torben-Nielsen and Cuntz, 2014). Conversely, abnormal dendrite morphology is seen in neurological diseases such as Alzheimer’s disease, schizophrenia, and Down Syndrome (Kaufmann and Moser, 2000; Elston, 2003; Kolomeets et al., 2007; Couch et al., 2010; Kulkarni and Firestein, 2012; Martinez-Cerdeño, 2017). While there is much to be discovered about the mechanisms of dendritic arborization, there are several known molecular mechanisms of control (Jan and Jan, 2010; de la Torre-Ubieta and Bonni, 2011; Arikkath, 2012). These mechanisms are carried out through different categories of proteins, which include cell adhesion molecules, cytoskeleton regulators, cell surface receptors, secreted molecules, postsynaptic density proteins, secreted molecules, signaling molecules, transcription factors, calcium signaling proteins, and molecules that control Golgi trafficking (Yacoubian and Lo, 2000; Redmond et al., 2002; Huang et al., 2005; Charych et al., 2006; Elia et al., 2006; Ye et al., 2007; Puram et al., 2011; Arikkath, 2012). Of these various classes, our study focuses on catenins, which are involved in cell adhesion and cytoskeletal reorganization. More specifically, this study investigates and compares how each member in the p120-catenin subfamily affects dendrite morphogenesis during neuron development.
The vertebrate p120-catenin subfamily is made up of four proteins: p120-catenin (gene name CTNND1), delta-catenin (gene name CTNND2), ARVCF-catenin (gene name ARVCF), and p0071-catenin (gene name PKP4). This subfamily of proteins is characterized by a central series of 9 or 10 Armadillo (ARM) repeats that are located between each catenin’s N- and C-terminal tails. Of these four proteins, all except p120-catenin contains a PDZ-binding motif at the C-terminus, which enables them to selectively bind to partner proteins that contain appropriate PDZ domains (Yuan and Arikkath, 2017). The domains and structures of each catenin are shown in Figure 1. Starting from the N-terminus, the coiled coil domain is thought to recruit other catenin binding partners to the cadherin-catenin complex (Markham et al., 2014). The ARM repeats in the middle of the catenins facilitate a variety of associations. Perhaps most notably, certain ARM repeats enable the binding of catenins to classical cadherins and are one of the sites of interactions with small Rho GTPases (Yanagisawa et al., 2008). Such interactions help the p120-subfamily of catenins to localize properly and to modulate the cytoskeleton, as well as enable additional functions such as stabilizing cadherin-catenin complexes at adherens junctions, participating in intracellular transport, and regulating cell polarity (Anastasiadis and Reynolds, 2000; Laura et al., 2002; Hatzfeld, 2005; McCrea and Park, 2007; Baumert et al., 2020).
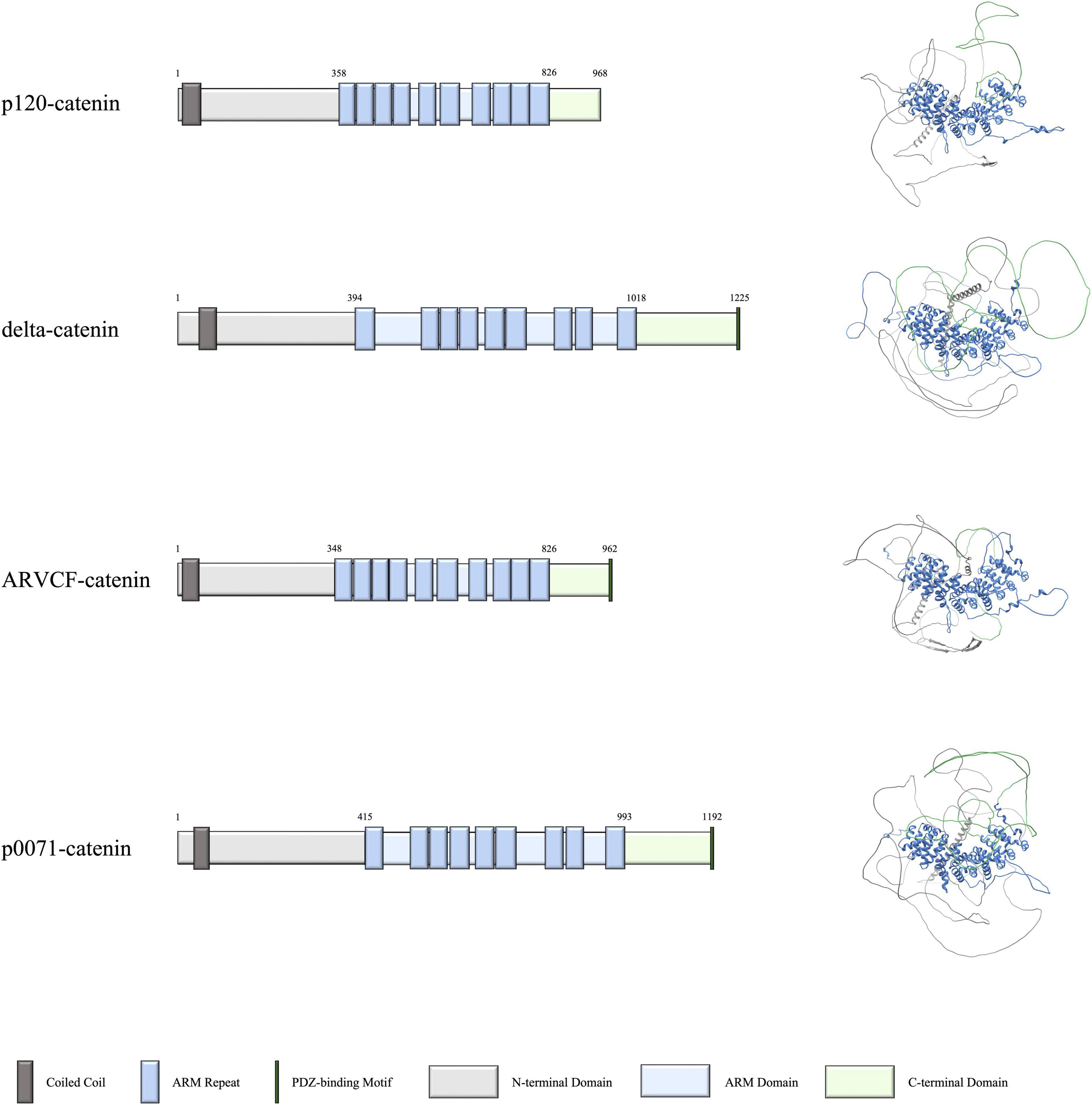
Figure 1. Domains and structures of human p120-catenin subfamily proteins. Schematics on the left depict the domains of p120-catenin, delta-catenin, ARVCF-catenin, and p0071-catenin, with the corresponding predicted structure of each protein on the right. Data for domain schematics was obtained from UniProt, and models were made using AlphaFold (The UniProt Consortium, 2018; Jumper et al., 2021).
While p120-catenin, ARVCF-catenin, and p0071-catenin are widely distributed throughout mammalian tissues, including the central nervous system, delta-catenin expression is primarily restricted to the central nervous system (Hatzfeld and Nachtsheim, 1996; Paffenholz and Franke, 1997; Sirotkin et al., 1997; Keirsebilck et al., 1998; Kawamura et al., 1999; Paulson et al., 2000; Duparc et al., 2006; Munton et al., 2007; Huttlin et al., 2010; Erickson et al., 2015; Foldy et al., 2016; Lu et al., 2016). Furthermore, p120-catenin and delta-catenin are also widely expressed at the protein level in the mammalian brain during early development. While studies have not yet shown the relative extent to which ARVCF-catenin and p0071-catenin are present at the protein level in early development, they are each present at the RNA level (Chauvet et al., 2003; Yuan and Arikkath, 2017). Within neurons, p120-catenin has been shown to localize to growth cones as well as presynaptic and postsynaptic terminals (Chauvet et al., 2003). Delta-catenin has been shown to localize to growth cones, postsynaptic terminals, and dendritic segments (Martinez et al., 2003; Arikkath et al., 2008; Yuan et al., 2015; Baumert et al., 2020). In contrast, the subcellular localization of ARVCF-catenin and p0071-catenin in neurons has not yet been investigated.
p120-catenin and delta-catenin have been found to regulate dendrite morphology in neurons through the cadherin-catenin complex and by modulating small Rho GTPase activity (Elia et al., 2006; Arikkath et al., 2008; Kim et al., 2008; Edbauer et al., 2009; Donta et al., 2022). Depletion of p120-catenin and delta-catenin each result in decreased dendritic length and branching, while increasing delta-catenin expression increased dendritic length and branching in neurons (Elia et al., 2006; Arikkath et al., 2008; Kim et al., 2008; Edbauer et al., 2009; Baumert et al., 2020). To date, fewer studies on ARVCF-catenin and p0071-catenin in neurons have been done but they have each been shown to modulate the cytoskeleton through the cadherin-catenin complex and by modulating small Rho GTPase activity in other cell types (Fang et al., 2004; Keil et al., 2013). While the effects of delta-catenin and p120-catenin on dendrite morphology have been partially characterized, we aimed for the first time to elucidate the roles ARVCF-catenin and p0071-catenin’s effects on dendrite morphogenesis during development, and also to directly compare phenotypic differences with those involving delta-catenin and p120-catenin. Indeed, even with some larger similarities in structure and function of the p120-catenin subfamily members, direct comparisons within the same primary neuronal culture model provide an opportunity to evaluate the relative protein distributions, and importantly, the gross morphologic roles of each subfamily member.
Results
p120-subfamily catenins exhibit unique distribution patterns in developing neurons
Given that there has not yet been a comprehensive comparative study of the p120-subfamily catenins in neurons, we began by examining the cellular localization patterns of p120-catenin, delta-catenin, ARVCF-catenin, and p0071-catenin. While RNA levels of each catenin in the brain are known, our aim was to determine subcellular protein expression. To conduct this study, primary rat hippocampal neurons were fixed at 1 DIV (day in vitro) and 5 DIV. The timepoint of 1 DIV was chosen to best visualize growth cones, while 5 DIV was chosen to better visualize dendritic processes. Using general criteria (dendrites, soma, nucleus, growth cones, etc.), antibodies specifically directed against the endogenous catenins were used to visualize and quantify the localization pattern of each p120-subfamily catenin (Figure 2). Since each antibody employed has a different inherent sensitivity to detecting its target catenin protein, we focused upon comparing the relative subcellular distributions within neurons of each catenin protein (not reporting on absolute levels). Each antibody employed was validated through shRNA-mediated knockdown and consequent loss of immunofluorescence staining (Supplementary Figure 1).
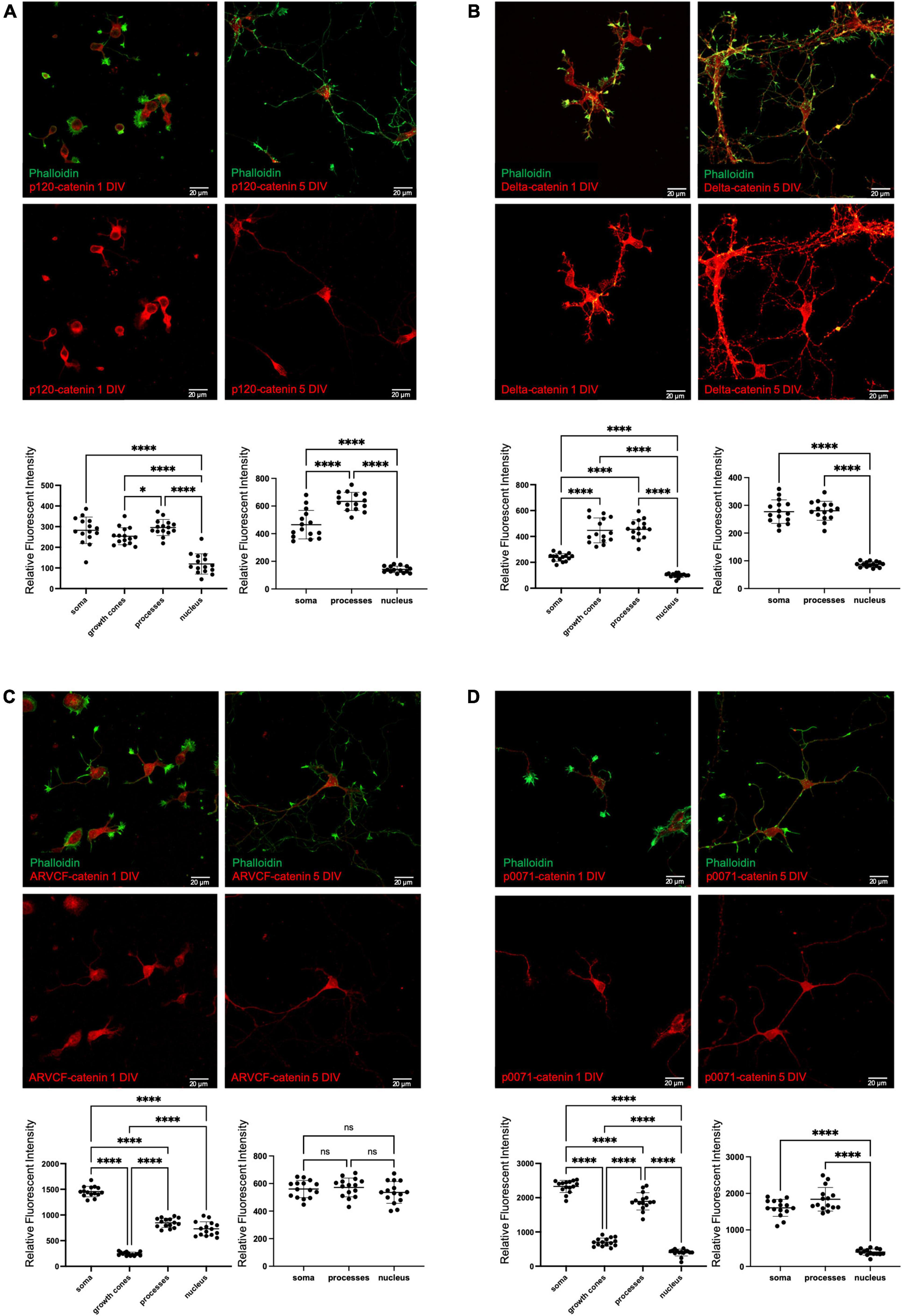
Figure 2. Endogenous localization of p120-subfamily catenins in primary neurons. Endogenous localization of (A) p120-catenin; (B) delta-catenin; (C) ARVCF-catenin; (D) p0071-catenin in primary rat hippocampal neurons at 1 DIV and 5 DIV. Relative fluorescent intensity in the soma growth cones, processes, and nucleus is shown below each example image for 1 DIV (left) and 5 DIV (right). Cells were co-stained with Phalloidin and DAPI to help visualize growth cones and nuclei for quantification, but for clarity these stains are not pictured in example images. Statistical significance was determined using a two-way Brown-Forsythe and Welch ANOVA tests with Dunnett’s T3 multiple comparisons post-hoc test. Significance was assigned at P < 0.05 for *, and P < 0.0001 for ****, and ns for non-significant. N = 15 neurons from three biological replicates for each catenin at each DIV. Graph values show mean and standard deviation.
At 1 DIV, p120-catenin is present at similar levels in the dendritic processes and soma, followed by the growth cones, and has relatively low presence in the nucleus. At 5 DIV, p120-catenin is most highly present in the processes, followed by the soma, and remains at relatively low levels in the nucleus (Figure 2A), which is consistent with previous studies (Elia et al., 2006). Delta-catenin localizes most to dendritic processes and growth cones, followed by the soma, then the nucleus at 1 DIV (Figure 2B). At 5 DIV, delta-catenin is present at similar higher relative levels in the processes and soma than in the nucleus (Figure 2B). Delta-catenin’s presence in growth cones, dendritic processes, cytoplasm, and cell membrane are consistent with previous findings from us and others (Martinez et al., 2003; Arikkath et al., 2008; Yuan et al., 2015; Baumert et al., 2020). ARVCF-catenin is most present in the soma at 1 DIV, followed by the processes and nucleus, and is least present in the growth cones (Figure 2C). However, at 5 DIV, ARVCF-catenin is about equally distributed throughout the soma, processes, and nucleus (Figure 2C). Finally, at 1 DIV, p0071-catenin is most present in the soma and processes, considerably less present in the growth cones, and even less present in the nucleus (Figure 2D). Similarly, at 5 DIV, p0071-catenin is more present in the soma and processes than the nucleus (Figure 2D).
p120-subfamily catenins exhibit both shared and distinct effects on dendrite morphology
We sought to determine how altering the expression levels of each p120-subfamily catenin affects dendrite morphology. To do this, we overexpressed versus knocked down each p120-catenin subfamily member in primary rat hippocampal neurons, and we then analyzed the resulting impact upon dendrite morphology. These neurons came from mixed cultures that included all regions in the hippocampus. Neuron-specific expression of each construct was achieved using the hSyn (synapsin 1) promoter so that we only scored neurons (Shevtsova et al., 2005). Furthermore, overexpression constructs contained a GFP reporter that was detached from the rest of the open reading frame via a P2A ribosomal skipping sequence. This was done to produce cell fills of GFP of the transfected neurons to visualize their entire morphology while marking the specific neurons expressing the protein of interest. For morphologic analysis, we utilized the Imaris Filament Tracer software, an example of the workflow is shown in Supplementary Figure 2.
We determined that increasing p120-catenin levels significantly increases dendritic length and branching, while decreasing p120-catenin levels decreases dendritic length and branching (Figure 3). Like p120-catenin, increasing delta-catenin levels increases dendritic length and branching, while decreasing levels decreases dendritic length and branching (Figure 4). Interestingly, we found that increasing the levels of ARVCF-catenin increases dendritic length and branching, but decreasing ARVCF-catenin levels has no effect on dendrite morphology (Figure 5). While p0071-catenin knockdown resulted in decreased dendritic length and branching, we found that overexpressing p0071-catenin increased dendritic branching but did not significantly increase total dendritic length (Figure 6). The effects of each p120-subfamily catenin on dendrite length and branching are shown in Table 1.
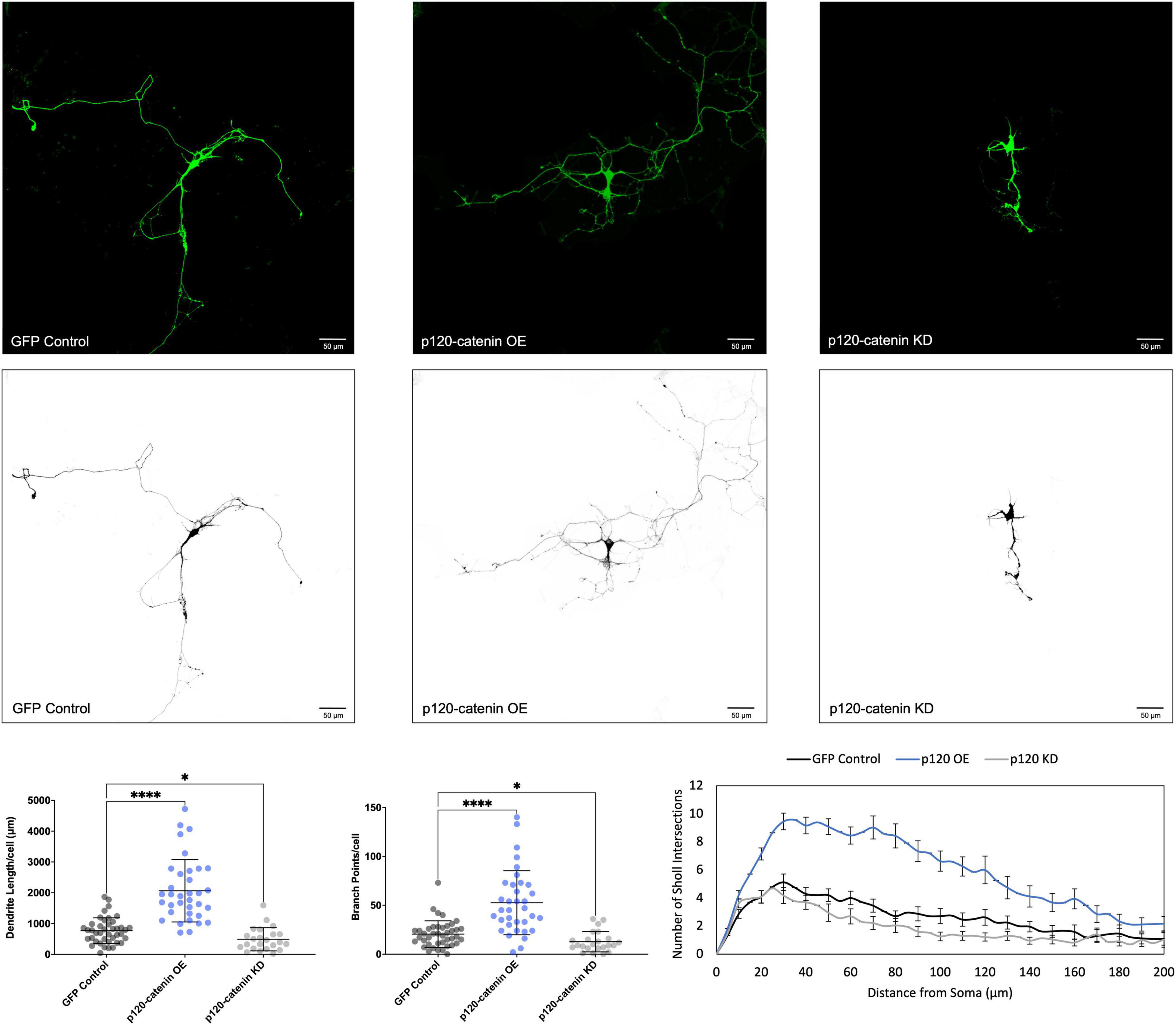
Figure 3. Morphological effects of p120-catenin expression in developing rat primary hippocampal neurons. Example images are shown of neurons that have been transfected with GFP, exogenous p120-catenin, and shRNA to knockdown p120-catenin. Cells were transfected at 3 DIV and analyzed at 7 DIV. These images have been masked to eliminate other cells in the visual field for clarity. Quantification of the average dendrite length per cell and average number of branch points per cell per each condition are shown below on the left, and Sholl analysis for each condition to the right. The number of cells analyzed in each condition were 35 for p120-catenin OE, 23 for p120-catenin KD, and 41 for GFP control. Samples were taken from five biological replicates. Statistical significance was determined using a two-way Brown-Forsythe and Welch ANOVA tests with Dunnett’s T3 multiple comparisons post-hoc test. Significance was assigned at P < 0.05 for * and P < 0.0001 for ****. Chart values show mean and standard deviation.
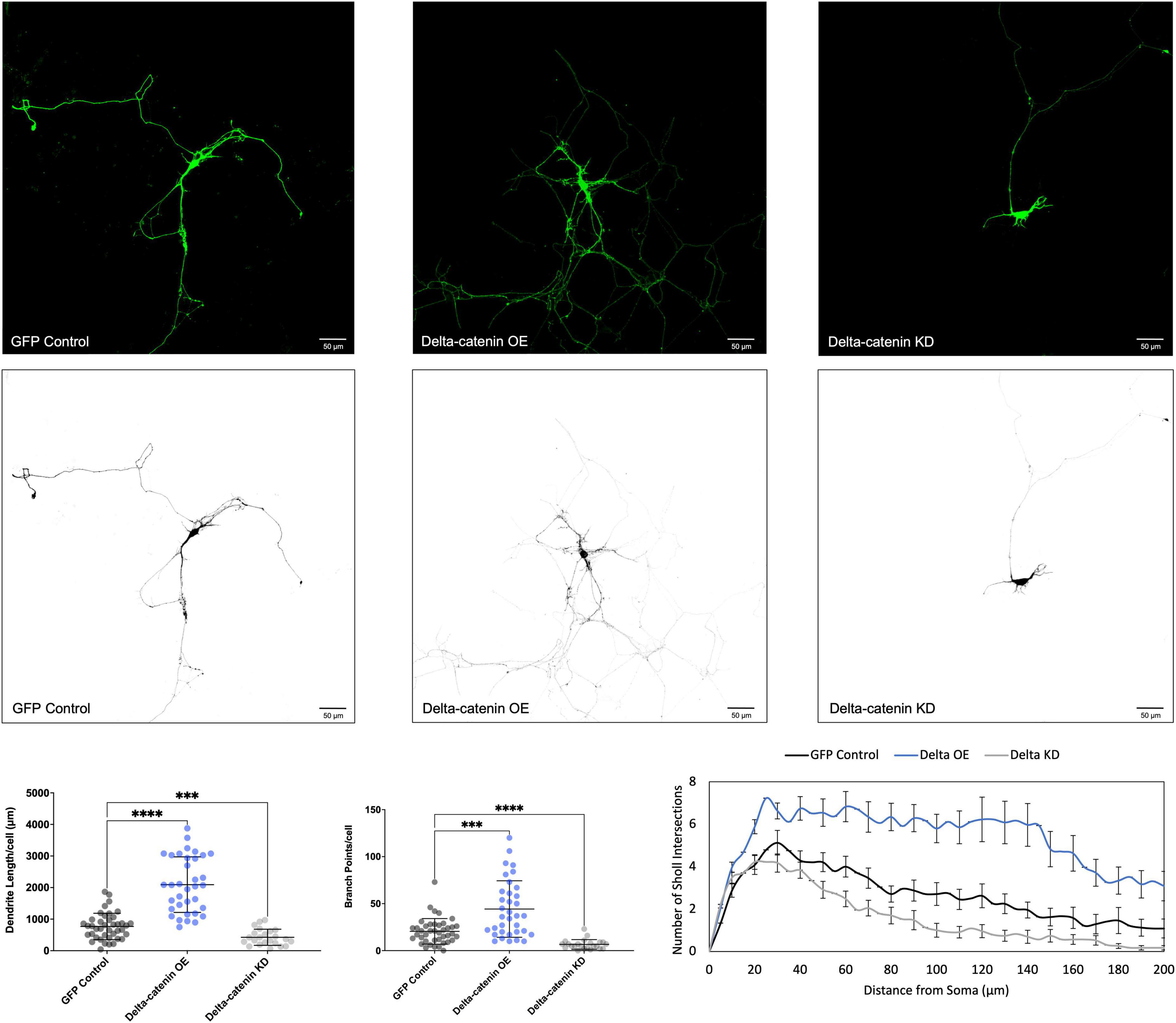
Figure 4. Morphological effects of delta-catenin expression in developing rat primary hippocampal neurons. Example images are shown of neurons that have been transfected with GFP, exogenous delta-catenin, and shRNA to knockdown delta-catenin. Cells were transfected at 3 DIV and analyzed at 7 DIV. These images have been masked to eliminate other cells in the visual field for clarity. Quantification of the average dendrite length per cell and average number of branch points per cell per each condition are shown below on the left, and Sholl analysis for each condition to the right. The number of cells analyzed in each condition were 35 for delta-catenin OE, 23 for delta-catenin KD, and 41 for GFP control. Samples were taken from five biological replicates. Statistical significance was determined using a two-way Brown-Forsythe and Welch ANOVA tests with Dunnett’s T3 multiple comparisons post-hoc test. Significance was assigned at P < 0.001 for *** and P < 0.0001 for ****. Chart values show mean and standard deviation.
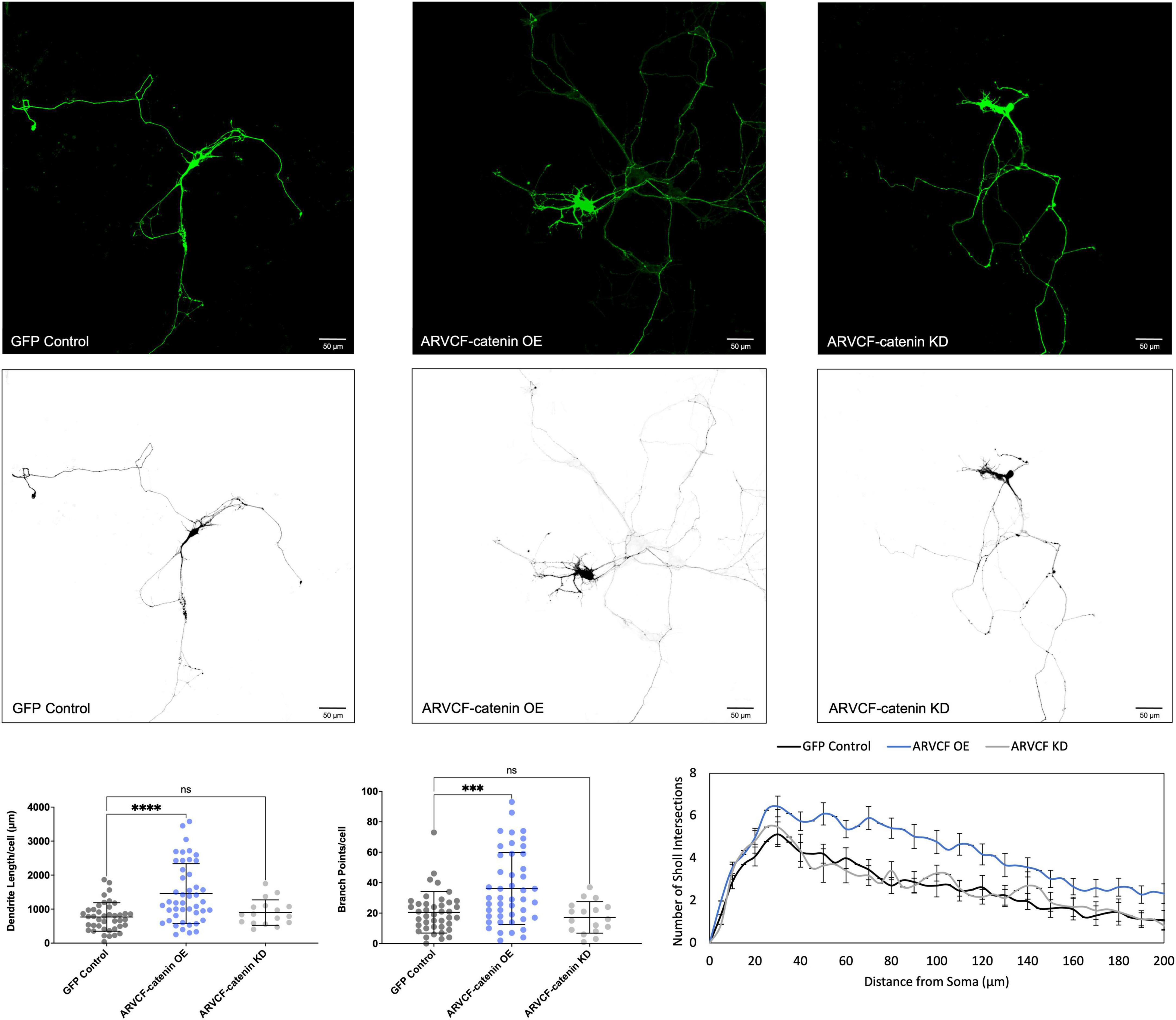
Figure 5. Morphological effects of ARVCF-catenin expression in developing rat primary hippocampal neurons. Example images are shown of neurons that have been transfected with GFP, exogenous ARVCF-catenin, and shRNA to knockdown ARVCF-catenin. Cells were transfected at 3 DIV and analyzed at 7 DIV. These images have been masked to eliminate other cells in the visual field for clarity. Quantification of the average dendrite length per cell and average number of branch points per cell per each condition are shown below on the left, and Sholl analysis for each condition to the right. The number of cells analyzed in each condition were 46 for ARVCF-catenin OE, 17 for ARVCF-catenin KD, and 41 for GFP control. Samples were taken from five biological replicates. Statistical significance was determined using a two-way Brown-Forsythe and Welch ANOVA tests with Dunnett’s T3 multiple comparisons post-hoc test. Significance was assigned at P < 0.001 for ***, P < 0.0001 for ****, and ns for non-significant. Chart values show mean and standard deviation.
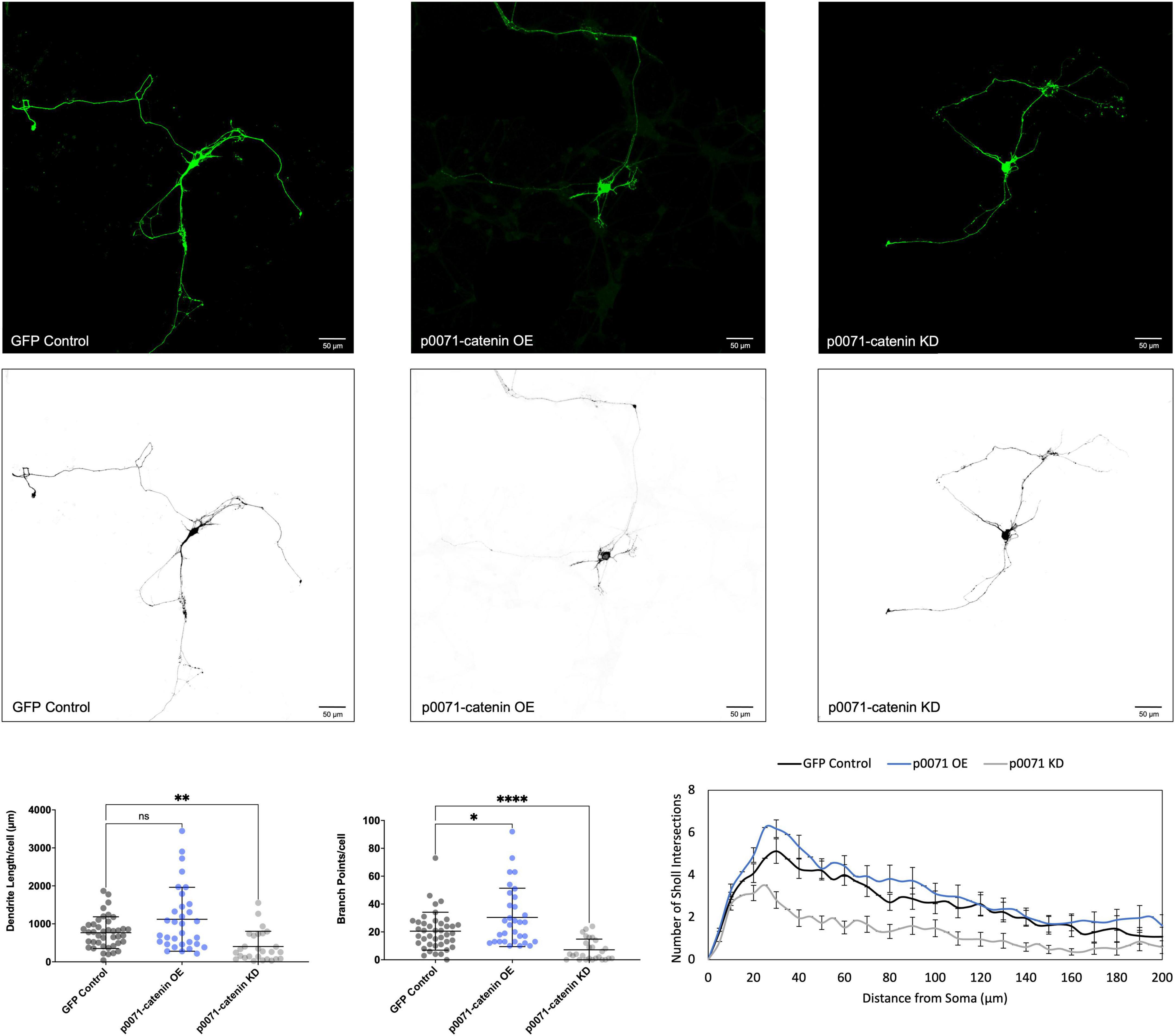
Figure 6. Morphological effects of p0071-catenin expression in developing rat primary hippocampal neurons. Example images are shown of neurons that have been transfected with GFP, exogenous p0071-catenin, and shRNA to knockdown p0071-catenin. Cells were transfected at 3 DIV and analyzed at 7 DIV. These images have been masked to eliminate other cells in the visual field for clarity. Quantification of the average dendrite length per cell and average number of branch points per cell per each condition are shown below on the left, and Sholl analysis for each condition to the right. The number of cells analyzed in each condition were 32 for p0071-catenin OE, 27 for p0071-catenin KD, and 41 for GFP control. Samples were taken from five biological replicates. Statistical significance was determined using a two-way Brown-Forsythe and Welch ANOVA tests with Dunnett’s T3 multiple comparisons post-hoc test. Significance was assigned at P < 0.05 for *, P < 0.01 for **, P < 0.0001 for ****, and ns for non-significant. Chart values show mean and standard deviation.
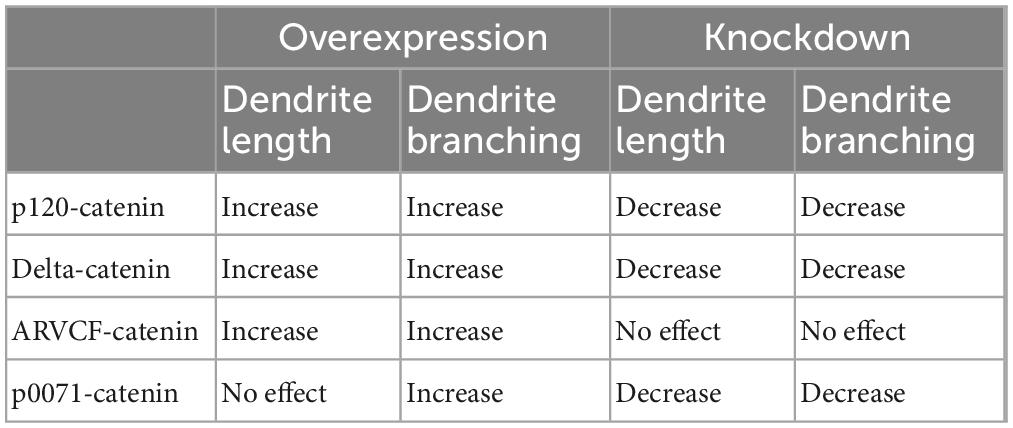
Table 1. Summary of p120-catenin subfamily expression effects on dendrite length and branching in rat primary hippocampal neurons.
Discussion
While we observe that each catenin is present in neuronal dendritic growth cones to some degree, a relatively larger proportion of p120-catenin and delta-catenin locate to growth cones compared to ARVCF-catenin and p0071-catenin. As growth cones are sites of neurite outgrowth, p120-catenin and delta-catenin may play a relatively more substantial role in dendritic morphogenesis than ARVCF-catenin and p0071-catenin (Halpain et al., 2009; Raper, 2009). All four catenins are present in dendritic processes, which may suggest that they all play roles in dendrite morphogenesis and/or maintenance. All four p120-subfamily catenins have some degree of localization to the nucleus, which is consistent with studies done in other cell types (McCrea et al., 2015; McCrea and Gottardi, 2016). However, their individual roles in regulating neuronal gene transcription have not been assessed. ARVCF-catenin clearly has the highest proportion of subcellular localization in the nucleus compared to the other three subfamily members. ARVCF-catenin, along with p120-catenin, has been shown to localize highly to the nucleus in non-neuronal cell types as well, but little is known about their potential roles in the nucleus (Daniel and Reynolds, 1999; Mariner et al., 2000; Kelly et al., 2004; Lee et al., 2014). None of the four p120-subfamily catenins have predicted DNA binding domains, but p120-catenin and delta-catenin have been shown to regulate transcription through other effectors, albeit in non-neuronal cell types (Daniel and Reynolds, 1999; Kelly et al., 2004; Rodova et al., 2004; Park et al., 2006; Hosking et al., 2007; Kim et al., 2008; Hong et al., 2010; Gu et al., 2011; Lee et al., 2014; Nopparat et al., 2015; Kulikov et al., 2016). Thus, it is currently unknown if the presence of any p120-subfamily catenin in the nucleus may be contributory to dendritic arborization.
The effects of p120-catenin knockdown on dendrite morphology align with previous work, which showed that p120-catenin knockdown decreases the dendritic arbor in CA1 primary hippocampal neurons from p120-catenin knockout mice in vitro (Elia et al., 2006). Our results showing that p120-catenin overexpression increases dendritic arborization are complimentary, as the impacts are the converse effect of p120-catenin knockdown. The neuronal phenotype corresponding to p120-catenin knockdown is thought to be primarily caused by p120-catenin’s interactions with cadherins and the small Rho GTPases RhoA and Rac1 (Elia et al., 2006). p120-catenin has been shown to inhibit RhoA and activate Rac1 in multiple cell types, including neurons (Anastasiadis et al., 2000; Noren et al., 2000; Grosheva et al., 2001; Elia et al., 2006). RhoA inhibition and Rac1 activation were established to increase dendritic arborization (Nakayama et al., 2000; Tashiro et al., 2000; Tashiro and Yuste, 2004; Guiler et al., 2021; Donta et al., 2022). Thus, our results are consistent with the hypothesis that p120-catenin expression increases dendritic arborization by inhibiting RhoA and activating Rac1, with the converse occurring upon p120-catenin depletion. Based on these depletion studies, it does not appear that p120-catenin depletion is compensated for by any other p120-subfamily catenin.
Morphological changes due to delta-catenin overexpression and knockdown are consistent with previous studies. There are multiple plausible mechanisms of how delta-catenin influences the dendritic arbor (Donta et al., 2022). Delta-catenin has been shown to inhibit RhoA and activate Rac1 (Martinez et al., 2003; Abu-Elneel et al., 2008; Kim et al., 2008; Gu et al., 2009; Gilbert and Man, 2016). Furthermore, delta-catenin is also thought to modulate dendrite morphology through its PDZ-binding motif, which p120-catenin lacks (Yuan et al., 2015). Interactions of delta-catenin’s PDZ-binding motif have also been shown to be phospho-dependent in certain cases, where the phosphorylation state of delta-catenin’s PDZ-binding motif dictates whether delta-catenin promotes dendritic length versus elongation (Baumert et al., 2020). Finally, delta-catenin was suggested to regulate dendrite morphogenesis by interactions with effectors like cortactin via delta-catenin’s C-terminal region (Martinez et al., 2003; Toyo-oka et al., 2014; Lee et al., 2015). While our results indicating that delta-catenin expression levels have a notable impact upon dendritic arborization are consistent with previous work, additional studies will be needed to reveal the exact mechanism(s) of action. Analogous to the morphological effects evident following the depletion of p120-catenin, those that relate to the knockdown of delta-catenin do not appear to be compensated for by delta’s remaining p120 sub-family members.
Although investigations of ARVCF-catenin in neurons are limited–particularly concerning its influence on dendrite morphology–studies in other cell types have characterized some of its roles in modulating the cytoskeleton. In X. laevis, ARVCF-catenin was shown to inhibit RhoA and activate Rac1 in a similar manner to p120-catenin (Fang et al., 2004; Valls et al., 2012). If ARVCF-catenin has the same effect on RhoA and Rac1 in neurons, this would lead to increased arborization upon overexpression, which is consistent with our results (Govek et al., 2005; Stankiewicz and Linseman, 2014). Studies in X. laevis and NIH 3T3 cells have also shown that p120-catenin and ARVCF-catenin can rescue each other’s depletions, with partial functional redundancy being a potential explanation for why ARVCF-catenin knockdown in neurons does not result in an altered phenotype (Mariner et al., 2000; Fang et al., 2004). p120-catenin is present in relatively higher protein levels compared to ARVCF-catenin in mammalian brain tissue (Mariner et al., 2000). In our current context of hippocampal neurons, this suggests the possibility that endogenous p120-catenin may largely rescue any gross morphological impact upon ARVCF-catenin depletion, but not the other way around. Furthermore, ARVCF-catenin contains a PDZ-binding motif as does delta-catenin so it perhaps share additionally interactions and thus functionality with delta-catenin and PDZ domain-containing proteins in regulating dendrite morphogenesis, though this has yet to be tested in neurons.
Studies centered on p0071-catenin in neurons are also limited, but our results are consistent with one study that found that p0071-catenin overexpression increased dendritic branching (Nolze et al., 2013). In non-neuronal tissues, p0071-catenin activates RhoA, which differs from p120-catenin, delta-catenin, and ARVCF-catenin (Wolf et al., 2006; Keil et al., 2009, 2013). As interactions with small Rho GTPases are thought to be a major pathway for how p120-subfamily catenins modulate the cytoskeleton, we would expect p0071-catenin to have the opposite morphological effects as the other three catenins. However, there are a few possibilities as to why we did not see such results. p0071-catenin’s interactions with small Rho GTPases could be tissue- and cell-type dependent, so studies showing that p0071-catenin activates RhoA in non-neuronal cell types may not hold true in neurons. Furthermore, as seen for delta-catenin, p0071-catenin may have multiple mechanisms of modulating the cytoskeleton (Elia et al., 2006; Kim et al., 2008). There may be an interplay between p0071-catenin’s interactions with small Rho GTPases and its functional association with other binding partners. This may also explain why p0071-catenin expression increases the number of branch points, but not the total dendritic length of neurons as is seen with p120-catenin, delta-catenin, and ARVCF-catenin. p0071-catenin depletion does not appear to be compensated for by any other p120-subfamily catenin.
Altering p120-subfamily expression in neurons increased sample variance within conditions (i.e., sample variance was relatively higher when altering p120-subfamily expression compared to the GFP control). This is likely due to the inclusion of multiple types of neurons in the cultures. These primary cultures originating from the rat hippocampus contained neurons from the CA1, CA2, CA3 regions, the dentate gyrus, and the subiculum complex (Lee et al., 2017). Therefore, it is possible that altering the expression of these catenins produces varied effects upon the distinct types of neurons present. Further, while our study focuses on the gross morphologies of dendrites, axons were not eliminated from analysis. This was due to the required high-density plating of neuronal cultures which generates extensive overlap of processes, raising difficulties in our ability to distinguish dendrites from axons using stains recognizing MAP2 and Tau. That considered, due to the multiplicity of dendrites per neuron (relative to the presence of a single axon), the predominance of observed morphological changes seen after altering expression of p120-subfamily members derives from effects upon dendrites.
Conclusion
Overall, the relative localization patterns of the p120-catenin subfamily are consistent with the possibility that they each play roles in dendritic arborization and maintenance, with p120-catenin and delta-catenin potentially playing a relatively stronger role in dendrite morphogenesis than ARVCF-catenin and p0071-catenin based on their relative abundance in growth cones. Furthermore, our morphologic analysis suggests that these catenins contain some shared and some distinct effects on dendrite shaping during neuronal development. The effects of the p120-subfamily catenins on dendrite morphology are likely due in part to their interactions with small Rho GTPases. However, further studies are needed to determine if the effects of each catenin are primarily caused by modulating small Rho GTPase activity, other effector molecules, or a combination of both. Finally, as abnormal dendrite morphology is seen in multiple neurological disorders like Alzheimer’s disease, schizophrenia, and autism spectrum disorder, determining the mechanisms of how each catenin modulates dendrite morphology may help point to new therapeutic approaches or considerations for these diseases. Interestingly, p120-subfamily catenins, most prominently delta, have associations with pathological states involving neurons and brain functions (Xie et al., 2005; Nolze et al., 2013; Donta et al., 2022).
Materials and methods
Cell culture and transfections
Primary hippocampal neurons were isolated from rat embryos at E18 as previously described (Baumert et al., 2020). All protocols involving vertebrate animals were approved by the Institutional Animal Care and Use Committee prior to initiating the studies. Once isolated, the hippocampal neurons were plated in 24-well tissue-culture plates with coverslips coated with 100 μg/mL poly-D-lysine at a density of 2 × 105 cells per well. The cultures were maintained in Neurobasal Medium supplemented with B-27, GlutaMAX, and penicillin-streptomycin. Transfections in neurons were done at 3 DIV using Lipofectamine 2000 according to the manufacturer’s protocol.
Immunocytochemistry
Neurons were fixed with 4% paraformaldehyde for 10 min at room temperature, incubated for 5 min in PBS supplemented with 100 mM glycine, washed with PBS for 30 min, then permeabilized with 0.5% Triton X-100 for 10 min at room temperature. The neurons were then blocked with PBS containing 1% bovine serum albumin overnight at 4°C. After blocking, the neurons were incubated with primary antibodies in blocking solution overnight at 4°C. The neurons were washed with PBS 3 times, then incubated with secondary antibodies for 1 h at room temperature. Following a second washing step of 3 washes at 5 min each, coverslips were mounted onto slides with 2 μL of VECTASHIELD Antifade Mounting Medium.
Confocal microscopy
Neurons were imaged using a Nikon T2i Inverted Confocal Microscope with a 20× Plan Achromat 0.75 NA lens for morphology studies and an Apo-Plan 60 × 1.4 NA oil objective for endogenous localization studies. Images were captured with a Nikon A1-DUG GaAsP hybrid four-channel multi-detector and Nikon NIS-Elements software. All z-series images were acquired at a pixel size of 100 nm and a step size of 1.1 μm at 20× and 0.2 μm at 60×. Excitation was done with a 488 nm laser line and emission was collected with a BP 525/50 m filter. The pinhole was set at 1 airy unit for all images. For imaging endogenous localization of catenins, the laser intensity, offset, and high voltage settings on the microscope were kept consistent between images of the same condition. All images were acquired below the threshold for pixel saturation. For morphological analysis of images acquired at 20×, montages of at least 5 × 5 tiles were captured and stitched together to visualize larger fields of view.
Antibodies
p120-catenin antibody (Invitrogen, Cat. #33-9600) was used at 1:250 dilution. Delta-catenin antibody (BD Technologies, Cat. #61137) was used at 1:250 dilution. ARVCF-catenin antibody (Santa Cruz Biotechnology, Cat. #sc-23874) was used at 1:100 dilution. p0071-catenin antibody (Invitrogen, Cat. #PA5-101752) was used at 1:250 dilution. For secondary antibodies, Alexa Fluor 488 (Cat. #A-11001, #A-11008) was used at 1:1,000 dilution, Alexa Fluor 555 (Cat. #A32732) was used at 1:1,000 dilution, and Alexa Fluor 568 (Cat. #A-11004) was used at 1:1,000 dilution.
Plasmids
All cDNA transfections for overexpression analyses utilized an AAV vector, an eGFP reporter, an hSyn promoter, and a P2A ribosomal skipping sequence to separate the exogenous catenin from the eGFP reporter to visualize the neurons’ full morphology. All shRNA-mediated knockdowns utilized a pSUPER vector with an eGFP reporter. The GFP controls contained only the vectors and eGFP. Overexpression plasmids were transfected with Lipofectamine 2000 according to the manufacturer’s protocol at 0.5 μg/well, and knockdown plasmids were transfected at 0.25 μg/well. The human forms of each catenin were used for exogenous expression plasmids and are as follows: (CTNND1, Gene ID:1500; CTNND2, Gene ID: 1501; ARVCF, Gene ID:421; PKP4, Gene ID:850). The sequences for the shRNA targeting each catenin are as follows: p120-catenin, 5′-CGATATTGGTAGGATTGTT-3′; delta-catenin, 5′-TAGAAGTCGACATAGTTGC-3′; ARVCF-catenin, 5′-CGAGTTAGCTATTTACTGA-3′; p0071-catenin, 5′-GGACTGTTCATGACATGGA-3′.
Image analysis
After capturing confocal images in the manner described above, several analyses were performed on the neurons. Endogenous localization was quantified using ImageJ by measuring the relative average fluorescent intensity of each catenin compared to itself in the nucleus, soma, processes, and growth cones. These measurements were obtained by going through each slice of the image, creating regions of interest for the four different subcellular regions, and using ImageJ to measure the average fluorescent intensity of each region. This process was repeated until all slices of the image were measured. The values presented for each cell are the average value for each slice to account for differences in thickness. Cells were co-stained with DAPI and phalloidin to determine subcellular regions. Measurements were averaged from 15 neurons for each condition from three separate cultures for endogenous localization studies. Fluorescent intensity is to be compared between subcellular localizations for the same catenin but is not to be compared between different catenins. For morphological analysis, cells were transfected at 3 DIV and analyzed at 7 DIV. Imaris Filament Tracer was used to create a skeleton of each neuron. From this tracing, the software automatically measures dendrite length, dendrite branching, and performs Sholl analysis. The radii interval for sholl analysis was set at 5 μm. Laser intensity and detector settings were kept the same between samples while collecting images, and all threshold settings were kept the same between samples when running the Imaris Filament Tracer software. Processes shorter than 5 μm were excluded from the analysis. Sample sizes were between 17 and 46 neurons from 5 biological replicates for each condition.
Antibody and shRNA validation
To validate antibodies and shRNA knockdowns, primary rat hippocampal neurons were transfected with shRNA to knockdown each p120-subfamily catenin at 3 DIV, then were fixed at 7 DIV. Samples were incubated with antibodies targeting the respective endogenous catenin, then imaged. Fiji was used to measure the fluorescent intensity of endogenous catenin in cells that were transfected with shRNA and were compared against cells that were transfected with GFP as a control.
Statistical analysis
Data were analyzed using GraphPad Prism. Statistical significance for all endogenous localization study and morphological analysis comparisons were determined using a two-way Brown-Forsythe and Welch ANOVA tests with Dunnett’s T3 multiple comparisons post-hoc test. Statistical significance for antibody and shRNA knockdown validation was determined using unpaired t-tests with Welch’s correction. Significance was assigned at P < 0.05 for *, P < 0.01 for **, P < 0.001 for ***, and P < 0.0001 for ****.
Data availability statement
The original contributions presented in this study are included in the article/Supplementary material, further inquiries can be directed to the corresponding authors.
Ethics statement
The animal study was reviewed and approved by the Institutional Animal Care and Use Committee.
Author contributions
MD: concept of study, research design, data collection and analysis, and manuscript preparation. YS: data collection and protein modeling. CD: hippocampal culture preparation and experimentation. AP-H: experimentation, imaging, and analysis. MW: concept of study, research design, and manuscript review and editing. PM: concept of study, research design, manuscript preparation, and review and editing. All authors contributed to the article and approved the submitted version.
Funding
This work was supported by 1RO1MH115717 (PM and MW). PM also acknowledges an Ashbel Smith Professorship Award, and MW an endowment from the William Wheless III Professorship. Use of the A1-Nikon (confocal images) was possible due to the UT MDACC Department of Genetics NIH Instrumentation Grant 1S10OD024976-01 (AP-H). DNA sequencing was provided from National Cancer Institute Core Grant CA-16672 to UT MDACC.
Acknowledgments
We thank Malgosia Kloc, Jae-Il Park, Rachel Miller, Mark Bedford, George Eisenhoffer, Michael Galko, Ryan Baumert, and Mark Corkins for helpful discussion. We also thank Leslie Thalmann and Lydia Mireles for their help with primary neuronal cultures.
Conflict of interest
The authors declare that the research was conducted in the absence of any commercial or financial relationships that could be construed as a potential conflict of interest.
Publisher’s note
All claims expressed in this article are solely those of the authors and do not necessarily represent those of their affiliated organizations, or those of the publisher, the editors and the reviewers. Any product that may be evaluated in this article, or claim that may be made by its manufacturer, is not guaranteed or endorsed by the publisher.
Supplementary material
The Supplementary Material for this article can be found online at: https://www.frontiersin.org/articles/10.3389/fncel.2023.1151249/full#supplementary-material
Supplementary Figure 1 | p120-catenin subfamily antibody validation via shRNA-mediated knockdown. Rat primary hippocampal neurons were transfected at 3 DIV with shRNA plasmids designed to knockdown p120-catenin, delta-catenin, ARVCF-catenin, and p0071-catenin, then fixed at 7 DIV. Green cells in the images are neurons that have been transfected with shRNA vectors corresponding to the labeled catenin on the image, and magenta cells depict the respective endogenous p120-subfamily catenin antibody. For each catenin, the image on the left includes the neuron(s) that has been transfected with shRNA knockdown, and the image on the right shows the neurons without the transfected neuron(s). Neurons that have been transfected with shRNA knockdown have significantly lower staining intensity, indicating that the shRNA knockdown decreased the amount of catenin present. Dots in each bar graph indicate number of cells analyzed for each condition and average and standard deviations are indicated. **** represent P-values < 0.0001.
Supplementary Figure 2 | The Imaris Filament Tracer software used to create a tracing of a transfected neuron. The image on the left is a 7 DIV rat primary hippocampal neuron that has been transfected with exogenous ARVCF-catenin with an eGFP reporter (magenta). The MAP2 neuronal marker (cyan) was used to depict neuronal dendrites, and DAPI (yellow) was used to visualize the nucleus. The image on the right is the skeleton of the transfected neuron produced by the Imaris Filament Tracer software. The tracings are used to quantify dendrite length and branching, as well as to perform Scholl analysis.
References
Abu-Elneel, K., Ochiishi, T., Medina, M., Remedi, M., Gastaldi, L., Caceres, A., et al. (2008). A delta-catenin signaling pathway leading to dendritic protrusions. J. Biol. Chem. 283, 32781–32791. doi: 10.1074/jbc.M804688200
Anastasiadis, P. Z., and Reynolds, A. B. (2000). The p120 catenin family: complex roles in adhesion, signaling and cancer. J. Cell Sci. 113(Pt. 8), 1319–1334. doi: 10.1242/jcs.113.8.1319
Anastasiadis, P. Z., Moon, S. Y., Thoreson, M. A., Mariner, D. J., Crawford, H. C., Zheng, Y., et al. (2000). Inhibition of RhoA by p120 catenin. Nat. Cell Biol. 2, 637–644. doi: 10.1038/35023588
Arikkath, J. (2012). Molecular mechanisms of dendrite morphogenesis. Front. Cell Neurosci. 6:61. doi: 10.3389/fncel.2012.00061
Arikkath, J., Israely, I., Tao, Y., Mei, L., Liu, X., and Reichardt, L. F. (2008). Erbin controls dendritic morphogenesis by regulating localization of delta-catenin. J. Neurosci. 28, 7047–7056. doi: 10.1523/JNEUROSCI.0451-08.2008
Baumert, R., Ji, H., Paulucci-Holthauzen, A., Wolfe, A., Sagum, C., Hodgson, L., et al. (2020). Novel phospho-switch function of delta-catenin in dendrite development. J. Cell Biol. 219, e201909166. doi: 10.1083/jcb.201909166
Charych, E. I., Akum, B. F., Goldberg, J. S., Jornsten, R. J., Rongo, C., Zheng, J. Q., et al. (2006). Activity-independent regulation of dendrite patterning by postsynaptic density protein PSD-95. J. Neurosci. 26, 10164–10176. doi: 10.1523/JNEUROSCI.2379-06.2006
Chauvet, N., Prieto, M., Fabre, C., Noren, N. K., and Privat, A. (2003). Distribution of p120 catenin during rat brain development: potential role in regulation of cadherin-mediated adhesion and actin cytoskeleton organization. Mol Cell Neurosci 22, 467–486. doi: 10.1016/s1044-7431(03)00030-7
Couch, B. A., DeMarco, G. J., Gourley, S. L., and Koleske, A. J. (2010). Increased dendrite branching in AbetaPP/PS1 mice and elongation of dendrite arbors by fasudil administration. J. Alzheimers Dis. 20, 1003–1008. doi: 10.3233/JAD-2010-091114
Daniel, J. M., and Reynolds, A. B. (1999). The catenin p120(ctn) interacts with Kaiso, a novel BTB/POZ domain zinc finger transcription factor. Mol. Cell Biol. 19, 3614–3623. doi: 10.1128/MCB.19.5.3614
de la Torre-Ubieta, L., and Bonni, A. (2011). Transcriptional regulation of neuronal polarity and morphogenesis in the mammalian brain. Neuron 72, 22–40. doi: 10.1016/j.neuron.2011.09.018
Donta, M. S., Srivastava, Y., and McCrea, P. D. (2022). Delta-catenin as a modulator of Rho GTPases in neurons. Front. Cell Neurosci. 16:939143. doi: 10.3389/fncel.2022.939143
Duparc, R. H., Boutemmine, D., Champagne, M. P., Tetreault, N., and Bernier, G. (2006). Pax6 is required for delta-catenin/neurojugin expression during retinal, cerebellar and cortical development in mice. Dev. Biol. 300, 647–655. doi: 10.1016/j.ydbio.2006.07.045
Edbauer, D., Cheng, D., Batterton, M. N., Wang, C. F., Duong, D. M., Yaffe, M. B., et al. (2009). Identification and characterization of neuronal mitogen-activated protein kinase substrates using a specific phosphomotif antibody. Mol. Cell Proteomics 8, 681–695. doi: 10.1074/mcp.M800233-MCP200
Elia, L. P., Yamamoto, M., Zang, K., and Reichardt, L. F. (2006). p120 catenin regulates dendritic spine and synapse development through Rho-family GTPases and cadherins. Neuron 51, 43–56. doi: 10.1016/j.neuron.2006.05.018
Elston, G. N. (2003). Cortex, cognition and the cell: new insights into the pyramidal neuron and prefrontal function. Cereb. Cortex 13, 1124–1138. doi: 10.1093/cercor/bhg093
Erickson, B. K., Jedrychowski, M. P., McAlister, G. C., Everley, R. A., Kunz, R., and Gygi, S. P. (2015). Evaluating multiplexed quantitative phosphopeptide analysis on a hybrid quadrupole mass filter/linear ion trap/orbitrap mass spectrometer. Anal. Chem. 87, 1241–1249. doi: 10.1021/ac503934f
Fang, X., Ji, H., Kim, S. W., Park, J. I., Vaught, T. G., Anastasiadis, P. Z., et al. (2004). Vertebrate development requires ARVCF and p120 catenins and their interplay with RhoA and Rac. J. Cell Biol. 165, 87–98. doi: 10.1083/jcb.200307109
Foldy, C., Darmanis, S., Aoto, J., Malenka, R. C., Quake, S. R., and Sudhof, T. C. (2016). Single-cell RNAseq reveals cell adhesion molecule profiles in electrophysiologically defined neurons. Proc. Natl. Acad. Sci. U.S.A. 113, E5222–E5231. doi: 10.1073/pnas.1610155113
Gilbert, J., and Man, H. Y. (2016). The X-linked autism protein KIAA2022/KIDLIA regulates neurite outgrowth via N-cadherin and delta-catenin signaling. eNeuro 3, ENEURO.238–ENEURO.216. doi: 10.1523/ENEURO.0238-16.2016
Govek, E. E., Newey, S. E., and Van Aelst, L. (2005). The role of the Rho GTPases in neuronal development. Genes Dev. 19, 1–49. doi: 10.1101/gad.1256405
Grosheva, I., Shtutman, M., Elbaum, M., and Bershadsky, A. D. (2001). p120 catenin affects cell motility via modulation of activity of Rho-family GTPases: a link between cell-cell contact formation and regulation of cell locomotion. J. Cell Sci. 114(Pt. 4), 695–707. doi: 10.1242/jcs.114.4.695
Gu, D., Sater, A. K., Ji, H., Cho, K., Clark, M., Stratton, S. A., et al. (2009). Xenopus delta-catenin is essential in early embryogenesis and is functionally linked to cadherins and small GTPases. J Cell Sci 122(Pt. 22), 4049–4061. doi: 10.1242/jcs.031948
Gu, D., Tonthat, N. K., Lee, M., Ji, H., Bhat, K. P., Hollingsworth, F., et al. (2011). Caspase-3 cleavage links delta-catenin to the novel nuclear protein ZIFCAT. J. Biol. Chem. 286, 23178–23188. doi: 10.1074/jbc.M110.167544
Guiler, W., Koehler, A., Boykin, C., and Lu, Q. (2021). Pharmacological modulators of small GTPases of Rho family in neurodegenerative diseases. Front. Cell Neurosci. 15:661612. doi: 10.3389/fncel.2021.661612
Halpain, S., Calabrese, B., and Dehmelt, L. (2009). “Actin cytoskeleton in growth cones, nerve terminals, and dendritic spines,” in Encyclopedia of neuroscience, ed. L. R. Squire (Cambridge: Academic Press), 15–21. doi: 10.1016/B978-008045046-9.00729-4
Hatzfeld, M. (2005). The p120 family of cell adhesion molecules. Eur. J. Cell Biol. 84, 205–214. doi: 10.1016/j.ejcb.2004.12.016
Hatzfeld, M., and Nachtsheim, C. (1996). Cloning and characterization of a new armadillo family member, p0071, associated with the junctional plaque: evidence for a subfamily of closely related proteins. J. Cell Sci. 109(Pt. 11), 2767–2778. doi: 10.1242/jcs.109.11.2767
Hong, J. Y., Park, J. I., Cho, K., Gu, D., Ji, H., Artandi, S. E., et al. (2010). Shared molecular mechanisms regulate multiple catenin proteins: canonical Wnt signals and components modulate p120-catenin isoform-1 and additional p120 subfamily members. J. Cell Sci. 123(Pt. 24), 4351–4365. doi: 10.1242/jcs.067199
Hosking, C. R., Ulloa, F., Hogan, C., Ferber, E. C., Figueroa, A., Gevaert, K., et al. (2007). The transcriptional repressor Glis2 is a novel binding partner for p120 catenin. Mol. Biol. Cell 18, 1918–1927. doi: 10.1091/mbc.e06-10-0941
Huang, Z., Zang, K., and Reichardt, L. F. (2005). The origin recognition core complex regulates dendrite and spine development in postmitotic neurons. J. Cell Biol. 170, 527–535. doi: 10.1083/jcb.200505075
Huttlin, E. L., Jedrychowski, M. P., Elias, J. E., Goswami, T., Rad, R., Beausoleil, S. A., et al. (2010). A tissue-specific atlas of mouse protein phosphorylation and expression. Cell 143, 1174–1189. doi: 10.1016/j.cell.2010.12.001
Jan, Y. N., and Jan, L. Y. (2010). Branching out: mechanisms of dendritic arborization. Nat. Rev. Neurosci. 11, 316–328. doi: 10.1038/nrn2836
Jumper, J., Evans, R., Pritzel, A., Green, T., Figurnov, M., Ronneberger, O., et al. (2021). Highly accurate protein structure prediction with AlphaFold. Nature 596, 583–589. doi: 10.1038/s41586-021-03819-2
Kaufmann, W. E., and Moser, H. W. (2000). Dendritic anomalies in disorders associated with mental retardation. Cereb. Cortex 10, 981–991. doi: 10.1093/cercor/10.10.981
Kawamura, Y., Fan, Q. W., Hayashi, H., Michikawa, M., Yanagisawa, K., and Komano, H. (1999). Expression of the mRNA for two isoforms of neural plakophilin-related arm-repeat protein/delta-catenin in rodent neurons and glial cells. Neurosci. Lett. 277, 185–188. doi: 10.1016/s0304-3940(99)00875-7
Keil, R., Kiessling, C., and Hatzfeld, M. (2009). Targeting of p0071 to the midbody depends on KIF3. J. Cell Sci. 122(Pt. 8), 1174–1183. doi: 10.1242/jcs.045377
Keil, R., Schulz, J., and Hatzfeld, M. (2013). p0071/PKP4, a multifunctional protein coordinating cell adhesion with cytoskeletal organization. Biol. Chem. 394, 1005–1017. doi: 10.1515/hsz-2013-0114
Keirsebilck, A., Bonne, S., Staes, K., van Hengel, J., Nollet, F., Reynolds, A., et al. (1998). Molecular cloning of the human p120ctn catenin gene (CTNND1): expression of multiple alternatively spliced isoforms. Genomics 50, 129–146. doi: 10.1006/geno.1998.5325
Kelly, K. F., Spring, C. M., Otchere, A. A., and Daniel, J. M. (2004). NLS-dependent nuclear localization of p120ctn is necessary to relieve Kaiso-mediated transcriptional repression. J. Cell Sci. 117(Pt. 13), 2675–2686. doi: 10.1242/jcs.01101
Kim, H., Han, J. R., Park, J., Oh, M., James, S. E., Chang, S., et al. (2008). Delta-catenin-induced dendritic morphogenesis. An essential role of p190RhoGEF interaction through Akt1-mediated phosphorylation. J. Biol. Chem. 283, 977–987. doi: 10.1074/jbc.M707158200
Kolomeets, N. S., Orlovskaya, D. D., and Uranova, N. A. (2007). Decreased numerical density of CA3 hippocampal mossy fiber synapses in schizophrenia. Synapse 61, 615–621. doi: 10.1002/syn.20405
Kulikov, A. V., Korostina, V. S., Kulikova, E. A., Fursenko, D. V., Akulov, A. E., Moshkin, M. P., et al. (2016). Knockout Zbtb33 gene results in an increased locomotion, exploration and pre-pulse inhibition in mice. Behav. Brain Res. 297, 76–83. doi: 10.1016/j.bbr.2015.10.003
Kulkarni, V. A., and Firestein, B. L. (2012). The dendritic tree and brain disorders. Mol. Cell Neurosci. 50, 10–20. doi: 10.1016/j.mcn.2012.03.005
Laura, R. P., Witt, A. S., Held, H. A., Gerstner, R., Deshayes, K., Koehler, M. F., et al. (2002). The Erbin PDZ domain binds with high affinity and specificity to the carboxyl termini of delta-catenin and ARVCF. J. Biol. Chem. 277, 12906–12914. doi: 10.1074/jbc.M200818200
Lee, H. B., He, Y., Yang, G. S., Oh, J. A., Ha, J. S., Song, O. H., et al. (2015). Determination of C-terminal delta-catenin responsible for inducing dendritic morphogenesis. J. Nanosci. Nanotechnol. 15, 5589–5592. doi: 10.1166/jnn.2015.10460
Lee, J. K., Johnson, E. G., and Ghetti, S. (2017). “Hippocampal development: structure, function and implications,” in The hippocampus from cells to systems, eds D. Hannula and M. Duff (Cham: Springer), doi: 10.1007/978-3-319-50406-3_6
Lee, M., Ji, H., Furuta, Y., Park, J. I., and McCrea, P. D. (2014). p120-catenin regulates REST and CoREST, and modulates mouse embryonic stem cell differentiation. J Cell Sci. 127(Pt. 18), 4037–4051. doi: 10.1242/jcs.151944
Lu, Q., Aguilar, B. J., Li, M., Jiang, Y., and Chen, Y. H. (2016). Genetic alterations of delta-catenin/NPRAP/Neurojungin (CTNND2): functional implications in complex human diseases. Hum. Genet. 135, 1107–1116. doi: 10.1007/s00439-016-1705-3
Mariner, D. J., Wang, J., and Reynolds, A. B. (2000). ARVCF localizes to the nucleus and adherens junction and is mutually exclusive with p120(ctn) in E-cadherin complexes. J. Cell Sci. 113(Pt 8), 1481–1490. doi: 10.1242/jcs.113.8.1481
Markham, N. O., Doll, C. A., Dohn, M. R., Miller, R. K., Yu, H., Coffey, R. J., et al. (2014). DIPA-family coiled-coils bind conserved isoform-specific head domain of p120-catenin family: potential roles in hydrocephalus and heterotopia. Mol. Biol. Cell 25, 2592–2603. doi: 10.1091/mbc.E13-08-0492
Martinez, M. C., Ochiishi, T., Majewski, M., and Kosik, K. S. (2003). Dual regulation of neuronal morphogenesis by a delta-catenin-cortactin complex and Rho. J. Cell Biol. 162, 99–111. doi: 10.1083/jcb.200211025
Martinez-Cerdeño, V. (2017). Dendrite and spine modifications in autism and related neurodevelopmental disorders in patients and animal models. Dev. Neurobiol. 77, 393–404. doi: 10.1002/dneu.22417
McCrea, P. D., and Gottardi, C. J. (2016). Beyond beta-catenin: prospects for a larger catenin network in the nucleus. Nat. Rev. Mol. Cell Biol. 17, 55–64. doi: 10.1038/nrm.2015.3
McCrea, P. D., and Park, J. I. (2007). Developmental functions of the P120-catenin sub-family. Biochim. Biophys. Acta 1773, 17–33. doi: 10.1016/j.bbamcr.2006.06.009
McCrea, P. D., Maher, M. T., and Gottardi, C. J. (2015). Nuclear signaling from cadherin adhesion complexes. Curr. Top. Dev. Biol. 112, 129–196. doi: 10.1016/bs.ctdb.2014.11.018
Munton, R. P., Tweedie-Cullen, R., Livingstone-Zatchej, M., Weinandy, F., Waidelich, M., Longo, D., et al. (2007). Qualitative and quantitative analyses of protein phosphorylation in naive and stimulated mouse synaptosomal preparations. Mol. Cell Proteomics 6, 283–293. doi: 10.1074/mcp.M600046-MCP200
Nakayama, A. Y., Harms, M. B., and Luo, L. (2000). Small GTPases Rac and Rho in the maintenance of dendritic spines and branches in hippocampal pyramidal neurons. J. Neurosci. 20, 5329–5338. doi: 10.1523/JNEUROSCI.20-14-05329.2000
Nolze, A., Schneider, J., Keil, R., Lederer, M., Huttelmaier, S., Kessels, M. M., et al. (2013). FMRP regulates actin filament organization via the armadillo protein p0071. RNA 19, 1483–1496. doi: 10.1261/rna.037945.112
Nopparat, J., Zhang, J., Lu, J. P., Chen, Y. H., Zheng, D., Neufer, P. D., et al. (2015). delta-Catenin, a Wnt/beta-catenin modulator, reveals inducible mutagenesis promoting cancer cell survival adaptation and metabolic reprogramming. Oncogene 34, 1542–1552. doi: 10.1038/onc.2014.89
Noren, N. K., Liu, B. P., Burridge, K., and Kreft, B. (2000). p120 catenin regulates the actin cytoskeleton via Rho family GTPases. J. Cell Biol. 150, 567–580. doi: 10.1083/jcb.150.3.567
Paffenholz, R., and Franke, W. W. (1997). Identification and localization of a neurally expressed member of the plakoglobin/armadillo multigene family. Differentiation 61, 293–304. doi: 10.1046/j.1432-0436.1997.6150293.x
Park, J. I., Ji, H., Jun, S., Gu, D., Hikasa, H., Li, L., et al. (2006). Frodo links dishevelled to the p120-catenin/Kaiso pathway: distinct catenin subfamilies promote Wnt signals. Dev. Cell 11, 683–695. doi: 10.1016/j.devcel.2006.09.022
Paulson, A. F., Mooney, E., Fang, X., Ji, H., and McCrea, P. D. (2000). Xarvcf, Xenopus member of the p120 catenin subfamily associating with cadherin juxtamembrane region. J. Biol. Chem. 275, 30124–30131. doi: 10.1074/jbc.M003048200
Puram, S. V., Riccio, A., Koirala, S., Ikeuchi, Y., Kim, A. H., Corfas, G., et al. (2011). A TRPC5-regulated calcium signaling pathway controls dendrite patterning in the mammalian brain. Genes Dev. 25, 2659–2673. doi: 10.1101/gad.174060.111
Raper, J. A. (2009). “Growth cones,” in Encyclopedia of neuroscience, ed. L. R. Squire (Cambridge: Academic Press), 981–985. doi: 10.1016/B978-008045046-9.00340-5
Redmond, L., Kashani, A. H., and Ghosh, A. (2002). Calcium regulation of dendritic growth via CaM kinase IV and CREB-mediated transcription. Neuron 34, 999–1010. doi: 10.1016/s0896-6273(02)00737-7
Rodova, M., Kelly, K. F., VanSaun, M., Daniel, J. M., and Werle, M. J. (2004). Regulation of the rapsyn promoter by kaiso and delta-catenin. Mol. Cell Biol. 24, 7188–7196. doi: 10.1128/MCB.24.16.7188-7196.2004
Shevtsova, Z., Malik, J. M., Michel, U., Bahr, M., and Kugler, S. (2005). Promoters and serotypes: targeting of adeno-associated virus vectors for gene transfer in the rat central nervous system in vitro and in vivo. Exp. Physiol. 90, 53–59. doi: 10.1113/expphysiol.2004.028159
Sirotkin, H., Morrow, B., Saint-Jore, B., Puech, A., Das Gupta, R., Patanjali, S. R., et al. (1997). Identification, characterization, and precise mapping of a human gene encoding a novel membrane-spanning protein from the 22q11 region deleted in velo-cardio-facial syndrome. Genomics 42, 245–251. doi: 10.1006/geno.1997.4734
Stankiewicz, T. R., and Linseman, D. A. (2014). Rho family GTPases: key players in neuronal development, neuronal survival, and neurodegeneration. Front. Cell Neurosci. 8:314. doi: 10.3389/fncel.2014.00314
Tashiro, A., and Yuste, R. (2004). Regulation of dendritic spine motility and stability by Rac1 and Rho kinase: evidence for two forms of spine motility. Mol. Cell Neurosci. 26, 429–440. doi: 10.1016/j.mcn.2004.04.001
Tashiro, A., Minden, A., and Yuste, R. (2000). Regulation of dendritic spine morphology by the rho family of small GTPases: antagonistic roles of Rac and Rho. Cereb. Cortex 10, 927–938. doi: 10.1093/cercor/10.10.927
The UniProt Consortium (2018). UniProt: the universal protein knowledgebase. Nucleic Acids Res. 46:2699. doi: 10.1093/nar/gky092
Torben-Nielsen, B., and Cuntz, H. (2014). “Introduction to dendrite morphology,” in The computing dendrite: from structure to function, Vol. 11, eds H. Cuntz, M. W. H. Remme, and B. Torben-Nielsen (Berlin: Springer), 3–22. doi: 10.1007/978-1-4614-8094-5
Toyo-oka, K., Wachi, T., Hunt, R. F., Baraban, S. C., Taya, S., Ramshaw, H., et al. (2014). 14-3-3epsilon and zeta regulate neurogenesis and differentiation of neuronal progenitor cells in the developing brain. J. Neurosci. 34, 12168–12181. doi: 10.1523/JNEUROSCI.2513-13.2014
Valls, G., Codina, M., Miller, R. K., Del Valle-Perez, B., Vinyoles, M., Caelles, C., et al. (2012). Upon Wnt stimulation, Rac1 activation requires Rac1 and Vav2 binding to p120-catenin. J. Cell Sci. 125(Pt. 22), 5288–5301. doi: 10.1242/jcs.101030
Wolf, A., Keil, R., Gotzl, O., Mun, A., Schwarze, K., Lederer, M., et al. (2006). The armadillo protein p0071 regulates Rho signalling during cytokinesis. Nat. Cell Biol. 8, 1432–1440. doi: 10.1038/ncb1504
Xie, L., Ju, G. Z., Liu, S. Z., Shi, J. P., Yu, Y. Q., and Wei, J. (2005). Searching for a schizophrenia susceptibility gene in the 22q11 region. Biomed .Environ. Sci. 18, 31–35.
Yacoubian, T. A., and Lo, D. C. (2000). Truncated and full-length TrkB receptors regulate distinct modes of dendritic growth. Nat. Neurosci. 3, 342–349. doi: 10.1038/73911
Yanagisawa, M., Huveldt, D., Kreinest, P., Lohse, C. M., Cheville, J. C., Parker, A. S., et al. (2008). A p120 catenin isoform switch affects Rho activity, induces tumor cell invasion, and predicts metastatic disease. J. Biol. Chem. 283, 18344–18354. doi: 10.1074/jbc.M801192200
Ye, B., Zhang, Y., Song, W., Younger, S. H., Jan, L. Y., and Jan, Y. N. (2007). Growing dendrites and axons differ in their reliance on the secretory pathway. Cell 130, 717–729. doi: 10.1016/j.cell.2007.06.032
Yuan, L., and Arikkath, J. (2017). Functional roles of p120ctn family of proteins in central neurons. Semin. Cell Dev. Biol. 69, 70–82. doi: 10.1016/j.semcdb.2017.05.027
Keywords: catenin, dendrite morphogenesis, dendrite branching, p120-catenin subfamily, neuron morphology
Citation: Donta MS, Srivastava Y, Di Mauro CM, Paulucci-Holthauzen A, Waxham MN and McCrea PD (2023) p120-catenin subfamily members have distinct as well as shared effects on dendrite morphology during neuron development in vitro. Front. Cell. Neurosci. 17:1151249. doi: 10.3389/fncel.2023.1151249
Received: 25 January 2023; Accepted: 21 March 2023;
Published: 04 April 2023.
Edited by:
Adrian Walton Moore, RIKEN Center for Brain Science (CBS), JapanReviewed by:
Ginger Withers, Whitman College, United StatesEmilie Pacary, INSERM U1215 Neurocentre Magendie, France
Copyright © 2023 Donta, Srivastava, Di Mauro, Paulucci-Holthauzen, Waxham and McCrea. This is an open-access article distributed under the terms of the Creative Commons Attribution License (CC BY). The use, distribution or reproduction in other forums is permitted, provided the original author(s) and the copyright owner(s) are credited and that the original publication in this journal is cited, in accordance with accepted academic practice. No use, distribution or reproduction is permitted which does not comply with these terms.
*Correspondence: M. Neal Waxham, TS5OLldheGhhbUB1dGgudG1jLmVkdQ==; Pierre D. McCrea, cGRtY2NyZWFAbWRhbmRlcnNvbi5vcmc=